- 1Third Institute of Oceanography, Ministry of Natural Resources, Xiamen, China
- 2Radiation Environment Supervision Station of Fujian Province, Fuzhou, China
Oceans are repositories of radionuclides. Radionuclides are transferred through the food chain and cause ionizing radiation hazards for marine organisms. In this study, the transfer characteristics of 226Ra, 40K, 14C, 3H, 137Cs and 90Sr in organisms at different trophic levels in the eastern coast of Yantai city were investigated. The risk of ionizing radiation to organisms was assessed using the ERICA Tool 2.0. The results show no significant changes in the concentration of any of the nuclides in the coastal area compared to the preoperation period of the nuclear power plant. The transfer factor of 137Cs, 40K, 226Ra, 14C, 90Sr and 3H at the different trophic levels of marine organisms were 2.09, 1.29, 1.17, 1.15, 1.06 and 0.74, respectively. The dose rates of ionizing radiation to organisms from six radionuclides ranged from 32.02 nGy·h-1 to 195.49 nGy·h-1 and had a mean value of 102.86 ± 57.30 nGy·h-1. The main artificial radionuclides (14C, 3H, 90Sr, 137Cs) released by nuclear power plants in the study area produced negligible radiation doses to marine organisms. However, other artificial radionuclides present in the effluents of nuclear power plants (99Tc, 110mAg and 131I) as well as other natural radionuclides (includes 210Po, 210Pb, etc) were not included, and further evaluation of these is recommended.
1 Introduction
The assessment of the exposure of biota to radiation is part of the environment protection system (Maystrenko and Rybak, 2022). Preventing or reducing the frequency of deleterious radiation effects to a level where they would have a negligible impact on the maintenance of biological diversity, the conservation of species, or the health and status of natural habitats, communities and ecosystems is one of the objectives of the International Commission on Radiological Protection (ICRP) (ICRP, 2008). Oceans are repositories of both naturally occurring and anthropogenic radionuclides (Qiao et al., 2023). Radionuclides present in the marine environment can be transferred in organisms through food chains. The organisms are themselves exposed internally to radiation from radionuclides that have been taken up from the environment and externally to radiation in their habitat (UNSCEAR, 2011). The direct hazards from ionizing radiation have been found to manifest at different levels of organization, from the subcellular level and individual organisms to populations and ecosystems (Sazykina and Kryshev, 2003; Garnier-Laplace et al., 2004). Radiation damage to genetic material can result in far-ranging disasters through genetic variation (ICRP, 1991; UNSCEAR, 2012). A comprehensive understanding of the behavior of radionuclides in the ocean and their radiological impact on the environment is of utmost importance (Lee et al., 2023). Therefore, it is essential to study the transfer of radionuclides in marine organisms and to conduct risk assessments to aid in the protection of marine wildlife and human health.
The large variety of species and radionuclides in the ocean, as well as the different behavior of organisms toward these radionuclides, contribute to multiple combinations of radionuclide transfer in marine organisms. This exacerbates many difficulties in accurately assessing the radiation risk to living species from radionuclides in marine environments (Beaugelin-Seiller et al., 2019). The identification of key radionuclides based on their potential contribution to the radiation dose to marine organisms is one of the key steps in assessing biological radiation risk. 226Ra and 40K are the naturally occurring radionuclides that show the highest specific activity in living organisms (Arogunjo et al., 2009; Lima et al., 2005). 14C and 3H are the two artificial radionuclides that contribute the most to the total radiation dose that affects wildlife during normal operation of a nuclear power plant (Beaugelin-Seiller et al., 2019; IAEA, 2021; Tani and Ishikawa, 2023). 137Cs and 90Sr are important artificial radionuclides released from nuclear power plants, and they easily accumulate in organisms (Konovalenko et al., 2016; Pinder et al., 2016). Therefore, 226Ra, 40K, 14C, 3H, 137Cs, and 90Sr were used as radionuclides of interest in this research.
The general approach to assessing radiation doses to organisms consists on constructing assessment models, including equilibrium and dynamic models. The former applies to chronic exposures under normal conditions, while the latter is more suitable for acute exposures under accidental conditions (Vives I Batlle et al., 2016). The Environmental Risk from Ionizing Contaminants: Assessment and Management (ERICA) Tool, developed by the EU, is an assessment model based on equilibrium conditions (Brown et al., 2016). In reality, there is no instantaneous equilibrium of radionuclides between organisms and environmental media. Therefore, the dynamic assessment model under accident conditions is close to the real situation. Current models related to dynamic assessment include the BURN-POSEIDON method (Lepicard et al., 2004), the ANL method (Vives I Batlle et al., 2016), the D-DAT method (Vives I Batlle et al., 2008), the ECOMOD method (Sazykina, 2000), the IRSN method (Fiévet et al., 2006), the NRPA method (Brown et al., 2004), and the multicompartment kinetic–allometric (MCKA) model (Bezhenar et al., 2021). The results calculated by the models tend to differ due to the different models and the model parameters, as well as influences from the uncertainties of the parameters being used (Vives I Batlle et al., 2016).
Food chains (webs) are the support of material cycles and energy flows in biological communities and ecosystems and are important mediators of the impacts of marine pollutants on ecosystems (Liu, 2013). Most radionuclides enter the biocenosis from lower levels of the food chain, including those of autotrophic organisms and bacteria, and then move with food to higher levels of the food chain (Fisher et al., 2000; Wang et al., 1996). The trophic level (TL) reflects an organism’s position in an ecosystem’s food chain/food web and can be used to indicate the energy consumption level of a species, as well as the ability of a particular population to assimilate energy (Bo, 2005). In recent years, it has been recognized that the changes of biological trophic level are influenced by a combination of biotic and abiotic factors (Zhang and Tang, 2004). The study of trophic levels has become an important indicator of the marine environment for assessing and monitoring ecosystem dynamics, biodiversity change and fisheries sustainability (Aydin et al., 2003; Pauly et al., 2001). The accumulation of radionuclides in marine organisms, which is similar to that of metal pollutants, is a complex and dynamic process that is determined by a combination of biological and environmental factors in the habitat and by the nature of the nuclide (Fakhri et al., 2022; Ishii et al., 2020; Suk et al., 2019). Kasamatsu and Ishikawa (1997) analyzed stomach contents of fish samples together with 137Cs concentrations in the stomach contents and demonstrated that the 137Cs concentration in preys of predators increased with their trophic levels (Kasamatsu and Ishikawa, 1997). Currently, carbon and nitrogen isotope components have been widely used to analyze the trophic levels and food sources of marine organisms to identify and determine the processes by which heavy metals or organic pollutants accumulate and flow in biological populations or food chains (Chouvelon et al., 2019; Gao et al., 2021; Liu et al., 2019). However, carbon and nitrogen isotope analysis techniques have not been reported in marine radioactivity studies.
To study the transfer properties of these six radionuclides (3H, 226Ra, 40K, 14C, 137Cs, and 90Sr) at the different trophic levels, and to assess the contribution of the major artificial radionuclides released from the Haiyang nuclear power plant on the radiation dose to marine species, the following studies were carried out in the surrounding 30km sea area of the Haiyang Nuclear Power Plant. First, the impact of Haiyang nuclear power plant operations on the marine environment was investigated in November 2022 by measuring radionuclide activity concentrations in the environment and in organisms. Second, through the analysis of carbon and nitrogen isotope contents in organisms, the transfer features of radionuclides in organisms at different trophic levels were investigated. Third, multivariate statistical analyses were performed to determine the correlation between different radionuclide activities in the organism and the trophic level of the organism. Finally, the radiation dose to organisms at different trophic levels in the marine environment was assessed with the ERICA Tool.
2 Materials and methods
2.1 Sampling and analysis
2.1.1 Sample collection
The Haiyang Nuclear Power Plant is located in Yantai city on the Yellow Sea coast of China’s Jiaodong Peninsula. It is surrounded by the sea on three sides. The commercial operation of the 2 AP1000 nuclear units in the plant started in October 2018 and January 2019 and have been in operation for more than 4 years. In November 2022, 18 sampling stations were deployed evenly along the direction of the tidal field within the 30 km sea area of the Nuclear Power Plant (Figure 1). A pump was used to collect 60 liters of surface seawater into plastic drums at each sampling station. The seawater was acidified to a pH less than 2 with 8 mol/L nitric acid and sealed. A 3-kg sample of marine surface sediment was collected with a Peterson grab dredge (sampling volume 5 L, opening area 15 cm × 30 cm) at each sampling station and stored frozen in polyethylene bags. Nine species of marine organisms were collected from small fishing boats at port terminals near the nuclear power plant (Table 1). These fishing boats were limited by power constraints and they could only catch organisms within 30km of the nuclear power plant which is consistent with the range of our survey stations. For each biological sample, 5 to 10 kg of fresh sample was collected and frozen for preservation. Finally, the samples were sent to the laboratory for further processing.
2.1.2 Sample processing and analysis
2.1.2.1 Sample processing
Seawater samples were decanted in the laboratory for 2-3 days, and the clear liquid was drawn off with a siphon. Afterward, the 137Cs, 226Ra, 40K, 90Sr, 3H, and 14C content in seawater were determined according to relevant national or industrial standards. Briefly, the 137Cs in the seawater was first adsorbed with ammonium phosphomolybdate (AMP) and then precipitated. Second, clear seawater was aspirated by siphoning, and the AMP after adsorption of 137Cs was filtered and collected (Third Institute Of Oceanography, 2018). 226Ra was processed by coprecipitation with barium sulfate. Afterward, the barium sulfate precipitates were combined with the AMP precipitates and ashed in a muffle furnace at 450°C. The ash samples were compacted and sealed in a Φ75 mm×75-mm cylindrical plastic sample box for 30 days before measurement (Third Institute Of Oceanography, 2018). 3H content in the seawater was measured by an ultralow-background liquid scintillation counter (Environment, 2020). First, 1 L of seawater was removed and distilled to reduce the conductivity, after which the solution was electrolytically concentrated. Second, 8 mL of sample was mixed with 12 mL of a liquid scintillation cocktail in a plastic vial. Third, the resulting solution was stored in an LSC sample holder for 12 hours in the dark before counting (Feng et al., 2020). 90Sr content in the seawater was measured by the di(2-ethylhexyl) phosphoric acid (HDEHP) extraction-b counting method (Third Institute Of Oceanography, 2018). First, a total of 2.00 ml of 100 mg/ml Sr(NO3)2, 1.00 ml of 20 mg/ml Y(NO3)2, 60 g of NH4Cl and 400 g of Na2CO3 were added to 40 L of seawater and then stirred for 30 minutes. Second, the precipitate was filtered, and then 10 mol/L HNO3 was used to dissolve the precipitate. The solution was extracted twice using 50 ml of 10% di(2-ethylhexyl) phosphoric acid (HDEHP), and the organic phase was re-extracted twice using 20 ml of 10 mol/l HNO3. Third, a total of 5 ml of C2H2O4 was added to form a saturated solution, and the solution was adjusted to pH=1.5–2.0 using a 6 mol/L NH3H2O solution and 2 mol/L HNO3. Finally, the YC2O4 sediment was produced. The YC2O4 was filtered and placed into an α/β counter to determine the activity of 90Y. The activity of 90Sr was then calculated from the 90Y data. 14C in seawater was measured by a wet oxidation-ultralow background liquid scintillation counter (China, 2019). First, 20 L of seawater was removed and placed in a four-necked flask. FeSO4, H2O2 and K2S2O8 were added, and the flask was subsequently heated. Second, N2 gas was passed through one side of the flask, and the other side was dried with H2SO4. The dried gas was then absorbed with NaOH solution. Third, 8 mL of CO2 absorption solution was mixed with 12 mL of a liquid scintillation cocktail in a plastic vial. Finally, the resulting solution was stored in an LSC sample holder for 12 hours in the dark before counting. A total of 1.5 L of filtered seawater was pipetted into the measuring cassette, and the cassette was subsequently placed on the gamma energy spectrometer to measure 40K (Commission, 2018).
Sediment samples were removed from gravel, larger plant and animal debris, then sequentially dried, ground, and finally sieved through an 80 mesh nylon sieve with a cover. Then, a 300-g prepared sediment sample was compacted and sealed in a Φ75 mm×75 mm cylindrical plastic sample box for 30 days before measurement. The activity of 226Ra was determined based on the gamma ray of 214Pb (351.92keV) and 214Bi (609.31keV). 40K and 137Cs radionuclides activity were determined directly from their respective emission at 1460.81keV and 661.65keV. The activity concentrations were determined by taking into account the net area of the photopeak, the gamma-ray emission probability, the absolute peak efficiency, and the mass of the sample (Patra et al., 2014; Yang et al., 2015). 90Sr in the sediment was also measured by the HDEHP extraction-b counting method, and the sample was counted using the gas-flow proportional alpha/beta counting system (Third Institute Of Oceanography, 2018).
The marine organism samples were dried to constant weight at 60°C in a drum dryer. The pulverized dried biological samples were ashed in a muffle furnace at 450°C for 24-40 hours. The ashed biosamples were then stored in sealed boxes (100 g per sample) for 30 days before analysis (China, 2020). The HDEHP extraction-β counting method and an α/β counter were used for 90Sr analysis (Third Institute Of Oceanography, 2018). A total of 100 g of dried biological sample was weighed, ground into powder form and subsequently placed in a combustion device for slow combustion. The water vapor and CO2 generated after combustion were collected. The subsequent steps were the same as those for monitoring 3H and 14C in water samples (Environment, 2020; Lin et al., 2020).
2.1.2.2 Radionuclide measurement
The 137Cs, 40K, and 226Ra activities were measured using a high-purity germanium γ spectrometer (Canberra GR4021, 40% relative efficiency). The following characteristic γ-ray peaks were selected to calculate the 226Ra (295.21, 351.92, 609.31 keV), 40K (1,460.8 keV), and 137Cs (661.7 keV) activities. To ensure that the data results met the quality control requirements, the instrumentation used in this study was used within the validity period of the calibration. Moreover, the standard materials were analyzed simultaneously with the sample for quality control. The point sources of 60Co (Laboratoire Etalons d’Activite, No-50321), 137Cs (Laboratoire Etalons d’Activite, No-50585), and 242Am (Laboratoire Etalons d’Activite, No-50236) were used for the instrumental scales before the measurements. 3H and 14C were measured by an ultralow-background liquid scintillation counter (LSC, Quantulus 1220, PerkinElmer). The efficiency of the LSC was measured by preparing standard sources in the same form as the samples to be measured using 3H standard solution (Physikalisch-Technische Bundesanstalt, 2005-1439) and 14C standard solution (Physikalisch-Technische Bundesanstalt, 2013-1055). 90Sr was counted using the gas-flow proportional alpha/beta counting system (Ortec MPC-9604). The counting efficiency was measured by using the 90Sr-90Y standard reagent (National Institute of Metrology China, Beijing, China). In addition, the testing programs in this study were approved by inspection and testing organizations. Three replicate measurements were analyzed for each sample.
2.1.2.3 Carbon and nitrogen isotope detection in biological samples
The dorsal fin muscles of fish, abdominal muscles of crustaceans, tentacle muscles of cephalopods, and closed shell muscles of shellfish were rinsed and freeze-dried in a freeze-dryer (SP Scientific FM 25EL). The tissues were then ground into powder in an agate mortar using a pestle. The biological powder was decarbonized and degreased with hydrochloric acid and degreasing solution. After drying, 0.1 mg and 0.5 mg of the sample powder were fed into a stable isotope ratio mass spectrometer (Thermo Fisher Mat 253). The power was turned on, and the samples were combusted at high temperatures to produce CO2 or N2, which was detected and analyzed by an elemental analyzer (Flash 2000HT) and a mass spectrometer (Mat 253) detector inside the instrument. The instrument provides an abundance ratio of 13C/12C or 15N/14N in the sample. The carbon and nitrogen stable isotope ratios (δ) in the sample were calculated as in Equation 1 (Yichen, 2021).
where Rsamples represent the ratios of carbon and nitrogen isotopes (13C/12C or 15N/14N) in the measured samples. Rstandard is the internationally recognized standard carbon isotope ratio and standard atmospheric nitrogen (N2) isotope ratio. δ13C values were determined with an accuracy of ±0.1‰. δ15N values were determined with an accuracy of ±0.2‰. To improve the stability of the instrument and the credibility of the data and to ensure that the results met the quality control requirements, one additional standard sample was added for calibration after every three samples were measured. Furthermore, three biological replicates were analyzed for each sample.
2.2 Data processing analysis
2.2.1 Bioconcentration factors
The bioaccumulation factor is the specific activity of an element or radionuclide in biological tissue relative to its concentration in the environment. For aquatic ecosystems, most approaches calculate CRwo-media using water, as shown in Equation 2 (IAEA, 2014):
where CRwo-media represents the bioaccumulation factor in aquatic ecosystems. In this study, CRwo-media represents the concentration of radionuclides in the whole organism to that in the filtered water.
2.2.2 Biological trophic level calculations
In this study, trophic levels were calculated using the carbon and nitrogen stable isotope contents of collected biological samples. Organisms fractionate carbon and nitrogen stable isotopes through processes such as ingestion, absorption, and metabolism. In general, the ratio of δ15 N in organisms increases by 2.5‰ to 5‰ from one trophic level to the next. The δ15N ratio was used to calculate the trophic level (TL) of the organisms as shown in Equation 3 (Peterson and Fry, 1987):
where δ15Nsample is the N isotope ratio in the measured sample and δ15Nbaseline represents N isotope baseline values. Nitrogen isotope measurements from mussel bodies (6.05‰) were used as the baseline in this study (Deling et al., 2005). Δδ15N is the N stable isotope enrichment factor (2.5‰) in the common food web of the Yellow-Bo Sea in China (Qu et al., 2016).
2.2.3 Magnification factors for radionuclides at the different trophic levels
The cumulative magnification or dissolution of radionuclides in the food web is expressed as a magnification factor, TMF (trophic magnification factor), which is calculated as shown in Equations 4 and 5 (Borga et al., 2012).
where Cm is the specific activity of radionuclides measured in the organism, TL is the biological trophic level, b is the slope of the linear regression equation between (radionuclide-specific activity) and the trophic level (TL), and a is an intercept. TMF is the magnification factor for radionuclides at the trophic level. A TMF > 1 indicates that there is a magnification effect of the radionuclide at the trophic level.
2.2.4 Radiation dose to marine biological species
In this study, the ERICA tool developed by the European Union and the default parameters (radionuclide weighting factors and radiation dose conversion factors) were used to conduct the biological radiation dose assessment. The size (length, width and height) and weight of the investigated biological species were entered into the ERICA Tool 2.0 to construct the assessment model. In the process of calculating the biological radiation dose, the 3H, 226Ra, 40K, 14C, 137Cs and 90Sr concentrations in seawater entered into the ERICA Tool were the average of 18 survey stations. The 226Ra, 40K, 137Cs and 90Sr concentrations in sediment entered were also averaged over 18 stations, and the default Kd of the ERICA tool was used to calculate the 3H and 14C concentrations of the sediments. Concentrations of six radionuclides in organisms were used as monitoring results.
2.2.5 Data statistics and analysis
One-way analysis of variance (ANOVA) was performed to analyze radionuclide bioaccumulation factors in different species using SPSS Statistics 26 to determine the significance of radionuclide bioaccumulation factors among species (Liu, 2013). Multifactor redundancy analysis of radionuclide mass activities in organisms and their relation with ash mass to fresh mass of organisms and trophic levels was performed using Origin 2021 (Yichen, 2021). The bioaccumulation and transfer of different types of radionuclides in organisms and their correlation with ash mass to fresh mass of organisms and biological trophic level were subsequently examined (Yichen, 2021). Origin 2021 was used to fit trophic magnification factors for radionuclides at different biological trophic levels to investigate the radionuclide transfer in marine organisms.
3 Results and discussion
3.1 Results for radionuclides in the seawater
The radionuclide data for the study area are shown in Supplementary Tables 1 and 2. The statistical analysis results are shown in Tables 2, 3 and Figure 2. There were no significant differences in 226Ra, 40K, 90Sr, or 3H at the 18 stations. The YT2, YT7 and YT8 stations had higher 137Cs activity in seawater than did the other stations. 14C was below the detection limit (1 mBq/L) in the seawater at stations YT10 and YT13. The average activity concentrations of radionuclides in seawater were in the following order: 40K (9.99 ± 0.48 Bq/L) > 3H (0.66 ± 0.20 Bq/L) > 226Ra (9.30 ± 2.31 mBq/L) >14C (5.03 ± 1.53 mBq/L) > 90Sr (1.56 ± 0.17 mBq/L) > 137Cs (1.16 ± 0.39 mBq/L).

Table 3. Results for radionuclides in sediments during different periods in the study area (Bq/kg-dry).
The activity concentrations of nuclides were at the same level as those in sea areas affected by discharges from nuclear power plants in China, such as the Changjiang nuclear power marine area, Tianwan nuclear power marine area, Yangjiang nuclear power marine area, and Fuqing nuclear power marine area (Shouxin et al., 2022; Xue et al., 2013). Compared to those in 1995-2009, the levels of 137Cs and 90Sr in seawater in the study area decreased (Haiyun et al., 2010). This was probably because 137Cs and 90Sr originate mainly from early nuclear explosion tests. As the nuclides decay, the activity concentrations of the residual components in the seawater gradually decrease. Compared with the radionuclide levels in the sea area before the operation of the nuclear power plant in 2010-2012, there were no significant changes in 40K, 226Ra, 137Cs or 3H in seawater, and 90Sr was reduced (Xue et al., 2013).
3.2 Results for radionuclides in the sediments
The results of radionuclide detection in the sediments of the sea area are shown in Table 3, and the statistical analysis is shown in Figure 2. The 226Ra, 40K, and 137Cs concentrations at stations YT1 and YT2 were lower than those at the other stations. These differences are probably because of the coarser sediment grain sizes at stations near the river mouth. Moreover, the 90Sr concentrations in the sediments were not significantly different. The average activities of radionuclides in the sediments were in the following order: 40K (650.44 ± 68.85 Bq/kg-dry) > 226Ra (27.04 ± 4.66 Bq/kg-dry) > 137Cs (1.34 ± 0.51 Bq/kg-dry) > 90Sr (0.38 ± 0.15 Bq/kg-dry).
Compared with the radionuclide levels in the period from 2010 to 2012, the levels of 40K, 226Ra, 137Cs, and 90Sr in the sediment did not change significantly and were at the same level as those in other nuclear power sea areas in China, including Daya Bay NPP, Changjiang NPP, and Tianwan NPP (Guiyuan et al., 2019; Konghua et al., 2005; Shouxin et al., 2022; Weirong et al., 2020). The 40K activity in the sediments of the investigated area is slightly greater than the global average (412 Bq/kg-dry) for marine areas. However, it is similar to that in the east coast region of Cyprus (628.1 Bq/kg-dry), Brazil (coast of Rio de Janeiro, 678 Bq/kg-dry), the Aqaba Gulf (641.1 Bq/kg-dry) and the Bay of Bengal (684.4 Bq/kg-dry) (Al-Mur and Gad, 2022; Al-Trabulsy et al., 2011; De Carvalho et al., 2016). The specific activity of 226Ra in sediments near the Haiyang Nuclear Power Plant is comparable to that in Tuban, southern coast of Albania (23 Bq/kg-dry) and Brazil (coast of Rio de Janeiro, 24 Bq/kg-dry) (Aryanti et al., 2021; De Carvalho et al., 2016; Tsabaris et al., 2007), and it is also similar to the world average (32 Bq/kg-dry) (UNSCEAR, 2000). However, the activity of these radionuclides is lower than the value of natural radionuclide activity in various marine areas worldwide, such as close to the Mawan coal-fired power plant (CFPP) in Shenzhen (204 Bq/kg-dry) (Liu et al., 2015). According to the statistical analysis in Figure 2, the specific activities of the natural radionuclides 40K and 226Ra in the sediments near stations YT1 and YT2 were significantly lower than those at the other stations. These differences are probably because of the coarser sediment grain sizes at stations near the river mouth.
3.3 Radionuclides in organisms
The results for radionuclides in organisms are shown in Supplementary Table 3. The 226Ra, 40K, 137Cs, 90Sr, 14C, and 3H activities in marine organisms ranged from 0.04 to 0.98 Bq/kg-fresh, 43.1 to 132 Bq/kg-fresh, 0.01 to 0.03 Bq/kg-fresh, 0.02 to 0.20 Bq/kg-fresh, 14.37 to 30.65 Bq/kg-fresh, and 0.41 to 2.21 Bq/kg-fresh, respectively. The mean specific activities of the radionuclides in organisms are as follows: 40K (74.58 Bq·kg-1·wet) >14C (22.90 Bq·kg-1·wet) >3H (1.05 Bq·kg-1·wet) >226Ra (0.33 Bq·kg-1·wet) >90Sr (0.08 Bq·kg-1·wet) >137Cs (0.02 Bq·kg-1·wet). The specific activities of 40K, 226Ra, 90Sr, and 137Cs in the organisms in the sea area of this study were within the same range as those in the sea areas of the Fuqing NPP, Ningde NPP, and Yangjiang NPP in China and were not significantly different (Li et al., 2021; Sun et al., 2021). There are two chemical forms of 3H in the ocean (tritiated water and tritiated organic molecules). Tritiated water combines with organic compounds through photosynthesis by primary producers to form organically bound tritium (OBT), which can accumulate in organisms higher up in the food chain (Lin et al., 2020). Tritiated water accounts for a large portion of the dose in the short term, and OBT accounts for a large portion of the dose in the longer term (IAEA, 2014). Therefore, this study measured the OBT activity of the examined organisms. The specific activity of OBT in organisms was comparable to that in organisms inhabiting waters adjacent to the Fangchenggang nuclear power plant and slightly greater than that in the nearshore waters of Zhejiang (Lin et al., 2020). However, it was lower than that in the English Channel (Fievet et al., 2013).
3.4 Carbon and nitrogen isotope contents of organisms and at different trophic levels
The results of the carbon and nitrogen isotope detection in marine organisms and calculations for different trophic levels in this study are shown in Supplementary Table A3 and in Figure 3. The δ13C content of the organisms ranged from -14.4‰ to -19.34‰, with a total span of 4.94‰ and a mean value of (-17.18 ± 1.43)‰. The δ15N content of the organisms ranged from 8.48‰ to 12.89‰, with a total span of 4.41‰ and a mean value of 11.44 ± 1.46‰. The carbon and nitrogen isotope contents of the organisms were the similar as those reported in previous investigations and studies in this marine area (Huaiyu et al., 2021; Yichen, 2021). The δ13C contents of the different living species in descending order were as follows: Loligo beka > Octopus variabilis > Cynoglossus semilaevis > Squilla oratoria > Anomiostrea coraliophila > Saurida elongata > Lepidotrigla micropterus > Trachypenaeus curvirostris > Pleuronichthys cornutus. The δ15N content in the organisms, in descending order, was as follows: Saurida elongata > Squilla oratoria > Loligo beka > Cynoglossus semilaevis > Lepidotrigla micropterus > Octopus variabilis > Trachypenaeus curvirostris > Pleuronichthys cornutus > Anomiostrea coraliophila. The order of carbon and nitrogen isotope contents among living species showed some variability.
The trophic level range of the organisms in this study ranged from 1.97 to 3.74, with a total span of 1.77 and a mean value of 3.16 ± 0.59. The results were similar to the coastal waters of Jiangsu Province in 2017 (1.52 ~ 4.28) and Xiaoqing River Estuary adjacent sea area in 2020 (1.65 ~ 3.54) in 2017 (Chuanxin et al., 2022; Nan et al., 2022). It has been shown that if the difference in stable carbon isotopes between 2 species in the same ecosystem is less than 0.60‰, the two species are not in a predatory relationship and may be at the same trophic level. When stable carbon isotopes are greater than 1.5‰, it is also assumed that the two species are not predatory but rather that at least 1 trophic level exists between them (Yukun, 2016). The total span of δ13C in the nine marine organisms in this study was 4.94‰, indicating they belonged to multiple trophic levels, which is consistent with their known trophic levels.
3.5 Bioconcentration factor and multivariate statistical analysis
The radionuclide concentration factors of marine organisms are shown in Table 4. As 226Ra and 40K are natural radionuclides, they are in dynamic equilibrium in the marine environment and in organisms. The average concentration factors of 226Ra and 40K in mollusks, fish and crustaceans in the study area were 4.86 and 4.89, 24.73 and 10.5, and 100.54 and 5.31, respectively. These findings showed that these organisms have a certain bioconcentration effect on the above radionuclides. The concentration factors of 226Ra in fish and mollusks were consistent with the IAEA report (IAEA, 2004, 2014). However, crustaceans had a lower concentration factor for 226Ra than that reported by the IAEA (IAEA, 2004, 2014). 3H, 14C, 90Sr and 137Cs might not reach equilibrium in the study area due to the discharge of nuclear power plants, and the concentration factors of these nuclides in organisms might also not reach equilibrium either. Therefore, the bioconcentration factor coefficients for 14C, 90Sr and 137Cs in organisms were somewhat different from those in the IAEA report. However, due to the high mobility of 3H in water, 3H can quickly reach equilibrium in organisms (IAEA, 2014). The concentration factor of 3H in organisms was the same as that reported by IAEA.
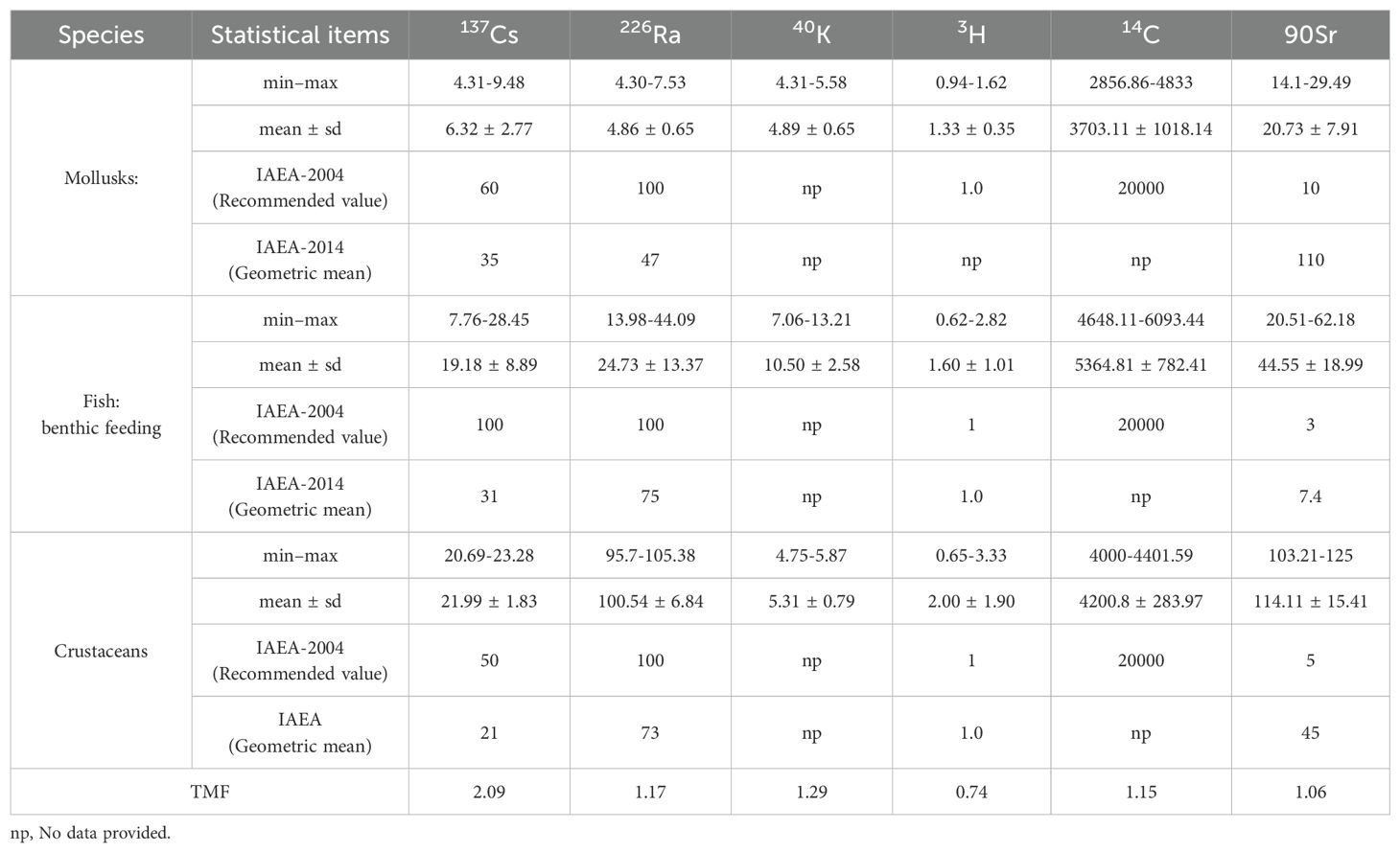
Table 4. Bioconcentration factor (CRwo-water) for wildlife groups in marine ecosystems (Bq/kg, fresh weight whole organism: Bq/L water, min–max, mean ± SD).
The results from the one-way ANOVA showed that the significance levels for radionuclide bioaccumulation factors among species of 226Ra and 40K were 0.0001 and 0.015, respectively, showing there was a significant difference in bioaccumulation factors for these radionuclides between the different species. The significance levels for 137Cs, 14C, and 3H were 0.32, 0.06, 0.08, and 0.80, respectively, between the different species, showing the differences were not significant.
Multivariate statistical analyses (MSAs) were performed to analyze the correlation of the radionuclides with respect to the ash-to-fresh weight ratio and trophic level of the organisms, and the results are shown in Figure 4. The cumulative inertia of the two constraint axes reached 91.38%, which meant that the variability of radionuclides in organisms can be well explained by ash mass to fresh mass of organisms and the trophic level. As shown in Figure 4, 40K, 137Cs, 14C, and 90Sr were strongly positively correlated with the biological trophic level and ash mass to fresh mass of organisms, with correlation sizes changing in the following order: 40K>90Sr>137Cs> 14C. This may be because K and C are the major elements of biological proteins, and Cs and K belong to the same group in the periodic system. They have similar chemical properties related to easy absorption by living organisms (Pentreath, 2019). The 226Ra and 90Sr concentrations in the organisms were strongly correlated and strongly positively correlated with ash mass to fresh mass of organisms. This may be because Ca is one of the main elements of organism bones, and Ra and Sr belong to the same group in the periodic system as Ca and both are osteophilic. Correlation analysis of the biological species revealed that Octopus variabilis and Loligo beka were strongly correlated. Lepidotrigla micropterus and Saurida elongata were more strongly correlated, and Trachypenaeus curvirostris and Squilla oratoria were even more strongly correlated. This was consistent with the morphological structures. and physiological habits of organisms.
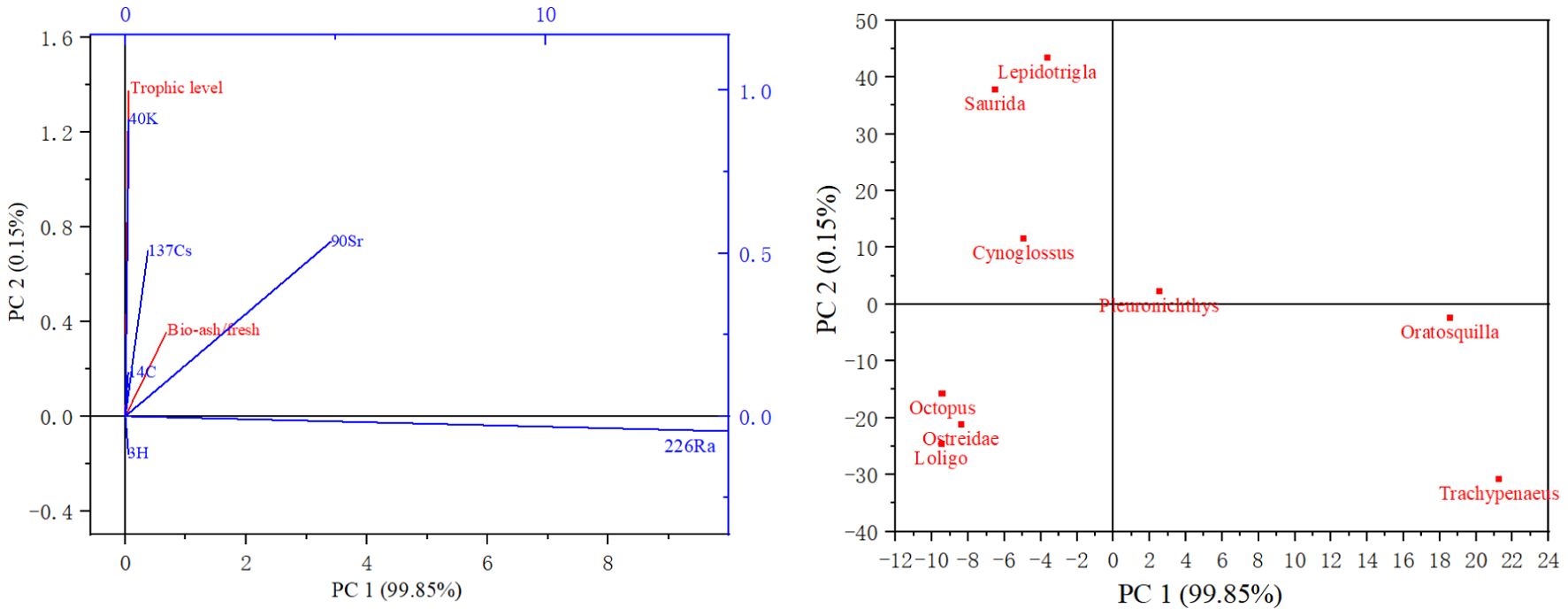
Figure 4. Multivariate statistical analysis of radionuclides versus biological carbon and nitrogen content and ash mass to fresh mass of organisms.
3.6 Cumulative transfer of radionuclides at the different trophic levels of marine organisms
Referring to the methodology of Gao et al. in 2021 (Gao et al., 2021), the trophic magnification factors (TMFs) for different types of radionuclides at different biological trophic levels were fitted. The fitting results are shown in Supplementary Figure 1, and the results from the magnification factor (TMF) calculation are shown in Supplementary Table 4. The TMF for 3H was less than 1.0, indicating that the increase in trophic level has a diluting effect on the activity of 3H. This result was similar to that for the heavy metals Co, Mn, Cd, Cu, and Zn in trophic level accumulation transfer in marine organisms (Bezhenar et al., 2021; Zheng et al., 2023). TMFs greater than 1.0 for 137Cs, 226Ra, 40K, 14C, and 90Sr suggested amplification of these radionuclides through the trophic levels of marine organisms. This result was similar to the transfer of the heavy metals Hg and Cr at marine trophic levels (Liu et al., 2019). The magnitudes of the cumulative transfer coefficients of radionuclides through the trophic levels of marine organisms were in the following order: 137Cs (2.09) > 40K (1.29) > 14C (1.15) > 226Ra (1.17) > 90Sr (1.06) > 3H (0.74). There is a wide variety of marine organisms, and the study is only based on radionuclide detections in nine different marine organisms. It is recommended that more detection data be used in the future to further optimize the fitting results. Using assimilation efficiency simulations, Bezhenar et al. (2021) calculated that concentrations of 137Cs increase with trophic level of marine organisms (Bezhenar et al., 2021). The magnification factor for 137Cs in the present study was equal to that used (TMF= 2.0) by Kasamatsu and Ishikawa (1997), who analyzed the concentration of 137Cs in the stomach and gastric contents of fish (Kasamatsu and Ishikawa, 1997).
The differences in the cumulative delivery of radionuclides at different marine trophic levels may be related to the feeding ecology of the different species and the variability in uptake efficiency by the digestive system (Konovalenko et al., 2016; Nakata and Sugisaki, 2015). When radionuclides are highly concentrated in prey organisms and when the intestinal transfer coefficient of the radionuclide to the predator is high, then the predator organisms are enriched in radionuclides mainly through the digestive absorption pathway (Carvalho, 2018). In addition, differences in the physicochemical properties of nuclides influence the mechanism of internal stabilization regulation of bioconcentrated radionuclides (Fowler and Carvalho, 1985). Some radioisotopes are chemically similar to certain life-essential elements, such as 137Cs and K, 90Sr, 226Ra, and Ca; therefore, they may be passed through the food chain once they are enriched in organisms (Rainbow, 1998). Equilibrium between tissue free water tritium and water is achieved in less than a day due to regulation of the water balance by respiration and osmoregulatory processes; thus, the differences in tritium in organisms are mainly due to differences in organically bound tritium (OBT) (Calmon and Garnier-Laplace, 2001). This is because OBT levels in aquatic biota are affected by a combination of different physicochemical forms of organic tritium present in the ecosystem, different uptake pathways and different transfer rates (Ferreira et al., 2023).
3.7 Assessment of radiation dose to marine species
The dose rates of ionizing radiation to the sampled organisms by the six radionuclides (3H, 226Ra, 40K, 14C, 137Cs and 90Sr) ranged from 32.02 nGy/h to 195.49 nGy/h, with a mean value of 102.86 ± 57.30 nGy/h. The dose rates to organisms from the six radionuclides in this study were about two orders of magnitude below the recommended value by ERICA tool (10 μGy/h). The ionizing radiation risk quotient was in the range of 0.003 to 0.019. The results of the assessment indicated that the ionizing radiation hazard to organisms from the investigated radionuclides were negligible. Trachypenaeus curvirostris, Squilla oratoria and Pleuronichthys cornutus received the highest doses of ionizing radiation. The doses of ionizing radiation to organisms from artificial radionuclides (14C, 3H, 90Sr, 137Cs) ranged from 0.45-1.08 nGy/h, with an average value of 0.86 ± 0.19 nGy/h. 226Ra and 40K produced approximately 99.16% of the total dose of ionizing radiation to organisms from these six radionuclides.
Many studies have been carried out on the assessment of radiation doses from radionuclides to marine organisms (Al-Mur and Gad, 2022; Keum et al., 2013). Based on monitoring data from Daya Bay from 2011–2017, Yue Yu et al. (2023) showed that the total dose rates of 137Cs, 90Sr, 40K, 226Ra, 232Th, 238U, and 210Po to the marine ecosystem of Daya Bay ranged from 230.5 to 853.9 nGy/h. 210Po, 226Ra, and 232Th were the main dose contributors. 137Cs and 90Sr accounted for approximately 0.01%-0.06% of the total radiation dose (Yu et al., 2023). Jiang Sun et al. (2021) showed that the total radiation dose rates of 210Po, 210Pb, 137Cs, 90Sr, 238U, 226Ra, and 40K to 12 marine organisms in the sea areas of the Fuqing NPP and Ningde NPP ranged from 37 to 1531 nGy/h. The dose contributions of 137Cs and 90Sr were <0.13% (Sun et al., 2021), and it’s similar to the results of the present study (0.03%~0.35%). A large contribution to the radiation dose received by marine fauna comes from members of the naturally occurring uranium series that accumulate in the body, especially polonium, such as 210Po (Carvalho, 1988; Cherry et al., 1994). Compared with the results from the above studies, the radiation doses generated by artificial radionuclides in this study were similar. Therefore, the proportion of radiation doses to organisms from the four artificial radionuclides (14C, 3H, 90Sr, 137Cs) would be further reduced if other natural radionuclides (including 210Po, 210Pb, 238U) were accounted for in the study area.
4 Conclusions
The activity concentrations of radionuclides (3H, 226Ra, 40K, 14C, 137Cs and 90Sr) in both the environment and organisms did not change significantly compared to those in the preoperational period.
Mollusks, fish and crustaceans showed bioaccumulative effects on 137Cs, 226Ra, 40K, 14C, 3H, and 90Sr. The bioaccumulation factors for 137Cs, 226Ra, and 40K in mollusks, fish, and crustaceans were different. This showed that there is some variability in the uptake of these three nuclides by different biological species. The results from the magnification factor (TMF) calculation in Supplementary Table A.4 showed that 3H dilution occurred with increasing trophic level. 137Cs, 226Ra, 40K, 14C, and 90Sr had amplification effects through the trophic levels. The cumulative magnification factors of radionuclides at the different trophic levels are in the following order: 137Cs (2.09) >40K (1.29) >14C (1.15) >226Ra (1.17) >90Sr (1.06) >3H (0.74).
The dose rates of ionizing radiation from six radionuclides, 3H, 226Ra, 40K, 14C, 137Cs and 90Sr, ranged from 32.02 nGy-h-1 to 195.49 nGy-h-1, with a mean value of 102.86 ± 57.30 nGy-h-1 for different species in the study area. The dose rates to organisms from the six radionuclides in this study were about two orders of magnitude below the standard limit of radiation dose (10 μGy/h). Compared to the biological radiation dose from natural radionuclides (226Ra, 40K), the percentage of radiation dose rate (0.84%) from the main artificial radionuclides (14C, 3H, 90Sr, 137Cs) released by the nuclear power plant in the marine environment was negligible in our study.
Data availability statement
The datasets presented in this study can be found in online repositories. The names of the repository/repositories and accession number(s) can be found in the article/Supplementary Material.
Author contributions
JN: Methodology, Writing – original draft. DC: Data curation, Validation, Writing – review & editing. ZQ: Data curation, Validation, Writing – review & editing. JL: Investigation, Writing – review & editing. FL: Investigation, Validation, Writing – review & editing. JJ: Investigation, Validation, Writing – review & editing. DH: Investigation, Writing – review & editing. TY: Funding acquisition, Validation, Writing – review & editing.
Funding
The author(s) declare that financial support was received for the research, authorship, and/or publication of this article. This project is supported by Natural Science Foundation of Fujian Province (2023J011370), Science and Technology Project of Fujian Province(Grant No.2019Y0073), the Marine Environment Radioactivity Monitoring and Early Warning Project (LSKJ202202903) and the Fundamental Research Funds of the Third Institute of Oceanology, Ministry of Natural Resources of China (Haisanke 2019001).
Conflict of interest
The authors declare that the research was conducted in the absence of any commercial or financial relationships that could be construed as a potential conflict of interest.
Publisher’s note
All claims expressed in this article are solely those of the authors and do not necessarily represent those of their affiliated organizations, or those of the publisher, the editors and the reviewers. Any product that may be evaluated in this article, or claim that may be made by its manufacturer, is not guaranteed or endorsed by the publisher.
Supplementary material
The Supplementary Material for this article can be found online at: https://www.frontiersin.org/articles/10.3389/fmars.2024.1377411/full#supplementary-material
References
Al-Mur B. A., Gad A. (2022). Radiation hazard from natural radioactivity in the marine sediment of jeddah coast, red sea, Saudi Arabia. J. Mar. Sci. Eng. 10, 1145. doi: 10.3390/jmse10081145
Al-Trabulsy H. A., Khater A. E. M., Habbani F. I. (2011). Radioactivity levels and radiological hazard indices at the saudi coastline of the gulf of aqaba. Radiat. Phys. Chem. Oxf. Engl. 1993. 80 (3), 343–348. doi: 10.1016/j.radphyschem.2010.09.002
Arogunjo A. M., Hoellriegl V., Giussani A., Leopold K., Gerstmann U., Veronese I., et al. (2009). Uranium and thorium in soils, mineral sands, water and food samples in a tin mining area in Nigeria with elevated activity. J. Environ. Radioact. 99, 232–240. doi: 10.1016/j.jenvrad.2008.12.004
Aryanti C. A., Suseno H., Muslim, Prihatiningsih W. R., Yahya M. N. (2021). Concentration of natural radionuclide and potential radiological dose of 226Ra to marine organism in tanjung awar-awar, tuban coal-fired power plant. Jurnal. Segara. 17 (3), 195–206. doi: 10.15578/segara.v17i3.10555
Aydin K. Y., Mcfarlane G. A., King J. R., Megrey B. A. (2003). The BASS/Model Report on Trophic Models of theSubarctic Pacific Basin Ecosystems (Sidney, B.C., Canada: North Pacific Marine Science Organization (PICES), 93.
Beaugelin-Seiller K., Howard B. J., Garnier-Laplace J. (2019). An approach to identifying the relative importance of different radionuclides in ecological radiological risk assessment: application to nuclear power plant releases. J. Environ. Radioact. 197, 116–126. doi: 10.1016/j.jenvrad.2018.11.011
Bezhenar R., Kim K. O., Maderich V., de With G., Jung K. T. (2021). Multi-compartment kinetic–allometric (mcka) model of radionuclide bioaccumulation in marine fish. Biogeosciences 18, 2591–2607. doi: 10.5194/bg-18-2591-2021
Bo Z. (2005). Preliminary studies on marine food wed and trophodynamics in China coastal seas (Qing Dao: Ocean University of China).
Borga K., Kidd K. A., Muir D. C., Berglund O., Conder J. M., Gobas F. A., et al. (2012). Trophic magnification factors: considerations of ecology, ecosystems, and study design. Integr. Environ. Assess. Manag. 8, 64–84. doi: 10.1002/ieam.v8.1
Brown J. E., Alfonso B., Avila R., Beresford N. A., Copplestone D., Hosseini A. (2016). A new version of the erica tool to facilitate impact assessments of radioactivity on wild plants and animals. J. Environ. Radioact. 153, 141–148. doi: 10.1016/j.jenvrad.2015.12.011
Brown J., Børretzen P., Dowdall M., Sazykina T., Kryshev I. (2004). The derivation of transfer parameters in the assessment of radiological impacts on arctic marine biota. Arctic 57, 279–289. doi: 10.14430/arctic505
Carvalho F. P. (1988). 210po in marine organisms: a wide range of natural radiation dose domains. Radiat. Prot. Dosimetry. 24, 113–117. doi: 10.1093/rpd/24.1-4.113
Carvalho F. P. (2018). Radionuclide concentration processes in marine organisms: a comprehensive review. J. Environ. Radioact. 186, 124–130. doi: 10.1016/j.jenvrad.2017.11.002
Cherry R., Heyraud M., Rindfuss R. (1994). Polonium-210 in teleost fish and in marine mammals: interfamily differences and a possible association between polonium-210 and red muscle content. J. Environ. Radioact. 24, 273–291. doi: 10.1016/0265-931X(94)90044-2
China H. M. O. E. (2019). Analytical method of 14C in liquid effluent of nuclear of power plant-wet oxidation (Beijing: China Environment Publishing Group Press).
China N. H. C. O. (2020). Gamma spectrometry method of analyzing radionuclides in biological samples (BeiJing: Standards Press of China).
Chouvelon T., Strady E., Harmelin-Vivien M., Radakovitch O., Brach-Papa C., Crochet S., et al. (2019). Patterns of trace metal bioaccumulation and trophic transfer in a phytoplankton-zooplankton-small pelagic fish marine food web. Mar. pollut. Bull. 146, 1013–1030. doi: 10.1016/j.marpolbul.2019.07.047
Chuanxin Z., Jing C., Yinglu J., Linlin C., Haihui L., Quanchao W., et al. (2022). Benthic food web structure of xiaoqing river estuary adjacent sea area revealed by carbon and nitrogen stable isotope analysis. Acta Oceanol. Sin. 44 (1), 89–100. doi: 10.12284/hyxb2022016
Commission N. H. A. F. (2018). Determination of radionuclides in water by gamma spectrometry (Beijing: Standards Press of China).
De Carvalho F. M., Da Costa Lauria D., Ribeiro F. C. A., Fonseca R. T., Da Silva P. S., Martins N. S. F. (2016). Natural and man-made radionuclides in sediments of an inlet in Rio de Janeiro state, Brazil. Mar. pollut. Bull. 107, 269–276. doi: 10.1016/j.marpolbul.2016.03.059
Deling C., Hongyan L., Qisheng T., Yao S. (2005). Establishment of trophic continuum in the food web of the yellow sea and east China sea ecosystem: insight from carbon and nitrogen stable isotopes. Sci. China 2, 123–130.
Environment R. M. T. C. (2020). Analysis method for tritium in water, HJ 1126-2020 (Beijing: China Environment Publishing group).
Fakhri Y., Sarafraz M., Pilevar Z., Khaneghah A. M. (2022). The concentration and health risk assessment of radionuclides in the muscle of tuna fish: a worldwide systematic review and meta-analysis. Chemosphere. 389, 133–149. doi: 10.1016/j.chemosphere.2021.133149
Feng L., Tao Y., Wen Y., Jialin N., Lin L. (2020). Electrolytic enrichment method for tritium determination in the arctic ocean using liquid scintillation counter. Acta Oceanol. Sin. 39, 73–77. doi: 10.1007/s13131-020-1647-4
Ferreira M. F., Turner A., Vernon E. L., Grisolia C., Lebaron-Jacobs L., Malard V., et al. (2023). Tritium: its relevance, sources and impacts on non-human biota. Sci. Total. Environ. 867, (162816). doi: 10.1016/j.scitotenv.2023.162816
Fievet B., Pommier J., Voiseux C., Bois P. B. D., Laguionie P., Cossonnet C., et al. (2013). Transfer of tritium released into the marine environment by french nuclear facilities bordering the english channel. Environ. Sci. Technol. 47, 6696–6703. doi: 10.1021/es400896t
Fiévet B., Voiseux C., Rozet M., Masson M., du Bois P. B. (2006). Transfer of radiocarbon liquid releases from the areva la hague spent fuel reprocessing plant in the english channel. J. Environ. Radioact. 90, 173–196. doi: 10.1016/j.jenvrad.2006.06.014
Fisher N. S., Stupakoff I., Sañudo-Wilhelmy S., Wang W. X., Crusius J. (2000). Trace metals in marine copepods: a field test of a bioaccumulation model coupled to laboratory uptake kinetics data. Mar. Ecol. Prog. 194, 211–218. doi: 10.3354/meps194211
Fowler S. W., Carvalho F. P. (1985). Americium biokinetics in benthic organisms as a function of feeding mode. Bull. Environ. Contamination. Toxicol. 35, 826–834. doi: 10.1007/BF01636594
Gao Y., Wang R., Li Y., Ding X., Jiang Y., Feng J., et al. (2021). Trophic transfer of heavy metals in the marine food web based on tissue residuals. Sci. Total. Environ. 772, 145064. doi: 10.1016/j.scitotenv.2021.145064
Garnier-Laplace J., Gilek M., Sundell-Bergman S., Larsson C. (2004). Assessing ecological effects of radionuclides: data gaps and extrapolation issues. J. Radiol. Prot. 24, 139–155. doi: 10.1088/0952-4746/24/4A/009
Guiyuan L., Fei D., Wanliang C., Guibiao W., Wentao C. (2019). Levels of radioactivity in marine sediments around the daya bay nuclear power plants site. Nucl. Saf. 18, 5.
Haiyun Y., Ling Z., Yan Z., Fei S., Meiyan L., Zhonggang C., et al. (2010). Monitoring of radioactivity levels of sea water in near coast marine environment in China during 1995-2009. Radiat. Prot. Bull. 35, 13–16.
Huaiyu B., Yukun W., Tingting Z., Lingfeng H., Yao S. (2021). Trophic levels and feeding characters of marine fishes in the yellow sea and northern east China sea based on stable isotope analysis. Prog. Fishery. Sci. 42, 10–17.
IAEA (2004). Sediment distribution coefficients and concentration factors for biota in the marine environment, TECHNICAL REPORTS SERIES No 422 (VIENNA).
IAEA (2014). Handbook of parameter values for the prediction of radionuclide transfer to wildlife, TECHNICAL REPORTS SERIES No. 479 (VIENNA).
IAEA (2021). Approaches for modelling of radioecological data to identify key radionuclides and associated parameter values for human and wildlife exposure assessments, IAEA TECDOC SERIES (VIENNA).
ICRP (1991). “Risk estimates for carcinogenic effects of radiation,” in Annals of the ICRP (New York: Annals of the ICRP).
ICRP (2008). Recommendations of the international commission on radiological protection. 2008. Environmental protection: the concept and use of reference animals and plants (New York: Annals of the ICRP).
Ishii Y., Matsuzaki S. I. S., Hayashi S. (2020). Different factors determine 137 cs concentration factors of freshwater fish and aquatic organisms in lake and river ecosystems. J. Environ. Radioact. 213, (106102). doi: 10.1016/j.jenvrad.2019.106102
Kasamatsu F., Ishikawa Y. (1997). Natural variation of radionuclide 137cs concentration in marine organisms with special reference to the effect of food habits and trophic level. Mar. Ecol. Prog. Ser. 160, 109–120. doi: 10.3354/meps160109
Keum D. K., Jun I. N., Lim K. M., Choi Y. H. (2013). Radiation dose to human and non-human biota in the republic of Korea resulting from the Fukushima nuclear accident. Nucl. Eng. Technol.: Int. J. Korean. Nucl. Soc. 45 (1), 1-12. doi: 10.5516/NET.03.2011.063
Konghua J., Qing T., Quanlu G., Zhigang C. (2005). Tnps environmental monitoring in 2004 (pre-operation). Radiat. Prot. 25, 321–333.
Konovalenko L., Bradshaw C., Andersson E., Lindqvist D., Kautsky U. (2016). Evaluation of factors influencing accumulation of stable sr and cs in lake and coastal fish. J. Environ. Radioact. 160, 64–79. doi: 10.1016/j.jenvrad.2016.04.022
Lee J., Yi S. C., Ko M. J. (2023). Monitoring of 137cs, 239 + 240pu, and 90sr in the marine environment of South Korea and their impact on marine biota: 10 years after the Fukushima accident. Sci. Total. Environ. 905, (167077). doi: 10.1016/j.scitotenv.2023.167077
Lepicard S., Heling R., Maderich V. (2004). Poseidon/rodos models for radiological assessment of marine environment after accidental releases: application to coastal areas of the baltic, black and north seas. J. Environ. Radioact. 72, 153–161. doi: 10.1016/S0265-931X(03)00197-8
Li D., Jiang Z., Zhao L., Zhao F., Zhou P. (2021). Assessment of radioactivity level in the terrestrial and marine organisms in yangjiang and its adjacent areas (China). Int. J. Environ. Res. Public Health 18, (16). doi: 10.3390/ijerph18168767
Lima A., Albanese S., Cicchella D. (2005). Geochemical baselines for the radioelements k, u, and th in the campania region, Italy: a comparison of stream-sediment geochemistry and gamma-ray surveys. Appl. Geochem. 20, 611–625. doi: 10.1016/j.apgeochem.2004.09.017
Lin F., Yu W., Guo J., Liao H., Wang Y. (2020). A method for the determination of organically bound tritium in marine biota based on an improved tubular-combustion system. J. Environ. Radioact. 211, 106084. doi: 10.1016/j.jenvrad.2019.106084
Liu J. (2013). Accumulation of heavy metals in multi-phase media and their transfer and biomagnification in food web of laizhou bay (Qingdao: Institute of Oceanology of Chinese Academy of Sciences).
Liu J., Cao L., Dou S. (2019). Trophic transfer, biomagnification and risk assessments of four common heavy metals in the food web of laizhou bay, the bohai sea. Sci. Total. Environ. 670, 508–522. doi: 10.1016/j.scitotenv.2019.03.140
Liu G., Luo Q., Ding M., Feng J. (2015). Natural radionuclides in soil near a coal-fired power plant in the high background radiation area, south China. Environ. Monit. Assess. 187, 356. doi: 10.1007/s10661-015-4501-y
Maystrenko T., Rybak A. (2022). Radiation exposure and risk assessment to earthworms in areas contaminated with naturally occurring radionuclides. Environ. Monit. Assess. 194, (10). doi: 10.1007/s10661-022-10382-4
Nakata K., Sugisaki H. (2015). “Impacts of the Fukushima nuclear accident on fish and fishing grounds,” in Impacts of the fukushima Nuclear Accident on Fish and Fishing Grounds. (Yokohama, Kanagawa, Japan: Fisheries Research Agency).
Nan L. Y., Rui Z., Hu Z., Shuo Z., Shike G. (2022). Seasonal variation in the trophic structure of food webs in coastal waters of Jiangsu province based on stable isotope techniques. Acta Oceanol. Sin. 44, 1–10.
Patra A. C., Mohapatra S., Sahoo S. K., Lenka P., Dubey J. S., Thakur V. K., et al. (2014). Assessment of ingestion dose due to radioactivity in selected food matrices and water near Vizag, India. J. Radioanal. Nucl. Chem. 300, 903–910. doi: 10.1007/s10967-014-3097-y
Pauly D., Palomares M. L., Froese R., Sa-A P., Vakily M., Preikshot D., et al. (2001). Fishing down Canadian aquatic food webs. Can. J. Fisheries. Aquat. Sci. 58, 51–62. doi: 10.1139/f00-193
Peterson B. J., Fry B. (1987). Stable isotopes in ecosystem studies. Annu. Rev. Ecol. Syst. 1, 293–320. doi: 10.1146/annurev.es.18.110187.001453
Pinder J. E., Rowan D. J., Smith J. T. (2016). Development and evaluation of a regression-based model to predict cesium-137 concentration ratios for saltwater fish. J. Environ. Radioact. 152, 101–111. doi: 10.1016/j.jenvrad.2015.11.004
Qiao J., Casacuberta N., Ginnity P. M. (2023). Editorial: natural and artificial radionuclides as tracers of ocean processes. Front. Mar. Sci 10. doi: 10.3389/fmars.2023.1170408
Qu P., Wang Q., Pang M., Zhang Z., Liu C., Tang X. (2016). Trophic structure of common marine species in the bohai strait, north China sea, based on carbon and nitrogen stable isotope ratios. Ecol. Indic. 66, 405–415. doi: 10.1016/j.ecolind.2016.01.036
Rainbow P. S. (1998). “Phylogeny of trace metal accumulation in crustaceans,” in Metal metabolism in aquatic environments. Eds. Langston W. J., Bebianno M. (Boston, MA: Springer). doi: 10.1007/978-1-4757-2761-6_9
Sazykina T. G. (2000). Ecomod — an ecological approach to radioecological modelling. J. Environ. Radioact. 50 (3), 207–220. doi: 10.1016/S0265-931X(99)00119-8
Sazykina T. G., Kryshev A. I. (2003). EPIC database on the effects of chronic radiation in fish: Russian/FSU data. J. Environ. Radioact. 68, 65–87. doi: 10.1016/S0265-931X(03)00030-4
Shouxin C., Honghai Z., Wu M. (2022). Marine environmental radioactive levels in the waters adjacent to Xiamen and environmental quality assessment. J. Appl. Oceanogr. 41, 443–453. doi: 10.3969/J.ISSN.2095-4972.2022.03.009
Suk H., Kim, Lee H., Lee S. H., Intae (2019). Distribution and accumulation of artificial radionuclides in marine products around Korean peninsula. Mar. pollut. Bull. 146, 521-531. doi: 10.1016/j.marpolbul.2019.06.082
Sun J., Men W., Wang F., Wu J. (2021). Activity levels of 210po, 210pb and other radionuclides (134cs, 137cs, 90sr, 110mag, 238u, 226ra and 40k) in marine organisms from coastal waters adjacent to fuqing and ningde nuclear power plants (China) and radiation dose assessment. Front. Mar. Sci. 8. doi: 10.3389/fmars.2021.702124
Tani T., Ishikawa Y. (2023). A deuterium tracer experiment for simulating accumulation and elimination of organically bound tritium in an edible flatfish, olive flounder. Sci. Total. Environ. 903, 166792. doi: 10.1016/j.scitotenv.2023.166792
Third Institute Of Oceanography S. O. A. (2018). Technical specification for marine environmental radionuclide monitoring, HY/T 235-2018 (Beijing: Standards Press of China).
Tsabaris C., Eleftheriou G., Kapsimalis V., Anagnostou C., Vlastou R., Durmishi C., et al. (2007). Radioactivity levels of recent sediments in the butrint lagoon and the adjacent coast of Albania. Appl. Radiat. Isot. 65, 445–453. doi: 10.1016/j.apradiso.2006.11.006
UNSCEAR. (2000). Sources and effects of ionizing radiation. In: UNSCEAR 2000 report to the General Assembly, with scientific annexes. v. 1: Sources.-v. 2: Effects. (New York).
UNSCEAR. (2011). UNSCEAR 2008 Report to the General Assembly with Scientific Annexes. In: Source and effects of ionizing radiation. In: Source and effects of ionizing radiation. (New York).
UNSCEAR. (2012). Biological Mechanisms of Radiation Actions At Low Doses (United Nations), 1–45. Available online at: http://www.unscear.org/docs/reports/. (New York).
Vives I Batlle J., Wilson R. C., Watts S. J., Jones S. R., Mcdonald P., Vives-Lynch S., et al. (2008). Dynamic model for the assessment of radiological exposure to marine biota. J. Environ. Radioact. 99 (11), 1711–1730. doi: 10.1016/j.jenvrad.2007.11.002
Vives I Batlle J., Beresford N. A., Beaugelin-Seiller K., Bezhenar R., Brown J., Cheng J. J., et al. (2016). Inter-comparison of dynamic models for radionuclide transfer to marine biota in a Fukushima accident scenario. J. Environ. Radioact. 153, 31–50. doi: 10.1016/j.jenvrad.2015.12.006
Wang W. X., Reinfelder J. R., Lee B. G., Fisher N. S. (1996). Assimilation and regeneration of trace elements by marine copepods. Limnol. Oceanogr. 1, 70–81. doi: 10.4319/lo.1996.41.1.0070
Weirong L., Yuhong Q., Feng L., Wei Z., Xin W. (2020). Results analysis of two marine radioactivity surveys in the adjacent water of hainan changjiang npp. J. Appl. Oceanogr. 39, 64–71.
Xue W., Chengpeng H., Chunlei W. (2013). Shandong haiyang nuclear power project unit 3&4project environmental impact report (Shanghai: Shanghai Nuclear Engineering Research and Design Institute Co., Ltd).
Yang B., Ha Y., Jin J. (2015). Assessment of radiological risk for marine biota and human consumers of seafood in the coast of Qingdao, China. Chemosphere 135, 363–369. doi: 10.1016/j.chemosphere.2015.04.097
Yichen L. (2021). Bioaccumulation and influential mechanisms of heavy metals in offshore seafood (Qingdao: Chinese Academy of Sciences).
Yu Y., Zhou P., Men W. (2023). Impact of long-term operation of nuclear power plants on the marine ecosystem of daya bay. Mar. pollut. Bull. 193, (115146). doi: 10.1016/j.marpolbul.2023.115146
Yukun W. (2016). Preliminary studies on the population ecology based on fish otolith microstructure and microchemistry (Qingdao: Ocean University of China).
Zhang B., Tang Q. (2004). Study on trophic level of important resources species at high trophic levels in the bohai sea,yellow sea and east China sea. Adv. Mar. Sci. 22, 393–404.
Keywords: radionuclides, amplification effects, biotrophic level, ionizing radiation, ERICA tool
Citation: Ni J, Chen D, Qian Z, Lin J, Lin F, Ji J, Huang D and Yu T (2024) A study on the transfer of radionuclides and of the resulting radiation dose assessment for marine organisms on the eastern coast of Yantai city. Front. Mar. Sci. 11:1377411. doi: 10.3389/fmars.2024.1377411
Received: 27 January 2024; Accepted: 04 October 2024;
Published: 22 October 2024.
Edited by:
Kun Chen, Jiangsu University, ChinaReviewed by:
Karine Beaugelin-Seiller, Institut de Radioprotection et de Sûreté Nucléaire, FranceLiwei Chen, Hefei Normal University, China
Copyright © 2024 Ni, Chen, Qian, Lin, Lin, Ji, Huang and Yu. This is an open-access article distributed under the terms of the Creative Commons Attribution License (CC BY). The use, distribution or reproduction in other forums is permitted, provided the original author(s) and the copyright owner(s) are credited and that the original publication in this journal is cited, in accordance with accepted academic practice. No use, distribution or reproduction is permitted which does not comply with these terms.
*Correspondence: Jialin Ni, bmlqaWFsaW5AdGlvLm9yZy5jbg==