- 1National Oceanic and Atmospheric Administration (NOAA) Center for Coastal and Marine Ecosystems, Tallahassee, FL, United States
- 2Harte Research Institute for Gulf of Mexico Studies, Texas A&M University-Corpus Christi, Corpus Christi, TX, United States
- 3Palacios Marine Agricultural Research, Palacios, TX, United States
Competing uses for nearshore coastal space, such as aquaculture, have complex environmental and ecological interactions with surrounding seagrass meadows. Oyster aquaculture is among the fastest-growing aquaculture segments in the United States, and it brings concerns such as increased sedimentation from farm maintenance operations and altered water quality through oyster filtration. Changes in seagrass coverage and growth are common indicators of ecological health used to determine anthropogenic impacts on nearshore environments. This study characterized the effects of a research-scale adjustable long-line oyster aquaculture system on the health of adjacent seagrass meadows in Copano Bay, TX. Four Halodule wrightii meadows were identified at various distances from the research site: 100 m upstream (upstream), directly adjacent (0 m), 30 m downstream (30 m), and 60 m downstream (60 m). Sites were monitored for 1) seagrass health, 2) water quality parameters, and 3) sediment deposition. Over the 18-week sampling period, no significant differences (p>0.05) were found for water quality variables or sediment grain-size analysis using a one-way ANOVA. A linear mixed-effects model was used for repeated measures of seagrass data, with no effect of the site found on mean seagrass length, coverage, or maximum length (p>0.05). These results suggest that seagrass health indicators were unaffected by proximity to the oyster system. It was concluded that an oyster research aquaculture system of the type and size at the project location had no major negative or positive impact on seagrass meadow health due to high background variability and the overall minor footprint of the oyster farm across a comparatively large spatial extent.
1 Introduction
Seagrasses are keystone species vital to estuary and marine habitats’ health and ecosystem function (Hemminga and Duarte, 2000; Dawes et al., 2004). Seagrass meadows offer shelter and habitat for marine food webs necessary for biodiversity (Duffy, 2006), recreational and commercial fisheries (Saenger et al., 2013; Williams et al., 2016; Nordlund et al., 2018; Unsworth et al., 2019), and other keystone species such as sea urchins (Prado et al., 2009) and sea stars (Pinn et al., 2014; Galasso et al., 2015). Other ecosystem services provided by seagrasses include the stabilization of coastal sediment (De Boer, 2007; Meysick et al., 2022), nutrient cycling (Cuddy and Dunton, 2023), and carbon sequestration (Bedulli et al., 2020; Ricart et al., 2020). With favorable environmental conditions, healthy seagrass will generally have robust root systems, long leaf lengths, and dense coverage (Dunton, 1994; Wood and Lavery, 2000; Congdon et al., 2023). Seagrasses can propagate sexually via seed production or asexually through clonal growth. Increased sexual reproduction has been well documented to occur in response to severe environmental disturbances, presumably as a mechanism to withstand harsh conditions that may be temporary (Alexandre et al., 2005; Cabaço et al., 2009; Cabaço and Santos, 2012; Paulo et al., 2019; Meling-López et al., 2021). Anthropogenic stressors, however, such as eutrophication, runoff, dredging, or shading, have altered hydrodynamics and chemical water quality, inhibiting seagrass health and reproduction (Dunton, 1994; Fitzpatrick and Kirkman, 1995; Roca et al., 2016; Fraser and Kendrick, 2017).
A variety of indicators have been used to measure the morphology and health of seagrass beds, such as above and belowground biomass ratios (Lee and Dunton, 2000; Kim et al., 2013), biomass-density relationships (Cabaço et al., 2007; Vieira et al., 2018, 2022), efficiency of space occupation (Vieira et al., 2018, 2022), and the use of molecular and biochemical metrics coupled with multivariate analysis (Romero et al., 2007; Cabaço et al., 2009; Garcıa-Marín et al., 2013). Worldwide, seagrass coverage has been decreasing throughout the 20th century. Between 1940 and 1990, the total overall seagrass coverage decreased annually by about 1.5 percent, with that rate rising to a 7 percent annual decrease after 1990 (Waycott et al., 2009). Between 1880 and 2021, seagrass in 547 sites worldwide decreased on average by 19.1 percent, with the majority of this decrease happening in non-tropical areas (Dunic et al., 2021). Human development and changes to coastal land use and hydrology, such as sediment loading, can kill off seagrass meadows by decreasing light availability (Lee and Dunton, 1997; Waycott et al., 2009; Kim et al., 2015; Saunders et al., 2017; Mehrubeoglu et al., 2024). Other stressors result from nutrient over-enrichment, which can lead to increased epiphyte and macroalgae bloom that compete for space and nutrients with seagrasses or potentially smother them entirely (Burkholder et al., 1992, 2007; Van Katwijk et al., 2011; Han and Liu, 2014).
In some cases, seagrasses are showing resilience to human impacts. In Tampa Bay, total nitrogen input was reduced by 50% in the 1970s, leading to an increase in seagrass coverage of more than 65 percent since the 1980s (Greening et al., 2018). In another study, it was found that all seagrass meadows in a heavily impacted area of the U.K. have increased in size over the last 10-15 years, with most populations appearing robust and healthy despite the high levels of nutrients and turbidity in the water column (Bertelli et al., 2018). Seagrass meadows in various regions affected by human activities have demonstrated resilience when anthropogenic pressures are reduced, leading to a slower rate of decline compared to the 20th century (de Los Santos et al., 2019; Burdick et al., 2020; Orth et al., 2020). These studies show that seagrass meadows have expanded in some areas despite human impacts like nitrification and sedimentation. This suggests that seagrass can thrive with mild nutrient and sediment loads, restoring the ecosystem and improving habitat health (Crawford et al., 2003; Orth et al., 2020; Vieira et al., 2022; Hodgson and Bucher, 2023). Although some limited geographic areas have documented increases in seagrass coverage, density, and biomass, the majority of seagrass beds adjacent to human populations worldwide are negatively affected by anthropogenic impacts. In addition to changes in seagrass meadow coverage, stress to seagrass can also result in morphological changes that may be harder to detect and that have the potential to lead to decreased resilience to ensuing stress events (Olivé et al., 2007; McMahon et al., 2013; Barry et al., 2018).
Commercially farmed oysters are commonly spawned in hatcheries and then placed in the water, where they are grown using different methods specific to the farming region. In areas with hard, rocky substrates, on-bottom culture or bottom cages are typically used (Lu, 2015). For off-bottom culture, methods consisting of cages, rack and bag culture, tray culture, floating cages, and suspended culture are typically utilized (Walton et al., 2013; Lu, 2015). Adjustable long-line systems (ALS) designs are commonly used in soft substrate, in which oysters are stocked in cages suspended mid-water by a series of lines and pilings (Lavoie, 2005). The advantages of such suspended or floating aquaculture methods are lower mortality as cages are situated farther from benthic predation and have access to higher food concentrations (Lu, 2015). The use of “off-bottom” oyster aquaculture can also reduce disruptions of benthic organisms, such as seagrass beds (Dumbauld and McCoy, 2015), and can also reduce shading as compared to “on-bottom” cultivation methods by being situated high in the water column and moving with the wind and tides. However, some disruptions occur as workers may have to access cages on foot. Because of the impact some methods of oyster aquaculture can have on benthic habitats, substantial regulatory concern exists for how oyster aquaculture could affect seagrass, primarily with a focus on entrainment of sediment from the farm to adjacent native seagrass beds and shading of the benthic environment (Handley et al., 2007; Dunton et al., 2011).
Since the recent legalization of off-bottom oyster aquaculture in Texas (2019), the impacts of oyster aquaculture on native ecosystems in potential growing areas have become a more novel and emerging topic of discussion. Ecosystems such as seagrass (Kowalski et al., 2009; Dunton and Wilson, 2010; Larkin et al., 2017; Congdon et al., 2019) and oyster reefs (Stunz et al., 2010; Blomberg et al., 2018) play a key role in providing essential habitat to a variety of marine species. Due to the strong potential for oysters to be cultured in areas overlapping seagrass ecosystems, a knowledge base must be built describing how aquaculture operations could affect them. Some studies have found oyster aquaculture to negatively impact seagrass by reducing light availability to seagrasses either by directly blocking the light with oyster aquaculture gear (Skinner et al., 2013) or indirectly through waste, leading to higher phytoplankton and increasing turbidity (Pietros and Rice, 2003). However, other studies have found that oyster aquaculture in well-mixed bodies of water has minimal effect on nearby water quality parameters (Crawford et al., 2003; Turner et al., 2019) or that using oyster aquaculture and seagrasses grown together may offer the most sustainable outcomes for both humans and ecological function (Hori et al., 2019; Cravo et al., 2022). To better understand the anthropogenic effects of off-bottom oyster cultivation, this study evaluated the short-term impact of ALS oyster culture on the health status of established Halodule wrightii (Aschers) meadows in the immediate project area. The study was conducted during the summer growing season (July) through the onset of fall (November). This period was chosen because it closely aligned with the timeline that the oyster system was set up and because analysis during this season would highlight any above-ground biomass changes caused by the oyster system. Research like this study could, over time, help inform rules and regulations related to oyster aquaculture, seagrass sensitivity, and day-to-day management activities associated with oyster cage culture operations.
2 Materials and methods
2.1 Copano bay research site
Copano Bay is a 180-km² secondary bay system and estuary located within the Mission-Aransas National Estuarine Research Reserve (NERR), the eastern portion of which connects to Aransas Bay. Due to its shallow depth and NW/SE orientation, it is well mixed by prevailing southeast winds. Copano Bay has an average depth of 2m (Mooney and McClelland, 2012) with typically low freshwater inflow and high winds (Spalt et al., 2020). There is a prevailing wind direction between 105 and 165 degrees in Copano Bay during the summer, with a shift to an NE wind during the colder months (NOAA, 2023). Three primary freshwater sources enter the bay: Copano Creek, the Mission River, and the Aransas River. Copano Bay salinity varies drastically between seasons, ranging from near zero to greater than 40ppt, depending on rain, hurricane, and other conditions (Turner et al., 2016; Bugica et al., 2020). Because of this, ephemeral seagrass coverage has been extraordinarily variable throughout the years, with ecosystems shifting with the changing salinity (Kowalski and DeYoe, 2016; Congdon et al., 2019). It is also possible that species composition changes over time as changing water parameters become more or less tolerant to certain species (Darnell et al., 2021). The surrounding terrestrial landscape forming Copano Bay is primarily agricultural, consisting of scrubland (31.57%), pasture/hay (24.49%), and cultivated crops (22.74%) (Wagner and Moench, 2009).
2.2 Oyster farm description and study design
On June 25th, 2020, oysters (Crassostrea virginica) (Gmelin, 1971) were stocked directly in Copano Bay using three parallel 25 m ALS lines. There were 76 cages at a density of 80 adult oysters per cage (typically 7.6 cm in shell height) for a total of 6,080 adult oysters (Figure 1). The orientation of the research system was approximately 100 m offshore and parallel to the shoreline. Adult oysters were stocked to simulate a mature culture and to amplify potential impacts that the research-scale farm might have. Losses of individual oysters due to mortality were periodically replaced throughout the study to maintain consistent system biomass. Cages within the research system were adjusted to a depth of approximately 1-1.5 m and periodically desiccated for the removal of biofouling. Four sites were selected parallel to the shoreline, with seagrass at each of the four sites. One site (0 m) (28.063214, -97.1872) was adjacent to the oyster farm. Two sites were downstream of the oyster farm at 30 m (28.0633475, -97.1875568) and 60 m (28.063517, -97.187867). The final site (upstream) was 100 m (28.062792, -97.186144) upstream of the oyster farm. The four sites together create a transect parallel with the coastline going in the direction of the prevailing current.
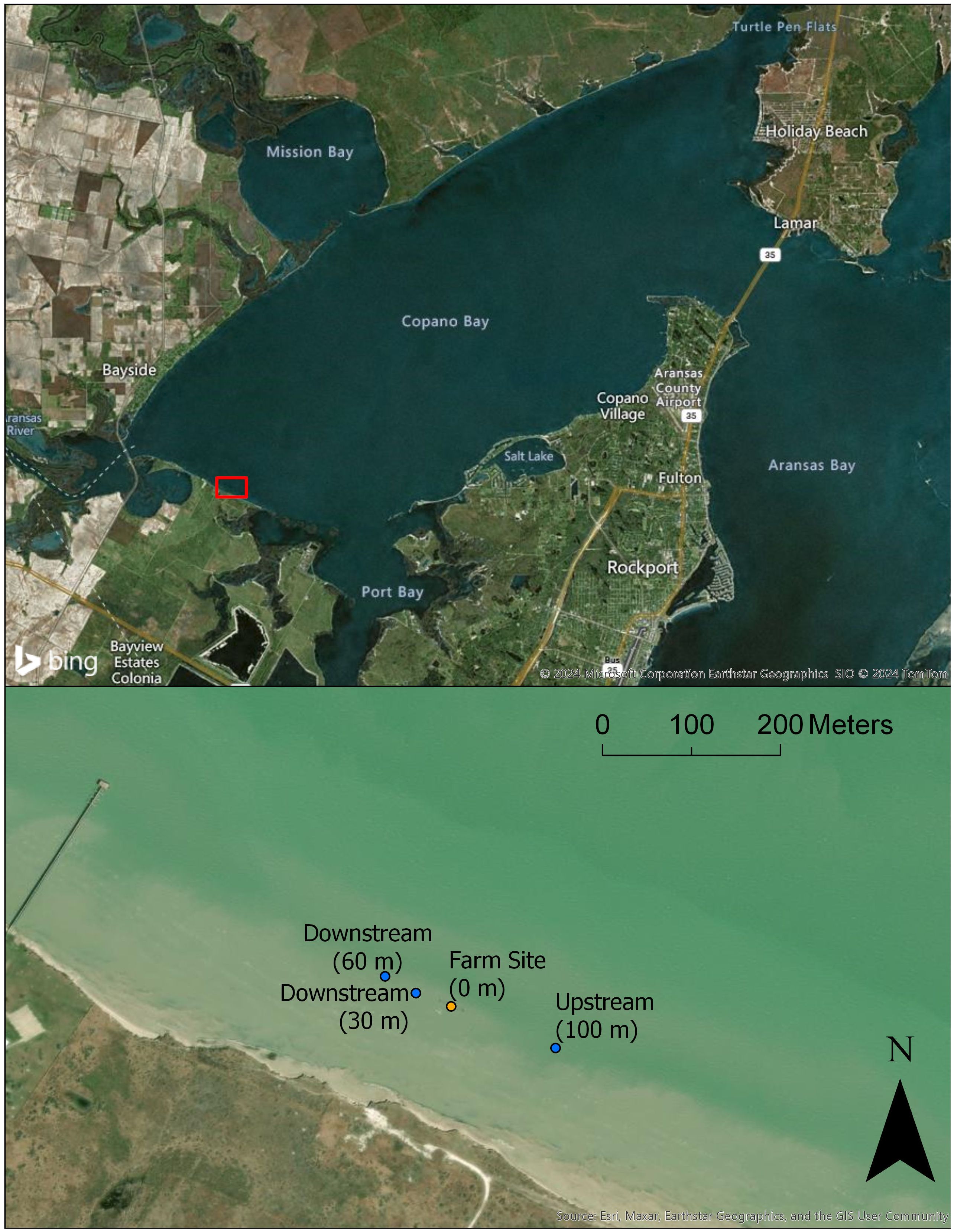
Figure 1. Copano Bay is a secondary bay system connected to Aransas Bay, with satellite imagery of each site (from left to right: 60m downstream, 30m downstream, 0m (orange), and 100m upstream).
2.3 Seagrass surveys
Preliminary surveys in April and May 2020 showed that seasonal, ephemeral seagrass meadows were beginning to sprout along the coast in the project area, providing an opportunity to initiate this study. All seagrass was identified as Halodule wrightii at the project site (Staugler, 2019) at a depth of 1-1.5 m accessible by wading from the shoreline without scuba equipment. To identify areas of seagrass at the project site that were both downstream and upstream of the oyster cages, an initial visual survey was conducted, followed by GPS recording. It was also noted that during warmer months, the prevailing current direction at the site is southeast (Mott and Lehman, 2005) and wind-driven, creating a calmer environment where these ephemeral seagrass patches can thrive. This was verified with data from wind direction collected from nearby Copano East and Copano West weather stations in the bay (NERR., n.d.) visualized with the “openair” R package (Carslaw and Ropkins, 2009) (Figure 2). Because currents in this bay are primarily wind-driven, the prevailing current direction was observed to typically align with the prevailing wind direction (southeast) along the coast. The hydrodynamic model TxBLEND also confirmed the current direction (Guthrie et al., 2010; Schoenbaechler et al., 2011). Once the predominant current direction was established and seagrass test meadows were identified, visual surveys were conducted on clear-water days.
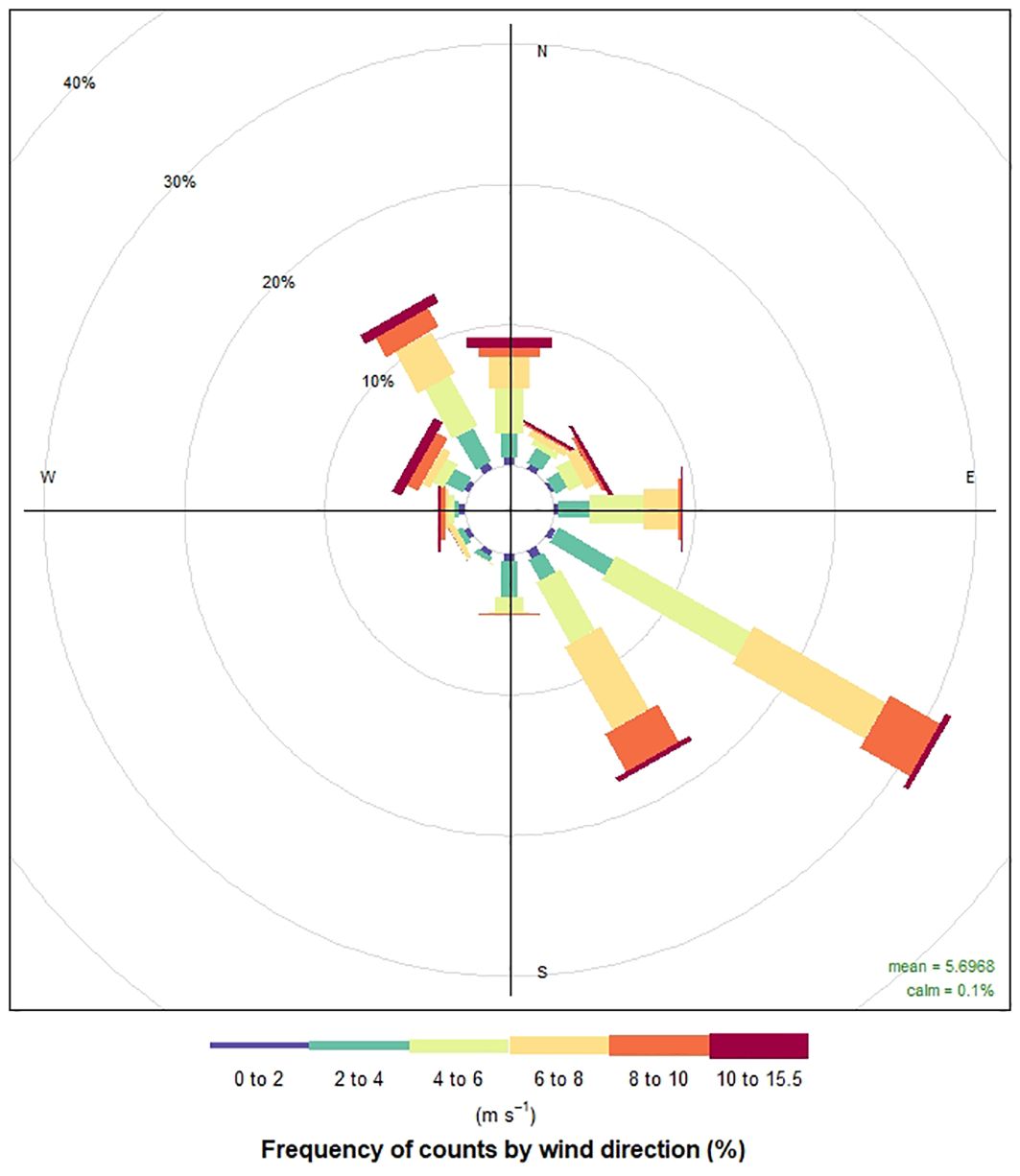
Figure 2. Chart of wind direction and speed (m s-1) throughout the timeframe of the experiment. Predominant southeast wind direction between 105 and 165 degrees is shown (NOAA, 2023).
Seagrass meadows in close proximity to the cages (within 2 m), 30 m downstream, 60 m downstream, and 100 m upstream from the system were marked and named for ease of identification (0 m, 30 m, 60 m, and Upstream). Seagrass sampling began on August 6th, 2020, and ended on October 22nd, 2020. The test meadow 100 m upstream from the system was chosen as an “untreated” control. The original research design included Before-after-control-impact (BACI), but due to factors not controllable by this study, sampling of seagrass sites began after the farm had been fully installed and stocked, as opposed to before the construction of the farm would be preferable. All test meadows were chosen to have similar depths and marked with transect lines mounted on two PVC posts per site. The PVC posts act as a visual indicator to ensure that trampling of seagrass beds does not occur or otherwise influence the study. Indicators of seagrass health at sampling sites included mean leaf length, coverage, and maximum leaf length (Heidelbaugh and Nelson, 1996). A 0.25 m by 0.25 m PVC quadrat divided into nine sections was used to determine the coverage (visually estimated) of test meadows following the methods of Dunton et al. (2011). Four random distance (but within 3m) placements of the quadrats in each respective seagrass test meadow were performed weekly along the transect line.
2.4 Water quality
Water quality parameters (e.g., temperature, salinity, dissolved oxygen (DO), and turbidity) were determined weekly beginning on August 6th, 2020, and ending on October 22nd, 2020 (n = 1 per sample seagrass meadow site) using a YSI Pro DSS data sonde (Yellow Spring Instruments, Yellow Springs, OH) (Table 1). Light intensity and temperature readings were taken every hour using a HOBO Pendant® Temperature/Light 8K Data Logger (Onset Computer Corporation, Bourne, MA) placed just above the seagrass surface, at the same depth, facing upright, at each of the four meadow sites and were regularly cleaned of biofouling. With Copano Bay’s minimal tidal range and strong winds, the tidal effect on depth here was negligible. The 0 m site loggers were placed beneath the oyster cage lines. Dissolved inorganic nutrients (e.g., total ammonia-nitrogen, nitrite plus nitrate-nitrogen, and phosphate) were determined on a twice-monthly basis by Woods Hole Oceanographic Institute using the Seal Analytical AutoAnalyzer Method G-171-96 (nitrate plus nitrite and ammonia) and Seal Analytical Continuous-Flow Analyzers Method G-297-03 (phosphate) from water samples taken (Seal Analytical, Mequon, WI) Data from the water samples (data sonde and grab-type) were acquired in 200ml bottles, 100 m upstream from the cage study and at 0 m, 30 m, and 60 m intervals downstream within the test seagrass meadows. All samples were filtered and frozen, then overnighted for processing. The sampling schedule for water samples coincided with another project and, therefore, differed slightly from the seagrass sampling schedule.
2.5 Sediment sampling
To evaluate the impact of sediment from routine farming activities on seagrass health, sediment traps (Eadie et al., 1991; Evans et al., 1998; Muzzi and Eadie, 2002) were deployed at the oyster culture system, 30 m downstream, 60 m downstream, and 100 m upstream within the test seagrass meadows with sampling between July 28th, 2020 through October 22nd, 2020. The sediment traps were constructed of 7.5-cm diameter PVC pipe fitted with a 7.5-cm diameter funnel inserted and glued into the opening to baffle wave action inside the trap and to prevent waves from washing out any collected sediment. Glass jars were inserted underneath the funnel to act as removable collection containers. The sediment content of these traps was analyzed at least twice monthly, more frequently, to account for weather events. The glass collection jars were removed, capped, bagged, and returned to the laboratory for immediate analysis. Contents were placed in tared ceramic drying pans and dried at 60°C for 72 hrs. The total weight of dried trap sediment was then determined. Dried sediment was sieved through sizes 5, 10, 35, 60, 120, and 230 sieves, and weights were recorded for each category. This data was reported as a percentage of total sediment weight by particle size.
2.6 Statistical analysis
Two statistical analyses were conducted in this study. First, a series of one-way ANOVAs was used to evaluate differences in water quality and sediment loading variables across four sites. Specifically, ten one-way ANOVAs were conducted for each dependent variable related to water quality, while seven additional ANOVAs were performed for sediment variables. All data were assessed for normality and homoscedasticity prior to analysis. The one-way ANOVA was performed using the “glht” function in the “multcomp” package in R (Hothorn et al., 2008; Pinheiro, 2009; Pinheiro et al., 2012). Post hoc comparisons between site means were made using Tukey’s HSD test and pairwise t-tests with Bonferroni correction. To analyze repeated measures data on seagrass health (mean leaf length, coverage, and maximum leaf length) at each site, a linear mixed-effects model was employed using the “nlme” package (Bates et al., 2015). This approach allowed the assessment of site effects on each seagrass health metric. All statistical analyses were performed in R (Version 4.0.3; Team, 2020), with significance set at α = 0.05.
3 Results
3.1 Water quality
There were no significant differences in mean turbidity, dissolved oxygen saturation, dissolved oxygen concentration, salinity, incident light, water temperature, nitrate + nitrite, ammonium, or phosphate among the four sampling sites regardless of proximity or orientation with the model oyster aquaculture site (Table 2).
3.2 Seagrass
Seagrass meadow health was evaluated using mean leaf length, coverage, and maximum leaf length. Mean leaf lengths were similar among sites, at 13.72, 14.12, 13.73, and 16.00 cm at the 100m upstream site, 0 m, 30 m, and 60 m sites, respectively (Table 3). Mean leaf length and seagrass coverage generally increased at most sites from early August to mid-September but sharply decreased after two major storms (September 19-22, mid-October) (Figures 3, 4). The linear mixed-effect model was used to detect whether the sites impacted each of the seagrass variables across repeated measures metrics. The results of the linear mixed-effects model for mean seagrass length (p = 0.397), coverage (p = 0.138), and maximum leaf length (p = 0.238) were not statistically significant, indicating that the site did not significantly influence any of these metrics (p > 0.05) (Table 4).
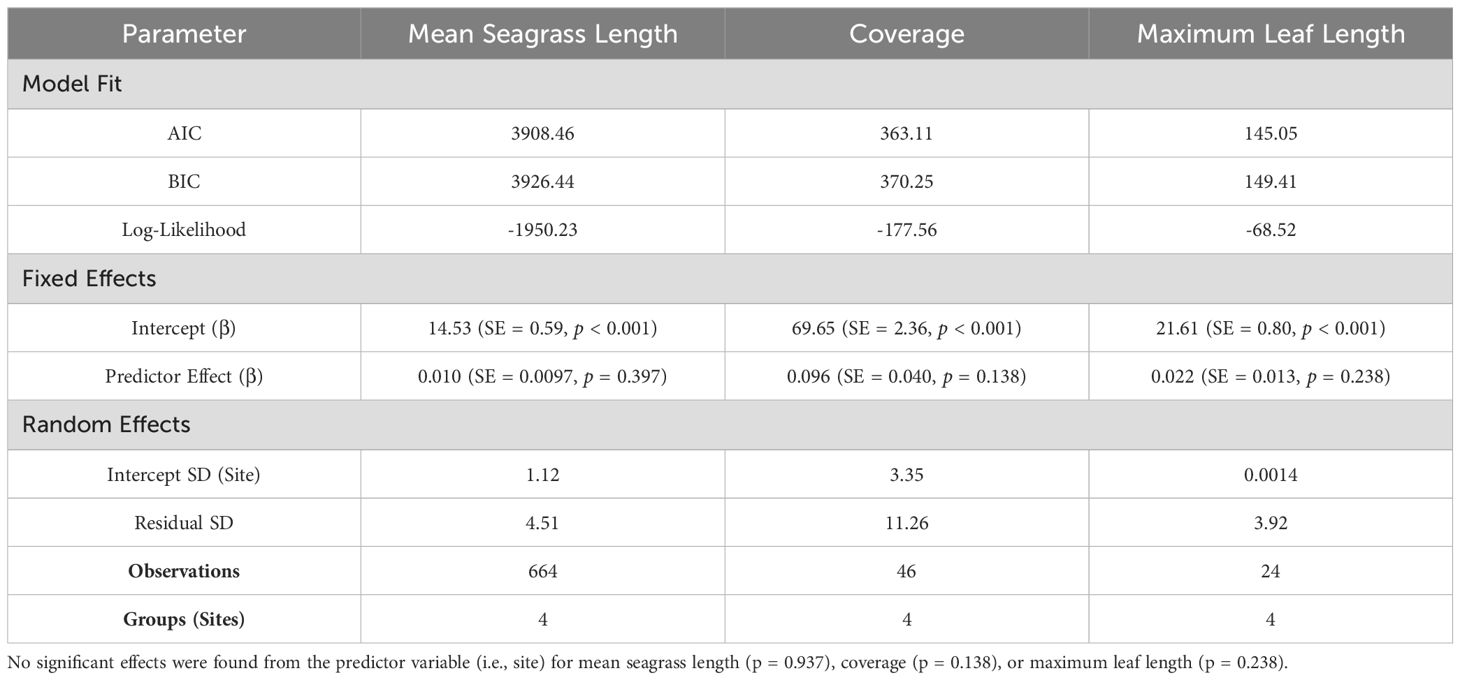
Table 4. Results of repeated measures linear mixed-effects models fit by REML on mean seagrass length, coverage, and maximum leaf length.
3.3 Sediment
The mean sediment deposition rate ranged from 9.15 to 11.42 g/day (Figure 5). More than half of the sediment (50.1–57.4%) was collected in the 250-µm to 125-µm size range across all sites (Figure 6). No significant differences in sediment particle size distribution were found among sites (p > 0.05). However, ANOVA revealed a significant difference in overall sediment deposition among sites (p = 0.0377) (Table 5). Despite this, post hoc analyses using Tukey’s HSD and pairwise t-tests with Bonferroni correction found no statistically significant pairwise differences in sediment deposition between any of the four sites (all p-values > 0.05) (Supplementary Tables A4, A5).
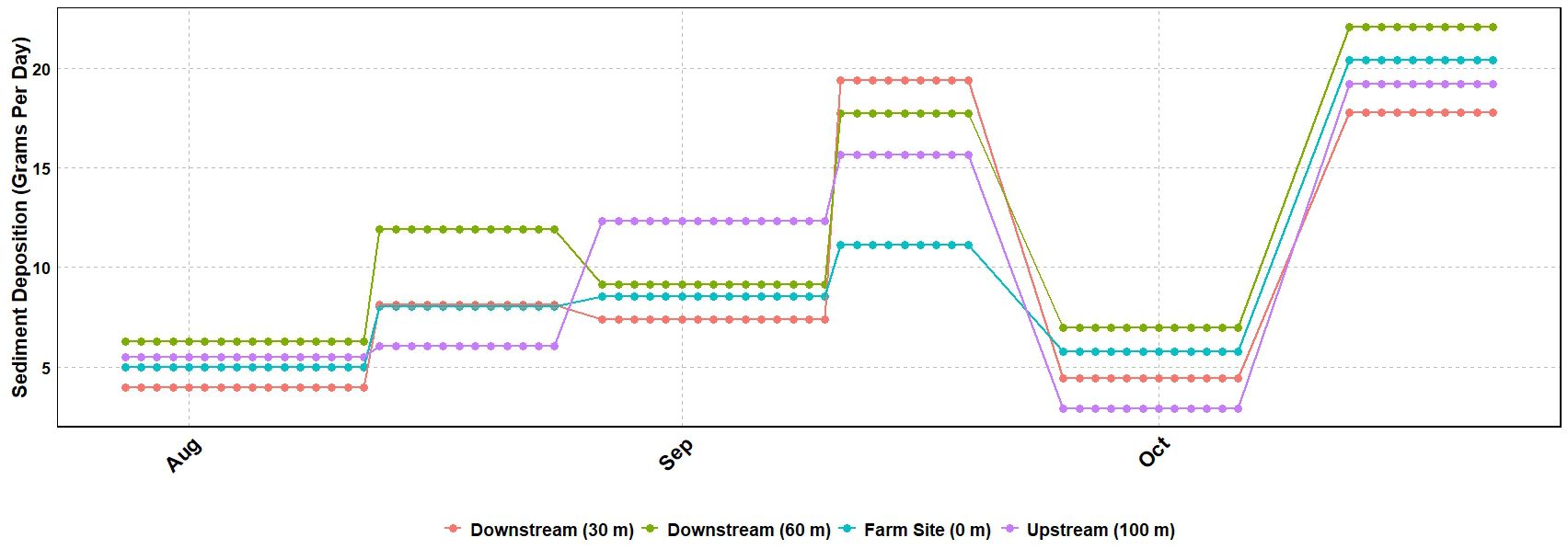
Figure 5. Mean (n=6) sediment deposited per day overtime at each seagrass site. Values show the total sediment captured during the deployment divided by the number of days the trap was deployed.
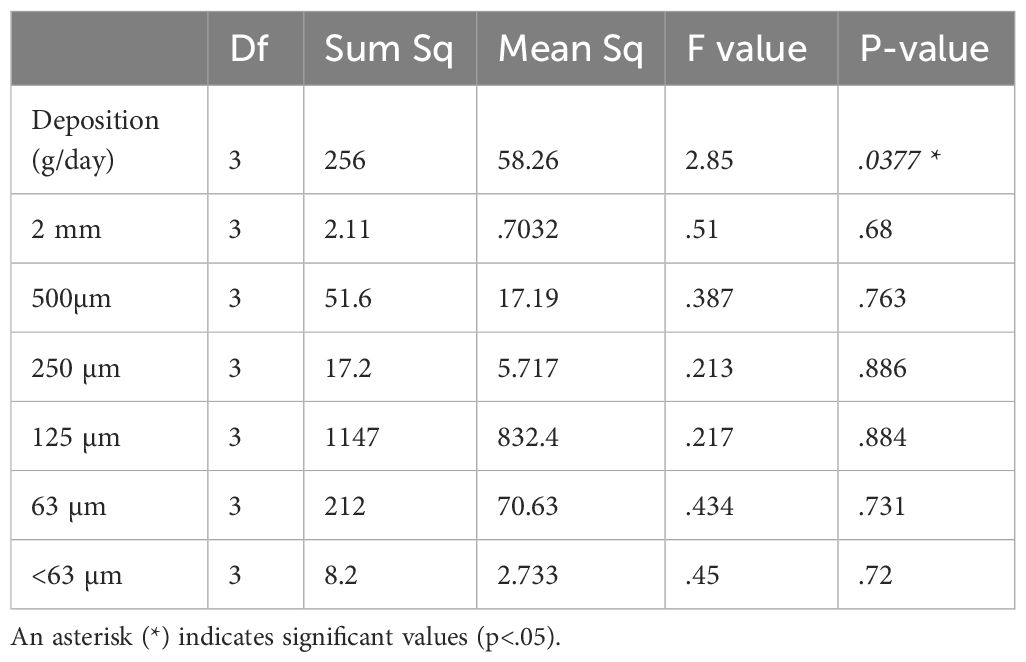
Table 5. ANOVA of sediment deposition variables, where deposition (.0377) was found to be significant among sites.
4 Discussion
4.1 Wind-driven vertical mixing and sediment loading
The present study focused on the potential effects of ALS cage farming of oysters on seagrass meadows. In the immediate area (maximum 100 m) from the research oyster operation, there were no significant differences in turbidity, temperature, light penetration, salinity, dissolved oxygen concentration, and percent saturation during the 18-week study. These findings indicate that human activities associated with access and management of the research system had no measurable effect on these factors. Due to the shallow water depth (1.0 - 1.5 m) and high turbidity driven by fetch (Cho, 2007), wind-driven vertical mixing and substantial longshore-oriented current were likely to offset these factors (Soria et al., 2021). Excess sediment loading is known to be detrimental to seagrass habitats through many studies of intensive anthropogenic activity (Dunton et al., 2003; De Boer, 2007; Van Katwijk et al., 2011; Saunders et al., 2017). Studies directly measuring sediment loading as a result of bivalve aquaculture typically occur at a much smaller scale compared to other anthropogenic activities such as dredging or construction (Onuf, 1994; Erftemeijer and Lewis, 2006). Impacts of bivalve aquaculture typically exhibit minor impacts on the benthic environment, although these results are highly dependent on experiment size and local ecosystem conditions (Bertin and Chaumillon, 2006; Mallet et al., 2006; Comeau et al., 2014; Testa et al., 2015). Stress to seagrass due to sedimentation from oyster farming is more likely to occur on larger farms at high densities, the use of more mechanized equipment involved with infrastructures and pilings, in systems with lower flushing potential, and dependence on gear type (Turner et al., 2019; Muething et al., 2020; Gadeken et al., 2021).
4.2 Nutrient impacts to seagrass meadows
Excess nutrients in the water column can directly influence growth patterns and morphometrics (Lee and Dunton, 2000) or lead to algal bloom or epiphyte shading that impacts seagrass resilience (Valiela et al., 1997). Eutrophication can further escalate drastic changes in the community composition of seagrasses and associated species (Deegan et al., 2002; Gil et al., 2006; Eklöf et al., 2009; Pérez-Ruzafa et al., 2012). Oysters are well-documented buffers against excess nutrient loading, consuming nitrogen and phosphorus-containing phytoplankton through the water column and sequestering it into tissue and shell (Kellogg et al., 2014; Bricker et al., 2014, 2018; Cubillo et al., 2023). On the other hand, oysters produce waste (feces, pseudofeces, and urea) that can harm seagrasses in high concentrations (Buzin et al., 2015; Pietros and Rice, 2003). However, this waste is rapidly absorbed by phytoplankton, algae, and bacteria, contributing to nutrient cycling (Pietros and Rice, 2003; Buzin et al., 2015; Moreno-Marín et al., 2016; Gray et al., 2022). Nutrient levels were not significantly or noticeably different among the four sampling sites, with the exception of nitrate plus nitrite, which was detectable at the 0 m site and barely detectable at the 60 m site but undetectable at the other sites. As nutrients and other water quality factors were similar, there were no significant differences in seagrass biomass or coverage between the sites.
Within this study, seagrass damage from storms had a more considerable impact than any impact from the model oyster farm. Seagrass damage from severe weather in the Gulf of Mexico is a common occurrence, which has accelerated from climate changes influencing the El Niño‐Southern Oscillation (ENSO) atmospheric patterns (Fodrie et al., 2010; Congdon et al., 2019, 2023). Subsequent decreases in leaf length can be seen from Tropical Storm Beta (September 19-22) and an unnamed storm towards the end of October (Figure 3). Severe weather has been observed to damage seagrasses through physical wave energy, destroying shoots directly or via sediment erosion, leaving seagrasses prone to uprooting (Congdon et al., 2019; Wilson et al., 2020). Sediment and seagrass biomass were not measured in this study, but future studies should aim to quantify sediment nutrient composition changes over time that are associated with commercial oyster aquaculture systems.
4.3 Balancing human needs and ecological preservation
Scholars have identified several pathways through which oyster aquaculture can influence seagrass habitats both positively and negatively, although much of the research findings include negligible or minor impacts (Crawford et al., 2003; Wagner et al., 2012; Smith et al., 2018; Turner et al., 2019). Individual studies typically provide valuable yet highly localized information that can be affected by seasonal patterns or site-specific factors. However, these studies can also be synthesized in a broader approach to identify techniques that minimize aquaculture effects on native seagrasses. Some identified practices to lessen the impact of commercial oyster aquaculture include site selection to avoid sensitive seagrass habitats, the use of off-bottom culture methods to help protect benthic habitats, and appropriate densities of cultured species to reduce competition and other environmental impacts (Booth and Heck, 2009; Tallis et al., 2009; Dumbauld et al., 2009; Dumbauld and McCoy, 2015; Ferretto et al., 2022).
In addition to the spatial expansion of aquaculture as a matter of food security and economic activity, other concepts of sustainability are also advancing bivalve aquaculture. Additional emphasis on expanding bivalve aquaculture exists through the growing attention to sustainable “no feed” aquaculture systems, generally more sustainable than other forms of fed aquaculture (Tacon and Metian, 2015; Cottrell et al., 2020). The utilization of bivalves as a method for the enhancement of water quality has also been championed as a prominent ecosystem function of the cultured species (Bricker et al., 2018, 2020; Ayvazian et al., 2021) These aspects, among others, provide an added level of intricacy when balancing multiple approaches to sustainability within limited and highly valuable estuarine environments.
4.4 Future study design considerations
One limitation of the present study was the spatial scale of the oyster culture system. As the culture system was originally designed as a research farm to evaluate whether farming oysters could be successful in Copano Bay, it was significantly smaller than a commercial-scale oyster farm and its culture systems. Any impact that the system and farm activities had on surrounding waters and seagrass meadows was smaller and more challenging to detect than at a commercial-scale farm. This farm had a total of 76 cages with a total of 6,080 adult oysters. A commercial farm might have over 500,000 oysters of various sizes in the same area. Adult oysters were used for the project to increase the system’s bio-load to make any impacts that the system might have had on seagrass more noticeable. In a commercial farm, there would be many more oysters ranging in size from seed (about 6-10mm) to market size (about 65 mm), and there would be many more pilings, cages, and lines depending on the style of the oyster farm. In the future, as more commercial-scale farms are established in Texas, similar studies should be repeated in much larger areas and over longer timescales. These longer, larger studies would highlight slight differences over smaller areas and time scales. Changes to seagrass health, water quality, or sedimentation may take several months or years to show significant measurable change, and additional studies across longer spatial scales can aid future management and conservations of seagrass when their habitats are shared with cultivated species, in particular aquaculture where large populations can be introduced quickly.
Additionally, other studies have used oysters in closer proximity over seagrass, which could be used to show greater relationships between the effects of both organisms on each other and the impacts of equipment on sediment transport and local hydrology (Booth and Heck, 2009). Smaller estuarine systems with less background variability and mixing (i.e., lagoons, saltwater ponds, or other waterbodies with low mixing) may be used to highlight ecological interactions. Extending the length of the study across multiple repeated seasons (i.e., multiple summers) may also highlight any long-term changes commonly seen in belowground, perennial biomass. Due to the ephemeral nature of seagrass beds in Copano Bay, long-term studies are still needed to characterize effects on seagrass beds via monitoring over multiple seasons with high and low environmental conditions. Future studies should also begin collecting baseline results far in advance of the addition of oyster aquaculture systems to better assess changes caused by the aquaculture system. This was not possible in this study but would provide extremely valuable information in future studies. Belowground biomass should also be considered in future works, as this would elucidate any changes or stress responses relating to anthropogenic impacts on belowground biomass that this current study was unable to observe.
5 Conclusion
The oyster culture system at the study site did not significantly affect seagrass health for the 18-week project duration. The seagrass presence appeared to be impacted more by aeolian forces and their influence on current and sediment transport. At present, in Texas, location of oyster farms is not permitted within 60 m of seagrass beds. The results of this study set up a first look at some of the effects that oyster aquaculture could have on seagrass ecosystems and highlight the research needs that future studies should focus on. Although the results could be interpreted as supportive of the conclusion that oyster farming’s potential impacts on seagrass could be avoided, or at least reduced, by situating farms in shallow, wind-driven bays, more research is needed across different and larger spatial areas, and using longer timescales to substantiate this conclusion. This study also shows that seagrass in slightly more sedimented areas can grow and thrive in high-wave action bays such as Copano Bay. Based on the results of the present study, it appears that any effects from the research oyster culture system were not noticeable in the sample sites. Therefore, the current 60 m buffer zone may be considered adequate in situating small, research-scale oyster farms where no effects (either positive or negative) will be detectable on nearby natural seagrass. Conducting a similar study with similar independent and dependent variables, but at a commercial scale, could further elucidate the relationship between commercial oyster farming in ALS-type cages and seagrass health. It is also recommended that similar studies be conducted with farming other gear types, gear such as on-bottom and floating cages, and within regions containing other species of seagrass. Seagrass has the potential to be resilient to various anthropogenic impacts; therefore, identifying, understanding, and mitigating the impacts of aquaculture activities on seagrasses is critical to support balancing human and ecological needs.
Data availability statement
The original contributions presented in the study are included in the article/Supplementary Material. Further inquiries can be directed to the corresponding author.
Author contributions
RR: Conceptualization, Data curation, Formal analysis, Methodology, Project administration, Software, Validation, Writing – original draft, Writing – review & editing. AL: Data curation, Project administration, Software, Supervision, Visualization, Writing – original draft, Writing – review & editing. JF: Conceptualization, Funding acquisition, Investigation, Methodology, Project administration, Resources, Supervision, Writing – review & editing.
Funding
The author(s) declare financial support was received for the research, authorship, and/or publication of this article. This publication was made possible by the National Oceanic and Atmospheric Administration, Office of Education Educational Partnership Program with Minority Serving Institutions award (NA16SEC4810009 and NA21SEC4810004). Its contents are solely the responsibility of the award recipient and do not necessarily represent the official views of the U.S. Department of Commerce, National Oceanic and Atmospheric Administration. Any opinions, findings, conclusions, or recommendations expressed in this publication are those of the author(s) and do not necessarily reflect the view of the U.S. Department of Commerce, National Oceanic and Atmospheric Administration.
Conflict of interest
The authors declare that the research was conducted in the absence of any commercial or financial relationships that could be construed as a potential conflict of interest.
Publisher’s note
All claims expressed in this article are solely those of the authors and do not necessarily represent those of their affiliated organizations, or those of the publisher, the editors and the reviewers. Any product that may be evaluated in this article, or claim that may be made by its manufacturer, is not guaranteed or endorsed by the publisher.
Supplementary material
The Supplementary Material for this article can be found online at: https://www.frontiersin.org/articles/10.3389/fmars.2024.1382153/full#supplementary-material
References
Alexandre A., Santos R., Serrão E. (2005). Effects of clam harvesting on sexual reproduction of the seagrass Zostera noltii. Mar. Ecol. Prog. Ser. 298, 115–122. doi: 10.3354/meps298115
Ayvazian S., Mulvaney K., Zarnoch C., Palta M., Reichert-Nguyen J., McNally S., et al. (2021). Beyond bioextraction: the role of oyster-mediated denitrification in nutrient management. Environ. Sci. Technol. 55, 14457–14465. doi: 10.1021/acs.est.1c01901
Barry S. C., Jacoby C. A., Frazer T. K. (2018). Resilience to shading influenced by differential allocation of biomass in Thalassia testudinum. Limnology Oceanography 63, 1817–1831. doi: 10.1002/lno.10810
Bates D., Mächler M., Bolker B., Walker S. (2015). Fitting linear mixed-effects models using lme4. J. Stat. Software 67, 1–48. doi: 10.18637/jss.v067.i01
Bedulli C., Lavery P. S., Harvey M., Duarte C. M., Serrano O. (2020). Contribution of seagrass blue carbon toward carbon neutral policies in a touristic and environmentally-friendly island. Front. Mar. Sci. 7, 1. doi: 10.3389/fmars.2020.00001
Bertelli C. M., Robinson M. T., Mendzil A. F., Pratt L. R., Unsworth R. K. (2018). Finding some seagrass optimism in Wales, the case of Zostera noltii. Mar. pollut. Bull. 134, 216–222. doi: 10.1016/j.marpolbul.2017.08.018
Bertin X., Chaumillon E. (2006). The implication of oyster farming in increasing sedimentation rates in a macrotidal bay: the Marennes-Oléron Bay, France. Cahiers biologie Mar. 47, 19.
Blomberg B. N., Palmer T. A., Montagna P. A., Pollack J. B. (2018). Habitat assessment of a restored oyster reef in South Texas. Ecol. Eng. 122, 48–61. doi: 10.1016/j.ecoleng.2018.07.012
Booth D. M., Heck K. L. Jr. (2009). Effects of the American oyster Crassostrea virginica on growth rates of the seagrass Halodule wrightii. Mar. Ecol. Prog. Ser. 389, 117–126. doi: 10.3354/meps08163
Bricker S. B., Ferreira J. G., Zhu C., Rose J. M., Galimany E., Wikfors G., et al. (2018). Role of shellfish aquaculture in the reduction of eutrophication in an urban estuary. Environ. Sci. Technol. 52, 173–183. doi: 10.1021/acs.est.7b03970
Bricker S. B., Grizzle R. E., Trowbridge P., Rose J. M., Ferreira J. G., Wellman K., et al. (2020). Bioextractive removal of nitrogen by oysters in Great Bay Piscataqua River estuary, New Hampshire, USA. Estuaries Coasts 43, 23–38. doi: 10.1007/s12237-019-00661-8
Bricker S. B., Rice K. C., Bricker O. P. (2014). From headwaters to coast: influence of human activities on water quality of the Potomac River Estuary. Aquat. Geochemistry 20, 291–323. doi: 10.1007/s10498-014-9226-y
Bugica K., Sterba-Boatwright B., Wetz M. S. (2020). Water quality trends in Texas estuaries. Mar. pollut. Bull. 152, 110903. doi: 10.1016/j.marpolbul.2020.110903
Burdick D. M., Edwardson K. J., Gregory T., Matso K., Mattera T., Paly M., et al. (2020). A Case for Restoration and Recovery of Zostera marina L. in the Great Bay Estuary. PREP Reports & Publications. 441. Available online at: https://scholars.unh.edu/prep/441.
Burkholder J. M., Mason K. M., Glasgow J. H. B. (1992). Water-column nitrate enrichment promotes decline of eelgrass Zostera marina: evidence from seasonal mesocosm experiments. Mar. Ecol. Prog. Ser. 81, 163–178. doi: 10.3354/meps081163
Burkholder J. M., Tomasko D. A., Touchette B. W. (2007). Seagrasses and eutrophication. J. Exp. Mar. Biol. Ecol. 350, 46–72. doi: 10.1016/j.jembe.2007.06.024
Buzin F., Dupuy B., Lefebvre S., Barillé L., Haure J. (2015). Storage of Pacific oysters Crassostrea gigas in recirculating tank: Ammonia excretion and potential nitrification rates. Aquacultural Eng. 64, 8–14. doi: 10.1016/j.aquaeng.2014.11.007
Cabaço S., Machás R., Santos R. (2007). Biomass–density relationships of the seagrass Zostera noltii: a tool for monitoring anthropogenic nutrient disturbance. Estuarine Coast. Shelf Sci. 74, 557–564. doi: 10.1016/j.ecss.2007.05.029
Cabaço S., Machás R., Santos R. (2009). Individual and population plasticity of the seagrass Zostera noltii along a vertical intertidal gradient. Estuarine Coast. Shelf Sci. 82, 301–308. doi: 10.1016/j.ecss.2009.01.020.1554
Cabaço S., Santos R. (2012). Seagrass reproductive effort as an ecological indicator of disturbance. Ecol. Indic. 23, 116–122. doi: 10.1016/j.ecolind.2012.03.022
Carslaw D. C., Ropkins K. (2009). Open-source tools for analysing air pollution data. Leeds UK 20, 18441.
Cho H. J. (2007). Effects of prevailing winds on turbidity of a shallow estuary. Int. J. Environ. Res. Public Health 4, 185–192. doi: 10.3390/ijerph2007040014
Comeau L. A., Mallet A. L., Carver C. E., Guyondet T. (2014). Impact of high-density suspended oyster culture on benthic sediment characteristics. Aquacultural Eng. 58, 95–102. doi: 10.1016/j.aquaeng.2013.12.004
Congdon V. M., Bonsell C., Cuddy M. R., Dunton K. H. (2019). In the wake of a major hurricane: differential effects on early vs. late successional seagrass species. Limnology Oceanography Lett. 4, 155–163. doi: 10.1002/lol2.10112
Congdon V. M., Hall M. O., Furman B. T., Campbell J. E., Durako M. J., Goodin K. L., et al. (2023). Common ecological indicators identify changes in seagrass condition following disturbances in the Gulf of Mexico. Ecol. Indic. 156, 111090. doi: 10.1016/j.ecolind.2023.111090
Cottrell R. S., Blanchard J. L., Halpern B. S., Metian M., Froehlich H. E. (2020). Global adoption of novel aquaculture feeds could substantially reduce forage fish demand by 2030. Nat. Food 1, 301–308. doi: 10.1038/s43016-020-0078-x
Cravo A., Barbosa A. B., Correia C., Matos A., Caetano S., Lima M. J., et al. (2022). Unravelling the effects of treated wastewater discharges on the water quality in a coastal lagoon system (Ria Formosa, South Portugal): Relevance of hydrodynamic conditions. Mar. pollut. Bull. 174, 113296. doi: 10.1016/j.marpolbul.2021.113296
Crawford C. M., Macleod C. K., Mitchell I. M. (2003). Effects of shellfish farming on the benthic environment. Aquaculture 224, 117–140. doi: 10.1016/S0044-8486(03)00210-2
Cubillo A. M., Lopes A. S., Ferreira J. G., Moore H., Service M., Bricker S. B. (2023). Quantification and valuation of the potential of shellfish ecosystem services in mitigating coastal eutrophication. Estuarine Coast. Shelf Sci. 293, 108469. doi: 10.1016/j.ecss.2023.108469
Cuddy M. R., Dunton K. H. (2023). Seagrass isoscapes and stoichioscapes reveal linkages to inorganic nitrogen sources in the lower laguna madre, western gulf of Mexico. Estuaries Coasts 46, 2115–2127. doi: 10.1007/s12237-023-01206-w
Darnell K. M., Furman B. T., Heck K. L. Jr., Byron D., Reynolds L., Dunton K. H. (2021). Seed reserve hot spots for the sub-tropical seagrass Halodule wrightii (Shoal Grass) in the Northern Gulf of Mexico. Estuaries Coasts 44, 339–351. doi: 10.1007/s12237-020-00808-y
Dawes C. J., Phillips R. C., Morrison G., Dawes C. J. (2004). Seagrass communities of the Gulf Coast of Florida: status and ecology. Florida Fish and Wildlife Conservation Commission Fish and Wildlife Research Institute, p. 74.
De Boer W. F. (2007). Seagrass–sediment interactions, positive feedbacks and critical thresholds for occurrence: a review. Hydrobiologia 591, 5–24. doi: 10.1007/s10750-007-0780-9
Deegan L. A., Wright A., Ayvazian S. G., Finn J. T., Golden H., Merson R. R., et al. (2002). Nitrogen loading alters seagrass ecosystem structure and support of higher trophic levels. Aquat. Conservation: Mar. Freshw. Ecosyst. 12, 193–212. doi: 10.1002/aqc.490
de Los Santos C. B., Krause-Jensen D., Alcoverro T., Marbà N., Duarte C. M., Van Katwijk M. M., et al. (2019). Recent trend reversal for declining European seagrass meadows. Nat. Commun. 10, 3356. doi: 10.1038/s41467-019-11340-4
Duffy J. E. (2006). Biodiversity and the functioning of seagrass ecosystems. Marine. Ecol. Prog. Ser. 311, 233–250. doi: 10.3354/meps311233
Dumbauld B. R., McCoy L. M. (2015). Effect of oyster aquaculture on seagrass Zostera marina at the estuarine landscape scale in Willapa Bay, Washington (USA). Aquaculture Environ. Interact. 7, 29–47. doi: 10.3354/aei00131
Dumbauld B. R., Ruesink J. L., Rumrill S. S. (2009). The ecological role of bivalve shellfish aquaculture in the estuarine environment: a review with application to oyster and clam culture in West Coast (USA) estuaries. Aquaculture 290, 196–223. doi: 10.1016/j.aquaculture.2009.02.033
Dunic J. C., Brown C. J., Connolly R. M., Turschwell M. P., Côté I. M. (2021). Long-term declines and recovery of meadow area across the world’s seagrass bioregions. Global Change Biol. 27, 4096–4109. doi: 10.1111/gcb.v27.17
Dunton K. H. (1994). Seasonal growth and biomass of the subtropical seagrass Halodule wrightii in relation to continuous measurements of underwater irradiance. Mar. Biol. 120, 479–489. doi: 10.1007/BF00680223
Dunton K. H., Burd A., Cifuentes L., Eldridge P. J., Morse J. W. (2003). Concluding Report. Effects of dredge deposits on seagrasses: an integrative model for Laguna Madre. Volume I: Executive Summary. U.S. Army Corps of Engineers, Galveston District, Galveston, Texas.
Dunton K. H., Pulich W. Jr., Mutchler T. R. O. Y. (2011). A seagrass monitoring program for Texas coastal waters. Final Report Contract, (0627), 1–39.
Dunton K. H., Wilson C. J. (2010). Assessment of Little Bay sediment and water quality in relation to indices of seagrass condition. University of Texas at Austin Marine Science Institute Technical Report Number TR/10-001.
Eadie B. J., Bell G. L., Hawley N. (1991). Sediment trap study in the Green Bay mass balance program: Mass and organic carbon fluxes, resuspension, and particle settling velocities.
Eklöf J. S., McMahon K., Lavery P. S. (2009). Effects of multiple disturbances in seagrass meadows: shading decreases resilience to grazing. Mar. Freshw. Res. 60, 1317–1327. doi: 10.1071/MF09008
Erftemeijer P. L., Lewis R. R. R. III (2006). Environmental impacts of dredging on seagrasses: a review. Mar. pollut. Bull. 52, 1553–1572. doi: 10.1016/j.marpolbul.2006.09.006
Evans M. S., Eadie B. J., Glover R. M. (1998). Sediment trap studies in southeastern Lake Michigan: fecal pellet express or the more traveled route? J. Great Lakes Res. 24, 555–568. doi: 10.1016/S0380-1330(98)70844-5
Ferretto G., Vergés A., Poore A. G., Gribben P. E., Glasby T. M. (2022). Floating bags have the potential to minimise oyster farming impacts on Posidonia australis seagrass meadows. Aquaculture 560, 738594. doi: 10.1016/j.aquaculture.2022.738594
Fitzpatrick J., Kirkman H. (1995). Effects of prolonged shading stress on growth and survival of seagrass Posidonia australis in Jervis Bay, New South Wales, Australia. Mar. Ecol. Prog. Ser. 127, 279–289. doi: 10.3354/meps127279
Fodrie F. J., Heck K. L. Jr., Powers S. P., Graham W. M., Robinson K. L. (2010). Climate-related, decadal-scale assemblage changes of seagrass-associated fishes in the northern Gulf of Mexico. Global Change Biol. 16, 48–59. doi: 10.1111/j.1365-2486.2009.01889.x
Fraser M. W., Kendrick G. A. (2017). Belowground stressors and long-term seagrass declines in a historically degraded seagrass ecosystem after improved water quality. Sci. Rep. 7, 14469. doi: 10.1038/s41598-017-14044-1
Gadeken K., Clemo W. C., Ballentine W., Dykstra S. L., Fung M., Hagemeyer A., et al. (2021). Transport of biodeposits and benthic footprint around an oyster farm, Damariscotta Estuary, Maine. PeerJ 9, e11862. doi: 10.7717/peerj.11862
Galasso N. M., Bonaviri C., Trapani F. D., Picciotto M., Gianguzza P., Agnetta D., et al. (2015). Fish-seastar facilitation leads to algal forest restoration on protected rocky reefs. Sci. Rep. 5, 12409. doi: 10.1038/srep12409
Garcıa-Marín P., Cabaço S., Hernandez I., Vergara J. J., Silva J., Santos R. (2013). Multimetric index based on the seagrass Zostera noltii (ZoNI) for ecological quality assessment of coastal and estuarine in S.W. Iberian peninsula. Mar. pollut. Bull. 68, 46–54. doi: 10.1016/j.marpolbul.2012.12.025
Gil M., Armitage A. R., Fourqurean J. W. (2006). Nutrient impacts on epifaunal density and species composition in a subtropical seagrass bed. Hydrobiologia 569, 437–447. doi: 10.1007/s10750-006-0147-7
Gray M. W., Pinton D., Canestrelli A., Dix N., Marcum P., Kimbro D., et al. (2022). Beyond residence time: Quantifying factors that drive the spatially explicit filtration services of an abundant native oyster population. Estuaries Coasts 45, 1343–1360. doi: 10.1007/s12237-021-01017-x
Greening H., Janicki A., Sherwood E. T. (2018). “Seagrass recovery in tampa Bay, Florida (USA),” in The Wetland Book: II: Distribution, Description, and Conservation (Springer, Dordrecht), 495–506.
Guthrie C. G., Matsumoto J., Lu Q. (2010). TxBLEND model calibration and validation for the Guadalupe and Mission-Aransas estuaries. Texas Water Dev. Board 46, 1–46.
Han Q., Liu D. (2014). Macroalgae blooms and their effects on seagrass ecosystems. J. Ocean Univ. China 13, 791–798. doi: 10.1007/s11802-014-2471-2
Handley L., Altsman D., DeMay R. (2007). Seagrass status and trends in the northern Gulf of Mexico: 1940–2002.
Heidelbaugh W. S., Nelson W. G. (1996). A power analysis of methods for assessment of change in seagrass cover. Aquat. Bot. 53 (3–4), 227–233.
Hodgson B. R., Bucher D. J. (2023). Biological processes in seagrass beds of coastal lagoons to maintain estuary-dependent marine fisheries. Mar. Environ. Res. 189, 106033. doi: 10.1016/j.marenvres.2023.106033
Hori M., Lagarde F., Richard M., Derolez V., Hamaguchi M., Makino M. (2019). Coastal management using oyster-seagrass interactions for sustainable aquaculture, fisheries and environment. Bull. Jpn. Fish. Res. Edu. Agen 49, 35–43.
Hothorn T., Bretz F., Westfall P. (2008). Simultaneous inference in general parametric models. Biometrical Journal: J. Math. Methods Biosci. 50, 346–363. doi: 10.1002/bimj.200810425
Kellogg M. L., Smyth A. R., Luckenbach M. W., Carmichael R. H., Brown B. L., Cornwell J. C., et al. (2014). Use of oysters to mitigate eutrophication in coastal waters. Estuarine Coast. Shelf Sci. 151, 156–168. doi: 10.1016/j.ecss.2014.09.025
Kim Y. K., Kim S. H., Lee K. S. (2015). Seasonal growth responses of the seagrass Zostera marina under severely diminished light conditions. Estuaries Coasts 38, 558–568. doi: 10.1007/s12237-014-9833-2
Kim J. B., Lee W. C., Lee K. S., Park J. I. (2013). Growth dynamics of eelgrass, Zostera marina, in the intertidal zone of Seomjin Estuary, Korea. Ocean Sci. J. 48, 239–250. doi: 10.1007/s12601-013-0021-2
Kowalski J. L., DeYoe H. R. (2016). Flowering and seed production in the subtropical seagrass, Halodule wrightii (shoal grass). Botanica Marina 59, 193–199. doi: 10.1515/bot-2015-0099
Kowalski J. L., DeYoe H. R., Allison T. C. (2009). Seasonal production and biomass of the seagrass, Halodule wrightii Aschers.(shoal grass), in a subtropical Texas lagoon. Estuaries Coasts 32, 467–482. doi: 10.1007/s12237-009-9146-z
Larkin P. D., Maloney T. J., Rubiano-Rincon S., Barrett M. M. (2017). A map-based approach to assessing genetic diversity, structure, and connectivity in the seagrass Halodule wrightii. Mar. Ecol. Prog. Ser. 567, 95–107. doi: 10.3354/meps12037
Lavoie R. E. (2005). Oyster Culture in North America History, Present and Future. The 1st International Oyster Symposium Proceedings. Available online at: https://worldoyster.org/wp/wp-content/uploads/2019/04/news_17e.pdf (Accessed February 12, 2021).
Lee K. S., Dunton K. H. (1997). Effect of in situ light reduction on the maintenance, growth and partitioning of carbon resources in Thalassia testudinum banks ex König. J. Exp. Mar. Biol. Ecol. 210, 53–73. doi: 10.1016/S0022-0981(96)02720-7
Lee K. S., Dunton K. H. (2000). Effects of nitrogen enrichment on biomass allocation, growth, and leaf morphology of the seagrass Thalassia testudinum. Mar. Ecol. Prog. Ser. 196, 39–48. doi: 10.3354/meps196039
Lu C. (2015). The Different Methods of Growing Oysters (Pangea Shellfish Company). Industry Website. Available online at: https://www.pangeashellfish.com/blog/the-different-methods-of-growing-oysters (Accessed June 12th, 2020).
Mallet A. L., Carver C. E., Landry T. (2006). Impact of suspended and off-bottom Eastern oyster culture on the benthic environment in eastern Canada. Aquaculture 255, 362–373. doi: 10.1016/j.aquaculture.2005.11.054
McMahon K., Collier C., Lavery P. S. (2013). Identifying robust bioindicators of light stress in seagrasses: a meta-analysis. Ecol. Indic. 30, 7–15. doi: 10.1016/j.ecolind.2013.01.030
Mehrubeoglu M., Cammarata K., Zhang H., Lifford McLauchlan L. (2024). Plume motion characterization in unmanned aerial vehicle aerial video and imagery. J. Appl. Remote Sens. 18, 16501. doi: 10.1117/1.JRS.18.016501
Meling-López A. E., Ibarra-Obando S. E., de la Cueva H., Ortega-Romero P., Navarro-Verdugo A. L. (2021). Effect of depth gradient and temperature on Zostera marina vegetative and reproductive phenology in the first year of the 1997-1998 El Niño. Cienc. marinas 47, 1–15. doi: 10.7773/cm.v47i1.3101
Meysick L., Infantes E., Rugiu L., Gagnon K., Boström C. (2022). Coastal ecosystem engineers and their impact on sediment dynamics: Eelgrass–bivalve interactions under wave exposure. Limnology Oceanography 67, 621–633. doi: 10.1002/lno.12022
Mooney R. F., McClelland J. W. (2012). Watershed export events and ecosystem responses in the Mission–Aransas National Estuarine Research Reserve, south Texas. Estuaries coasts 35, 1468–1485. doi: 10.1007/s12237-012-9537-4
Moreno-Marín F., Vergara J. J., Pérez-Llorens J. L., Pedersen M. F., Brun F. G. (2016). Interaction between ammonium toxicity and green tide development over seagrass meadows: a laboratory study. PloS One 11, e0152971. doi: 10.1371/journal.pone.0152971
Mott J. B., Lehman R. L. (2005). Bacteria source tracking in Copano Bay phase II final report Vol. 24 (Corpus Christi: Texas A&M University). Available at: http://www.cbbep.org/publications/virtuallibrary/2008table/0404.pdf.
Muething K. A., Tomas F., Waldbusser G., Dumbauld B. R. (2020). On the edge: assessing fish habitat use across the boundary between Pacific oyster aquaculture and eelgrass in Willapa Bay, Washington, USA. Aquaculture Environ. Interact. 12, 541–557. doi: 10.3354/aei00381
Muzzi R. W., Eadie B. J. (2002). The design and performance of a sequencing sediment trap for lake research. Mar. Technol. Soc. J. 36, 23–28. doi: 10.4031/002533202787914025
NOAA (2023). N. Centralized Data Management Office | Data Export System. Available online at: https://cdmo.baruch.sc.edu//dges/ (Accessed 10 April 2023).
Nordlund L. M., Unsworth R. K., Gullström M., Cullen-Unsworth L. C. (2018). Global significance of seagrass fishery activity. Fish Fisheries 19, 399–412. doi: 10.1111/faf.2018.19.issue-3
Olivé I., Brun F. G., Vergara J. J., Pérez-Lloréns J. L. (2007). Effects of light and biomass partitioning on growth, photosynthesis and carbohydrate content of the seagrass Zostera noltii Hornem. J. Exp. Mar. Biol. Ecol. 345, 90–100. doi: 10.1016/j.jembe.2007.02.008
Onuf C. P. (1994). Seagrasses, dredging and light in Laguna Madre, Texas, USA. Estuarine Coast. Shelf Sci. 39, 75–91. doi: 10.1006/ecss.1994.1050
Orth R. J., Lefcheck J. S., McGlathery K. S., Aoki L., Luckenbach M. W., Moore K. A., et al. (2020). Restoration of seagrass habitat leads to rapid recovery of coastal ecosystem services. Sci. Adv. 6, eabc6434. doi: 10.1126/sciadv.abc6434
Paulo D., Diekmann O., Ramos A. A., Alberto F., Serrão E. A. (2019). Sexual reproduction vs. clonal propagation in the recovery of a seagrass meadow after an extreme weather event. Scientia Marina 83, 357–363. doi: 10.3989/scimar.04843.06A
Pérez-Ruzafa A., Marcos C., Bernal C. M., Quintino V., Freitas R., Rodrigues A. M., et al. (2012). Cymodocea nodosa vs. Caulerpa prolifera: Causes and consequences of a long term history of interaction in macrophyte meadows in the Mar Menor coastal lagoon (Spain, southwestern Mediterranean). Estuarine Coast. Shelf Sci. 110, 101–115. doi: 10.1016/j.ecss.2012.04.004
Pietros J. M., Rice M. A. (2003). The impacts of aquacultured oysters, Crassostrea virginica (Gmelin 1791) on water column nitrogen and sedimentation: results of a mesocosm study. Aquaculture 220, 407–422. doi: 10.1016/S0044-8486(02)00574-4
Pinheiro J. (2009). nlme: linear and nonlinear mixed effects models. R package version 3.1-96. Available online at: http://cran.r-project.org/web/packages/nlme/ (Accessed March 20, 2020).
Pinheiro J., Bates D., DebRoy S., Sarkar D. (2012). Nonlinear mixed-effects models. R package version, Vol. 3. 1–89.
Pinn W. S., Fang A. N. P., Razalli N. M., Nilamani N., Peng T. C., Yasin Z., et al. (2014). New records of sea stars (Echinodermata Asteroidea) from Malaysia with notes on their association with seagrass beds. Biodiversity J. 5, 453–458.
Prado P., Romero J., Alcoverro T. (2009). Welcome mats? The role of seagrass meadow structure in controlling post-settlement survival in a keystone sea-urchin species. Estuarine Coast. Shelf Sci. 85, 472–478. doi: 10.1016/j.ecss.2009.09.012
Ricart A. M., York P. H., Bryant C. V., Rasheed M. A., Ierodiaconou D., Macreadie P. I. (2020). High variability of Blue Carbon storage in seagrass meadows at the estuary scale. Sci. Rep. 10, 5865. doi: 10.1038/s41598-020-62639-y
Roca G., Alcoverro T., Krause-Jensen D., Balsby T. J., Van Katwijk M. M., Marbà N., et al. (2016). Response of seagrass indicators to shifts in environmental stressors: a global review and management synthesis. Ecol. Indic. 63, 310–323. doi: 10.1016/j.ecolind.2015.12.007
Romero J., Martínez-Crego B., Alcoverro T., Pérez M. (2007). A multivariate index based on the seagrass Posidonia oceanica (POMI) to assess ecological status of coastal waters under the water framework directive (WFD). Mar. pollut. Bull. 55, 196–204. doi: 10.1016/j.marpolbul.2006.08.032
Saenger P., Gartside D., Funge-Smith S. (2013). A review of mangrove and seagrass ecosystems and their linkage to fisheries and fisheries management (Food and Agriculture Organization of the United Nations Regional Office for Asia and the Pacific).
Saunders M. I., Atkinson S., Klein C. J., Weber T., Possingham H. P. (2017). Increased sediment loads cause non-linear decreases in seagrass suitable habitat extent. PloS One 12, e0187284. doi: 10.1371/journal.pone.0187284
Schoenbaechler C., Guthrie C. G., Matsumoto J., Lu Q. (2011). TxBlend model calibration and validation for the laguna madre estuary. Tex Water Dev. Board 60.
Skinner M. A., Courtenay S. C., McKindsey C. W. (2013). Reductions in distribution, photosynthesis, and productivity of eelgrass Zostera marina associated with oyster Crassostrea virginica aquaculture. Mar. Ecol. Prog. Ser. 486, 105–119. doi: 10.3354/meps10345
Smith C. S., Ito M., Namba M., Nakaoka M. (2018). Oyster aquaculture impacts Zostera marina epibiont community composition in Akkeshi-ko estuary, Japan. PloS One 13, e0197753. doi: 10.1371/journal.pone.0197753
Soria J., Jover M., Domínguez-Gómez J. A. (2021). Influence of wind on suspended matter in the water of the albufera of Valencia (Spain). J. Mar. Sci. Eng. 9, 343. doi: 10.3390/jmse9030343
Spalt N., Murgulet D., Abdulla H. (2020). Spatial variation and availability of nutrients at an oyster reef in relation to submarine groundwater discharge. Sci. Total Environ. 710, 136283. doi: 10.1016/j.scitotenv.2019.136283
Staugler B. (n.d). Eyes on Seagrass Field Guides: Seaweed, Seagrasses, Percent Cover, Epibionts. Available online at: https://sarasotabay.org/wp-content/uploads/Eyes-on-Seagrass-Field-Guides-Seaweed_Seagrasses_PercentCover_Epibionts_FGCUAlgae.pdf (Accessed March 10, 2020).
Stunz G. W., Minello T. J., Rozas L. P. (2010). Relative value of oyster reef as habitat for estuarine nekton in Galveston Bay, Texas. Mar. Ecol. Prog. Ser. 406, 147–159. doi: 10.3354/meps08556
Tacon A. G., Metian M. (2015). Feed matters: satisfying the feed demand of aquaculture. Rev. Fisheries Sci. Aquaculture 23, 1–10. doi: 10.1080/23308249.2014.987209
Tallis H. M., Ruesink J. L., Dumbauld B., Hacker S., Wisehart L. M. (2009). Oysters and aquaculture practices affect eelgrass density and productivity in a Pacific Northwest estuary. J. Shellfish Res. 28, 251–261. doi: 10.2983/035.028.0207
Team, R. C. (2020). R: A Language and Environment for Statistical Computing Ver. 4.0. 3. Vienna, Austria: R Foundation for Statistical Computing.
Testa J. M., Brady D. C., Cornwell J. C., Owens M. S., Sanford L. P., Newell C. R., et al. (2015). Modeling the impact of floating oyster (Crassostrea virginica) aquaculture on sediment-water nutrient and oxygen fluxes. Aquaculture Environ. Interact. 7, 205–222. doi: 10.3354/aei00151
Turner J. S., Kellogg M. L., Massey G. M., Friedrichs C. T. (2019). Minimal effects of oyster aquaculture on local water quality: Examples from southern Chesapeake Bay. PloS One 14, e0224768. doi: 10.1371/journal.pone.0224768
Turner E. L., Savage K., Trungale J., Palmer T. A., Montagna P. A. (2016). Effect of freshwater inflow on habitat suitability change in Texas bays. Report to the Texas Environmental Flows Working Group and National Fish and Wildlife Foundation (Corpus Christi, Texas: Harte Research Institute, Texas A&M University-Corpus Christi), 35.
Unsworth R. K., Nordlund L. M., Cullen-Unsworth L. C. (2019). Seagrass meadows support global fisheries production. Conserv. Lett. 12, e12566. doi: 10.1111/conl.2019.12.issue-1
Valiela I., McClelland J., Hauxwell J., Behr P. J., Hersh D., Foreman K. (1997). Macroalgal blooms in shallow estuaries: controls and ecophysiological and ecosystem consequences. Limnology oceanography 42, 1105–1118. doi: 10.4319/lo.1997.42.5_part_2.1105
Van Katwijk M. M., van der Welle M. E. W., Lucassen E. C. H. E. T., Vonk J. A., Christianen M. J. A., Kiswara W., et al. (2011). Early warning indicators for river nutrient and sediment loads in tropical seagrass beds: a benchmark from a near-pristine archipelago in Indonesia. Mar. pollut. Bull. 62, 1512–1520. doi: 10.1016/j.marpolbul.2011.04.007
Vieira V. M., Lobo-Arteaga J., Santos R., Leitão-Silva D., Veronez A., Neves J. M., et al. (2022). Seagrasses benefit from mild anthropogenic nutrient additions. Front. Mar. Sci. 9, 960249. doi: 10.3389/fmars.2022.960249
Vieira V. M., Lopes I. E., Creed J. C. (2018). The biomass–density relationship in seagrasses and its use as an ecological indicator. BMC Ecol. 18, 1–16. doi: 10.1186/s12898-018-0200-1
Wagner E., Dumbauld B. R., Hacker S. D., Trimble A. C., Wisehart L. M., Ruesink J. L. (2012). Density-dependent effects of an introduced oyster, Crassostrea gigas, on a native intertidal seagrass, Zostera marina. Mar. Ecol. Prog. Ser. 468, 149–160. doi: 10.3354/meps09952
Wagner K., Moench E. (2009). Education Program for Improved Water Quality in Copano Bay Task Two Report (Texas Water Resources Institute). (Accessed May 5th, 2021).
Walton W. C., Davis J. E., Supan J. (2013). Off-bottom culture of oysters in the Gulf of Mexico (Southern Regional Aquaculture Center). (Accessed May 5th, 2021).
Waycott M., Duarte C. M., Carruthers T. J., Orth R. J., Dennison W. C., Olyarnik S., et al. (2009). Accelerating loss of seagrasses across the globe threatens coastal ecosystems. Proc. Natl. Acad. Sci. 106, 12377–12381. doi: 10.1073/pnas.0905620106
Williams J. A., Holt G. J., Robillard M. M. R., Holt S. A., Hensgen G., Stunz G. W. (2016). Seagrass fragmentation impacts recruitment dynamics of estuarine-dependent fish. J. Exp. Mar. Biol. Ecol. 479, 97–105. doi: 10.1016/j.jembe.2016.03.008
Wilson S. S., Furman B. T., Hall M. O., Fourqurean J. W. (2020). Assessment of Hurricane Irma impacts on South Florida seagrass communities using long-term monitoring programs. Estuaries Coasts 43, 1119–1132. doi: 10.1007/s12237-019-00623-0
Keywords: seagrass, oyster aquaculture, Crassostrea virginica, Halodule wrightii, Gulf of Mexico
Citation: Rubino R, Lima AR and Fox JM (2024) Short-term effects of a research-scale oyster cage aquaculture system on sediment transport, water quality, and seagrass meadow health in Copano Bay, TX, USA. Front. Mar. Sci. 11:1382153. doi: 10.3389/fmars.2024.1382153
Received: 05 February 2024; Accepted: 15 October 2024;
Published: 14 November 2024.
Edited by:
Shaochun Xu, Chinese Academy of Sciences (CAS), ChinaReviewed by:
Gorka Bidegain, University of the Basque Country, SpainVasco Manuel Nobre de Carvalho da Silva Vieira, University of Lisbon, Portugal
Copyright © 2024 Rubino, Lima and Fox. This is an open-access article distributed under the terms of the Creative Commons Attribution License (CC BY). The use, distribution or reproduction in other forums is permitted, provided the original author(s) and the copyright owner(s) are credited and that the original publication in this journal is cited, in accordance with accepted academic practice. No use, distribution or reproduction is permitted which does not comply with these terms.
*Correspondence: Anthony R. Lima, YW50aG9ueS5saW1hQG5vYWEuZ292