- 1Animal Genetics and Genome Evolutionary Lab (AGAGEL), Division of Genetics and Molecular Biology, Institute of Biological Sciences, Faculty of Science, University of Malaya, Kuala Lumpur, Malaysia
- 2Centre for Research in Biotechnology for Agriculture (CEBAR), University of Malaya, Kuala Lumpur, Malaysia
- 3Malaysian Genome Vaccine Institute, National Institute Biotechnology Malaysia, Bangi, Selangor, Malaysia
- 4Infectious Diseases Translational Research Programme, Department of Medicine, Yong Loo Lin School of Medicine, National University of Singapore, Singapore, Singapore
- 5Department of Biotechnology and Bioinformatics, University of Hyderabad, Hyderabad, Telangana, India
Shrimp aquaculture has been growing rapidly over the last three decades. However, high-density aquaculture together with environmental degradation has led to increased incidence of shrimp infections. Thus, devising and implementing effective strategies to predict, diagnose and control the spread of infections of shrimps are crucial, also to ensure biosecurity and sustainability of the food industry. With the recent advancements in biotechnology, more attention has been given to develop novel promising therapeutic tools with potential to prevent disease occurrence and better manage shrimp health. Furthermore, owing to the advent of the next-generation sequencing (NGS) platforms, it has become possible to analyze the genetic basis of susceptibility or resistance of different stocks of shrimps to infections and how sustainable aquaculture could be made free of shrimp diseases.
1 Introduction to shrimp farming and disease management
Shrimp farming has been identified as one of the most profitable aquaculture sectors in the Asia-Pacific region (De Silva et al., 2007). Aquaculture production of shrimp has been increasing globally, dominated by Southeast Asia, China, India, and America (Anderson et al., 2019). Shrimps belonging to the Penaeidae family are recognized as a valuable economic resource in crustacean aquaculture sector. Among all farmed shrimps, the black tiger shrimp (Penaeus monodon) and the pacific white shrimp (Litopenaeus vannamei) contribute to more than 90-95% of the world production. Initially, P. monodon was identified as the dominant species among cultured shrimps. However, owing to factors such as lower levels of protein requirements and disease resistance, L. vannamei surpassed P. monodon, and now attributes to more than 70% of crustacean aquaculture production (Flegel et al., 2008; Arulmoorthy et al., 2020).
Over the past few decades, there has been a rapid growth in penaeid shrimp aquaculture sector. This over-intensification, along with environmental degradation and the introduction of new varieties in the tropics and subtropics, has resulted in an increased occurrence of emerging shrimp diseases (Walker and Mohan, 2009; Thitamadee et al., 2016; Xiong et al., 2016). The production of shrimp is impaired by diseases primarily caused by various microbial pathogens such as viruses, bacteria, fungi, and protozoa. The World Animal Health Organization (OIE: Office International des Epizooties) recognizes certain diseases as the most significant, which were included in their list for penaeid shrimp diseases (Lightner et al., 2012).
Since the late 1980s, unknown diseases have emerged and spread in shrimp aquaculture farms across the globe, causing staggering economic losses in some countries. For instance, Taiwan faced a loss of US$3 billion of farmed shrimp in 1994, and US$0.5 billion of P. monodon cultures between 1987 and 1988 (Lundin, 1995). White spot syndrome virus (WSSV) was discovered in Taiwan in the early 1990s, and it quickly spread to major shrimp aquaculture farms in Asian countries such as Japan. WSSV caused high mortality rates, leading to an economic loss of US$6 billion (Afsharnasab et al., 2014). In addition to WSSV, other viral pathogens have also caused epidemics in different regions. While Taiwan encountered monodon baculovirus (MBV) epidemic during the mid-1980s, shrimp farms in America were impacted by infectious hypodermal and hematopoietic necrosis virus (IHHNV) and taura syndrome virus (TSV) in 1981 and 1992, respectively. Simultaneously, in 1990, yellow head virus (YHV) first emerged in cultivated shrimps in Thailand (Walker and Mohan, 2009).
Other significant pathogenic diseases include necrotizing hepatopancreatic bacterium (NHPB) disease (Cuéllar-Anjel et al., 2018) and vibriosis (Chandrakala and Priya, 2017) caused by bacteria. Moreover, there are some other fungal and protozoan parasitic diseases that can infect shrimps, as well. Owing to such diseases, mostly caused by viruses, it was projected that since 1994, the worldwide aquaculture sector has incurred an annual loss of US$3 billion (Lundin, 1995; Lightner, 1999). Although there has been a noticeable recovery over the last few years, the growth and profitability of this sector remains impacted by infectious diseases caused by various microbial pathogens.
Penaeid shrimps, unlike vertebrates, lack adaptive immune system and separate lymphatic systems to protect themselves against invading pathogens; thus, increasing the risk of mortality within a few days of infection. This also makes it challenging to develop vaccines against various pathogens (Johnson et al., 2008; Aguirre-Guzman et al., 2009). Moreover, some pathogens infect shrimps at different life stages, even larvae, that has little or no innate immune response (Lightner et al., 2012). Therefore, the enhancement of biosecurity at shrimp farms, development of rapid diagnostic methods and disease prevention strategies are crucial. This review should serve as a compendium of the major shrimp diseases caused by pathogens, the significance of shrimp gut microbiota, and its correlation with the emergence and occurrence of diseases. Furthermore, in addition to the available pathogen detection, diagnostic, and control strategies, novel technologies for improved detection methods and promising therapeutic tools for shrimp diseases are also reviewed in this paper.
2 Pathogen diversity in shrimp aquaculture
2.1 Microbial diseases of penaeid shrimps
Microbial pathogens that cause diseases in shrimps belong to various types of viruses, bacteria, fungi, and protozoan parasites. Among these, certain diseases that cause major economic losses, are recognized by OIE as most significant (Table 1).
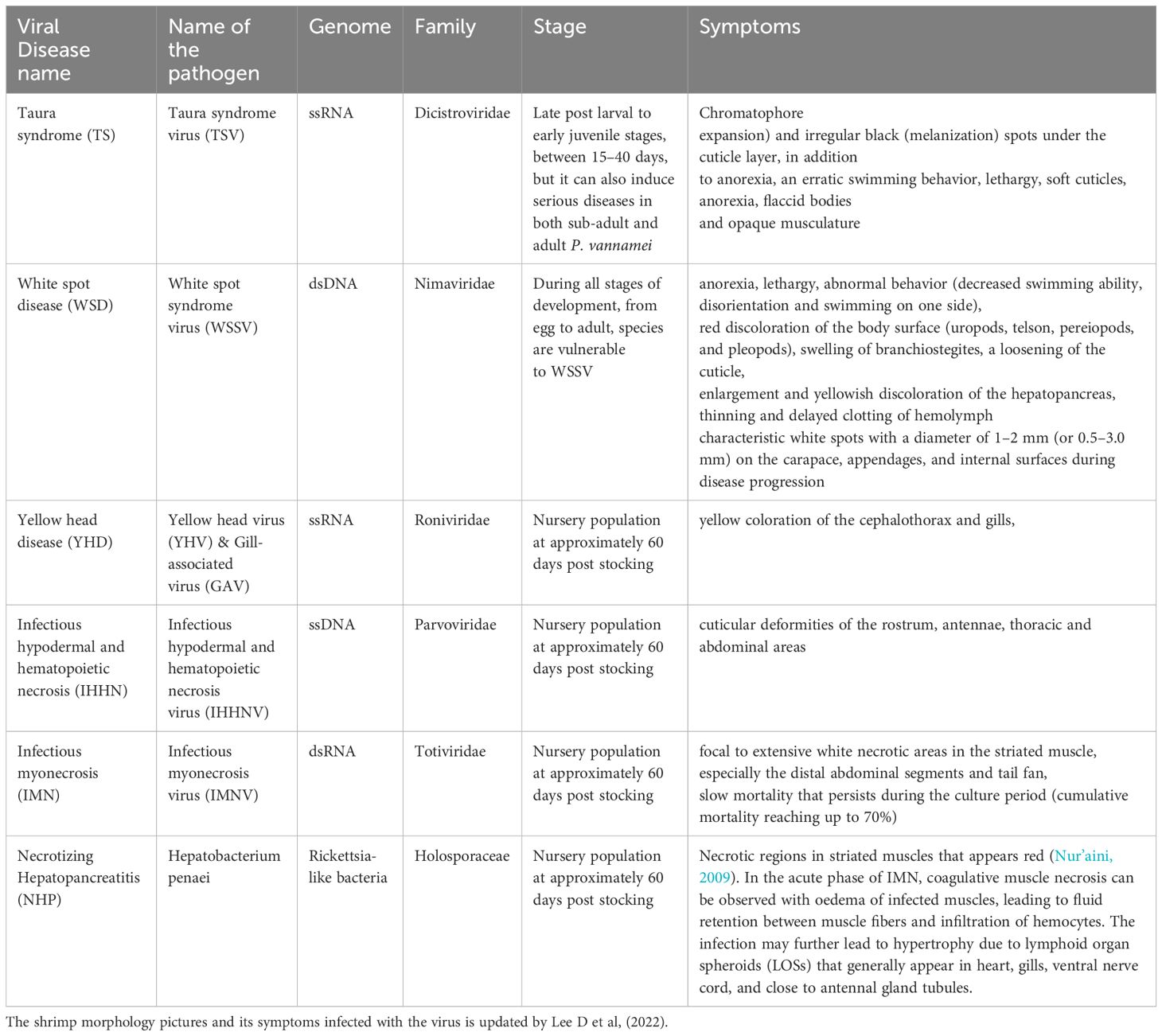
Table 1. Viral shrimp diseases that are listed by OIE (Lightner et al., 2012) and updated by Lee D et al., 2022.
2.1.1 Viral diseases
2.1.1.1 Taura syndrome (TS)
Taura syndrome virus (TSV) which belongs to the family Dicistroviridae is the causative agent of TS. With a diameter of 32 nm, the virion is a naked (without an envelope) icosahedron. TSV’s genetic material consists of 10,205 nucleotides of positive-sense, single-stranded RNA (+ssRNA). TSV’s genome has two open reading frames (ORFs): ORF 1, which codes for non-structural proteins, and ORF 2, which contains TSV structural protein sequences such as capsid proteins (Mari et al., 2002). Studies conducted on the TSV using molecular tools indicated that a single virus strain was behind the first TSV pandemic in American (Ecuador) aquaculture farms from 1991-1992. However, when cDNA sequences of TSV capsid protein 2 (CP2) were compared (Tang and Lightner, 2005), four different genetic variants of TSV were discovered (Wertheim et al., 2009). TS has caused a huge loss to the global shrimp aquaculture industry and has been reported in the Middle East, America, and Asia. The spread of TS was mainly due to the transfer of live broodstocks across regional and international borders (Walker and Mohan, 2009; Lightner, 2011).
With a cumulative death rate of 40-90%, L. vannamei is one of the most vulnerable species to TSV. TS has been reported in this species at post-larvae (PL), juvenile, and adult stages of its life cycle (Ochoa et al., 2020). This virus is also known to infect other varieties of shrimps such as Penaeus stylirostris and Penaeus setiferus. In addition, experiments on PL and juveniles of Penaeus japonicus, P. monodon, Penaeus duorarum, Fenneropenaeus chinensis, Penaeus aztecus and Penaeus schmitti were also reported to be prone to TSV. The viral replication of TSV is in the cytoplasm of the host cell (Ganjoor, 2015). TS occurs in shrimps in three phases. In the peracute/acute phase, the shrimps are more likely to die, and is characterized by the showing of tail fan, pale reddish color in pleopods, softening of coats and hollow intestines. Individuals that survive this phase will go through the regeneration process, which begins with multifocal melanoid lesions. In the chronic infectious phase, the shrimp remains persistently infected with subclinical infections. The TSV is spread to susceptible shrimps by contaminated water and horizontal transmission via cannibalism of diseased, moribund or dead shrimps. It is also hypothesized that TSV can be vertically transmitted, although this is yet to be experimentally validated. Furthermore, the aquatic insect, water boatman Trichocorixa reticulata (Guerin-Meneville, 1857), has been identified as a TSV vector (Dhar et al., 2004). Since the mid-1990s, various research and commercial breeding programs have employed TSV specific pathogen resistance (SPR1) selective breeding to combat TSV disease, significantly decreasing its incidence (Sookruksawong et al., 2013). Notably, between 1999 and 2004, there were no TSV outbreaks reported in Colombian shrimp farms, demonstrating the effectiveness of a TSV-resistant breeding program where 100% of the raised shrimp were TSV-SPR1.
2.1.1.2 White spot disease (WSD)
WSD is a serious disorder caused by WSSV that causes rapid death, most notably in juvenile shrimp. WSSV is an enveloped virus of the genus Whispovirus with double-stranded DNA (dsDNA) and a genome size of 290 to 305 Kbp on average. The size of the genome of isolates from various geographical regions varies, indicating genetic instability that might lead to alterations in virulence (Ganjoor, 2015).
WSSV is widely regarded as one of the most serious threats to the shrimp aquaculture sector (Flegel and Alday-Sanz, 1998). Between 1990 and 1994, the first WSD outbreak was observed in Taiwan and Japan, in P. japonicus (Zhu et al., 2019). In 1999, WSSV was discovered in the United States and Latin America, inflicting massive losses in L. vannamei and P. stylirostris aquaculture. Subsequently, infections were discovered in P. indicus, P. setiferus, F. chinensis, P. merguiensis, and P. monodon, as well. The geographical spread of WSSV became a severe threat to shrimp farming in Asia and America. This restricted the import requirements for shrimp broodstocks in different countries with a ban on animal imports from regions with viral infection (Sangamaheswaran and Jeyaseelan, 2001; Citarasu et al., 2006; Zhang et al., 2006).
Significant signs of acute WSD include a sudden drop in food intake, lethargy, and loosened cuticles with white spots 0.5 to 2.0 mm wide, visible beneath the carapace. In some cases, infected shrimp may exhibit a pink to reddish-brown color due to increased chromatophores. Additionally, white spots are occasionally observed in infected P. vannamei from America. WSSV can infect mesodermal and ectodermal cells, such as the subcuticular epithelium, in various crustacean species, leading to inconsistent mortality rates. The virus spreads through vertical and horizontal transmission, including cannibalism of infected dead shrimp and water-borne pathways. Furthermore, WSSV can reach uncontaminated areas via organisms exposed to contaminated effluents from shrimp farms. Vectors or reservoirs of WSSV include aquatic insect larvae, invertebrates, and copepods (Ganjoor, 2015).
2.1.1.3 Yellow head disease (YHD)
Yellow head virus (YHV) genotype 1 is the causative agent of YHD. YHV is a rod-shaped, enveloped virus with +ssRNA, belonging to the family Roniviridae (Ganjoor, 2015). When tissues infected with YHV were observed under transmission electron microscopy (TEM), vesicles encapsulating virions were observed in the cytoplasm, and in the intracellular spaces. These virions were reported to have a diameter of approximately 40-50 nm and 150-200 nm in length (Walker and Mohan, 2009; Lightner, 2011). First cases of YHV were reported in Thailand in 1990 in a P. monodon culture, and eventually was spread widely in cultured shrimps all over the country (Cowley et al., 2000). Subsequently, YHD has been reported in cultured shrimps in Malaysia, Sri Lanka, India, Taiwan, Indonesia, Vietnam, and Mexico (Mohan et al., 1998; Wang and Chang, 2000; Walker and Mohan, 2009). Apart from P. monodon, other susceptible species to YHD include L. vannamei, L. stylirostris, P. styliferus, Macrobrachium sintangense and Macrobrachium lanchesteri (Ganjoor, 2015).
There are now eight recognized genotypes of YHV (Arulmoorthy et al., 2020). Gill associated virus (GAV) is the genotype 2, recognized as the Australian strain of YHV, belonging to the genus Okavirus in Roniviridae (Spann et al., 1997). GAV is a rod-shaped, enveloped, positive-strand RNA nidovirus (Cowley et al., 1999; Cowley and Walker, 2002). Infections caused by genotypes 3 to 6 were reported in P. monodon in Asia, Australia, and East Africa with no associated disease symptoms (Lee D et al, 2022). Furthermore, genotypes 7 and 8 were reported recently in diseased P. monodon (Mohr et al., 2015) and F. chinensis (Liu et al., 2014).
YHD in Asian intensive cultivation systems are considered as a dangerous P. monodon disease. However, YHV has also been reported to infect other species such as P. aztecus, P. duorarum, P. japonicus, L. vannamei, P. setiferus, and P. stylirostris (Stentiford et al., 2009). Although there is a higher chance for YHD-associated mass mortality in early to juvenile stages of shrimps, there is a chance that individuals in late post larval stages may also die due to infection (Limsuwan, 1991).
As the name “Yellowhead” implies, one of the major characteristics of this disease is yellowish or bleached appearance of the cephalothorax. Other gross signs include high feeding rates followed by a cessation in feed intake, and the presence of moribund shrimp along the pond’s edge (Stentiford et al., 2009; Walker and Mohan, 2009). Moreover, a reddish discoloration is observed in infected shrimps. Although GAV infection is identified as less severe due to low mortality, YHV can infect and cause necrosis in ectodermal and mesodermal tissue, especially in lymphoid organ and gills (Walker and Mohan, 2009). YHV is spread via horizontal transmission by cannibalism of moribund and weak shrimp, and vertical transmission by survivors of the disease, which suffer from persistent subclinical infections (Stentiford et al., 2009; Lightner et al., 2012).
2.1.1.4 Infectious hypodermal and hematopoietic necrosis (IHHN)
IHHN is caused by IHHNV, which belongs to the family Parvoviridae. This virus is 22 nm in diameter, making it the smallest known virus to infect penaeid shrimps. IHHNV is a nonenveloped icosahedral virus with single-stranded DNA of 3.9 kb (Mari et al., 1993; Lightner et al., 2012; Ganjoor, 2015). IHHNV was first discovered in L. vannamei and P. stylirostris in America in the early 1980s, which then rapidly spread to Central America, Brazil, Mexico, Peru, Philippines, Thailand, Indonesia, Singapore, Malaysia (Lightner, 1996a) Australia (Krabsetsve et al., 2004), China (Arulmoorthy et al., 2020) and India (Rai et al., 2009).
Molecular testing identified significant variation within the IHHNV isolates from Asia (Tang et al., 2003; Krabsetsve et al., 2004; Tang and Lightner, 2006). However, American isolates showed lower sequence variation (99.6 to 100% identity) (Tang and Lightner, 2002) and 99.8% sequence identity to IHHNV isolates from the Philippines (Tang et al., 2003). Based on molecular and epidemiology studies of this virus, three different genotypes were identified: (I) Southeast Asia; (II) America/Philippines; and (III) East Africa, Madagascar, Mauritius, and Australia (Lightner, 1996a; Tang and Lightner, 2002; Tang et al., 2003; Lightner, 2011). The host species P. monodon and L. vannamei were only susceptible to IHHNV-I and IHHNV-II. Studies show that genetically diverse cultures of P. monodon belonging to the Indo-Pacific region carried an IHHNV-III DNA fragment in their genome, which prevented infection by the genotype III (Duda and Palumbi, 1999; Tang and Lightner, 2006).
In P. stylirostris, IHHNV can cause high mortality and low virulence in juvenile shrimps and adults, respectively (Motte et al., 2003). Gross signs of this viral infection in P. stylirostris include reduced food intake, changes in behavior, stunted growth, and appearance of white or buff-colored spots (these spots appear different from the white spots observed in WSSV-infected shrimps). These spots are often observed in the cuticular epidermis of the shrimp, making it appear mottled. In moribund shrimps of P. stylirostris and P. monodon suffering from the terminal stage of the infection, the mottled appearance changes to a bluish color with opaqueness in the abdomen (Brock and Lightner, 1990; Lightner, 1996a). In L. vannamei, “Runt-deformity syndrome” (RDS) is observed, which can be characterized by reduced growth and deformed cuticles (Duda and Palumbi, 1999). RDS in juvenile shrimps can also be distinguished by a bent or malformed rostrum, wrinkly antennal flagella, roughness and the appearance of ‘bubble-heads’ on the cuticles (Kalagayan et al., 1991; Browdy et al., 1993; Carr et al., 1996; Lightner, 1996a; Motte et al., 2003).
IHHNV infects the ectodermal and mesodermal tissues such as gills, hypodermis, connective tissues, nerve cord, lymphoid organs, and antennal gland (Lightner, 2011). This virus is spread via horizontal transmission through cannibalism and contaminated water (Lightner, 1996a), and vertical transmission through infected eggs (Motte et al., 2003).
2.1.1.5 Infectious myonecrosis (IMN)
IMN is a novel viral infection caused by the Infectious myonecrosis virus (IMNV), which belongs to the Totiviridae family. IMNV is a non-enveloped icosahedral virus of 40 nm in size. The genome of this virus consists of a single double-stranded RNA (dsRNA) of 7,560 bp (Ganjoor, 2015). Two ORFs are present in the IMNV genome. ORF1 codes for capsid proteins and RNA-binding proteins, while putative RNA-dependent RNA polymerase is encoded by the second ORF (Poulos et al., 2006).
IMNV was initially discovered in L. vannamei of northeast Brazil in the year 2002, and later spread to countries in southeast Asia, including India and Indonesia (Senapin et al., 2007; Sahul Hameed et al., 2017). Genome sequencing analysis showed a 99.6% nucleotide sequence identity between the IMNV genomes from Brazil and Indonesia. This suggests that IMNV may have been transferred to Indonesia from Brazil in 2006 (Arulmoorthy et al., 2020).
IMNV can lead to cumulative mortality ranging from 40% to 70%. Experiments have demonstrated that P. stylirostris, Fenneropenaeus subtilis, and P. monodon can also be infected with IMNV, with shrimps at both juvenile and subadult stages being more susceptible (Arulmoorthy et al., 2020). Apart from increased mortality, lethargy, loss of coordination, and reduced food intake are gross signs of IMN. Furthermore, infected shrimps may appear at the water surface throughout the day and may exhibit necrotic regions in striated muscles that appears red (Nur’aini, 2009). In the acute phase of IMN, coagulative muscle necrosis can be observed with oedema of infected muscles, leading to fluid retention between muscle fibers and infiltration of hemocytes. The infection may further lead to hypertrophy due to lymphoid organ spheroids (LOSs) that generally appear in heart, gills, ventral nerve cord, and areas proximal to antennal gland tubules. LOS lesions are extremely consistent with IMNV lesions that are associated with acute, or chronic stages of the infection (Arulmoorthy et al., 2020). Primary target areas of IMNV include, striated muscles, hemocytes, lymph organ parenchyma cells, and connective tissues (Tang et al., 2005). IMNV is spread via horizontal transmission through cannibalism of the infected shrimps and contaminated water (Poulos et al., 2006; Lightner, 2011).
2.1.1.6 Monodon baculovirus disease (MBD)
MBD is caused by a rod-shaped, enveloped virus which is known as Monodon baculovirus (MBV). This virus belongs to the Baculoviridae family and has circular dsDNA, with a genome size between 80 and 160 Kbp (Mari et al., 1993; Ganjoor, 2015). The first cases of MBD were discovered in 1977 in P. monodon shrimp farms in Taiwan. Subsequently it spread to other countries such as Australia, Philippines, China, Malaysia, Indonesia, Sri Lanka, South Africa, and USA. MBV is also known as P. monodon singly enveloped nuclear polyhedrosis virus (PmSNPV). Apart from MBV, two SNPV strains have been reported. They are the plebejus baculovirus and benettae baculovirus (Arulmoorthy et al., 2020).
Although the primary host species of MBV is P. monodon, multiple cases have been reported from other species including Macrobrachium rosenbergii, Penaus penicillatus, Penaeus semisulcatus, Metapenaeus ensus, P. merguiensis, Penaeus esculentus, L. vannamei, and Penaeus kerathurus (Lightner et al., 1987; Doubrovsky et al., 1988; Lightner, 1988; Chen et al., 1989; Colorni, 1989; Vijayan et al., 1995; Lightner, 1996a, 1996; Rajendran et al., 2012). The most susceptible individuals for MBV are larval and juvenile shrimps. However, the disease has been observed in all developmental stages of P. monodon (Brock and Lightner, 1990). This viral infection causes anorexia, subsequent retarded growth (Ganjoor, 2015), lethargy, and fouling of the surface with darkened appearance. Moreover, when compared to healthy individuals, the infected shrimps are smaller. MBV targets the anterior midgut and the hepatopancreas (Lightner, 1993). The virus spreads through horizontal transmission via the fecal oral route (Chen et al., 1992).
2.1.2 Bacterial diseases
2.1.2.1 Necrotizing hepatopancreatitis (NHP)
NHP is a bacterial disease caused by Hepatobacter penai, a bacterium like Rickettsia. This is a Gram-negative, dimorphic bacterium found in the cytoplasm of infected hepatopancreatic cells. The rod-shaped rickettsia-like body (0.35-0.9 μm) is the most common form. The spiral (helical) form (0.25 x 2-3.5 μm) possesses eight flagella at the basal apex (Lightner et al., 1992). The first cases of NHP were discovered in L. vannamei cultures in Texas, in the year 1985. Later in 1993, a similar disease surfaced in Peru, which was later confirmed as NHP through molecular diagnostic methods such as polymerase chain reaction (PCR) and restriction fragment length polymorphism (RFLP) (Loy et al., 1996). The isolates were further analyzed to have morphologies that are extremely similar, to be considered as identical. With a cumulative prevalence of 39.3%, Latin American countries have reported the highest number of NHP cases (Cuéllar-Anjel et al., 2018). NHP caused mass mortality in countries such as Peru, Costa Rica, Venezuela, Mexico, Panama, and Brazil. In the year 2006, Mexico also encountered an NHP outbreak (del Río-Rodríguez et al., 2006). NHP disease is more likely to occur in regions with high water temperature (29-35°C) and salinity (30-40 ppt) (Lightner, 1996a).
Primary host species susceptible to NHP are F. aztecus, L. vannamei, F. californiensis, P. setiferus, and P. stylirostris. The gross signs of NHP consists of reduced food consumption, slow growth, anorexia, softened shells, flaccid bodies, and expanded chromatophores, along with fouling of the body surface (Lightner, 1996a). NHP generally occurs in hepatopancreatic cell types (Lightner et al., 2012) and can be spread via horizontal transmission through cannibalism of infected tissue (Vincent et al., 2004).
2.1.2.2 Vibriosis
Vibriosis is one of the major diseases that affect shrimp aquaculture farms and has been associated with mortality of shrimp cultures around the globe. Vibriosis is caused by Gram-negative bacteria, and multiple species may be associated with the disease. In this regard, species that have caused vibriosis include Vibrio harveyi, Vibrio alginolyticus, Vibrio splendidus, and Vibrio parahaemolyticus. These bacteria belonging to the Vibrionaceae family, have caused mortality in P. monodon larvae in shrimp farms. It has been demonstrated that only some isolates of V. harveyi have shown to possess virulence, indicating molecular and genetic variation. The over-intensification of shrimp aquaculture may have been associated with the emergence of vibriosis, as the disease has been known to occur in shrimps in stressful conditions (Ishimaru et al., 1995; Lavilla-Pitogo and de la Pena, 1998).
V. harveyi is a luminous bacterium, hence it is visible in infected shrimps at nighttime. Gross signs of this disease include reduced growth rate, lethargy, opaque muscles, and presence of patches (Karunasagar et al., 1994). Furthermore, necrosis of the appendages, gut emptiness, and expansion of chromatophores can also be observed in vibriosis-infected larvae (Chandrakala and Priya, 2017).
2.1.2.3 Acute hepatopancreatic necrosis disease (AHPND)
AHPND is a bacterial disease which is recognized as a major threat to shrimp aquaculture (Tran et al., 2013). In 2009, China reported an outbreak in shrimp cultures which was diagnosed as AHPND (Nunan et al., 2014). Subsequently, Malaysia, Philippines, Mexico, Vietnam, Thailand (Shinn et al., 2018), Bangladesh (Eshik et al., 2017), and the United States (Dhar et al., 2019) also encountered AHPND outbreaks which caused huge losses to their shrimp aquaculture productivity.
AHPND is caused by strains of V. parahaemolyticus, which are highly omnipresent, opportunistic, marine bacteria (Tran et al., 2013). The Gram-negative bacteria belonging to the family Vibrionaceae, contains the plasmid pVa1, which causes virulence. This plasmid carries transposons, binary toxin genes and conjugate transfer genes. This indicates that it is possible for plasmid transfer to other strains of this bacteria or other species (Lee et al., 2015).
This bacterium infects the shrimp by colonizing the gut (Tran et al., 2013; Lai et al., 2015). The pVa1 plasmid then expresses the binary toxins that invade the hepatopancreas (Prachumwat et al., 2019) and trigger shedding of the epithelial cell lining of the tubule (Tran et al., 2013). This makes the hepatopancreas appear pale. The severity of the disease can be influenced by the gut microbiota of the shrimp. When infected, the bacterial communities residing in the gut and hepatopancreas are exposed to an imbalance in their local distribution, known as dysbiosis (Chen et al., 2017). On the other hand, the host can gain protection against the pathogen by the enrichment of certain bacterial species (Yu et al., 2018).
Penaeid shrimp species that have been identified as highly susceptible hosts to AHPND-causing bacteria include L. vannamei and P. monodon (Tran et al., 2013; Zorriehzahra and Banaederakhshan, 2015). Gross signs of infected shrimps include exhibition of shrunken and pale hepatopancreas, gut emptying, low feed intake, swimming spirally, and sluggishness (Zorriehzahra and Banaederakhshan, 2015). This disease is spread via horizontal transmission through co-habituation or ingestion of the pathogen. In this regard, cannibalism of infected and dead shrimp, fecal-oral transmission, and feed pellets colonized by the bacteria can also spread AHPND (Tang et al., 2020).
2.1.3 Fungal diseases
Like viruses and bacteria, fungal pathogens such as Lagenidium callinectes and Sirolpidium spp., have been known to cause diseases in penaeid shrimps as well. Generally, fungal infections are found in larval stages of the shrimps with gross signs including lethargy, presence of mycelia and fungal spores, especially in appendages and gills. Larval mycosis and Fusariosis are common fungal diseases of penaeid shrimp (Karunasagar et al., 2004). A recent study on a new biofloc system also reported that a Fusarium verticilliodes infection resulted in cumulative mortalities in white leg shrimps (Hussein et al., 2024).
2.1.3.1 Larval mycosis
Larval mycosis is a fungal disease caused by Haliphthoros philippinensis, Lagenidium callinectes, Sirolpidium sp., and Lagenidium sp. This disease can affect P. monodon eggs, larvae, and post-larvae. In shrimps infected by Lagenidium, vesicles with zoospores of high motility are formed at the end of the discharge tube, invading the host. On the other hand, when affected by Haliphthoros, vesicles are not formed, but long discharge tubes are observed. In contrast, Sirolpidium infection results in short discharge tubes, and vesicles do not form either (Baticados et al., 1990).
Significant signs of infection include whitish appearance, weakness and high risk of death. Moreover, the mortality rate may reach 100% within 2 days. The inner tissue of the individual is replaced by the zoospores, with discharge tubes protruding out from the body. If the egg is infected, they are prevented from hatching and respiratory difficulties are observed in infected larvae (Baticados et al., 1990).
2.1.3.2 Fusariosis
Fusariosis is caused by Fusarium spp., such as Fusarium solani, which are opportunistic soil fungi that have been reported to infect penaeid shrimps. Cases of fusariosis has been found in P. californiensis, L. vannamei and P. stylirostris from Mexico (Lightner et al., 1979). Moreover, cultivated P. japonicus shrimps from Japan and France have also been affected by this disease (Bian and Egusa, 1981; Criado-Fornelio et al., 1988). Gross signs of this infection include large melanized lesions on cephalothorax and abdomen, with degradation and ulceration of cuticles (Colorni, 1989). Moreover, fungal hyphae can also be observed in infected tissue, under a light microscope (Karunasagar et al., 2004).
2.1.4 Protozoan diseases
2.1.4.1 Microsporidiosis
Microsporidiosis is caused by an endoparasite, Microsporidia. This protozoan has been shown to infect shrimps belonging to the species P. indicus, P. monodon, and P. merguiensis. Generally causing infection in juveniles and adult shrimps, the infected regions can be characterized by an opaque white appearance. The pathogen is known to form spores in infected tissue and may lead to white ovaries, which can cause sterility in spawners. Though the infection rate was estimated to be as low as < 10%, Microsporidia have been known to be extremely pathogenic (Baticados et al., 1990).
2.1.4.2 Gregarine disease
Gregarine disease is caused by gregarine, which are usually present in the gut of penaeid shrimps. They can infect P. monodon shrimps at larval, post larval, juvenile, and adult stages. When gregarines are prevalent, they can interfere with functions of the hepatopancreatic duct, such as particle infiltration. Gregarine infection rates up to 94% have been reported in shrimp cultures (Baticados et al., 1990).
2.2 Microbiome of penaeid shrimps
‘Microbiota’ refers to the bacterial community that resides and maintains a symbolic or commensal relationship with a host organism. The genetic material or metagenome linked with a certain microbiota is known as the ‘Microbiome’ (Kumar et al., 2020). They function as an endocrine organ by serving as a barrier against pathogenic invasion and stimulating the acquisition of host nutrients through several metabolic pathways (Xiong et al., 2017). In penaeid shrimps, the composition of gut microbiota can be influenced by both intrinsic and extrinsic factors. This includes, the diet of the shrimp, consumption of probiotics, physiochemical properties of the water and the growth stage (Xiong et al., 2015b; Yan et al., 2016; Vargas-Albores et al., 2017; Garibay-Valdez et al., 2019).
In shrimp aquaculture systems, shrimp share the ecosystem with invading pathogens. Some studies indicate that the intricate interaction between the shrimp, environmental factors, and the microbiota of the surroundings may cause certain pathogenic diseases to emerge (Boutin et al., 2013; Xiong et al., 2015a). Moreover, the severity of the disease in shrimps is correlated with the composition of gut microbiota (Xiong et al., 2015b). On the other hand, recovery of the gut bacterial composition has shown to increase survival rate in shrimps (Rungrassamee et al., 2016). Hence, it can be concluded that the bacterial community in the intestine is a good indicator of the status of shrimp health (Round and Mazmanian, 2009; Clemente et al., 2012).
The microbiome of penaeid shrimps is dominated by Gram-negative bacteria belonging to the phylum Proteobacteria (Holt et al., 2021). In P. monodon and L. vannamei, the gut microbiota mostly comprises of Vibrio and Photobacterium spp., which belong to the class Gamma-proteobacteria (Chaiyapechara et al., 2012; Rungrassamee et al., 2013; Tzuc et al., 2014; Rungrassamee et al., 2016; Zheng et al., 2016). Apart from this, other phylum including Actinobacteria, Bacteroidetes, Firmicutes and Fusobacteria are also a part of shrimp microbiota (Rungrassamee et al., 2013). With recent technological advancements, using the gut microbiome as a tool to address gut microbiota in various shrimp populations from farm to table will ensure that food safety and hygiene are achieved. This is in line with sustainable development goal strategies, which ensure the fast detection of gut microbiome diversity. Later, strategies using control measures to reverse the pathogens will enable quick control. The intestinal microbiota is crucial for the diverse host physiological processes, such as immunity growth, metabolism upkeep (Lin and Zhang, 2017; Thursby and Juge, 2017), pathogen defense, health maintenance, and nutrient absorption (Blancheton et al., 2013). Following the widespread application of high throughput next-generation sequencing (NGS) technology, there has been an increase in NGS application in 16S rRNA-based microbial community analysis due to cost and effectiveness concerns (Caporaso et al., 2011). These studies are vital for the identification of microbial diversity (Ritchie et al., 2008), trend of microbial gene content (Konstantinidis and Tiedje, 2005), and their correlation to host or environmental parameters (Hamady and Knight, 2009). A study conducted by Soo and Bhassu, 2022 showed that both biochemical tests and 16S rRNA analysis can be proposed as a combined strategy for shrimp health diagnosis, ensuring shrimp health maintenance, disease control, and food safety. This was illustrated in a graphical abstract (Figure 1) that shows the simplified method on how gut microbiome can serve as biomarkers for healthy shrimps.
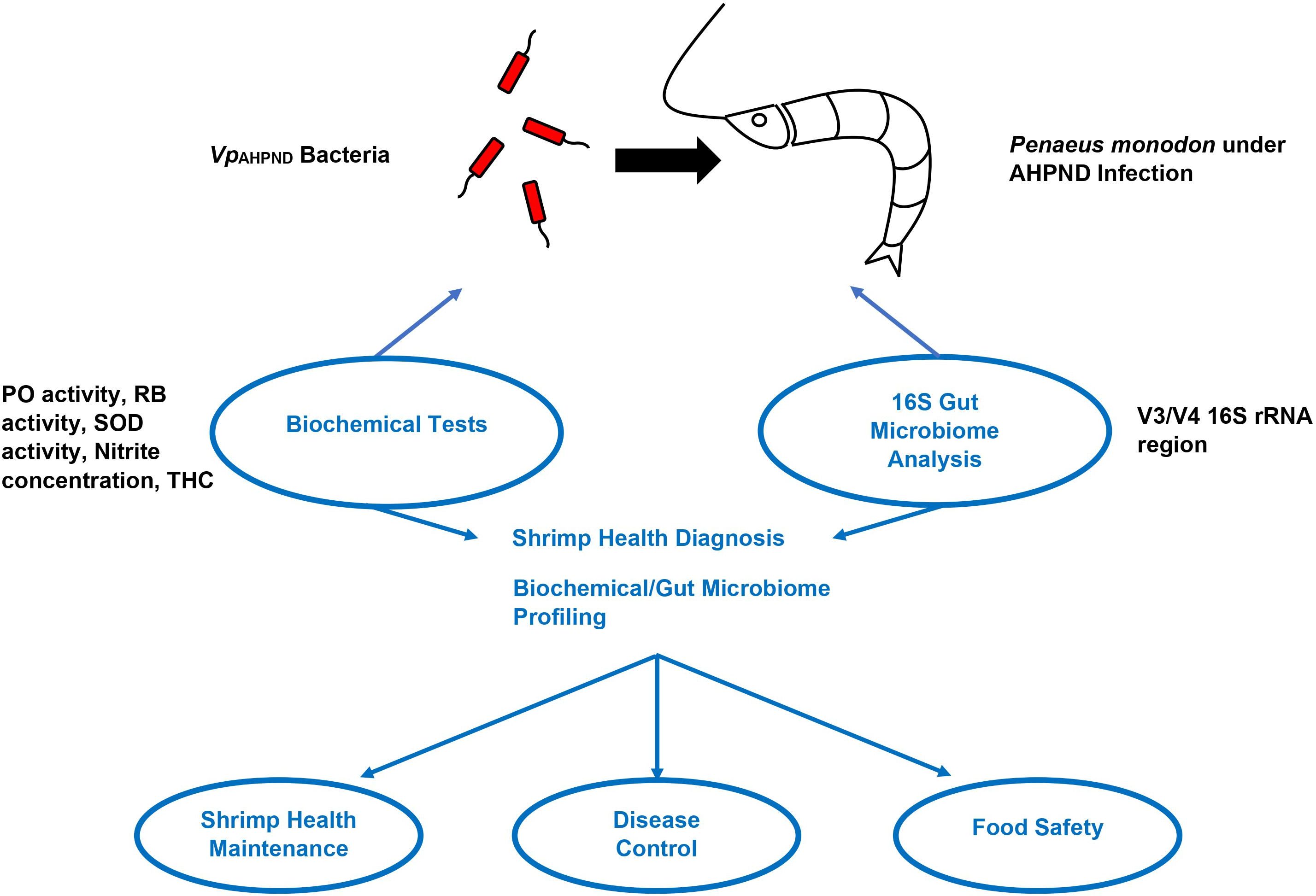
Figure 1. Diagnostic methods for Penaeus monodon health during AHPND infection. Note: Adapted from “Biochemical indexes and gut microbiota testing as diagnostic methods for Penaeus monodon health and physiological changes during AHPND infection with food safety concerns” (Soo and Bhassu, 2022).
16S rRNA V3/V4 hypervariable region is commonly selected in 16S amplicon sequencing analysis as shown previously (Kommedal et al., 2008; Edwards et al., 2012; Derakhshani et al., 2016; Wu et al., 2016). 16S amplicon sequencing analysis is also advantageous due to its lesser reliance on the quality of extracted DNA samples (Rintala et al., 2017). In spite of the widespread application of 16S amplicon sequencing technology in recent years, there have only been relatively few publications reported for the shrimp aquaculture industry, especially those involving diseased conditions (Rungrassamee et al., 2016; Zheng et al., 2016; Pilotto et al., 2018).
The composition of penaeid shrimp microbiota is different at various developmental stages. For instance, Photobacterium spp. is found abundantly (80%) in P. monodon shrimps at post-larval stages, while Vibrio dominates the guts of juvenile shrimp. Other bacteria such as Fusobacteria are observed in PL15, while Spirochaetes are only present in J2 shrimps. Furthermore, while Actinobacteria, are observed in both post-larval and juvenile stages, Listonella is only found in juvenile stages (Rungrassamee et al., 2013). In L. vannamei, similar trends were observed, where Photobacterium spp. and Vibrio spp. dominated in the early stages of life, while Actinobacteria were more abundant in the adult stages (Cicala et al., 2020). The differences in bacterial diversity at various life stages may also be associated with differences in diet composition at different life stages. At the post-larval stage, the shrimps are given live feed, while at the juvenile stage, they are fed commercial pellets (Rungrassamee et al., 2013).
The changes in the microbiome may influence the onset and development of pathogenic diseases (Holt et al., 2021). To fight against bacterial diseases, antibiotics and probiotics have been used in shrimps to modulate the microbiota and develop resistance against specific pathogens. However, the use of antibiotics in shrimps, is restricted to avoid the development of antibiotic-resistance in prevailing bacterial pathogens. Hence, developmental strategies are required to reduce the occurrence of disease outbreaks, decrease the usage of antibiotics, and reinvigorate the health of the shrimp. Consequently, it is crucial to understand the association between the microbiota and shrimp immune system. Additionally, enhancing on the microbial dynamics of the shrimp microbiome and the ecosystem during disease outbreaks is also important (Kumar et al., 2020). For instance, if the gut microbiota is maintained, the risk of diseases caused by opportunistic pathogens in shrimps could be reduced (Rungrassamee et al., 2013).
2.3 Management and diagnostic approaches of penaeid shrimp diseases
Penaeid shrimps do not have an adaptive immune system that can facilitate natural protection against various pathogens and allow for immunization against viruses via standard vaccination (Arala-Chaves and Sequeira, 2000; Johnson et al., 2008). The main objectives of the shrimp health administration in aquaculture or disease management techniques are thus to exclude pathogens, and to avoid stressful environmental conditionals that might favor the emergence and spread of diseases (Walker and Mohan, 2009). This includes implementation of a structured biosecurity at shrimp farms, breeding programs for SPR1 or Specific pathogen free (SPF) stocks, the use of probiotics, and the development of pathogen detection and diagnostic methods. Hence, bacterial species, such as Lactobacillus or Nitrobacter help to improve survival rate, water quality, immunity, and disease resistance through space competition with disease-causing bacteria, such as Vibrio spp., as demonstrated in a recent study conducted by Amiin et al. (2023). The use of prebiotics, probiotics and synbiotics are key ingredients to maintain shrimp gut health at optimum levels throughout the production cycle, ensuring high survival and growth (Noman et al., 2024).
2.3.1 Biosecurity in shrimp aquaculture
Biosecurity practices involves strategies aimed at excluding certain pathogens from shrimp farms to prevent the occurrence of diseases (Walker and Mohan, 2009). It is imperative to establish effective biosecurity measures during all stages of shrimp cultivation, from breeding to harvest, to prevent pathogen entry or release into the environment. There are multiple measures that are implemented on-farm to ensure maximum biosecurity. Firstly, the aquaculture habitat or ponds are initially prepared by drying and exposing them to the sun to eradicate residual pathogens. Secondly, the water is filtered and disinfected before the shrimps are stocked or exchanged during grow-out stage (Walker and Mohan, 2009). The water quality is also frequently monitored and controlled by carrying out tests such as Water Quality Index (WQI), which can allow for the qualitative estimation of shrimp diseases in case of an outbreak (Xiong et al., 2016). Moreover, fences and nets are built to prevent potential carrier organisms and birds from feeding on or spreading infected or moribund shrimps. Furthermore, the effluent from the farm is treated before discharge to avoid the releasing of contaminated water into the ecosystem (Fegan and Clifford, 2001; Vanpatten et al., 2004; Subasinghe, 2005). In addition, shrimps are monitored during all stages of life cycle for early signs of infections by carrying out frequent histological examinations (Walker and Mohan, 2009).
In medium to large semi-intensive shrimp farms, the management of diseases are carried out at a higher level compared to small-holder farms. In more equipped and semi-intensive farms, aspects such as the aquaculture pond and land, treatment of wastes, water usage and maintenance records are managed more effectively. Moreover, they restrict the import of broodstocks from the wild and reduce usage of chemicals and antibiotics in the shrimp farms (Walker and Mohan, 2009). On the other hand, low-income farms have limited awareness, education, and resources to comprehensively practice disease management strategies (Padiyar, 2009). Hence, Better Management Practices (BMP) were developed. BMP are more affordable and effective measures that can be implemented by small-holder farmers to reduce shrimp diseases. The main objectives of BMP are to reduce the risks associated with health problems in shrimps, thereby maximizing the efficiency and production. Moreover, it also aims to minimize the adverse effects of shrimp aquaculture on the environment and enhance safety and quality of cultivated shrimps. Furthermore, BMP are also implemented by small producers to improve social benefits, acceptance, and sustainability of shrimp cultivation (Walker and Mohan, 2009).
The use of artifical intelligence (AI) and machine learning (ML) aided by molecular images are the latest technologies to understand the disease outbreaks in recent decades, necessitating an AI and ML approach. A study conducted by Ping and Liem (2000), attempted to predict shrimp disease occurrence using artificial neural networks versus logistic regression. However the study was inconclusive due to differences in farm and pond management practices. The integration of advanced technologies such as image-based machine learning, augmented reality (AR), surface-enhanced Raman scattering (SERS), and sensor technology, coupled with Internet of Things (IoT), big data, AI, 5G networks, cloud computing, and robotics is expected to have a high impact on disease management in aquaculture (Malik et al., 2017a; Islam et al., 2024; Malik et al. 2017b; Jothiswaran et al., 2020; Li and Li, 2020).
2.3.2 Specific pathogen free stocks
To reduce the impact of diseases on shrimp aquaculture productivity and to guarantee sustainability, it is crucial to acquire high-quality seed or post larvae to stock shrimp farms (Walker and Mohan, 2009). The deployment of SPF stocks has been an emerging trend in the shrimp aquaculture sector and has been recognized as an effective strategy to control shrimp stocks to enhance biosecurity in shrimp farms (Alday Sanz et al., 2020; Walker and Mohan, 2009; Turkmen and Toksen, 2010). SPF stocks are cultured under strict quarantine and screening at breeding centers to acquire shrimp populations devoid of specific or more pathogens. These pathogens should meet a certain criterion. In this regard, the pathogen should be effectively diagnosed and physically removable from the farm. In addition, the pathogen should be classified as a significant threat to the shrimp aquaculture industry (Lotz, 1997; Lightner, 2003).
Currently, SPF stocks that are free of WSSV, YHV, IHHNV, TSV and IMNV are available. However, on a large scale, SPF populations of only L. vannamei are currently obtainable (Walker and Mohan, 2009). It is significant to note that SPF stocks are not resistant to diseases or free of diseases. This is because there is a high chance that they might be infected with a known pathogen that is not listed by the breeding center. On the other hand, the stocks may also be infected by an unknown pathogen. Additionally SPF lists does not include the genus Vibrio, even though they can potentially cause shrimp diseases. Vibrio resides in the gut microbiome of shrimps, and hence cannot be physically eliminated from shrimp farms. SPF shrimps lack innate resistance to pathogens; therefore, disease resistant shrimps can only be bred into a line via selective breeding (Walker and Mohan, 2009).
2.3.3 Specific pathogen resistant stocks
SPR1 stocks are refractory to pathogenic infections and when exposed to such pathogens, they do not exhibit any gross symptoms of the disease. The difference between SPF and SPR1 stocks is that SPF relates to an individual’s health, whereas SPR1 pertains to its genetic status (Alday‐Sanz et al., 2020). Followed by the development of SPF stocks, in 1990, shrimp breeding programs were established to selectively breed shrimp stocks with commercially desirable traits such as resistance to certain diseases. These breeding programs were based on the understanding of the quantitative genetics of penaeid shrimps (Walker and Mohan, 2009).
The development of SPR1 stocks were more focused on certain viral shrimp diseases that inflicted major economic losses to the sector. They include TS, IHHN, and WSD (Argue et al., 2002; Kong et al., 2003; Jiang et al., 2004; Gitterle et al., 2005; Cuéllar-Anjel et al., 2011). In the past two decades, many researchers have managed to enhance penaeid shrimp resistance to TSV via selective breeding (White et al., 2002; Kong et al., 2003). In this regard, L. vannamei stocks that have been selectively bred over 15 generations, were reported to exhibit 100% survival followed by TSV infection (Moss et al., 2011). On the other hand, for WSSV, minor improvements have been made in developing SPR1 stocks. In 2005, a mean selection of 2.8% (Gitterle et al., 2005) was reported in L. vannamei, while a 22.7% survival rate was reported in 2010 (Huang et al., 2011). In addition, in 2011, survival rates between 23% to 57% against WSSV were reported in L. vannamei (Cuéllar-Anjel et al., 2011).
2.3.4 Probiotics management for shrimp growth
The use of antibiotics against bacterial pathogens in shrimp farms have led to the development of antibiotic-resistant bacteria, decreasing the efficiency of antibiotics (Vaseeharan and Ramasamy, 2003). Moreover, other traditional approaches such as the use of disinfectants to eliminate all microbiota in the aquaculture pond (Summerfelt et al., 2009) have led to imbalance in microbial community which decreases the competition within the niche and provides the opportunity for opportunistic pathogens to multiply. Examples of such bacteria include Vibrio species (Attramadal et al., 2012). Hence, it has been advised to avoid the use of antibiotics and disinfectants in shrimp aquaculture systems. Consequently, the application of probiotics was suggested, and some farmers have been adding probiotics to shrimp cultivation ponds as a control strategy against bacterial pathogens (Flegel et al., 2008).
Probiotics are live microorganisms that are orally administered as feed supplements, to enhance the balance of gut microbiota (Newaj-Fyzul et al., 2014). Some studies have suggested that the composition of gut microbiome is different in diseased shrimps, compared to their healthier counterparts (Xiong et al., 2015a; Zheng et al., 2016). These studies indicated that most common species found in healthy shrimp gut are known and have been used as probiotics, whereas the species of microbes isolated from diseased shrimps are labeled as potential pathogens (Zheng et al., 2016). The use of probiotics in shrimp health management has been reported to enhance the immune response and decrease the prevalence of Vibrio species (Li et al., 2007).
Multiple studies have validated the successful application of probiotics in shrimp disease management (Li et al., 2007; Cao et al., 2015; Solanki et al., 2015). Generally, the application of probiotics is standardized throughout the shrimp life cycle, although studies have shown that susceptibility to potential pathogens may vary based on the developmental stages (Zheng et al., 2016). This may limit the extent of probiotic effectiveness (Wang et al., 2008; Ninawe and Selvin, 2009). A study counter-indicated that probiotics do not improve the survival rate and productivity of shrimp aquaculture. Moreover, it is recommended to tailor the probiotic administration to the composition of intestinal microbiota at different developmental stages of the shrimp life cycle (Xiong et al., 2015a).
2.3.5 Currently available diagnostic methods
For effective management of penaeid shrimp diseases, the availability of rapid, sensitive, convenient, and reliable diagnostic methods is significant for early diagnosis and prevention of the spread of disease. The most commonly available diagnostic methods include histological methods such as hematoxylin and eosin (H&E) staining, light microscopy, transmission electron microscopy (TEM), and fluorescent microscopy. Modern diagnostic and research laboratories for penaeid shrimp rely on traditional methods adapted from fish, veterinary, and human diagnostics. These include case history analysis, gross signs and behavioral observation, morphological pathology using bright-field or phase-contrast light microscopy, electron microscopy, and classical microbiology techniques (bacteriology and mycology). However, techniques involving tissue and cell culture, hematology, and clinical chemistry, which are staples in vertebrate diagnostics, have either been unsuccessful or provided unreliable diagnostic data for shrimp (Lightner and Redman, 1998). In contrast, serological methods using polyclonal and monoclonal antibodies, and molecular methods like gene probes and Polymerase Chain Reaction (PCR), have proven to be accurate and standardizable for disease diagnosis and pathogen detection in penaeid shrimp, particularly for certain viruses. It is crucial to understand the workflow from classical methods to the latest diagnostic techniques, including new AI methods in molecular imaging, understanding cellular events within the shrimp innate immune system, and applying the latest technologies such as single-cell sequencing.
In addition, molecular methods such as PCR, nested PCR, multiplex PCR, multiplex reverse transcription-PCR (mRT-PCR), real-time RT-PCR, multiplex RT-nested PCR, and in-situ DNA hybridization methods are also very common. Furthermore, monoclonal antibody based tests such as ELISA, dot-blot assay, and lateral flow chromatographic assay are also shown to be efficient diagnostic methods of shrimp pathogens (Ganjoor, 2015). In this regard, lateral flow chromatographic assay strips have been developed for the detection of certain pathogens such as WSSV and are being applied in Japan and Thailand (Flegel, 2006). These strips were also used by unskilled farmers to diagnose shrimp pathogens immediately at the pond site, without the need for technical expertise (Flegel et al., 2008). Additionally, a similar rapid lateral flow immunoassay test kit for WSSV detection has also been developed commercially in Taiwan (Hsu et al., 2022). On the other hand, methods such as PCR and RT-PCR require sophisticated equipment and skilled personnel to operate them (Flegel et al., 2008; Ganjoor, 2015). Moreover, loop-mediated isothermal amplification (LAMP) in combination with amplicon detection via chromatographic lateral flow dipsticks (LFD) has also been recognized as an easier detection method (Nimitphak et al., 2008). Recently, nanotechnology has been applied in shrimp pathogen diagnosis through nanopores, protein arrays, nanoarrays, nanoparticles, nano-vaccines, and nano-based sensors (Govindaraju et al., 2020).
2.3.6 Contribution of next-generation sequencing in genomic studies
NGS technologies were developed in the early 2000s, transforming the field of biological sciences. These DNA and RNA sequencing technologies have revolutionized the studies of OMICS, allowing novel directions in relevant fields of research that have never been considered in the past (Moorthie et al., 2011). NGS is a high-resolution technology that is cost-effective and efficient. Millions of fragments of DNA can be sequenced concurrently in a short period of time. Examples of NGS platforms include Illumina or Solexa, Roche, Helicos, ABI SOLiD, and Oxford Nanopore (Slatko et al., 2018). These technologies have provided exceptional opportunities for high-throughput applications in the field of functional genomics research (Moorthie et al., 2011).
Conventional approaches for the detection of pathogens are time-consuming and laborious. Through NGS platforms, various information regarding pathogenic diseases can be interpreted. For example, genome sequencing allows for the identification of species, strain of pathogens, its virulence, and the mechanism of pathogenesis. Furthermore, studies on molecular epidemiology and antibiotic-resistance of a pathogen can also be carried out using this technology (Fournier et al., 2014). For instance, Oxford nanopore (Oxford, UK) developed a DNA/RNA sequencing device that can be plugged to a computer and connected to central databases for the assembly and analysis of sequences using the internet. This allows for the detection and analysis of pathogens in real-time at shrimp farms. Moreover, these approaches will also be able to detect unanticipated nucleotide sequences belonging to new or currently unidentified strains of pathogens (Flegel, 2019).
Metagenomic approaches can be used to profile microbial communities present in the aquaculture system (Martínez-Porchas and Vargas-Albores, 2017). The data obtained from these studies can be used to understand the interactions between existing microbes and invading pathogens, that are both synergic and antagonistic. These studies can be applied in developing more cost-effective control strategies for disease management in shrimps. Simultaneously, NGS technologies has also made studies on environmental DNA (eDNA) of shrimp aquaculture systems possible (Shaw et al., 2016). The interaction between organisms in the ecosystem, lead to shedding of eDNA. eDNA is a significant tool that can be applied in ecological studies to monitor the biodiversity and to detect invading species (Barnes et al., 2014; Goldberg et al., 2016). These studies can be used to identify the optimum microbial community in the aquaculture systems for enhanced productivity and long-term sustainability (Flegel, 2019).
The advancement of NGS platforms has also been applied in the establishment of new breeding programs. Marker-assisted selection (MAS) allows for the selection of individuals with economically desirable traits, based on genetic markers, and has shown to increase the efficiency of breeding programs (Shekhar et al., 2021). Selective breeding for enhanced growth has increased the productivity of L. vannamei in the shrimp aquaculture industry. Other determinants of cultivated shrimp productivity include traits that have low heritability and are difficult to measure, such as resistance to diseases (Yáñez et al., 2015). NGS offers platforms for low-cost whole genome (DNA) and transcriptome (RNA) sequencing that can be used to identify genetic markers for traits of interest (Bayliss et al., 2017).
To enhance the sustainability of penaeid shrimp culture, it is important to understand the genomic architecture of the complete genome of penaeid shrimps. However, due to the presence of large number of repeat sequences, it has been challenging to generate a reference genome for all shrimp species (Rodriguez-Anaya et al., 2018). Nevertheless, owing to NGS technologies, a remarkable progress has been made to the advancement of other molecular genetic resources, such as generation of genetic maps, transcriptomes, and identification of qualitative trait loci (QTL) (Shekhar et al., 2021). For instance, the genome sequence and draft assembly of M. japonicus and P. monodon genomes have been generated with sequencing data up to 132.86 Gb and 132 Gb, respectively (Yuan et al., 2018). Recently constructed linkage maps of shrimps allowed for the identification of QTL that are economically important. They include shrimp weight and length of the body in L. vannamei (Andriantahina et al., 2013; Yu et al., 2015), resistance to WSSV in P. monodon (Robinson et al., 2014) and sex linkage in P. monodon (Staelens et al., 2008; Guo et al., 2019). Moreover, QTL related to high pH tolerance has also been mapped, very recently (Huang et al., 2020).
RNA sequencing (RNA-Seq) via NGS platforms has allowed for the development and implementation of transcriptomic analysis of L. vannamei. The potential functional genes and subsequent proteins that are engaged in the route of V. parahaemolyticus infection have been identified from the transcriptome analysis of pathogen-free larvae of L. vannamei. These results indicated that the immune response of the shrimp has evolved against Vibrio infection (Li et al., 2012). Moreover, the identification of gene expression involved in immune response against other pathogens such as TSV (Sookruksawong et al., 2013), and WSSV (Chen et al., 2013; Peruzza et al., 2019, 2020) has also been made possible by using transcript profiling data.
Concurrently, RNA-Seq has been used to generate the transcriptome of gonads in L. vannamei reproductive systems. These data were used to identify genes that are expressed in metabolic routes of the reproductive system of shrimp including sexual differentiation, ovarian follicle growth, development and maturation of gonads and oocytes (Peng et al., 2015). These results can further be applied in MAS for the selection of high-quality stocks with inheritable traits that can improve growth, nutrition, maturation, tolerance to various environmental stress and tolerance against pathogens (Rodriguez-Anaya et al., 2018).
To sum up, NGS platforms have enhanced the application of OMIC technologies in the field of shrimp disease management research. Further advancement of these technologies may be a key to increase the sustainability of shrimp aquaculture industry.
3 Microbial control strategies
3.1 Improvement of pathogen detection methods
The development of rapid, reliable, and convenient diagnostic or detection methods is a significant aspect of penaeid shrimp disease control programs. The most reliable and commonly used diagnostic methods such as PCR-based methods are not readily available for diagnosis in shrimp aquaculture farms. Moreover, these methods require advanced equipment and skilled personnel to operate such machines and interpret data (Flegel et al., 2008). Hence, there is a high demand for point-of-care (POC) methods that farmers can use at pond side, such as the recently developed lateral flow chromatographic immunodiagnostic strips (Flegel, 2006).
There have been several advancements in the development of early disease detection technologies. In the past few years, researchers have developed a method called Sensitive High Efficiency Reporter unLOCKing (SHERLOCK). This method is highly sensitive, rapid and was able to develop lyophilized paper strips for the diagnostic testing of human Zika and Dengue virus. This POC method allowed the device to be used in places with no power or infrastructure (Gootenberg et al., 2017, 2018; Myhrvold et al., 2018). With the increase in knowledge and innovation in Clustered Regularly Interspaced Short Palindromic Repeats (CRISPR) technology, this tool has been applied in many fields of scientific research. In 2019, a CRISPR-based SHERLOCK diagnostic method was developed which allowed for accurate single copy detection of WSSV in penaeid shrimps. This assay is rapid and highly sensitive with potential applications in early detection of penaeid shrimp viruses at pond side (Sullivan et al., 2019). The recent study by Major et al. (2023) successfully adapted a SHERLOCK assay, initially designed for human diagnostics, to detect RNA and DNA pathogens in shrimp. This innovative RT-LAMP CRISPR/Cas diagnostic assay targets TSV and WSSV in L. vannamei, offering significant potential for field-deployable applications. This breakthrough is set to enhance biomonitoring in shrimp aquaculture and lays the groundwork for developing rapid and efficient diagnostics in the broader agricultural sector.
Concurrently, advanced multiplex PCR methods have also been developed to simultaneously detect DNA and RNA viruses in shrimps. In this regard, a study conducted in 2021 developed a dual priming oligonucleotide (DPO)- based multiplex PCR system which is cost effective. This multiplex PCR kit is time-saving, as results can be obtained within a day (See et al., 2021).
Another technology that has been implemented widely in this field is biosensing. Biosensors detect pathogens or diagnose diseases based on the conversion of biological responses generated during protein or nucleic acid interactions into electrical signals (Santos et al., 2020). This method can be used to diagnose and detect pathogens in aquaculture systems as well. Graphene oxide immobilized with methylene blue was used to develop an electrochemical immunosensor to successfully detect the presence of WSSV in penaeid shrimp (Natarajan et al., 2017). This method allows for the electrochemical immunosensing of the virus in the tissues of shrimps. In 2018, DNA Schottky diodes were used to derive electronic properties of DNA to develop a biosensor which can detect both bacterial and viral pathogens in penaeid shrimp samples (Rizan et al., 2018). DNA-based biosensors can become a significant tool in the diagnosis of microbial pathogens in shrimp farms in the field.
Nanotechnology is another advanced technology that has been applied in multiple areas of scientific research. However, the application of nanotechnology in the aquaculture industry is still novel. In Thailand, a rapid and efficient immunochromatographic test strip was developed using a monoclonal antibody W29 coupled with colloidal gold nanoparticles, to detect WSSV in M. rosenbergii (Sithigorngul et al., 2006). In addition, gold nanoparticles were also used to develop a Surface Plasmon Resonance (SPR2) device for the detection of WSSV (Lei et al., 2008).
ELISA is the currently used viral pathogen detection method in shrimp aquaculture (Ganjoor, 2015), which can be further improved to detect microbial diseases. For instance, white tail disease in M. rosenbergii was detected via a sandwich enzyme-linked immunosorbent assay (S-ELISA). This method was based on unlabeled antibody and biotinylated antibody coatings to trap antigens. S-ELISA is a rapid, sensitive, and cost-effective method that can be used in epidemiological studies of shrimp diseases (Romestand and Bonami, 2003).
Alternative perspectives can also be taken in the development of effective diagnostic methods for shrimp pathogens. An example would be the possible development and utilization of a new type of pathogen detection approach involving Raman spectra analysis using deep learning methods (Yu et al., 2021).
3.2 Vaccination/immunostimulants and immune memory of shrimps
Conventional vaccines consist of inactive pathogen-derived molecules such as antigens, that can trigger a host memory-based immune response to fight certain pathogens. This mechanism is known as adaptive immunity and is absent in invertebrates such as penaeid shrimps (Flegel, 2019). Hence, it has been a challenge to develop vaccines against viral and bacterial diseases of shrimps. It is significant to comprehensively understand the immune system of penaeid shrimps to develop effective control strategies. Immunological studies of shrimps have discovered a phenomenon that is similar to acquired immunity observed in vertebrates. In shrimps, the innate immune system can form immunological memory, and this is known as ‘trained innate immunity’ or ‘immune memory’ (Netea et al., 2011; Quintin et al., 2014; Boraschi and Italiani, 2018).
Multiple studies have reported the activation of innate immune responses in shrimps following an infection or the administration of immunization, which decreased the risk of getting infected by the same virus or reduced the spread of infection. Penaeid shrimps have exhibited resistance to experimentally induced WSSV for 4 months (Venegas et al., 2000; Wu et al., 2002). Moreover, when formalin inactivated WSSV or envelope proteins of recombinant WSSV were administered to shrimps, it also induced resistance (Namikoshi et al., 2004; Vaseeharan et al., 2006; Witteveldt et al., 2006; Ha et al., 2008). Protective levels of 50-90% have been observed with the administration WSSV envelope proteins, however, the duration of immunity depends on the formulation (Wu and Zhang, 2007; Wu et al., 2008). A recent study has suggested an epitope that could be used in the development of a vaccine against WSSV as well (Momtaz et al., 2019). The precise mechanism of action of DNA vaccines in shrimps is not completely understood, hence further research is required in this area. The use of immunostimulants is preferred as natural derivatives that range from bacteria, fungi, plants, animals, phytochemicals, and hormones, serving as primers for a chain of events in the PAMPs defense mechanism to clear the pathogen (Lee et al., 2020; Kumar et al., 2023). It is proposed that immunostimulants are a better chemotherapeutic than vaccines, as immune priming can be administered at the larval stage. Hence, disease intervention at the larval stage is a crucial step in ensuring higher survival rate in shrimp aquaculture. This strategy will pave way for reducing antibiotic use in shrimp aquaculture (Kumar et al., 2023). It is proposed that immunostimulants may be more effective than vaccines as a chemotherapeutic strategy in shrimp aquaculture. Applications of vaccines range from traditional killed/inactivated and live attenuated vaccines to new generation ones, including recombinant, synthetic peptides, mucosal and DNA, subunit, nanoparticle-based and plant-based edible vaccines, reverse vaccinology, and monovalent and polyvalent vaccines (Mondal and Thomas, 2022). In shrimp vaccines, the most promising vaccine that has been tested is against vibriosis, as discussed in a recent review by Amatul-Samahah et al., 2020. The aim of a good vaccine is based on a few criteria: 1) safe for shrimp and humans, 2) long lasting protection of the pathogens throughout the entire production cycle, 3) cost-effective and sustainable 4) trans-generational potential that can be passed to progeny, and 5) applicability across different shrimp species (Sivasankar et al., 2017; Kumar et al., 2018).
3.3 Phage therapy
Antibiotics are frequently used in shrimp aquaculture to treat bacterial outbreaks; however, there have been concerns regarding development of antibiotic-resistance in bacterial cultures and environmental pollution. One of the most common bacterial diseases reported in penaeid shrimp farm is vibriosis. Phage therapy is used to control and prevent bacterial infections in aquaculture systems through the use of lytic phages, which are viruses that infect bacteria, called bacteriophages (Flegel et al., 2008; Kalatzis et al., 2018). This technology has been promising (Defoirdt et al., 2011; Oliveira et al., 2012; Richards, 2014) ever since it was first applied in Japan to control Lactococcus garvieae communities (Nakai et al., 1999).
Over the last decade, phage therapy has been applied to treat diseases caused by various species of bacteria. In L. vannamei, Lomelí-Ortega and Martínez-Díaz (2014) administered A3S and Vp1 bacteriophages after 6 hours after a vibriosis infection caused by V. parahaemolyticus and reported a reduced mortality rate (Lomelí-Ortega and Martínez-Díaz, 2014). A similar study on the same species induced experimental phage therapy against V. parahaemolyticus causing AHPND and reported an increase in survival rates (Jun et al., 2018). This technology has also been applied against V. alginolyticus in Apostichopus japonicus (Zhang et al., 2015) and V. harveyi in P. monodon (Stalin and Srinivasan, 2017). However, there is a limitation of field studies involving this technology. Abiotic factors such as the quality of water and the composition of organic matter in the culture or pond, may influence the efficiency of phage therapy. Hence, further studies are required to establish models that determine the various external factors impacting the efficiency of phage therapy. Moreover, it is significant to conduct more studies to determine the optimal dosage and administration schedule of this treatment (Santos et al., 2020). Phage therapy has the potential to be a biologically safe, commercial, and environmentally friendly alternative to antibiotic treatment of bacterial diseases in penaeid shrimp aquaculture.
3.4 Quorum sensing (QS) to control virulence of bacteria
The development of antibiotic-resistance in bacterial species such as Vibrio is a major concern. There have been reports of antimicrobial-resistant (AMR) genes being transferred to human pathogens by Vibrio sp. Hence, it is imperative to develop methods to control AMR bacterial species in aquaculture systems (Bramhachari et al., 2019). QS is a bacterial mechanism that allows cell-to-cell communication and regulation of gene expression in response to cell density (Pawar and Lahiri, 2018). The production of virulence factors in bacteria is controlled by this mechanism. Consequently, QS has been extensively studied in disciplines such as medicine, environmental science, and technology. Recently, QS has been applied in the regulation of bacterial virulence and infections caused by Vibrio species in aquaculture (De Decker et al., 2013; Benitez and Silva, 2016). In this regard, QS was used to reduce the mortality associated with V. campbelli infection in brine shrimp larvae and M. rosenbergii (Pande et al., 2013). These studies suggest that QS system may be a potential target to develop treatments against shrimp infections caused by Vibrio spp.
3.5 RNA interference
RNA interference (RNAi) is a post-transcriptional process wherein dsRNA is introduced into cells to trigger the silencing of specific genes. When dsRNA is delivered into cells, it, along with proteins including Argonaute, forms the RNA-induced silencing complex (RISC). The RISC then targets, identifies, and degrades specific messenger RNA (mRNA) sequences, inhibiting translation of subsequent proteins (Agrawal et al., 2003). RNAi has potential applications in the disease management of penaeid shrimps (Walker and Mohan, 2009). This mechanism was first applied in shrimps against WSSV in the US (Robalino et al., 2004, 2005). Concurrently, RNAi was reported effective in protecting shrimps against YHV and TSV. Moreover, WSSV replication was inhibited in M. japonicus via the introduction of inactive bacteria expressing vp28-siRNA construct (Yodmuang et al., 2006; Xu et al., 2007; Zhu and Zhang, 2011). Furthermore, via the introduction of non-specific dsRNA constructs, the mortality rates of M. japonicus decreased when infected with WSSV (Maralit et al., 2015).
RNAi can also be used to study the function of proteins that are involved in the immune system of shrimps. Furthermore, the addition of dsRNA as feed additives in shrimp farms and breeding programs, may have the potential to protect against pathogens and generate pathogen-free progeny (Itsathitphaisarn et al., 2017). Limitations of RNAi in shrimp disease management include inadequate assessments of the long-term durability of the immunity induced by this strategy (Walker and Mohan, 2009). Moreover, the application of RNAi is still novel in this industry, as majority of the studies are conducted in laboratories (Loy et al., 2013). Hence, it is imperative to develop economic and practical techniques for RNAi application in shrimp disease management on a commercial level.
3.6 The future of NGS analysis in shrimp aquaculture
NGS platforms offer rapid, high throughput and large amounts of sequencing data. Third-generation sequencers such as PacBio and Oxford Nanopore, allow longer read lengths of ~20kb without the requirement for assembly processing (Amarasinghe et al., 2020). One such example is the complete transcriptome of L. vannamei by long-read sequencing (Zeng et al., 2018). Currently, the only reported complete shrimp genome is of L. vannamei (Zhang et al., 2019), while there are reports on the full-length transcriptomes of P. indicus and P. monodon (Huerlimann et al., 2018; Katneni et al., 2020). The development of bioinformatics offers novel advanced software for the assembly of genomes and genome annotation. Hence, with the advancement of NGS technologies and algorithms, future research can focus on generating reference genomes and transcriptomes for other commercially significant shrimp species. These sequencing data create valuable genomic resources that can be utilized in areas such as MAS in breeding programs, enhancing the sustainability of shrimp aquaculture (Shekhar et al., 2021).
Future research on whole genome sequencing of shrimp can focus on identifying genome-wide variations in wild and pond-reared shrimps from different regions of the world. Moreover, the development of experimental and statistical methods is required to identify variations in genes and alleles that lead to desirable traits. In addition, it is crucial to enhance the integration of molecular and genomics tools in both academic and industrial settings. Furthermore, epigenomics studies help in understanding the influence of abiotic factors on shrimp phenotypes, thereby increasing the sustainability and productivity (Metzger and Schulte, 2016; Wan et al., 2016; Yue and Wang, 2017).
4 Epilogue
Since the outbreaks of shrimp diseases emerged in the 1980s, control measures have been implemented to prevent and reduce the spread of the diseases, primarily bacterial and viral infections. These measures mostly involved onsite biosecurity, the use of probiotics, and the development of SPF and SPR1 stocks. Multiple molecular diagnostic tools, including PCR-based methods, monoclonal antibody assays, and lateral flow chromatographic assay test strips, were developed to detect and diagnose various pathogens. Crucially, some of these methods enabled farmers to detect and diagnose pathogenic microorganisms directly at shrimp farms, significantly enhancing disease management and prevention efforts. However, a significant concern persists regarding the lack of effective strategies to prevent the emergence of new or previously controlled infections and to treat them. Due to the absence of an adaptive immune system, conventional disease prevention measures like vaccination are ineffective in shrimp. Consequently, numerous studies have concentrated on elucidating the underlying mechanisms of shrimp immune responses, potentially advancing our understanding of immunomodulation. Over the past few years, improved pathogen detection methods, including the application of NGS platforms, nanotechnology, CRISPR-Cas9, and DNA biosensors, have gained traction. Additionally, novel technologies with the potential to treat shrimp diseases, such as the development of vaccines, phage therapy, quorum sensing to control bacterial virulence, and gene silencing using RNA interference, show great promise. However, additional field studies are necessary to evaluate the effectiveness of these strategies in treating or preventing the emergence of diseases in shrimp aquaculture systems. This research is crucial to ensure that these systems become profitable and sustainable for farmers, while also being safe for consumers. The next frontier research should be directed towards the development of culture-free diagnostics. This approach would ideally require transdisciplinary methodologies such as those harnessing physiochemical biosensors.
Author contributions
SB: Conceptualization, Formal analysis, Project administration, Supervision, Writing – original draft, Writing – review & editing. MS: Data curation, Formal analysis, Writing – original draft. ST: Data curation, Formal analysis, Writing – review & editing. TS: Formal analysis, Investigation, Visualization, Writing – review & editing. NA: Formal analysis, Investigation, Resources, Writing – review & editing. KY: Investigation, Validation, Visualization, Writing – review & editing.
Funding
The author(s) declare financial support was received for the research, authorship, and/or publication of this article. The work was completed under the funding of RU004G-2020 grant awarded to SB under Institut Pengurusan dan Pemantauan Penyelidikan (IPPP), University of Malaya.
Acknowledgments
The authors would like to thank Shuvarnah Mohan who helped in obtaining the materials for writing.
Conflict of interest
The authors declare that the research was conducted in the absence of any commercial or financial relationships that could be construed as a potential conflict of interest.
The author(s) declared that they were an editorial board member of Frontiers, at the time of submission. This had no impact on the peer review process and the final decision.
Publisher’s note
All claims expressed in this article are solely those of the authors and do not necessarily represent those of their affiliated organizations, or those of the publisher, the editors and the reviewers. Any product that may be evaluated in this article, or claim that may be made by its manufacturer, is not guaranteed or endorsed by the publisher.
References
Afsharnasab M., Kakoolaki S., Afzali F. (2014). The Status of white spot syndrome virus (WSSV) in Islamic Republic of Iran. Iran. J. Fish. Sci. 13, 1021–1055. doi: 10.22092/IJFS.2018.114414
Agrawal N., Dasaradhi P. V. N., Mohmmed A., Malhotra P., Bhatnagar R. K., Mukherjee S. K. (2003). RNA interference: biology, mechanism, and applications. Microbiol. Mol. Biol. Rev. 67, 657–685. doi: 10.1128/MMBR.67.4.657-685.2003
Aguirre-Guzman G., Sanchez-Martinez J. G., Campa-Cordova A. I., Luna-Gonzalez A., Ascencio F. (2009). Penaeid shrimp immune system. Thai J. Vet. Med. 39, 205–215. doi: 10.56808/2985-1130.2175
Alday-Sanz V., Brock J., Flegel T. W., McIntosh R., Bondad-Reantaso M. G., Salazar M., et al. (2020). Facts, truths and myths about SPF shrimp in Aquaculture. Rev. Aquac. 12, 76–84. doi: 10.1111/raq.12305
Amarasinghe S. L., Su S., Dong X., Zappia L., Ritchie M. E., Gouil Q. (2020). Opportunities and challenges in long-read sequencing data analysis. Genome Biol. 21, 30. doi: 10.1186/s13059-020-1935-5
Amatul-Samahah M. A., Wan Omar W. H. H., Mohd Ikhsan N. F., Azmai M. N.A., Zamri-Saad M., Ina-Salwany M. Y. (2020). Vaccination trials against vibriosis in shrimp: A review. Aquac. Rep. 18, 100471. doi: 10.1016/j.aqrep.2020.100471
Amiin M. K., Lahay A. F., Putriani R. B., Reza M., Putri S. M. E., Sumon M. A. A., et al. (2023). The role of probiotics in vannamei shrimp aquaculture performance - A review. Vet. World. 16, 638–649. doi: 10.14202/vetworld.2023.638-649
Anderson J. L., Valderrama D., Jory D. E. (2019). GOAL 2019: Global Shrimp Production Review. Available at: https://www.aquaculturealliance.org/advocate/goal-2019-global-shrimp-production-review/ (Accessed June 16, 2021).
Andriantahina F., Liu X., Huang H. (2013). Genetic map construction and quantitative trait locus (QTL) detection of growth-related traits in Litopenaeus vannamei for selective breeding applications. PloS One 8, e75206. doi: 10.1371/journal.pone.0075206
Arala-Chaves M., Sequeira T. (2000). Is there any kind of adaptive immunity in invertebrates? Aquaculture 191, 247–258. doi: 10.1016/S0044-8486(00)00430-0
Argue B. J., Arce S. M., Lotz J. M., Moss S. M. (2002). Selective breeding of Pacific white shrimp (Litopenaeus vannamei) for growth and resistance to Taura Syndrome Virus. Aquaculture 204, 447–460. doi: 10.1016/S0044-8486(01)00830-4
Arulmoorthy M. P., Anandajothi E., Vasudevan S., Suresh E. (2020). Major viral diseases in culturable penaeid shrimps: a review. Aquac. Int. 28, 1939–1967. doi: 10.1007/s10499-020-00568-3
Attramadal K. J., Salvesen I., Xue R., Øie G., Størseth T. R., Vadstein O., et al. (2012). Recirculation as a possible microbial control strategy in the production of marine larvae. Aquac. Eng. 46, 27–39. doi: 10.1016/j.aquaeng.2011.10.003
Barnes M. A., Turner C. R., Jerde C. L., Renshaw M. A., Chadderton W. L., Lodge D. M. (2014). Environmental conditions influence eDNA persistence in aquatic systems. Environ. Sci. Technol. 48, 1819–1827. doi: 10.1021/es404734p
Baticados M. C. L., Cruz-Lacierda E. R., de la Cruz M., Duremdez-Fernandez R. C., Gacutan R. Q., Lavilla-Pitogo C. R., et al. (1990). Diseases of penaeid shrimps in the Philippines (Tigbauan, Iloilo, Philippines: Aquaculture Department, Southeast Asian Fisheries Development Center).
Bayliss S. C., Verner-Jeffreys D. W., Bartie K. L., Aanensen D. M., Sheppard S. K., Adams A., et al. (2017). The promise of whole genome pathogen sequencing for the molecular epidemiology of emerging aquaculture pathogens. Front. Microbiol. 8. doi: 10.3389/fmicb.2017.00121
Benitez J. A., Silva A. J. (2016). Vibrio cholerae hemagglutinin (HA)/protease: an extracellular metalloprotease with multiple pathogenic activities. Toxicon 115, 55–62. doi: 10.1016/j.toxicon.2016.03.003
Bian B. Z., Egusa S. (1981). Histopathology of black gill disease caused by Fusarium solani (Martius) infection in the Kuruma prawn, Penaeus japonicus Bate. J. Fish Dis. 4, 195–201. doi: 10.1111/j.1365-2761.1981.tb01126.x
Blancheton J. P., Attramadal K. J. K., Michaud L., d’Orbcastel E. R., Vadstein O. (2013). Insight into bacterial population in aquaculture systems and its implication. Aquac. Eng. 53, 30–39. doi: 10.1016/j.aquaeng.2012.11.009
Boraschi D., Italiani P. (2018). Innate immune memory: time for adopting a correct terminology. Front. Immunol. 9. doi: 10.3389/fimmu.2018.00
Boutin S., Bernatchez L., Audet C., Derôme N. (2013). Network analysis highlights complex interactions between pathogen, host and commensal microbiota. PloS One 8, e84772. doi: 10.1371/journal.pone.0084772
Bramhachari P. V., Prathyusha A. M. V. N., Sheela G. M., Rao Reddy N. N., Berde C. V., Yugandhar N. M. (2019). “Strategies for disruption of biofilm formation ability and intricate quorum sensing networks in aquaculture Vibrio pathogens,” in Implication of Quorum Sensing and Biofilm Formation in Medicine, Agriculture and Food Industry. Ed. Bramhachari P. (Springer, Singapore), 313–321.
Brock J. A., Lightner D. V. (1990). “Diseases of crustacea. Diseases caused by microorganisms,” in Diseases of Marine Animals. Ed. Kinne O. (Biologische Anstalt Helgoland, Hamburg, Germany), 245–349.
Browdy C. L., Holloway J. D. Jr., King C. O., Stokes A. D., StephenHopkins J., Sandifer P. A. (1993). IHHN virus and intensive culture of Penaeus vannamei: effects of stocking density and water exchange rates. J. Crust. Biol. 13, 87–94. doi: 10.1163/193724093X00462
Cao H., An J., Zheng W., He S. (2015). Vibrio cholerae pathogen from the freshwater-cultured whiteleg shrimp Penaeus vannamei and control with Bdellovibrio bacteriovorus. J. Invertebr. Pathol. 130, 13–20. doi: 10.1016/j.jip.2015.06.002
Caporaso J. G., Lauber C. L., Walters W. A., Berg-Lyons D., Lozupone C. A., Turnbaugh P. J., et al. (2011). Global patterns of 16S rRNA diversity at a depth of millions of sequences per sample. Proc. Natl. Acad. Sci. U.S.A. 108, 4516–4522. doi: 10.1073/pnas.1000080107
Carr W. H., Sweeney J. N., Nunan L., Lightner D. V., Hirsch H. H., Reddington J. J. (1996). The use of an infectious hypodermal and hematopoietic necrosis virus gene probe serodiagnostic field kit for the screening of candidate specific pathogen-free Penaeus vannamei broodstock. Aquaculture 147, 1–8. doi: 10.1016/S0044-8486(96)01291-4
Chaiyapechara S., Rungrassamee W., Suriyachay I., Kuncharin Y., Klanchui A., Karoonuthaisiri N., et al. (2012). Bacterial community associated with the intestinal tract of P. monodon in commercial farms. Microb. Ecol. 63, 938–953. doi: 10.1007/s00248-011-9936-2
Chandrakala N., Priya S. (2017). Vibriosis in shrimp aquaculture a review. Int. J. Sci. Res. Sci. Eng. 3, 27–33.
Chen S. N., Chang P. S., Kou G. H. (1992). “Infection route and eradication of Penaeus monodon baculovirus (MBV) in larval giant tiger prawns, Penaeus monodon,” in Diseases of cultured penaeid shrimp in Asia and the United States. Eds. Fulks W., Main K. L. (The Oceanic Institute, Makapuu Point, Honolulu, Hawaii), 177–184.
Chen S. N., Chang P. S., Kou G. H., Lightner D. V. (1989). Studies on virogenesis and cytopathology of Penaeus monodon baculovirus (MBV) in the giant tiger prawn (Penaeus monodon) and the red tail prawn (Penaeus penicillatus). Fish Pathol. 24, 89–100. doi: 10.3147/jsfp.24.89
Chen W. Y., Ng T. H., Wu J. H., Chen J. W., Wang H. C. (2017). Microbiome dynamics in a shrimp grow-out pond with possible outbreak of acute hepatopancreatic necrosis disease. Sci. Rep. 7, 9395. doi: 10.1038/s41598-017-09923-6
Chen X., Zeng D., Chen X., Xie D., Zhao Y., Yang C., et al. (2013). Transcriptome analysis of Litopenaeus vannamei in response to white spot syndrome virus infection. PloS One 8, e73218. doi: 10.1371/journal.pone.0073218
Cicala F., Lago-Lestón A., Gomez-Gil B., Gollas-Galván T., Chong-Robles J., Cortés-Jacinto E., et al. (2020). Gut microbiota shifts in the giant tiger shrimp, Penaeus monodon, during the postlarvae, juvenile, and adult stages. Aquac. Int. 28, 1421–1433. doi: 10.1007/s10499-020-00532-1
Citarasu T., Sivaram V., Immanuel G., Rout N., Murugan V. (2006). Influence of selected Indian immunostimulant herbs against white spot syndrome virus (WSSV) infection in black tiger shrimp, Penaeus monodon with reference to haematological, biochemical and immunological changes. Fish Shellfish Immunol. 21, 372–384. doi: 10.1016/j.fsi.2006.01.002
Clemente J. C., Ursell L. K., Parfrey L. W., Knight R. (2012). The impact of the gut microbiota on human health: an integrative view. Cell 148, 1258–1270. doi: 10.1016/j.cell.2012.01.035
Colorni A. (1989). Fusariosis in the shrimp Penaeus semisulcatus cultured in Israel. Mycopathologia 108, 145–147. doi: 10.1007/BF00436066
Cowley J. A., Dimmock C. M., Spann K. M., Walker P. J. (2000). Gill-associated virus of Penaeus monodon prawns: an invertebrate virus with ORF1a and ORF1b genes related to arteri-and coronaviruses. J. Gen. Virol. 81, 1473–1484. doi: 10.1099/0022-1317-81-6-1473
Cowley J. A., Dimmock C. M., Wongteerasupaya C., Boonsaeng V., Panyim S., Walker P. J. (1999). Yellow head virus from Thailand and gill-associated virus from Australia are closely related but distinct prawn viruses. Dis. Aquat. Org. 36, 153–157. doi: 10.3354/dao036153
Cowley J. A., Walker P. J. (2002). The complete genome sequence of gill-associated virus of Penaeus monodon prawns indicates a gene organisation unique among nidoviruses. Arch. Virol. 147, 1977–1987. doi: 10.1007/s00705-002-0847-x
Criado-Fornelio A., Constantin E., Mialhe E., Grizel H. (1988). “Biochemical characterization of an isolate of Fusarium solani pathogenic for Penaeus japonicus,” in Third International Colloquium on Pathology in Marine Aquaculture (Virginia Institute of Marine Science, Gloucester Point, Virginia), 2–6.
Cuéllar-Anjel J., Chamoro R., White-Noble B., Schofield P., Lightner D. (2011). Testing finds resistance to WSSV in shrimp from Panamanian breeding program. Glob. Aquacult. Advocate, 65–66. doi: 10.33997/j.afs.2018.31.S1.005
Cuéllar-Anjel J., Morales-Covarrubias M. S., Varela-Mejías A., Elizondo-Ovares C. (2018). Shrimp bacterial infections in Latin America: a review. Asian Fish. Sci. 31S, 76–87.
De Decker S., Reynaud Y., Saulnier D. (2013). First molecular evidence of cross-species induction of metalloprotease gene expression in Vibrio strains pathogenic for Pacific oyster Crassostrea gigas involving a quorum sensing system. Aquaculture 392, 1–7. doi: 10.1016/j.aquaculture.2013.01.033
Defoirdt T., Sorgeloos P., Bossier P. (2011). Alternatives to antibiotics for the control of bacterial disease in aquaculture. Curr. Opin. Microbiol. 14, 251–258. doi: 10.1016/j.mib.2011.03.004
del Río-Rodríguez R. E., Soto-Rodríguez S., Lara-Flores M., Cu-Escamilla A. D., Gomez-Solano M. I. (2006). A necrotizing hepatopancreatitis (NHP) outbreak in a shrimp farm in Campeche, Mexico: a first case report. Aquaculture 255, 606–609. doi: 10.1016/j.aquaculture.2005.12.014
Derakhshani H., Tun H. M., Khafipour E. (2016). An extended single-index multiplexed 16S rRNA sequencing for microbial community analysis on MiSeq illumina platforms. J. Basic Microbiol. 56, 321–326. doi: 10.1002/jobm.201500420
De Silva S. S., Mohan C. V., Phillips M. J. (2007). A different form of dumping: The need for a precautionary approach for yet another new species for shrimp farming in Asia. Aquac. Asia. 12, 3–5.
Dhar A. K., Cowley J. A., Hasson K. W., Walker P. J. (2004). Genomic organization, biology, and diagnosis of Taura syndrome virus and yellowhead virus of penaeid shrimp. Adv. Virus Res. 63, 353–421. doi: 10.1016/S0065-3527(04)63006-5
Dhar A. K., Piamsomboon P., Caro L. F. A., Kanrar S., Adami R. Jr., Juan Y. S. (2019). First report of acute hepatopancreatic necrosis disease (AHPND) occurring in the USA. Dis. Aquat. Org. 132, 241–247. doi: 10.3354/dao03330
Doubrovsky A., Paynter J. L., Sambhi S. K., Atherton J. G., Lester R. J. G. (1988). Observations on the Ultrastracture of Baculovirus in Australian Penaeus monodon and Penaeus merguiensis. Mar. Freshw. Res. 39, 743–749. doi: 10.1071/MF9880743
Duda T. F. Jr., Palumbi S. R. (1999). Population structure of the black tiger prawn, Penaeus monodon, among western Indian Ocean and western Pacific populations. Mar. Biol. 134, 705–710. doi: 10.1007/s002270050586
Edwards K. J., Logan J. M., Langham S., Swift C., Gharbia S. E. (2012). Utility of real-time amplification of selected 16S rRNA gene sequences as a tool for detection and identification of microbial signatures directly from clinical samples. J. Med. Microbiol. 61, 645–652. doi: 10.1099/jmm.0.041764-0
Eshik M. M. E., Abedin M. M., Punom N. J., Begum M. K., Rahman M. S. (2017). Molecular identification of AHPND positive Vibrio parahaemolyticus causing an outbreak in south-west shrimp farming regions of Bangladesh. J. Bangladesh Acad. Sci. 41, 127–135. doi: 10.3329/jbas.v41i2.35492
Fegan D. F., Clifford H. C. (2001). “Health management for viral diseases in shrimp farms,” in The New Wave, Proceedings of the Special Session on Sustainable Shrimp Culture, Aquaculture. Eds. Browdy C. L., Jory D. E. (The World Aquaculture Society, Sorrento, Louisiana), 168–198.
Flegel T. W. (2006). Detection of major penaeid shrimp viruses in Asia, a historical perspective with emphasis on Thailand. Aquaculture 258, 1–33. doi: 10.1016/j.aquaculture.2006.05.013
Flegel T. W. (2019). A future vision for disease control in shrimp aquaculture. J. World Aquac. Soc 50, 249–266. doi: 10.1111/jwas.12589
Flegel T. W., Alday-Sanz V. (1998). The crisis in Asian shrimp aquaculture: current status and future needs. J. Appl. Ichthyol. 14, 269–273. doi: 10.1111/j.1439-0426.1998.tb00654.x
Flegel T. W., Lightner D. V., Lo C. F., Owens L. (2008). Shrimp disease control: past, present and future. Asian Fish. Soc 505, 355–377.
Fournier P. E., Dubourg G., Raoult D. (2014). Clinical detection and characterization of bacterial pathogens in the genomics era. Genome Med. 6, 1–15. doi: 10.1186/s13073-014-0114-2
Ganjoor M. (2015). A short review on infectious viruses in cultural shrimps (Penaeidae family). J. FisheriesSciences.com 9, 9–33. doi: 10.4172/2150-3508
Garibay-Valdez E., Martínez-Córdova L. R., Vargas-Albores F., Gollas-Galván T., Lago-Leston A., Calderón K., et al. (2019). Biofilm consumption shapes the intestinal microbiota of shrimp (Penaeus vannamei). Aquac. Nutr. 25, 427–435. doi: 10.1111/anu.2019.25.issue-2
Gitterle T., Salte R., Gjerde B., Cock J., Johansen H., Salazar M., et al. (2005). Genetic (co) variation in resistance to White Spot Syndrome Virus (WSSV) and harvest weight in Penaeus (Litopenaeus) vannamei. Aquaculture 246, 139–149. doi: 10.1016/j.aquaculture.2005.02.011
Goldberg C. S., Turner C. R., Deiner K., Klymus K. E., Thomsen P. F., Murphy M. A., et al. (2016). Critical considerations for the application of environmental DNA methods to detect aquatic species. Methods Ecol. Evol. 7, 1299–1307. doi: 10.1111/2041-210X.12595
Gootenberg J. S., Abudayyeh O. O., Kellner M. J., Joung J., Collins J. J., Zhang F. (2018). Multiplexed and portable nucleic acid detection platform with Cas13, Cas12a, and Csm6. Science 360, 439–444. doi: 10.1126/science.aaq0179
Gootenberg J. S., Abudayyeh O. O., Lee J. W., Essletzbichler P., Dy A. J., Joung J., et al. (2017). Nucleic acid detection with CRISPR-Cas13a/C2c2. Science 356, 438–442. doi: 10.1126/science.aam9321
Govindaraju K., Dilip Itroutwar P., Veeramani V., Ashok Kumar T., Tamilselvan S. (2020). Application of nanotechnology in diagnosis and disease management of white spot syndrome virus (WSSV) in aquaculture. J. Clust. Sci. 31, 1163–1171. doi: 10.1007/s10876-019-01724-3
Guo L., Xu Y. H., Zhang N., Zhou F. L., Huang J. H., Liu B. S., et al. (2019). A high-density genetic linkage map and QTL mapping for sex in black tiger shrimp (Penaeus monodon). Front. Genet. 10. doi: 10.3389/fgene.2019.00326
Guérin-Méneville F. É. (1857). Description of Trichocorixa reticulata. Revue et magasin de zoologie pure et appliquée 9, 123–125.
Ha Y. M., Gong S. J., Nguyen T. H., Ra C. H., Kim K. H., Nam Y. K., et al. (2008). Vaccination of shrimp (Penaeus chinensis) against white spot syndrome virus (WSSV). J. Microbiol. Biotechnol. 18, 964–967.
Hamady M., Knight R. (2009). Microbial community profiling for human microbiome projects: tools, techniques, and challenges. Genome Res. 19, 1141–1152. doi: 10.1101/gr.085464.108
Holt C. C., Bass D., Stentiford G. D., van der Giezen M. (2021). Understanding the role of the shrimp gut microbiome in health and disease. J. Invertebr. Pathol. 186, 107387. doi: 10.1016/j.jip.2020.107387
Hsu J. C. K., Hsu T. K., Kannan J., Wang H. C., Tassanakajon A., Chen L. L. (2022). Diagnostic performance of a Rapid Test Kit for white spot syndrome virus (WSSV). Aquaculture 558, 738379. doi: 10.1016/j.aquaculture.2022.738379
Huang W., Cheng C., Liu J., Zhang X., Ren C., Jiang X., et al. (2020). Fine mapping of the high-pH tolerance and growth trait-related quantitative trait loci (QTLs) and identification of the candidate genes in Pacific white shrimp (Litopenaeus vannamei). Mar. Biotechnol. 22, 1–18. doi: 10.1007/s10126-019-09932-8
Huang Y. C., Yin Z. X., Ai H. S., Huang X. D., Li S. D., Weng S. P., et al. (2011). Characterization of WSSV resistance in selected families of Litopenaeus vannamei. Aquaculture 311, 54–60. doi: 10.1016/j.aquaculture.2010.11.032
Huerlimann R., Wade N. M., Gordon L., Montenegro J. D., Goodall J., McWilliam S., et al. (2018). De novo assembly, characterization, functional annotation and expression patterns of the black tiger shrimp (Penaeus monodon) transcriptome. Sci. Rep. 8, 13553. doi: 10.1038/s41598-018-31148-4
Hussein M. M. A., Hassan W. H., Abd El-Ghany N. N. A., Ghanem N. H. (2024). Cumulative mortalities in white leg shrimp, Litopenaeus vannamei Boone 1931, cultured in biofloc system in Egypt reflected new record of Fusarium verticillioides infection. Aquacult Int. 32, 562–575. doi: 10.1007/s10499-024-01513-4
Ishimaru K., Akagawa-Matsushita M., Muroga K. (1995). Vibrio penaeicida sp. nov., a pathogen of kuruma prawns (Penaeus japonicus). Int. J. Syst. Evol. Microbiol. 45, 134–138. doi: 10.1099/00207713-45-1-134
Islam S. I., Ahammad F., Mohammed H. (2024). Cutting-edge technologies for detecting and controlling fish diseases: Current status, outlook, and challenges. J. World Aquaculture Soc. 55, e13051. doi: 10.1111/jwas.13051
Itsathitphaisarn O., Thitamadee S., Weerachatyanukul W., Sritunyalucksana K. (2017). Potential of RNAi applications to control viral diseases of farmed shrimp. J. Invertebr. Pathol. 147, 76–85. doi: 10.1016/j.jip.2016.11.006
Jiang D., Rocha J., Ciobanu D., Mileham A., van der Steen H., White-Noble B., et al. (2004). Quantitative, molecular genetic selection for shrimp disease resistance. Genetic improvement. Glob. Aquacult. Advocate, 52–55.
Johnson K. N., van Hulten M. C., Barnes A. C. (2008). Vaccination” of shrimp against viral pathogens: phenomenology and underlying mechanisms. Vaccine 26, 4885–4892. doi: 10.1016/j.vaccine.2008.07.019
Jothiswaran V., Velumani T., Jayaraman R. (2020). Application of artificial intelligence in fisheries and aquaculture. Biotica Res. Today 2, 499–502.
Jun J. W., Han J. E., Giri S. S., Tang K. F., Zhou X., Aranguren L. F., et al. (2018). Phage application for the protection from acute hepatopancreatic necrosis disease (AHPND) in Penaeus vannamei. Indian J. Microbiol. 58, 114–117. doi: 10.1007/s12088-017-0694-9
Kalagayan H., Godin D., Kanna R., Hagino G., Sweeney J., Wyban J., et al. (1991). IHHN virus as an etiological factor in runt-deformity syndrome (RDS) of juvenile Penaeus vannamei cultured in Hawaii. J. World Aquac. Soc 22, 235–243. doi: 10.1111/j.1749-7345.1991.tb00740.x
Kalatzis P. G., Castillo D., Katharios P., Middelboe M. (2018). Bacteriophage interactions with marine pathogenic Vibrios: implications for phage therapy. Antibiotics 7, 15. doi: 10.3390/antibiotics7010015
Karunasagar I., Karunasagar I., Umesha R. K. (2004).Microbial diseases in shrimp aquaculture. Available at: https://drs.nio.org/drs/bitstream/handle/2264/78/Karunasagar_chap13.pdf?sequence=1 (Accessed May 30, 2021).
Karunasagar I., Pai R., Malathi G. R., Karunasagar I. (1994). Mass mortality of Penaeus monodon larvae due to antibiotic-resistant Vibrio harveyi infection. Aquaculture 128, 203–209. doi: 10.1016/0044-8486(94)90309-3
Katneni V. K., Shekhar M. S., Jangam A. K., Prabhudas S. K., Krishnan K., Kaikkolante N., et al. (2020). Novel isoform sequencing based full-length transcriptome resource for Indian white shrimp, penaeus indicus. Front. Mar. Sci. 7. doi: 10.3389/fmars.2020.60
Kommedal Ø., Karlsen B., Sæbø Ø. (2008). Analysis of mixed sequencing chromatograms and its application in direct 16S rRNA gene sequencing of polymicrobial samples. J. Clin. Microbiol. 46, 3766–3771. doi: 10.1128/JCM.00213-08
Kong J., Zhang Q., Liu P., Yue Z., Wang Q. (2003). Selective breeding raises WSSV resistance in fleshy prawns. Glob. Aquacult. Advocate 6, 25–26.
Konstantinidis K. T., Tiedje J. M. (2005). Genomic insights that advance the species definition for prokaryotes. Proc. Natl. Acad. Sci. U.S.A. 102, 2567–2572. doi: 10.1073/pnas.0409727102
Krabsetsve K., Cullen B. R., Owens L. (2004). Rediscovery of the Australian strain of infectious hypodermal and haematopoietic necrosis virus. Dis. Aquat. Org. 61, 153–158. doi: 10.3354/dao061153
Kumar M., Gupta G., Vikas, Sharma S. (2018). Feed based vaccine in aquaculture farm. Mar. Biol. 1, 180013.
Kumar R., Ng T. H., Wang H. C. (2020). Acute hepatopancreatic necrosis disease in penaeid shrimp. Rev. Aquac. 12, 1867–1880. doi: 10.1111/raq.12414
Kumar S., Verma A. K., Singh S. P., Awasthi A. (2023). Immunostimulants for shrimp aquaculture: paving pathway towards shrimp sustainability. Environ. Sci. pollut. Res. 30, 25325–25343. doi: 10.1007/s11356-021-18433-y
Lai H. C., Ng T. H., Ando M., Lee C. T., Chen I. T., Chuang J. C., et al. (2015). Pathogenesis of acute hepatopancreatic necrosis disease (AHPND) in shrimp. Fish Shellfish Immunol. 47, 1006–1014. doi: 10.1016/j.fsi.2015.11.008
Lavilla-Pitogo C. R., de la Pena L. D. (1998). Bacterial diseases in shrimp (Penaeus monodon) culture in the Philippines. Fish Pathol. 33, 405–411. doi: 10.3147/jsfp.33.405
Lee C. L., Chang C. C., Kuo H. W., Cheng W. (2020). Pectin of cacao pod husk, an efficient immunostimulant for white shrimp, Litopenaeusvannamei. Fish Shellfish Immunol. 107, 357–366. doi: 10.1016/j.fsi.2020.10.026
Lee C. T., Chen I. T., Yang Y. T., Ko T. P., Huang Y. T., Huang J. Y., et al. (2015). The opportunistic marine pathogen Vibrio parahaemolyticus becomes virulent by acquiring a plasmid that expresses a deadly toxin. Proc. Natl. Acad. Sci. U.S.A. 112, 10798–10803. doi: 10.1073/pnas.1503129112
Lee D., Yu Y.-B., Choi J.-H., Jo A.-H., Hong S.-M., Kang J.-C., et al. (2022). Viral shrimp diseases listed by the OIE: a review. Viruses. 14 (3), 585. doi: 10.3390/v14030585
Lei Y., Chen H., Dai H., Zeng Z., Lin Y., Zhou F., et al. (2008). Electroless-plated gold films for sensitive surface plasmon resonance detection of white spot syndrome virus. Biosens. Bioelectron. 23, 1200–1207. doi: 10.1016/j.bios.2007.10.004
Li D., Li C. (2020). Intelligent aquaculture. J. World Aquaculture Soc. 51, 808–814. doi: 10.1111/jwas.12736
Li C., Weng S., Chen Y., Yu X., Lü L., Zhang H., et al. (2012). Analysis of Litopenaeus vannamei transcriptome using the next-generation DNA sequencing technique. PloS One 7, e47442. doi: 10.1371/journal.pone.0047442
Li K., Zheng T., Tian Y., Xi F., Yuan J., Zhang G., et al. (2007). Beneficial effects of Bacillus licheniformis on the intestinal microflora and immunity of the white shrimp, Litopenaeus vannamei. Biotechnol. Lett. 29, 525–530. doi: 10.1007/s10529-006-9291-4
Lightner D. V. (1988). “Diseases of cultured penaeid shrimp and prawns,” in Disease diagnosis and control in North American marine aquaculture. Eds. Sinderman C. J., Lightner D. V. (Elsevier, Amsterdam, Netherlands), 8–127.
Lightner D. V. (1993). “Diseases of cultured penaeid shrimp,” in CRC Handbook of Mariculture: Crustacean Aquaculture. Ed. McVey J. P. (CRC Press, Boca Raton, Florida), 289–320.
Lightner D. V. (1996a). A handbook of shrimp pathology and diagnostic procedures for diseases of cultured penaeid shrimp (Baton Rouge, LA: World Aquaculture Society).
Lightner D. V. (1996b). Epizootiology, distribution and the impact on international trade of two penaeid shrimp viruses in the Americas. Rev. Sci. Tech. 15, 579–601. doi: 10.20506/rst.issue.15.2.2491
Lightner D. V. (1999). The penaeid shrimp viruses TSV, IHHNV, WSSV, and YHV: current status in the Americas, available diagnostic methods, and management strategies. J. Appl. Aquac. 9, 27–52. doi: 10.1300/J028v09n02_03
Lightner D. V. (2003). “Exclusion of specific pathogens for disease control in a penaeid shrimp biosecurity program,” in Biosecurity in aquaculture production systems: Exclusion of pathogens and other undesirables. Eds. Lee C. S., O’Bryen P. J. (The World Aquaculture Society, Baton Rouge, Louisiana), 81–116.
Lightner D. V. (2011). Virus diseases of farmed shrimp in the Western Hemisphere (the Americas): a review. J. Invertebr. Pathol. 106, 110–130. doi: 10.1016/j.jip.2010.09.012
Lightner D. V., Hedrick R. P., Fryer J. L., Chen S. N., Liao I. C., Kou G. H. (1987). A survey of cultured penaeid shrimp in Taiwan for viral and other important diseases. Fish Pathol. 22, 127–140. doi: 10.3147/jsfp.22.127
Lightner D. V., Moore D., Danald D. A. (1979). “A mycotic disease of cultured penaeid shrimp caused by the fungus Fusarium solani,” in Proc. Second Biennial Crustacean Health Workshop. Eds. Lewis D. H., Leong J. K. (Texas A&M University, College Station, Texas), 137–158.
Lightner D. V., Redman R. M. (1998). Shrimp diseases and current diagnostic methods. Aquaculture. 164 (1-4), 201–220. doi: 10.1016/S0044-8486(98)00187-2
Lightner D. V., Redman R. M., Bonami J. R. (1992). Morphological evidence for a single bacterial etiology in Texas necrotizing hepatopancreatitis in Penaeus vannamei (Crustacea: Decapoda). Dis. Aquat. Org. 13, 235–239. doi: 10.3354/dao013235
Lightner D. V., Redman R. M., Pantoja C. R., Tang K. F. J., Noble B. L., Schofield P., et al. (2012). Historic emergence, impact and current status of shrimp pathogens in the Americas. J. Invertebr. Pathol. 110, 174–183. doi: 10.1016/j.jip.2012.03.006
Limsuwan C. (1991). Handbook for cultivation of black tiger prawns (Bangkok, Thailand: Tansetakit Co. Ltd).
Lin L., Zhang J. (2017). Role of intestinal microbiota and metabolites on gut homeostasis and human diseases. BMC Immunol. 18, 1–25. doi: 10.1186/s12865-016-0187-3
Liu Q., Huang J., Yang H., Yang B., Liu S., Wang H., et al. (2014). Detection of a new genotype of yellow-head virus in farmed shrimp suspicious of EMS/AHPNS infection. Oceanologia Limnologia Sinica/Hai Yang Yu Hu Chao 45, 703–709.
Lomelí-Ortega C. O., Martínez-Díaz S. F. (2014). Phage therapy against Vibrio parahaemolyticus infection in the whiteleg shrimp (Litopenaeus vannamei) larvae. Aquaculture 434, 208–211. doi: 10.1016/j.aquaculture.2014.08.018
Lotz J. M. (1997). Viruses, biosecurity and specific pathogen-free stocks in shrimp aquaculture. World J. Microbiol. Biotechnol. 13, 405–413. doi: 10.1023/A:1018572132529
Loy J. K., Frelier P. F., Varner P., Templeton J. W. (1996). Detection of the etiologic agent of necrotizing hepatopancreatitis in cultured Penaeus vannamei from Texas and Peru by polymerase chain reaction. Dis. Aquat. Org. 25, 117–122. doi: 10.3354/dao025117
Loy J. D., Loy D. S., Mogler M. A., Janke B., Kamrud K., Harris D. H., et al. (2013). Sequence-optimized and targeted double-stranded RNA as a therapeutic antiviral treatment against infectious myonecrosis virus in Litopenaeus vannamei. Dis. Aquat. Org. 105, 57–64. doi: 10.3354/dao02600
Lundin C. G. (1995).Global attempts to address shrimp disease. In: Report of the land, water and natural habitats division, environment department (The World Bank, Washington). Available at: http://library.enaca.org/Shrimp/Publications/ShrimpDisease.pdf (Accessed May 26, 2021).
Major S. R., Harke M. J., Cruz-Flores R., Dhar A. K., Bodnar A. G., Wanamaker S. A. (2023). Rapid detection of DNA and RNA shrimp viruses using CRISPR-based diagnostics. Appl. Environ. Microbiol. 89, e0215122. doi: 10.1128/aem.02151-22
Malik S., Kumar T., Sahoo A. (2017a). Image Processing Techniques for Identification of Fish Disease. In IEEE 2nd International Conference on Signal and Image Processing (ICSIP). New York, NY: IEEE, pp. 55–59. doi: 10.1109/SIPROCESS.2017.8124505
Malik S., Kumar T., Sahoo A. (2017b). A novel approach to fish disease diagnostic system based on machine learning. Adv. Image Video Process. 5, 49–57. doi: 10.14738/aivp.51.2017
Maralit B. A., Komatsu M., Hipolito S. G., Hirono I., Kondo H. (2015). Microarray analysis of immunity against WSSV in response to injection of non-specific long dsRNA in kuruma shrimp, Marsupenaeus japonicus. Mar. Biotechnol. 17, 493–501. doi: 10.1007/s10126-015-9637-9
Mari J., Bonami J. R., Lightner D. (1993). Partial cloning of the genome of infectious hypodermal and haematopoietic necrosis virus, an unusual parvovirus pathogenic for penaeid shrimps; diagnosis of the disease using a specific probe. J. Gen. Virol. 74, 2637–2643. doi: 10.1099/0022-1317-74-12-2637
Mari J., Poulos B. T., Lightner D. V., Bonami J. R. (2002). Shrimp Taura syndrome virus: genomic characterization and similarity with members of the genus Cricket paralysis-like viruses. J. Gen. Virol. 83, 915–926. doi: 10.1099/0022-1317-83-4-915
Martínez-Porchas M., Vargas-Albores F. (2017). Microbial metagenomics in aquaculture: a potential tool for a deeper insight into the activity. Rev. Aquac. 9, 42–56. doi: 10.1111/raq.12102
Metzger D. C., Schulte P. M. (2016). Epigenomics in marine fishes. Mar. Genom. 30, 43–54. doi: 10.1016/j.margen.2016.01.004
Mohan C. V., Shankar K. M., Kulkarni S., Sudha P. M. (1998). Histopathology of cultured shrimp showing gross signs of yellow head syndrome and white spot syndrome during 1994 Indian epizootics. Dis. Aquat. Org. 34, 9–12. doi: 10.3354/dao034009
Mohr P. G., Moody N. J., Hoad J., Williams L. M., Bowater R. O., Cummins D. M., et al. (2015). New yellow head virus genotype (YHV7) in giant tiger shrimp Penaeus monodon indigenous to northern Australia. Dis. Aquat. Org. 115, 263–268. doi: 10.3354/dao02894
Momtaz F., Foysal J., Rahman M., Fotedar R. (2019). Design of epitope based vaccine against shrimp white spot syndrome virus (WSSV) by targeting the envelope proteins: an immunoinformatic approach. Turkish J. Fish. Aquat. Sci. 19, 59–69. doi: 10.4194/1303-2712-v19_2_07
Mondal H., Thomas J. (2022). A review on the recent advances and application of vaccines against fish pathogens in aquaculture. Aquac. Int. 30 (4), 1971–2000. doi: 10.1007/s10499-022-00884-w
Moorthie S., Mattocks C. J., Wright C. F. (2011). Review of massively parallel DNA sequencing technologies. Hugo J. 5, 1–12. doi: 10.1007/s11568-011-9156-3
Moss D. R., Arce A., Otoshi C. A., Moss S. M. (2011). Shrimp breeding for resistance to Taura syndrome virus. Glob. Aquacult. Advocate 36, 40–41.
Motte E., Yugcha E., Luzardo J., Castro F., Leclercq G., Rodríguez J., et al. (2003). Prevention of IHHNV vertical transmission in the white shrimp Litopenaeus vannamei. Aquaculture 219, 57–70. doi: 10.1016/S0044-8486(02)00631-2
Myhrvold C., Freije C. A., Gootenberg J. S., Abudayyeh O. O., Metsky H. C., Durbin A. F., et al. (2018). Field-deployable viral diagnostics using CRISPR-Cas13. Science 360, 444–448. doi: 10.1126/science.aas8836
Nakai T., Sugimoto R., Park K. H., Matsuoka S., Mori K. I., Nishioka T., et al. (1999). Protective effects of bacteriophage on experimental Lactococcus garvieae infection in yellowtail. Dis. Aquat. Org. 37, 33–41. doi: 10.3354/dao037033
Namikoshi A., Wu J. L., Yamashita T., Nishizawa T., Nishioka T., Arimoto M., et al. (2004). Vaccination trials with Penaeus japonicus to induce resistance to white spot syndrome virus. Aquaculture 229, 25–35. doi: 10.1016/S0044-8486(03)00363-6
Natarajan A., Devi K. S., Raja S., Senthil Kumar A. (2017). An elegant analysis of white spot syndrome virus using a graphene oxide/methylene blue based electrochemical immunosensor platform. Sci. Rep. 7, 46169. doi: 10.1038/srep46169
Netea M. G., Quintin J., van der Meer J. W. (2011). Trained immunity: a memory for innate host defense. Cell Host Microbe 9, 355–361. doi: 10.1016/j.chom.2011.04.006
Newaj-Fyzul A., Al-Harbi A. H., Austin B. (2014). Developments in the use of probiotics for disease control in aquaculture. Aquaculture 431, 1–11. doi: 10.1016/j.aquaculture.2013.08.026
Nimitphak T., Kiatpathomchai W., Flegel T. W. (2008). Shrimp hepatopancreatic parvovirus detection by combining loop-mediated isothermal amplification with a lateral flow dipstick. J. Virol. Methods 154, 56–60. doi: 10.1016/j.jviromet.2008.09.003
Ninawe A. S., Selvin J. (2009). Probiotics in shrimp aquaculture: avenues and challenges. Crit. Rev. Microbiol. 35, 43–66. doi: 10.1080/10408410802667202
Noman M., Kazmi S. S. U. H., Saqib H. S. A., Fiaz U., Pastorino P., Barcelò D., et al. (2024). Harnessing probiotics and prebiotics as eco-friendly solution for cleaner shrimp aquaculture production: A state of the art scientific consensus. Sci. Total Environ. 915, 169921. doi: 10.1016/j.scitotenv.2024.169921
Nunan L., Lightner D., Pantoja C., Gomez-Jimenez S. (2014). Detection of acute hepatopancreatic necrosis disease (AHPND) in Mexico. Dis. Aquat. Org. 111, 81–86. doi: 10.3354/dao02776
Nur’aini Y. L. (2009). Infectious myonecrosis virus (IMNV) in Pacific white shrimp (Litopenaeus vannamei) in Indonesia. Isr. J. Aquac. 61, 255–262. doi: 10.46989/001c.20553
Ochoa L. M., Cruz-Flores R., Dhar A. K. (2020). Detection and phylogenetic analyses of taura syndrome virus from archived davidson’s-fixed paraffin-embedded shrimp tissue. Viruses 12, 1030. doi: 10.3390/v12091030
Oliveira J., Castilho F., Cunha A., Pereira M. J. (2012). Bacteriophage therapy as a bacterial control strategy in aquaculture. Aquac. Int. 20, 879–910. doi: 10.1007/s10499-012-9515-7
Padiyar A. (2009). Epidemiology and management of Penaeus monodon farms in Andhra Pradesh, India. Deakin University, Victoria, Australia. Doctoral dissertation/PhD Thesis.
Pande G. S. J., Scheie A. A., Benneche T., Wille M., Sorgeloos P., Bossier P., et al. (2013). Quorum sensing-disrupting compounds protect larvae of the giant freshwater prawn Macrobrachium rosenbergii from Vibrio harveyi infection. Aquaculture 406, 121–124. doi: 10.1016/j.aquaculture.2013.05.015
Pawar S., Lahiri C. (2018). Quorum sensing: An imperative longevity weapon in bacteria. Afr. J. Microbiol. Res. 12, 96–104. doi: 10.5897/AJMR2017.8751
Peng J., Wei P., Zhang B., Zhao Y., Zeng D., Chen X., et al. (2015). Gonadal transcriptomic analysis and differentially expressed genes in the testis and ovary of the Pacific white shrimp (Litopenaeus vannamei). BMC Genom. 16, 1006. doi: 10.1186/s12864-015-2219-4
Peruzza L., Shekhar M. S., Kumar K. V., Swathi A., Karthic K., Hauton C., et al. (2019). Temporal changes in transcriptome profile provide insights of White Spot Syndrome Virus infection in Litopenaeus vannamei. Sci. Rep. 9, 13509. doi: 10.1038/s41598-019-49836-0
Peruzza L., Thamizhvanan S., Vimal S., Kumar K. V., Shekhar M. S., Smith V. J., et al. (2020). A comparative synthesis of transcriptomic analyses reveals major differences between WSSV-susceptible Litopenaeus vannamei and WSSV-refractory Macrobrachium rosenbergii. Dev. Comp. Immunol. 104, 103564. doi: 10.1016/j.dci.2019.103564
Pilotto M. R., Goncalves A. N., Vieira F. N., Seifert W. Q., Bachère E., Rosa R. D., et al. (2018). Exploring the impact of the biofloc rearing system and an oral WSSV challenge on the intestinal bacteriome of Litopenaeus vannamei. Microorganisms 6, 83. doi: 10.3390/microorganisms6030083
Ping S. L., Liem T. T. (2000). Predicting shrimp disease occurrence: artificial neural networks vs. logistic regression. Aquaculture 187, 35–49. doi: 10.1016/S0044-8486(00)00300-8
Poulos B., Tang K., Pantoja C., Bonami J. R., Lightner D. (2006). Purification and characterization of infectious myonecrosis virus of penaeid shrimp. J. Gen. Virol. 87, 987–996. doi: 10.1099/vir.0.81127-0
Prachumwat A., Taengchaiyaphum S., Mungkongwongsiri N., Aldama-Cano D. J., Flegel T. W., Sritunyalucksana K. (2019). Update on early mortality syndrome/acute hepatopancreatic necrosis disease by April 2018. J. World Aquac. Soc 50, 5–17. doi: 10.1111/jwas.12559
Quintin J., Cheng S. C., van der Meer J. W., Netea M. G. (2014). Innate immune memory: towards a better understanding of host defense mechanisms. Curr. Opin. Immunol. 29, 1–7. doi: 10.1016/j.coi.2014.02.006
Rai P., Pradeep B., Karunasagar I., Karunasagar I. (2009). Detection of viruses in Penaeus monodon from India showing signs of slow growth syndrome. Aquaculture 289, 231–235. doi: 10.1016/j.aquaculture.2008.12.035
Rajendran K. V., Makesh M., Karunasagar I. (2012). Monodon baculovirus of shrimp. Indian J. Virol. 23, 149–160. doi: 10.1007/s13337-012-0086-z
Richards G. P. (2014). Bacteriophage remediation of bacterial pathogens in aquaculture: a review of the technology. Bacteriophage 4, e975540. doi: 10.4161/21597081.2014.975540
Rintala A., Pietilä S., Munukka E., Eerola E., Pursiheimo J. P., Laiho A., et al. (2017). Gut microbiota analysis results are highly dependent on the 16S rRNA gene target region, whereas the impact of DNA extraction is minor. J. Biomol. Tech. 28, 19–30. doi: 10.7171/jbt.17-2801-003
Ritchie L. E., Steiner J. M., Suchodolski J. S. (2008). Assessment of microbial diversity along the feline intestinal tract using 16S rRNA gene analysis. FEMS Microbiol. Ecol. 66, 590–598. doi: 10.1111/fem.2008.66.issue-3
Rizan N., Yew C. Y., Niknam M. R., Krishnasamy J., Bhassu S., Hong G. Z., et al. (2018). Electronic properties of synthetic shrimp pathogens-derived DNA schottky diodes. Sci. Rep. 8, 896. doi: 10.1038/s41598-017-18825-6
Robalino J., Bartlett T., Shepard E., Prior S., Jaramillo G., Scura E., et al. (2005). Double-stranded RNA induces sequence-specific antiviral silencing in addition to nonspecific immunity in a marine shrimp: convergence of RNAInterference and innate immunity in the invertebrate antiviral response? J. Virol. 79, 13561–13571. doi: 10.1128/JVI.79.21.13561-13571.2005
Robalino J., Browdy C. L., Prior S., Metz A., Parnell P., Gross P., et al. (2004). Induction of antiviral immunity by double-stranded RNA in a marine invertebrate. J. Virol. 78, 10442–10448. doi: 10.1128/JVI.78.19.10442-10448.2004
Robinson N. A., Gopikrishna G., Baranski M., Katneni V. K., Shekhar M. S., Shanmugakarthik J., et al. (2014). QTL for white spot syndrome virus resistance and the sex-determining locus in the Indian black tiger shrimp (Penaeus monodon). BMC Genom. 15, 731. doi: 10.1186/1471-2164-15-731
Rodriguez-Anaya L. Z., Casillas-Hernández R., Lares-Jiménez L. F., Gonzalez-Galaviz J. R. (2018). Next-generation sequencing technologies and the improvement of aquaculture sustainability of Pacific white shrimp (Litopenaeus vannamei). Curr. Sci. 115, 202–203. doi: 10.18520/cs/v115/i2/202-203
Romestand B., Bonami J. R. (2003). A sandwich enzyme linked immunosorbent assay (S-ELISA) for detection of MrNV in the giant freshwater prawn, Macrobrachium rosenbergii (de Man). J. Fish Dis. 26, 71–75. doi: 10.1046/j.1365-2761.2003.00432.x
Round J. L., Mazmanian S. K. (2009). The gut microbiota shapes intestinal immune responses during health and disease. Nat. Rev. Immunol. 9, 313–323. doi: 10.1038/nri2515
Rungrassamee W., Klanchui A., Chaiyapechara S., Maibunkaew S., Tangphatsornruang S., Jiravanichpaisal P., et al. (2013). Bacterial population in intestines of the black tiger shrimp (Penaeus monodon) under different growth stages. PloS One 8, e60802. doi: 10.1371/journal.pone.0060802
Rungrassamee W., Klanchui A., Maibunkaew S., Karoonuthaisiri N. (2016). Bacterial dynamics in intestines of the black tiger shrimp and the Pacific white shrimp during Vibrio harveyi exposure. J. Invertebr. Pathol. 133, 12–19. doi: 10.1016/j.jip.2015.11.004
Sahul Hameed A. S., Abdul Majeed S., Vimal S., Madan N., Rajkumar T., Santhoshkumar S., et al. (2017). Studies on the occurrence of infectious myonecrosis virus in pond-reared Litopenaeus vannamei (Boone 1931) in India. J. Fish Dis. 40, 1823–1830. doi: 10.1111/jfd.12655
Sangamaheswaran A. P., Jeyaseelan M. J. P. (2001). White spot viral disease in penaeid shrimp-A review. Naga ICLARM Q. 24, 16–22.
Santos H. M., Tsai C. Y., Maquiling K. R. A., Tayo L. L., Mariatulqabtiah A. R., Lee C. W., et al. (2020). Diagnosis and potential treatments for acute hepatopancreatic necrosis disease (AHPND): a review. Aquac. Int. 28, 169–185. doi: 10.1007/s10499-019-00451-w
See S. A., Goh Z. H., Chan Y. Y., Chong K. E., Tan G. Y. A., Bhassu S., et al. (2021). Biosafety evaluation and detection of shrimp viruses on field samples using dual priming oligonucleotide (DPO) system based multiplex PCR assay. Gene Rep. 23, 101158. doi: 10.1016/j.genrep.2021.101158
Senapin S., Phewsaiya K., Briggs M., Flegel T. W. (2007). Outbreaks of infectious myonecrosis virus (IMNV) in Indonesia confirmed by genome sequencing and use of an alternative RT-PCR detection method. Aquaculture 266, 32–38. doi: 10.1016/j.aquaculture.2007.02.026
Shaw J. L., Weyrich L., Cooper A. (2016). Using environmental (e) DNA sequencing for aquatic biodiversity surveys: a beginner’s guide. Mar. Freshw. Res. 68, 20–33. doi: 10.1071/MF15361
Shekhar M. S., Katneni V. K., Jangam A. K., Krishnan K., Kaikkolante N., Vijayan K. K. (2021). The genomics of the farmed shrimp: current status and application. Rev. Fish. Sci. Aquac. 29, 654–665. doi: 10.1080/23308249.2020.1858271
Shinn A. P., Pratoomyot J., Griffiths D., Trong T. Q., Vu N. T., Jiravanichpaisal P., et al. (2018). Asian shrimp production and the economic costs of disease. Asian Fish. Sci. 31, 29–58. doi: 10.33997/j.afs.2018.31.S1.003
Sithigorngul W., Rukpratanporn S., Pecharaburanin N., Longyant S., Chaivisuthangkura P., Sithigorngul P. (2006). A simple and rapid immunochromatographic test strip for detection of white spot syndrome virus (WSSV) of shrimp. Dis. Aquat. Org. 72, 101–106. doi: 10.3354/dao072101
Sivasankar P., John K. R., George M. R., Anushalini S. V., Kaviarasu D., Petchimuthu M. (2017). Prophylactics in shrimp aquaculture health management: a review. J. Entomol. Zool. Stud. 5, 1049–1055.
Slatko B. E., Gardner A. F., Ausubel F. M. (2018). Overview of next-generation sequencing technologies. Curr. Protoc. Mol. Biol. 122, e59. doi: 10.1002/cpmb.59
Solanki H. G., Bhatt J. H., Gopal C., Patil P. K., Pillai S. M. (2015). Effect of Vibrio bacterial product CIBASTIM administration on productivity in commercial tiger shrimp Penaeus monodon culture ponds in Gujarat. J. Appl. Aquac. 27, 107–112. doi: 10.1080/10454438.2015.1006495
Soo T. C. C., Bhassu S. (2022). Biochemical indexes and gut microbiota testing as diagnostic methods for Penaeus monodon health and physiological changes during AHPND infection with food safety concerns. Food Sci. Nutr. 10 (8), 2694–2709. doi: 10.1002/fsn3.2873
Sookruksawong S., Sun F., Liu Z., Tassanakajon A. (2013). RNA-Seq analysis reveals genes associated with resistance to Taura syndrome virus (TSV) in the Pacific white shrimp Litopenaeus vannamei. Dev. Comp. Immunol. 41, 523–533. doi: 10.1016/j.dci.2013.07.020
Spann K. M., Cowley J. A., Walker P. J., Lester R. J. G. (1997). A yellow-head-like virus from Penaeus monodon cultured in Australia. Dis. Aquat. Org. 31, 169–179. doi: 10.3354/dao031169
Staelens J., Rombaut D., Vercauteren I., Argue B., Benzie J., Vuylsteke M. (2008). High-density linkage maps and sex-linked markers for the black tiger shrimp (Penaeus monodon). Genetics 179, 917–925. doi: 10.1534/genetics.107.080150
Stalin N., Srinivasan P. (2017). Efficacy of potential phage cocktails against Vibrio harveyi and closely related Vibrio species isolated from shrimp aquaculture environment in the south east coast of India. Vet. Microbiol. 207, 83–96. doi: 10.1016/j.vetmic.2017.06.006
Stentiford G. D., Bonami J. R., Alday-Sanz V. (2009). A critical review of susceptibility of crustaceans to Taura syndrome, Yellowhead disease and White Spot Disease and implications of inclusion of these diseases in European legislation. Aquaculture 291, 1–17. doi: 10.1016/j.aquaculture.2009.02.042
Subasinghe R. P. (2005). Epidemiological approach to aquatic animal health management: opportunities and challenges for developing countries to increase aquatic production through aquaculture. Prev. Vet. Med. 67, 117–124. doi: 10.1016/j.prevetmed.2004.11.004
Sullivan T. J., Dhar A. K., Cruz-Flores R., Bodnar A. G. (2019). Rapid, CRISPR-based, field-deployable detection of white spot syndrome virus in shrimp. Sci. Rep. 9, 19702. doi: 10.1038/s41598-019-56170-y
Summerfelt S. T., Sharrer M. J., Tsukuda S. M., Gearheart M. (2009). Process requirements for achieving full-flow disinfection of recirculating water using ozonation and UV irradiation. Aquac. Eng. 40, 17–27. doi: 10.1016/j.aquaeng.2008.10.002
Tang K. F., Bondad-Reantaso M. G., Arthur J. R., MacKinnon B., Hao B., Alday-Sanz V., et al. (2020). Shrimp acute hepatopancreatic necrosis disease strategy manual. FAO Fish. Aquac. Circ.
Tang K. F., Lightner D. V. (2002). Low sequence variation among isolates of infectious hypodermal and hematopoietic necrosis virus (IHHNV) originating from Hawaii and the Americas. Dis. Aquat. Org. 49, 93–97. doi: 10.3354/dao049093
Tang K. F., Lightner D. V. (2005). Phylogenetic analysis of Taura syndrome virus isolates collected between 1993 and 2004 and virulence comparison between two isolates representing different genetic variants. Virus Res. 112, 69–76. doi: 10.1016/j.virusres.2005.03.023
Tang K. F., Lightner D. V. (2006). Infectious hypodermal and hematopoietic necrosis virus (IHHNV)-related sequences in the genome of the black tiger prawn Penaeus monodon from Africa and Australia. Virus Res. 118, 185–191. doi: 10.1016/j.virusres.2006.01.003
Tang K. F., Pantoja C. R., Poulos B. T., Redman R. M., Lightner D. V. (2005). In situ hybridization demonstrates that Litopenaeus vannamei, L. stylirostris and Penaeus monodon are susceptible to experimental infection with infectious myonecrosis virus (IMNV). Dis. Aquat. Org. 63, 261–265. doi: 10.3354/dao063261
Tang K. F., Poulos B. T., Wang J., Redman R. M., Shih H. H., Lightner D. V. (2003). Geographic variations among infectious hypodermal and hematopoietic necrosis virus (IHHNV) isolates and characteristics of their infection. Dis. Aquat. Org. 53, 91–99. doi: 10.3354/dao053091
Thitamadee S., Prachumwat A., Srisala J., Jaroenlak P., Salachan P. V., Sritunyalucksana K., et al. (2016). Review of current disease threats for cultivated penaeid shrimp in Asia. Aquaculture 452, 69–87. doi: 10.1016/j.aquaculture.2015.10.028
Thursby E., Juge N. (2017). Introduction to the human gut microbiota. Biochem. J. 474, 1823–1836. doi: 10.1042/BCJ20160510
Tran L., Nunan L., Redman R. M., Mohney L. L., Pantoja C. R., Fitzsimmons K., et al. (2013). Determination of the infectious nature of the agent of acute hepatopancreatic necrosis syndrome affecting penaeid shrimp. Dis. Aquat. Org. 105, 45–55. doi: 10.3354/dao02621
Turkmen G., Toksen E. (2010). Biosecurity and major diseases in shrimp culture. Sarajevo, Bosnia and Herzegovina: International Burch University.
Tzuc J. T., Escalante D. R., Rojas Herrera R., Gaxiola Cortés G., Ortiz M. L. A. (2014). Microbiota from Litopenaeus vannamei: digestive tract microbial community of Pacific white shrimp (Litopenaeus vannamei). SpringerPlus 3, 280. doi: 10.1186/2193-1801-3-280
Vanpatten K. A., Nunan L. M., Lightner D. V. (2004). Seabirds as potential vectors of penaeid shrimp viruses and the development of a surrogate laboratory model utilizing domestic chickens. Aquaculture 241, 31–46. doi: 10.1016/j.aquaculture.2004.08.012
Vargas-Albores F., Porchas-Cornejo M. A., Martínez-Porchas M., Villalpando-Canchola E., Gollas-Galván T., Martínez-Córdova L. R. (2017). Bacterial biota of shrimp intestine is significantly modified by the use of a probiotic mixture: a high throughput sequencing approach. Helgol. Mar. Res. 71, 1–10. doi: 10.1186/s10152-017-0485-z
Vaseeharan B., Prem Anand T., Murugan T., Chen J. C. (2006). Shrimp vaccination trials with the VP292 protein of white spot syndrome virus. Lett. Appl. Microbiol. 43, 137–142. doi: 10.1111/j.1472-765X.2006.01941.x
Vaseeharan B. A. R. P., Ramasamy P. (2003). Control of pathogenic Vibrio spp. by Bacillus subtilis BT23, a possible probiotic treatment for black tiger shrimp Penaeus monodon. Lett. Appl. Microbiol. 36, 83–87. doi: 10.1046/j.1472-765X.2003.01255.x
Venegas C. A., Nonaka L., Mushiake K., Nishizawa T., Muroga K. (2000). Quasi-immune response of Penaeus japonicus to penaeid rod-shaped DNA virus (PRDV). Dis. Aquat. Org. 42, 83–89. doi: 10.3354/dao042083
Vijayan K. K., Alavandi S. V., Rajendran K. V., Alagarswami K. (1995). Prevalence and Histopathology of Monodon Baculovirus (MBV) infection in Penaeus monodon and P. indicus in shrimp farms in the south–east coast of India. Asian Fish. Sci. 8, 267–272. doi: 10.33997/j.afs.1995.8.3-4.010
Vincent A. G., Breland V. M., Lotz J. M. (2004). Experimental infection of Pacific white shrimp Litopenaeus vannamei with necrotizing hepto-pancreatitis (NHP) bacterium by per os exposure. Dis. Aquat. Org. 61, 227–233. doi: 10.3354/dao061227
Walker P. J., Mohan C. V. (2009). Viral disease emergence in shrimp aquaculture: origins, impact and the effectiveness of health management strategies. Rev. Aquac. 1, 125–154. doi: 10.1111/j.1753-5131.2009.01007.x
Wan Z. Y., Xia J. H., Lin G., Wang L., Lin V. C., Yue G. H. (2016). Genome-wide methylation analysis identified sexually dimorphic methylated regions in hybrid tilapia. Sci. Rep. 6, 35903. doi: 10.1038/srep35903
Wang Y. C., Chang P. S. (2000). Yellow head virus infection in the giant tiger prawn Penaeus monodon cultured in Taiwan. Fish Pathol. 35, 1–10. doi: 10.3147/jsfp.35.1
Wang Y. B., Li J. R., Lin J. (2008). Probiotics in aquaculture: challenges and outlook. Aquaculture 281, 1–4. doi: 10.1016/j.aquaculture.2008.06.002
Wertheim J. O., Tang K. F., Navarro S. A., Lightner D. V. (2009). A quick fuse and the emergence of Taura syndrome virus. Virology 390, 324–329. doi: 10.1016/j.virol.2009.05.010
White B. L., Schofield P. J., Poulos B. T., Lightner D. V. (2002). A laboratory challenge method for estimating Taura syndrome virus resistance in selected lines of Pacific white shrimp Litopenaeus vannamei. J. World Aquac. Soc 33, 341–348. doi: 10.1111/j.1749-7345.2002.tb00510.x
Witteveldt J., Vlak J. M., Van Hulten M. C. (2006). Increased tolerance of Litopenaeus vannamei to white spot syndrome virus (WSSV) infection after oral application of the viral envelope protein VP28. Dis. Aquat. Org. 70, 167–170. doi: 10.3354/dao070167
Wu J. L., Nishioka T., Mori K., Nishizawa T., Muroga K. (2002). A time-course study on the resistance of Penaeus japonicus induced by artificial infection with white spot syndrome virus. Fish Shellfish Immunol. 13, 391–403. doi: 10.1006/fsim.2002.0414
Wu W., Zhang X. (2007). Characterization of a Rab GTPase up-regulated in the shrimp Peneaus japonicus by virus infection. Fish Shellfish Immunol. 23, 438–445. doi: 10.1016/j.fsi.2007.01.001
Wu X., Zhang H., Chen J., Shang S., Wei Q., Yan J., et al. (2016). Comparison of the fecal microbiota of dholes high-throughput Illumina sequencing of the V3–V4 region of the 16S rRNA gene. Appl. Microbiol. Biotechnol. 100, 3577–3586. doi: 10.1007/s00253-015-7257-y
Wu W., Zong R., Xu J., Zhang X. (2008). Antiviral phagocytosis is regulated by a novel Rab-dependent complex in shrimp Penaeus japonicus. J. Proteome Res. 7, 424–431. doi: 10.1021/pr700639t
Xiong J., Chen H., Hu C., Ye X., Kong D., Zhang D. (2015a). Evidence of bacterioplankton community adaptation in response to long-term mariculture disturbance. Sci. Rep. 5, 15274. doi: 10.1038/srep15274
Xiong J., Dai W., Li C. (2016). Advances, challenges, and directions in shrimp disease control: the guidelines from an ecological perspective. Appl. Microbiol. Biotechnol. 100, 6947–6954. doi: 10.1007/s00253-016-7679-1
Xiong J., Dai W., Zhu J., Liu K., Dong C., Qiu Q. (2017). The underlying ecological processes of gut microbiota among cohabitating retarded, overgrown and normal shrimp. Microb. Ecol. 73, 988–999. doi: 10.1007/s00248-016-0910-x
Xiong J., Wang K., Wu J., Qiuqian L., Yang K., Qian Y., et al. (2015b). Changes in intestinal bacterial communities are closely associated with shrimp disease severity. Appl. Microbiol. Biotechnol. 99, 6911–6919. doi: 10.1007/s00253-015-6632-z
Xu J., Han F., Zhang X. (2007). Silencing shrimp white spot syndrome virus (WSSV) genes by siRNA. Antiviral Res. 73, 126–131. doi: 10.1016/j.antiviral.2006.08.007
Yan Q., Li J., Yu Y., Wang J., He Z., Van Nostrand J. D., et al. (2016). Environmental filtering decreases with fish development for the assembly of gut microbiota. Environ. Microbiol. 18, 4739–4754. doi: 10.1111/1462-2920.13365
Yáñez J. M., Newman S., Houston R. D. (2015). Genomics in aquaculture to better understand species biology and accelerate genetic progress. Front. Genet. 6. doi: 10.3389/fgene.2015.00
Yodmuang S., Tirasophon W., Roshorm Y., Chinnirunvong W., Panyim S. (2006). YHV-protease dsRNA inhibits YHV replication in Penaeus monodon and prevents mortality. Biochem. Biophys. Res. Commun. 341, 351–356. doi: 10.1016/j.bbrc.2005.12.186
Yu S., Li X., Lu W., Li H., Fu Y. V., Liu F. (2021). Analysis of Raman spectra by using deep learning methods in the identification of marine pathogens. Anal. Chem. 93, 11089–11098. doi: 10.1021/acs.analchem.1c00431
Yu W., Wu J. H., Zhang J., Yang W., Chen J., Xiong J. (2018). A meta-analysis reveals universal gut bacterial signatures for diagnosing the incidence of shrimp disease. FEMS Microbiol. Ecol. 94, fiy147. doi: 10.1093/femsec/fiy147
Yu Y., Zhang X., Yuan J., Li F., Chen X., Zhao Y., et al. (2015). Genome survey and high-density genetic map construction provide genomic and genetic resources for the Pacific White Shrimp Litopenaeus vannamei. Sci. Rep. 5, 15612. doi: 10.1038/srep15612
Yuan J., Zhang X., Liu C., Yu Y., Wei J., Li F., et al. (2018). Genomic resources and comparative analyses of two economical penaeid shrimp species, Marsupenaeus japonicus and Penaeus monodon. Mar. Genom. 39, 22–25. doi: 10.1016/j.margen.2017.12.006
Yue G. H., Wang L. (2017). Current status of genome sequencing and its applications in aquaculture. Aquaculture 468, 337–347. doi: 10.1016/j.aquaculture.2016.10.036
Zeng D., Chen X., Peng J., Yang C., Peng M., Zhu W., et al. (2018). Single-molecule long-read sequencing facilitates shrimp transcriptome research. Sci. Rep. 8, 16920. doi: 10.1038/s41598-018-35066-3
Zhang J., Cao Z., Li Z., Wang L., Li H., Wu F., et al. (2015). Effect of bacteriophages on Vibrio alginolyticus infection in the sea cucumber, Apostichopus japonicus (Selenka). J. World Aquac. Soc 46, 149–158. doi: 10.1111/jwas.12177
Zhang J. S., Dong S. L., Tian X. L., Dong Y. W., Liu X. Y., Yan D. C. (2006). Studies on the rotifer (Brachionus urceus Linnaeus 1758) as a vector in white spot syndrome virus (WSSV) transmission. Aquaculture 261, 1181–1185. doi: 10.1016/j.aquaculture.2006.09.002
Zhang X., Yuan J., Sun Y., Li S., Gao Y. I., Yu Y., et al. (2019). Penaeid shrimp genome provides insights into benthic adaptation and frequent molting. Nat. Commun. 10, 356. doi: 10.1038/s41467-018-08197-4
Zheng Y., Yu M., Liu Y., Su Y., Xu T., Yu M., et al. (2016). Comparison of cultivable bacterial communities associated with Pacific white shrimp (Litopenaeus vannamei) larvae at different health statuses and growth stages. Aquaculture 451, 163–169. doi: 10.1016/j.aquaculture.2015.09.020
Zhu F., Twan W. H., Tseng L. C., Peng S. H., Hwang J. S. (2019). First detection of white spot syndrome virus (WSSV) in the mud shrimp Austinogebia edulis in Taiwan. Sci. Rep. 9, 18572. doi: 10.1038/s41598-019-54837-0
Zhu F., Zhang X. (2011). The antiviral vp28-siRNA expressed in bacteria protects shrimp against white spot syndrome virus (WSSV). Aquaculture 319, 311–314. doi: 10.1016/j.aquaculture.2011.04.003
Keywords: shrimp, pathogens, diseases, aquaculture, biosecurity
Citation: Bhassu S, Shama M, Tiruvayipati S, Soo TCC, Ahmed N and Yusoff K (2024) Microbes and pathogens associated with shrimps - implications and review of possible control strategies. Front. Mar. Sci. 11:1397708. doi: 10.3389/fmars.2024.1397708
Received: 26 March 2024; Accepted: 23 July 2024;
Published: 07 October 2024.
Edited by:
Kwang-Sik Albert Choi, Jeju National University, Republic of KoreaReviewed by:
Ming Zhang, Shantou University, ChinaThavasimuthu – Citarasu, Manonmaniam Sundaranar University, India
Suvra Roy, Central Inland Fisheries Research Institute (ICAR), India
Copyright © 2024 Bhassu, Shama, Tiruvayipati, Soo, Ahmed and Yusoff. This is an open-access article distributed under the terms of the Creative Commons Attribution License (CC BY). The use, distribution or reproduction in other forums is permitted, provided the original author(s) and the copyright owner(s) are credited and that the original publication in this journal is cited, in accordance with accepted academic practice. No use, distribution or reproduction is permitted which does not comply with these terms.
*Correspondence: Subha Bhassu, c3ViaGFiaGFzc3VAdW0uZWR1Lm15