- 1Leibniz Centre for Tropical Marine Research – ZMT, Bremen, Germany
- 2Institute of Geology, Universität Hamburg, Hamburg, Germany
- 3Oceans and Coasts Research Branch, Department of Environment, Forestry and Fisheries, Cape Town, South Africa
- 4Department of Oceanography, University of Cape Town, Rondebosch, South Africa
- 5Bayworld Centre for Research and Education, Cape Town, South Africa
- 6National Marine Information and Research Centre, Swakopmund, Namibia
Bottom-trawl fishery is known to cause major disturbances to marine sediments as the dragging of trawl gears across the seabed fosters sediment resuspension, which can lead to organic particle remineralization and release of benthic CO2 and nutrients into bottom waters. However, its effects on carbon cycling and biological productivity, especially in highly productive regions like the Benguela Upwelling System (BUS), are less well studied. Here, we simulated carbon (C) and nutrient pathways from the trawled coastal seabed to overlying water masses that are being upwelled into the sunlit surface within the BUS, using shipboard data on sea surface and water column characteristics and published benthic CO2 emission estimates from bottom-trawled sediments. The latter reports 4.35 and 0.64 Tg C year-1 to be released from the seabed into upwelling source waters after bottom trawling in the northern (NBUS) and southern (SBUS) subsystems, respectively. Based on these values, we estimated a corresponding nitrate (N) input of 1.39 and 0.47 µmol kg-1 year-1, enhancing source water nitrate concentrations by ~5% and ~2%. Trawl-induced nitrate input into the sunlit surface could support a new production of 3.14 and 0.47 Tg C year-1 in the NBUS and SBUS, respectively, recapturing only 2/3 of CO2 released after bottom trawling into biomass, mainly due to differences in stoichiometric C:N ratios between the sediment (~9) and surface biomass (Redfield, 6.6). The remaining benthic CO2 can thereby lead to an increase in surface CO2 concentration and its partial pressure (pCO2), impeding CO2 uptake of the biological carbon pump in the BUS by 1.3 Tg C year-1, of which 1 Tg C year-1 is emitted to the atmosphere across the northern subsystem. Our results demonstrate the extent to which bottom trawling may affect the CO2 storage potential of coastal sediments on a basin-wide level, highlighting the need to better resolve small-scale sediment characteristics and C:N ratios to refine trawl-induced benthic carbon and nutrient effluxes within the BUS.
1 Introduction
The burial of carbon as organic matter in ocean sediments forms an integral part in the global carbon cycle by removing carbon from its three main reservoirs: the ocean, the atmosphere and the terrestrial biosphere (Siegenthaler and Sarmiento, 1993; Avelar et al., 2017; Rixen, 2023). The underlying mechanism refers to the assimilation of CO2 through the generation of biomass by plants on land and by phytoplankton in the ocean and its transfer into marine sediments. In the ocean, the fixation of CO2 into biomass in the sunlit surface ocean and its transport across the water column and into sediments is commonly termed as the biological carbon pump (Volk and Hoffert, 1985; Boyd et al., 2019). Hereby, coastal upwelling ecosystems, especially along the eastern margins of the Pacific and Atlantic basins, play a crucial role for the sediment carbon storage. They belong to one of the most productive regions in the ocean, contributing 11% to global new production, which refers to biomass largely produced based on upwelled nutrients (Eppley and Peterson, 1979; Chavez and Toggweiler, 1995; Messié et al., 2009), while supporting high amounts of carbon being transferred and stored in the ocean and sediments. Due to their outstanding biological productivity, these upwelling systems are particularly vulnerable to anthropogenic pressures like fisheries (Sala et al., 2021). Hereby, bottom trawling fisheries are being regarded as the greatest source of physical disturbance to the seafloor (Amoroso et al., 2018; O’Hara et al., 2021), with the potential to alter carbon-rich sea sediments and their capacity to store atmospheric CO2 (De Borger et al., 2021; Epstein et al., 2022). However, the impact of bottom trawling on biogeochemical cycling and release of carbon into the water column and atmosphere is still subject to intense discussion (Palanques et al., 2014; Pusceddu et al., 2014; Hale et al., 2017; Paradis et al., 2019; Tiano et al., 2019).
The ocean’s carbon storage received broad recognition and became part of nationwide climate change mitigation strategies through the concept of ‘Blue Carbon’ (BC) (Hilmi et al., 2021; Christianson et al., 2022). BC was promoted in 2009 and intended to facilitate carbon quantification and to provide guidance for sustainable resource management and conservation of carbon stored by marine ecosystems within the coastal and open ocean (Nellemann et al., 2009). Although coastal ecosystems like mangroves, seagrasses and saltmarshes are currently assigned to BC (Pendleton et al., 2012), biomass carbon storages within the water column and sediment across the continental shelf, slope and deep ocean remain unconsidered, despite their relevance in mitigating greenhouse gas (GHG) emissions (Howard et al., 2017; Luisetti et al., 2019). The inclusion of sediments, especially those located in productive upwelling ecosystems into the BC framework should therefore be perceived as a key interest to sustain and manage carbon storages and to foster climate change mitigation strategies. As a precondition, various criteria have to be met before establishing marine ecosystems as BC, such as whether they can be managed to facilitate climate change mitigation or are currently affected by anthropogenic disturbances, with the impact being observable and quantifiable (Lovelock and Duarte, 2019). However, a lack of data to elaborate carbon emissions and sequestration capacities, human impacts and the effectiveness of management strategies to reduce GHG emissions in coastal sediments are all key criteria that currently prevent these coastal habitats from being assigned to the BC concept, albeit of rising scientific concerns on the quantity and vulnerability of sediment carbon storages to anthropogenic activities (Avelar et al., 2017; Atwood et al., 2020; Sala et al., 2021).
Hence, the goal of our study is to further incentivize the integration of sediments in the BC discourse by elucidating the impact of human interventions through bottom trawl fisheries on the sedimentary carbon storage and its potential role in driving atmospheric CO2 emissions in one of the most productive coastal upwelling systems, namely the Benguela Upwelling System (BUS). We thereby focus on the effects of bottom trawling on the resuspension of particulate matter at the seafloor (Oberle et al., 2016; Breimann et al., 2021) that has further been linked to increased oxygen consumption and organic matter remineralization which, in turn, can cause high amounts of nutrients and dissolved inorganic carbon (DIC) to be released into the water column (Almroth-Rosell et al., 2012; Bradshaw et al., 2021; Breimann et al., 2021) (Figure 1). By combining sea surface measurements with water column profiles of the upwelling source waters from the past two decades across the BUS, we simulated the impact of bottom trawling on the water column and sea surface partial pressure of CO2 (pCO2) using CO2SYS simulations (Lewis and Wallace, 1998; Humphreys et al., 2020) and recently published global benthic CO2 emission estimates from bottom trawling activities (Sala et al., 2021). Hereby, CO2 emissions of bottom trawling are defined as the labile fraction of carbon released into the bottom water as aqueous CO2 (DIC). This DIC stems from the remineralization process of resuspended sediment after the occurrence of bottom trawling, and is referred to as the benthic CO2 efflux. By estimating the effect of the benthic CO2 efflux on the biological productivity, we additionally shed light on the role of carbon to nutrient ratios in mitigating CO2 emissions from bottom trawling activities.
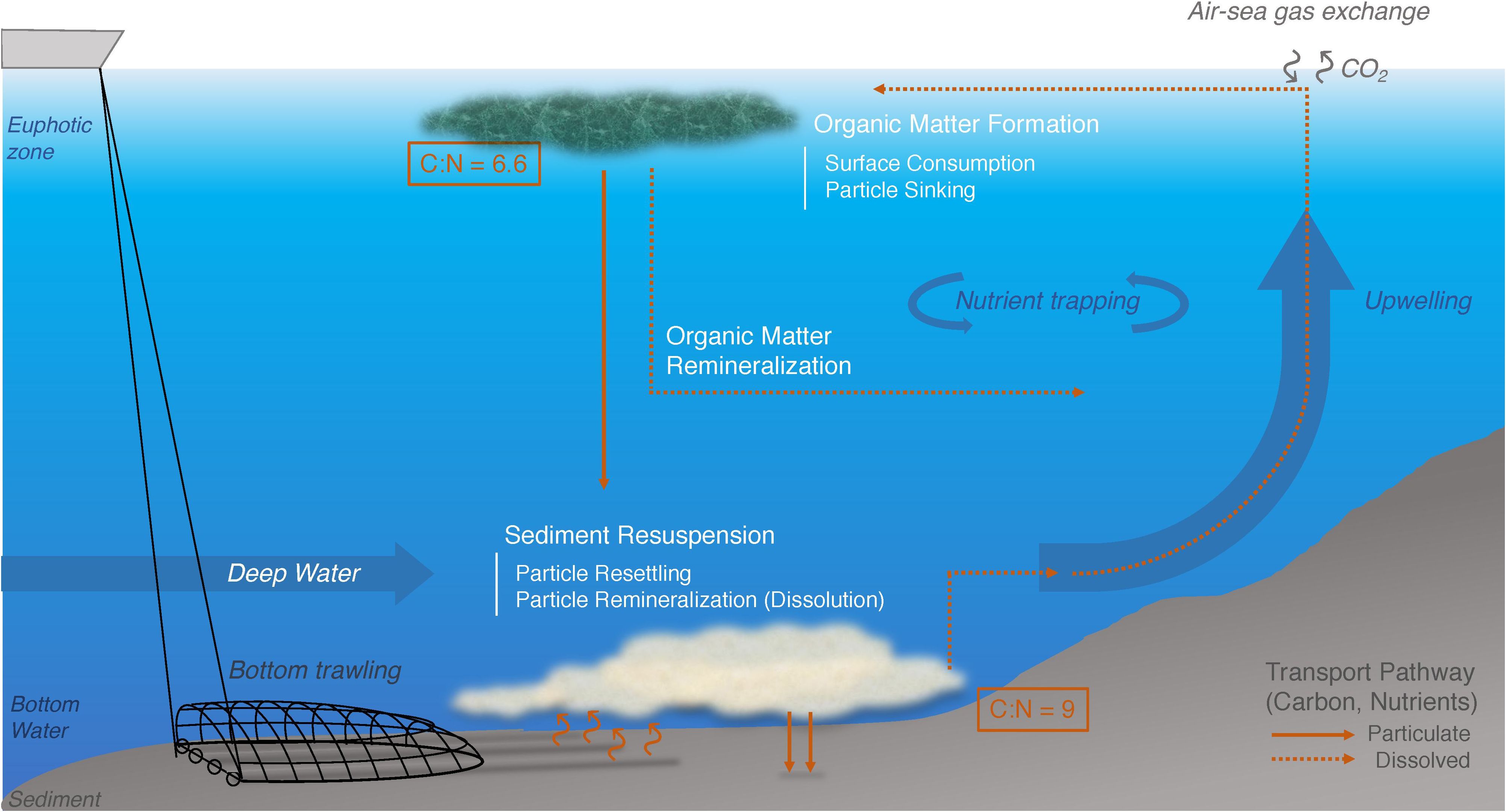
Figure 1. Schematic overview depicting the impact of bottom trawling on the transport pathways of carbon and nutrients within the Benguela Upwelling System.
2 Study site
The region under study is the Benguela Upwelling System (BUS), which is located along the west coast of southern Africa and stretches from the Angola Benguela Frontal Zone at around 17°S to the south-western tip of South Africa at approximately 34°S (Kämpf and Chapman, 2016). Due to the offshore wind-driven advection of water masses, this region is dominated by coastal upwelling, a process known to uplift cold, CO2- and nutrient-rich water masses from the deep ocean into the surface region. With multiple upwelling cells along the shoreline, the Lüderitz Cell at around 26°S is the strongest one separating the BUS into a northern (NBUS) and southern part (SBUS) (Hutchings et al., 2009), which cover areas of 377,400 and 177,600 km2, respectively (Siddiqui et al., 2023). Both subsystems are influenced by two distinct source water masses dominating the bottom shelf region, namely South Atlantic Central Water (SACW) in the NBUS, and Eastern South Atlantic Central Water (ESACW) in the SBUS (McCartney, 1977; Gordon et al., 1992; Shillington et al., 2006). Near the coast, upwelling of CO2- and nutrient-rich waters leads to an initial rise in the sea surface partial pressure of CO2 (pCO2) above that of the atmosphere and outgassing of CO2 at the air-sea interface, which is further amplified by the warming of upwelling waters and ultimate reduction of the CO2 solubility in seawater. In turn, the availability of nutrients creates a favorable environment for primary producers to fix CO2 into biomass, which could also be displayed e.g., by enhanced satellite chlorophyll concentrations in close proximity to the coast (Weeks et al., 2006; Demarcq et al., 2007; Lamont et al., 2019), leading to a continual decrease in pCO2 within the offshore-advecting upwelling waters. In the SBUS, the biologically-mediated CO2 uptake offsets the increase of CO2 due to surface warming of upwelling waters, causing this region to act as an atmospheric CO2 sink, while in the NBUS, the impact of surface warming on the pCO2 exceeds the effect of the biological carbon pump and promotes CO2 outgassing (Siddiqui et al., 2023). The biologically-mediated CO2 uptake is strongly affected by the upwelling source water’s nutrient concentration, which comprise of (a) biologically-unused, so-called preformed nutrients, (b) regenerated nutrients originating from remineralization of organic matter within the water column, and (c) those nutrients released by the remineralization of organic matter in sediments across the sediment-water interface via diffusional processes (Neumann et al., 2016).
The biological productiveness of the BUS promotes the sinking and subsequent remineralization of organic matter (OM) previously formed at the sea surface. Biogeochemical oxygen consumption linked to organic matter remineralization causes low dissolved oxygen concentrations, leading to the development of an Oxygen Minimum Zone (OMZ) in both the NBUS and SBUS. In the latter case, the OMZ is mostly controlled by the seasonality in biological productivity (Pitcher et al., 2014; Lamont et al., 2015), while in the NBUS, the OMZ is mainly governed by the seasonality in the poleward advection of poorly oxygenated SACW, leading to a greater expansion of the OMZ across the Namibian shelf and continental slope (Monteiro et al., 2006; Mohrholz et al., 2008). The high biological productivity and low bottom oxygen in the water column foster the accumulation of OM at the seafloor. In the NBUS, this has led to the formation of a mud belt region of mainly diatomaceous ooze (Emeis et al., 2004) in shallow depths across the 100 – 200 m isobath from where OM is further transported laterally and deposited across the upper to central slope off Namibia (Monteiro et al., 2005; Inthorn et al., 2006; van der Plas et al., 2007) (Figure 2A).
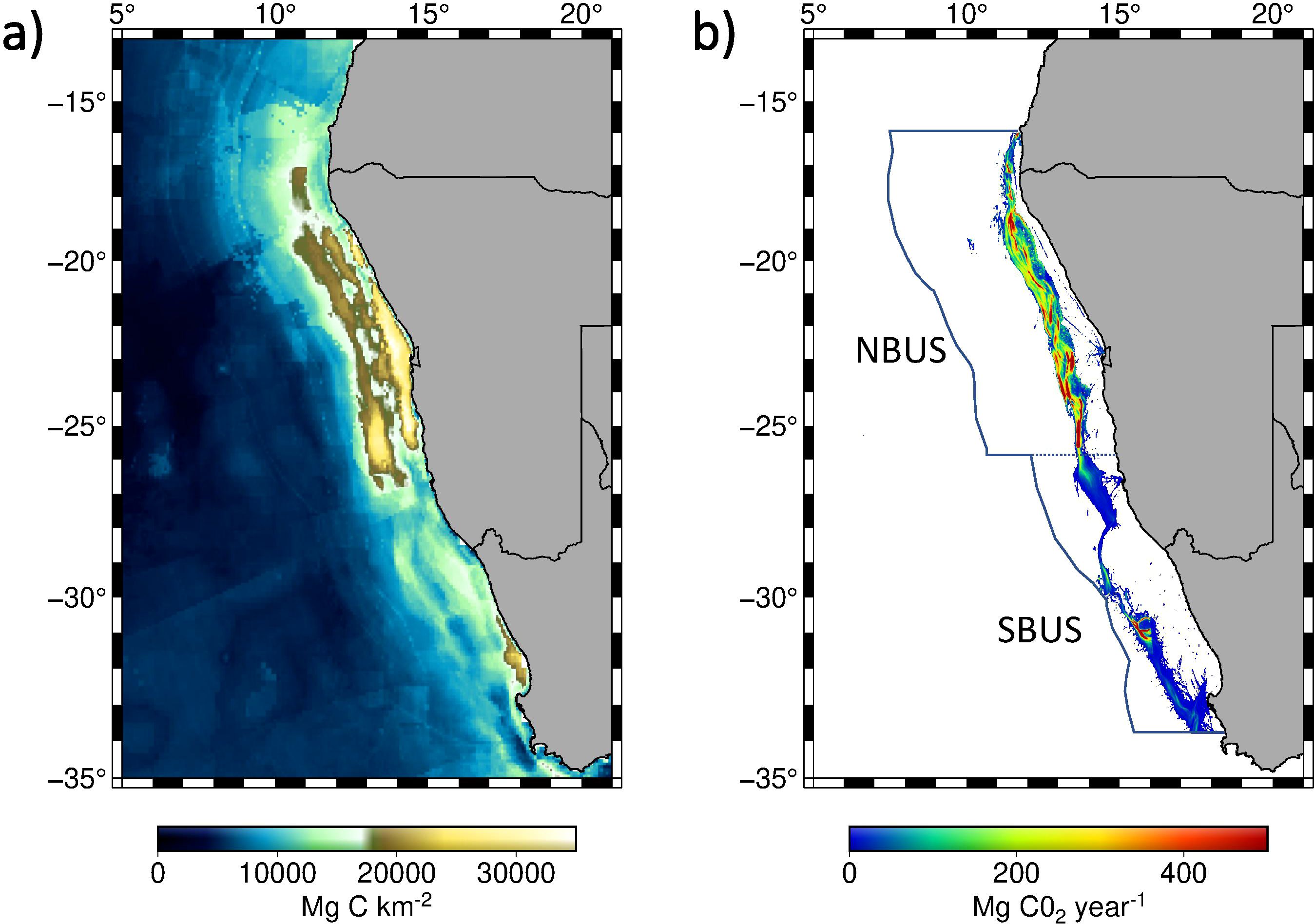
Figure 2. Map showing (A) published carbon storage within the first meter of sediment (Mg C km-2) (Atwood et al., 2020) and (B) the benthic CO2 emission estimates from bottom trawling activities (Mg CO2 year-1) (Sala et al., 2021) within the Benguela Upwelling System’s northern (NBUS) and southern (SBUS) part.
Meanwhile, at the base of the marine food chain, primary producers further support a vast richness in marine species that are relevant for the fishing industry, with the hake directed bottom-trawl fishery being the most economically valuable one in both Namibia and South Africa (Mwafila, 2017). Bottom trawling activities in the BUS mainly occur along the outer continental shelf and upper slope region within water depths between 200 and 1000 m (Figure 2B). The inner shelf (<200 m) remains largely unaffected as a result of trawl restrictions within the Namibian Exclusive Economic Zone (EEZ). These restrictions were previously passed for the protection of juvenile fish, as well as due to fishing legislations within Marine Protected Areas (MPAs) that are located within the shallow coastal zone, with the MPAs’ spatial extent making up less than 2% of the Namibian EEZ (Finke et al., 2020). In the BUS, trawling gears are dragged across unconsolidated sediments that can mainly be classified as terrigenous, biogenic and authigenic (Rogers and Rau, 2010). Surficial sediments from the north are further characterized by relict, nitrogen-poor particulate organic matter, which originated from the inner-shelf zone from where it was redistributed under low oxygen conditions across the bottom shelf to offshore depocenters off Namibia (Monteiro et al., 2005; Inthorn et al., 2006; Bruni et al., 2022).
3 Material and methods
3.1 Modelling concept
We elucidate the impact of bottom trawling on the biological carbon pump by focusing on changes in the water column, sea surface pCO2 and new production that could arise from the additional release of CO2 into the bottom water after the remineralization of resuspended sediments. It was shown that the release of sedimentary carbon through bottom trawling may extend up to 10 m or more above the seafloor (Churchill, 1989) and lead to resuspended sediments being remineralized and further advected laterally and vertically across the water column (Pusceddu et al., 2014; De Borger et al., 2021; Morys et al., 2021). Additional experimental model results from a study in the Eastern Mediterranean indicated bottom trawl-induced plumes of sediment along the outer continental shelf and upper slope promoted the supply of remineralized benthic nutrients into the euphotic zone during coastal upwelling, increasing annual net primary productivity by 15% (Dounas et al., 2007). Thus, we assumed the released sedimentary carbon as reported by (Sala et al., 2021) to be fed into the upwelling source waters that are overlaying the bottom shelf region, and subsequently, to be upwelled together with the source waters into the surface region within the individual subsystems. We also took into account the release of nutrients during the remineralization process of resuspended organic matter and their transfer into the upwelling source water masses. The extent to which source water mass concentrations of carbon and nutrients are raised by bottom trawling is hereby controlled by the volume of upwelling waters which the benthic nutrients and carbon are dispersed into. To address the effect of bottom trawling on the air-sea gas exchange, we simulated sea surface pCO2 using the CO2SYS routine with source water masses’ DIC, Total Alkalinity (TA) and nutrient concentrations, as well as source water temperature and salinity (SWT, SWS) as input parameters (Table 1). We assumed upwelled nutrients and carbon of the source water masses to be consumed at the surface following the Redfield C:N ratio of 106:16 (Rixen et al., 2023), and ultimately to be transformed into organic matter and exported into deeper water layers below the euphotic zone. With this, we follow the bottom-up principle which has been previously applied within the BUS to outline new production rates and carbon export fluxes on the basis of upwelling source water mass inventories (Eppley and Peterson, 1979; Messié et al., 2009; Waldron et al., 2009). With a constant Redfield-ratio of 106:16 (C:N), the impact of biology was estimated by subtracting the amount of nitrate-associated carbon from the source water’s initial DIC concentration, and adding the associated release of total alkalinity (TA) to the original source water TA. Additionally, we considered the surface warming effect of the upwelling source waters to account for the decrease in the CO2 solubility of seawater and its impact on pCO2 by using the locally measured sea surface temperatures and salinities (SST, SSS) instead of those of the source water masses. In order to quantify the effect of bottom trawling on the sink and source functionalities of the BUS, we translated the increase in pCO2 into equivalent changes in CO2 fluxes. Therefore, we used the offshore increase in pCO2 through bottom trawling to add to the basin-scale average pCO2 as estimated from ordinary kriging interpolations (see section 3.5) for calculating the annual CO2 fluxes within both subsystems. Lastly, we shed light on changes in new production rates on the basis of nutrients and carbon that were released after bottom trawling and upwelled into the surface region, and by taking note of the efficiency at which these nutrients and carbon are being recaptured into biomass. A schematic overview of the bottom-up approach is given in Supplementary Figure 1.
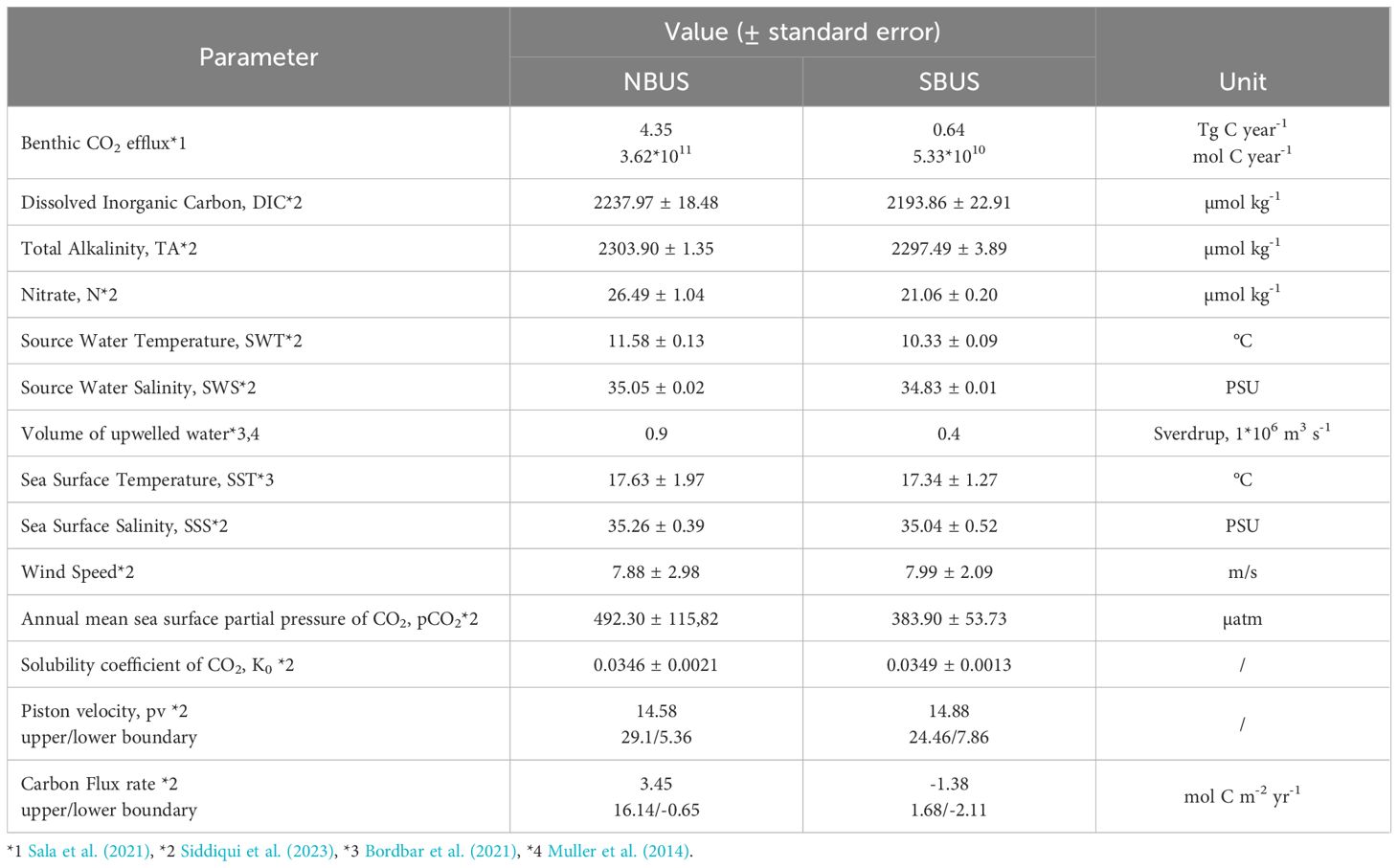
Table 1. Parameters used for simulating impacts of bottom trawling, including the benthic CO2 efflux, average hydrographic conditions of source water masses, CO2 exchange coefficients and flux rates for the northern and southern Benguela Upwelling System.
3.2 Surface sediment characteristics
We used undisturbed sediment core samples that were collected on board RV Africana (AFR258), Meteor (M48/2, M76/2, M103/1) and Maria S. Merian (MSM17/3) (see Table 2) with a multicorer (Oktopus Kiel) to outline the stoichiometric carbon to nutrient ratios within the top sediment layer. The sampler was equipped with acrylic tubes of 10 cm diameter and 60 cm length, and was used to retrieve sediment cores that were sliced in 1cm intervals, kept frozen under -20°C and freeze-dried in the home laboratory, and analyzed for concentrations of total nitrogen and organic carbon with an Elemental Analyzer (Carlo Erba NA 1500).
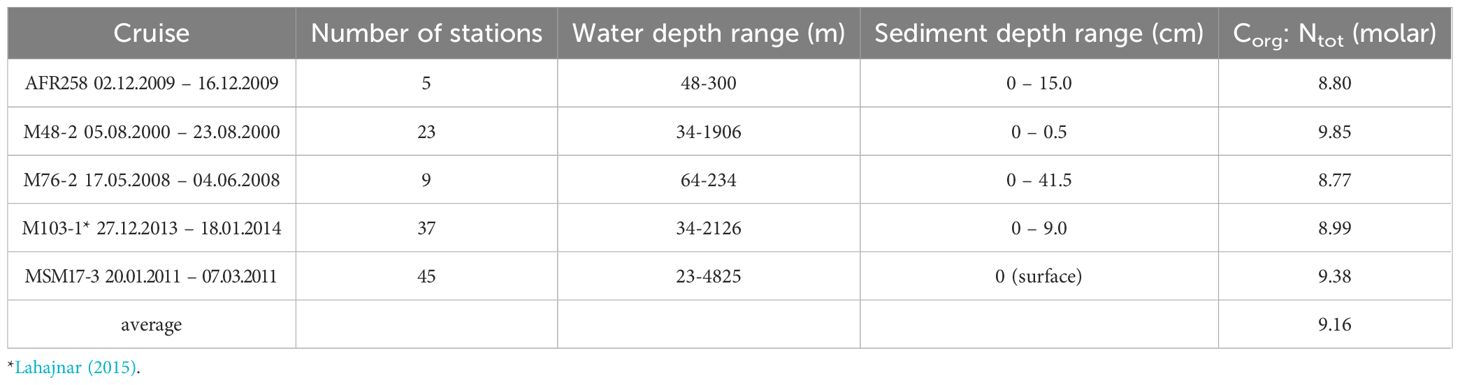
Table 2. Average stoichiometric carbon to nutrient ratios of multicore samples collected during various cruises to the northern Benguela Upwelling System.
3.3 Benthic CO2 efflux
We used published global benthic CO2 efflux estimates of bottom trawling and dredging fishing practices (Sala et al., 2021). The benthic CO2 efflux was derived from the amount of carbon stored within the top layer of the sediment as based on recently published global carbon stocks (Atwood et al., 2020), and the labile fraction of carbon that is remineralized and released at the sediment-water interface after the occurrence of bottom trawling. The latter is inferred from the sediment type and trawl activity as estimated from automatic identification systems (AIS) data that was used for tracking the fishing trawlers’ distance, speed, and the applied gear type with its corresponding penetration depth. The sediment type was thereby used as a proxy for the labile carbon fraction due to its impact on organic matter preservation and remineralization. For each sediment type (coarse, sandy, fine and biogenic), the proportion of labile organic carbon was assigned using literature values, amounting e.g., to 0.04 for sandy sediments and to 0.7 for muds, silts or biogenic sediments. The CO2 efflux was then modelled using an average first-order reaction constant k for the degradation process as a function of oceanic region (Atlantic = 1.00).
Hereby, several studies outlined the underlying first-order reaction constant k as applied by Sala et al. (2021) to be overestimated, since it based on a reactivity value for highly reactive, fresh organic carbon that has recently been transferred to the sediment surface, and applied to a bulk of less reactive compounds within deeper sediment layers.
To shed light on the applicability of k by Sala et al. (2021) for the BUS, we took into account the degradation index (DI) inferred from amino acids of sediment samples collected during cruise MSM17/3. According to Dauwe et al. (1999), the DI derived from amino acids of particulate matter samples can be directly linked to the degradation rate, allowing to assess the quality of organic matter and first-order reaction constants. We estimated an average DI of 0.12 (standard deviation ±0.1) for surface sediment samples collected at water depths between 200 – 1000 m (in line with the bottom trawled area), with 0.36 as the highest value. Given the linear correlation between DI and k, these values imply the reactivity constant k to fall within a spectrum of 0.1 – 1 (Dauwe et al., 1999), where k=1 is used by Sala et al. (2021) to infer the benthic CO2 efflux of the BUS. Hereby, higher values are indicative for well-preserved, fresh organic matter and lower values implying less well-preserved organic matter and faster degradation process.
3.4 Physical and biogeochemical water column characteristics
We used data on upwelling source water mass compositions within the NBUS and SBUS taken from Siddiqui et al. (2023). This dataset is based on water samples collected with multiple CTD/Rosette systems during various cruises to the BUS which we listed in Supplementary Table 1, including data from the Global Ocean Data Analysis Project version 2.2020 (GLODAPv2_2020). Water samples were analyzed for temperature, salinity, oxygen, DIC, total alkalinity (TA) and nutrients (phosphate P, nitrate N) following the methods described in Emeis et al. (2018) and Flohr et al. (2014). The upwelling waters, SACW and ESACW, were defined using potential temperatures (theta, ) and definitions by Mohrholz et al. (2014) following Equations 1, 2, respectively:
Additional samples for suspended matter were retrieved with the multiple CTD/Rosette system during Meteor cruise M153, Sonne cruise SO283 and SO285. The filtration volume of sea water on pre-combusted (450°C) and tarred glass fibre filters (WHATMAN GF/F, ~0.7 μm, 47 mm diameter) varied between 5 and 30 L. The filtration was stopped when filters were satisfactorily covered. After filtration, the samples were rinsed with deionised water to remove sea salt and subsequently dried in the ship’s dry oven at 40 °C for 48 hours prior to analysis of total nitrogen and organic carbon.
3.5 Air-sea interface conditions
The analysis and quantification of air-sea CO2 fluxes was based on data taken from Siddiqui et al. (2023), comprising of continuous underway measurements collected between 2008 and 2019 according to methods described in Emeis et al. (2018), and additional quality-controlled measurements from the Surface Ocean CO2 Atlas (SOCAT) v2020 (Bakker et al., 2016). Data on the sea surface CO2 partial pressure (pCO2) were normalized to the reference year 2020 (Takahashi et al., 2009, 2014) and spatially interpolated on a 0.1° x 0.1° grid using ordinary kriging performed with the R automap package (Hiemstra et al., 2009). Annual variogram models applied during the ordinary kriging procedure, together with autocorrelation length scales that were used to correct for spatial autocorrelation following Landschützer et al. (2014), can be found in Siddiqui et al. (2023).
Carbon flux rates (FCO2) were determined using the partial pressure at the sea surface (pCO2,sw) and of the atmosphere (pCO2,at) following Equation 3:
with K0 as the solubility coefficient of CO2 (Weiss, 1974) and k as the gas transfer velocity of CO2 (Wanninkhof, 2014), calculated using Equation 4:
with Sc as the Schmidt number of CO2 in seawater, 660 as Sc at 20°C water temperature, and u referring to wind speed (m s-1) at 10 m above sea surface. Sc was determined using shipboard data on wind speed, sea surface temperature (SST) and salinity (SSS) that were spatially interpolated using the ordinary kriging procedures as outlined for pCO2. The annual mean CO2 exchange coefficients, sea surface pCO2 and flux rates for the northern and southern Benguela Upwelling System that we used in this study are presented in Table 1.
4 Results and discussion
4.1 Effect of bottom trawling on benthic fluxes and upwelling source waters
4.1.1 Benthic fluxes
According to published estimates across the northern and southern upwelling region of the BUS (Sala et al., 2021), the amount of CO2 released during the remineralization of resuspended sediment after bottom trawling added up to 4.35 and 0.64 Tg C year-1 (3.62*1011 and 5.33*1010 mol C year-1), respectively. Hereby, the CO2 release was highest from regions with water depths of 200-400 m, contributing over 50% to the trawl-induced benthic CO2 efflux, adding DIC into bottom waters overlying the sediment surface. To take note of the associated release of nutrients like nitrate due to bottom trawling, we applied the average molar carbon to nitrate (C/N) ratio (9.16) found within the top layer of the sediment as derived from multicore samples across the NBUS region (Table 2). This resulted in 3.95*1010 mol N year-1 (= 3.62*1011 mol C year-1/9.16) for the NBUS and 5.82*109 mol N year-1 for the SBUS, respectively, that are assumed to be released during the remineralization of resuspended sediment into bottom waters.
Hereby, a preferential remineralization of nitrogen containing compounds should have led to an increase in the C/N ratio of suspended organic matter (SPM) in the water column. SPM collected along the continental shelf break at water-depths between 200 and 1000 m showed C/N ratios between 2.7 and 15.0 (see Supplementary Figure 2). Apart from two exceptions with a C/N ratio above 10, the maximum C/N ratios increase with a decreasing distance to the surface sediments, reflecting an increased contribution of resuspended sediments to the SPM. With C/N ratios of SPM hardly exceeding 9.6, there appears to be no preferential remineralization of nitrogen containing compounds that would have otherwise significantly increased C/N ratios within the SPM.
One of the factors controlling the benthic CO2 efflux is the reactivity of organic carbon in sediments. Hereby, high reactivity values, as applied by Sala et al. (2021) for the BUS, can be traced back to environmental factors limiting OM degradation due to enhanced vertical or lateral transfer of OM to the seafloor, or due to factors impeding OM degradation directly, such as diminished oxygen availability (Arndt et al., 2013). In case of the BUS, the presence of OMZs could be responsible for highly reactive, fresh OM settlement at the sediment surface as implicated by k values derived from the sediment samples. Together with other studies reporting intermediate to highest values for organic matter reactivity in the BUS (Arndt et al., 2013; Pika et al., 2023; Xu et al., 2023), the applicability of k = 1, and amount of the trawl-induced benthic CO2 efflux, appear plausible. However, the spatial variability in OM reactivity as seen in our DI estimates and seasonal variability in poorly oxygenated SACW inflow exerting control over the OMZ highlights the need to refine the reaction constant on a higher spatio-temporal resolution.
A further concern with the approach of Sala et al. (2021) is that both natural and bottom trawl-induced remineralization of organic carbon across the sediment-water interface are being accounted for in calculating the trawl-related benthic CO2 efflux, therefore overestimating the CO2 release after bottom trawling [Hiddink et al., (2023)]. In this regard, the bottom trawl-induced nitrate efflux of 3.95*1010 mol N year-1 in the NBUS corresponds to more than ten times the diffusive benthic nitrate efflux of 3.5 *109 mol N year-1 as calculated by Neumann et al. (2016) based on pore water gradients across the inner-shelf mud belt off Namibia. Thus, the amount of nutrients released after bottom trawling seems to be higher than the natural benthic efflux from inner-shelf sediment that is profoundly rich in fresh organic matter, which, together with the rise in bottom water DIC concentrations, highlights the intrusive nature of bottom trawling on the benthic-pelagic coupling. Hereby, it should be noted that we only accounted for the nutrients associated with the remineralization of resuspended organic matter, which we derived via applying the C:N ratio to the benthic CO2 efflux by Sala et al. (2021). Hence, we neglected the dissolved nutrients within pore waters that could be released during bottom trawling as shown by a study in the Gulf of Lion, where 2-5 orders of magnitude more nutrients were released from pore waters of muddy sediments after trawling as compared to the natural benthic efflux (Durrieu de Madron et al., 2005).
However, results of other studies displayed the trawl-released nutrients to only affect nutrient availability on a short timeframe without long-term consequences for the overall nutrient budget (Trimmer et al., 2005), while others noted elevated nutrient concentrations to be detectable even within a 100 m distance to the trawl track (Bradshaw et al., 2021). These partially contradicting outcomes are likely site-specific, with sediments being subject to various factors governing their response to trawl-induced disturbances, such as sediment type (cohesive vs. non-cohesive) (Palanques et al., 2014; Hale et al., 2017), trawl frequency and gear type (Eigaard et al., 2016; Depestele et al., 2019). Hereby, studies on trawl-induced carbon and nutrient releases found remineralization of particulate organic matter at sites with muddy sediments to be either enhanced by the deposition of fresh organic matter on a nutrient-deprived trawled area (Paradis et al., 2019), or decreased with the degradation of sedimentary habitats and depletion in organic matter content (Pusceddu et al., 2014). Further contradicting findings were reported for sedimentary organic carbon concentrations, which either showed an increase (Pusceddu et al., 2005; Palanques et al., 2014; Sciberras et al., 2016) or decrease (Mayer et al., 1991; Hale et al., 2017; Tiano et al., 2019) in the aftermath of bottom trawling, as well as for organic matter degradation pathways to be increased (Polymenakou et al., 2005; Paradis et al., 2019) or decreased (Tiano et al., 2019), with no clear effects on sedimentary organic carbon reported along the Southern Benguela Upwelling System (Atkinson et al., 2011). In addition, remineralization of organic matter after trawling events was found to be enhanced if resuspended from low oxygenated sediment environments into well oxygenated bottom waters (Sciberras et al., 2016; Bradshaw et al., 2021). In this context, oxygen-depleted zones within the BUS might limit remineralization of resuspended sediments which could reduce the effect of bottom trawling on benthic carbon and nutrient fluxes, or might be promoted by trawl-induced OM oxidation, especially with the entrainment of oxygen-depleted SACW into the NBUS shelf system (Mohrholz et al., 2008).
4.1.2 Upwelling source waters
On the basis of the published benthic CO2 efflux (Sala et al., 2021), we estimated the increase of DIC and nutrients within the upwelling source waters due to bottom trawling by dividing the release of sedimentary carbon and nitrate (in mol year-1) by the annual volume of waters (in Sverdrup, Sv) that are being upwelled within the NBUS and SBUS region. With an upwelling volume of 0.9 Sv (= 106 m3 s-1) for the NBUS (Muller et al., 2014; Bordbar et al., 2021) and 0.4 Sv for the SBUS (Bordbar et al., 2021), we estimated 2.83*1013 and 1.26*1013 m3 year-1 of water masses to be upwelled, respectively. Dividing the benthic efflux of 3.62*1011 and 5.33*1010 mol C year-1 of the NBUS and SBUS by the volume of upwelling waters (m3 year-1) resulted in a DIC enrichment of 12.8 and 4.3 µmol kg-1 per year, respectively. Nitrate concentrations would be enhanced by 1.39 and 0.47 µmol kg-1 year-1 in the NBUS and SBUS, respectively, making up ~5 and ~2% of the corresponding average source water nitrate concentrations of 26.49 ± 1.04 and 21.06 ± 0.2 µmol kg-1 year-1 (Table 1).
Although our average source water mass DIC concentration might already be influenced by bottom trawling activities, we add the trawl-induced release of CO2 and nutrients to our respective DIC and N concentration of the upwelling source water masses, as bottom trawling is an ongoing fishing practice where carbon and nutrients are being released from the sediment-water interface.
4.2 Biological response and feedback to air-sea gas exchange
To estimate the impact of bottom trawling on the biological productivity, we applied the bottom-up approach to calculate new production rates for each subsystem within the BUS based on respective upwelling volumes and benthic nitrate concentrations released into the upwelling source waters after bottom trawling (NBUS: 1.39 µmol N kg-1, SBUS: 0.47 µmol N kg-1). Quantifying this amount of N available within the euphotic zone and translating it into equivalent grams of C using the Redfield-ratio (6.6) resulted in new production rates of 3.14 and 0.47 Tg C year-1 for the NBUS and SBUS, respectively. Compared to new production rates based on average DIC and N concentrations of the source water masses (NBUS: 59.83 Tg C year-1, SBUS: 21.14 Tg C year-1), the release of N in the aftermath of bottom trawling could increase new production by 5.25% in the NBUS and 2.22% in the SBUS.
However, compared to the corresponding benthic CO2 efflux of 4.35 and 0.64 Tg C year-1 in the north and south, only 2/3 of the benthic CO2 emissions could be recaptured and assimilated into organic matter by benthic nutrients as a result of the difference in C:N ratios between the sediment (Table 2) and surface biomass (Redfield, 6.6). Although this could support the overall productivity of the system, the remaining 1/3 of the benthic CO2 emissions could lead to an ongoing enrichment of DIC and rise of pCO2 in the surface region. To elaborate the latter, we estimated the impact of enhanced DIC and nutrient concentrations through bottom trawling on the air-sea gas exchange through sea surface pCO2 simulations using CO2SYS. We therefore compared our results with the measured and simulated pCO2 of the NBUS and SBUS coastal and offshore region as presented in Siddiqui et al. (2023).
According to Siddiqui et al. (2023), highest pCO2 prevails in the coastal region (Figures 3A, C) where the upwelling of carbon-rich waters fosters the outgassing of CO2, as also indicated by elevated pCO2 measurements. Hereby, the average modelled pCO2 in the upwelled water lies within the upper range of the nearshore measured pCO2 (NBUS:294 – 1012 µatm, SBUS: 334 – 610 µatm). This implies biologically-mediated nutrient consumption and fixation of DIC into biomass to occur simultaneously with coastal upwelling, as also indicated by increased chlorophyll concentrations that have been observed by satellites along a narrow belt across the coastal region within the BUS (Weeks et al., 2006; Demarcq et al., 2007; Lamont et al., 2019). In addition, pCO2 measurements were taken during different times of the year, covering (non-) upwelling seasons and variable intensities of solar radiation that could have led to variabilities in the warming of upwelling waters and its effect on sea water CO2 solubility. The latter is addressed by considering the effect of the biologically-mediated nutrient consumption on pCO2 within the offshore region, while also accounting for the warming of upwelled waters in a subsequent stage. This caused a decrease in pCO2 with respect to the coast due to degassing and the biologically-mediated uptake of CO2 within the offshore flowing waters (Figures 3B, D). Hereby, the simulated pCO2 falls into the spectrum of measured estimates in cases when the surface warming effect is being accounted for. Depending on its strength, pCO2 can be drawn below or above the atmospheric pCO2, which, in the end, controls the regional CO2 sink and source functionality of the two subsystems (Siddiqui et al., 2023).
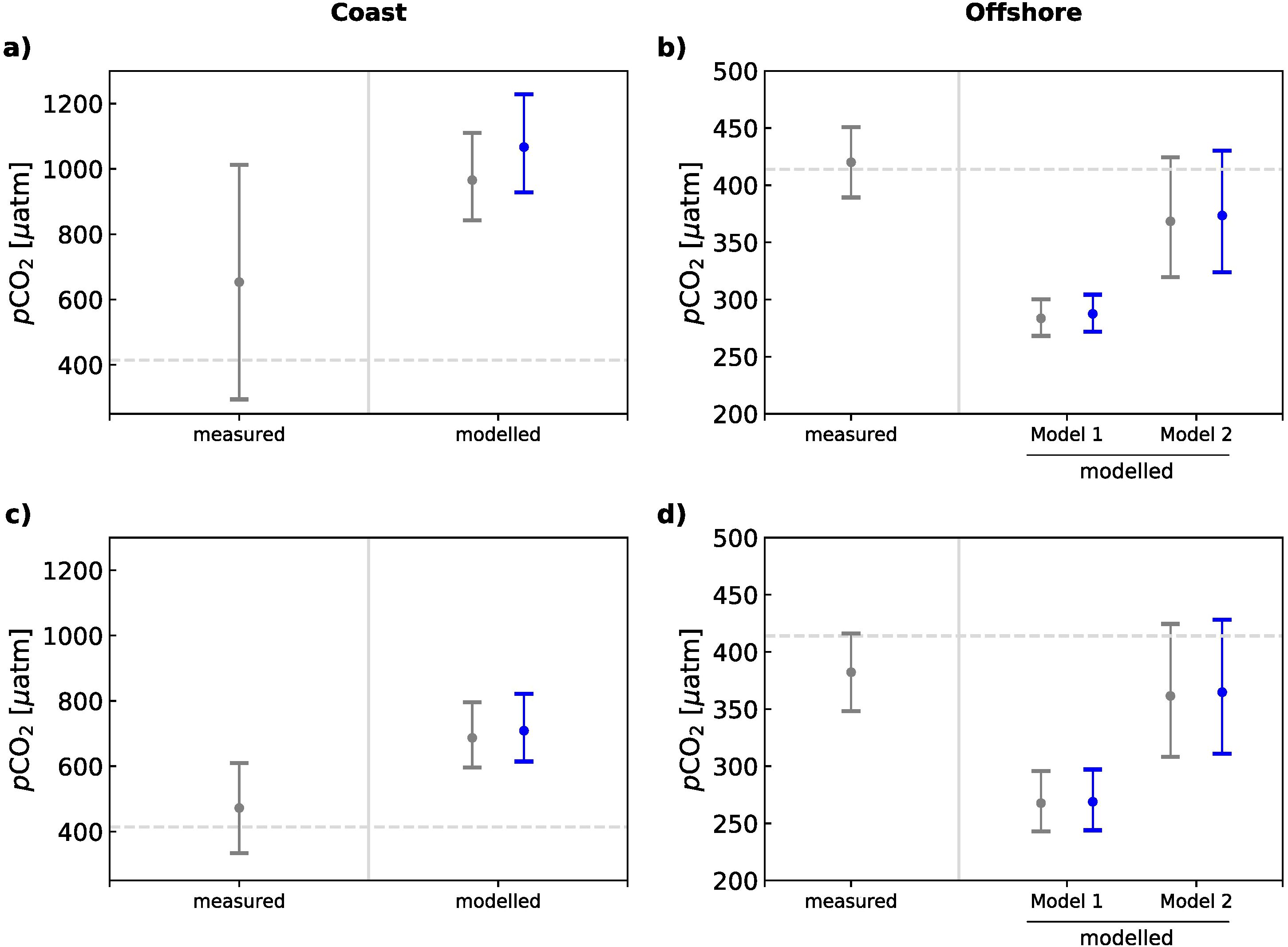
Figure 3. Simulations of the sea surface partial pressure of CO2 with CO2SYS (Lewis and Wallace, 1998; Humphreys et al., 2020) as estimated from bottom trawling activities within the Benguela Upwelling System. Measured sea surface pCO2 (in µatm) normalized to reference year 2020 (Takahashi et al., 2009, 2014) and modelled pCO2 during coastal upwelling and nitrate (N) consumption through biologically-mediated CO2 uptake across the shelf and offshore boundary for the (A, B) NBUS and (C, D) SBUS, adapted from Siddiqui et al. (2023), licensed under CC BY 4.0. The simulated pCO2 after biological consumption is shown without (Model 1) and with (Model 2) the surface warming effect. The effect of bottom trawling on pCO2 as simulated in this study is shown in blue. The grey dashed line mirrors atmospheric pCO2 of the reference year 2020 based on Mauna Loa records (414 µatm). Sea surface pCO2 concentrations below (above) the atmospheric level indicate a source (sink) of atmospheric CO2. The uncertainties in measured pCO2 are presented as the standard deviation, whereas uncertainties in the modelled pCO2 are based on the standard error of the average source water mass characteristics.
In order to account for the impact of bottom trawling, we repeated the simulations of pCO2 for the coast and offshore regions by adding the bottom trawl-induced efflux of DIC and nutrients as previously outlined to the given source water concentrations. The effect of bottom trawling thereby led to an additional increase in pCO2 at the coast of +100 and +20 µatm in the NBUS and SBUS, respectively, that gradually decreased towards offshore, where pCO2 was merely raised by 5 µatm in the north and 3 µatm in the south.
Given the increase in pCO2 through bottom trawling by 5 µatm in the NBUS, the corresponding annual CO2 flux would increase by ~1 Tg C year-1, resulting in 17.13 (-2.64 – 77.25) Tg C year-1 that would be emitted into the atmosphere. In the SBUS, the bottom trawl-induced rise in pCO2 would offset the annual CO2 flux by 0.3 Tg C year-1, leading to a comparatively lower CO2 uptake of -2.79 (-4.55 – 4.25) Tg C year-1. Thus, an additional release of sedimentary carbon into upwelling source waters could on average reduce the SBUS’s sink functionality of atmospheric carbon by ~10%, and increase the rate of outgassing by 6.5% in the NBUS.
These values are in the order of CO2 emissions by land use and land cover changes as ascribed to the AFOLU (agriculture, forestry, and other land use) sector for Namibia, which takes into account carbon stocks of coastal reservoirs like mangrove forests, tidal marshes, and seagrass meadows (“blue carbon”) (Rixen et al., 2023). The bottom trawl-induced release of CO2 into the atmosphere within the BUS (~1Tg C year-1) corresponds to ~3% of the CO2 currently stored by AFOLU (-30.6 Tg C year-1) (Rixen et al., 2023), showing the scale to which bottom trawling could potentially alter CO2 emissions in coastal settings.
Hence, the efficiency of the biological carbon pump in sequestering and storing atmospheric CO2 is likely affected by bottom trawling: On the one side, such fishing practices impair the sink function of the BUS by reactivating ~5 Tg C year-1 previously stored in the sediment of which 2/3 may be transformed back into organic matter. On the other side, the trawl-induced release of sedimentary CO2 into upwelling source water masses can potentially foster an additional outgassing of ~1 Tg C year-1 into the atmosphere. Hereby, our estimations are representative for an upper limit, assuming the entirety of the benthic CO2 efflux and associated nutrients to be upwelled into the surface region and assimilated into organic matter, while neglecting any processes hampering the biologically-mediated CO2 uptake, such as light and iron limitations or water column denitrification. The rise in pCO2 through bottom trawling is thereby related to the efficiency at which benthic nutrients and DIC are recaptured and assimilated into organic matter after being released from the sediment and upwelled into the surface region. Thus, using a constant stoichiometric carbon to nutrient ratio further disregards any variability in nutrient utilization and remineralization, which, in the end, is a pivotal factor that constitutes the recapture efficiency of the bottom trawl-induced CO2 efflux. Additionally, the amount of the benthic CO2 efflux and associated nutrients as elucidated for the BUS are subject to uncertainty because underlying calculations of the labile carbon fraction and remineralization were based on basin-scale average values that curtailed spatial variabilities within the BUS (Sala et al., 2021; Epstein et al., 2022). In this regard, the remineralization potential is related to oxygen availability, with oxygen-rich environments likely promoting organic matter degradation and oxygen deficiencies limiting remineralization (Sciberras et al., 2016; Bradshaw et al., 2021). Hereby, a potential expansion of Oxygen Minimum Zones (OMZs) could add further pressure on benthic ecosystems under the influence of bottom trawling by impeding the recovery potential and protection of benthic flora and fauna, which would otherwise have a stabilizing effect on the sediment and increase its resistance to physical disruptions (Roberts et al., 2017). Hence, in addition to monitoring of hydrographic changes and benthic ecosystem responses, higher spatially resolved data is needed to account for heterogeneity in sedimentary carbon characteristics and to refine trawl-induced benthic carbon and nutrient effluxes within the BUS.
5 Conclusion
In this study, we examined how coastal sediments exposed to trawl-induced disturbances may impact the biological carbon pump efficiency of the Benguela Upwelling System by applying the bottom-up approach to simulate benthic C and N pathways from the bottom trawled seafloor to the sunlit surface via coastal upwelling of source water masses encompassing the bottom shelf region. Using shipboard data on sea surface and water column characteristics and published benthic CO2 emission estimates from bottom-trawled sediments, we estimated a release of ~5 Tg C year-1 from the trawled sediment into bottom waters within the BUS, together with nutrient inputs that could enhance source water nitrate concentrations by ~2-5%. Despite of supporting the biological productivity, benthic nitrate inputs merely lead to 2/3 of CO2 released from bottom trawling to be recaptured into organic matter due to stoichiometric C:N ratio differences between the sediment (~9) and surface biomass (Redfield, 6.6), impeding the biological carbon pump efficiency in sequestering atmospheric CO2 by ~1.3 Tg C year-1. Hence, our results suggests that C:N stoichiometry should be considered when determining how trawl-induced disturbances at the seafloor may affect carbon and nutrient cycling in coastal upwelling ecosystems. Hereby, the heterogeneity in sediment organic matter reactivity and site-specific conditions such as sediment type and hydrographic changes affecting OM remineralization give further incentive to refine sedimentary and pelagic C and N variabilities in order to better understand effects of trawl-induced sediment resuspension on the biological carbon pump.
Data availability statement
The raw data supporting the conclusions of this article will be made available by the authors, without undue reservation. We have adopted the python code previously published by Siddiqui et al. (2023) for sea surface pCO2 simulations (thermally and non-thermally controlled pCO2), CO2 flux calculations and new production calculations. These codes incorporate all necessary equations and parameters for reproducing the output of this study. The python code is available in Figshare under the accession code doi:10.6084/m9.figshare.21436494.
Author contributions
CS: Conceptualization, Investigation, Visualization, Writing – original draft, Writing – review & editing. TR: Conceptualization, Supervision, Writing – review & editing. NL: Investigation, Writing – review & editing. TL: Writing – review & editing. AvdP: Writing – review & editing.
Funding
The author(s) declare financial support was received for the research, authorship, and/or publication of this article. The German Federal Ministry of Education and Research (BMBF) funded the research under the grant no. 03F0797A (ZMT) and 03F0797C (Universität Hamburg).
Acknowledgments
We would like to thank the scientists, technicians, captains and crew members for their support and assistance during the cruises that were embedded in this study. F. Hüge and M. Birkicht are thanked for their support in the laboratories. The Surface Ocean CO2 Atlas (SOCAT) is an international effort, endorsed by the International Ocean Carbon Coordination Project (IOCCP), the Surface Ocean Lower Atmosphere Study (SOLAS) and the Integrated Marine Biosphere Research (IMBeR) program, to deliver a uniformly quality-controlled surface ocean CO2 database. The many researchers and funding agencies responsible for the collection of data and quality control are thanked for their contribution to SOCAT. We also thank P. Wessels and W.H.F. Smith for providing the Generic Mapping Tools (GMT).
Conflict of interest
The authors declare that the research was conducted in the absence of any commercial or financial relationships that could be construed as a potential conflict of interest.
The author(s) declared that they were an editorial board member of Frontiers, at the time of submission. This had no impact on the peer review process and the final decision.
Publisher’s note
All claims expressed in this article are solely those of the authors and do not necessarily represent those of their affiliated organizations, or those of the publisher, the editors and the reviewers. Any product that may be evaluated in this article, or claim that may be made by its manufacturer, is not guaranteed or endorsed by the publisher.
Supplementary material
The Supplementary Material for this article can be found online at: https://www.frontiersin.org/articles/10.3389/fmars.2024.1387121/full#supplementary-material
References
Almroth-Rosell E., Tengberg A., Andersson S., Apler A., Hall P. (2012). Effects of simulated natural and massive resuspension on benthic oxygen, nutrient and dissolved inorganic carbon fluxes in Loch Creran, Scotland. J. Sea Res. 72, 38–48. doi: 10.1016/j.seares.2012.04.012
Amoroso R. O., Pitcher C.R., Rijnsdorp A. D., McConnaughey R. A., Parma A. M., Suuronen P., et al. (2018). Bottom trawl fishing footprints on the world’s continental shelves. Proc. Natl. Acad. Sci. 115, E10275. doi: 10.1073/pnas.1802379115
Arndt S., Jørgensen B. B., LaRowe D. E., Middelburg J. J., Pancost R. D., Regnier P. (2013). Quantifying the degradation of organic matter in marine sediments: A review and synthesis. Earth-Sci. Rev. 123, 53–86. doi: 10.1016/j.earscirev.2013.02.008
Atkinson L. J., Field J. G., Hutchings L. (2011). Effects of demersal trawling along the west coast of southern Africa: multivariate analysis of benthic assemblages. Mar. Ecol. Prog. Ser. 430, 241–255. doi: 10.3354/meps08956
Atwood T. B., Witt A., Mayorga J., Hammill E., Sala E. (2020). Global patterns in marine sediment carbon stocks. Front. Mar. Sci. 7. doi: 10.3389/fmars.2020.00165
Avelar S., van der Voort T., Eglinton T. (2017). Relevance of carbon stocks of marine sediments for national greenhouse gas inventories of maritime nations. Carbon Balance Manage. 12. doi: 10.1186/s13021-017-0077-x
Bakker D., Pfeil B., Landa C., Metzl N., O’Brien K., Olsen A., et al. (2016). A multi-decade record of high-quality fCO2 data in version 3 of the Surface Ocean CO2 Atlas (SOCAT). Earth System Science Data 8, 313–413. doi: 10.5194/essd-8-383-2016
Bordbar M. H., Mohrholz V., Schmidt M. (2021). The relation of wind-driven coastal and offshore upwelling in the benguela upwelling system. J. Phys. Oceanogr. 51, 3117–3133. doi: 10.1175/JPO-D-20-0297.1
Boyd P., Claustre H., Levy M., Siegel D., Weber T. (2019). Multi-faceted particle pumps drive carbon sequestration in the ocean. Nature 568, 327–335. doi: 10.1038/s41586-019-1098-2
PubMed Abstract | PubMed Abstract | Crossref Full Text | Google Scholar
Bradshaw C., Jakobsson M., Brüchert V., Bonaglia S., Mörth C.-M., Muchowski J., et al. (2021). Physical disturbance by bottom trawling suspends particulate matter and alters biogeochemical processes on and near the seafloor. Front. Mar. Sci. 8. doi: 10.3389/fmars.2021.683331
Breimann S., O’Neill F., Summerbell K., Mayor D. (2021). Quantifying the resuspension of nutrients and sediment by demersal trawling. Continent. Shelf Res. 233, 104628. doi: 10.1016/j.csr.2021.104628
Bruni E. T., Blattmann T. M., Haghipour N., Louw D., Lever M., Eglinton T. I. (2022). Sedimentary hydrodynamic processes under low-oxygen conditions: implications for past, present, and future oceans. Front. Earth Sci. 10. doi: 10.3389/feart.2022.886395
Chavez F., Toggweiler J. R. (1995). “Physical estimates of global new production: The upwelling contribution,” in Upwelling in the ocean: Modern processes and ancient records. Eds. Summerhayes C. P., Emeis K.-C., Angel M. V., Smith R. L., Zeitschel B. (Wiley & Sons, Chichester).
Christianson A. B., Cabré A., Bernal B., Baez S. K., Leung S., Pérez-Porro A., et al. (2022). The promise of blue carbon climate solutions: where the science supports ocean-climate policy. Front. Mar. Sci. 9. doi: 10.3389/fmars.2022.851448
Churchill J. H. (1989). The effect of commercial trawling on sediment resuspension and transport over the Middle Atlantic Bight continental shelf. Continent. Shelf Res. 9, 841–865. doi: 10.1016/0278-4343(89)90016-2
Dauwe B., Middelburg J. J., Herman P. M.J., Heip C. H.R. (1999). Linking diagenetic alteration of amino acids and bulk organic matter reactivity. Limnol. Oceanogr. 44, 1809–1814. doi: 10.4319/lo.1999.44.7.1809
De Borger E., Tiano J., Braeckman U., Rijnsdorp A. D., Soetaert K. (2021). Impact of bottom trawling on sediment biogeochemistry: a modelling approach. Biogeosciences 18, 2539–2557. doi: 10.5194/bg-18-2539-2021
Demarcq H., Barlow R., Hutchings L. (2007). Application of a chlorophyll index derived from satellite data to investigate the variability of phytoplankton in the Benguela ecosystem. Afr. J. Mar. Sci. - AFR J. Mar. Sci. 29, 271–282. doi: 10.2989/AJMS.2007.29.2.11.194
Depestele J., Degrendele K., Esmaeili M., Ivanović A., Kröger S., O’Neill F. G., et al. (2019). Comparison of mechanical disturbance in soft sediments due to tickler-chain SumWing trawl vs. electro-fitted PulseWing trawl. ICES J. Mar. Sci. 76, 312–329. doi: 10.1093/icesjms/fsy124
Dounas C., Davies I., Triantafyllou G., Koulouri P., Petihakis G., Arvanitidis C., et al. (2007). Large-scale impacts of bottom trawling on shelf primary productivity. Continent. Shelf Res. 27, 2198–2210. doi: 10.1016/j.csr.2007.05.006
Durrieu de Madron X., Ferré B., Le Corre G., Grenz C., Conan P., Pujo-Pay M., et al. (2005). Trawling-induced resuspension and dispersal of muddy sediments and dissolved elements in the Gulf of Lion (NW Mediterranean). Continent. Shelf Res. 25, 2387–2409. doi: 10.1016/j.csr.2005.08.002
Eigaard O. R., Bastardie F., Breen M., Dinesen G. E., Hintzen N. T., Laffargue P., et al. (2016). Estimating seabed pressure from demersal trawls, seines, and dredges based on gear design and dimensions. ICES J. Mar. Sci. 73, i27–i43. doi: 10.1093/icesjms/fsw116
Emeis K., Brüchert V., Currie B., Endler R., Ferdelman T., Kiessling A., et al. (2004). Shallow gas in shelf sediments of the Namibian coastal upwelling ecosystem. Continent. Shelf Res. 24, 627–642. doi: 10.1016/j.csr.2004.01.007
Emeis K., Eggert A., Flohr A., Lahajnar N., Nausch G., Neumann A., et al. (2018). Biogeochemical processes and turnover rates in the Northern Benguela Upwelling System. J. Mar. Syst. 188, 63–80. doi: 10.1016/j.jmarsys.2017.10.001
Eppley R. W., Peterson B. J. (1979). Particulate organic matter flux and planktonic new production in the deep ocean. Nature 282, 677–680. doi: 10.1038/282677a0
Epstein G., Middelburg J., Hawkins J., Norris C., Roberts C. (2022). The impact of mobile demersal fishing on carbon storage in seabed sediments. Global Change Biol. 28, 2875–2894. doi: 10.1111/gcb.16105
Finke G., Gee K., Kreiner A., Amunyela M., Braby R. (2020). Namibia’s way to Marine Spatial Planning – Using existing practices or instigating its own approach? Mar. Policy 121, 104107. doi: 10.1016/j.marpol.2020.104107
PubMed Abstract | PubMed Abstract | Crossref Full Text | Google Scholar
Flohr A., van der Plas A., Emeis K., Mohrholz V., Rixen T. (2014). Spatio-temporal patterns of C: N: P ratios in the northern Benguela upwelling regime. Biogeosciences 11, 885–897. doi: 10.5194/bg-11-885-2014
Gordon A., Weiss R., Smethie W. M., Warner M. J. (1992). Thermocline and intermediate water communication between the south Atlantic and Indian oceans. J. Geophys. Res. 97, 7223–7240. doi: 10.1029/92JC00485
Hale R., Godbold J. A., Sciberras M., Dwight J., Wood C., Hiddink J. G., et al. (2017). Mediation of macronutrients and carbon by post-disturbance shelf sea sediment communities. Biogeochemistry 135, 121–133. doi: 10.1007/s10533-017-0350-9
PubMed Abstract | PubMed Abstract | Crossref Full Text | Google Scholar
Hiddink J., van de Velde S., McConnaughey R., De Borger E., Tiano J., Kaiser M., et al. (2023). Quantifying the carbon benefits of ending bottom trawling. Nature 617, E1–E2. doi: 10.1038/s41586-023-06014-7
PubMed Abstract | PubMed Abstract | Crossref Full Text | Google Scholar
Hiemstra P., Pebesma E., Twenhöfel C., Heuvelink G. (2009). Real-time automatic interpolation of ambient gamma dose rates from the Dutch Radioactivity Monitoring Network. Comput. Geosci. 35, 1711–1721. doi: 10.1016/j.cageo.2008.10.011
Hilmi N., Chami R., Sutherland M. D., Hall-Spencer J. M., Lebleu L., Benitez M. B., et al. (2021). The role of blue carbon in climate change mitigation and carbon stock conservation. Front. Climate 3. doi: 10.3389/fclim.2021.710546
Howard J., Sutton-Grier A., Herr D., Kleypas J., Landis E., McLeod E., et al. (2017). Clarifying the role of coastal and marine systems in climate mitigation. Front. Ecol. Environ. 15, 42–50. doi: 10.1002/fee.1451
Humphreys M. P., Gregor L., Pierrot D., van Heuven S. M. A. C., Lewis E., Wallace D. (2020). PyCO2SYS: marine carbonate system calculations in Python. Zenodo. doi: 10.5281/zenodo.3744275
Hutchings L., van der Lingen C. D., Shannon L. J., Crawford R. J. M., Verheye H. M. S., Bartholomae C. H., et al. (2009). “The Benguela Current: An ecosystem of four components,” in Eastern Boundary Upwelling Ecosystems: Integrative and Comparative Approaches (Progress in Oceanography), vol. 83. , 15–32. doi: 10.1016/j.pocean.2009.07.046
Inthorn M., Wagner T., Scheeder G., Zabel M. (2006). Lateral transport controls distribution, quality, and burial of organic matter along continental slopes in high-productivity areas. Geology 34, 205–208. doi: 10.1130/G22153.1
Kämpf J., Chapman P. (2016). “The Benguela Current Upwelling System,” in Upwelling Systems of the World (Springer International Publishing, Switzerland).
Lamont T., Barlow R. G., Brewin R. J. W. (2019). [amp]]lsquo;Long-term trends in phytoplankton chlorophyll a and size structure in the benguela upwelling system’. J. Geophys. Res.: Oceans 124, 1170–1195. doi: 10.1029/2018JC014334
Lahajnar (2015). Surface Sediments analysis from Meteor Cruise M103/1 in the Benguela Upwelling System off Namibia in December 2013 and January 2014. In.: PANGAEA. doi: 10.1594/PANGAEA.854182
Lamont T., Hutchings L., van den Berg M. A., Goschen W. S., Barlow R. G. (2015). Hydrographic variability in the St. Helena Bay region of the southern Benguela ecosystem. J. Geophys. Res.: Oceans 120, 2920–2944. doi: 10.1002/2014JC010619
Landschützer P., Gruber N., Bakker D. C. E., Schuster U. (2014). Recent variability of the global ocean carbon sink. Global Biogeochem. Cycles 28, 927–949. doi: 10.1002/2014GB004853
Lewis E., Wallace D. (1998). Program Developed for CO2 system Calculations (Oak Ridge, TN, USA: Carbon Dioxide Information Analysis Center, Oak Ridge National Laboratory, U.S. Department of Energy). ORNL/CDIAC-105.
Lovelock C. E., Duarte C. M. (2019). Dimensions of Blue Carbon and emerging perspectives. Biol. Lett. 15, 20180781. doi: 10.1098/rsbl.2018.0781
PubMed Abstract | PubMed Abstract | Crossref Full Text | Google Scholar
Luisetti T., Turner R.K., Andrews J. E., Jickells T. D., Kröger S., Diesing M., et al. (2019). Quantifying and valuing carbon flows and stores in coastal and shelf ecosystems in the UK. Ecosys. Serv. 35, 67–76. doi: 10.1016/j.ecoser.2018.10.013
Mayer L. M., Schick D. F., Findlay R. H., Rice D. L. (1991). Effects of commercial dragging on sedimentary organic matter. Mar. Environ. Res. 31, 249–261. doi: 10.1016/0141-1136(91)90015-Z
McCartney M. S. (1977). Subantarctic Mode Water A Voyage of Discovery. Angel M. V. (Pergamon Press), 103–119, George Deacon 70th Anniversary.
Messié M., Ledesma J., Kolber D., Michisaki R., Foley D., Chavez F. (2009). Potential new production estimates in four eastern boundary upwelling ecosystems. Prog. In Oceanogr. 83, 151–158. doi: 10.1016/j.pocean.2009.07.018
Mohrholz V., Bartholomae C. H., van der Plas A. K., Lass H. U. (2008). The seasonal variability of the northern Benguela undercurrent and its relation to the oxygen budget on the shelf. Continent. Shelf Res. 28, 424–441. doi: 10.1016/j.csr.2007.10.001
Mohrholz V., Eggert A., Junker T., Nausch G., Ohde T., Schmidt M. (2014). Cross shelf hydrographic and hydrochemical conditions and their short term variability at the northern Benguela during a normal upwelling season. J. Mar. Syst. 140, 92–110. doi: 10.1016/j.jmarsys.2014.04.019
Monteiro P. M. S., Nelson G., van der Plas A., Mabille E., Bailey G. W., Klingelhoeffer E. (2005). Internal tide—shelf topography interactions as a forcing factor governing the large-scale distribution and burial fluxes of particulate organic matter (POM) in the Benguela upwelling system. Continent. Shelf Res. 25, 1864–1876. doi: 10.1016/j.csr.2005.06.012
Monteiro P. M. S., van der Plas A., Mohrholz V., Mabille E., Pascall A., Joubert W. (2006). [amp]]lsquo;Variability of natural hypoxia and methane in a coastal upwelling system: Oceanic physics or shelf biology? Geophys. Res. Lett. 33, L16614. doi: 10.1029/2006GL026234
Morys C., Brüchert V., Bradshaw C. (2021). Impacts of bottom trawling on benthic biogeochemistry in muddy sediments: Removal of surface sediment using an experimental field study. Mar. Environ. Res. 169, 105384. doi: 10.1016/j.marenvres.2021.105384
PubMed Abstract | PubMed Abstract | Crossref Full Text | Google Scholar
Muller A. A., Schmidt M., Reason C., Mohrholz V., Eggert A. (2014). Computing transport budgets along the shelf and across the shelf edge in the northern Benguela during summer (DJF) and winter (JJA). J. Mar. Syst. 140, 82–91. doi: 10.1016/j.jmarsys.2014.02.007
Mwafila S. K. (2017). The community structure of demersal fish species from bottom-trawls off Namibia and the West coast of South Africa. International Journal of Life Sciences, 5 (2), 180–188.
Nellemann C., Corcoran E., Duarte C., Valdes L., Young C., Fonseca L., et al. (2009). Blue Carbon - The Role of Healthy Oceans in Binding Carbon. (United Nations Environment Programme, GRID-Arendal).
Neumann A., Lahajnar N., Emeis K. (2016). Benthic remineralisation rates in shelf and slope sediments of the northern Benguela upwelling margin. Continent. Shelf Res. 113, 47–61. doi: 10.1016/j.csr.2015.12.009
O’Hara C. C., Frazier M., Halpern S.B. (2021). At-risk marine biodiversity faces extensive, expanding, and intensifying human impacts. Science 372, 84–87. doi: 10.1126/science.abe6731
PubMed Abstract | PubMed Abstract | Crossref Full Text | Google Scholar
Oberle F. K.J., Storlazzi C. D., Hanebuth T. J.J. (2016). [amp]]lsquo;What a drag: Quantifying the global impact of chronic bottom trawling on continental shelf sediment. J. Mar. Syst. 159, 109–119. doi: 10.1016/j.jmarsys.2015.12.007
Palanques A., Puig P., Guillén J., Demestre M., Martín J. (2014). Effects of bottom trawling on the Ebro continental shelf sedimentary system (NW Mediterranean). Continent. Shelf Res. 72, 83–98. doi: 10.1016/j.csr.2013.10.008
Paradis S., Pusceddu A., Masqué P., Puig P., Moccia D., Russo T., et al. (2019). Organic matter contents and degradation in a highly trawled area during fresh particle inputs (Gulf of Castellammare, southwestern Mediterranean). Biogeosciences 16, 4307–4320. doi: 10.5194/bg-16-4307-2019
Pendleton L., Donato D. C., Murray B. C., Crooks S., Aaron Jenkins W., Sifleet S., et al. (2012). Estimating global “Blue carbon” Emissions from conversion and degradation of vegetated coastal ecosystems. PloS One 7, e43542. doi: 10.1371/journal.pone.0043542
PubMed Abstract | PubMed Abstract | Crossref Full Text | Google Scholar
Pika P. A., Hülse D., Eglinton T. I., Arndt S. (2023). Regional and global patterns of apparent organic matter reactivity in marine sediments. Global Biogeochem. Cycles 37, e2022GB007636. doi: 10.1029/2022GB007636
Pitcher G. C., Probyn T. A., du Randt A., Lucas A. J., Bernard S., Evers-King H., et al. (2014). Dynamics of oxygen depletion in the nearshore of a coastal embayment of the southern Benguela upwelling system. J. Geophys. Res.: Oceans 119, 2183–2200. doi: 10.1002/2013JC009443
Polymenakou P. N., Pusceddu A., Tselepides A., Polychronaki T., Giannakourou A., Fiordelmondo C., et al. (2005). Benthic microbial abundance and activities in an intensively trawled ecosystem (Thermaikos Gulf, Aegean Sea). Continent. Shelf Res. 25, 2570–2584. doi: 10.1016/j.csr.2005.08.018
Pusceddu A., Bianchelli S., Martín J., Puig P., Palanques A., Masqué P., et al. (2014). Chronic and intensive bottom trawling impairs deep-sea biodiversity and ecosystem functioning. Proc. Natl. Acad. Sci. United States America 111. doi: 10.1073/pnas.1405454111
Pusceddu A., Grémare A., Escoubeyrou K., Amouroux J. M., Fiordelmondo C., Danovaro R. (2005). Impact of natural (storm) and anthropogenic (trawling) sediment resuspension on particulate organic matter in coastal environments. Continent. Shelf Res. 25, 2506–2520. doi: 10.1016/j.csr.2005.08.012
Rixen T. (2023). Past changes in and present status of the coastal carbon cycle. Cambridge Prisms: Coast. Futures, 1–35. doi: 10.1017/cft.2023.20
Rixen T., Lahajnar N., Lamont T., Koppelmann R., Martin B., Meiritz L., et al. (2023). “The marine carbon footprint: Challenges in the quantification of CO2 emissions from southern Africa,” in Sustainability of southern African ecosystems under global change: Science for management and policy interventions. Eds. Maltitz G.v., Midgley G. F., Veitch J., Brümmer C., Rötter R., Viehberg F., Veste M. (Springer, Berlin, Heidelberg, New York).
Roberts C. M., O’Leary B. C., McCauley D. J., Cury P. M., Duarte C. M., Lubchenco J., et al. (2017). Marine reserves can mitigate and promote adaptation to climate change. Proc. Natl. Acad. Sci. 114, 6167–6175. doi: 10.1073/pnas.1701262114
Rogers J., Rau A. J. (2010). Surficial sediments of the wave-dominated Orange River Delta and the adjacent continental margin off south-western Africa. Afr. J. Mar. Sci. 28, 511–524. doi: 10.2989/18142320609504202
Sala E., Mayorga J., Bradley D., Cabral R. B., Atwood T. B., Auber A., et al. (2021). Protecting the global ocean for biodiversity, food and climate. Nature 592, 397–402. doi: 10.1038/s41586-021-03371-z
PubMed Abstract | PubMed Abstract | Crossref Full Text | Google Scholar
Sciberras M., Parker R., Powell C., Robertson C., Kröger S., Bolam S., et al. (2016). Impacts of bottom fishing on the sediment infaunal community and biogeochemistry of cohesive and non-cohesive sediments’. Limnol. Oceanogr. 61, 2076–2089. doi: 10.1002/lno.10354
Shillington F. A., Reason C. J. C., Duncombe Rae C. M., Florenchie P., Penven P. (2006). “4 Large scale physical variability of the Benguela Current Large Marine Ecosystem (BCLME),” in Large Marine Ecosystems. Eds. Shannon V., Hempel G., Malanotte-Rizzoli P., Moloney C., Woods J. (Amsterdam, Netherlands: Elsevier).
Siddiqui C., Rixen T., Lahajnar N., Plas A. K.v. d., Louw D. C., Lamont T., et al. (2023). Regional and global impact of CO2 uptake in the Benguela Upwelling System through preformed nutrients. Nat. Commun. 1, 2582. doi: 10.1038/s41467-023-38208-y
Siegenthaler U., Sarmiento J. L. (1993). [amp]]lsquo;Atmospheric carbon dioxide and the ocean’. Nature 365, 119–125. doi: 10.1038/365119a0
Takahashi T., Sutherland S. C., Chipman D. W., Goddard J. G., Ho C., Newberger T., et al. (2014). Climatological distributions of pH, pCO2, total CO2, alkalinity, and CaCO3 saturation in the global surface ocean, and temporal changes at selected locations. Mar. Chem. 164, 95–125. doi: 10.1016/j.marchem.2014.06.004
Takahashi T., Sutherland S. C., Wanninkhof R., Sweeney C., Feely R. A., Chipman D. W., et al. (2009). Climatological mean and decadal change in surface ocean pCO2, and net sea–air CO2 flux over the global oceans. Deep-Sea Res. II 56, 554–577. doi: 10.1016/j.dsr2.2008.12.009
Tiano J., Witbaard R., Bergman M., Rijswijk P., Tramper A., Oevelen D., et al. (2019). Acute impacts of bottom trawl gears on benthic metabolism and nutrient cycling. ICES J. Mar. Sci. 76, 1917–1930. doi: 10.1093/icesjms/fsz060
Trimmer M., Petersen J., Sivyer D. B., Mills C., Young E., Parker E. R. (2005). Impact of long-term benthic trawl disturbance on sediment sorting and biogeochemistry in the southern North Sea. Mar. Ecol. Prog. Ser. 298, 79–94. doi: 10.3354/meps298079
van der Plas A. K., Monteiro P. M. S., Pascall A. (2007). Cross-shelf biogeochemical characteristics of sediments in the central Benguela and their relationship to overlying water column hypoxia. Afr. J. Mar. Sci. 29, 37–47. doi: 10.2989/AJMS.2007.29.1.3.68
Volk T., Hoffert M. I. (1985). “Ocean Carbon Pumps: Analysis of Relative Strengths and Efficiencies in Ocean-Driven Atmospheric CO2 Changes,” in The Carbon Cycle and Atmospheric CO2: Natural Variations Archean to Present, 99–110. doi: 10.1029/GM032p0099
Waldron H. N., Monteiro P. M. S., Swart N. C. (2009). [amp]]lsquo;Carbon export and sequestration in the southern Benguela upwelling system: lower and upper estimates’. Ocean Sci. 5, 711–718. doi: 10.5194/os-5-711-2009
Wanninkhof R. (2014). Relationship between wind speed and gas exchange over the ocean revisited. Limnol. Oceanogr.: Methods 12, 351–362. doi: 10.4319/lom.2014.12.351
Weeks S. J., Barlow R., Roy C., Shillington F. A. (2006). Remotely sensed variability of temperature and chlorophyll in the southern Benguela: upwelling frequency and phytoplankton response. Afr. J. Mar. Sci. 28, 493–509. doi: 10.2989/18142320609504201
Weiss R. F. (1974). Carbon dioxide in water and seawater: the solubility of a non-ideal gas. Mar. Chem. 2, 203–215. doi: 10.1016/0304-4203(74)90015-2
Keywords: Benguela coastal upwelling system, bottom trawling effects, biological carbon pump, carbon and nutrient cycling, CO2 emissions
Citation: Siddiqui C, Rixen T, Lahajnar N, Lamont T and van der Plas AK (2024) Simulating potential impacts of bottom trawling on the biological carbon pump: a case study in the Benguela Upwelling System. Front. Mar. Sci. 11:1387121. doi: 10.3389/fmars.2024.1387121
Received: 16 February 2024; Accepted: 02 October 2024;
Published: 05 November 2024.
Edited by:
Alex J. Poulton, Heriot-Watt University, United StatesCopyright © 2024 Siddiqui, Rixen, Lahajnar, Lamont and van der Plas. This is an open-access article distributed under the terms of the Creative Commons Attribution License (CC BY). The use, distribution or reproduction in other forums is permitted, provided the original author(s) and the copyright owner(s) are credited and that the original publication in this journal is cited, in accordance with accepted academic practice. No use, distribution or reproduction is permitted which does not comply with these terms.
*Correspondence: Claire Siddiqui, Y2xhaXJlLnNpZGRpcXVpQGFuZW1vcy5kZQ==
†Present address: Claire Siddiqui, anemos Gesellschaft für Umweltmeteorologie mbH, Reppenstedt, Germany