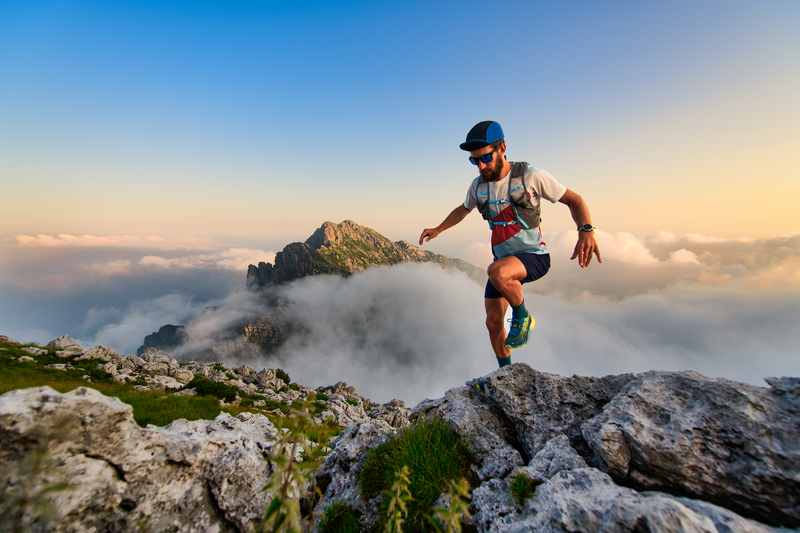
95% of researchers rate our articles as excellent or good
Learn more about the work of our research integrity team to safeguard the quality of each article we publish.
Find out more
ORIGINAL RESEARCH article
Front. Mar. Sci. , 23 May 2024
Sec. Marine Ecosystem Ecology
Volume 11 - 2024 | https://doi.org/10.3389/fmars.2024.1390074
This article is part of the Research Topic Degradation, Conservation and Ecological Restoration of Seagrass Beds under Intensifying Global Changes View all 8 articles
The ascorbate-glutathione (AsA-GSH) cycle plays a critical role in scavenging hydrogen peroxide in plants and contributes significantly to plant stress tolerance. This study examines the cycle’s response in Zostera marina seedlings to warming, specifically under conditions of abnormal sea temperature increase. Three temperature gradients were established: 18°C (control group), 23°C (high-temperature group), and 28°C (abnormally high-temperature group). Results after 7 days of exposure to mild high temperature (23°C) showed decreased MDA content in the HT group, increased AsA/DHA ratio, and enhanced activity of enzymes related to the AsA-GSH cycle. However, exposure to extreme high temperatures resulted in increased oxidative damage and redox imbalance in the AHT group. Initially, enzymes associated with the AsA-GSH cycle, such as APX, MDHAR, GPX, and γ-ECS, increased but significantly decreased later under stress. In contrast, DHAR and GaILDH levels significantly rose on the seventh day. Transcriptome analysis revealed upregulation of APX, MDHAR, DHAR, GR, and γ-ECS genes in the HT group, with a decline in other enzyme gene expressions by the seventh day, except for APX. Under extreme high temperatures, APX expression was downregulated early in the stress period, while DHAR was upregulated, indicating Z. marina seedlings can mitigate oxidative damage under short-term high temperatures by activating the AsA-GSH cycle. Conversely, extreme high temperatures may inhibit this cycle, disrupt redox balance, and adversely affect Z. marina seedling establishment, potentially leading to their demise.
Seagrass beds, recognized as highly productive global ecosystems (Hemming and Duarte, 2000; Unsworth et al., 2018), serve crucial ecological functions in coastal zones (Duarte, 2002) by providing habitats (McDevitt-Irwin et al., 2016) and food sources (Edgar, 1994) for numerous marine organisms and playing significant roles in water purification, sediment stabilization (Orth et al., 2020), and carbon burial (Mtwana Nordlund et al., 2016; Duarte and Dorte, 2017). However, climate change and human disturbances have posed severe threats and accelerated the degradation of these habitats (Waycott et al., 2009). Global warming stands out as a critical factor (Hoegh-Guldberg and Bruno, 2010; Arias-Ortiz et al., 2018; Strydom et al., 2020; Trisos et al., 2020). Ocean temperatures between 2001–2020 were 0.88°C warmer than in 1850–1990 (Jiang et al., 2022). The frequency and duration of extreme heat events, such as summer heatwaves, have increased and are expected to continue rising through the 21st century (Karl and Trenberth, 2003; Christidis et al., 2015; Jiang et al., 2022). These extreme temperatures pose a significant threat, leading to substantial mortality in seagrass beds (Arias-Ortiz et al., 2018; Strydom et al., 2020). Based on global warming projections, the functional extinction of seagrasses could be imminent, emphasizing the urgency for conservation and mitigation efforts (Marbà and Duarte, 2010; Jordà et al., 2012; Chefaoui et al., 2018).
Recent research has highlighted the impact of global warming on the way seagrasses respond to marine events, examining differences at various levels such as growth, physiology, and molecular aspects (Nguyen et al., 2021; Wang et al., 2023; Yan et al., 2023a, b). For instance, severe occurrences like ocean warming or marine heatwaves can restrict the growth of Zostera marina, findings that align with transcriptomic and metabolomic studies (Yan et al., 2023a, b). However, the impact of temperature changes, including the frequency and duration of abnormal events, varies on seagrass (Marbà and Duarte, 2010; Kaldy et al., 2017; Kendrick et al., 2019; Magel et al., 2022). Notably, Z. marina exhibits different reactions to temperature fluctuations at various stages of its life cycle (Wang et al., 2023; Yan et al., 2023a), with seedlings being particularly temperature-sensitive (Wang et al., 2023).
Marine stress events disrupt the balance of reactive oxygen species (ROS) in seagrasses, challenging their survival. The antioxidant system plays a crucial role in enabling seagrasses to cope with environmental stress, regulating ROS signal transmission and managing adversity (Lee et al., 2016; Olsen et al., 2016). Elevated temperatures have been found to significantly enhance the gene expression of Ascorbate peroxidase (APX), superoxide dismutase (SOD), and catalase (CAT) in Thalassia hemprichii, with APX showing the highest increase. This suggests the ascorbate-glutathione cycle is central to the antioxidant system’s function (Rakhmad et al., 2019). Additionally, an increase in Mn-SOD gene expression was observed, promoting SOD production (Rakhmad et al., 2019), which breaks down the superoxide anion (O2-) produced in excess under high temperatures, thereby maintaining ROS homeostasis (Rakhmad et al., 2019). This mechanism is also evident in Posidonia oceanica and Cymodocea nodosa (Marín-Guirao et al., 2016). Other antioxidant enzymes, including glutathione S-transferase, are upregulated in response to high temperatures, as observed in Z. noltii and Z. marina (Massa et al., 2011; Yan et al., 2019). As mentioned above, the response of some typical antioxidant enzymes to environmental stress has also been studied.
The ascorbate-glutathione cycle (AsA-GSH) is essential for enhancing plant tolerance to prolonged stress and for scavenging ROS in plants (Alscher et al., 1997), clearing H2O2 and facilitating the regeneration of AsA and GSH (Kang et al., 2013; Bartoli et al., 2017). Paeonia ostii T. has been shown to increase the levels of AsA, GSH, and related enzyme activities under treatment with multi-walled carbon nanotubes (MWCNTs), mitigating the effects of high temperatures (Zhao et al., 2021). Similarly, the presence of endogenous nitric oxide assists salicylic acid in enhancing Capsicum annuum L.’s tolerance to salt by upregulating activities of enzymes related to the AsA-GSH cycle (Kaya et al., 2019). Externally applied AsA and GSH can regulate the AsA-GSH cycle and reduce cold damage in stored postharvest bell pepper fruits (Yao et al., 2021). While much of the research on the AsA-GSH cycle has focused on terrestrial plants, its role in seagrasses, especially in response to temperature changes, remains underexplored. Understanding the AsA-GSH cycle in seagrasses is vital for comprehending their resilience against oxidative damage caused by environmental stresses (Zhao et al., 2021; Kaya et al., 2019; Karam et al., 2017; Yao et al., 2021).
Given the importance of understanding the vulnerability of seagrass seedlings, this study focuses on the response of the typical temperate seagrass, Zostera marina, to increased temperatures through the lens of the AsA-GSH cycle. Utilizing a mesoscale experimental approach as outlined by Yan et al. (Yan et al., 2023a, b) and Wang et al. (2023), the study integrates physiology and transcriptomics to elucidate the AsA-GSH cycle’s behavior under short-term, extreme high temperature. This study aims to provide theoretical support for the protection and restoration of seagrass beds.
Zostera marina reproduces through both sexual and asexual means. The population survey of Tangshan Z. marina shows that the seed banks typically germinate in the spring, and the seedlings are established from April to May (Xu, 2021). In our study, Z. marina seedlings were collected on May 2023 from Caofeidian, Tangshan, Hebei (39°5’40.45” N, 118°40’57.15” E). Upon collection, 100 plants exhibiting healthy growth and of approximately equal lengths were promptly transported to the laboratory within 30 minutes. These plants were meticulously cleaned with a fine-bristle brush to remove debris and organisms attached to the leaves, stolons, and root system. Subsequently, four plants were randomly grouped together and placed in an acrylic isolation box measuring 15 cm × 15 cm × 15 cm, with stolons secured using ceramic grains.
Five such groups were accommodated in each mesoscale incubator. The incubators, each 1.5 m in length, 1 m in width, and 1 m in height, featured a recirculation system that introduced natural seawater to simulate the Z. marina’s natural habitat accurately. Real-time monitoring of temperature and pH was conducted, maintaining a temperature of ± 1°C with a heating rod and a pH of ± 0.05 using a carbon dioxide enrichment device, as detailed by Yan et al (Yan et al., 2023a, b). Before starting the main experiments, all plants underwent a 7-day acclimatization phase under specific conditions: 18°C, a light intensity of 240 μmol·m-2·s-1, a 12 D: 12 L light cycle, a pH of 8.2 ± 0.05, and a salinity level of 32, mirroring the natural seawater conditions in Tangshan.
To investigate how Z. marina seedlings respond to ocean warming and particularly to abnormal Sea Surface Temperature (SST) events, this study established three temperature conditions: 18°C (control group, representing the midday mean SST of the sampling month), 23°C (the peak temperature during the sampling month), and 28°C (an abnormally high temperature). A growth box containing a single Z. marina specimen exhibiting consistent, healthy development was placed in an incubator. For each temperature condition, three replicates were set up, with each replicate comprising nine plants, totaling 81 Z. marina seedlings across all groups. The seedlings were exposed to their respective temperatures for 14 days, with continuous monitoring of temperature and pH levels. To prevent rhizome hypoxia, the ceramic support was rotated daily. Leaf samples were collected at specified intervals throughout the experiment (0 d, 1/4 d, 1/2 d, 3/4 d, 1 d, 1.5 d, 2 d, 3 d, 4 d, and 7 d) to minimize variations due to sampling time and individual plant differences. Care was taken to exclude damaged leaves from sampling to avoid the impact of physical damage. Each sampled group was quickly frozen in liquid nitrogen and preserved at -80°C.
For the analysis, Z.marina seedling leaf samples were prepared using reagent kits appropriate for plant tissue, focusing on indicators of oxidative damage and the levels of enzymes and substances linked to ASA and GSH metabolism. The samples were processed with a fast preparation instrument (MP/FastPrep-24, USA) using Lysing Matrix D, containing 1.4 mm ceramic beads, with a parameter of 6 m/s and a cracking time of 40 s) and then centrifuged in a benchtop freezer centrifuge (SIGMA-3K15, Germany). The experiments were conducted with the following parameters: 4 °C, 10,000 rpm, and 10 minutes. These parameters were adjusted as per the instructions provided by the reagent kits. The assays were performed using a full-wavelength enzyme labeler (MD VERSAmax, USA), with the instrument’s wavelength being adjusted according to the reagent kits’ guidelines. Additionally, GPX family genes displayed various trends throughout the stress period, associated with the roles of different GPX family members (Islam et al., 2015). To assess the extent of oxidative damage following high temperature stress, various assay kits were employed: the Plant ROS enzyme-linked immunoassay kit (MLBIO, Shanghai, China) for reactive oxygen species (ROS), the hydrogen peroxide (H2O2) content assay kit (Beyotime, Shanghai, China), and the lipid oxidation assay kit (Beyotime, Shanghai, China) for measuring malondialdehyde (MDA) levels. Additionally, the total antioxidant capacity (TAC) was determined using a TAC assay kit (ABTS method, S0116, Beyotime, Shanghai, China) (Buege and Aust, 1978; Jung et al., 2019).
Redox status, characterized by ASA/DHA and GSH/GSSG ratios, was assessed using assay kits for ASA/total ASA (Beyotime, Shanghai, China) and for GSH and GSSG contents (Solarbio, Beijing, China). The key enzymes in the ASA-GSH cycle, including ascorbate peroxidase (APX), dehydroascorbate reductase (DHAR), monodehydroascorbate reductase (MDHAR), and glutathione reductase (GR), were measured using activity assay kits for APX, DHAR, and MDHAR (Solarbio, Beijing, China), drawing on methodologies from Groden and Beck (1979), Chew et al. (2003), and Foyer and Noctor (2011). Additionally, Glutathione Peroxidase (GPX), which uses GSH produced by GR to eliminate H2O2, was measured with activity assay kits for GPX (Solarbio, Beijing, China). This measurement indirectly reflects the GR content of key enzymes in the AsA-GSH cycle. The content of other critical enzymes in the ASA-GSH cycle, L-1,4-galactonolactone dehydrogenase (GalLDH) and γ-glutamylcysteine synthetase (γ-ECS), was determined using assay kits for GalLDH (Solarbio, Beijing, China) and γ-ECS (D&B, Shanghai, China), based on findings by Wheeler et al. (1998), Linster and Clarke (2008), Oppenheimer et al. (1979), and Noctor and Foyer (1998).
Seedlings exposed to temperatures of 18°C, 23°C, and 28°C for 1 day (early stage of stress), 4 days (mid stage of stress), and 7 days (late stage of stress) were selected for transcriptomic analysis. From the total RNA extracted from all samples, rRNA was removed, and mRNA was enriched and amplified by PCR after reverse transcription, end repair, and splicing. The data underwent quality checks using agarose gel electrophoresis (for DNA contamination), a NanoPhotometer spectrophotometer (for RNA purity), a Qubit 2.0 Fluorometer (for RNA concentration), and an Agilent 2100 bioanalyzer (for RNA integrity), ensuring the quality of library construction. Low-quality data and base quality were analyzed using fastq (Chen et al., 2018). Further rRNA removal was performed by ribosome comparison (Langmead and Salzberg, 2012), and comparison with the reference genome (Zosma_marina.v.2.1) was carried out (Olsen et al., 2016). Genetic analysis included calculating and counting sample expression using RSEM (Li and Dewey, 2011; Pertea et al., 2015) to identify related genes and their expression changes. Outlier samples were excluded through sample relationship analysis. Intergroup differences in read counts were analyzed using DESeq software (Love et al., 2014), identifying significantly differentially expressed genes (DEGs) with an FDR < 0.05. DEGs underwent cluster analysis, Gene Ontology (GO) enrichment analysis, and Kyoto Encyclopedia of Genes and Genomes (KEGG) enrichment analysis to elucidate the biochemical metabolism and signaling pathways related to the ASA-GSH cycle.
Microsoft Excel 2019 was utilized for statistical analysis of the collected data, focusing on physiological indices as detailed in Section 2.3. Data were presented as mean ± standard deviation (SD). For graphical representation, Origin 2021 facilitated the creation of double Y-axis histograms. IBM SPSS Statistics version 27.0 enabled the execution of normality assessments, variance homogeneity tests, and one-way analysis of variance (ANOVA) on the experimental data. Further analyses included multiple comparisons using LSD, Tamhane, and Dunnett methods, with Duncan’s test identifying homogeneous subsets. Significant differences were noted with p-values < 0.05. In transcriptome analysis, Pearson correlation coefficient was applied to identify differentially expressed genes (DEGs) based on p-values and FDR < 0.05. Gene Ontology (GO) and Kyoto Encyclopedia of Genes and Genomes (KEGG) enrichment analyses were conducted on these DEGs.
Relative to the Control group, reactive oxygen species (ROS), hydrogen peroxide (H2O2), and malondialdehyde (MDA) levels were markedly elevated in both HT and AHT groups, as shown in Figure 1. Specifically, ROS levels in the HT group surged significantly at 0.25 days, 1.5 days, 2 days, and 3 days, registering increases of 40.23%, 13.73%, 27.56%, and 19.22% over the Control group, respectively (p < 0.05), with a 7.35% rise at 7 days (Figure 1A). H2O2 levels showed significant upticks at 0.5 days, 1 day, and 1.5 days, with increases of 45.68%, 65.49%, and 45.48% above the Control, respectively (p < 0.05), and a 5.17% increase at 7 days (Figure 1B). MDA levels also significantly rose at 0.25 days and 1 day, up by 35.22% and 27.56% over the Control, respectively (p < 0.05), but were 13.15% lower at 7 days (Figure 1C). While no significant difference was observed in total antioxidant capacity (TAC), a trend of initial decrease followed by an increase was noted at 0.25 days, 0.5 days, and 0.75 days (Figure 1D). Compared to the Control group, TAC dropped by 5.67%, 9.53%, and 4.06%, but increased by 6.20% and 4.37% at 4 days and 7 days, respectively (Figure 1D).
Figure 1 Effect of high temperature on reactive oxygen species (ROS), hydrogen peroxide (H2O2), malonaldehyde (MDA), and total antioxidant capacity (TAC) for Zostera marina seedlings. The data notation is as follows: (A) denotes ROS content; (B) denotes H2O2 content; (C) denotes MDA content; and (D) denotes TAC in the seedling leaves. The experiment involved three groups: the Control group, maintained at 18°C; the High Temperature (HT) group, maintained at 23°C; and the Advanced High Temperature (AHT) group, maintained at 28°C. Lowercase letters (a, b, c, and d) indicate statistically significant differences, with identical letters signifying no significant difference among values. Dashed lines in the graphs represent the baseline levels of these indicators at the onset of stress (t=0).
In the AHT group, ROS levels significantly exceeded those in the Control group at 0.5 days, 0.75 days, 2 days, and 7 days, by 8.21%, 27.75%, 27.56%, 19.22%, and 18.57%, respectively (p < 0.05; Figure 1A). H2O2 levels were considerably higher at 0.5 days, 0.75 days, 1 day, 1.5 days, and 7 days, exceeding Control levels by 62.82%, 84.48%, 43.41%, 50.59%, and 65.59%, respectively (p < 0.05; Figure 1B). MDA levels, higher than those in the Control on day 0, 1 day, and 7 days, were notably increased on 0.25 days, 0.5 days, 0.75 days, 1 day, and 7 days by 5.26%, 19.54%, 43.90%, 12.84%, and 19.56%, with significant hikes observed at 0.75 days and 7 days (p < 0.05; Figure 1C). TAC showed a significant reduction of 15.41% at 0.25 days (p < 0.05), and decreases of 4.22% and 4.35% at 1 day and 3 days compared to the Control, with a 6.78% increase at 7 days (Figure 1D).
Compared with the Control group, the HT group exhibited three increases (0.25 d, 0.5 d, and 3 d-7 d) and two decreases (0.5 d and 2 d) in AsA content by the 7th day. The AsA content was significantly higher by 217.24% at 0.25 d (p < 0.05), significantly lower by 74.60% at 2 d, and 59.26% higher at 7 d (Figure 2A). GSH content showed significant differences only at 1.5 d and 3 d, by 11.59% and 18.09% respectively, with no significant difference at 7 d (Figure 2B). DHA content significantly varied (p < 0.05) at 2 d and 3 d, showing decreases of 65.01% and 47.24% respectively; the difference was not significant at 7 d (Figure 2C). GSSG content differences were all non-significant, being slightly higher than the Control group by 1.32% and 2.24% at 1.5 d and 3 d (Figure 2D). The AsA/DHA ratio was higher than the Control group, except at 0.5 d and 2 d, with significant increases at 0.25 d, 1 d, 3 d, and 7 d by 145.26%, 56.80%, 137.29%, and 65.03%, respectively (Figure 2E). The GSH/GSSG ratio was significantly higher than the Control group at 1.5 d and 3 d, by 10.13% and 15.51%, respectively (Figure 2F).
Figure 2 Effect of high temperature on redox status (AsA/DHA, GSH/GSSG) for Zostera marina seedlings. (A): Content of ascorbate (AsA); (B): Content of glutathione (GSH); (C): Content of DHA; (D) Content of GSSG; (E): Ratio of AsA/DHA; and (F): Ratio of GSH/GSSG. Similar to the first note, the Control group was kept at 18°C; the HT group at 23°C; and the AHT group at 28°C. The significance of differences between groups is indicated by lowercase letters (a, b, c, and d), with identical letters denoting no significant difference. The initial levels of these biomarkers at the beginning of the stress period (t=0) are depicted by dashed lines.
In group AHT, AsA content was higher than in the Control group from 0 d-4 d, including a significant increase of 124.66% at 0.5 d (p < 0.05) and a decrease of 25.70% at 7 d (Figure 2A). GSH content significantly decreased throughout the entire experiment (p < 0.05), with a 33.36% decrease observed at 7 d (Figure 2B). DHA content experienced two decreases (0 d-1.5 d and 4 d-7 d) and one increase (1.5 d-4 d), with significant increases of 43.90%, 49.98%, and 84.23% (p < 0.05) at 0.25 d, 3 d, and 4 d, respectively, a significant decrease of 37.47% at 1.5 d (p < 0.05), and no significant difference at 7 d (Figure 2C). GSSG content increased by 5.16% at 7 d (Figure 2D). The AsA/DHA ratio was significantly higher at 0.5 d and 1.5 d (p < 0.05), by 97.90% and 113.50% respectively, and decreased by 32.54% and 24.93% at 4 d and 7 d (Figure 2E). The GSH/GSSG ratio significantly decreased (p < 0.05), with a 36.63% decrease at 7 d (Figure 2F).Key enzymes for ASA-GSH cycle
Compared to the Control group, the APX enzyme activity in the HT group significantly increased at 0.5 days, 1 day, and 1.5 days by 202.63%, 81.08%, and 77.78%, respectively. It significantly decreased by 65.22% at 0.75 days and showed no significant difference at 7 days (Figure 3A). MDHAR enzyme activity tripled, significantly increasing at 0.25 days, 1 day, and 7 days by 54.38%, 84.43%, and 122.48%, respectively (p < 0.05), but significantly decreased by 71.80% at 4 days (p < 0.05; Figure 3B). DHAR enzyme activity initially increased, then decreased, peaking between 1.5 days and 2 days (Figure 3C). GPX enzyme activity significantly increased at 1.5 days, 3 days, and 7 days, surpassing the Control group by 88.70%, 108.71%, and 157.93%, respectively (p < 0.05; Figure 3D). GalLDH enzyme activity significantly increased at 1.5 days, 2 days, and 7 days, respectively exceeding the Control group by 40.58%, 48.20%, and 36.92% (p < 0.05; Figure 3E). γ-ECS enzyme activity significantly increased by 30.13% at 0.75 days (p < 0.05), and was lower at all other times except for 0.25 days and 0.75 days (Figure 3F).
Figure 3 The effect of high temperature on AsA-GSH cycle related enzymes (APX, MDHAR, DHAR and GPX) and key enzymes for AsA and GSH synthesis (GalLDH and γ-ECS) in Zostera marina. (A): Content of ascorbate peroxidase (APX); (B): Content of monodehydroascorbate reductase (MDHAR); (C): Content of dehydroascorbate reductase (DHAR); (D) Content of glutathione peroxidase (GPX); (E): Content of L-1,4-galactonolactone dehydrogenase (GalLDH); and (F): Content of γ-glutamylcysteine synthetase (γ-ECS). The study grouped seedlings into three temperature conditions: Control at 18°C, HT at 23°C, and AHT at 28°C. Significant differences in enzyme content are denoted by lowercase letters (a, b, c, and d), with identical letters indicating no significant difference. Dashed lines illustrate the initial content levels of these indicators at the start of stress exposure (t=0).
In the AHT group, APX enzyme activity increased by 51.02%, 71.05%, and 13.33% at 0.25 days, 0.5 days, and 1.5 days; the lowest levels were at 0.75 days and 4 days, 28.26% and 53.19% lower than the Control group, respectively (Figure 3A). MDHAR enzyme activity at 0.25 days and 1 day was 47.09% and 56.58% higher than the Control group (p < 0.05) but was lower at other times (Figure 3B). DHAR enzyme activity was significantly higher than the Control, increasing by 31.81%, 122.63%, 195.52%, and 106.04% at 0.5 days, 1 day, 2 days, and 7 days, respectively (p < 0.05; Figure 3C). GPX enzyme activity was higher than the Control group by 13.36%, 4.05%, 38.74%, and 35.78% at 0.25 days, 1.5 days, 2 days, and 4 days, respectively, and declined by 8.49% at 7 days (Figure 3D). GalLDH enzyme activity increased by 41.18%, 23.91%, and 29.84% compared to the Control at 0.25 days, 1.5 days, and 7 days (p < 0.05), and was significantly lower by 41.31% at 0.75 days (p < 0.05; Figure 3E). γ-ECS enzyme activity was higher than the Control by 10.93%, 9.69%, and 14.16% at 0.25 days, 0.5 days, and 0.75 days, respectively, then decreased (Figure 3F).
Samples from the 18°C (Control), 23°C (HT), and 28°C (AHT) groups were selected for transcriptome sequencing at 1 day, 4 days, and 7 days, totaling 27 samples across the three groups. A total of 1,177,247,620 clean reads, representing 99.60% of the raw reads, were obtained. The Q30 base distribution ranged from 93.07% to 95.42%, with an average GC content of 44.87%. Comparison with the reference genome showed an average matching rate of 94.81%, indicating the sequencing data’s high integrity and quality.
Correlation analysis reveals that as temperature and stress duration increase, the Pearson correlation coefficient tends to decrease, with the differences in correlation becoming more pronounced. Specifically, the difference in correlation between Group AHT and the Control group was larger than between the Control and HT groups. The Pearson correlation coefficients decreased as stress duration increased, with the largest correlation difference observed after 7 days.
In Group HT, the numbers of differentially expressed genes (DEGs) at 1 day, 4 days, and 7 days compared to the Control group were 41, 140, and 204, respectively. The proportion of up-regulated DEGs increased with stress duration, being 48.78%, 67.14%, and 76.96%, respectively. In Group AHT, the numbers of DEGs at 1 day, 4 days, and 7 days compared to the Control group were 3024, 1032, and 2255, respectively. The number of DEGs first increased, then decreased, and finally increased again with the duration of stress. The proportion of up-regulated DEGs decreased as stress duration increased, with percentages of 69.35%, 64.73%, and 63.73%, respectively. This indicates that the number of DEGs in Z. marina seedlings rose with increasing temperature, with Group AHT showing ten times more DEGs than Group HT after 7 days.
Key enzyme genes of the AsA-GSH cycle were analyzed to produce a heatmap (Figure 4). Compared to the Control group, the APX gene in Group HT was up-regulated during 7 days of stress, peaking on the 7th day, with its expression positively correlating with the stress duration. Genes MDHAR, DHAR, GR, GaILDH, and γ-ECS were up-regulated, showing a pattern of initial increase followed by a decrease, with the highest expression on the 4th day, although γ-ECS’s expression was lower than that of MDHAR, DHAR, and GR. In Group AHT, the APX gene was down-regulated initially before increasing again. MDHAR gene expression was up-regulated but less than in Group HT, peaking on the 4th day. DHAR, GaILDH, GR, and γ-ECS gene expressions were higher than those in the Control and AHT groups. The expressions of DHAR and GaILDH peaked on the 7th day, positively correlating with stress duration. GR and γ-ECS expressions initially increased then decreased, reaching their maximum on the 4th and 1st days, respectively. Additionally, GPX family genes displayed various trends throughout the stress period, associated with the roles of different GPX family members. GPX6 was notably up-regulated early in the stress period and down-regulated afterwards, while GPX1, GPX3, and GPX7 were up-regulated in the middle to late stages of stress, with the highest expression levels on the 4th day.
Figure 4 Heatmap of Pearson’s correlation coefficient (r) for APX, MDHAR, DHAR, GR, γ-ECS (A) and GPX (B) of Z. marina seedings. The color coding within the study—red for high and blue for low—illustrates the Pearson’s correlation coefficient (r), indicating the abundance of target gene expression. Specifically, a redder hue signifies higher abundance, whereas a bluer hue indicates lower abundance. The samples from days 1, 4, and 7 of stress exposure are marked as “_1”, “_4”, and “_7” respectively.
The phenomenon of global warming, particularly the occurrence of extreme high temperatures, presents a significant risk to the survival of seagrass beds. Such temperature stresses may compromise plant viability by elevating respiration rates in seagrasses, thereby generating excessive Reactive Oxygen Species (ROS) and causing metabolic imbalances (Lee et al., 2007; Koch et al., 2013). Alternatively, high temperatures might directly impair the seagrass’s photosynthetic system (PSII) or trigger non-photochemical quenching, leading to a sustained reduction in photosynthetic activity. This could constrain seagrass growth or even result in death (Collier and Waycott, 2014; Yan et al., 2023a, b). Our prior studies have demonstrated that seasonal temperature rises negatively affect the growth of Z. marina seedlings, with notable reductions in leaf size under thermal stress (Yan et al., 2023a, b). Building on this background, the current study investigates the role of the AsA-GSH antioxidant system in mitigating high-temperature stress, including conditions of extreme heat, in Z. marina seedlings.
The research findings indicate that, in response to high temperatures, Z. marina seedlings enhance the gene expression and activity levels of key enzymes within the AsA-GSH cycle. This adjustment increases enzyme content and the AsA/DHA ratio, enabling the seedlings to counteract high-temperature stress effectively by neutralizing excess ROS through the AsA-GSH cycle and GPX. However, under conditions of extreme high temperature, the AsA-GSH cycle’s effectiveness is compromised; the concentration of essential substances and enzymes declines, disrupting the redox balance (Figure 5). While moderate temperature elevations can activate the AsA-GSH cycle, bolstering the heat tolerance of Z. marina seedlings and minimizing oxidative harm, it proves insufficient under extreme high temperatures, leading to significant oxidative damage.
Figure 5 Changes in contents and gene expressions of key substances and enzymes of the AsA-GSH cycle in eelgrass seedlings at 1st, 4th and 7th day in HT group (A) and AHT group (B) under high temperature stress. From left to right or top to bottom represents the changes at days 1, 4, and 7; the graphs are labeled with the changes in oxidative stress, antioxidant capacity, and redox pairs of AsA/DHA and GSH/GSSG within the AsA-GSH cycle in seedlings from the HT group (A) and AHT group (B) across days 1, 4, and 7 under high-temperature stress. These graphics also depict changes in the content of key substances, enzymes, and gene expressions related to the AsA-GSH cycle, including modifications in enzyme content and gene expression (marked by rectangles for the cycle changes and ellipses for enzyme and gene changes). Key terms defined include ROS (reactive oxygen species), MDA (malondialdehyde), TAC (total antioxidant capacity), AsA (ascorbate, reduced form), DHA (dehydroascorbate, oxidized form), MDHA (monodehydroascorbate), GSH (glutathione, reduced form), GSSG (glutathione disulfide, oxidized form), along with the enzymes APX, MDHAR, DHAR, GR (glutathione reductase), GaILDH (L-galactono-1, 4-lactone dehydrogenase), and γ-ECS. NADPH and NADP+ (reduced and oxidized forms of nicotinamide adenine dinucleotide phosphate, respectively) are also included in the study’s scope.
Reactive oxygen species (ROS) are unintended by-products of aerobic metabolism in plants, potentially causing oxidative harm when they accumulate excessively (Sharma et al., 2012; Tutar et al., 2017). The antioxidant system serves as a crucial defensive mechanism against environmental stress, playing a vital role in protecting seagrass from temperature-induced stress (Sharma et al., 2012; Tutar et al., 2017). In this study, exposure to short-term high temperature resulted in elevated levels of ROS, H2O2, and MDA in Z. marina seedlings. However, seedlings subjected to mild high temperatures (HT group) managed to revert to normal levels after 7 days, with MDA levels even dropping below control levels. In contrast, seedlings exposed to extreme high temperatures (AHT group) exhibited significantly higher levels of ROS, H2O2, and MDA compared to the control (Figure 1). Additionally, the Total Antioxidant Capacity (TAC) of both HT and AHT groups initially fell, then rose, and finally declined again during the stress period, peaking on the 4th and 7th day, respectively (Figure 1D). These observations suggest that the antioxidant system is initially activated to combat oxidative damage in Z. marina seedlings under high temperature stress. While the system is effective against mild high temperatures, it becomes overwhelmed and inadequate under extreme heat, leading to reduced TAC and potentially inhibiting the AsA-GSH cycle’s ability to prevent oxidative damage.
The two crucial non-enzymatic antioxidants, ascorbic acid (AsA) and glutathione (GSH), within the AsA-GSH cycle play pivotal roles in both directly and indirectly scavenging reactive oxygen species (ROS) through the antioxidant system and participating in redox signaling. The balance between their reduced and oxidized forms is key in maintaining cellular redox balance and activating various defense mechanisms (Chew et al., 2003; Fotopoulos et al., 2010). This study observed that under high temperature, the reduced AsA levels in eelgrass seedlings increased (p > 0.05), while reduced GSH, oxidized DHA, and GSSG levels tended to stabilize by the seventh day after initial fluctuations, with the AsA/DHA ratio increasing and the GSH/GSSG ratio normalizing (Figure 2). However, under extreme high temperatures, while reduced AsA and oxidized DHA contents, as well as AsA/DHA ratios, tended to stabilize, reduced GSH content and GSH/GSSG ratio significantly decreased, and oxidized GSSG increased during the stress period (Figure 2). This indicates that under high temperatures, eelgrass seedlings can maintain redox balance by modulating AsA and GSH metabolism to scavenge ROS, but extreme temperatures severely inhibited GSH metabolism, disrupting the redox state.
The AsA-GSH cycle is the primary pathway for hydrogen peroxide (H2O2) metabolism in plants. It involves the decomposition of H2O2 into water and monodehydroascorbate (MDHA) by ascorbate peroxidase (APX), the reduction of MDHA to ascorbate (AsA) or its disproportionation to dehydroascorbate (DHA) by MDHA reductase (MDHAR), and the reduction of DHA back to AsA by dehydroascorbate reductase (DHAR) using glutathione (GSH). Glutathione disulfide (GSSG), which is produced in this process, can be reduced back to GSH via a NADPH-dependent glutathione reductase (GR; Escudero-Feliu et al., 2023). This cycle not only decomposes H2O2 but also regenerates two critical antioxidants, AsA and GSH, aiding in the maintenance of reactive oxygen species (ROS) and redox balance in plants (Chew et al., 2003; Escudero-Feliu et al., 2023, 2023). In this study, under high temperatures, the contents of APX and DHAR initially fluctuated before normalizing, whereas MDHAR and glutathione peroxidase (GPX) enzyme levels significantly increased, particularly MDHAR at day 7 during the stress period (Figure 3). The variations in APX, MDHAR, DHAR, GR, gamma-glutamylcysteine synthetase (γ-ECS), and glutamate-ligase (GaILDH) levels were in line with gene expression upregulation. With the exception of APX, the expression of these cycle-related enzymes decreased later in the stress period (Figure 5). At extremely high temperatures, however, APX and MDHAR levels dropped, DHAR significantly increased, and GPX levels were substantially lower than those in the high-temperature (HT) group (Figure 3). Gene expression also proves the accuracy of the trend of enzyme content changes. The APX gene expression is downregulated in the early stages of stress, and upregulation of MDHAR and GR is also lower than that of the HT group. DHAR and GaILDH are significantly upregulated, while the gene expression level of MDHAR and γ-ECS decreases in the later stage of stress (Figure 5). The study indicates that high temperature stress activates AsA-GSH cycle enzymes in eelgrass seedlings, with the cycle prioritizing the consumption of reduced GSH to ensure the production of reduced AsA and excess H2O2 scavenging, aided by APX. The increase in GPX content, along with a rise in reduced GSH and GR content, suggests a robust GSH pool, essential for the AsA-GSH cycle’s operation and effective H2O2 scavenging (Bela et al., 2015; Rajput et al., 2021). The decrease in gene expression of key enzymes and the reduction of H2O2 and malondialdehyde (MDA) content towards the late stage of stress suggest the AsA-GSH cycle’s role in enhancing heat resistance in eelgrass seedlings. Yet, at extremely high temperatures, a significant increase in DHA content and the substantial depletion of reduced GSH led to a redox imbalance, as evidenced by GSH, GSSG, and the GSH/GSSG ratio (Figure 2), aligning with transcriptomic data (Figure 5). Under extreme temperatures, early stress period downregulation of APX genes, alongside continuous upregulation of GaILDH and DHAR genes, highlights the rapid consumption of reductive AsA and production of oxidative DHA. However, the downregulation of MDHAR, GR, and γ-ECS genes by day 7 suggests reduced GSH production inhibition and GSH/GSSG redox balance disruption. This imbalance indicates that AsA reduction and H2O2 scavenging become unsustainable, leading to a hypothesis of prolonged stress causing complete redox imbalance and eventual death of eelgrass seedlings—a hypothesis confirmed by the complete death of these seedlings after 14 days of stress. Previous research by the group also observed significant decreases in physiological indicators such as wet weight, and leaf dimensions under high temperature stress, aligning with these findings (Yan et al., 2023a, b). Moreover, the significant increase in GaILDH content under high temperature and the decrease in key enzymes for GSH synthesis under extreme temperatures (Figure 3) suggest an inhibition of GSH synthesis, impacting the AsA-GSH cycle’s operation and forcing a larger reliance on the AsA ab initio pathway for reductional AsA generation.
GO and KEGG analysis reveals that the DEGs in the HT and AHT groups, under the biological processes category, predominantly encompass cellular processes, metabolic processes, single-organism processes, responses to stimuli, cellular component organization or biogenesis, biological regulation, localization, and regulation of biological processes. Within the cellular components category, enrichment is notably seen in cells, cell walls, organelle parts, membrane parts, and macromolecular complexes. For molecular functions, enrichment is primarily in binding, catalytic activity, and transporter activity. The DEGs in the HT and Control groups are chiefly enriched in metabolic pathways and biosynthesis of secondary metabolites, whereas those in the AHT and Control groups focus on the biosynthesis of secondary metabolites. Specifically, the DEGs in the AHT and CT groups are enriched in the biosynthesis of amino acids, carbon metabolism, ribosome biogenesis in eukaryotes, DNA replication, starch and sucrose metabolism, carbon fixation in photosynthetic organisms, and nucleocytoplasmic transport. This suggests that the amino acid and carbon metabolism of Zostera marina seedlings are significantly impacted by high temperature stress, aligning with studies on the effects of ocean warming and acidification on Zostera marina (Yan et al., 2023b). Additionally, transcriptome analysis indicates significant enrichment in glutathione metabolism, arachidonic acid metabolism, and ascorbate and aldarate metabolism under high-temperature stress, highlighting the crucial role of the AsA-GSH cycle in the heat stress response of eelgrass seedlings. The analysis also reveals significant up-regulation of HSP70 and HSP90 related genes, consistent with findings by Marín-Guirao et al. (2016).
In summary, high-temperature conditions trigger the activation of the AsA-GSH cycle in eelgrass seedlings, utilizing AsA to reduce H2O2 and maintain the AsA/DHA redox balance via reduced GSH. This process also involves up-regulating various enzymes within the cycle to regulate H2O2 clearance and redox balance. However, at extreme high temperatures, the suppression of circulating APX enzymes leads to rapid H2O2 accumulation, resulting in the oxidation of AsA to DHA and a swift depletion of the GSH pool. This disrupts the GSH/GSSG equilibrium and affects the AsA/DHA balance, exacerbating oxidative damage over time, potentially leading to death. After 14 days of stress at extreme high temperatures, all eelgrass seedlings died, affirming the severe impact of prolonged high-temperature stress. Thus, while high-temperature stress activates the AsA-GSH cycle to enhance heat tolerance in eelgrass seedlings, it is insufficient to maintain redox balance under extreme conditions, leading to significant oxidative damage.
In this study, we demonstrated that under high-temperature stress (23°C), Z. marina seedlings could increase the expression of AsA-GSH cycle-related enzymes and their synthetase genes to maintain redox homeostasis. This adaptation potentially enhances heat resistance and prevents oxidative damage. However, at extremely high temperatures (28°C), the AsA-GSH cycle was insufficient to preserve the redox balance in eelgrass seedlings. The DHAR content significantly rose after the 7th day of stress, yet the redox state was disrupted due to a substantial accumulation of GSSG. Consequently, the AsA-GSH cycle nearly ceased, leading to death after 14 days of stress. Thus, extreme high temperatures significantly impair the growth of seedlings. With the increasing frequency of such temperatures, seagrass beds, including their establishment and expansion, will be severely impacted. This research is valuable for further exploration and identification of heat-resistant genes in seagrass. It also provides theoretical support for the restoration of Z. marina seagrass beds.
The datasets presented in this study can be found in online repositories. The names of the repository/repositories and accession number(s) can be found below: The transcriptome data can be found in Bioproject accession number: PRJNA1069623. Further inquiries can be directed to the corresponding authors.
YP: Data curation, Formal analysis, Methodology, Visualization, Writing – original draft, Writing – review & editing. ZW: Data curation, Formal analysis, Methodology, Writing – original draft. WY: Conceptualization, Funding acquisition, Supervision, Writing – original draft, Writing – review & editing. BZ: Conceptualization, Funding acquisition, Supervision, Writing – review & editing.
The author(s) declare financial support was received for the research, authorship, and/or publication of this article. The study was supported by the National Natural Science Foundation of China (grant number: 42076147; 42306135). The funder is the corresponding author BZ and WY, respectively.
We thank editors and reviewers for very helpful comments on the manuscript.
The authors declare that the research was conducted in the absence of any commercial or financial relationships that could be construed as a potential conflict of interest.
All claims expressed in this article are solely those of the authors and do not necessarily represent those of their affiliated organizations, or those of the publisher, the editors and the reviewers. Any product that may be evaluated in this article, or claim that may be made by its manufacturer, is not guaranteed or endorsed by the publisher.
Alscher R. G., Donahue J. L., Cramer C. L. (1997). Reactive oxygen species and antioxidants: relationships in green cells. Physiol. Plant. 100, 224–233. doi: 10.1111/j.1399-3054.1997.tb04778.x
Arias-Ortiz A., Serrano O., Masqué P., Lavery P. S., Mueller U., Kendrick G. A., et al. (2018). A marine heatwave drives massive losses from the world’s largest seagrass carbon stocks. Nat. Climate Change. 8, 338–344. doi: 10.1038/s41558-018-0096-y
Bartoli C. G., Buet A., Grozeff G. G., Galatro A., Simontacchi M. (2017). “Ascorbate-glutathione cycle and abiotic stress tolerance in plants,” in Ascorbic Acid in Plant Growth, Development and Stress Tolerance, eds. Hossain M., Munné-Bosch S., Burritt D., Diaz-Vivancos P., Fujita M., Lorence A. (Cham: Springer), 177–200. doi: 10.1007/978–3-319–74057-7_7
Bela K., Horváth E., Gallé Á, Szabados L., Tari I., Csiszár J. (2015). Plant glutathione peroxidases: emerging role of the antioxidant enzymes in plant development and stress responses. J. Plant Physiol. 176, 192–201. doi: 10.1016/j.jplph.2014.12.014
Buege J. A., Aust S. D. (1978). Microsomal lipid peroxidation. Methods Enzymol. 52, 302–310. doi: 10.1016/S0076-6879(78)52032-6
Chefaoui R. M., Duarte C. M., Serrão E. A. (2018). Dramatic loss of seagrass habitat under projected climate change in the Mediterranean Sea. Glob Chang Biol. 24, 4919–4928. doi: 10.1111/gcb.14401
Chen S., Zhou Y., Chen Y., Gu J. (2018). fastp: an ultra-fast all-in-one FASTQ preprocessor. Bioinformatics 34, i884–i890. doi: 10.1093/bioinformatics/bty560
Chew O., Whelan J., Millar A. H. (2003). Molecular definition of the ascorbate-glutathione cycle in Arabidopsis mitochondria reveals dual targeting of antioxidant defenses in plants. J. Biol. Chem. 278, 46869–46877. doi: 10.1074/jbc.M307525200
Christidis N., Jones G. S., Stott P. A. (2015). Dramatically increasing chance of extremely hot summers since the 2003 European heatwave. Nat. Climate Change. 5, 46–50. doi: 10.1038/nclimate2468
Collier C. J., Waycott M. (2014). Temperature extremes reduce seagrass growth and induce mortality. Mar. pollut. Bull. 83, 483–490. doi: 10.1016/j.marpolbul.2014.03.050
Duarte C. M. (2002). The future of seagrass meadows. Environ. Conserv. 29, 192–206. doi: 10.1017/S0376892902000127
Duarte C. M., Dorte K. J. (2017). Export from seagrass meadows contributes to marine carbon sequestration. Front. Mar. Sci. 4. doi: 10.3389/fmars.2017.00013
Edgar G. J. (1994). Comparisons of species richness, size-structure and production of benthos in vegetated and unvegetated habjtats in Western Port, Victoria. J. Exp. Mar. Biol. Ecol. 176, 201–226. doi: 10.1016/0022-0981(94)90185-6
Escudero-Feliu J., Lima-Cabello E., Rodríguez de Haro E., Morales-Santana S., Jimenez-Lopez J. C. (2023). Functional association between storage protein mobilization and redox signaling in narrow-leafed lupin (Lupinus angustifolius L.) seed germination and seedling development. Genes (Basel) 14, 1889. doi: 10.3390/genes14101889
Fotopoulos V., Ziogas V., Tanou G., Molassiotis A. (2010). “Involvement of asA/DHA and GSH/GSSG ratios in gene and protein expression and in the activation of defence mechanisms under abiotic stress conditions,” in Ascorbate-Glutathione Pathway and Stress Tolerance in Plants, eds. Anjum N., Chan M. T., Umar S. (Dordrecht: Springer), 265–302. doi: 10.1007/978–90-481–9404-9_10
Foyer C. H., Noctor G. (2011). Ascorbate and glutathione: the heart of the redox hub. Plant Physiol. 155, 2–18. doi: 10.1104/pp.110.167569
Groden D., Beck E. (1979). H2O2 destruction by ascorbate-dependent systems from chloroplasts. Biochim. Biophys. Acta 546, 426–435. doi: 10.1016/0005-2728(79)90078-1
Hemming M. A., Duarte C. M. (2000). Seagrass Ecology (Cambridge: Cambridge University Press), 20–23.
Hoegh-Guldberg O., Bruno J. F. (2010). The impact of climate change on the world’s marine ecosystems. Science 328, 1523–1528. doi: 10.1126/science.1189930
Islam T., Manna M., Kaul T., Pandey S., Subramanyam Reddy C., Reddy M. K. (2015). Genome-wide dissection of arabidopsis and rice for the identification and expression analysis of glutathione peroxidases reveals their stress-specific and overlapping response patterns. Plant Mol. Biol. Report. 33, 1413–1427. doi: 10.1007/s11105-014-0846-6
Jiang T., Zhai J., Luo Y., Su B., Chao Q., Wang Y., et al. (2022). Understandings of assessment reports on climate change impacts, adaptation and vulnerability: progress from IPCC AR5 to AR6. Trans. Atmos Sci. 45, 502–511. doi: 10.13878/j.cnki.dqkxxb.20220529013
Jordà G., Marbà N., Duarte C. M. (2012). Mediterranean seagrass vulnerable to regional climate warming. Nat. Climate Change 2, 821–824. doi: 10.1038/nclimate1533
Jung H. I., Kong M. S., Lee B. R., Kim T. H., Chae M. J., Lee E. J., et al. (2019). Exogenous glutathione increases arsenic translocation into shoots and alleviates arsenic-induced oxidative stress by sustaining ascorbate-glutathione homeostasis in rice seedlings. Front. Plant Sci. 10. doi: 10.3389/fpls.2019.01089
Kaldy J. E., Brown C. A., Nelson W. G., Frazier M. (2017). Macrophyte community response to nitrogen loading and thermal stressors in rapidly flushed mesocosm systems. J. Exp. Mar. Biol. Ecol. 497, 107–119. doi: 10.1016/j.jembe.2017.09.022
Kang G. Z., Li G. Z., Liu G. Q., Xu W., Peng X. Q., Wang C. Y., et al. (2013). Exogenous salicylic acid enhances wheat drought tolerance by influence on the expression of genes related to ascorbate-glutathione cycle. Biol. Plant 57, 718–724. doi: 10.1007/s10535-013-0335-z
Karam E. A., Maresca V., Sorbo S., Keramat B., Basile A. (2017). Effects of triacontanol on ascorbate-glutathione cycle in Brassica napus L. exposed to cadmium-induced oxidative stress. Ecotoxicol. Environ. Saf. 144, 268–274. doi: 10.1016/j.ecoenv.2017.06.035
Karl T. R., Trenberth K. E. (2003). Modern global climate change. Science 302, 1719–1723. doi: 10.1126/science.1090228
Kaya C., Ashraf M., AlYemeni M. N., Ahmad P. (2019). The role of endogenous nitric oxide in salicylic acid-induced up-regulation of ascorbate-glutathione cycle involved in salinity tolerance of pepper (Capsicum annuum L.) plants. Plant Physiol. Biochem. 147, 10–20. doi: 10.1016/j.plaphy.2019.11.040
Kendrick G. A., Nowicki R. J., Olsen Y. S., Strydom S., Fraser M. W., Sinclair E. A., et al. (2019). A systematic review of how multiple stressors from an extreme event drove ecosystem-wide loss of resilience in an iconic seagrass community. Front. Mar. Sci. 6. doi: 10.3389/fmars.2019.00455
Koch M., Bowes G., Ross C., Zhang X. H. (2013). Climate change and ocean acidification effects on seagrasses and marine macroalgae. Glob Chang Biol. 19, 103–132. doi: 10.1111/j.1365-2486.2012.02791.x
Langmead B., Salzberg S. L. (2012). Fast gapped-read alignment with Bowtie 2. Nat. Methods 9, 357–359. doi: 10.1038/nmeth.1923
Lee H., Golicz A. A., Bayer P. E., Jiao Y., Tang H., Paterson A. H., et al. (2016). The genome of a southern hemisphere seagrass species (Zostera muelleri). Plant Physiol. 172, 272–283. doi: 10.1104/pp.16.00868
Lee K. S., Park S. R., Kim Y. K. (2007). Effects of irradiance, temperature, and nutrients on growth dynamics of seagrasses: A review. J. Exp. Mar. Biol. Ecol. 350, 144–175. doi: 10.1016/j.jembe.2007.06.016
Li B., Dewey C. N. (2011). RSEM: accurate transcript quantification from RNA-Seq data with or without a reference genome. BMC Bioinf. 12, 323. doi: 10.1186/1471-2105-12-323
Linster C. L., Clarke S. G. (2008). L-Ascorbate biosynthesis in higher plants: the role of VTC2. Trends Plant Sci. 13, 567–573. doi: 10.1016/j.tplants.2008.08.005
Love M. I., Huber W., Anders S. (2014). Moderated estimation of fold change and dispersion for RNA-seq data with DESeq2. Genome Biol. 15, 550. doi: 10.1186/s13059-014-0550-8
Magel C. L., Chan F., Hessing-Lewis M., Hacker S. D. (2022). Differential responses of eelgrass and macroalgae in Pacific Northwest estuaries following an unprecedented NE Pacific Ocean marine heatwave. Front. Mar. Sci. 9. doi: 10.3389/fmars.2022.838967
Marbà N., Duarte C. M. (2010). Mediterranean warming triggers seagrass (Posidonia oceanica) shoot mortality. Glob Chang Biol. 16, 2366–2375. doi: 10.1111/j.1365–2486.2009.02130.x
Marín-Guirao L., Ruiz J. M., Dattolo E., Garcia-Munoz R., Procaccini G. (2016). Physiological and molecular evidence of differential short-term heat tolerance in Mediterranean seagrasses. Sci. Rep. 6, 28615. doi: 10.1038/srep28615
Massa S. I., Pearson G. A., Aires T., Kube M., Olsen J. L., Reinhardt R., et al. (2011). Expressed sequence tags from heat-shocked seagrass Zostera noltii (Hornemann) from its southern distribution range. Mar. Genomics 4, 181–188. doi: 10.1016/j.margen.2011.04.003
McDevitt-Irwin J., Iacarella J., Baum J. (2016). Reassessing the nursery role of seagrass habitats from temperate to tropical regions: A meta-analysis. Mar. Ecol. Prog. Series. 557, 133–143. doi: 10.3354/meps11848
Mtwana Nordlund L., Koch E. W., Barbier E. B., Creed J. C. (2016). Seagrass ecosystem services and their variability across genera and geographical regions. PloS One 11, e0163091. doi: 10.1371/journal.pone.0163091
Nguyen H. M., Ralph P. J., Marín-Guirao L., Pernice M., Procaccini G. (2021). Seagrasses in an era of ocean warming: a review. Biol. Rev. Camb Philos. Soc 96, 2009–2030. doi: 10.1111/brv.12736
Noctor G., Foyer C. H. (1998). ASCORBATE AND GLUTATHIONE: keeping active oxygen under control. Annu. Rev. Plant Physiol. Plant Mol. Biol. 49, 249–279. doi: 10.1146/annurev.arplant.49.1.249
Olsen J. L., Rouzé P., Verhelst B., Lin Y. C., Bayer T., Collen J., et al. (2016). The genome of the seagrass Zostera marina reveals angiosperm adaptation to the sea. Nature 530, 331–335. doi: 10.1038/nature16548
Oppenheimer L., Wellner V. P., Griffith O. W., Meister A. (1979). Glutathione synthetase. Purification from rat kidney and mapping of the substrate binding sites. J. Biol. Chem. 254, 5184–5190. doi: 10.1016/S0021-9258(18)50577-9
Orth R. J., Lefcheck J. S., McGlathery K. S., Aoki L., Luckenbach M. W., Moore K. A., et al. (2020). Restoration of seagrass habitat leads to rapid recovery of coastal ecosystem services. Sci. Adv. 6, eabc6434. doi: 10.1126/sciadv.abc6434
Pertea M., Pertea G. M., Antonescu C. M., Chang T. C., Mendell J. T., Salzberg S. L. (2015). StringTie enables improved reconstruction of a transcriptome from RNA-seq reads. Nat. Biotechnol. 33, 290–295. doi: 10.1038/nbt.3122
Rajput V. D., Harish, Singh R. K., Verma K. K., Sharma L., Quiroz-Figueroa F. R., et al. (2021). Recent developments in enzymatic antioxidant defence mechanism in plants with special reference to abiotic stress. Biol. (Basel). 10, 267. doi: 10.3390/biology10040267
Rakhmad P., Hariyanto S, Sri Y., Purnobasuki H. (2019). Gene expression of antioxidant enzymes and heat shock proteins in tropical seagrass Thalassia hemprichii under heat Stress. Taiwania. 64, 117–123. doi: 10.6165/tai.2019.64.117
Sharma P., Jha A. B., Dubey R. S., Pessarakli M. (2012). Reactive oxygen species, oxidative damage, and antioxidative defense mechanism in plants under stressful conditions. J. Bot. 2012, 26. doi: 10.1155/2012/217037
Strydom S., Murray K., Wilson S., Huntley B., Rule M., Heithaus M., et al. (2020). Too hot to handle: Unprecedented seagrass death driven by marine heatwave in a World Heritage Area. Glob. Chang. Biol. 26, 3525–3538. doi: 10.1111/gcb.15065
Trisos C. H., Merow C., Pigot A. L. (2020). The projected timing of abrupt ecological disruption from climate change. Nature 580, 496–501. doi: 10.1038/s41586-020-2189-9
Tutar O., Marín-Guirao L., Ruiz J. M., Procaccini G. (2017). Antioxidant response to heat stress in seagrasses. A gene expression study. Mar. Environ. Res. 132, 94–102. doi: 10.1016/j.marenvres.2017.10.011
Unsworth R. K. F., Ambo-Rappe R., Jones B. L., La Nafie Y. A., Irawan A., Hernawan U. E., et al. (2018). Indonesia's globally significant seagrass meadows are under widespread threat. Sci. Total Environ. 634, 279–286. doi: 10.1016/j.scitotenv.2018.03.315
Wang Z., Pei Y., Yan W., Lu L., Zhou B. (2023). Seasonal temperature variation in Zostera marina seedlings under ocean acidification. Front. Mar. Sci. 10. doi: 10.3389/fmars.2023.1304132
Waycott M., Duarte C. M., Carruthers T. J., Orth R. J., Dennison W. C., Olyarnik S., et al. (2009). Accelerating loss of seagrasses across the globe threatens coastal ecosystems. Proc. Natl. Acad. Sci. U S A. 106, 12377–12381. doi: 10.1073/pnas.0905620106
Wheeler G. L., Jones M. A., Smirnoff N. (1998). The biosynthetic pathway of vitamin C in higher plants. Nature 393, 365–369. doi: 10.1038/30728
Xu S. (2021). Study on population characteristics and ecological restoration of Zostera marina in the Yellow Sea and Bohai Sea (Qingdao: University of the Chinese Academy of Sciences, Institute of Oceanology, Chinese Academy of Sciences). doi: 10.27551/d.cnki.gzkhs.2021.000102
Yan W., Liu J., Seng S., Zhou B., Ding K. (2019). Cloning, characterization and expression analysis of a microsomal glutathione S-transferase gene from the seagrass Zostera marina. Acta Oceanol. Sin. 38, 111–115. doi: 10.1007/s13131-019-1429-z
Yan W., Wang Z., Pei Y., Zhou B. (2023a). Adaptive responses of eelgrass (Zostera marina L.) to ocean warming and acidification. Plant Physiol. Biochem. 206, 108257. doi: 10.1016/j.plaphy.2023.108257
Yan W., Wang Z., Pei Y., Zhou B. (2023b). How does ocean acidification affect Zostera marina during a marine heatwave? Mar. Pollut. Bull. 194, 115394. doi: 10.1016/j.marpolbul.2023.115394
Yao M., Ge W., Zhou Q., Zhou X., Luo M., Zhao Y., et al. (2021). Exogenous glutathione alleviates chilling injury in postharvest bell pepper by modulating the ascorbate-glutathione (AsA-GSH) cycle. Food Chem. 352, 129458. doi: 10.1016/j.foodchem.2021.129458
Keywords: Zostera marina seedlings, ascorbate-glutathione cycle, high temperature stress, reactive oxygen species, transcriptome
Citation: Pei Y, Wang Z, Yan W and Zhou B (2024) Characterization of ascorbate-glutathione cycle response in Zostera marina seedlings under short-term temperature surge. Front. Mar. Sci. 11:1390074. doi: 10.3389/fmars.2024.1390074
Received: 26 March 2024; Accepted: 06 May 2024;
Published: 23 May 2024.
Edited by:
Shaochun Xu, Chinese Academy of Sciences (CAS), ChinaReviewed by:
QuanSheng Zhang, Yantai University, ChinaCopyright © 2024 Pei, Wang, Yan and Zhou. This is an open-access article distributed under the terms of the Creative Commons Attribution License (CC BY). The use, distribution or reproduction in other forums is permitted, provided the original author(s) and the copyright owner(s) are credited and that the original publication in this journal is cited, in accordance with accepted academic practice. No use, distribution or reproduction is permitted which does not comply with these terms.
*Correspondence: Wenjie Yan, eWFud2VuamllQG91Yy5lZHUuY24=; Bin Zhou, WmhvdWJpbkBvdWMuZWR1LmNu
†These authors have contributed equally to this work
Disclaimer: All claims expressed in this article are solely those of the authors and do not necessarily represent those of their affiliated organizations, or those of the publisher, the editors and the reviewers. Any product that may be evaluated in this article or claim that may be made by its manufacturer is not guaranteed or endorsed by the publisher.
Research integrity at Frontiers
Learn more about the work of our research integrity team to safeguard the quality of each article we publish.