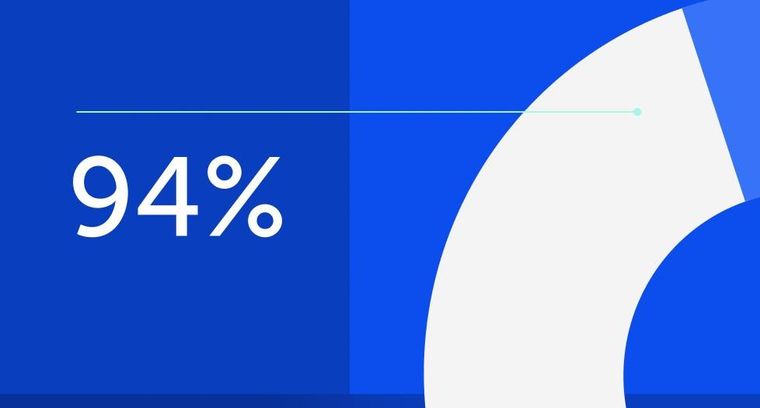
94% of researchers rate our articles as excellent or good
Learn more about the work of our research integrity team to safeguard the quality of each article we publish.
Find out more
ORIGINAL RESEARCH article
Front. Mar. Sci., 05 March 2024
Sec. Aquatic Microbiology
Volume 11 - 2024 | https://doi.org/10.3389/fmars.2024.1371296
This article is part of the Research TopicWomen in Aquatic Microbiology: 2023View all 18 articles
The heterotrophic dinoflagellate Oxyrrhis marina is an essential microzooplankton in coastal waters, linking the energy transfer from phytoplankton to higher trophic levels. It is of general significance to investigate how it responds and acclimates to ocean acidification (OA), especially under varied availabilities of food. Here, O. marina was exposed and acclimated to three pCO2 levels (LC: 415, MC:1000, HC:1500 μatm) for 60 days, and then was further grown under the CO2 levels with different levels of food (the microalgae Dunaliella salina) availability for about 8 generations. The OA treatments did not significantly hamper its growth and ingestion rates even under the reduced food availability and starvation (deprived of the microalgae), which significantly reduced its growth rate. While the impacts of OA on the growth and ingestion rates of O. marina were insignificant, the OA treatments appeared to have resulted in a faster decline of the heterotrophic dinoflagellate cells during the starvation period. Nevertheless, the acidic stress under the elevated pCO2 of 1000 or 1500 μatm decreased its respiration by about 53% or 59% with the high and by about 26% or 23% with the low food availability, respectively. Such OA-repressed respiration was also significant during the starvation period. On the other hand, the OA treatments and deprivation of the microalgae synergistically reduced the cellular quota of particulate organic C, N and P, resulting in a reduction of food value of the heterotrophic dinoflagellate as prey. In conclusion, our results show that O. marina is highly resilient to future ocean acidification by reducing its respiration and sustaining its ingestion of microalgae.
Ocean acidification (OA) caused by increasingly dissolved anthropogenic CO2 in seawater is known to affect many marine organisms along with other global change drivers (Häder and Gao, 2023). Atmospheric CO2 concentration is increasing by over 2 ppmv annually and is predicted to reach 1000 μatm by the end of this century and 1900 μatm by the year 2300 (Caldeira and Wickett, 2003; Gattuso et al., 2015). Seawater chemical changes associated with ocean acidification include increased concentrations of H+ and HCO3- and decreased CO32- concentration and CaCO3 saturation state, which have been documented to influence both marine primary, secondary producers and even higher tropic levels (see the review by (Jin et al., 2020) and literatures therein). OA has been shown to disturb the sperm flagella motility of reef invertebrates (Morita et al., 2010) and intracellular acid-base stability (Whiteley, 2011), reducing metabolism and disrupting acid-base homeostasis in many heterotrophs (Michaelidis et al., 2005; Miles et al., 2007). Compared to the large organisms, smaller ones may be more sensitive to the acidic stress due to large surface to volume ratios (Flynn et al., 2012). However, the effects of OA on microzooplankton, a heterotroph group in the size of 20-200 µm, have been scarcely documented (Caron and Hutchins, 2012; López-Abbate, 2021).
Microzooplankton consumes about 60-75% of daily phytoplankton production (Calbet and Landry, 2004; Schmoker et al., 2013), serving as food for mesozooplankton and most marine fish larvae (Fukami et al., 1999; Calbet, 2008). Therefore, responses of microzooplankton species to environmental changes are of general significance, considering their cascade effects on marine ecosystems. Progressive ocean acidification is suggested to affect zooplankton directly due to acidic stress and indirectly because of altered phytoplankton food quality (Jin et al., 2015). Results from mesocosm experiments using field plankton communities showed that OA negatively affected microzooplankton in coastal waters (Park et al., 2014; Horn et al., 2020; Spisla et al., 2021), though opposite results were reported in another study conducted in the coastal North Sea (Moreno et al., 2022). While decreased pH had no detectable effect on the growth and grazing of microzooplankton communities (Suffrian et al., 2008; Aberle et al., 2013; Horn et al., 2016; Wang et al., 2019), it has little been documented on how heterotrophic dinoflagellates respond to OA. It has been shown that 4-day exposure to high seawater pCO2 did not alter the growth of the heterotrophic dinoflagellate Oxyrrhis marina (Meunier et al., 2017). Conversely, O. marina was negatively impacted by acidic stress under lowered pH by about 0.3 unit (Pedersen and Hansen, 2003). Such controversial findings need to be verified under different environmental conditions and food availabilities. Here, we hypothesize that OA affects heterotrophic dinoflagellates to different extents under different levels of food availability because a sufficient energy supply derived from the digestion of food may help them to cope with the acidic stress. We investigated how O. marina responds to elevated CO2 concentrations under different levels of food availability, including starvation, and found that it could reduce its respiratory cost and its growth rate was insensitive to the carbonate chemistry changes projected for future acidification even under the conditions without supplying microalgae as food.
The heterotrophic dinoflagellate Oxyrrhis marina (CCMA174; ca 16μm in ESD) initially isolated from Dongshan Bay (117°29’-117°36’ E, 23°34’-23°54’ N), southeast China, was obtained from the Center for Collections of Marine Algae (CCMA, Xiamen University). The cells were inoculated into sterilized artificial seawater at temperature 24°C and salinity 30‰ under 20 µmol photons m-2 s-1, with a light: dark cycle set to 12h:12h. The dim light level was chosen to limit the growth of possible residual alga food, so that carbonate chemistry stability was maintained. The temperature and salinity were close to the strain’s original environment (Chen et al., 2014). The green microalgae, Dunaliella salina (CCMA352; ESD, ca 6.88 μm), was separately grown as food stock. Before initiating the experiments, O. marina cells in 500 mL polycarbonate flasks were exposed and acclimated to ambient (low, 415 µatm), medium (1000 µatm), and high (1500 µatm) CO2 levels, representing the ambient, that by the end of this century (Gattuso et al., 2015) and that predicted for 2160 (Vargas et al., 2017) for about two months with the pH variations in cultures under each pCO2 level being less than 0.05 unit (Supplementary Figure 1). The different CO2 concentrations were achieved using ambient outdoor air or a mixture of pure CO2 with ambient air using a CO2 enricher (HP1000G-D, Ruihua, China). All cultures were gently aerated (about 300 mL min-1, 0.22μm filtered) to ensure the carbonate chemistry stability. Such pre-acclimation under the different pCO2 levels was carried out in semi-continuous cultures (diluted every 3 days) with an initial cell density of about 1500 cells/mL and fed with the microalgae food (15,000 cells mL-1). O. marina cultures were diluted about once a week and the cell densities were generally not more than 10,000cells mL-1 before the diluting. To minimize the photosynthetic effects of the microalgae on pH, the food supply to O. marina was carried out at the early night period. In order not to dilute the O. marina cultures by adding the food, the microalgae cells (about 15,000 cells mL-1) were harvested by centrifugation at 2,000 g for 10 min then resuspended in 1.5-3 mL sterilized seawater prior to each feeding. No residual microalgae cells were microscopical found before the feeding.
The experiment was designed to investigate the effects of future-projected OA under different levels of food availability on O. marina. The whole experiment was divided into two phases, the feeding phase (from Day 1 to Day 19) and the subsequent starvation phase (from Day 19 to Day 43). In the feeding period, the dinoflagellate cells were fed with the microalgae daily under the targeted pCO2 levels. During the starvation phase, the microalgae was not supplied. The CO2 levels were maintained the same as mentioned above. Two food concentrations in the feeding period were set: about 8,000 cells mL-1day-1(LF), and 24,000cells mL-1day-1 (HF), respectively. Both of the food biomass densities (< 72 μg C L−1d-1), reflecting the mean values reported in coastal waters (Majewska et al., 2017; Ok et al., 2021), are however below the mean satiation values (100 μg C L-1d-1) for O. marina (Jeong et al., 2001). The culture conditions were the same as in the pre-acclimation mentioned above. The cell concentration of O. marina was semi-continuously controlled with an initial density of approximately 1,500 cells mL-1 by dilutions every 3 days to ensure cell concentrations maintained in the exponential growth phase (Figure 1) and under stable carbonate chemistry condition (Supplementary Table 1). The cell ranges for exponential growth phase under the targeted pCO2 and food levels were determined by a primary test (Supplementary Figure 2). The dinoflagellate cells were grown under the different food availabilities for about 8 generations before being used for physiological and biochemical measurements. Due to the different growth rates, the acclimation time for each treatment was different (Figure 1): 10 days for HF, and 19 days for LF treatments. Subsequently, the starvation period lasted for 24 days under the same conditions without any algal food being supplied.
Figure 1 Changes of cell density of O. marina and microalgae food D. salina under three pCO2 levels (LC of 415, MC of 1000, and HC of 1500 μatm) in the semi-continuously diluted cultures. The experiment included the feeding period (about 8 generations growth of O. marina, from Day 1 to Day 19 for LF and from Day 10 to Day 19 for HF) and the starvation period (from Day 19 to Day 43). The physiological main parameters were measured on Day 19 and Day 27 (arrows), for the fed and starved cells, respectively. Values are represented as the means ± SD of triplicate cultures.
The ingestion rates were measured during the feeding period. The respiration rates, and cellular particulate C/N/P (POC, PON, and POP) of O. marina were determined on the first day and the ninth day after stripping of algal food, representing the fed and starved status of the cells, respectively. Because no residual alga food remained in the cultures after 12 hours of feeding (verified microscopically), the dinoflagellate samples were taken without any algal cells involved. Abundance of bacteria that naturally existed in the cultures was measured since they can be the food for O. marina (Jeong et al., 2008; Roberts et al., 2011). Dissolved organic carbon was also monitored for it could support the growth of bacteria.
The specific growth rate was calculated using the following equation:
where N3 and N0 are respectively the cell concentrations at t3 and t0. The (t3-t0) was 3 days. A 1 mL sample was fixed with acidifying Lugol’s iodine (2%) and then counted using an inverted microscope (Olympus CK30) to measure predator and alga food densities.
Ingestion rate (cells O. marina-1 h-1) was calculated according to the equation (Frost, 1972) as follows:
where Ct′ and Ct (cells mL−1) represent the food concentrations at the end of the incubation in control and experimental cultures, respectively; C0 is the food concentration at the start of the incubation; V is the culture volume (mL), t (hour) is the incubation time and n is the number of O. marina; F represents the volume of ambient medium from which cells are completely removed by predators to achieve the measured ingestion rate; [C] is the food concentrations in the experimental cultures averaged over the incubation period. In parallel, the cultures with the microalgae cells alone were served as controls. This parameter was measured at night to avoid the photosynthetic CO2 removal that may affect pH.
The filtrate samples were fixed with glutaraldehyde (0.5%, v/v) for 15 minutes in the dark before being freezed in liquid nitrogen, and then stored at -80°C till analysis. Before bacteria counting, samples were thawed at room temperature and diluted 1:10 with 0.2μm-filtered Tris-EDTA buffer, and finally, samples were stained with SYBR Green I nucleic acid gel stain (final concentration 1:10,000; Invitrogen, America) for 15 minutes. These SYBR-stained samples were enumerated using a Flow Cytometer (Epics Altra II, Beckman Coulter, America). Millipore water and 0.2μm filtered seawater were used as controls.
The respiration rate (pmol O2 O. marina-1 h-1) was estimated based on the oxygen consumption. Dissolved O2 concentration was measured using an optical oxygen sensor (Microx, PreSence, Germany), and a mini-magnetic stirrer was placed at the bottom of a sealed flat-bottomed glass vessel (2.5cm in diameter and 50mL in volume) to homogenize the oxygen concentration. The measurements of respiration rate were carried out with the same density of O. marina cells collected from HF and LF treatments. The samples were incubated in the 50 mL centrifuge tube for 24 h (during which a decrease in O2 concentration was confirmed). All measurements were done under conditions similar to that of the cultures. There was no food residual (confirmed microscopically) in the measured samples. Additional samples filtered off O. marina cells were used as controls to correct the background disturbance from bacterial respiration, which was measured in parallel. pH in each tube was stable before and after the determination.
To measure the cellular particulate carbon (POC), nitrogen (PON) and phosphorus (POP), the dinoflagellate cells alone (without the microalgae) were filtered onto pre-combusted (450°C,4h) Whatman GF/F membranes (25mm, Whatman, America), and then stored at -20°C until analysis. For cellular carbon and nitrogen analysis, the membranes were fumed with HCl in a closed container for at least 12 h and dried at 60°C overnight to remove the inorganic elements as gas. A CHN element analyzer (Vario EL cube, Langenselbold, Germany) was used to analyze the dried samples. To measure cellular phosphorus, the Solórzano method (Solórzano and Sharp, 1980) was followed. Filters were soaked with 17 mM MgSO4, dried at 95°C, and then baked for 2 h at 450°C to convert the organic phosphorus to inorganic one. Then, the samples were acidified in 0.2 M HCl at 80°C for 30 minutes and subsequently centrifuged (10,000g, 10 minutes, room temperature). Concentration of phosphate ion in the supernatant was measured with an auto-analyzer (AA3, Seal, Germany), and was used to estimate the cellular POP quota.
40 mL cultures were filtered onto pre-combusted (450°C,4h) GF/F membranes (25mm, Whatman, America), the filtrate was collected using the acid cleaned brown glass bottle and stored at -20°C until analysis. Before analyzing, aqueous samples were thawed at room temperature and blended with phosphoric acid (0.1%, v/v). Finally, DOC was measured using a TOC-analyzer (TOC-VCPH, Shimadzu, Japan).
To assure the stability of seawater carbonate systems in cultures, pHNBS was measured before the dark period by a pH meter (OAKTON, USA), which was frequently calibrated with standard National Bureau of Standards (NBS) buffers. Total alkalinity (TA) was measured with the titration method (Lewis et al., 1998). Then, the CO2SYS software was used to convert pHNBS values to pHT and other carbonate chemistry parameters were calculated based on TA and pHT with the known temperature and salinity values (Roy et al., 1993). The seawater carbonate chemistry was stable, all the parameters are shown in Supplementary Table 1.
Data were analyzed by SPSS statistics 26 and plotted by GraphPad Prism 9.0. Two-way ANOVA was employed to test the differences among pCO2 (three levels), food availability (two levels), and their interactions. One-way ANOVA and Tukey test were applied to analyze the significance among different treatments. The normality, independence and homogeneity of variance were checked before analysis. The significance level was set to p< 0.05. All values represented the means ± SD of triplicate independent cultures.
After the dinoflagellate cells had pre-acclimated for about 23 generations under the different pCO2 levels, further treatments under the different pCO2 levels for another 8 generations did not bring out any difference in its specific growth rate, either under low (LF) or high (HF) food concentration (Figure 2A). During the starvation phase, the dinoflagellate cell density stayed stable in the first 4 days after stopping feeding, dramatically declined in the next 4 days, and further decreased to the minimal values till day 43 (Figure 1). In the feeding period, the growth rates of HF treatments increased dramatically by 212%, 209% and 258% under LC, MC, and HC levels compared with that of LF (all p< 0.001, Figure 2A), respectively. While the elevated pCO2 did not alter the growth rates under HF, but slightly decreased it (about 18%) under LF, though the difference was not significant (p = 0.087, Figure 2A). There were no interactive effects between food concentration and pCO2 level on the growth rate (two-way ANOVA, p = 0.152). During the starvation period, the cell concentrations decreased with time (- 0.045 day-1). However, the pCO2 treatments neither resulted in significant changes in the specific growth rates (p = 0.6661 for HF and p = 0.8146 for LF, Figure 2B).
Figure 2 Effect of CO2 concentrations and food supplies on the specific growth rate (μ, d-1) of O. marina during the feeding period (A) and the subsequent starvation [24 days, (B)]. In the feeding period, O. marina was fed with two concentrations of microalgae (HF: 24000 cells mL-1day-1 and LF: 8000 cells mL-1day-1). In the starvation period, all treatments were deprived of the microalgae. Starved-HF and Starved-LF, respectively, indicate the starved cells from HF or LF cultures. (C) The respiration rate (pmol O2 O. marina cell-1 h-1) with two alga food concentrations (HF and LF) under the different pCO2 levels. (D) The respiration rates of O. marina cells (Starved-HF and Starved-LF) after 8 d of starvation under different pCO2 levels. Values are the means ± SD of triplicate cultures. Different letters above the data indicate significant differences (p< 0.05) among the treatments.
The respiration rates were significantly reduced by the elevated pCO2 levels (p<0.001, both for fed and starved cells) and the increased food availability (p< 0.001), but not by their interaction (Figures 2C, D, two-way ANOVA, p = 0.590 for fed cells and p = 0.107 for starved cells). In the feeding period, LF-grown cells increased respiration rate by 48% under LC (p< 0.001), 67% under MC (p< 0.001), and 72% under HC (p<0.001), compared with that of HF-grown ones, respectively (Figure 2C). In the starvation period, LF-starved cells increased respiration rates by 40% under LC (p = 0.005), 66% under MC (p< 0.001), and 73% under HC (p< 0.001), compared with that of the HF-starved cells, respectively (Figure 2D). Obviously, the OA treatment decreased the dinoflagellate’s respiration either with HF or LF or during starvation. The respiration rates of LC-grown cells were significantly higher than those of MC (by 53% with HF and 26% with LF; by 55% and 21% in HF-starved and LF-starved cells, respectively) and HC (by 59% and 23% with HF and LF; by 66% and 24% in HF-starved and LF-starved cells, respectively) (Figures 2C, D, with p values for the above difference ranged from 0.0001 to 0.0222). However, there were no statistical differences between MC and HC either for fed or starved periods. Furthermore, in all the cultures, the respiration rates during the starved phase were lower compared that of the feeding period (Figures 2C, D, with p values ranged 0.001-0.0358) regardless of the pCO2 levels. The highest pCO2 resulted in the most reduction in the respiration ratio of the fed to the starved cells (Supplementary Table 2), by up to 30-36% for the fed cells transferred to the starved condition.
Ingestion rates of O. marina during the feeding period were 185%, 199%, and 185% higher in the HF cultures compared with LF at LC (p = 0.001), MC (p = 0.001), and HC (p = 0.003) (Figure 3). It appears that elevated pCO2 gradually decreased the ingestion rate under either HF or LF, but the difference was insignificant (Figure 3, p = 0.0979 for HF and p = 0.2743 for LF). No interactive effects were detected between food concentration and pCO2 level on the ingestion rate (two-way ANOVA, p = 0.529).
Figure 3 The ingestion rate (cells O. marina-1 h-1) of the dinoflagellate grown with two different alga food concentrations (HF and LF as in Figure 2) under three pCO2 levels. One D.salina cell had 0.003 ± 0.0004 ng C. Values are the means ± SD of triplicate cultures. Different letters above the data indicate significant differences (p< 0.05) among the treatments.
The cellular quotas of POC, PON, and POP were significantly affected by food availability (p = 0.007, p = 0.035, p = 0.001 for the fed cells; p = 0.001, p<0.001, p<0.001 for the starved cells, correspondingly), however, they were not influenced by the pCO2 levels (p = 0.985, p = 0.943, p = 0.542 for the fed cells, p = 0.341, p = 0.599, p = 0.107 for the starved cells, Figure 4). There was no interaction of pCO2 and food availability (Figure 4, p values ranged 0.061- 0.982). HF-fed cells appeared to possess higher quotas of POC, PON, and POP than those of LF-fed cells (Figures 4A–C), and such pattern continued to the starvation period, though the differences were not always significant (Figures 4D–F).
Figure 4 (A–C) The cellular particular organic carbon (POC), particular organic nitrogen (PON), and particular organic phosphorus (POP) in O. marina cells fed with two alga food concentrations (HF and LF as in Figure 2) under the different pCO2 levels. (D–F) The cellular POC, PON, and POP in O. marina after 8 d of starvation (Starved-HF and Starved-LF as in Figure 2) under the different pCO2 levels. Values are the means ± SD of triplicate cultures. Different letters above the data indicate significant differences (p< 0.05) among treatments.
After the 8-days starvation, the survived O. marina cells displayed significant decreases in the quotas compared to that in the feeding period (Figure 4), reflecting that the starvation reduced the cells’ nutritional value. The decreased percentage of the quotas from the fed to the corresponding starved cells were as follows: POC by 32-42%, PON by 22-36%, and POP by 7-19%. Such decrease of the quotas was pronounced with the increased pCO2 levels (Supplementary Table 2). The elevated pCO2 reduced the quota in the Starved-LF cells (Figures 4D–F). Compared to the LC, POC quotas of starved-LF cells decreased by 10% under MC (p = 0.23) and 13% (p = 0.059) under HC, quotas of PON decreased by 11% under MC (p = 0.203) and 12% (p = 0.162) under HC, quotas of POP decreased by 16% under MC (p = 0.066) and 15% (p = 0.017) under HC, respectively. However, the elevated pCO2 levels did not influence the molar ratios of C: N, C: P and N: P in both the fed and starved cells (Figure 5, p values ranged from 0.277 to 0.942). Compared to the fed O. marina cells, all the starved ones showed significant decreases in the C: N (Figures 5A, D), C:P ratios (Figures 5B, E) and insignificant decrease in the N: P ratios (Figures 5C, F). The larger decline was observed in the C: P, especially in the cells transferred from fed to starvation under the highest pCO2 level (Supplementary Table 2).
Figure 5 (A–C) The molar ratios of C: N, C: P, and N: P in O. marina cells fed with two alga food concentrations (HF and LF as in Figure 2) under the different pCO2 levels. (D–F) The molar ratios of O. marina (Starved-HF and Starved-LF as in Figure 2) after 8 d of starvation. Values are the means ± SD of triplicate cultures. Different letters above the data indicate significant differences (p< 0.05) among treatments.
Our results showed that the heterotrophic dinoflagellate O. marina could reduce its respiration to cope with the acidic stress associated with future ocean acidification (OA) even under reduced levels of food availability or during starvation, disapproving our hypothesis that OA can decrease the growth of the heterotrophic dinoflagellate under food scarcity. Nevertheless, we found that OA did reduce the quotas of POC, PON, and POP in O. marina after depriving of microalgae, implying nutritional deterioration.
The growth and ingestion rates of microzooplankton can be food availability-dependent (Jeong et al., 2001; Adolf et al., 2007). However, to date, the combined effects of ocean acidification and food availability has been little examined. It has been suggested that the OA effects could be species-specific in heterotrophic dinoflagellates (Meunier et al., 2017; Moreno et al., 2022) and other zooplankton grazers (Crook et al., 2013; Hurst et al., 2017; Li et al., 2017; Brown et al., 2018). In the present study, no interactive effects between food availability and OA were observed. Such resilience to OA could be attributed to the dinoflagellate’s niche where naturally strong pCO2 fluctuations enable them to possess the tolerating capacity (Majewska et al., 2017; Meunier et al., 2017). Food scarcity has been shown to exacerbate the impacts of OA on several predators (Thomsen et al., 2013; Pedersen et al., 2014; Li et al., 2017). In this work, the high and low food concentrations that reflected different levels of food availability below the satiate feeding (Roberts et al., 2011) and were close to that in the environment where O. marina is common (Jung et al., 2021; Ok et al., 2021), did not alter the impacts of OA, which reduced its respiration (Figures 2C, D) but did not alter its growth and ingestion (Figures 2A, B, 3).
The resistance for the O. marina cells to expel respiratory CO2 could be stronger under the elevated pCO2 since CO2 diffuses very slow in water (about 8,000 times slower compared to that in air). This could be partially responsible for the reduction of the dinoflagellate’s respiration. Alternatively, repressing respiration under OA could be a strategy for O. marina to cope with acidic stress. Metabolic responses to the acidic stress associated with elevated pCO2 are thought to be related to energetic trade-offs (Gao, 2017). Acidification-driven energy cost coupled with acid-base regulation has been reported in heterotrophic species (Pan et al., 2015; Li et al., 2017; Frieder et al., 2018). The respiration depression observed at lowered pH (Figures 2C, D) was reported in other organisms, such as in a starfish (Collard et al., 2013). On the contrary, other studies showed that OA either stimulated respiration (Li and Gao, 2012; Meunier et al., 2017) or did not affect it (Runge et al., 2016) in copepods. For the heterotrophic dinoflagellate O. marina, reduced metabolic cost due to repressed respiration could save energy for it to sustain its growth (Osma et al., 2016). In the present work, the saved energy from the down-regulated respiration under elevated pCO2 could be utilized to sustain cellular acid-base homeostasis. Thus, no obvious differences in growth rates were found between the LC, MC and HC treatments. Most aquatic organisms can use diverse ionic pumps and enzymes including H+-ATPases and Na+/K+-ATPases to maintain the intracellular acid-base stability to counteract external acidic stress under lowered pH (Pörtner et al., 2000). O. marina might also employ similar mechanism. On the other hand, decreased pH may inhibit the motility of O. marina and thus save the energy for its growth, since OA treatment was shown to impede the motility of flagellated microalgae (Wang et al., 2020). In natural environments, microalgae food is not always enough, O. marina often lives near the bottom where bacteria are abundant (Jeong et al., 2008). Provided that the motility of O. marina be inhibited under lowered pH, it might graze relatively more bacteria since they are easier to be caught than the moving microalgae, though higher ingestion rate on microalgae than on bacteria has been suggested under normal conditions (Roberts et al., 2011). On the other hand, enhanced motility could give rise to increased respiration. In the present work, regardless of the pCO2 levels, O. marina exhibited higher respiration when fed with the low food concentration (Figure 2C), which could be due to more movements searching for food (it can swim as fast as 700 μm s-1) (Cosson et al., 1988) and its swimming activities negatively correlate with food availability (Bartumeus et al., 2003). Contrastingly, the OA-induced repression of respiration reflects less swimming activity, though future studies are expected to provide experimental evidence.
Starvation by depriving of microalgae did resulted negative growth rates under all the pCO2 levels (Figure 2B), but the dinoflagellate could survive by ingesting bacteria for 24 days (Figures 1, 6A, B), even longer than a month (Jeong et al., 2008). The relatively higher abundance of bacteria under the elevated pCO2 during the starvation (after 8-days depriving of microalgae) could be attributed to enhanced decomposition of dissolved organic matter under OA (Lønborg et al., 2020). In the present study, compared with the LC cultures, DOC in the HC cultures was significantly higher (Figure 6C), which indeed coincided with a lower decreased ratio of bacterial abundance (Figures 6A, B). While the O. marina cell abundance declined faster under the elevated pCO2 levels during starvation (Figure 1), the dead cells could have led to increased levels of DOC (Strom et al., 1997) due to enhance decomposition by bacteria (Figure 6). Although we did not directly measure the ingestion rate of bacteria by O. marina, OA-repressed respiration of the dinoflagellate might have led to a reduced ingestion of bacteria during the starvation period, therefore, resulting apparently higher levels of bacterial abundance (Figure 6B).
Figure 6 (A) Bacteria abundances (105 ind mL-1) in fed O. marina cultures (cells supplied with microalgae) at Day 19 under the different pCO2 levels. (B) Bacteria abundances (105 ind mL-1) in the subsequent starved cultures (deprived of microalgae) on Day 27 under the different pCO2 levels. (C) The dissolved organic carbon (DOC, μmol L-1) in the cultures during the starvation, measured at Day 27. The values represent the means ± SD of triplicate cultures. Different letters above the data indicate significant differences (p< 0.05) among treatments.
It has been suggested that O. marina is a weak homeostasis species for its prompt alteration in stoichiometric composition (C:N:P) (Golz et al., 2015). Our results demonstrated that the stoichiometric composition remained relatively stable irrespective of the pCO2 levels and food availabilities (Figure 5). The cellular quotas of organic C/N/P and their ratios (Figures 4, 5) fell within the range measured for O. marina in view of the fed cells (Hantzsche and Boersma, 2010; Golz et al., 2015). During the starvation, however, all the cellular quotas declined significantly, which was especially exacerbated under the elevated pCO2 levels during the starvation (Figure 4). The decline of PON and POP was significantly stronger compared to POC. This implies that starvation and OA act synergistically to reduce the nutritional quality of the heterotrophic dinoflagellate. Comparing the data between the fed and starved cells, the OA treatment led to enhanced repression of respiration along with pronounced reduction of POC, PON, and POP quotas (Figures 2C, D, 4; Supplementary Table 2). Consequently, the combination of food scarcity and OA can deteriorate the dinoflagellate’s nutritional value. The greater loss in PON and POP (Figure 4) hints a more serious inhibition of flagella motility because flagella is composed of plentiful protein components (Gagnon et al., 1996), which in turn is responsible for the repressed respiration (Figures 2C, D).
The heterotrophic dinoflagellate O. marina is a cosmopolitan microzooplankton species often found in coastal waters (Watts et al., 2011). It usually experiences fluctuating pCO2 and pH (Dai et al., 2009; Waldbusser and Salisbury, 2014) as well as phytoplankton food variation (Sherr and Sherr, 2009; Ok et al., 2021). To cope with those stresses, it can adjust cellular components and processes, such as structural proteins (Osma et al., 2016), ion pumps (Bailey et al., 2017), respiration rate and nutritional compositions (this work). Consequently, its robust resilience to acidic stress under future ocean acidification scenarios reflects that the heterotrophic dinoflagellate will be most likely one of the “winners” in future ocean, though the degradation of its nutritional values would influence organisms of high trophic levels, which needs to be explored under impacts of multiple climate change drivers in future studies.
The original contributions presented in the study are included in the article/Supplementary Material. Further inquiries can be directed to the corresponding author.
The manuscript presents research on animals that do not require ethical approval for their study.
KG: Conceptualization, Funding acquisition, Supervision, Validation, Writing – review & editing. NW: Data curation, Formal analysis, Investigation, Methodology, Software, Writing – original draft.
The author(s) declare financial support was received for the research, authorship, and/or publication of this article. This study was supported by the national key R&D program (2022YFC3105303) and National Natural Science Foundation of China (42361144840).
The authors are grateful to the former Ph.D. student He Li for his invaluable assistance in installing the CO2 enrichers, to the Ph.D. student Chuze Zou for his technical support in bacteria counting, and to the Ph.D. student Weijia for contributing to the part of sampling. We also thank the laboratory engineers Xianglan Zeng and Wenyan Zhao for their logistical and technical supports.
The authors declare that the research was conducted in the absence of any commercial or financial relationships that could be construed as a potential conflict of interest.
All claims expressed in this article are solely those of the authors and do not necessarily represent those of their affiliated organizations, or those of the publisher, the editors and the reviewers. Any product that may be evaluated in this article, or claim that may be made by its manufacturer, is not guaranteed or endorsed by the publisher.
The Supplementary Material for this article can be found online at: https://www.frontiersin.org/articles/10.3389/fmars.2024.1371296/full#supplementary-material
Aberle N., Schulz K. G., Stuhr A., Malzahn A. M., Ludwig A., Riebesell U. (2013). High tolerance of microzooplankton to ocean acidification in an Arctic coastal plankton community. Biogeosciences 10, 1471–1481. doi: 10.5194/bg-10-1471-2013
Adolf J. E., Krupatkina D., Bachvaroff T., Place A. R. (2007). Karlotoxin mediates grazing by Oxyrrhis marina on strains of Karlodinium veneficum. Harmful Algae 6, 400–412. doi: 10.1016/j.hal.2006.12.003
Bailey A., De Wit P., Thor P., Browman H. I., Bjelland R., Shema S., et al. (2017). Regulation of gene expression is associated with tolerance of the Arctic copepod Calanus glacialis to CO2-acidified sea water. Ecol. Evol. 7, 7145–7160. doi: 10.1002/ece3.3063
Bartumeus F., Peters F., Pueyo S., Marrasé C., Catalan J. (2003). Helical Lévy walks: Adjusting searching statistics to resource availability in microzooplankton. Proc. Natl. Acad. Sci. U.S.A. 100, 12771–12775. doi: 10.1073/pnas.2137243100
Brown N. E. M., Bernhardt J. R., Anderson K. M., Harley C. D. G. (2018). Increased food supply mitigates ocean acidification effects on calcification but exacerbates effects on growth. Sci. Rep. 8, 9800. doi: 10.1038/s41598-018-28012-w
Calbet A. (2008). The trophic roles of microzooplankton in marine systems. ICES J. Mar. Sci. 65, 325–331. doi: 10.1093/icesjms/fsn013
Calbet A., Landry M. R. (2004). Phytoplankton growth, microzooplankton grazing, and carbon cycling in marine systems. Limnol. Oceanogr. 49, 51–57. doi: 10.4319/lo.2004.49.1.0051
Caldeira K., Wickett M. E. (2003). Anthropogenic carbon and ocean pH. Nature 425, 365. doi: 10.1038/425365a
Caron D. A., Hutchins D. A. (2012). The effects of changing climate on microzooplankton grazing and community structure: drivers, predictions and knowledge gaps. J. Plankton Res. 35, 235–252. doi: 10.1093/plankt/fbs091
Chen B. H., Ji W. D., Zhou K. W., He Q., Fu T. T. (2014). Nutrient and eutrophication characteristics of the Dongshan Bay, South China. Chin. J. Oceanol. Limnol. 32, 886–898. doi: 10.1007/s00343-014-3214-3
Collard M., Catarino A. I., Bonnet S., Flammang P., Dubois P. (2013). Effects of CO2-induced ocean acidification on physiological and mechanical properties of the starfish Asterias rubens. J. Exp. Mar. Biol. Ecol. 446, 355–362. doi: 10.1016/j.jembe.2013.06.003.
Cosson J., Cachon M., Cachon J., Cosson M.-P. (1988). Swimming behaviour of the unicellular biflagellate Oxyrrhis marina: in vivo and in vitro movement of the two flagella. Biol. Cell. 63, 117–128. doi: 10.1016/0248-4900(88)90050-0
Crook E. D., Cooper H., Potts D. C., Lambert T., Paytan A. (2013). Impacts of food availability and pCO2 on planulation, juvenile survival, and calcification of the azooxanthellate scleractinian coral Balanophyllia elegans. Biogeosciences 10, 7599–7608. doi: 10.5194/bg-10-7599-2013
Dai M. H., Lu Z. M., Zhai W. D., Chen B. S., Cao Z. M., Zhou K. B., et al. (2009). Diurnal variations of surface seawater pCO2 in contrasting coastal environments. Limnol. Oceanogr. 54, 735–745. doi: 10.4319/lo.2009.54.3.0735
Flynn K. J., Blackford J. C., Baird M. E., Raven J. A., Clark D. R., Beardall J., et al. (2012). Changes in pH at the exterior surface of plankton with ocean acidification. Nat. Clim. Change 2, 510–513. doi: 10.1038/nclimate1489
Frieder C. A., Applebaum S. L., Pan T. C. F., Manahan D. T. (2018). Shifting balance of protein synthesis and degradation sets a threshold for larval growth under environmental stress. Biol. Bull. 234, 45–57. doi: 10.1086/696830
Frost B. W. (1972). Effects of size and concentration of food particles on the feeding behavior of the marine planktonic copepod Calanus pacificus. Limnol. Oceanogr. 17, 805–815. doi: 10.4319/lo.1972.17.6.0805
Fukami K., Watanabe A., Fujita S., Yamaoka K., Nishijima T. (1999). Predation on naked protozoan microzooplankton by fish larvae. Mar. Ecol. Prog. Ser. 185, 285–291. doi: 10.3354/meps185285
Gagnon C., White D., Cosson J., Huitorel P., Eddé B., Desbruyéres E., et al. (1996). The polyglutamylated lateral chain of alpha-tubulin plays a key role in flagellar motility. J. Cell Sci. 109, 1545–1553. doi: 10.1242/jcs.109.6.1545
Gao K. (2017). Changes in bioenergetics associated with ocean acidification and climate changes. Bioenergetics 06, 147. doi: 10.4172/2167-7662.1000147
Gattuso J. P., Magnan A., Billé R., Cheung W. W. L., Howes E. L., Joos F., et al. (2015). Contrasting futures for ocean and society from different anthropogenic CO2 emissions scenarios. Science 349, aac4722. doi: 10.1126/science.aac4722
Golz A. L., Burian A., Winder M. (2015). Stoichiometric regulation in micro- and mesozooplankton. J. Plankton Res. 37, 293–305. doi: 10.1093/plankt/fbu109
Häder D. P., Gao K. S. (2023). Aquatic productivity under multiple stressors. Water 15, 817. doi: 10.3390/w15040817
Hantzsche F. M., Boersma M. (2010). Dietary-induced responses in the phagotrophic flagellate Oxyrrhis marina. Mar. Biol. 157, 1641–1651. doi: 10.1007/s00227-010-1437-1
Horn H. G., Boersma M., Garzke J., Löder M. G. J., Sommer U., Aberle N. (2016). Effects of high CO2 and warming on a Baltic Sea microzooplankton community. ICES J. Mar. Sci. 73, 772–782. doi: 10.1093/icesjms/fsv198
Horn H. G., Boersma M., Garzke J., Sommer U., Aberle N. (2020). High CO2 and warming affect microzooplankton food web dynamics in a Baltic Sea summer plankton community. Mar. Biol. 167, 1–17. doi: 10.1007/s00227-020-03683-0
Hurst T. P., Laurel B. J., Hanneman E., Haines S. A., Ottmar M. L. (2017). Elevated CO2 does not exacerbate nutritional stress in larvae of a Pacific flatfish. Fish.Oceanogr. 26, 336–349. doi: 10.1111/fog.12195
Jeong H. J., Kang H. J., Shim J. H., Park J. K., Kim J. S., Song J. Y., et al. (2001). Interactions among the toxic dinoflagellate Amphidinium carterae, the heterotrophic dinoflagellate Oxyrrhis marina, and the calanoid copepods Acartia spp. Mar. Ecol. Prog. Ser. 218, 77–86. doi: 10.3354/meps218077
Jeong H. J., Seong K. A., Yoo Y. D., Kim T. H., Kang N. S., Kim S., et al. (2008). Feeding and grazing impact by small marine heterotrophic dinoflagellates on heterotrophic bacteria. J. Eukaryot. Microbiol. 55, 271–288. doi: 10.1111/j.1550-7408.2008.00336.x
Jin P., Hutchins D. A., Gao K. (2020). The Impacts of ocean acidification on marine food quality and its potential food chain consequences. Front. Mar. Sci. 7. doi: 10.3389/fmars.2020.543979
Jin P., Wang T. F., Liu N. N., Dupont S., Beardall J., Boyd P. W., et al. (2015). Ocean acidification increases the accumulation of toxic phenolic compounds across trophic levels. Nat. Commun. 6, 8714. doi: 10.1038/ncomms9714
Jung M. K., Yin T. Y., Moon S. J., Park J., Yoon E. Y. (2021). Taxonomy and physiology of Oxyrrhis marina and Oxyrrhis maritima in Korean waters. Water 13, 2057. doi: 10.3390/w13152057
Lewis E., Wallace D., Allison L. J. (1998). Program developed for CO2 system calculations (ORNL/CDIAC-105(Oak Ridge Natl.Lab), 33pp. doi: 10.2172/639712
Li W., Gao K. S. (2012). A marine secondary producer respires and feeds more in a high CO2 ocean. Mar. Pollut. Bull. 64, 699–703. doi: 10.1016/j.marpolbul.2012.01.033
Li F., Shi J., Cheung S. G., Shin P. K. S., Liu X., Sun Y., et al. (2017). The combined effects of elevated pCO2 and food availability on Tigriopus japonicus Mori larval development, reproduction, and superoxide dismutase activity. Mar. Pollut. Bull. 126, 623–628. doi: 10.1016/j.marpolbul.2017.02.054
Lønborg C., Carreira C., Jickells T., Álvarez-Salgado X. A. (2020). Impacts of global change on ocean dissolved organic carbon (DOC) Cycling. Front. Mar. Sci. 7. doi: 10.3389/fmars.2020.00466
López-Abbate M. C. (2021). Microzooplankton communities in a changing ocean: a risk assessment. Diversity 13, 82. doi: 10.3390/d13020082
Majewska R., Adam A., Mohammad-Noor N., Convey P., Stefano M., Marshall D. J. (2017). Spatio-temporal variation in phytoplankton communities along a salinity and pH gradient in a tropical estuary (Brunei, Borneo, South East Asia). Trop. Ecol. 58, 251–269.
Meunier C. L., Alguero-Muniz M., Horn H. G., Lange J. A. F., Boersma M. (2017). Direct and indirect effects of near-future pCO2 levels on zooplankton dynamics. Mar. Freshw. Res. 68, 373–380. doi: 10.1071/MF15296
Michaelidis B., Ouzounis C., Paleras A., Pörtner H. O. (2005). Effects of long-term moderate hypercapnia on acid-base balance and growth rate in marine mussels Mytilus galloprovincialis. Mar. Ecol. Prog. Ser. 293, 109–118. doi: 10.3354/meps293109
Miles H., Widdicombe S., Spicer J. I., Hall-Spencer J. (2007). Effects of anthropogenic seawater acidification on acid-base balance in the sea urchin Psammechinus miliaris. Mar. Pollut. Bull. 54, 89–96. doi: 10.1016/j.marpolbul.2006.09.021
Moreno H. D., Köring M., Di Pane J., Tremblay N., Wiltshire K. H., Boersma M., et al. (2022). An integrated multiple driver mesocosm experiment reveals the effect of global change on planktonic food web structure. Commun. Biol. 5, 179. doi: 10.1038/s42003-022-03105-5
Morita M., Suwa R., Iguchi A., Nakamura M., Shimada K., Sakai K., et al. (2010). Ocean acidification reduces sperm flagellar motility in broadcast spawning reef invertebrates. Zygote 18, 103–107. doi: 10.1017/S0967199409990177
Ok J. H., Jeong H. J., You J. H., Kang H. C., Park S. A., Lim A. S., et al. (2021). Phytoplankton bloom dynamics in incubated natural seawater: predicting bloom magnitude and timing. Front. Mar. Sci. 8. doi: 10.3389/fmars.2021.681252
Osma N., Aristizabal M., Fernández-Urruzola I., Packard T. T., Gámez M. (2016). Influence of starvation on respiratory metabolism and pyridine nucleotide levels in the marine dinoflagellate Oxyrrhis marina. Protist 167, 136–147. doi: 10.1016/j.protis.2016.01.002
Pan T. C. F., Applebaum S. L., Manahan D. T. (2015). Experimental ocean acidification alters the allocation of metabolic energy. Proc. Natl. Acad. Sci. U.S.A 112, 4696–4701. doi: 10.1073/pnas.1416967112
Park K. T., Lee K., Shin K., Yang E. J., Hyun B., Kim J. M., et al. (2014). Direct Linkage between dimethyl sulfide production and microzooplankton grazing, resulting from prey composition change under high partial pressure of carbon dioxide conditions. Environ. Sci. Technol. 48, 4750–4756. doi: 10.1021/es403351h
Pedersen S. A., Håkedal O. J., Salaberria I., Tagliati A., Gustavson L. M., Jenssen B. M., et al. (2014). Multigenerational exposure to ocean acidification during food Limitation reveals consequences for copepod scope for growth and vital rates. Environ. Sci. Technol. 48, 12275–12284. doi: 10.1021/es501581j
Pedersen M. F., Hansen P. J. (2003). Effects of high pH on the growth and survival of six marine heterotrophic protists. Mar. Ecol. Prog. Ser. 260, 33–41. doi: 10.3354/meps260033
Pörtner H. O., Bock C., Reipschläger A. (2000). Modulation of the cost of pHi regulation during metabolic depression: a 31P-NMR study in invertebrate (Sipunculus nudus) isolated muscle. J. Exp. Biol. 203, 2417–2417. doi: 10.1242/JEB.203.16.2417
Roberts E. C., Wootton E. C., Davidson K., Jeong H. J., Lowe C. D., Montagnes D. J. S. (2011). Feeding in the dinoflagellate Oxyrrhis marina: linking behaviour with mechanisms. J. Plankton Res. 33, 603–614. doi: 10.1093/plankt/fbq118
Roy R. N., Roy L. N., Vogel K. M., Porter-Moore C., Pearson T., Good C. E., et al. (1993). The dissociation constants of carbonic acid in seawater at salinities 5 to 45 and temperatures 0 to 45°C. Mar. Chem. 44, 249–267. doi: 10.1016/0304-4203(93)90207-5
Runge J. A., Fields D. M., Thompson C. R. S., Shema S. D., Bjelland R. M., Durif C. M. F., et al. (2016). End of the century CO2 concentrations do not have a negative effect on vital rates of Calanus finmarchicus, an ecologically critical planktonic species in North Atlantic ecosystems. ICES J.Mar.Sci. 73, 937–950. doi: 10.1093/icesjms/fsv258
Schmoker C., Hernández-León S., Calbet A. (2013). Microzooplankton grazing in the oceans: impacts, data variability, knowledge gaps and future directions. J. Plankton Res. 35, 691–706. doi: 10.1093/plankt/fbt023
Sherr E. B., Sherr B. F. (2009). Capacity of herbivorous protists to control initiation and development of mass phytoplankton blooms. Aquat. Microb. Ecol. 57, 253–262. doi: 10.3354/ame01358
Solórzano L., Sharp J. H. (1980). Determination of total dissolved phosphorus and particulate phosphorus in natural waters. Limnol. Oceanogr. 25, 754–757. doi: 10.4319/lo.1980.25.4.0754
Spisla C., Taucher J., Bach L. T., Haunost M., Boxhammer T., King A. L., et al. (2021). Extreme levels of ocean acidification restructure the plankton community and biogeochemistry of a temperate coastal ecosystem: a Mesocosm Study. Front. Mar. Sci. 7. doi: 10.3389/fmars.2020.611157
Strom S. L., Benner R., Ziegler S., Dagg M. J. (1997). Planktonic grazers are a potentially important source of marine dissolved organic carbon. Limnol. Oceanogr. 42, 1364–1374. doi: 10.4319/lo.1997.42.6.1364
Suffrian K., Simonelli P., Nejstgaard J. C., Putzeys S., Carotenuto Y., Antia A. N. (2008). Microzooplankton grazing and phytoplankton growth in marine mesocosms with increased CO2 levels. Biogeosciences 5, 1145–1156. doi: 10.5194/bg-5-1145-2008
Thomsen J., Casties I., Pansch C., Körtzinger A., Melzner F. (2013). Food availability outweighs ocean acidification effects in juvenile Mytilus edulis: laboratory and field experiments. Glob. Change Biol. 19, 1017–1027. doi: 10.1111/gcb.12109
Vargas C. A., Lagos N. A., Lardies M. A., Duarte C., Manriquez P. H., Aguilera V. M., et al. (2017). Species-specific responses to ocean acidification should account for local adaptation and adaptive plasticity. Nat. Ecol. Evol. 1, 1–7. doi: 10.1038/s41559-017-0084
Waldbusser G. G., Salisbury J. E. (2014). Ocean acidification in the coastal zone from an organism’s perspective: multiple system parameters, frequency domains, and habitats. Annu. Rev. Mar. Sci. 6, 221–247. doi: 10.1146/annurev-marine-121211-172238
Wang Y., Fan X., Gao G., Beardall J., Inaba K., Hall-Spencer J. M., et al. (2020). Decreased motility of flagellated microalgae long-term acclimated to CO2-induced acidified waters. Nat. Clim. Change 10, 561–567. doi: 10.1038/s41558-020-0776-2
Wang T. F., Jin P., Wells M. L., Trick C. G., Gao K. S. (2019). Insensitivities of a subtropical productive coastal plankton community and trophic transfer to ocean acidification: Results from a microcosm study. Mar. Pollut. Bull. 141, 462–471. doi: 10.1016/j.marpolbul.2019.03.002
Watts P. C., Martin L. E., Kimmance S. A., Montagnes D. J. S., Lowe C. D. (2011). The distribution of Oxyrrhis marina: a global disperser or poorly characterized endemic? J. Plankton Res. 33, 579–589. doi: 10.1093/plankt/fbq148
Keywords: CO2, food availability, growth, heterotrophic dinoflagellate, ingestion, ocean acidification, Oxyrrhis marina, respiration
Citation: Wang N and Gao K (2024) Ocean acidification and food availability impacts on the metabolism and grazing in a cosmopolitan herbivorous protist Oxyrrhis marina. Front. Mar. Sci. 11:1371296. doi: 10.3389/fmars.2024.1371296
Received: 16 January 2024; Accepted: 12 February 2024;
Published: 05 March 2024.
Edited by:
Feixue Fu, University of Southern California, United StatesReviewed by:
Marco J. Cabrerizo, University of Granada, SpainCopyright © 2024 Wang and Gao. This is an open-access article distributed under the terms of the Creative Commons Attribution License (CC BY). The use, distribution or reproduction in other forums is permitted, provided the original author(s) and the copyright owner(s) are credited and that the original publication in this journal is cited, in accordance with accepted academic practice. No use, distribution or reproduction is permitted which does not comply with these terms.
*Correspondence: Kunshan Gao, a3NnYW9AeG11LmVkdS5jbg==
Disclaimer: All claims expressed in this article are solely those of the authors and do not necessarily represent those of their affiliated organizations, or those of the publisher, the editors and the reviewers. Any product that may be evaluated in this article or claim that may be made by its manufacturer is not guaranteed or endorsed by the publisher.
Research integrity at Frontiers
Learn more about the work of our research integrity team to safeguard the quality of each article we publish.