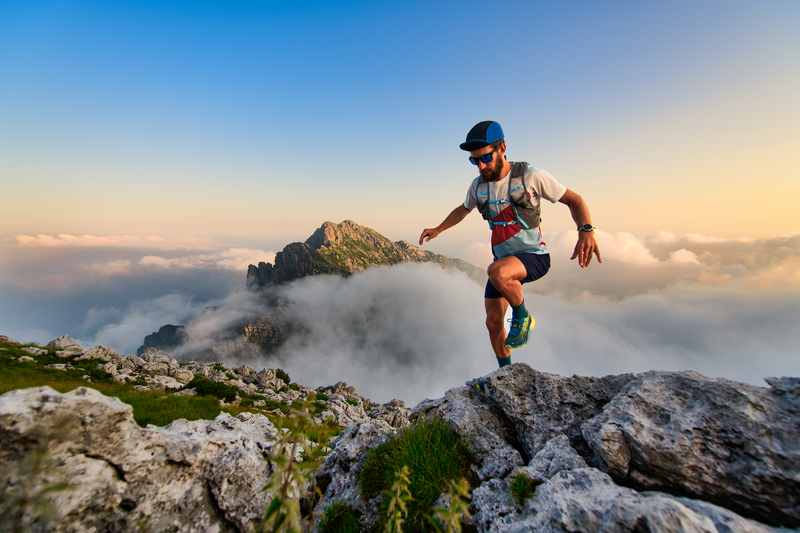
94% of researchers rate our articles as excellent or good
Learn more about the work of our research integrity team to safeguard the quality of each article we publish.
Find out more
ORIGINAL RESEARCH article
Front. Mar. Sci. , 25 March 2024
Sec. Marine Ecosystem Ecology
Volume 11 - 2024 | https://doi.org/10.3389/fmars.2024.1370768
Seagrass and macroalgae beds are key blue carbon ecosystems in the ocean. However, coastal development and climate change are sparking a growing concern about the vulnerability of sediment organic carbon (OC) to remineralization after macrophyte perturbation. Thus, the aim of this study was to assess the potential of long-term remineralization of sediment OC stocks (1 year) in coastal vegetated habitats (i.e., seagrasses Zostera noltei and Cymodocea nodosa, macroalgae Caulerpa prolifera and unvegetated sediment) after complete disturbance of macrophyte meadows under conducive conditions to microorganisms growth (i.e., oxygen saturated, non-nutrient limitation, turbulence and dark). Leached dissolved organic carbon (DOC) from particulate organic carbon (POC) remineralization, carbonate dissolution and photo-reactivity of long-term persistent DOC were also evaluated. Our results evidenced that, sediment OC from Z. noltei and unvegetated habitats were entirely remineralized to CO2. However, sediment OC from C. nodosa and C. prolifera communities exhibited a significant fraction of recalcitrant OC, and therefore, a 42 and 46% of the sediment OC still remained after 1 year of culture, respectively. POC remineralization released relevant amounts of both labile and recalcitrant DOC, which showed low photo-reactivity. Finally, we discuss that the main argument to promote management, monitoring, and restoration programs for macrophytes is usually based on their sediment OC deposit, which favor larger species. The study presented here adds arguments to also include small macrophyte species, since their sediment OC may be highly labile and entirely remineralized to CO2 once these habitats are disturbed.
Marine sediments are one of the largest and most important organic carbon (OC) reservoirs on Earth (deemed among 2,239–2,391 PgC in the top 1 m; nearly twice that of terrestrial soils; Atwood et al., 2020), which makes them cornerstone for climate regulation. Although less than 1% of the gross primary production on the planet ends up on the seafloor (Burdige, 2007; Emerson, 2013), OC buried in the sediments of the ocean can remain there for millennia if left undisturbed (Mcleod et al., 2011; Estes et al., 2019). However, enhanced human activities and climate change are onsetting growing interest about the vulnerability of the OC stocks to resuspension and remineralization once coastal habitats are disturbed, an issue that will be presumably worsen under climate change (Salinas et al., 2020; Casal-Porras et al., 2022). Among the marine environments and ecosystems, coastal areas are sites of intensive OC production, turnover, and burial, efficiently linking the terrestrial, oceanic and atmospheric carbon reservoirs (Chen and Borges, 2009). Seagrasses have been acknowledged for their carbon storage potential, which leads us to consider coastal habitat as a key elements for carbon sequestration in marine areas (i.e., blue carbon) (Röhr et al., 2016; de los Santos et al., 2023). Like seagrasses, benthic macroalgal communities have also been recently suggested as an important contributor to the carbon sequestered in marine sediments (Krause-Jensen and Duarte, 2016). Countries and states are increasingly considering their blue carbon ecosystems as nature-based allies in the fight against climate change (Hilmi et al., 2021). However, the growth of climate change mitigation approaches based on their protection and restoration, similar to those already operating on terrestrial ecosystems (e.g., REDD+ program), enjoin a full understanding of the sediment OC deposits remineralization processes once macrophyte populations are lost (Chen et al., 2022a).
The remineralization of OC is determined by recycling processes in which OC, as dead cells or metabolites, is decomposed into smaller organic compounds and further degraded to CO2 and dissolved inorganic carbon (DIC) (Emerson, 2013). OC remineralization can occur both in sediment and water column (Chen et al., 2022a). Whilst several studies have investigated the OC remineralization processes on sediments (e.g., Macreadie et al., 2017; Trevathan-Tackett et al., 2017), remineralization processes taken place in the water column have received less attention (e.g., Arıstegui et al., 2002). Probably, this is a consequence of the widely assumed fate (i.e., entirely remineralized to CO2) for the resuspended sediment OC from vegetated coastal communities, either in the area itself or in adjacent ecosystems where it can be exported and deposited (Macreadie et al., 2014). However, this assumption still largely lacks empirical support (Moksnes et al., 2021). Previous studies reported a partial decline of carbon stocks following the loss of macrophyte cover (Macreadie et al., 2014; Marbà et al., 2015; Serrano et al., 2016). However, most of them are based on carbon chronosequences in sediments (e.g., with 210Pb) once the meadows were lost, which evidences sediment erosion or resuspension rather than OC remineralization. A part of this resuspended OC can be remineralized as it becomes exposed to aerobic conditions (Macreadie et al., 2019; Salinas et al., 2020). However, the relatively low nutrient content (high C:N and C:P ratios) and the presence of complex aromatic structural biopolymers such as lignocellulose, which characterize the detritus of some vegetated coastal communities like seagrasses, may affect the capacity of the microbial community to decompose such debris (Trevathan-Tackett et al., 2017; Egea et al., 2023a). On the other hand, since the resuspension time of the sediment OC is usually limited to few hours or days (Lande and Wood, 1987), the OC remineralization in water column has been usually neglected compared to OC remineralization in sediments once the OC is deposited. However, the decomposition of sediment OC in the water column can be relevant and even higher than those in sediments in coastal areas where the current regime can produce a higher resuspension time of the sediment OC (Zhao et al., 2018). The sediment OC resuspension time in the water column in coastal systems depends on particle size and water turbulence, and it is usually limited to few days (< 30 days; Lande and Wood, 1987; Omand et al., 2020). That explains that most of the previous studies about sediment OC remineralization from marine ecosystems under oxic conditions were limited to short-term experimental periods (i.e., few weeks or months; Zhao et al., 2018; Zhang et al., 2023). However, shallow waters are systems in which interactions between sediment and water column are continuous (Fabiano et al., 2000), and where the carbon cycle is coupled closely with the water column through a succession of erosion/redeposition cycles as a consequence of higher (both, in number and intensity) turbulence events, such as storms (Pusceddu et al., 2005; Arndt et al., 2013), which may increase the resuspension time of sediments, and therefore the time OC is subjected to oxic remineralization conditions in the water column. Water column in areas dominated by vegetated coastal communities has generally high concentrations of dissolved oxygen, while these communities are often located in close proximity to the mouths of rivers, estuaries or lagoons where input of external nutrient sources often occur (Ralph et al., 2006). These conducive conditions for microorganisms growth can facilitate the decomposition of the most refractory organic carbon from sediments, a process described as ‘microbial priming’ (Kuzyakov et al., 2000). Therefore, in order to enhance our understanding about the sediment OC-remineralization processes from degraded vegetated coastal communities, the sediment OC remineralization under oxic conditions at the long-term (i.e., several months or years) in the water column needs to be assessed to both, an adequate understanding of coastal carbon cycle, and to quantify the potential of sediment OC stock remineralization in some important blue carbon habitats (i.e., the OC fraction that could be eventually oxidized to CO2 by microorganisms under favorable conditions).
During the remineralization of OC, dissolved organic materials (DOM) such as dissolved organic carbon and nitrogen (DOC and DON) are produced (Zhang et al., 2023). To date, the few recent studies analysing the OC resuspension and remineralization in vegetated coastal communities has been limited to particulate fraction (POC; Marin-Diaz et al., 2020; Barcelona et al., 2021), meanwhile the dissolved organic fraction (DOC) remains largely unexplored. The DOC released by vegetated coastal ecosystems has been highlighted in the last years as a key factor in the coastal carbon cycle. A significant fraction of this DOC is formed by bioavailable material (i.e., the labile fraction; DOCL) being an important driver of secondary production, acting as a quick transfer of carbon across the food web (Egea et al., 2019; Moran et al., 2022). Otherwise, another fraction of this DOC is apparently non-accessible to rapid microbial degradation (i.e., recalcitrant fraction; DOCR) and it can be exported and sequestered in the deep ocean, and therefore it is considered nowadays a cornerstone in the contribution of oceans to carbon sequestration (Krause-Jensen and Duarte, 2016; Jiménez-Ramos et al., 2022; Zhang et al., 2023; Egea et al., 2023b). On the other hand, seagrass and macroalgae are highly variable in terms of size, biomass, and meadow canopy complexity, which ultimately affect the properties of sediment deposits –main OM origin (autochthonous vs allochthonous), dry bulk density (DBD), OC and CaCO3 contents, etc (Mazarrasa et al., 2018). For instance, in a review of sediment OC deposits among seagrass meadows in Andalusia region –south Spain; Mateo et al. (2018) – showed that the top meter of Posidonia oceanica (Linnaeus) Delile sediment holds on average 309 tons of total OC per hectares (tTOC/ha), one order of magnitude more than the other smaller temperate seagrass species Cymodocea nodosa (Ucria) Ascherson (22 to 59 tTOC/ha) and Zostera noltei Horneman (38 tTOC/ha). In general, small species (e.g., Z. noltei) are typically ‘colonizers’, having high growth and turnover rates, which leads to lower biomass accumulation, while sediment OC deposits are typically formed by allochthonous material (Mazarrasa et al., 2021). On the other hand, larger species tend to be long-living and more persistent (e.g., P. oceanica and C. nodosa; Lavery et al., 2013; Serrano et al., 2016), while sediment OC deposits use to have a large contribution of autochthonous material (Serrano et al., 2012). Since the structure and composition of OM determine its reactivity (LaRowe et al., 2020), these differences in sediment properties may trigger important effects on OC remineralization after vegetation is lost and OC is exposed to hydrodynamics and resuspension (Egea et al., 2023a). Therefore, studies analysing the remineralization of sediment OC from benthic communities dominated by macrophytes with different complexity and/or structure are increasingly necessary to predict the role of these important ecosystem as blue carbon sinks.
The present study addresses the long-term (1-year) oxic remineralization of OM (i.e., the fraction of resuspended OC that could be oxidized or transported elsewhere) in sediments from vegetated and unvegetated coastal communities under favourable remineralization conditions, in order to determine the potential CO2 emissions from disturbed blue carbon benthic communities. In this study, 1-year was considered as long-term, since this study focuses on the oxic remineralization of sediment OC in water column. Favorable conditions for oxic remineralization of resuspended sediments are difficult to find on nature for extended periods, since sediment particles tend to sink (Lande and Wood, 1987; Omand et al., 2020) and finally get buried under layers of sediments within days or weeks (Sanford, 1992). Such long-term exposure (1-year) allowed us to quantify the potential of OC remineralization in sediments from blue carbon coastal habitats when favorable conditions are sustained over time. We expect that the remineralization curve of recalcitrant OC turned asymptotic before 1-year of exposure, evidencing the fraction of OC that is poorly bioavailable regardless of the exposure time. A set of laboratory experiments were designed to quantify (1) how much OC from macrophyte habitats is entirely remineralized as CO2 or remain as recalcitrant OC after resuspension? and (2) what is the labile/recalcitrant ratio of DOC produced by the leaching of resuspended POC? Specific responses depending on the type of benthic macrophyte communities are expected. Four different benthic communities were chosen: two monospecific communities of the temperate seagrasses Z. noltei and C. nodosa; one community dominated by the macroalgae Caulerpa prolifera (Forsskål) J. V. Lamouroux; and one unvegetated community.
Sediment samples from dense and monospecific communities of the seagrasses Zostera noltei Hornemann and Cymodocea nodosa (Ucria) Ascherson, from the rhizophyte macroalgae Caulerpa prolifera (Forsskål) J. V. Lamouroux and unvegetated areas were collected at a depth of 1–2m (high tide) in submerged meadows at Cádiz Bay (southern Spain, 36°29′19.79”N; 6°15′53.05″E). Sediment grain size in the study area is predominantly mud (size grain< 63 μm; Carrasco et al., 2003; de los Santos et al., 2023). Fifty small cores (60 mL-plastic syringes with their bases cut off; separated by at least 50 cm) were taken at random within each community. The cores were pushed into the sediment to a depth of 10 cm; that correspond to the sediment fraction accumulated during the last 40–50 years (depending on the meadow), as indicated by 210Pb sediment dating results obtained in de los Santos et al. (2023) for the study area. Sediment samples from each community were transferred to large plastic bags to set up four sediment pools (i.e., one for each community studied) and transported to the laboratory within 1 h of collection. Macrophytes samples of each community (n=3) were also collected using areas of 20 x 20 cm to describe meadow characteristics at the laboratory (i.e., aboveground (AG–) and belowground (BG–) macrophyte biomass, and shoot or frond densities). Each sediment pool from each community was mixed, homogenized and sieved through 8 mm (coarse leaf litter) and 1 mm (fine leaf litter) to remove fauna, living macrophyte tissues and litter (mostly, leaf litter). Macrophyte samples were processed by counting the number of shoots and, then, splitting into aboveground and belowground biomasses in the case of C. nodosa. Similarly, the number of fronds was counted and then divided into fronds and rhizoids biomasses in the case of C. prolifera. Biomass samples were rinsing and drying at 60 °C to estimate the dry weight biomass (gDW m-2).
The experiment was run in a temperature-controlled indoor mesocosm system at the Faculty of Marine and Environmental Sciences of the University of Cadiz set at 20°C and darkness conditions. A total of 108-replicates borosilicate glass bottles (600 mL) were used as incubators (27 replicates per community) filled with sediment samples (60 cm3) and autoclaved artificial seawater (440 mL; ~35 g Tropic Marin® Sea Salt Classic · L-1 distilled water) with a similar salinity to measure in situ areas (33‰ psu). The sea salt used (Tropic Marin, St. Niklausen Switzerland) contained all the main and trace elements of seawater in a natural ratio. All incubators were covered with clean aluminium foil to prevent air contamination. Long-term OC remineralization process was evaluated under conducive conditions to microorganisms growth, including oxygen conditions and non-water stagnation (through gently aeration using airstones) and, when it was necessary, adjusting nutrients (N, P and C) during 365 days (Figure 1). In this way, the potential of remineralization of OC stocks at the long-term (i.e., the OC fraction that could be eventually oxidized to CO2 by microorganisms under favourable conditions after 365 days) was assessed. The total seawater volume was adjusted by adding artificial seawater or Milli-Q as needed to keep salinity. Ammonium (NH4Cl) and phosphate (NaH2PO4) concentrations were maintained to ~3 and ~0.7 µM respectively (Supplementary Table 2 in the Supplementary Material) (the minimum concentration in experimental area; Egea et al., 2020), to avoid bacterial growth limitation by either nitrogen or phosphorus availability. Three incubators (replicates) from each sediment type were randomly retrieved for POC and DOC measurements (see below), at 8 different sampling times on days 0, 5, 10, 15, 30, 60, 180 and 365. Bacterial abundance was monitored in three replicates per community on days 0, 30, 60, 180 and 365 by collecting samples of water (1.5 mL). Samples were fixed immediately with cold 10% glutaraldehyde (final concentration, 1%), left in the dark for 10 min at room temperature and then, stored at −80 °C. Bacterial abundance was counted with a FacsAriaII (Cell Sorter) flow cytometer as described previously in Gasol and Del Giorgio (2000).
Figure 1 Simplified diagram of experimental procedures and design for (A) the sediment samples collection and (B) the long-term sediment organic carbon remineralization. See detailed description in the text.
In order to confirm the bioavailability of released DOC from sediment OC, an extra assay of DOC bioavailability was also carried out after 30 days of incubation using new microbial inoculum from the field. The bioavailability assay was done at day 30th, since it represents the maximum sediment OC resuspension time in the water column reported in previous works (Lande and Wood, 1987). In this assay, the only OC available for bacteria was DOC, since sediment OC was removed. Twelve incubations (i.e., hermetic glass bottles; four communities and three replicates per community) were filled filtering the hermetic glass bottles according to Jiménez-Ramos et al. (2022). For this purpose, 270 mL of water from each incubation were collected using 50-ml acid-washed syringe and filtered through a 0.2 µm polycarbonate filter and added to each hermetic glass bottle. Then, newly microbial inoculum from field was added, by collecting 30 mL of water and filtering through a 0.8 µm polycarbonate filter to eliminate bacterium predators, such as small flagellates. Filtered water with the natural bacterial community was also added to the hermetic glass bottle to complete the culture medium (300 mL; ratio 9:1). In this way, the DOC bioavailability was evaluated using the DOC released from sediment OC degradation as the only C source for bacteria (i.e., without POC from sediments in the incubations). Hermetic glass bottles were maintained during 14 days in dark, under oxygen saturation and temperature-controlled conditions (Jiménez-Ramos et al., 2022). DOC samples were collected in each treatment at different sampling times, on days 0, 1, 2, 3, 6, 10 and 14. In this study, the term bioavailable/labile fraction of DOC (DOCL) refers to the fraction of DOC that is used by heterotrophic bacteria during the incubation time (i.e., 14 days). Likewise, we used the term recalcitrant fraction of DOC (DOCR) to refers to the remaining DOC fraction.
After 365 days, the remaining incubators from the experiment were used to evaluate the photo-reactivity of released–DOC. All water inside remaining incubations (n = 3 for each sediment community) were filtered into acid-washed glass vials through a 0.2 µm polycarbonate filter to eliminate POC and bacterial community and then, they were subjected to sunlight conditions (ca. 50 mol photon m-2 d-1) in order to test the UV–degradation effect in DOC released (photo-reactivity of DOC) in an outdoor experimental area in the Faculty of Marine and Environmental Sciences during 48h. This time span was enough to evaluate the magnitude of photo-reactivity of DOC, since previous studies indicated that most of DOC photodegradation occurs rapidly in few hours (Zhang et al., 2009; Li et al., 2020). After this sunlight period, water samples were kept with 0.08 ml of H3PO4 (diluted 30%) at 4 °C (glass vials encapsulated with silicone-PTFE caps) until DOC analysis.
The sediment organic carbon (OC) and the released dissolved organic carbon (DOC) in each sediment type in the long-term OC remineralization culture and both in the bioavailability and photo-reactivity of released–DOC assays were measured by collecting three incubations (i.e., replicates) per sediment type and sampling days. For each sample, the sediment OC content and sediment properties for each incubation were measured by collecting all the sediment inside the incubation (~60 cm3) and drying at 60°C until constant weight. Dry bulk density (DBD) was calculated as the dry weight of the sediment divided by the volume of the original sediment sample. Subsequently, sediment samples were ground to fine powder and homogenized and subdivided into two subsamples for organic carbon content (A–subsample) and inorganic carbon content (B–subsample). The measurement of carbon content in the sediment samples was performed at the Institute of Marine Research (INMAR) at University of Cádiz (Spain), following the procedures described by Howard et al. (2014). Total carbon (and nitrogen) content (%) were measured in ca. 0.15 g dry weight of A–subsample through an automated elemental analyzer (LECO CNS928). Inorganic carbon content (%) was determined in ca. 2 g of dry weight from B–subsamples using the acidification method with HCL 1N (Howard et al., 2014). Then, the sediment organic carbon content (OC, %) was calculated as the difference between the total and inorganic carbon (Howard et al., 2014).
DOC samples were taken from each treatment by filling glass vials (20 mL) through a 50 ml acid-washed syringe using pre-combusted (500 °C for 4 h) Whatman GF/F filters. Water samples were kept with 0.08 ml of H3PO4 (diluted 30%) at 4 °C in acid-washed material (glass vials encapsulated with silicone-PTFE caps) until subsequently DOC analysis. The DOC concentration in DOC samples (mg C L-1) were derived by catalytic oxidation at high temperature (680°C) and coordination with NDIR by using a Shimadzu TOC-L analyser (detection limit of 4 μg DOC L-1). DOC-certified reference material (Low and Deep), provided by D. A. Hansell and W. Chen (DSR: 44–45 of μM for DOC, University of Miami), were used to assess the accuracy of the estimations. The results were in good agreement with certified DSR values (deviation:<5%). Since all sediments samples (~60 cm3) were diluted in seawater to fill the experimental units (~500 ml) in the experimental set-up, the DOC released by sediments (mg · L sediment-1) were obtained from DOC samples concentration in those incubations (mg · L incubation-1) and corrected over the effect of sediment dilution through the following Equation (1):
where L Sed-1 is the volume of sediment (L), DOCInc and VolIncare the DOC concentration and volume of the incubations and VolSedis the volume of the sediment sample.
Data are presented as mean ± SE. Differences among communities on each response variable were tested using generalized linear models (GLMs). For each response variable, we selected a particular family error structure and link function to reach the assumptions of linearity, homogeneity of variances, and no overdispersion, which were checked by visual inspection of residuals and Q-Q plots (Harrison et al., 2018) after modelling. Levene’s test for equality of variances was used to verify this assumption. Aboveground–, belowground– and total–biomass, AG : BG ratio, DBD, OC stocks, N content, OC : IC ratio and DOC concentrations were modelled using Gaussian distribution with identity link. Shoot or frond density and IC contents were modelled using Gamma distribution with inverse link. We performed post-hoc comparisons among communities using estimated marginal means with a Bonferroni correction (“emmeans” R package, Lenth et al., 2022). Assumptions of normality and homoscedasticity were assessed by examining the residuals of all linear models. Statistical analyses were computed using R 4.2.2 statistical software (R Development Core Team, 2022).
Zostera notei and Cymodocea nodosa showed similar aboveground biomass and total biomass, significantly higher than their Caulerpa prolifera counterpart. All vegetated communities showed significant differences in belowground biomass, with a trend of C. nodosa > Z. noltei > C. prolifera. The density of Z. noltei was, on average, x6.9-fold higher than the mean of the other two macrophytes (Table 1 and Supplementary Table 1 in the Supplementary Material). The initial average of both sediment organic and inorganic carbon stocks (OC and IC; %DW) in the upper sediment surface (i.e., top 10 cm) showed significant differences among communities. C. nodosa was the community with the highest OC stock, followed by C. prolifera, both significantly higher than Z. noltei and unvegetated sediments counterparts. C. nodosa and C. prolifera exhibited similar IC stocks, ca. x1.9-fold higher than those found, on average, in Z. noltei and unvegetated sediments. As a result, the initial ratio of organic vs inorganic carbon (OC : IC) was<1 in unvegetated sediment and Z. noltei, meanwhile it was >1 for C. nodosa and C. prolifera (Table 1 and Supplementary Table 1 in the Supplementary Material).
During the experiment, sediment OC stocks experienced a sharp decline from the onset until the first 30 days for vegetated communities, especially in Z. noltei sediments, which a reduction of ca. 27% with respect to the initial OC stock (Figure 2A). The same tendency was recorded for OC stocks in sediments from C. nodosa and C. prolifera communities, although these reductions were milder, reaching reductions of ca. 22% and 10% with respect to the initial OC stocks for C. nodosa and C. prolifera respectively. These reductions concurred with a significant increase in the bacterial abundance in all the communities (Supplementary Figure 1 in the Supplementary Material). This initial phase of sharp decrease in the OC concentration of vegetated communities was followed by a slower degradation phase until the end of the experimental period for C. nodosa and C. prolifera communities (Figure 2A). Both, unvegetated sediment and Z. noltei community evidenced a complete OC remineralization after 365 days (i.e., 100% of their initial OC stocks). By contrast, C. nodosa and C. prolifera treatments exhibited a partial OC remineralization during that period (ca. 58% and 54% of their initial OC stocks remained, respectively) (Figure 2A). Regarding IC deposits, vegetated communities showed a sharp decline during the first 30 days reaching reductions of ca. 30%, 29% and 36% with respect to the initial IC stock for C. prolifera, C. nodosa and Z. noltei, respectively (Figure 2B). This initial phase of sharp decrease in the IC concentration of vegetated communities was followed by a final phase of stagnation until the end of experimental period, where the decrease in IC was neglected.
Figure 2 Reduction of (A) sediment organic carbon (OC) and (B) sediment inorganic carbon (IC) stocks during the experimental period (365 days). Data are expressed as remaining percentages with respect to initial concentrations ± SE (n =3). Note difference in Y-axis scale.
The four communities exhibited a sharp increase in the dissolved organic carbon (DOC) concentrations during the first 60 days, from, on average, 19.0 ± 2.5 mg DOC · L Sed-1 (t = 0) to 58.1 ± 4.1 (t = 60; i.e., x3.1-fold higher). After that, the DOC concentration fell up, on average, to 32.0 ± 2.9 mg DOC · L Sed-1 (i.e., x1.7-fold respect t = 0) after 1 year of experimental period (Figure 3). Although the four communities followed similar trends throughout the experimental period, significant differences were found among them. Sediments from vegetated communities showed a large DOC release compared with unvegetated ones, with a trend of C. prolifera>C. nodosa>Z. noltei. Two peaks of DOC concentration were found in sediments from vegetated bottoms, meanwhile only one peak was recorded in unvegetated sediment through the experimental period. The first peak was at t = 5 days (peaking at 29.9 ± 1.5, 35.2 ± 4.8 and 34.8 ± 4.4 mg DOC · L Sed-1 for C. prolifera, C. nodosa and Z. noltei communities, respectively; Figure 3), and the second one at t = 60 days (peaking at 72.5 ± 0.8, 65.2 ± 2.2, 57.3 ± 3.2 and 37.3 ± 1.5 mg DOC · L Sed-1 for C. prolifera, C. nodosa, Z. noltei communities and unvegetated sediment, respectively). It is noteworthy the high DOC concentration in the Z. noltei treatment at t = 0 days with respect to the others communities (31.5 ± 5 mg DOC · L Sed-1, ~2.3-fold higher than unvegetated sediments). After the first 60 days, the sediments evidenced a progressive decrease in DOC concentration until reaching values around 23.6 ± 1.7 mg DOC · L Sed-1 for unvegetated and Z. noltei sediments, and 40.4 ± 2.5 mg DOC · L Sed-1 (i.e., 1.7-fold higher) in C. nodosa and C. prolifera sediments at the end of the experimental period.
Figure 3 (A) DOC concentration produced by the degradation of sediment OC in vegetated and unvegetated sediments (mean ± SE) during the experimental period (365 days). To facilitate the visualization, the first part of the graph was enlarged in (B).
Once experimental period ended (365 days) and samples were exposed to 48h of sunlight to assess the photodegradation of remaining DOC, the concentration of DOC fell up, on average, to 29.9 ± 2.7 mg DOC · L Sed-1, which represent a slight decrease of 6.6% with respect to DOC concentration measured at the end of the experimental period (Table 2). DOC from C. nodosa showed the lowest photodegradation (~0.3-fold than the average of the other sediments), followed by C. prolifera, Z. noltei communities and unvegetated sediments (Table 2).
Table 2 Average of DOC concentrations, both after bacterial degradation (365 days) and photodegradation phases (mg DOC · L sediment-1).
The bioavailability of released-DOC assay showed a sharp decline in DOC concentration during the first 6–10 days, depending on the community, followed by a final phase of stagnation in most of the treatments. At the end of the assay, the 74%, 66%, 65% and 64% of initial DOC remained in samples from Z. noltei, C. prolifera, C. nodosa and unvegetated sediments, respectively (Figure 4). All communities showed DOCR : DOCL ratios higher than 1, with a trend of Z. noltei>C. prolifera>C. nodosa>Unvegetated (2.78>1.97>1.85>1.79, respectively).
Figure 4 Bioavailability assay using DOC pool coming from experimental units at day 30. Data are expressed as remaining percentages with respect to initial concentrations ± SE (n =3).
The sediment organic carbon (OC) stock found in upper sediments was in the same range than those reported for Cádiz Bay habitats by de los Santos et al. (2023), and similar to those reported by Bañolas et al. (2020) for seagrasses and by Holmer et al. (2004) for the macroalgae. This high sediment OC stock is explained by the high macrophyte density and fine grain sizes, which feature the donor sampling area (de los Santos et al., 2023; Egea et al., 2023b). A significant decrease in sediment OC content was recorded in all the communities during the 365 days experimental period. However, long-term OC remineralization results evidenced a considerable variability depending on the macrophyte species. A relevant proportion of initial OC stock remained in C. nodosa (ca. 42%), which is a relatively larger species compared with Z. noltei, which showed a full OC remineralization, similar to those found in unvegetated sediments. In general, small species are typically ‘colonizers’, with higher growth and turnover rates, which leads to lower biomass accumulation and OC deposits than larger and typically ‘long-lived and persistent’ species (Mateo et al., 2018; Mazarrasa et al., 2018). Our results evidence that different seagrasses hold substantial differences in OC remineralization rates. The high OC stock and relatively low OC remineralization rate recorded in C. nodosa can be explained by the presence of complex aromatic structural biopolymers such as lignocellulose, which characterizes the detritus of seagrasses and affect the capacities of the microbial community to decompose those debris (Trevathan-Tackett et al., 2017; Egea et al., 2023a). However, similar biopolymers are present in tissues of Z. noltei and hence, a similar trend would be expected. The high rate of OC remineralization in Z. noltei can be explained by the high contribution of microphytobenthos (highly labile) and allochthonous seston POM that can be found in the sediment of this seagrass species (up to ca. 30% and 45%, respectively; Santos et al., 2019). The low contributions of Z. noltei as autochthonous sources of sedimentary organic matter can be explained by the low belowground biomass and from the high turnover and exportation of leaves. The leaves of this species are quite light, with a leaf mass area of 34.4 ± 7.4 g DW m−2, much lower than seagrass mean values (55.8 ± 25.7 g DW m−2; de los Santos et al., 2016). These characteristics give the leaves a high buoyancy, making them float away with the tidal flow (Jiménez-Ramos et al., 2023). On the other hand, C. prolifera evidenced a relatively low OC remineralization rate (ca. 46% of initial OC remained at the end of the experiment), which contrast with the labile features of tissues from this macroalgae species (Caulerpales does not contain cellulose and shows low C:N ratio; Kloareg and Quatrano, 1988). However, the high capacity of C. prolifera beds to sequester and store OC relies in its high efficiency in trapping fine suspended particles, in this meadow mainly from the large nearby seagrass meadows (de los Santos et al., 2023). This trapping ability is attributed to the very dense canopies that form this species and to its ability to root, which translates into a very complex frond structure and subterranean network of stolons and rhizoids (Vergara et al., 2012).
Our results also evidence a relatively faster degradation of OC during the first 30 days of the experimental period (27%, 22%, 10% and 15% respect the initial OC stock for Z. noltei, C. nodosa, C. prolifera, and unvegetated sediments, respectively). The sediment OC resuspension time in the water column of coastal areas depends on particle biomass and water turbulence, but it is usually limited to few days (< 30 days; Lande and Wood, 1987). Therefore, our results show that a significant amount of sediment OC can be remineralized in the water column when vegetated coastal ecosystems are degraded or lost. This released organic matter may enhance heterotrophic microbial production and serve as an OC supplement for some autotrophic phytoplankton (e.g., cyanobacteria and chlorophyte), which ultimately can boost zooplankton growth (Cavan et al., 2017; Steinberg and Landry, 2017). Zooplankton plays a central role in marine ecosystems as trophic link between primary producers and higher trophic levels (Kruse et al., 2010). Hence, changes in zooplankton abundance and composition derived of OC remineralization in the water column may trigger important ecological implications to food webs and to the ocean carbon cycle. Regarding carbonate (CaCO3) stocks, they fell in the lower part of the range previously recorded in these macrophytes (0.3−174 mg CaCO3 cm−3, Mazarrasa et al., 2015), which was expectable since latitude, and because the seagrass species assessed here usually exhibit lower CaCO3 content than larger species (e.g., Posidonia spp. and Thalassia spp.) (Mazarrasa et al., 2015). The higher concentration in CaCO3 observed in C. prolifera may be caused by anoxia and the presence of toxic sulfides in their sediments that benefit carbonate precipitation (Holmer et al., 2009).
Although the methodology used here was similar to previous studies on sediment OC and DOC degradation (e.g., Zhao et al., 2018; Jiménez-Ramos et al., 2022; Chen et al., 2022a), it also has some limitations. The growth and structure of the bacterial community may be altered when confined during a long-term incubation period, since the systems is completely isolated –there is no continuous supply of bacteria or nutrient inputs as occur in nature (Baltar et al., 2012). To reduce this effect, we periodically supplied incubations with nutrients (see materials and methods). Moreover, we bubbled incubations preventing water stagnation in a process similar to rolling tanks used for long-term studies of POM-bacteria interactions (Ionescu et al., 2015). Continuous aeration might have caused the input of volatile organic compounds and air-borne microorganisms inside incubations, but allowed the most favorable conditions for OC degradation and, therefore, to estimate at the long-term the potential of remineralization. In spite of that, previous controls with and without aeration showed that these possible inputs were negligible. In addition, bioavailability of DOC exported by vegetated communities was also evaluated with newly microbial inoculum from field. For these reasons, the possible artifacts introduced by our experimental setup are considered negligible and affecting in the same manner to all the treatments, and therefore it would hardly modify the trends found in the long-term experiment. Regarding to the photodegradation assay, it is important to note that borosilicate glass bottles typically have a sharp cut-off at 320 nm (e.g., it can absorb ≈ 95 % of UVB, ≈37 % of UVA radiation and ≈10 % of PAR; Reche et al., 1999), that can affect to the UV levels in our experimental assay. However, the experimental bottles were also subjected to significantly higher sunlight doses than use to occur in nature (i.e., no turbidity, reduced water layer in our experimental design), and therefore it can balance the reduction in UV caused by the borosilicate bottles. However, since we didn´t measure the levels of UV reaching the culture medium, our results about photo-reactivity should be considered with caution, although observed trends among communities are presumably reliable.
Our results showed that the sediment resuspension and subsequently OC remineralization resulted in an important source of DOC to coastal systems. DOC concentration increased substantially in all treatments until day 60th, probably as a consequence of OC degradation and leaching as DOC, as recorded in previous studies (Komada et al., 2013; Egea et al., 2023a). After this initial increase, DOC concentration showed a marked decrease, denoting that a high fraction of leached DOC is labile (DOCL), and mainly during the first weeks. This has important ecological implications as DOCL constitutes an important source of carbon for heterotrophic bacteria (Lønborg et al., 2018). This supply of carbon can boost the trophic web (Egea et al., 2019; Moran et al., 2022) and may promote the production of recalcitrant DOC (DOCR) as bacteria’s metabolic by-product (Jiao et al., 2014; Koch et al., 2014; Chen et al., 2020). A considerable amount of DOC remained in the C. prolifera and C. nodosa incubations after 365 days under favorable conditions for remineralization, which were significantly higher than values measured in Z. noltei and unvegetated sediment (Table 2). This low bio-reactive fraction of DOC can be considered as DOCR (Hansell, 2013; Jiao et al., 2014), and is probably formed by complex humic-like components, which are harder to be consumed by microbes as carbon source (Dittmar, 2015; Jiménez-Ramos et al., 2022). The time course assay conducted with the leached DOC after 30 days of incubation using new microbial inoculum from the field, supported that DOC leached from OC remineralization produces a considerable amount of DOCR (Figure 4). In this assay, the only OC available for bacteria was DOC, since OC was removed. In this short-time assay, DOC concentration showed a rapid decrease in the first 2–3 days followed by a milder decrease until a stationary phase was reached at 6th day for C. prolifera and 10th day for C. nodosa and unvegetated sediments. Although at the end of the assay, the proportion of DOC was higher in Z. noltei, the leached DOC from this community did not reach the stationary phase, denoting a high proportion of DOCL and a relatively lower efficiency in DOC consumption by microorganisms.
Besides the remineralization of DOC by microorganisms, some DOC components typically show photo-reactivity when they are exposed to sunlight irradiation. Photochemical oxidation of DOC induced by sunlight can reduce some DOC to CO2 and carbon monoxide (Cory et al., 2014), which may explain the slight decrease in DOC concentrations found here after samples were exposed during 48h to sunlight (Table 2) with C. nodosa showing the lowest photochemical oxidation of released DOC. Although the photodegradation assay was limited to 48 hours, we considered this time span enough to evaluate the variability on photo-reactivity of DOC among communities, since previous studies indicated that most of DOC photodegradation occurs rapidly in few hours (Zhang et al., 2009; Li et al., 2020). According to previous works (Bertilsson et al., 2009; Chen and Jaffé, 2014), our results suggest that the photodegradation of DOC from coastal vegetated sediments is relatively low. Some previous studies indicated that UV-irradiated DOCR can be more bioavailable for heterotrophs (Chen et al., 2022b), which has not been tested here. However, the role of photodegradation of marine macrophytes-derived DOC is still unclear, since other studies found that photodegradation in algae-derived DOC did not stimulate bacterial growth (e.g., Pausz and Herndl, 1999; Bertilsson et al., 2004). This is still an unresolved question in the research of DOC remineralization that needs to be further assessed in future studies.
Seagrass and macroalgae meadows are one of the main blue carbon ecosystems, accumulating huge amounts of OC in their sediments or in adjacent soft bottom systems (Duarte et al., 2005). Their inclusion in the voluntary carbon markets has opened the door to develop CO2 offset projects through the restoration of these ecosystems (Needelman et al., 2018; Junta de Andalucía, 2021). However, the differences in OC stocks among macrophytes, makes that most management, monitoring and restoration programs focus on larger species such as Posidonia oceanica (Kilminster et al., 2015; IUCN, 2021), which typically exhibit higher OC deposits (Mazarrasa et al., 2018). The study presented here adds arguments to also include smaller macrophyte species. Although their OC deposits may be relatively minor than larger species, our results showed that they can be entirely remineralized, releasing this sediment OC as CO2 or other greenhouse gases to the environment. By contrast, a high fraction of sediment OC from larger macrophytes species can remain in the habitat itself, in the water column or in adjacent ecosystems where this OC may be deposited, once the original habitat is perturbed.
In summary, we present the first empirical experiment that confirm that the sediment OC from vegetated coastal communities can be entirely remineralized to CO2 if conditions are favorable for microorganism metabolism during long-term, although the magnitude has a species-specific dependence. A significant fraction of sediment OC from vegetated coastal communities can be recalcitrant, especially in larger macrophytes. Therefore, the magnitude of OC remineralization depends on size and macrophyte species. Our results showed that the sediment resuspension and subsequently OC remineralization also resulted in an important source of both labile and recalcitrant DOC to coastal systems.
The original contributions presented in the study are included in the article/Supplementary Material. Further inquiries can be directed to the corresponding author.
AY-M: Data curation, Formal analysis, Investigation, Methodology, Writing – original draft, Writing – review & editing. RJ-R: Conceptualization, Data curation, Formal analysis, Funding acquisition, Investigation, Methodology, Project administration, Supervision, Writing – original draft, Writing – review & editing. IC-P: Investigation, Writing – review & editing. FB: Methodology, Supervision, Writing – review & editing. LE: Conceptualization, Data curation, Formal analysis, Funding acquisition, Investigation, Methodology, Project administration, Supervision, Writing – review & editing.
The author(s) declare financial support was received for the research, authorship, and/or publication of this article. This study was supported by the Spanish National Project RECOUNT (PID2020-120237RJ-I00), financed by MCIN/AEI/10.13039/501100011033; the SER-CADY project (FEDERUCA18-107451) supported by the, 2014–2020 ERDF Operational Programme and by the Department of Economy, Knowledge, Business and University of the Regional Government of Andalusia. AY-M was supported by a FPU fellowship from the Spanish Ministry of Universities. The open access fee was co-funded by the Plan Propio – UCA, 2023-2024 and by the QUALIFICA Project (QUAL21-0019, Junta de Andalucía).
Thanks to the Integration and Application Network for courtesy in supplying vector symbols (ian.umces.edu/symbols/).
The authors declare that the research was conducted in the absence of any commercial or financial relationships that could be construed as a potential conflict of interest.
All claims expressed in this article are solely those of the authors and do not necessarily represent those of their affiliated organizations, or those of the publisher, the editors and the reviewers. Any product that may be evaluated in this article, or claim that may be made by its manufacturer, is not guaranteed or endorsed by the publisher.
The Supplementary Material for this article can be found online at: https://www.frontiersin.org/articles/10.3389/fmars.2024.1370768/full#supplementary-material
Arıstegui J., Denis M., Almunia J., Montero M. F. (2002). Water-column remineralization in the Indian sector of the Southern Ocean during early spring. Deep Sea Res. Part II Top. Stud. Oceanogr. 49, 1707–1720. doi: 10.1016/S0967-0645(02)00008-5
Arndt S., Jørgensen B. B., LaRowe D. E., Middelburg J. J., Pancost R. D., Regnier P. (2013). Quantifying the degradation of organic matter in marine sediments: A review and synthesis. Earth-Science Rev. 123, 53–86. doi: 10.1016/j.earscirev.2013.02.008
Atwood T. B., Witt A., Mayorga J., Hammill E., Sala E. (2020). Global patterns in marine sediment carbon stocks. Front. Mar. Sci. 7. doi: 10.3389/fmars.2020.00165
Baltar F., Lindh M. V., Parparov A., Berman T., Pinhassi J. (2012). Prokaryotic community structure and respiration during long-term incubations. Microbiologyopen 1, 214–224. doi: 10.1002/mbo3.25
Bañolas G., Fernández S., Espino F., Haroun R., Tuya F. (2020). Evaluation of carbon sinks by the seagrass Cymodocea nodosa at an oceanic island: Spatial variation and economic valuation. Ocean Coast. Manage. 187, 105112. doi: 10.1016/j.ocecoaman.2020.105112
Barcelona A., Oldham C., Colomer J., Garcia-Orellana J., Serra T. (2021). Particle capture by seagrass canopies under an oscillatory flow. Coast. Eng. 169, 103972. doi: 10.1016/j.coastaleng.2021.103972
Bertilsson S., Tranvik L. J. (2000). Photochemical transformation of dissolved organic matter in lakes. Limnol. Oceanogr. 45, 753–762. doi: 10.4319/lo.2000.45.4.0753
Bertilsson S., Carlsson P., Granéli W. (2004). Influence of solar radiation on the availability of dissolved organic matter to bacteria in the Southern Ocean. Deep Sea Res. Part II Top. Stud. Oceanogr. 51, 2557–2568. doi: 10.1016/j.dsr2.2000.07.001
Burdige D. J. (2007). Preservation of organic matter in marine sediments: Controls, mechanisms, and an imbalance in sediment organic carbon budgets? Chem. Rev. 107, 467–485. doi: 10.1021/cr050347q
Carrasco M., López-Ramırez J., Benavente J., López-Aguayo F., Sales D. (2003). Assessment of urban and industrial contamination levels in the bay of Cádiz, SW Spain. Mar. Pollut. Bull. 46, 335–345. doi: 10.1016/S0025-326X(02)00420-4
Casal-Porras I., de los Santos C. B., Martins M., Santos R., Pérez-Lloréns J. L., Brun F. G. (2022). Sedimentary organic carbon and nitrogen stocks of intertidal seagrass meadows in a dynamic and impacted wetland: Effects of coastal infrastructure constructions and meadow establishment time. J. Environ. Manage. 322, 115841. doi: 10.1016/j.jenvman.2022.115841
Cavan E. L., Henson S. A., Belcher A., Sanders R. (2017). Role of zooplankton in determining the efficiency of the biological carbon pump. Biogeosciences 14, 177–186. doi: 10.5194/bg-14-177-2017
Chen C.-T. A., Borges A. V. (2009). Reconciling opposing views on carbon cycling in the coastal ocean: Continental shelves as sinks and near-shore ecosystems as sources of atmospheric CO2. Deep Sea Res. Part II Top. Stud. Oceanogr. 56, 578–590. doi: 10.1016/j.dsr2.2009.01.001
Chen M., Jaffé R. (2014). Photo- and bio-reactivity patterns of dissolved organic matter from biomass and soil leachates and surface waters in a subtropical wetland. Water Res. 61, 181–190. doi: 10.1016/j.watres.2014.03.075
Chen J., Li H., Zhang Z., He C., Shi Q., Jiao N., et al. (2020). DOC dynamics and bacterial community succession during long-term degradation of Ulva prolifera and their implications for the legacy effect of green tides on refractory DOC pool in seawater. Water Res. 185, 116268. doi: 10.1016/j.watres.2020.116268
Chen Z., Nie T., Zhao X., Li J., Yang B., Cui D., et al. (2022a). Organic carbon remineralization rate in global marine sediments: A review. Reg. Stud. Mar. Sci. 49, 102112. doi: 10.1016/j.rsma.2021.102112
Chen Y., Sun K., Sun H., Yang Y., Li Y., Gao B., et al. (2022b). Photodegradation of pyrogenic dissolved organic matter increases bioavailability: Novel insight into bioalteration, microbial community succession, and C and N dynamics. Chem. Geol. 605, 120964. doi: 10.1016/j.chemgeo.2022.120964
Cory R. M., Ward C. P., Crump B. C., Kling G. W. (2014). Sunlight controls water column processing of carbon in arctic fresh waters. Science 345, 925–928. doi: 10.1126/science.1253119
de los Santos C. B., Egea L. G., Martins M., Santos R., Masqué P., Peralta G., et al. (2023). Sedimentary organic carbon and nitrogen sequestration across a vertical gradient on a temperate wetland seascape including salt marshes, seagrass meadows and rhizophytic macroalgae beds. Ecosystems 26, 826–842. doi: 10.1007/s10021-022-00801-5
de los Santos C., Onoda Y., Vergara J., Pérez-Lloréns J., Bouma T., La Nafie Y., et al. (2016). A comprehensive analysis of mechanical and morphological traits in temperate and tropical seagrass species. Mar. Ecol. Prog. Ser. 551, 81–94. doi: 10.3354/meps11717
Dittmar T. (2015). “Reasons behind the long-term stability of dissolved organic matter,” in Biogeochemistry of Marine Dissolved Organic Matter. Eds. Hansell D. A., Carlson C. A. (Elsevier Inc), 369–388. doi: 10.1016/B978-0-12-405940-5.00007-8
Duarte C. M., Middelburg J. J., Caraco N. (2005). Major role of marine vegetation on the oceanic carbon cycle. Biogeosciences 2, 1–8. doi: 10.5194/bg-2-1-2005
Egea L. G., Barrón C., Jiménez–Ramos R., Hernández I., Vergara J. J., Pérez–Lloréns J. L., et al. (2019). Coupling carbon metabolism and dissolved organic carbon fluxes in benthic and pelagic coastal communities. Estuar. Coast. Shelf Sci. 227, 106336. doi: 10.1016/j.ecss.2019.106336
Egea L. G., Infantes E., Jiménez-Ramos R. (2023a). Loss of POC and DOC on seagrass sediments by hydrodynamics. Sci. Total Environ. 901, 165976. doi: 10.1016/j.scitotenv.2023.165976
Egea L. G., Jiménez-Ramos R., Hernández I., Brun F. G. (2020). Differential effects of nutrient enrichment on carbon metabolism and dissolved organic carbon (DOC) fluxes in macrophytic benthic communities. Mar. Environ. Res. 162, 105179. doi: 10.1016/j.marenvres.2020.105179
Egea L. G., Jiménez-Ramos R., Romera-Castillo C., Casal-Porras I., Bonet-Melià P., Yamuza-Magdaleno A., et al. (2023b). Effect of marine heat waves on carbon metabolism, optical characterization, and bioavailability of dissolved organic carbon in coastal vegetated communities. Limnol. Oceanogr. 68, 467–482. doi: 10.1002/lno.12286
Emerson S. (2013). “Organic carbon preservation in marine sediments,” in The Carbon Cycle and Atmospheric CO2: Natural Variations Archean to Present. Eds. Sundquist E. T., Broecker W. S. (American Geophysical Union, Washington D. C), 78–87.
Estes E. R., Pockalny R., D’Hondt S., Inagaki F., Morono Y., Murray R. W., et al. (2019). Persistent organic matter in oxic subseafloor sediment. Nat. Geosci. 12, 126–131. doi: 10.1038/s41561-018-0291-5
Fabiano M., Sarà G., Mazzola M., Pusceddu A. (2000). “Environmental constrains on pathways of organic detritus in a semi-enclosed marine system (W-Mediterranean),” in Structures and processes in the mediterranean ecosystems. Eds. Faranda F. M., Guglielmo L., Spezie G. (Springer, Italy), 435–445.
Gasol J. M., Del Giorgio P. A. (2000). Using flow cytometry for counting natural planktonic bacteria and understanding the structure of planktonic bacterial communities. Sci. Mar. 64, 197–224. doi: 10.3989/scimar.2000.64n2197
Hansell D. A. (2013). Recalcitrant dissolved organic carbon fractions. Ann. Rev. Mar. Sci. 5, 421–445. doi: 10.1146/annurev-marine-120710-100757
Harrison X. A., Donaldson L., Correa-Cano M. E., Evans J., Fisher D. N., Goodwin C. E. D., et al. (2018). A brief introduction to mixed effects modelling and multi-model inference in ecology. PeerJ 6, e4794. doi: 10.7717/peerj.4794
Hilmi N., Chami R., Sutherland M. D., Hall-Spencer J. M., Lebleu L., Benitez M. B., et al. (2021). The role of blue carbon in climate change mitigation and carbon stock conservation. Front. Clim. 3. doi: 10.3389/fclim.2021.710546
Holmer M., Duarte C., Boschker H., Barrón C. (2004). Carbon cycling and bacterial carbon sources in pristine and impacted Mediterranean seagrass sediments. Aquat. Microb. Ecol. 36, 227–237. doi: 10.3354/ame036227
Holmer M., Marbà N., Lamote M., Duarte C. M. (2009). Deterioration of Sediment Quality in Seagrass Meadows (Posidonia oceanica) Invaded by Macroalgae (Caulerpa sp.). Estuaries Coasts 32, 456–466. doi: 10.1007/s12237-009-9133-4
Howard J., Hoyt S., Isensee K., Telszewski M., Pidgeon E. (2014). Coastal blue carbon: methods for assessing carbon stocks and emissions factors in mangroves, tidal salt marshes, and seagrasses (Arlington, Virginia, USA: Conservation International, Intergovernmental Oceanographic Commission of UNESCO, International Union for Conservation of Nature).
Ionescu D., Bizic-Ionescu M., Khalili A., Malekmohammadi R., Morad M. R., de Beer D., et al. (2015). A new tool for long-term studies of POM-bacteria interactions: overcoming the century-old Bottle Effect. Sci. Rep. 5, 14706. doi: 10.1038/srep14706
IUCN. (2021). “Manual for the creation of Blue Carbon projects in Europe and the Mediterranean,” in International Union for Conservation of Nature and Natural Resources. Ed. Otero M. (Center for Marine Cooperation, SOLPRINT S.L), 1–144.
Jiao N., Robinson C., Azam F., Thomas H., Baltar F., Dang H., et al. (2014). Mechanisms of microbial carbon sequestration in the ocean – future research directions. Biogeosciences 11, 5285–5306. doi: 10.5194/bg-11-5285-2014
Jiménez-Ramos R., Henares C., Egea L. G., Vergara J. J., Brun F. G. (2023). Leaf senescence of the seagrass cymodocea nodosa in Cádiz Bay, Southern Spain. Diversity 15, 187. doi: 10.3390/d15020187
Jiménez-Ramos R., Tomas F., Reynés X., Romera-Castillo C., Pérez-Lloréns J. L., Egea L. G. (2022). Carbon metabolism and bioavailability of dissolved organic carbon (DOC) fluxes in seagrass communities are altered under the presence of the tropical invasive alga Halimeda incrassata. Sci. Total Environ. 839, 156325. doi: 10.1016/j.scitotenv.2022.156325
Junta de Andalucía. (2021). Andalusian carbon standard for the certification of blue carbon credits, the absorption calculator and the Andalusian catalog of blue carbon projects (Spain). Available online at: https://www.juntadeandalucia.es/medioambiente/portal/web/cambio-climatico/indice/-/asset_publisher/hdxWUGtQGkX8/content/compensaci-c3-b3n-de-emisiones/328613.
Kilminster K., McMahon K., Waycott M., Kendrick G. A., Scanes P., McKenzie L., et al. (2015). Unravelling complexity in seagrass systems for management: Australia as a microcosm. Sci. Total Environ. 534, 97–109. doi: 10.1016/j.scitotenv.2015.04.061
Kloareg B., Quatrano R. S. (1988). Structure of the cell walls of marine algae and ecophysiological functions of the matrix polysaccharides. Oceanogr. Mar. Biol. Annu. Rev. 26, 259–315.
Koch B. P., Kattner G., Witt M., Passow U. (2014). Molecular insights into the microbial formation of marine dissolved organic matter: recalcitrant or labile? Biogeosciences 11, 4173–4190. doi: 10.5194/bg-11-4173-2014
Komada T., Burdige D. J., Crispo S. M., Druffel E. R. M., Griffin S., Johnson L., et al. (2013). Dissolved organic carbon dynamics in anaerobic sediments of the Santa Monica Basin. Geochim. Cosmochim. Acta 110, 253–273. doi: 10.1016/j.gca.2013.02.017
Krause-Jensen D., Duarte C. M. (2016). Substantial role of macroalgae in marine carbon sequestration. Nat. Geosci. 9, 737–742. doi: 10.1038/ngeo2790
Kruse S., Brey T., Bathmann U. (2010). Role of midwater chaetognaths in Southern Ocean pelagic energy flow. Mar. Ecol. Prog. Ser. 416, 105–113. doi: 10.3354/meps08773
Kuzyakov Y., Friedel J., Stahr K. (2000). Review of mechanisms and quantification of priming effects. Soil Biol. Biochem. 32, 1485–1498. doi: 10.1016/S0038-0717(00)00084-5
Lande R., Wood A. M. (1987). Suspension times of particles in the upper ocean. Deep Sea Res. Part A. Oceanogr. Res. Pap. 34, 61–72. doi: 10.1016/0198-0149(87)90122-1
LaRowe D. E., Arndt S., Bradley J. A., Estes E. R., Hoarfrost A., Lang S. Q., et al. (2020). The fate of organic carbon in marine sediments - New insights from recent data and analysis. Earth-Science Rev. 204, 103146. doi: 10.1016/j.earscirev.2020.103146
Lavery P., McMahon K., Weyers J., Boyce M., Oldham C. (2013). Release of dissolved organic carbon from seagrass wrack and its implications for trophic connectivity. Mar. Ecol. Prog. Ser. 494, 121–133. doi: 10.3354/meps10554
Lenth R. V., Buerkner P., Herve M., Love J., Miguez F., Riebl H., et al. (2022). Emmeans: estimated marginal means, aka least-squares means. J. Stat. Software 69, 1–33. doi: 10.1080/00031305.1980.10483031
Li S., Hou X., Shi Y., Huang T., Yang H., Huang C. (2020). Rapid photodegradation of terrestrial soil dissolved organic matter (DOM) with abundant humic-like substances under simulated ultraviolet radiation. Environ. Monit. Assess. 192, 103. doi: 10.1007/s10661-019-7945-7
Lønborg C., Álvarez-Salgado X. A., Duggan S., Carreira C. (2018). Organic matter bioavailability in tropical coastal waters: The Great Barrier Reef. Limnol. Oceanogr. 63, 1015–1035. doi: 10.1002/lno.10717
Macreadie P. I., Atwood T. B., Seymour J. R., Fontes M. L. S., Sanderman J., Nielsen D. A., et al. (2019). Vulnerability of seagrass blue carbon to microbial attack following exposure to warming and oxygen. Sci. Total Environ. 686, 264–275. doi: 10.1016/j.scitotenv.2019.05.462
Macreadie P. I., Baird M. E., Trevathan-Tackett S. M., Larkum A. W. D., Ralph P. J. (2014). Quantifying and modelling the carbon sequestration capacity of seagrass meadows – A critical assessment. Mar. Pollut. Bull. 83, 430–439. doi: 10.1016/j.marpolbul.2013.07.038
Macreadie P. I., Nielsen D. A., Kelleway J. J., Atwood T. B., Seymour J. R., Petrou K., et al. (2017). Can we manage coastal ecosystems to sequester more blue carbon? Front. Ecol. Environ. 15, 206–213. doi: 10.1002/fee.1484
Marbà N., Arias-Ortiz A., Masqué P., Kendrick G. A., Mazarrasa I., Bastyan G. R., et al. (2015). Impact of seagrass loss and subsequent revegetation on carbon sequestration and stocks. J. Ecol. 103, 296–302. doi: 10.1111/1365-2745.12370
Marin-Diaz B., Bouma T. J., Infantes E. (2020). Role of eelgrass on bed-load transport and sediment resuspension under oscillatory flow. Limnol. Oceanogr. 65, 426–436. doi: 10.1002/lno.11312
Mateo M.Á., Díaz-Almela E., Piñeiro-Juncal N., Leiva-Dueñas C., Giralt S., Marco-Méndez C. (2018). “Carbon stocks and fluxes associated to Andalusian seagrass meadows,” in Deliverable C1: Results Report LIFE Blue Natura (Centre for Advanced Studies of Blanes, Spanish Council for Scientific Research, Blanes), 1–94.
Mazarrasa I., Lavery P., Duarte C. M., Lafratta A., Lovelock C. E., Macreadie P. I., et al. (2021). Factors determining seagrass blue carbon across bioregions and geomorphologies. Global Biogeochem. Cycles 35, e2021GB006935. doi: 10.1029/2021GB006935
Mazarrasa I., Marbà N., Lovelock C. E., Serrano O., Lavery P. S., Fourqurean J. W., et al. (2015). Seagrass meadows as a globally significant carbonate reservoir. Biogeosciences 12, 4993–5003. doi: 10.5194/bg-12-4993-2015
Mazarrasa I., Samper-Villarreal J., Serrano O., Lavery P. S., Lovelock C. E., Marbà N., et al. (2018). Habitat characteristics provide insights of carbon storage in seagrass meadows. Mar. Pollut. Bull. 134, 106–117. doi: 10.1016/j.marpolbul.2018.01.059
Mcleod E., Chmura G. L., Bouillon S., Salm R., Björk M., Duarte C. M., et al. (2011). A blueprint for blue carbon: toward an improved understanding of the role of vegetated coastal habitats in sequestering CO2. Front. Ecol. Environ. 9, 552–560. doi: 10.1890/110004
Moksnes P., Röhr M. E., Holmer M., Eklöf J. S., Eriander L., Infantes E., et al. (2021). Major impacts and societal costs of seagrass loss on sediment carbon and nitrogen stocks. Ecosphere 12, e03658. doi: 10.1002/ecs2.3658
Moran M. A., Ferrer-González F. X., Fu H., Nowinski B., Olofsson M., Powers M. A., et al. (2022). The Ocean’s labile DOC supply chain. Limnol. Oceanogr. 67, 1007–1021. doi: 10.1002/lno.12053
Needelman B. A., Emmer I. M., Emmett-Mattox S., Crooks S., Megonigal J. P., Myers D., et al. (2018). The science and policy of the verified carbon standard methodology for tidal wetland and seagrass restoration. Estuaries Coasts 41, 2159–2171. doi: 10.1007/s12237-018-0429-0
Omand M. M., Govindarajan R., He J., Mahadevan A. (2020). Sinking flux of particulate organic matter in the oceans: Sensitivity to particle characteristics. Sci. Rep. 10, 5582. doi: 10.1038/s41598-020-60424-5
Pausz C., Herndl G. (1999). Role of ultraviolet radiation on phytoplankton extracellular release and its subsequent utilization by marine bacterioplankton. Aquat. Microb. Ecol. 18, 85–93. doi: 10.3354/ame018085
Pusceddu A., Grémare A., Escoubeyrou K., Amouroux J. M., Fiordelmondo C., Danovaro R. (2005). Impact of natural (storm) and anthropogenic (trawling) sediment resuspension on particulate organic matter in coastal environments. Cont. Shelf Res. 25, 2506–2520. doi: 10.1016/j.csr.2005.08.012
Ralph P. J., Tomasko D., Moore K., Seddon S., Macinnis-Ng C. M. O. (2006). “Human impacts on seagrasses: eutrophication, sedimentation and contamination,” in Seagrasses: Biology, Ecology and Conservation. Eds. Larkum A. W. D., Orth R. J., Duarte C. M. (Springer), 567–593.
R Development Core Team. (2022). R: A Language and Environment for Statistical Computing (Vienna, Austria: R Foundation for Statistical Computing). Available at: https://www.R-project.org/.
Reche I., Pace M. L., Cole J. J. (1999). Relationship of trophic and chemical conditions to photobleaching of dissolved organic matter in lake ecosystems. Biogeochemistry 44, 259–280. doi: 10.1007/BF00996993
Röhr M. E., Boström C., Canal-Vergés P., Holmer M. (2016). Blue carbon stocks in Baltic Sea eelgrass (Zostera marina) meadows. Biogeosciences 13, 6139–6153. doi: 10.5194/bg-13-6139-2016
Salinas C., Duarte C. M., Lavery P. S., Masque P., Arias-Ortiz A., Leon J. X., et al. (2020). Seagrass losses since mid-20th century fuelled CO2 emissions from soil carbon stocks. Glob. Change Biol. 26, 4772–4784. doi: 10.1111/gcb.15204
Sanford L. P. (1992). New sedimentation, resuspension, and burial. Limnol. Oceanogr. 37, 1164–1178. doi: 10.4319/lo.1992.37.6.1164
Santos R., Duque-Núñez N., de los Santos C. B., Martins M., Carrasco A. R., Veiga-Pires C. (2019). Superficial sedimentary stocks and sources of carbon and nitrogen in coastal vegetated assemblages along a flow gradient. Sci. Rep. 9, 610. doi: 10.1038/s41598-018-37031-6
Serrano O., Lavery P., Masque P., Inostroza K., Bongiovanni J., Duarte C. (2016). Seagrass sediments reveal the long-term deterioration of an estuarine ecosystem. Glob. Change Biol. 22, 1523–1531. doi: 10.1111/gcb.13195
Serrano O., Mateo M. A., Renom P., Julià R. (2012). Characterization of soils beneath a Posidonia oceanica meadow. Geoderma 185–186, 26–36. doi: 10.1016/j.geoderma.2012.03.020
Steinberg D. K., Landry M. R. (2017). Zooplankton and the ocean carbon cycle. Ann. Rev. Mar. Sci. 9, 413–444. doi: 10.1146/annurev-marine-010814-015924
Trevathan-Tackett S. M., Seymour J. R., Nielsen D. A., Macreadie P. I., Jeffries T. C., Sanderman J., et al. (2017). Sediment anoxia limits microbial-driven seagrass carbon remineralization under warming conditions. FEMS Microbiol. Ecol. 93, fix033. doi: 10.1093/femsec/fix033
Vergara J. J., García-Sánchez M. P., Olivé I., García-Marín P., Brun F. G., Pérez-Lloréns J. L., et al. (2012). Seasonal functioning and dynamics of Caulerpa prolifera meadows in shallow areas: An integrated approach in Cadiz Bay Natural Park. Estuar. Coast. Shelf Sci. 112, 255–264. doi: 10.1016/j.ecss.2012.07.031
Zhang Y., Liu M., Qin B., Feng S. (2009). Photochemical degradation of chromophoric-dissolved organic matter exposed to simulated UV-B and natural solar radiation. Hydrobiologia 627, 159–168. doi: 10.1007/s10750-009-9722-z
Zhang M., Qin H., Ma Y., Qi Y., Zhao Y., Wang Z., et al. (2023). Carbon sequestration from refractory dissolved organic carbon produced by biodegradation of Saccharina japonica. Mar. Environ. Res. 183, 105803. doi: 10.1016/j.marenvres.2022.105803
Keywords: sediment organic carbon, dissolved organic carbon, remineralization, recalcitrant DOC, labile DOC, Cymodocea nodosa, Zostera noltei, Caulerpa prolifera
Citation: Yamuza-Magdaleno A, Jiménez-Ramos R, Casal-Porras I, Brun FG and Egea LG (2024) Long-term sediment organic carbon remineralization in different seagrass and macroalgae habitats: implication for blue carbon storage. Front. Mar. Sci. 11:1370768. doi: 10.3389/fmars.2024.1370768
Received: 15 January 2024; Accepted: 12 March 2024;
Published: 25 March 2024.
Edited by:
Elva G. Escobar-Briones, National Autonomous University of Mexico, MexicoReviewed by:
Yunchao Wu, Chinese Academy of Sciences (CAS), ChinaCopyright © 2024 Yamuza-Magdaleno, Jiménez-Ramos, Casal-Porras, Brun and Egea. This is an open-access article distributed under the terms of the Creative Commons Attribution License (CC BY). The use, distribution or reproduction in other forums is permitted, provided the original author(s) and the copyright owner(s) are credited and that the original publication in this journal is cited, in accordance with accepted academic practice. No use, distribution or reproduction is permitted which does not comply with these terms.
*Correspondence: Rocío Jiménez-Ramos, cm9jaW8uamltZW5lekB1Y2EuZXM=
Disclaimer: All claims expressed in this article are solely those of the authors and do not necessarily represent those of their affiliated organizations, or those of the publisher, the editors and the reviewers. Any product that may be evaluated in this article or claim that may be made by its manufacturer is not guaranteed or endorsed by the publisher.
Research integrity at Frontiers
Learn more about the work of our research integrity team to safeguard the quality of each article we publish.