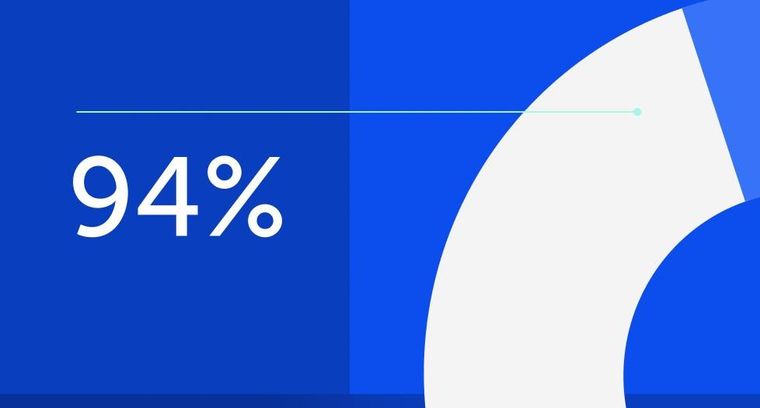
94% of researchers rate our articles as excellent or good
Learn more about the work of our research integrity team to safeguard the quality of each article we publish.
Find out more
ORIGINAL RESEARCH article
Front. Mar. Sci., 07 June 2024
Sec. Global Change and the Future Ocean
Volume 11 - 2024 | https://doi.org/10.3389/fmars.2024.1375839
This article is part of the Research TopicOcean Carbon Sink and ManagementView all 5 articles
Ocean Negative Carbon Emission (ONCE) involves utilizing natural marine chemistry and biology, along with mariculture, to achieve carbon sink goals. Growing awareness of the interplay between aquaculture and the coastal carbonate system has drawn researchers’ attention amid ring CO2 concentrations and the negative impacts of aquaculture on the environment. In this study, twelve sites representing different maricultural types were selected, including macroalgae, shellfish, fish, and non-farming areas. The environmental factors, dissolved inorganic carbon (DIC), total alkalinity (TA), and pCO2, were measured monthly during kelp farming periods. Nitrate is a major component of total nitrogen, and the NO3-N concentration in the macroalgal culture zone was lower than others, indicating effective nitrogen removal by macroalgae aquaculture. TA and DIC in non-farmed areas demonstrated larger variation ranges than in farming areas, probably due to the effects of precipitation on salinity. Aquaculture activities effectively maintained TA and DIC, with macroalgae cultivation playing an important role in TA stability, potentially resisting acidification. The pCO2sea-air of macroalgae culture areas in spring was slightly negative, suggesting carbon sink potential. However, further research is needed to assess the full extent of this “fourth type” of blue carbon, including accurate carbon footprint calculation and the contributions of particulate organic carbon and recalcitrant dissolved organic carbon. This study provided insight into the comprehensive contribution of different aquaculture types to the fishery environment and carbonate system, which can help guide aquaculture management and facilitate the carbon-neutral transition of aquaculture.
Rising atmospheric carbon dioxide (CO2) concentrations over the past two centuries have led to greater CO2 uptake by the oceans, altering the saturation state of the oceans with respect to the carbonate system (Feely et al., 2004). Despite growing awareness of the seriousness of acidification and unremitting efforts to mitigate these global changes, ocean acidification is still on the rise, with CO2 concentrations ongoingly increasing (Kroeker et al., 2013; Osborne et al., 2020; Burger et al., 2022; Nagelkerken and Connell, 2022). Most studies have focused on the negative impacts of increasing CO2 concentration in seawater on marine organisms, including the calcification of coral reefs and shellfish (Hoegh-Guldberg et al., 2007; Talmage and Gobler, 2010; Ekstrom et al., 2015; Lagos et al., 2016), physiology and biochemistry of seagrasses and algae (Roleda et al., 2012; Koch et al., 2013), growth, reproduction, and behavior of fish (Nagelkerken et al., 2016; Cattano et al., 2018), and other structure and function in population or community levels as well (Meakin and Wyman, 2011; Gaylord et al., 2015; Coni et al., 2021). However, as biological pumps contribute to the oceanic carbon cycle, how the adaptation and feedback of these marine organisms affect the marine carbonate system is poorly understood. Recent studies have highlighted the importance of the ocean as a carbon sink, buffering ocean acidification and global warming (Heinze et al., 2015; DeVries, 2022; Wang et al., 2023a).
Aquaculture is a critically high-protein food source, supplying the growing population of the world (Jones et al., 2022). However, aquaculture has faced criticism for excessive greenhouse gas emissions, eutrophication from feeding, and other environmental issues (Yuan et al., 2019; Xu et al., 2022; Zhang et al., 2022). For example, macroalgae blooms in aquaculture ponds lead to green tides (Liu et al., 2021; Sun et al., 2022; Liu et al., 2022a). Methane emissions offset atmospheric carbon dioxide uptake in coastal macroalgae (Roth et al., 2023). The concept of fishery carbon sink has gradually emerged, linking aquaculture with the response to global warming (Ahmed et al., 2017; Ren, 2021; Jia et al., 2023). In particular, the maricultural potential contribution of macroalgae and non-feeding shellfish may become an important driving force in addressing climate change (Zhang et al., 2017; Tamburini et al., 2022). Mariculture blue carbon is also considered an important component of China’s “blue granary” (Zhang et al., 2017; Dong et al., 2022).
As global CO2 emissions continue to rise, there is debate over whether aquaculture acts as a carbon sink or a new source of emissions (Ahmed et al., 2017; Guan et al., 2022; Jones et al., 2022; Tamburini et al., 2022). In addition to the growth and metabolism of cultured organisms themselves, there are few studies on the effect of the biological pump on the carbonate system in aquacultural waters (Morris and Humphreys, 2019; Han et al., 2021). Where the open ocean is difficult to define to account for the contributions and sources of carbon cycle changes, mariculture in the closed bay provides an important place for understanding the temporal and spatial distribution of seawater carbonate systems in fishery waters and their relationship with aquacultural processes (Li et al., 2021). In addition, the closed bay forms an excellent aquacultural environment, including the advantages of small wind and waves as well as adequate nutrient input from rivers. As a result, large-scale aquaculture is concentrated here, especially the formation of complex aquaculture patterns, including macroalgae, bivalve, fish, and other invertebrate species.
Sansha Bay (26.50°~26.96°N, 119.43°~120.17°E), a semi-enclosed bay (about 714 km2) with a depth of more than 10 meters, lies on the coast of Fujian Province, China. The bay has served as one of the most intensively used mariculture bays in China for more than 30 years, where the annual production of shellfish and macroalgae cultivation reached 56.61×104 tons (Song et al., 2023). The aquaculture modes and species are so complex that the main cultivation species included non-feeding shellfish and macroalgae, such as kelp (Saccharina japonica), gracilaria (Gracilaria lemaneiformis), and oysters (Crassostrea gigas), and cage aquaculture, such as abalone (Haliotis discus), sea cucumber (Stichopus japonicus), and yellow croaker (Larimichthys crocea). Water exchange between the bay and East China Sea through the only 3 km opening of the bat is driven by semi-diurnal tides. Three rivers (Huotong River, Baima River, and Bei River) flow into Sansha Bay, which have formed many natural harbors with busy shipping transport (Lin et al., 2017, 2019). In addition to the developed aquaculture and port resources, industry along the coast has been developed with the rapid expansion of new-energy batteries in recent years. Here, twelve sites representing different maricultural types were selected to analyze the spatio-temporal distribution of water quality, dissolve inorganic carbon (DIC), and total alkalinity (TA). The aims of this study are to understand the dynamics of water quality and carbonate systems in different aquaculture types during the whole aquacultural process, to determine the key factors of carbonate systems in coastal fishery waters, and, in addition, to guide the green upgrading of mariculture and provide the scientific basis for fishery carbon sink accounting and management.
Monthly survey cruises were conducted five times in Sansha Bay during kelp farming time (January to June 2023). A total of 12 sites in Sansha Bay were continuously sampled and monitored. The 12 sites basically cover different areas of Sansha Bay and also correspond to different aquacultural modes (Figure 1). Hence, they were grouped into four groups: macroalgae farming area (A), shellfish farming area (S), fish farming area (F), and non-farmed area (N).
A multi-parametric sonde (EXO2, YSI, and US) was used in situ for measurements of water temperature, salinity, DO concentration, and pH at surface seawater. Water samples were manually collected with a water sample collector (1L) from 0.3 meters below the surface at each station for measurement of nutrients, DIC, and TA. All water samples were taken to the laboratory and analyzed immediately.
Total nitrogen (TN) and total phosphorus (TP) in water samples were analyzed by the method of simultaneous digestion introduced by Valderrama (1981). Then, these water samples were filtrated through a cellulose acetate membrane (Merck Millipore Ltd., Ireland) to analyze NO3-N, NO2-N, NH4-N, and PO4-P concentrations. NO3-N was determined with the cadmium−copper column reduction method, according to Grasshoff et al. (2009). NO2-N was measured by the method described by Bendschneider and Robinson (1952). NH4-N was determined with the indophenol blue method, according to Sagi (1966). PO4-P was analyzed by the method introduced by Murphy and Riley (1962).
DIC was measured by acidifying 0.3–0.7 mL of water samples and subsequently quantifying released CO2 using an infrared CO2 detector (Apollo ASC-3) with a precision of ± 2 μmol·L-1 (Cai et al., 2004). TA was determined on 25 mL samples using an open-cell setting based on the Gran titration technique with a Kloehn digital syringe pump. The analytical precision was ± 2 μmol·L-1 (Cai et al., 2010; Zhao et al., 2020).
The aqueous partial pressure of CO2 (pCO2) was calculated with the program CO2SYS-Excel (Pelletier et al., 2007; Xu et al., 2017) based on TA and DIC. Meanwhile, the component composition of the carbon system in surface water was also calculated. The composition of carbonate ions (CO32-), bicarbonate ions (HCO3-), and dissolved CO2 was found to be dynamic. pCO2sea-air (ΔpCO2) is the pCO2 difference between surface seawater and the atmosphere. In this study, the value of atmospheric pCO2 is selected at 420 ppm (NOAA’s Global Monitoring Laboratory). Statistical analysis was performed using SPSS v20.0 and GraphPad Prism v8.0 software. An analysis of variance (ANOVA) was used to analyze the effects of season and zone on environmental factors, the dynamics of DIC and TA, pCO2, and ΔpCO2. According to the results of the homogeneity test, Tukey’s honestly significant difference, or Tamhane’s T2 test, was used to evaluate the significance of differences between groups (P <0.05) after ANOVA. The differences in environmental variables between different cultural systems were analyzed using a Student’s t-test. The correlations between DIC, TA, and pCO2 and the measured variables were tested using Pearson correlation analysis. A stepwise multiple regression analysis was used to identify the relationships between pCO2 and environmental variables. pCO2 was considered the dependent variable, and the measured environmental factors served as the independent variables. To investigate the influence of environmental factors on the parameters of the carbonate system, the best-fit multiple regression equations for pCO2 in different cultural systems, as well as the P value and adjusted R2 value of the models, were determined using the Pearson correlation coefficient.
The variations in environmental parameters are shown in Table 1. The average water temperature and salinity during the macroalgae farming period (January to June) ranged from 11.80°C to 28.60°C and 1.52°C to 30.82°C, respectively. The water temperature increases gradually with each mouth, while the salinity is at its lowest in April and May, mainly due to heavy rainfall, especially the very low salinity of the estuary (N1, N2, and N3). Although rainfall affected salinity, both farming zones showed a small range of salinity variation except for S1, suggesting that the increase of runoff due to precipitation in estuaries is the main factor in salinity changes. Dissolved oxygen (DO) at all stations peaked in March and then continued to decline. As the temperature warms up, DO in summer is significantly lower than that in winter and early spring. On the other hand, pH is much more stable, with a small variation ranging from 7.35 to 8.40 (Table 1).
The concentrations of NO2-N, NH4-N, and PO4-P in farming areas had no difference from those in non-farmed areas, except that the TN and NO3-N concentrations in farming areas were significantly lower than those in non-farmed areas. In particular, with the growth of kelp, NO3-N concentration decreased from close to non-farmed area in January (0.82, 0.65, 0.67, and 0.86 mg·L-1 in macroalgae, shellfish, fish, and non-farming groups, respectively) to half of that from non-farmed area (0.70, 1.07, 1.03, and 1.91 mg·L-1 in macroalgae, shellfish, fish, and non-farming groups, respectively). The algal culture zone showed much lower NO3-N concentrations in May and June, only one-third to one-fourth of those in non-farmed areas. There was no significant difference in PO4-P concentration during the growth of these culture organisms.
The mean TA of macroalgae, shellfish, fish, and non-farming groups were 2218 μmol·L-1, 1917 μmol·L-1, 2111 μmol·L-1, and 1570 μmol·L-1, respectively. Temporally, different farming times had a significant impact on changes in TA (F = 15.60, P-value < 0.01). Especially for non-farmed areas in April and May, TA decreased sharply as the rains increased. TA generally showed a downward trend from January to May and a slight rebound in June, both in farming areas and non-farmed areas, except for macroalgae farming areas, where the region has remained stable in TA (Figure 2). Spatially, aquaculture modes did not have a significant impact on TA (F = 2.25, P-value = 0.14). Algae farming area is always the highest region of TA, while non-farmed area is the lowest with wide ranges. Shellfish farming areas exhibit a relatively lower TA than macroalgae and fish farming areas (Figure 2).
Figure 2 Spatio-temporal distribution of total alkalinity in different farming seasons. * and ** represent p-value < 0.05, and 0.01, respectively.
The surface seawater DIC was highest in January, followed by March and June, which ranged from 486 μmol·L-1 to 2274 μmol·L-1. The surface seawater DIC was highest in macroalgae farming areas and lowest in non-farmed areas. There was no significant difference in DIC between the farmed areas and the non-farmed areas from January to March and June, but it was significantly higher than the non-farmed areas from April to May. DIC showed a slightly downward trend from January to May and then rebounded in June from non-farmed areas. Among the aquaculture zones, the DIC of the macroalgae farming areas are slightly higher than that of the fish and shellfish farming areas, but both of them remain at a significantly higher level than the non-farmed areas (Figure 3).
Figure 3 Spatio-temporal distribution of dissolved inorganic carbon in different farming seasons. *, **, and *** represent p-value < 0.05, 0.01 and 0.001, respectively.
Oceanic dissolved inorganic carbon is the largest pool of carbon that substantially interacts with the atmosphere on human timescales (Humphreys et al., 2022). Hence, the concentration of inorganic carbon in surface water was calculated and found significant variation among different farming areas during the farming season (Table 2). HCO3- is an important component, accounting for 94 percent of the annual average DIC. Except for January, the concentration of HCO3- is significantly higher than that in other areas. The concentration of HCO3- in fish and shellfish farming areas stays stable for their composition in the inorganic system, ranging from 94.20% to 95.09%. Meanwhile, the concentration of CO32- in fish and shellfish is significantly higher than macroalgae farming areas, suggesting that calcification and physiological processes in shellfish and fish potentially facilitate the conversion of the inorganic carbon to organic carbon (Boudreau et al., 2018; Bianchi et al., 2021). Spatially, there was no significant difference in the composition of the inorganic carbon system in different months.
The farming time and aquaculture mode all had a significant impact on the spatio-temporal changes in surface seawater pCO2 (Figure 4A). For macroalgae farming areas, the surface seawater pCO2 was highest in January, followed by June and May, ranging from 213.24 μatm to 2039.25 μatm. With the growth of macroalgae, pCO2 remained at a low level. Especially for A3 station, it showed negative pCO2sea-air from March to May, suggesting the potential for carbon sink (Figure 4B). For shellfish farming areas, the fluctuation of surface seawater pCO2 is very small and low. In addition, although pCO2sea-air is positive, it is still lower than in other areas of the nearshore ocean. In the fish farming area, there was no difference from other aquaculture modes. With the increase in water temperature, the change in pCO2 and pCO2sea-air is greater than that of macroalgae and shellfish. In non-farmed zones, pCO2 fluctuations are the largest and peak in May to June, which is significantly different from farmed zones. In April, however, negative pCO2sea-air has been detected from all the sites in non-farmed zones, which might have resulted from heavy rainfall and no dramatic warming in spring (Figure 4B).
Figure 4 Spatio-temporal distribution of (A) surface water pCO2 and (B) sea-air pCO2 in different farming seasons. * and ** represent p-value < 0.05, and 0.01, respectively.
In order to investigate the influence of environmental factors on the parameters of the carbonate system, Pearson correlation analysis was employed between all environmental factors and TA and DIC. The results showed that salinity played a decisive role in the changes of TA (Pearson R=0.99, P-value < 0.01) and DIC (Pearson R=0.99, P-value < 0.01) (Figure 5). In addition, no other environmental factors showed a significant correlation with TA or DIC. The concentrations of total nitrogen and total phosphorus, however, had no significant effects on the concentrations of TA and DIC. The concentration of total nitrogen was negatively correlated with the distribution of DIC (TN, Pearson R=-0.54, P-value = 0.28) and TA (TN, Pearson R=-0.53, P-value = 0.17). Especially for total nitrogen between 1 mg/L and 4 mg/L, total nitrogen and TA or DIC show a significant negative correlation. While total phosphorus had no significant effect on TA and DIC (Figure 6).
Figure 5 Pearson correlation analyzes salinity with (A) total alkalinity and (B) dissolved inorganic carbon.
Figure 6 Correlation between the concentrations of total nitrogen and total phosphorus with total alkalinity and dissolved inorganic carbon.
Aquaculture has a substantial impact on the water environment of coastal ecosystems (Wu et al., 2022). As an important aquaculture bay, Sansha Bay has been reported in a state of heavy pollution and high eutrophication level after a long-period observation (Wang et al., 2020). The variation of nutrients was controlled by river discharge (Niu et al., 2021; Chen et al., 2022). The concentrations of nitrogen in spring and summer were higher than those in autumn, and the mean concentration of phosphorus was the lowest in spring. In spring and summer, terrestrial input was the dominant source for nitrogen and phosphorus pollution in the surface water of Sansha Bay. In autumn, concentrations of nitrogen and phosphorus were determined by the combination of multiple processes such as endogenous release, seawater dilution, cage culture, and nutrient uptake of macroalgae or phytoplankton (Lin et al., 2021; Niu et al., 2021; Huang et al., 2023). Here, seawater quality is still not optimistic that the concentrations of NO2-N, NO3-N, NH4-N, and PO4-P are over the Category IV seawater quality standards of the national standards of China (GB 3097–1997). The NO3-N concentration in the kelp culture area was significantly lower than that in other areas, indicating the ability of macroalgae to eutrophicate. The capacity, however, is potentially so minimal that the amount of N removed by the cultured seaweed was estimated to be 288 tons/a, accounting for only 2% of the N loaded from fish farming in another quantitative study (Ji et al., 2021). As an important part of ecosystem services, the contribution of shellfish and algae culture to eutrophication still needs to be integrated and evaluated against the background of carbon sinks for aquaculture ecosystems (Duarte et al., 2022; Lin et al., 2023). More importantly, the results also suggested that the current aquaculture plan might not meet the cultivation capacity of the bay. In the future, we should carry out reasonable aquaculture planning to ensure that the service function of the aquaculture ecosystem can be maximized. Referring to the experience and ecological carrying capacity model of IMTA from Sanggou Bay (Gao et al., 2020; Lin et al., 2020), Sansha Bay should also take a path of sustainable development of aquaculture, including the adjustment of aquaculture mode, species composition, and the balance between aquaculture with water flow and nutrient input.
Calcium carbonate formation is the primary pathway by which carbon is returned from the ocean-atmosphere system to the solid Earth (Isson et al., 2020). The removal of dissolved inorganic carbon from seawater by precipitation of carbonate minerals—the marine carbonate factory—plays a critical role in shaping marine biogeochemical cycling (Wang et al., 2023b). Macroalgae cultivation contributed more than 30% TOC in the local area (Wang et al., 2023b). TA refers to the total amount of all substances contained in water that can neutralize and react with strong acids. TA plays an important role in fishery production, and the appropriate TA can stabilize the pH value of water, improve the buffering force of water, and maintain the stability of the aquaculture environment. In general, macroalgae farming areas can absorb carbonate, thereby increasing the TA of water, which results from absorbing CO2 and releasing oxygen during photosynthesis (Chi et al., 2013; Alami et al., 2021; Onyeaka et al., 2021). In contrast, shellfish and fish generally do not significantly alter TA. They may have other effects on water quality, such as the production of wastes such as ammonia and nitrogen through excretion, but these effects are usually not directly related to changes in alkalinity. The same results were reported in the oyster aquaculture system (Han et al., 2021; Villasuso-Palomares et al., 2022). Although there was no significant difference in TA dynamics among different aquaculture modes, the trend of TA change was different from winter to summer. Here, we found that the decrease in salinity caused by precipitation is the most important influencing factor of TA and DIC, which resulted from the dilution of low inorganic carbon from rivers (Ge et al., 2022). However, the cultivation of macroalgae plays an important role in maintaining TA stability but has little impact on DIC. The changes in TA in the shellfish culture area may be caused by biological activities such as excretion and assimilation after water temperature increases. There was no significant decrease in the fish culture area. We speculated that the eutrophication caused by feeding in the fish culture area would indirectly support the increase of local phytoplankton biomass, so that the photosynthesis of phytoplankton maintained a high level of TA in the surrounding area in spring and summer after the temperature increased. Subsequently, it is necessary to supplement the bottom water samples to determine whether there is vertical stratification, which results in a carbon source through the continuous accumulation of the bottom acidification (Zhai et al., 2012).
More and more studies have shown that macroalgae and shellfish aquaculture have certain carbon sink potential (Zhang et al., 2017; Liu et al., 2022b). Macroalgae culture is undoubtedly carbon sinking through sequestering carbon by photosynthesis, which directly absorbs and uses carbon dioxide (Krause-Jensen and Duarte, 2016; Ortega et al., 2019). Through in-situ mesocosm cultivation experiments, the kelp aquaculture area became a source of CO2 at the aging stage of kelps but a sink of CO2 at the fast-growth stage (Xiong et al., 2024). In addition, macroalgal farms can utilize the excess inorganic nutrients supplied by other anthropogenic activities, although the generated organic matter remains in the water body (Xie et al., 2020). In this study, in the maturity stage of kelp culture, the pCO2 of the kelp culture area decreased significantly, and the pCO2sea-air in some stations was negative, indicating that it had a direct effect on carbon sinks. However, because the harvested product is soon eaten, its value as a carbon sink is greatly diminished. We should evaluate the benefits of the whole ecosystem scientifically by integrating its potential as a carbon sink and other ecological service values like water purification.
The carbon sink properties of shellfish are even more controversial (Fodrie et al., 2017; Mariani et al., 2020; Gu et al., 2022). On the one hand, from the perspective of marine chemistry, calcification in forming shells undoubtedly produces CO2 (Ray et al., 2018; Morris and Humphreys, 2019). In the present study, the results of pCO2, calculated by DIC and TA, are positive. However, this study has also proved that the shellfish aquaculture zone has a crucial contribution to the excess of inorganic carbon to organic carbon and the potential resistance to ocean acidification (Filgueira et al., 2015). In addition, shellfish also play an important role in the downward deposition of organic carbon through biological pumps. Hence, filter-feeding shellfish aquaculture has an indirect carbon sink capacity. Overall, we still need to quantitatively study the contribution of carbon sources and sinks in aquaculture through containment experiments in the future.
From 2003 to 2016, the areas dedicated to cage and macroalgae culture in Sansha Bay expanded rapidly, with expansion rates of 1.7 km2/a and 9.3 km2/a, respectively (Xue et al., 2019; Ying et al., 2020; Chen, 2021). In 2018, the intensive cage aquaculture area was renovated by the local government for ecological restoration in Sansha Bay. Hence, the environmental status has improved (Xie et al., 2020). In this study, the seawater quality in Sansha Bay is still under great pressure. With the population return caused by the epidemic and the vigorous development of new energy, a rising upward trend in aquaculture has been seen in Sansha Bay over the past two years. Rational planning of aquaculture layout and continuous environmental monitoring remain top priorities not only for the government but also for the relevant practitioners around the bay. Under the ambition of carbon neutrality, there is a road to green development transformation in Sansha Bay. More attention should be paid to the carbon sink mechanism of macroalgae and shellfish aquaculture, as well as the evaluation of new ecological service values.
The original contributions presented in the study are included in the article/supplementary material. Further inquiries can be directed to the corresponding author.
ZZ: Formal Analysis, Funding acquisition, Investigation, Methodology, Writing – original draft. FW: Investigation, Resources, Writing – original draft. LL: Formal Analysis, Investigation, Resources, Visualization, Writing – original draft. NZ: Formal Analysis, Methodology, Writing – review & editing. ZS: Writing – review & editing. JM: Conceptualization, Funding acquisition, Supervision, Writing – review & editing.
The author(s) declare that financial support was received for the research, authorship, and/or publication of this article. This research was funded by the Special Funding Project for Promoting High-quality Development of Marine and Fishery of Fujian Province (FJHJF-TH-2023–3), the Natural Science Foundation of Fujian Province of China (2022J011136; 2023N0028), and the Marine Economy Development Project of Fujian Province (FJHJF-L-2022–12).
The authors would like to thank Xiaolong Li, Xiaoyun Zhang, Miao Tian, Chaoyue Zhang, and other colleagues from Minjiang University for their help in sample collection and laboratory analysis.
The authors declare that the research was conducted in the absence of any commercial or financial relationships that could be construed as a potential conflict of interest.
All claims expressed in this article are solely those of the authors and do not necessarily represent those of their affiliated organizations, or those of the publisher, the editors and the reviewers. Any product that may be evaluated in this article, or claim that may be made by its manufacturer, is not guaranteed or endorsed by the publisher.
Ahmed N., Bunting S. W., Glaser M., Flaherty M. S., Diana J. S. (2017). Can greening of aquaculture sequester blue carbon? Ambio 46, 468–477. doi: 10.1007/s13280–016-0849–7
Alami A. H., Alasad S., Ali M., Alshamsi M. (2021). Investigating algae for CO2 capture and accumulation and simultaneous production of biomass for biodiesel production. Sci. Total Environ. 759, 143529. doi: 10.1016/j.scitotenv.2020.143529
Bendschneider K., Robinson R. J. (1952). A new spectrophotometric method for the determination of nitrite in sea water (Washington: University of Washington Oceanographic Laboratories).
Bianchi D., Carozza D. A., Galbraith E. D., Guiet J., Devries T. (2021). Estimating global biomass and biogeochemical cycling of marine fish with and without fishing. Sci. Adv. 7, eabd7554. doi: 10.1126/sciadv.abd7554
Boudreau B. P., Middelburg J. J., Luo Y. (2018). The role of calcification in carbonate compensation. Nat. Geosci. 11, 894–900. doi: 10.1038/s41561-018-0259-5
Burger F. A., Terhaar J., Frölicher T. L. (2022). Compound marine heatwaves and ocean acidity extremes. Nat. Commun. 13, 4722. doi: 10.1038/s41467-022-32120-7
Cai W. J., Dai M., Wang Y., Zhai W., Huang T., Chen S., et al. (2004). The biogeochemistry of inorganic carbon and nutrients in the Pearl River estuary and the adjacent Northern South China Sea. Continent. Shelf Res. 24, 1301–1319. doi: 10.1016/j.csr.2004.04.005
Cai W. J., Hu X., Huang W. J., Jiang L. Q., Wang Y., Peng T. H., et al. (2010). Alkalinity distribution in the western North Atlantic Ocean margins. J. Geophys. Res.: Oceans 115, C08014. doi: 10.1029/2009JC005482
Cattano C., Claudet J., Domenici P., Milazzo M. (2018). Living in a high CO2 world: A global meta-analysis shows multiple trait-mediated fish responses to ocean acidification. Ecol. Monogr. 88, 320–335. doi: 10.1002/ecm.1297
Chen C., Lao Q., Shen Y., Jin G., Chen F., Su Q., et al. (2022). Comparative study of nitrogen cycling between a bay with riverine input and a bay without riverine input, inferred from stable isotopes. Front. Mar. Sci. 9. doi: 10.3389/fmars.2022.885037
Chen S. (2021). Spatiotemporal dynamics of mariculture area in Sansha Bay and its driving factors. Chin. J. Ecol. 40, 1137–1145. doi: 10.13292/j.1000-4890.202104.033
Chi Z., Xie Y., Elloy F., Zheng Y., Hu Y., Chen S. (2013). Bicarbonate-based integrated carbon capture and algae production system with alkalihalophilic cyanobacterium. Biores. Technol. 133, 513–521. doi: 10.1016/j.biortech.2013.01.150
Coni E. O., Nagelkerken I., Ferreira C. M., Connell S. D., Booth D. J. (2021). Ocean acidification may slow the pace of tropicalization of temperate fish communities. Nat. Climate Change 11, 249–256. doi: 10.1038/s41558-020-00980-w
DeVries T. (2022). The ocean carbon cycle. Annu. Rev. Environ. Resour. 47, 317–341. doi: 10.1146/annurev-environ-120920-111307
Dong S. L., Dong Y. W., Cao L., Verreth J., Olsen Y., Liu W. J., et al. (2022). Optimization of aquaculture sustainability through ecological intensification in China. Rev. Aquacult. 14, 1249–1259. doi: 10.1111/raq.12648
Duarte C. M., Bruhn A., Krause-Jensen D. (2022). A seaweed aquaculture imperative to meet global sustainability targets. Nat. Sustainabil. 5, 185–193. doi: 10.1038/s41893-021-00773-9
Ekstrom J. A., Suatoni L., Cooley S. R., Pendleton L. H., Waldbusser G. G., Cinner J. E., et al. (2015). Vulnerability and adaptation of US shellfisheries to ocean acidification. Nat. Climate Change 5, 207–214. doi: 10.1038/nclimate2508
Feely R. A., Sabine C. L., Lee K., Berelson W., Kleypas J., Fabry V. J., et al. (2004). Impact of anthropogenic CO2 on the CaCO3 system in the oceans. Science 305, 362–366. doi: 10.1126/science.1097329
Filgueira R., Byron C. J., Comeau L. A., Costa-Pierce B., Cranford P. J., Ferreira J. G., et al. (2015). An integrated ecosystem approach for assessing the potential role of cultivated bivalve shells as part of the carbon trading system. Mar. Ecol. Prog. Ser. 518, 281–287. doi: 10.3354/meps11048
Fodrie F. J., Rodriguez A. B., Gittman R. K., Grabowski J. H., Lindquist N. L., Peterson C. H., et al. (2017). Oyster reefs as carbon sources and sinks. Proc. R. Soc. B: Biol. Sci. 284, 20170891. doi: 10.1098/rspb.2017.0891
Gao Y., Fang J., Lin F., Li F., Li W., Wang X., et al. (2020). Simulation of oyster ecological carrying capacity in Sanggou Bay in the ecosystem context. Aquacult. Int. 28, 2059–2079. doi: 10.1007/s10499-020-00576-3
Gaylord B., Kroeker K. J., Sunday J. M., Anderson K. M., Barry J. P., Brown N. E., et al. (2015). Ocean acidification through the lens of ecological theory. Ecology 96, 3–15. doi: 10.1890/14-0802.1
Ge T., Luo C., Ren P., Zhang H., Fan D., Chen H., et al. (2022). Stable carbon isotopes of dissolved inorganic carbon in the Western North Pacific Ocean: Proxy for water mixing and dynamics. Front. Mar. Sci. 9. doi: 10.3389/fmars.2022.998437
Grasshoff K., Kremling K., Ehrhardt M. (Eds.) (2009). Methods of seawater analysis (Weinheim, Germany: John Wiley & Sons).
Gu Y., Lyu S., Wang L., Chen Z., Wang X. (2022). Assessing the carbon sink capacity of coastal mariculture shellfish resources in China from 1981–2020. Front. Mar. Sci. 9. doi: 10.3389/fmars.2022.981569
Guan H., Sun Z., Zhao A. (2022). Spatio-temporal evolution and influencing factors of net carbon sink in marine aquaculture in China. Front. Environ. Sci. 10. doi: 10.3389/fenvs.2022.978073
Han T., Shi R., Qi Z., Huang H., Gong X. (2021). Impacts of large-scale aquaculture activities on the seawater carbonate system and air-sea CO2 flux in a subtropical mariculture bay, southern China. Aquacult. Environ. Interact. 13, 199–210. doi: 10.3354/aei00400
Heinze C., Meyer S., Goris N., Anderson L., Steinfeldt R., Chang N., et al. (2015). The ocean carbon sink–impacts, vulnerabilities and challenges. Earth Sys. Dynam. 6, 327–358. doi: 10.5194/esd-6-327-2015
Hoegh-Guldberg O., Mumby P. J., Hooten A. J., Steneck R. S., Greenfield P., Gomez E., et al. (2007). Coral reefs under rapid climate change and ocean acidification. Science 318, 1737–1742. doi: 10.1126/science.1152509
Huang Y., Li Y., Chen Z., Li R., Chen H., Mu J. (2023). Spatiotemporal distributions of nutrients and their potential influencing factors in Sansha bay. Mar. Environ. Sci. 42, 440–448. doi: 10.12111/j.mes.2022-x-0111
Humphreys M. P., Lewis E. R., Sharp J. D., Pierrot D. (2022). PyCO2SYS v1. 8: marine carbonate system calculations in Python. Geosci. Model. Dev. 15, 15–43. doi: 10.5194/gmd-15-15-2022
Isson T. T., Planavsky N. J., Coogan L. A., Stewart E. M., Ague J. J., Bolton E. W., et al. (2020). Evolution of the global carbon cycle and climate regulation on earth. Global Biogeochem. Cycles 34, e2018GB006061. doi: 10.1029/2018GB006061
Ji W., Yokoyama H., Fu J., Zhou J. (2021). Effects of intensive fish farming on sediments of a temperate bay characterised by polyculture and strong currents. Aquacult. Rep. 19, 100579. doi: 10.1016/j.aqrep.2020.100579
Jia R., Li P., Chen C., Liu L., Li Z. H. (2023). Shellfish-algal systems as important components of fisheries carbon sinks: Their contribution and response to climate change. Environ. Res. 224, 115511. doi: 10.1016/j.envres.2023.115511
Jones A. R., Alleway H. K., McAfee D., Reis-Santos P., Theuerkauf S. J., Jones R. C. (2022). Climate-friendly seafood: The potential for emissions reduction and carbon capture in marine aquaculture. BioScience 72, 123–143. doi: 10.1093/biosci/biab126
Koch M., Bowes G., Ross C., Zhang X. H. (2013). Climate change and ocean acidification effects on seagrasses and marine macroalgae. Global Change Biol. 19, 103–132. doi: 10.1111/j.1365-2486.2012.02791.x
Krause-Jensen D., Duarte C. M. (2016). Substantial role of macroalgae in marine carbon sequestration. Nat. Geosci. 9, 737–742. doi: 10.1038/ngeo2790
Kroeker K. J., Kordas R. L., Crim R., Hendriks I. E., Ramajo L., Singh G. S., et al. (2013). Impacts of ocean acidification on marine organisms: quantifying sensitivities and interaction with warming. Global Change Biol. 19, 1884–1896. doi: 10.1111/gcb.12179
Lagos N. A., Benítez S., Duarte C., Lardies M. A., Broitman B. R., Tapia C., et al. (2016). Effects of temperature and ocean acidification on shell characteristics of Argopecten purpuratus: implications for scallop aquaculture in an upwelling-influenced area. Aquacult. Environ. Interact. 8, 357–370. doi: 10.3354/aei00183
Li J., Zhang W., Ding J., Xue S., Huo E., Ma Z., et al. (2021). Effect of large-scale kelp and bivalve farming on seawater carbonate system variations in the semi-enclosed Sanggou Bay. Sci. Total Environ. 753, 142065. doi: 10.1016/j.scitotenv.2020.142065
Lin H., Chen Z., Hu J., Cucco A., Sun Z., Chen X., et al. (2019). Impact of cage aquaculture on water exchange in Sansha Bay. Continent. Shelf Res. 188, 103963. doi: 10.1016/j.csr.2019.103963
Lin H., Chen Z., Hu J., Cucco A., Zhu J., Sun Z., et al. (2017). Numerical simulation of the hydrodynamics and water exchange in Sansha Bay. Ocean Eng. 139, 85–94. doi: 10.1016/j.oceaneng.2017.04.031
Lin C. Y., Dai G. L., Liu Y., Zhang M. Q., Liu Y., Jiang W., et al. (2023). Ecological value of mariculture shellfish resources in China: Assessment and management. Mar. Policy 148, 105406. doi: 10.1016/j.marpol.2022.105406
Lin F., Du M., Liu H., Fang J., Asplin L., Jiang Z. (2020). A physical-biological coupled ecosystem model for integrated aquaculture of bivalve and seaweed in sanggou bay. Ecol. Model. 431, 109181. doi: 10.1016/j.ecolmodel.2020.109181
Lin M., Yu J., Liu X., Niu S., Tong M., Guo H., et al. (2021). Abnormalities, mechanisms and effects of nitrite nitrogen, ammonia nitrogen and phosphate in Sansha Bay. Earth Sci. 46, 4107–4117. doi: 10.3799/dqkx.2020.368
Liu J., Jia X., Zhuang M., Zhang J., Yu K., Zhao S., et al. (2021). Controlling the source of green tides in the Yellow Sea: NaClO treatment of Ulva attached on Pyropia aquaculture rafts. Aquaculture 535, 736378. doi: 10.1016/j.aquaculture.2021.736378
Liu J., Tong Y., Jia X., Sun Y., Zhao X., Sun J., et al. (2022a). Ulva macroalgae within local aquaculture ponds along the estuary of Dagu River, Jiaozhou Bay, Qingdao. Mar. pollut. Bull. 174, 113243. doi: 10.1016/j.marpolbul.2021.113243
Liu Y., Zhang J., Wu W., Zhong Y., Li H., Wang X., et al. (2022b). Effects of shellfish and macro-algae IMTA in north China on the environment, inorganic carbon system, organic carbon system, and sea–air CO2 fluxes. Front. Mar. Sci. 9. doi: 10.3389/fmars.2022.864306
Mariani G., Cheung W. W., Lyet A., Sala E., Mayorga J., Velez L., et al. (2020). Let more big fish sink: Fisheries prevent blue carbon sequestration—half in unprofitable areas. Sci. Adv. 6, eabb4848. doi: 10.1126/sciadv.abb4848
Meakin N. G., Wyman M. (2011). Rapid shifts in picoeukaryote community structure in response to ocean acidification. ISME J. 5, 1397–1405. doi: 10.1038/ismej.2011.18
Morris J. P., Humphreys M. P. (2019). Modelling seawater carbonate chemistry in shellfish aquaculture regions: Insights into CO2 release associated with shell formation and growth. Aquaculture 501, 338–344. doi: 10.1016/j.aquaculture.2018.11.028
Murphy J. A. M. E. S., Riley J. P. (1962). A modified single solution method for the determination of phosphate in natural waters. Anal. Chimica Acta 27, 31–36. doi: 10.1016/S0003-2670(00)88444-5
Nagelkerken I., Connell S. D. (2022). Ocean acidification drives global reshuffling of ecological communities. Global Change Biol. 28, 7038–7048. doi: 10.1111/gcb.16410
Nagelkerken I., Russell B. D., Gillanders B. M., Connell S. D. (2016). Ocean acidification alters fish populations indirectly through habitat modification. Nat. Climate Change 6, 89–93. doi: 10.1038/nclimate2757
Niu S., Li M., Tong M., Liu X., Lin M., Yin G. (2021). Responses of distribution of water quality in the Sansha bay on the land discharge and aquaculture. J. Mar. Environ. Sci. 40, 41–49. doi: 10.12111/j.mes.20190186
Onyeaka H., Miri T., Obileke K., Hart A., Anumudu C., Al-Sharify Z. T. (2021). Minimizing carbon footprint via microalgae as a biological capture. Carbon Capture Sci. Technol. 1, 100007. doi: 10.1016/j.ccst.2021.100007
Ortega A., Geraldi N. R., Alam I., Kamau A. A., Acinas S. G., Logares R., et al. (2019). Important contribution of macroalgae to oceanic carbon sequestration. Nat. Geosci. 12, 748–754. doi: 10.1038/s41561-019-0421-8
Osborne E. B., Thunell R. C., Gruber N., Feely R. A., Benitez-Nelson C. R. (2020). Decadal variability in twentieth-century ocean acidification in the California Current Ecosystem. Nat. Geosci. 13, 43–49. doi: 10.1038/s41561-019-0499-z
Pelletier G., Lewis E., Wallace D. (2007). CO2sys.xls: A Calculator for the CO2 System in Seawater for Microsoft Excel/VBA (Olympia, WA/Upton, NY, USA: Washington State Department of Ecology/Brookhaven National Laboratory).
Ray N. E., O’Meara T., Wiliamson T., Izursa J. L., Kangas P. C. (2018). Consideration of carbon dioxide release during shell production in LCA of bivalves. Int. J. Life Cycle Assess. 23, 1042–1048. doi: 10.1007/s11367-017-1394-8
Ren W. (2021). Study on the removable carbon sink estimation and decomposition of influencing factors of mariculture shellfish and algae in China—a two-dimensional perspective based on scale and structure. Environ. Sci. pollut. Res. 28, 21528–21539. doi: 10.1007/s11356-020-11997-1
Roleda M. Y., Morris J. N., McGraw C. M., Hurd C. L. (2012). Ocean acidification and seaweed reproduction: increased CO2 ameliorates the negative effect of lowered pH on meiospore germination in the giant kelp Macrocystis pyrifera (Laminariales, Phaeophyceae). Global Change Biol. 18, 854–864. doi: 10.1111/j.1365-2486.2011.02594.x
Roth F., Broman E., Sun X., Bonaglia S., Nascimento F., Prytherch J., et al. (2023). Methane emissions offset atmospheric carbon dioxide uptake in coastal macroalgae, mixed vegetation and sediment ecosystems. Nat. Commun. 14, 42. doi: 10.1038/s41467-022-35673-9
Sagi T. (1966). Determination of ammonia in sea water by the indophenol method and its application to the coastal and off-shore waters. Oceanogr. Mag. 18, 43–51.
Song Y., Li M., Fang Y., Liu X., Yao H., Fan C., et al. (2023). Effect of cage culture on sedimentary heavy metal and water nutrient pollution: Case study in Sansha Bay, China. Sci. Total Environ. 899, 165635. doi: 10.1016/j.scitotenv.2023.165635
Sun Y., Yao L., Liu J., Tong Y., Xia J., Zhao X., et al. (2022). Prevention strategies for green tides at source in the Southern Yellow Sea. Mar. pollut. Bull. 178, 113646. doi: 10.1016/j.marpolbul.2022.113646
Talmage S. C., Gobler C. J. (2010). Effects of past, present, and future ocean carbon dioxide concentrations on the growth and survival of larval shellfish. Proc. Natl. Acad. Sci. 107, 17246–17251. doi: 10.1073/pnas.0913804107
Tamburini E., Turolla E., Lanzoni M., Moore D., Castaldelli G. (2022). Manila clam and Mediterranean mussel aquaculture is sustainable and a net carbon sink. Sci. Total Environ. 848, 157508. doi: 10.1016/j.scitotenv.2022.157508
Valderrama J. C. (1981). The simultaneous analysis of total nitrogen and total phosphorus in natural waters. Mar. Chem. 10, 109–122. doi: 10.1016/0304-4203(81)90027-X
Villasuso-Palomares S., Gutiérrez-Wing M. T., Paniagua-Chávez C. G. (2022). Water quality and the CO2-carbonate system during the preconditioning of Pacific oyster (Crassostrea gigas) in a recirculating aquaculture system. Sci. Rep. 12, 22245. doi: 10.1038/s41598–022-26661–6
Wang W. L., Fu W., Le Moigne F. A., Letscher R. T., Liu Y., Tang J. M., et al. (2023a). Biological carbon pump estimate based on multidecadal hydrographic data. Nature 624, 579–585. doi: 10.1038/s41586-023-06772-4
Wang Y., Yang W., Zhao X., Zhang Q., Chen H., Fang Z., et al. (2023b). Changes in the carbon source and storage in a cultivation area of macro-algae in Southeast China. Mar. pollut. Bull. 188, 114680. doi: 10.1016/j.marpolbul.2023.114680
Wang X., Zhou T., Ying Z., Wu J., Yang W. (2020). Analyses of water quality and driving forces in Ningde aquaculture area. Acta Ecol. Sin. 40, 1766–1778. doi: 10.5846/stxb201901240180
Wu P., Wang T., Liu Y., Li C., Xiao Y., Xu S., et al. (2022). Differences of macroalgal consumption by eight herbivorous coral reef fishes from the Xisha Islands, China. Front. Mar. Sci. 9. doi: 10.3389/fmars.2022.882196
Xie B., Huang J., Huang C., Wang Y., Shi S., Huang L. (2020). Stable isotopic signatures (δ13C and δ15N) of suspended particulate organic matter as indicators for fish cage culture pollution in Sansha Bay, China. Aquaculture 522, 735081. doi: 10.1016/j.aquaculture.2020.735081
Xiong T., Li H., Hu Y., Zhai W. D., Zhang Z., Liu Y., et al. (2024). Seaweed farming environments do not always function as CO2 sink under synergistic influence of macroalgae and microorganisms. Agricult. Ecosyst. Environ. 361, 108824. doi: 10.1016/j.agee.2023.108824
Xu Y. Y., Pierrot D., Cai W. J. (2017). Ocean carbonate system computation for anoxic waters using an updated CO2SYS program. Mar. Chem. 195, 90–93. doi: 10.1016/j.marchem.2017.07.002
Xu C., Su G., Zhao K., Xu X., Li Z., Hu Q., et al. (2022). Current status of greenhouse gas emissions from aquaculture in China. Water Biol. Secur. 1, 100041. doi: 10.1016/j.watbs.2022.100041
Xue M., Chen Y., Tian X., Yan M., Zhang Z. (2019). Detection of marine aquaculture in Sansha bay by remote sensing. Mar. Environ. Sci. 38, 730–735. doi: 10.12111/j.mes20190513
Ying Z., Wu J., Valle T. M. D., Yang W. (2020). Spatiotemporal dynamics of coastal aquaculture and driving force analysis in Southeastern China. Ecosys. Health Sustainabil. 6, 1851145. doi: 10.1080/20964129.2020.1851145
Yuan J., Xiang J., Liu D., Kang H., He T., Kim S., et al. (2019). Rapid growth in greenhouse gas emissions from the adoption of industrial-scale aquaculture. Nat. Climate Change 9, 318–322. doi: 10.1038/s41558-019-0425-9
Zhai W., Zhao H., Zheng N., Xu Y. (2012). Coastal acidification in summer bottom oxygen-depleted waters in northwestern-northern Bohai Sea from June to August in 2011. Chin. Sci. Bull. 57, 1062–1068. doi: 10.1007/s11434-011-4949-2
Zhang Y., Tang K. W., Yang P., Yang H., Tong C., Song C., et al. (2022). Assessing carbon greenhouse gas emissions from aquaculture in China based on aquaculture system types, species, environmental conditions and management practices. Agricult. Ecosyst. Environ. 338, 108110. doi: 10.1016/j.agee.2022.108110
Zhang Y., Zhang J., Liang Y., Li H., Li G., Chen X., et al. (2017). Carbon sequestration processes and mechanisms in coastal mariculture environments in China. Sci. China Earth Sci. 60, 2097–2107. doi: 10.1007/s11430-017-9148-7
Keywords: carbonate system, pCO2, mariculture, kelp farming season, marine carbon sink
Citation: Zhang Z, Wang F, Lei L, Zheng N, Shen Z and Mu J (2024) Spatio-temporal dynamics of the carbonate system during macroalgae farming season in a semi-closed bay in southeast China. Front. Mar. Sci. 11:1375839. doi: 10.3389/fmars.2024.1375839
Received: 24 January 2024; Accepted: 13 May 2024;
Published: 07 June 2024.
Edited by:
Licheng Peng, Hainan University, ChinaCopyright © 2024 Zhang, Wang, Lei, Zheng, Shen and Mu. This is an open-access article distributed under the terms of the Creative Commons Attribution License (CC BY). The use, distribution or reproduction in other forums is permitted, provided the original author(s) and the copyright owner(s) are credited and that the original publication in this journal is cited, in accordance with accepted academic practice. No use, distribution or reproduction is permitted which does not comply with these terms.
*Correspondence: Jingli Mu, amxtdUBtanUuZWR1LmNu
†These authors have contributed equally to this work
Disclaimer: All claims expressed in this article are solely those of the authors and do not necessarily represent those of their affiliated organizations, or those of the publisher, the editors and the reviewers. Any product that may be evaluated in this article or claim that may be made by its manufacturer is not guaranteed or endorsed by the publisher.
Research integrity at Frontiers
Learn more about the work of our research integrity team to safeguard the quality of each article we publish.