- 1Division of Marine Science and Conservation, Nicholas School of the Environment, Duke University, Beaufort, NC, United States
- 2INSPIRE Environmental, Newport, RI, United States
Bioturbation is a key ecosystem function with a fundamental role in mediating major biogeochemical cycles. The intensity and depth of bioturbation is influenced by the taxa present, which is often a function of food supply. The deep sea is generally oligotrophic with sediments composed predominantly of small, shallow burrowing macrofauna (<10 cm). Human activity is increasingly introducing POC to the deep-sea, however, organic enrichment of the deep-sea, and the subsequent response of bioturbators is poorly understood. Here we present data on benthic function in deep-sea systems that have experienced organic enrichment. We show that organic enrichment enhances deep-sea bioturbation through larger, advanced successional taxa, and deeper bioturbation depths. Enhanced bioturbation in the deep-sea should confer positive ecosystem functions (nutrient recycling, microbiological activity, remineralization), but adherence to approaches and interpretations guided by the paradigm of small, shallow-burrowing infauna could significantly underestimate deep-sea benthic processes at a global scale.
Highlights
● Evidence showing anthropogenic organic enrichment of the deep-sea enhances deep-sea function by stimulating bioturbation.
Introduction
The deep sea (>1000 m water depth) is the most extensive habitat on our planet (Snelgrove, 1997; Danovaro et al., 2017) and plays a critical role in global ecosystem function via its influence on the biological pump and nutrient recycling (Snelgrove, 1997; Ramirez-Llodra et al., 2011; Thurber et al., 2014). More than 60% of the Earth’s surface is below 1000 m of water (Glover and Smith, 2003); from about 1000 m to the deepest depths of the ocean floor there is virtually no seasonal variation and water temperatures are consistently ~2°C (Rex and Etter, 2010). This once remote ecosystem is threatened by the cumulative effects of anthropogenic activities (e.g., climate change; fisheries; oil, gas, and mineral extraction), which could result in ecological impairment and potentially catastrophic regime-shifts (Levin and Le Bris, 2015; Wedding et al., 2015; Sweetman et al., 2017). Awareness of increased human disturbances in the deep sea has led to recent calls for the development of an ecosystem-based strategy for global cooperation in deep-sea preservation (Danovaro et al., 2017; Boetius and Haeckel, 2018), but effective conservation requires a robust and accurate understanding of the environment (Le Saout et al., 2013). As our understanding of the deep sea is refined, our perceptions have been reshaped from viewing this environment as homogeneous to one that is complex and diverse (Brandt et al., 2007; Danovaro et al., 2014; Costello and Chaudhary, 2018).
Deep-sea sediments have been traditionally viewed as oligotrophic and food-limited, devoid of the requisite carbon and nutrient input to support large-bodied and deep burrowing macrofauna (Thiel, 1979; Grassle and Grassle, 1994); for this review macrofauna are defined as individuals retained on a sieve size greater than 300 µm, commonly used in deep-sea studies (Supplementary Table SI; Montagna et al., 2016). Chemotrophic production provides organic enrichment in localized instances in the deep sea (Van Dover, 2000), however, the deposition of phytodetritus and/or the horizontal advection of organic matter from the continental shelf supplies carbon and nutrients to much of the deep sea (Jahnke et al., 1990; Honjo et al., 2008). In the latter two instances, the amount of available organic matter to the deep-sea benthos is limited by surface production and the subsequent flux to the seafloor (Honjo et al., 2008). The majority of material transported to the deep seafloor tends to be refractory and a fraction of the quantity that was produced in surface waters (Rowe and Staresinic, 1979), though there are episodic instances where POC produced in surface water is rapidly transported to the deep sea delivering large amounts of fresh (i.e., nonrefractory) material to the sea floor (Smith et al., 2018). As a result of the predominant process of POC transport to the deep sea (Rowe and Staresinic, 1979; Jahnke et al., 1990; Honjo et al., 2008), macrofauna present in deep-sea sediments tend to be small and confined to the upper few centimeters of the sediment column (Rex and Etter, 2010). Thus, methods for sampling deep-sea macrofauna have largely focused on the upper 10 cm of the sediment (Rex and Etter, 2010; Montagna et al., 2016; Supplementary Table SI). This perspective has been challenged recently (Uchman and Wetzel, 2011) but does not appear to have shifted the predominant view of deep-sea ecologists conducting benthic community analysis. The majority of deep-sea benthic community studies employing traditional sampling methods (sediment grabs, box cores, or multi-core arrays) limited the depth of sieving to 10 cm, regardless of the sediment depth cores or grabs were collected (~70% from our literature review presented in Supplementary Table SI; Rex and Etter, 2010). However, there are numerous places in the deep sea that are not oligotrophic (Graf, 1989; Diaz et al., 1994; Witte, 2000) and adherence to approaches (sieving <10 cm) and interpretations guided by a paradigm of small, shallow bioturbators in deep-sea sediments could significantly underestimate deep-sea benthic processes.
Bioturbation describes the biological displacement or mixing of sediments by flora, fauna or microbial activity (Meysman et al., 2006). In marine sediments macrofauna are predominantly responsible for sediment reworking, which has been shown to play a vital role in regulating marine sediment geochemical and physical properties (Aller, 1978; Rhoads and Boyer, 1982; Nicholaus et al., 2023) as well as affecting ecosystem function (Meysman et al., 2006). Sediment permeability, chemical gradients in pore water, remineralization (supplying electron acceptors that fuel organic mineralization), and inorganic nutrient efflux are a few of the sediment properties and functions regulated by macrofauna bioturbation (Lohrer et al., 2004).
There are two main approaches to assessing bioturbation in marine sediments: as a diffusion process or through direct measurements from cores. The diffusion process is a modelling technique that allows the distribution and exchange rates of pore water solutes, or sediment particles across the sediment-water interface to be quantified and compared by calculating an intensity coefficient and/or a mixed layer depth (Teal et al., 2008). Direct core measurements calculate the depth of bioturbation by measuring the depth of biogenic features in the sediment column relative to the sediment-water interface (Germano et al., 2011). In this study bioturbation was quantified using direct measurements from optical cores (SPI).
Over the years a number of deep-sea benthic surveys have been conducted around anthropogenically disturbed sites using sediment profile cameras (Figure 11*; See S1 to S5). Sediment profile images (SPI) provide a cross-section of the sediment column from the sediment-water interface (SWI) down to ca. 22 cm (Rhoads and Cande, 1971; Solan et al., 2003). The most striking observation from these surveys was the extent of sediment reworking and depth of macrofauna burrowing. Bioturbation depths, the depth beneath the sediment-water interface that biogenic structures (burrows, feeding voids) were detected, exceeded 15–21 cm. Burrows were defined as open or partially filled channels in the sediment surrounded by brownish sediment (oxic halo), and voids as water filled pockets.
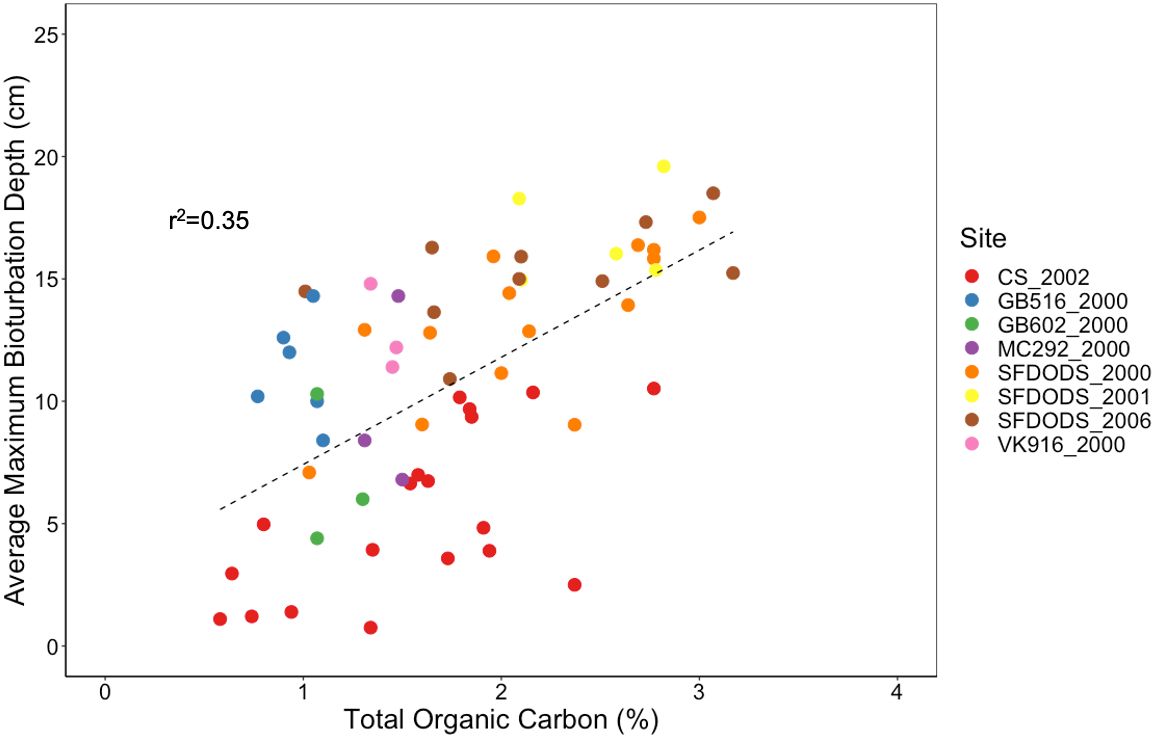
Figure 1 Relationship between sediment Total Organic Carbon (%) and maximum bioturbation depth (cm). A significant positive relationship between sediment percent total organic carbon (TOC) and bioturbation depth (df=61, F=33.44, p<0.0005), with bioturbation depth increasing linearly with increasing TOC. Sediment TOC (assessed from sediment grabs) was compared to maximum bioturbation depth, measured by sediment profile imaging, from 8 different deep-sea surveys around the world: CS_2002 – Caspian Sea survey in 2002 related to drilling muds discharge; GB516_2000 and GB602_2000 – surveys around two wells in the Gulf of Mexico in 2000 related to synthetic drilling muds discharge; MC292_2000 and VK916_2000 – surveys around two wells in the Gulf of Mexico in 2000 related to drilling muds discharge; and SFDODS_2000, SFDODS_2001, and SFDODS_2006 – surveys in the eastern Pacific Ocean at a deep-sea dredged material disposal site off the coast of San Francisco Bay.
These observations indicate larger deeper dwelling macrofauna may play a more important role in biogeochemical cycling in the deep sea than previously assumed. This insight prompted us to revisit current and past paradigms of deep-sea benthic community structure and function. In order to shift from the current shallow-community structure approach to sampling deep-sea sediments, to one that focuses on benthic function, will require sampling deeper than the 10 cm approach commonly applied (Montagna et al., 2016; Supplementary Table SI). Especially as we gain a better understanding of how organic enrichment from human activity or localized organic input reshapes the response and function of deep-sea communities, resulting in sediments with functional characteristics (deep bioturbation) similar to productive shallow coastal systems (Zhang et al., 2024).
Organic enrichment and bioturbation in the deep sea
Large areas of the deep sea are oligotrophic (Druffel and Robison, 1999) and, in these areas, macrofauna are generally small-bodied and primarily reside in the upper 2–3 cm of the sediment column (Snider et al., 1984; Witte, 2000; Rex and Etter, 2010). Macrofauna, relatively sessile organisms that spend the vast majority of their lives inhabiting the sediment, influence many important benthic functions through bioturbation (Solan et al., 2003), and secondary production (Nilsen et al., 2006). Numerous studies have attempted to document the biological response of deep-sea macrofauna to the flux of particulate organic carbon (POC), but the responses are complex, and dependent on the source and type of POC enrichment (e.g., carcass falls, anthropogenic sources, upwelling zones, density flows) (Pfannkuche, 1993; Diaz et al., 1994; Smith et al., 2014). Deep-sea macrobenthic response to POC flux has ranged from limited or nonexistent (Pfannkuche, 1993; Gooday et al., 1996; Drazen et al., 1998; Smith et al., 2002), to a rapid response occurring on the order of days (Graf, 1989; Witte et al., 2003). The contrast in response between these endpoints suggests fine-scale differences in the quantity, quality, and rate of POC deposition to the deep seafloor, even though most carbon hitting the deep sea is well reworked (Honjo et al., 2008).
It is generally accepted in deep sea ecology that 90% of meio- and macrofauna occupy the top few cm of the sediment, and in organically rich continental shelf sediments macrofauna can extend down to 10 cm, but it is not common (Rex and Etter, 2010; Montagna et al., 2016). Our data review suggests an alternative and presents a picture of deep-sea sediment communities where organic enrichment can facilitate deep burrowing often extending well below 10 cm (Figure 1). In a synthesis of deep-sea sites around the globe (S1-S5), we found bioturbation depth to be significantly correlated with the percent of total organic carbon (TOC) in the sediment (df=61, F=33.44, p<0.0005), with TOC explaining more than 35% of the variability in bioturbation depth. Further, there was evidence that organic enrichment of the deep sea facilitated a community of deep burrowing (>10 cm) bioturbators (Figure 2). These findings were consistent with recent observations assessing the influence of organic matter on deep-sea bioturbation (Zhang et al., 2024).
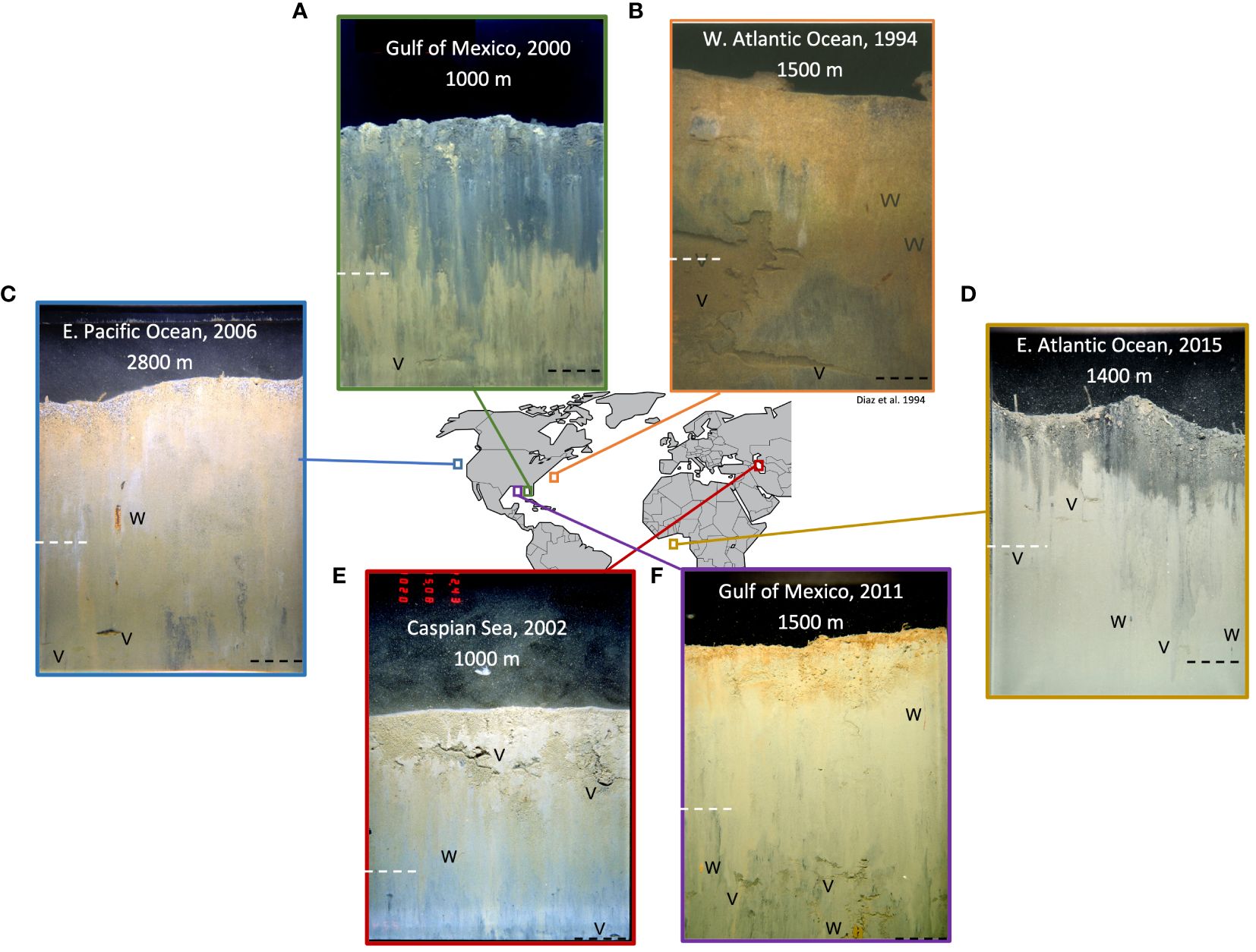
Figure 2 Locations around the globe where deep-sea organic enrichment and enhanced deep-sea bioturbation were observed. Organic enrichment of the deep sea and subsequently enhanced bioturbation documented at a number of locations around the globe. The mechanism of enrichment varied between location, but the process in each instance was similar (large input of organic material to the deep sea over a short timeframe). The dotted white lines denote 10 cm depth in the sediment column, representing the depth to which traditional deep-sea sampling studies process the sediment. The black dotted lines are the maximum depth of bioturbation observed in each SPI; on occasion the black line is at the bottom edge of the field of view (FOV), suggesting bioturbation extends deeper than the FOV of the SPI. Feeding voids [V] and active burrows [W] are also indicated. (A) Enrichment of the deep-sea Gulf of Mexico from synthetic drilling muds. The signature of disturbance (synthetic drilling muds) was visible in the sediment profile (gray sediment), and feeding voids were present deep within the sediment column. (B) Enrichment of the outer continental shelf and upper slope off Cape Hatteras bight due to hydrodynamic confluence. Numerous voids and burrows were visible throughout the sediment column. (C) Enrichment of the Eastern Pacific deep sea following the disposal of dredged material. Signatures of dredged material were visible at the sediment-water interface (gray coarse grains and grayish white mottled clay), a large worm was visible in its burrow extending deep into sediment, and there was a presence of feeding voids near the bottom edged of the FOV. (D) Enrichment of the eastern Atlantic continental slope from drilling muds. Drill cuttings were visible in the sediment profile (black sediment just below the sediment-water interface), and numerous voids and burrows were present deep in the sediment column. (E) Enrichment in the Caspian Sea around a well from drilling activity. Drilling muds were visible in profile image (brown surface sediment) with voids observed in the drilling mud. A worm was observed in its burrow just below the drilling muds, and a void is visible at the bottom edge of the FOV. (F) Enrichment of the deep-sea Gulf of Mexico following the Deepwater Horizon oil spill. Numerous feeding voids were observed deep within the sediment column, and a large worm was visible against faceplate near the penetration maximum of the prism. Scale of the images is 21 x 15 cm.
Diaz et al. (1994) found deep-sea bioturbation extending past 10 cm off the coast of Cape Hatteras, NC (Figure 2B). The outer continental shelf and upper slope off Cape Hatteras bight is located at an important hydrodynamic confluence that results in this part of the Atlantic slope receiving high rates of organic and inorganic materials (Blake and Grassle, 1994) supporting high macrofauna production (Blake and Hilbig, 1994). Using x-ray cores, 210Pb, and SPI, Diaz et al. (1994) found surface sedimentary structures to be dominated by the bioturbation activities of deep burrowing macrofauna to at least 30 cm depth in the sediment column at stations up to 2,000 m water depth.
Similar observations were made in the early 2000s along the continental slope in the Gulf of Mexico from 1,000 to 1,075 m depths (Figure 2A) (CSA, 2006). A survey was commissioned by the U.S. Mineral Management Service (now the Bureau of Ocean Energy Management) to assess the physical, chemical, and biological effects of oil and gas development by investigating sediments and biota at several well sites (Supplementary Table S1–1; Supplementary Figure S1–1). SPI results from these studies showed active bioturbation deep within the sediment column both near (in the immediate vicinity of drilling activity) and far (stations located 10 to 25 km away from the well sites) from the wells in all examined scenarios. At each of the four well sites influenced by drilling activity, which included organic-rich synthetic drilling muds (TOC up to 2.6%), the maximum bioturbation depths in SPI ranged from 10.3 to 19.0 cm with a mean of 15.1 cm (SD±2.8) (Supplementary Table S1–1; Supplementary Figure S1–3). Synthetic drilling muds are a nonaqueous emulsion in which the external phase is synthetic fluid rather than petroleum. Synthetic muds were developed as environmentally friendly alternatives to conventional petroleum-derived oil-based muds due to their lower toxicity (Growcock et al., 1994). Due to these properties synthetic muds have become popular for offshore drilling projects because of the environmental acceptance to disposing of synthetic muds into the environment. These observations show that use of synthetic drilling muds organically enriches deep-sea sediments resulting in deep bioturbation.
This pattern was also observed in deep-sea sediments surrounding well sites located between 1,200 and 1,400 m depth on the upper continental slope of the Ghanaian continental shelf (Figure 2D) in the Deep Ivorian Basin. SPI were collected as part of a multidisciplinary sampling program to assess impacts of drilling mud discharge on the seafloor near four offshore exploration/production platforms (S2). Sampling around the four production platforms occurred between 150 m and 1000 m from the location where the wells were being drilled. Maximum bioturbation depths ranging from 6.2 to 21.1 cm, with a mean of 14.8 cm (SD±3.2), were observed in the sediments around the four well sites.
Deep bioturbation was also observed in the abyssal plains of the Pacific Ocean following the disposal of dredged spoils (Figure 2C). In the northeastern Pacific Ocean, organically rich dredged material (TOC up to 3.2%) was deposited on an annual basis (material in excess of 16 million cubic yards between 1993 and 2009) at depths of 2,500 to 3,000 m at the San Francisco Deep Ocean Disposal Site (SF-DODS). Monitoring surveys were commissioned by the U.S. Environmental Protection Agency (USEPA) as part of their site monitoring and management plan; those surveys that included SPI took place in 2000, 2001, 2006, and 2009. A synthesis of these four surveys (S3) found maximum bioturbation depths at SF-DODS ranged from 18.6 to 21.7 cm with a mean of 19.5 cm (SD ± 1.0).
A similar pattern of deep bioturbation depths occurred following the Deepwater Horizon oil spill in the Gulf of Mexico (Figure 2F) (S4). The Macondo wellhead blowout and subsequent activities to control the spill resulted in the deposition of large amounts of hydrocarbons and organic drilling muds to the seabed (Atlas and Hazen, 2011; McNutt et al., 2012; Guarinello et al., 2022). In response to the spill, SPI was collected at depths ranging from 1,040 to 1,689 m in spring 2011 (12 months following the spill), fall 2011 (18 months post-spill), and spring 2014 (37 months post-spill) (Supplementary Figure S4–1). The mean of maximum bioturbation depth from the three surveys ranged from 10.9 to 21.0 cm with a mean of 16.4 cm (SD ± 1.6). At every station sampled from the three surveys, evidence of deep-burrowing macrofauna (>10 cm) were observed (Supplementary Figures S4–2, S4–3, S4–4).
A paradox in deep-sea enrichment and bioturbation
Interpretations of deep-sea benthic community function can be influenced by the methods employed. In comparing assumptions behind traditional methods of sediment collection and selective sieving, with observations from SPI, we postulate traditional methods may potentially lead to an underestimate of benthic function, especially in areas affected by human activities. We found a striking consistency in deep-sea benthic response to organic enrichment at multiple locations around the globe. Each case study represented a different mechanism of anthropogenic enrichment to the deep sea (drilling muds, dredged material, hydrocarbons) with a commonality of large quantities of organic material being deposited on the seafloor over a relatively short period of time. It is precisely these two components, the quantity of material and the timeframe of deposition, that we hypothesize is important for the observations we presented. Within a defined spatial area, over a relatively short-time period human activity can enrich deep-sea sediments in excess of the natural processes supplying carbon to an otherwise oligotrophic seabed. Organic enrichment also occurs in otherwise oligotrophic environments from natural sources such as oil seeps (Levin et al., 2016; Kennicutt, 2017), vents (Marcus et al., 2009), or ocean fronts (Diaz et al., 1994).
Analogous to shallow systems that experience organic enrichment, anthropogenic enrichment of the deep sea is a balance between organic enrichment and consumption. Optically, there is a stark contrast in the sediment profiles of deep-sea sediments experiencing enrichment and those from an oligotrophic seafloor (Figure 3). Physical signatures of the enrichment mechanism (dredged material, drilling muds, hydrocarbons) are often visible on the seabed or in the sediment, the sediment column is “pocked” with feeding voids (the products of advanced successional taxa) (Pearson and Rosenberg, 1978; Germano et al., 2011), and bioturbation extends >10 cm beneath the sediment-water interface. The activity of macrofauna is enhanced and positive functions related to enhanced bioturbation should be realized (nutrient recycling, enhanced microbiological activity, remineralization) (Aller et al., 2001; Meysman et al., 2006). While organic enrichment of the deep sea can elicit a positive response in macrofauna activity and function (Baguley et al., 2006), we hypothesize that over-enrichment should promote anaerobic conditions, as frequently occurs in shallow coastal systems (Sturdivant et al., 2011). At a certain threshold of enrichment, deep-sea macrobenthic bioturbation should decline, reduced conditions should become prominent, and microbial processes should dominate (Figure 4). An example of this can be seen in S1, where the impact of synthetic drilling muds on the deep seafloor were assessed. Supplementary Figure S1–5 depicts a SPI captured at a station immediately adjacent the well. In this example, the impact of drilling muds on the surrounding seafloor is a function of distance from the well. Drilling muds and organic enrichment are greatest at stations closest to the well and decreases with increasing distance from the well. The sediment profile in Supplementary Figure S1–5 is entirely composed of organic rich drilling muds. There is low optical reflectance of the sediment in this image indicating high sediment oxygen demand, and the presence of Thiophilic bacteria are visible at the sediment-water interface. These features are evidence of a reduced environment experienced high organic enrichment.
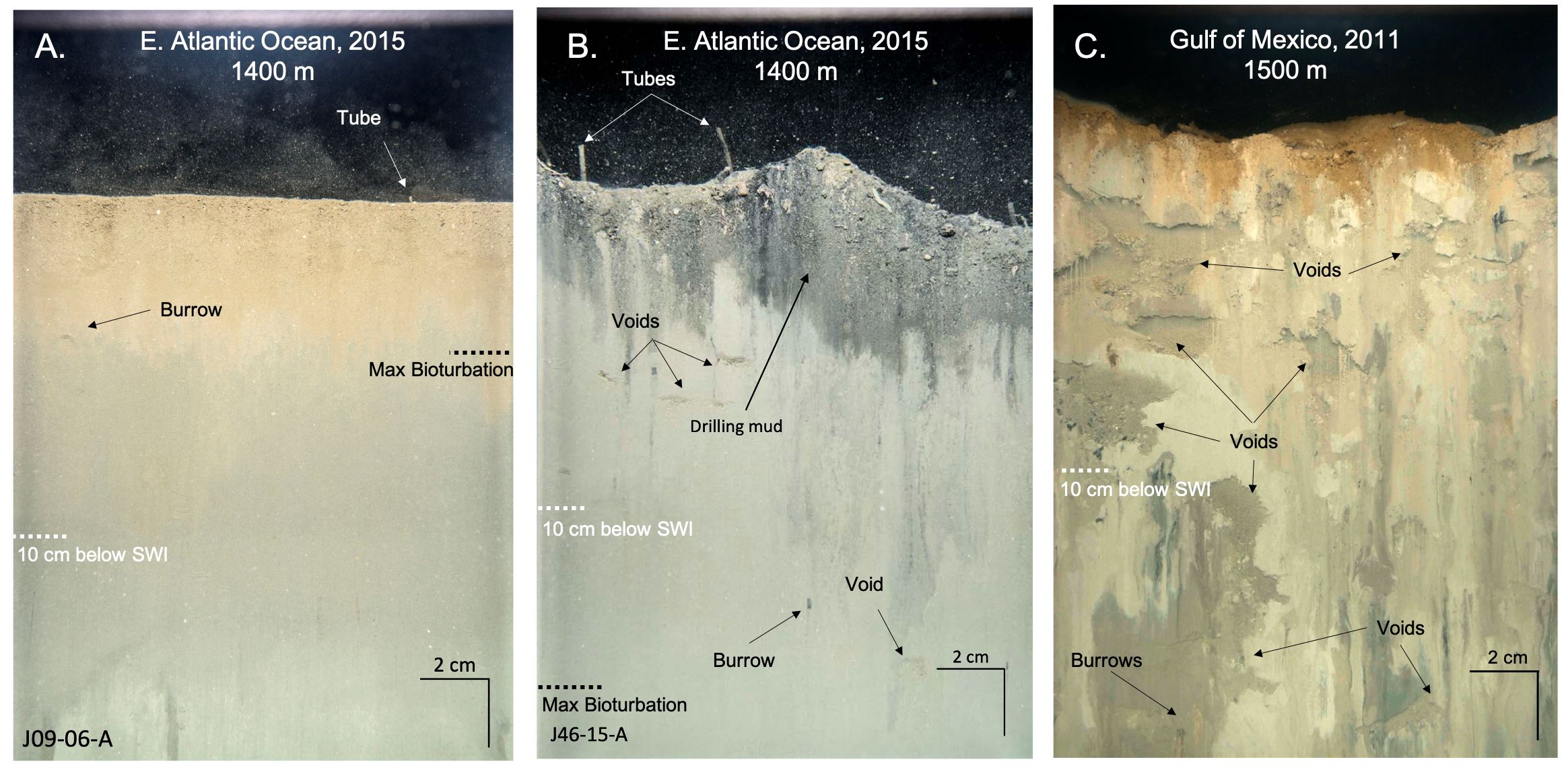
Figure 3 Optical comparison between (A) oligotrophic deep-sea sediment profile, and (B, C) deep-sea sediments experiencing organic enrichment. (A) Representation of oligotrophic deep-sea sediments. Image was captured in the Ivorian Basin. The maximum depth of bioturbation is ~2–3 cm below the sediment-water interface, and small tubes were present on the sediment surface. (B) Representation of deep-sea sediments experiencing enrichment from drilling muds. Image was captured in the Ivorian Basin located ~150 m from a well. Drilling muds were visible just below the sediment-water interface (black sediment). Numerous voids and burrows were present throughout the sediment column, including deep within the sediment (>10 cm). Medium-sized tubes were present at the sediment surface. (C) Representation of deep-sea sediments experiencing enrichment from hydrocarbons and drilling muds. Image was captured in the Gulf of Mexico following the Deepwater Horizon incident in the footprint of the “top-kill” effort (an effort to plug the well by pumping drilling muds into the blowout – resulted in drilling muds with oil particles scattering out in an NW direction from the well). The majority of the sediment profile was drilling muds from “top-kill” and the sediment column was pocked with voids and burrows. Maximum bioturbation depth extended past the prism penetration (see voids and burrows at the lower extent of the field of view). Scale of the images is 21 x 15 cm.
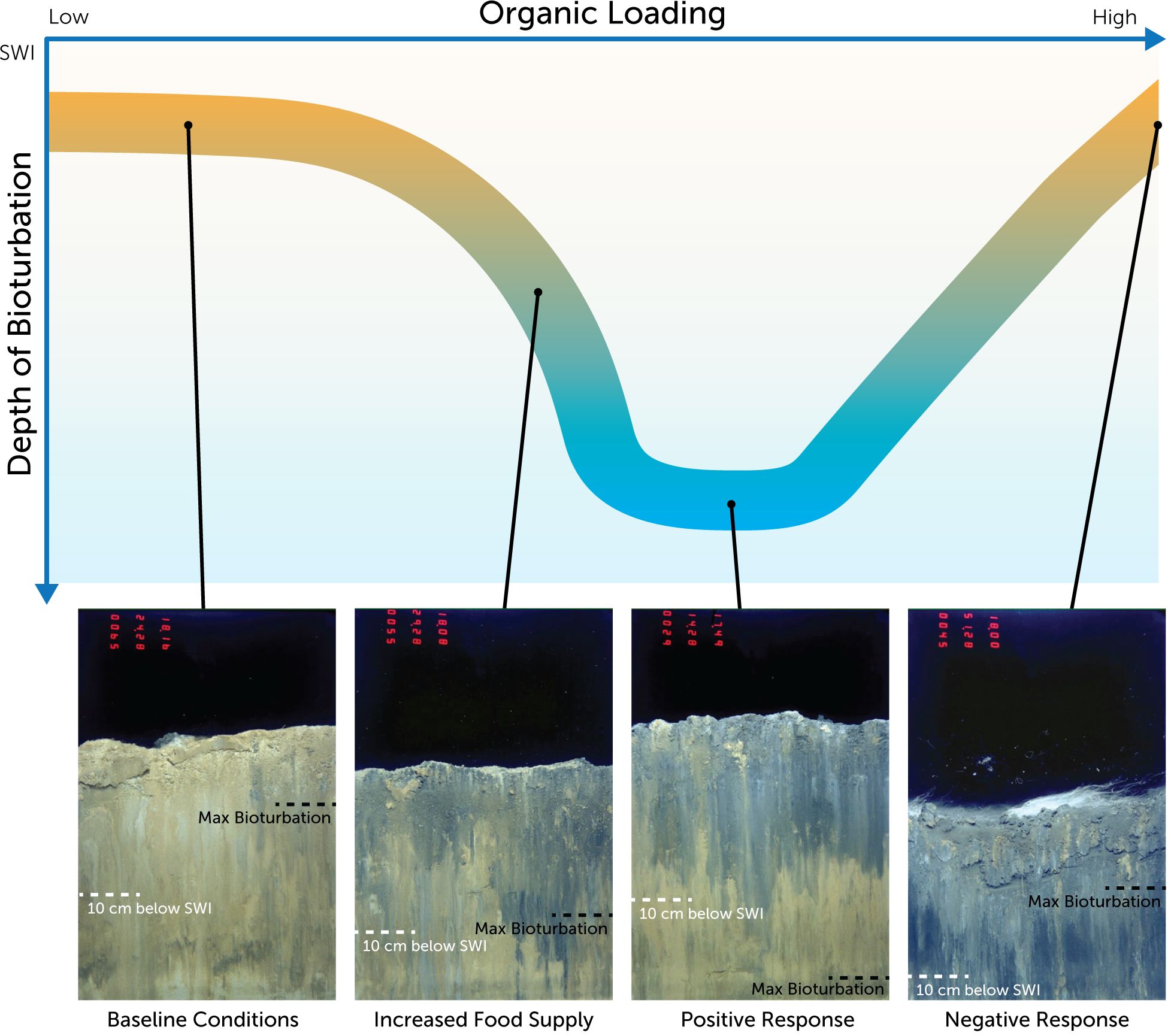
Figure 4 The deep-sea enrichment paradox: a theoretical response of bioturbators to organic enrichment of the deep sea. A conceptual model of how deep-sea sediments respond functionally to organic enrichment. The representative sediment profile images (SPI) were captured in the Gulf of Mexico and the mechanism of enrichment was from the deposition of drilling muds onto the deep-sea floor. Traditional oligotrophic deep-sea sediments are represented at the left of the graph and indicated by a profile image (Baseline Conditions) with no drilling mud and shallow bioturbation depth. Moving along the x-axis as organic enrichment increases (in this case the deposition of drilling muds increases on the seafloor) a positive macrobiological response is triggered (Increased Food Supply) and bioturbation increases in depth. As organic enrichment continues to increase this results in the stimulation of deep burrowing bioturbators (Positive Response). Evidence of the enrichment mechanism (drilling muds) is visible in the profile images (grey sediment). As organic enrichment increases to the point of over enrichment a negative macrobiological response occurs (Negative Response) decreasing bioturbation depths and stimulating anaerobic conditions. A key diagnostic feature of over-enrichment visible in the representative profile image is the presence of thiophilic bacterial mats (white filaments at the sediment-water interface) and a darkening of the sediment as sediment oxygen demand increases due to the available organic content. Scale of the images is 21 x 15 cm.
The deep sea is generally described as an oligotrophic environment with the concept of organic enrichment perceived as somewhat of a paradox (Menzel and Ryther, 1970; Druffel et al., 1989; Rex and Etter, 2010). The notable exceptions to the oligotrophic paradigm are natural seeps and hydrothermal vents (Tunnicliffe et al., 1997) and the occasional phenomena of hydrodynamic confluence (Diaz et al., 1994). Very little is known about macrobenthic communities surrounding seeps and vents and areas of hydrodynamic confluence that enrich the deep sea, such as that observed by Diaz et al. (1994). Based on our observations with SPI, we hypothesize a deep-sea enrichment paradox that counters the typical oligotrophic profile. Given sufficient organic enrichment, the biological response of deep-sea macrofauna can mimic conditions observed in shallow coastal and estuarine systems (Zhang et al., 2024): advanced successional taxa are present (Vetter and Dayton, 1998) and deep bioturbation occurs (Diaz et al., 1994). However, our observations are singular in approach, viewed largely through the “prism” of the sediment profile window. SPI allowed us to document this phenomenon of enhanced benthic function in the deep sea and provide a theoretical conceptualization of deep-sea macrobenthic response to organic enrichment (Figure 4), but questions remain. Does an enhanced deep-sea macrofauna community confer similar positive ecosystem functions to those observed in shallow benthic systems (e.g., nutrient recycling, enhanced microbiological activity, remineralization)? What are the thresholds for organic input (quantity, quality, supply rate) that are necessary to stimulate macrobenthic production and subsequent deep bioturbative activity in the deep sea, and at what point does organic input become over-enrichment with deleterious consequences? When organic enrichment of the deep sea occurs, what proportion of bioturbators occur at depths deeper than 10 cm? All our observations were from large-scale organic inputs over relatively short time frames (days to months). These questions, and more, need to be resolved to better understand the observations we made at the deep-sea sites that were the focus of this study.
Discussion: moving forward in deep-sea ecology
Understanding how a system as important, but difficult to assess, as the deep-sea functions is an imperative. If an area of the deep sea has experienced organic enrichment (anthropogenic or naturally occurring), traditional approaches to benthic community assessment that often do not assess function could lead to underestimating deep-sea benthic function by sampling a small slice (<10 cm) of the sediment. Shallow subsampling can overlook a community that is thriving deep within the sediment and influencing deep-sea sediment processes. This can result in unintentional misinterpretations and misrepresentations of the benthic community and related processes in the deep sea. The ramifications of such underestimations and subsequent misinterpretations can be wide ranging. These have the potential to skew not only our ecological understanding of the deep sea but to distort policy, management, and public opinion related to anthropogenic activity in the deep sea, which has widely been viewed as catastrophically disruptive (Joye, 2016).
Acknowledgement of a paradox in deep-sea enrichment will require ecologists to rethink their approach to deep-sea benthic studies and possibly revisit interpretations of previous studies. Such shifts in approach are most critical in areas where anthropogenic enrichment of the deep-sea may have occurred, especially if such areas are in regions with naturally occurring seeps where benthic communities (microbial and macrofauna) are well-adapted to influxes of carbon (Glover et al., 2010; Smith et al., 2014; Levin et al., 2016). Recent work highlights microbial consumption of hydrocarbons in the global ocean that is occurring on a much larger scale than previously realized (Love et al., 2021). With human influence on the deep sea increasing, our observations are critically relevant for deep-sea ecology moving forward (Ramirez-Llodra et al., 2011). Consideration of ecological and anthropogenic factors will be a critical step in planning deep-sea benthic studies. For assessing community structure, application of the traditional approach and sieving sediments to a depth of 10 cm is acceptable (Montagna et al., 2016). However, to assess community function, it will be important that studies are designed to capture and hopefully provide more data on larger deeper burrowing macrofauna.
Data availability statement
The original contributions presented in the study are included in the article/Supplementary Material. Further inquiries can be directed to the corresponding author.
Ethics statement
The manuscript presents research on animals that do not require ethical approval for their study.
Author contributions
SS: Conceptualization, Data curation, Formal Analysis, Investigation, Methodology, Project administration, Resources, Software, Supervision, Validation, Visualization, Writing – original draft, Writing – review & editing. MG: Formal Analysis, Investigation, Writing – review & editing. JG: Conceptualization, Funding acquisition, Investigation, Methodology, Resources, Supervision, Writing – review & editing. DC: Methodology, Project administration, Resources, Supervision, Writing – review & editing.
Funding
The author(s) declare financial support was received for the research, authorship, and/or publication of this article. Support for this review paper was provided by INSPIRE Environmental.
Acknowledgments
We thank A. Murphy and M. Solan for helpful critique and critical discussions, and S. Sabo for support with figure development.
Conflict of interest
Authors SS, MG, JG, and DC founded the company INSPIRE, which was the funding source for the authors’ time and the publishing fees. The company had no other involvement in the study.
Publisher’s note
All claims expressed in this article are solely those of the authors and do not necessarily represent those of their affiliated organizations, or those of the publisher, the editors and the reviewers. Any product that may be evaluated in this article, or claim that may be made by its manufacturer, is not guaranteed or endorsed by the publisher.
Supplementary material
The Supplementary Material for this article can be found online at: https://www.frontiersin.org/articles/10.3389/fmars.2024.1383754/full#supplementary-material
Footnotes
- ^ *A linear regression was conducted to determine if there was a correlation between TOC% and Bioturbation Depth. This analysis utilized station location independent data, and the residuals were determined to be random, (i.e. independent variables drawn from a normal [0, sigma] distribution).
References
Aller R. C. (1978). Experimental studies of changes produced by deposit feeders on pore water, sediment and overlying water chemistry. Am. J. Sci. 278, 1185–1234. doi: 10.2475/ajs.278.9.1185
Aller J., Woodin S., Aller R. (2001). Organism-sediment interactions (Columbia: University of South Carolina Press), 403 pp.
Atlas R. M., Hazen T. C. (2011). Oil biodegradation and bioremediation: A tale of the two worst spills in U.S. history. Environ. Sci. Technol. 45, 6709–6715. doi: 10.1021/es2013227
Baguley J. G., Montagna P. A., Hyde L. J., Kalke R. D., Rowe G. T. (2006). Metazoan meiofauna abundance in relation to environmental variables in the northern Gulf of Mexico deep sea. Deep Sea Res. I 53, 1344–1362. doi: 10.1016/j.dsr.2006.05.012
Blake J. A., Grassle J. F. (1994). Benthic community structure on the U.S. South Atlantic slope off the Carolinas: Spatial heterogeneity in a current-dominated system. Deep Sea Res. II 41, 835–874. doi: 10.1016/0967-0645(94)90051-5
Blake J. A., Hilbig B. (1994). Dense infaunal assemblages on the continental slope off Cape Hatteras, North Carolina. Deep Sea Res. II 41, 875–899. doi: 10.1016/0967-0645(94)90052-3
Brandt A., Gooday A. J., Brandão S. N., Brix S., Brökeland W., Cedhagen T., et al. (2007). First insights into the biodiversity and biogeography of the Southern Ocean deep-sea. Nature 447, 307–310. doi: 10.1038/nature05827
Continental Shelf Associates, Inc (2006). Effects of Oil and Gas Exploration and Development at Selected Continental Slope Sites in the Gulf of Mexico. Volume I: Executive Summary (New Orleans, LA: U.S. Department of the Interior, Minerals Management Service, Gulf of Mexico OCS Region), 45 pp. Available at: https://www.boem.gov/Gulf-of-Mexico-OCS-Region-Publications-B/. OCS Study MMS 2006–044.
Costello M. J., Chaudhary C. (2018). Marine biodiversity, biogeography, deep-sea gradients, and conservation. Curr. Biol. 27, 511–527. doi: 10.1016/j.cub.2017.04.060
Danovaro R., Aguzzi J., Fanelli E., Billett D., Gjerde K., Jamieson A., et al. (2017). An ecosystem-based deep-ocean strategy. Science 355, 452–454. doi: 10.1126/science.aah7178
Danovaro R., Snelgrove P. V. R., Tyler P. (2014). Challenging the paradigms of deep-sea ecology. Trend Ecol. Evol. 29, 465–475. doi: 10.1016/j.tree.2014.06.002
Diaz R. J., Cutter G. R., Rhoads D. C. (1994). The importance of bioturbation to continental slope sediment structure and benthic processes off Cape Hatteras, North Carolina. Deep-Sea Res. II 41, 719–734. doi: 10.1016/0967-0645(94)90044-2
Drazen J. C., Baldwin R. J., Smith K. L. (1998). Sediment community response to a temporally varying food supply at an abyssal station in the NE Pacific. Deep-Sea Res. II 45, 893–913. doi: 10.1016/S0967-0645(98)00007-1
Druffel E. R. M., Robison B. H. (1999). Is the deep sea on a diet? Science 284, 1139–1140. doi: 10.1126/science.284.5417.1139
Druffel E. R. M., Williams P. M., Susuki Y. (1989). Concentrations and radiocarbon signatures of dissolved organic matter in the Pacific Ocean. Geophys. Res. Lett. 16, 991–994. doi: 10.1029/GL016i009p00991
Germano J. D., Rhoads D. C., Valente R. M., Carey D. C., Solan M. (2011). The use of sediment profile imaging (SPI) for environmental impact assessments and monitoring studies: lessons learned from the past four decades. Oceanogr. Mar. Biol. 49, 235–298. doi: 10.1201/b11009-7
Glover A. G., Gooday A. J., Bailey D. M., Billett D. S., Chevaldonné P., Colaço A., et al. (2010). Temporal change in deep-sea benthic ecosystems: a review of the evidence from recent time-series studies. Adv. Mar. Biol. 58, 1–95. doi: 10.1016/B978-0-12-381015-1.00001-0
Glover A. G., Smith C. R. (2003). The deep-sea floor ecosystem: current status and prospects of anthropogenic change by the year 2025. Env. Conserv. 30, 219–241. doi: 10.1017/S0376892903000225
Gooday A. J., Pfannkuche O., Lambshead P. J. D. (1996). An apparent lack of response by metazoan meiofauna to phytodetritus deposition in the bathyal north-eastern Atlantic. J. Mar. Biol. Ass. UK 76, 297–310. doi: 10.1017/S0025315400030563
Graf G. (1989). Benthic-pelagic coupling in a deep-sea benthic community. Nature 341, 437–439. doi: 10.1038/341437a0
Grassle J. F., Grassle J. P. (1994). “Notes from the abyss: the effects of a patchy supply of organic material and larvae on soft-sediment benthic communities,” in Aquatic Ecology: Scale, Pattern and Process. Eds. Giller P. S., Hildrew A. D., Raffaelli D. G. (Blackwell, Oxford UK), 499–515.
Growcock F. B., Andrews S. L., Frederick T. P. (1994). “Physicochemical properties of synthetic drilling fluids,” in Paper presented at the IADC/SPE Drilling Conference, Dallas, Texas, February 1994. doi: 10.2118/27450-MS
Guarinello M. L., Sturdivant S. K., Murphy A. E., Brown L., Godbold J. A., Solan M., et al. (2022). Evidence of rapid functional benthic recovery following the deepwater horizon oil spill. Envi Sci Tech. 2 (10), 1760–1771. doi: 10.1021/acsestwater.2c00272
Honjo S., Manganini S. J., Krishfield R. A., Francois R. (2008). Particulate organic carbon fluxes to the ocean interior and factors controlling the biological pump: A synthesis of global sediment trap programs since 1983. Prog. Oceanogr. 76, 217–285. doi: 10.1016/j.pocean.2007.11.003
Jahnke R. A., Reimers C. E., Craven D. B. (1990). Intensification of recycling of organic matter at the sea floor near ocean margins. Nature 348, 50–54. doi: 10.1038/348050a0
Joye S. (2016). The Gulf of Mexico ecosystem – Before, during and after the Deepwater Horizon oil well blowout. Deep-Sea Res. II 129, 1. doi: 10.1016/j.dsr2.2016.04.022
Kennicutt M. C. (2017). “Oil and gas seeps in the Gulf of Mexico,” in Habitats and Biota of the Gulf of Mexico: Before the Deepwater Horizon Oil Spill. Ed. Ward C. (Springer, New York, NY).
Le Saout S., Hoffmann M., Shi Y., Hughes A., Bernard C., Brooks T. M., et al. (2013). Protected areas and effective biodiversity conservation. Science 342, 803–805. doi: 10.1126/science.1239268
Levin L. A., Baco A. R., Bowden D. A., Colaço A., Cordes E. E., Cunha M. R., et al. (2016). Hydrothermal vents and methane seeps: Rethinking the sphere of influence. Front. Mar. Sci. 3, 1–23. doi: 10.3389/fmars.2016.00072
Levin L. A., Le Bris N. (2015). The deep ocean under climate change. Science 350, 766–768. doi: 10.1126/science.aad0126
Lohrer A. M., Thrush S. F., Gibbs M. M. (2004). Bioturbators enhance ecosystem function through complex biogeochemical interactions. Nature 431, 1092–1095. doi: 10.1038/nature03042
Love C. R., Arrington E. C., Gosselin K. M., Reddy C. M., Van Mooy B. A. S., Nelson R. K., et al. (2021). Microbial production and consumption of hydrocarbons in the global ocean. Nat. Microbiol. 6, 489–498. doi: 10.1038/s41564–020-00859–8
Marcus J., Tunnicliffe V., Butterfield D. A. (2009). Post-eruption succession of macrofaunal communities at diffuse flow hydrothermal vents on Axial Volcano, Juan de Fuca Ridge, Northeast Pacific. Deep Sea Res. II 56, 1586–1598. doi: 10.1016/j.dsr2.2009.05.004
McNutt M. K., Camilli R., Crone T. J., Guthrie G. D., Hsieh P. A., Ryerson T. B., et al. (2012). Review of flow rate estimates of the Deepwater Horizon oil spill. Proc. Natl. Acad. Sci. 10, 20260–20267. doi: 10.1073/pnas.1112139108
Menzel D. W., Ryther J. H. (1970). Distribution and cycling of organic carbon in the oceans. Inst. Mar. Sci. Univ. Alaska Publ. 1, 31–54.
Meysman F. J. R., Middelburg J. J., Heip C. H. R. (2006). Bioturbation: a fresh look at Darwin’s last idea. Trends Ecol. Evol. 21, 688–695. doi: 10.1016/j.tree.2006.08.002
Montagna P. A., Baguley J. G., Hsiang C. Y., Reuscher M. G. (2016). Comparison of sampling methods for deep-sea infauna. Limnol. Oceanogr. Methods 15, 166–183. doi: 10.1002/lom3.10150
Nicholaus R., Lukwambe B., Zheng Z. (2023). The effects of Cyclina sinensis bioturbation on alkaline phosphatase and total microbial hydrolytic activities in marine clam–shrimp integrated ponds. FEMS Microbiol. Ecol. 97, 1–9. doi: 10.1093/femsec/fiad063
Nilsen M., Pedersen T., Nilssen E. M. (2006). Macrobenthic biomass, productivity (P/B), and production in a high-latitude ecosystem, North Norway. Mar. Ecol. Prog. Ser. 321, 67–77. doi: 10.3354/meps321067
Pearson T. H., Rosenberg R. (1978). Macrobenthic succession in relation to organic pollution. Oceanogr. Mar. Biol. Ann. Rev. 16, 229–311.
Pfannkuche O. (1993). Benthic response to the sedimentation of particulate organic matter at the BIOTRANS station 47° N, 20° W. Deep-Sea Res. II 40, 135–149. doi: 10.1016/0967-0645(93)90010-K
Ramirez-Llodra E., Tyler P. A., Baker M. C., Bergstad O. A., Clark M. R., Escobar E., et al. (2011). Man and the last great wilderness: human impact on the deep sea. PloS One 6, e22588. doi: 10.1371/journal.pone.0022588
Rex M. A., Etter R. J. (2010). Deep-sea biodiversity: Pattern and scale (Cambridge, Massachusetts, USA: Harvard University Press), 354 pp.
Rhoads D. C., Boyer L. F. (1982). “Effects of marine benthos on physical properties of sediments: a successional perspective,” in Animal-Sediment Relations. Eds. McCall P. L., Tevesz M. J. S. (Plenum Press, New York), 3–51.
Rhoads D. C., Cande S. (1971). Sediment profile camera for in situ study of organism- sediment relations. Limnol. Oceanogr. 16, 110–114. doi: 10.4319/lo.1971.16.1.0110
Rowe G. T., Staresinic N. (1979). Sources of organic matter to the deep-sea benthos. Ambio. Special Rep. 6, 19–23. doi: 10.2307/25099603
Smith K. L., Baldwin R. J., Karl D. M., Boetius A. (2002). Benthic community response to pulses in pelagic food supply: North Pacific Subtropical Gyre. Deep-Sea Res. 49, 971–990. doi: 10.1016/S0967-0637(02)00006-7
Smith C. R., Bernardino A. F., Baco A., Hannides A., Altamira I. (2014). Seven-year enrichment: macrofaunal succession in deep-sea sediments around a 30 tonne whale fall in the Northeast Pacific. Mar. Ecol. Prog. Ser. 515, 133–149. doi: 10.3354/meps10955
Smith K. L., Henry A. R., Huffard C. L., Messie M., Kahru M. (2018). Episodic organic carbon fluxes from surface ocean to abyssal depths during long-term monitoring in NE Pacific. Proceed Natl. Acad. Sci. 115, 12235–12240. doi: 10.1073/pnas.1814559115
Snelgrove P. V. R. (1997). The importance of marine sediment biodiversity in ecosystem processes. Ambio 26, 578–583.
Snider L. J., Burnett B. R., Hessler R. R. (1984). The composition and distribution of meiofauna and nanobiota in a central North Pacific deep-sea area. Deep-Sea Res. 31, 1225–1249. doi: 10.1016/0198-0149(84)90059-1
Solan M., Germano J. D., Rhoads D. C., Smith C., Michaud E., Parry D., et al. (2003). Toward a greater understanding of pattern, scale and process in marine benthic systems: a picture is worth a thousand worms. J. Exp. Mar. Biol. Ecol. 285–286, 313–338. doi: 10.1016/S0022-0981(02)00535-X
Sturdivant S. K., Diaz R. J., Cutter G. R. (2011). Bioturbation in a declining oxygen environment, in situ observations from Wormcam. PloS One 7, e34539. doi: 10.1371/journal.pone.0034539
Sweetman A. K., Thurber A. R., Smith C. R., Levin L. A., Mora C., Wei C.-L., et al. (2017). Major impacts of climate change on deep-sea benthic ecosystems. Elem. Sci. Anth. 5, 4. doi: 10.1525/elementa.203
Teal LR, Bulling MT, Parker ER, Solan M. (2008). Global patterns of bioturbation intensity and mixed depth of marine soft sediments. Aquat Biol. 2, 207–218. doi: 10.3354/ab00052
Thurber A. R., Sweetman A. K., Narayanaswamy B. E., Jones D. O. B., Ingels J., Hansman R. L. (2014). Ecosystem function and services provided by the deep sea. Biogeosciences 12, e1001947. doi: 10.1371/journal.pbio.1001947
Tunnicliffe V., Embley R. W., Holden J. F., Butterfield D. A., Massoth G. J., Juniper S. K. (1997). Biological colonization of new hydrothermal vents following an eruption on Juan de Fuca Ridge. Deep Sea Res. I 44, 1627–1644. doi: 10.1016/S0967-0637(97)00041-1
Uchman A., Wetzel A. (2011). “Deep-sea ichnology: the relationships between depositional environment and endobenthic organisms,” in Developments in Sedimentology. Eds. HüNeke H., Mulder T. (Elsevier, Amsterdam), 517–556.
Van Dover C. L. (2000). The Ecology of Deep-sea Hydrothermal Vents (Princeton, USA: Princeton University Press), 474 pp.
Vetter E. W., Dayton P. K. (1998). Macrofaunal communities within and adjacent to a detritus-rich submarine canyon system. Deep-Sea Res. II 45, 25–54. doi: 10.1016/S0967-0645(97)00048-9
Wedding L. M., Reiter S. M., Smith C. R., Gjerde K. M., Kittinger J. N., Friedlander A. M., et al. (2015). Managing mining of the deep seabed. Science 349, 144–145. doi: 10.1126/science.aac6647
Witte U. (2000). Vertical distribution of metazoan macrofauna within the sediment at four sites with contrasting food supply in the deep Arabian Sea. Deep-Sea Res. II 47, 2979–2997. doi: 10.1016/S0967-0645(00)00055-2
Witte U., Wenzhöfer F., Sommer S., Boetius A., Heinz P., Aberle N., et al. (2003). In situ experimental evidence of the fate of a phytodetritus pulse at the abyssal sea floor. Nature 424, 763–766. doi: 10.1038/nature01799
Keywords: bioturbation, bioturbation activity, macrofauna, deep sea, human disturbance, sediment profile imaging, SPI
Citation: Sturdivant SK, Guarinello ML, Germano JD and Carey DA (2024) Reshaping perspectives of deep-sea benthic function. Front. Mar. Sci. 11:1383754. doi: 10.3389/fmars.2024.1383754
Received: 08 February 2024; Accepted: 12 June 2024;
Published: 01 July 2024.
Edited by:
Elva G. Escobar-Briones, National Autonomous University of Mexico, MexicoReviewed by:
Olmo Miguez-Salas, Senckenberg Nature Research Society, GermanyJesse Van Der Grient, South Atlantic Environmental Research Institute, Falkland Islands
Regan Nicholaus, Mbeya University of Science and Technology, Tanzania
Copyright © 2024 Sturdivant, Guarinello, Germano and Carey. This is an open-access article distributed under the terms of the Creative Commons Attribution License (CC BY). The use, distribution or reproduction in other forums is permitted, provided the original author(s) and the copyright owner(s) are credited and that the original publication in this journal is cited, in accordance with accepted academic practice. No use, distribution or reproduction is permitted which does not comply with these terms.
*Correspondence: S. Kersey Sturdivant, a2Vyc2V5QGluc3BpcmVlbnZpcm9ubWVudGFsLmNvbQ==