- 1Fishery College, Guangdong Ocean University, Zhanjiang, China
- 2Department of Water Resources and Aquaculture Management, School of Sustainable Development, University of Environment and Sustainable Development, Somanya, Eastern Region, Ghana
- 3Department of Biological Sciences, School of Natural and Environmental Sciences, University of Environment and Sustainable Development, Somanya, Eastern Region, Ghana
- 4Department of Fisheries and Aquatic Resources Management, University for Development Studies, Nyankpala, Ghana
- 5Southern Marine Science and Engineering Guangdong Laboratory (Zhanjiang), Zhanjiang, China
- 6Guangdong Provincial Key Laboratory of Pathogenic Biology and Epidemiology for Aquatic Economic Animals, Zhanjiang, China
There has been a surge of research in the aquaculture industry investigating probiotic, prebiotic, and synbiotic interventions on the physiological mechanisms of fish, specifically digestive enzymes, oxidative stress, and antioxidant defense. In fish, probiotics have been shown to improve nutrient utilization and growth performance by stimulating digestive enzymes. Meanwhile, probiotics, prebiotics and synbiotics have also been studied for their ability to modulate oxidative stress and antioxidant defense mechanisms in fish, highlighting their multifaceted health benefits. This review identified current trends, research gaps, and future considerations in this evolving field. Although promising findings have been made, a significant research gap exists in understanding the specific role of probiotics prebiotics, and synbiotics in modulating digestive enzymes, oxidative stress, and antioxidant defense systems in a variety of fish species. As this study investigate into the existing body of literature, it becomes evident that while certain aspects of these interactions have been elucidated, a nuanced and comprehensive understanding still needs to be discovered. The variations in experimental design, species-specific responses, and the lack of standardized methodologies contribute to the complexity of the field. Digestive physiology and antioxidant defense mechanisms vary among different fish species, so future research should focus on species-specific responses to probiotic, prebiotic, and synbiotic formulations. It will also be possible to establish robust correlations between dietary interventions and observed effects through a systematic experimental design and methodology approach. Accordingly, further research is needed to understand the interactions between probiotics, prebiotics, and synbiotics in fish and digestive enzymes, oxidative stress, and antioxidant defense. Identifying research gaps and adopting standardized methodologies can help develop tailored strategies to optimize aquaculture fish health and growth performance.
1 Introduction
In the ever-evolving landscape of aquaculture, the delicate balance between maximizing fish production and ensuring the health and well-being of aquatic organisms remains a paramount concern (Ribas and Piferrer, 2014; Ibrahem, 2015; Dawood and Koshio, 2016; Mukherjee et al., 2019). One of the pivotal aspects in achieving this equilibrium lies in comprehending the complex interaction between digestive enzymes, oxidative stress, and antioxidant defense mechanisms in fish (Hoseinifar et al., 2015a; Hoseinifar et al., 2015b; Hoseinifar et al., 2021). Over the years, significant strides have been made in elucidating the multifaceted relationship between these physiological processes (Schieber and Chande, 2014; Hoseinifar et al., 2021). Nonetheless, a conspicuous research gap persists, necessitating a comprehensive review to synthesize existing knowledge, identify current limitations, and chart the course for future investigations.
The digestive system of fish is a complex network of enzymatic reactions that orchestrate the breakdown and assimilation of nutrients essential for growth, development, and overall physiological function (Mata-Sotres et al., 2016; Assan et al., 2022; Amenyogbe et al., 2022a; Amenyogbe et al., 2022b; Amenyogbe et al., 2022c). In tandem with these digestive processes, oxidative stress and antioxidant defense mechanisms play a pivotal role in maintaining cellular homeostasis (Hoseinifar et al., 2021). Recent studies have underscored the potential modulatory effects of probiotics, prebiotics, and synbiotics on these crucial physiological pathways in fish (Olmos-Soto, 2014; Wang C. et al., 2019; Wang et al., 2021; Assan et al., 2022). Probiotics, live microorganisms with documented health benefits, prebiotics, non-digestible compounds that selectively stimulate the growth and activity of beneficial microorganisms, and synbiotics, combinations of probiotics and prebiotics, have emerged as promising candidates for enhancing the digestive efficiency and oxidative stress resilience of fish (FAO/WHO, 2001; Beck et al., 2014; Olmos-Soto, 2014; Dawood et al., 2016a, b; Van Doan et al., 2016; Xia et al., 2018; Hoseinifar et al., 2019; Wang et al., 2019; Zuo et al., 2019; Wang et al., 2021).
While individual studies have investigated the influence of probiotics, prebiotics, and synbiotics on either digestive enzymes or oxidative stress in fish (Table 1), there exists a discernible gap in the literature that systematically integrates these disparate aspects into a unified framework (Olmos-Soto, 2014; Hoseinifar et al., 2015a, b; Wang et al., 2019; Wang et al., 2021; Assan et al., 2022). This review aims to bridge this gap by comprehensively synthesizing current research findings, highlighting interactions, and identifying areas that require further exploration. By doing so, the study aspire to offer a holistic understanding of how probiotics, prebiotics, and synbiotics collectively impact fish’s digestive enzymes and oxidative stress responses.
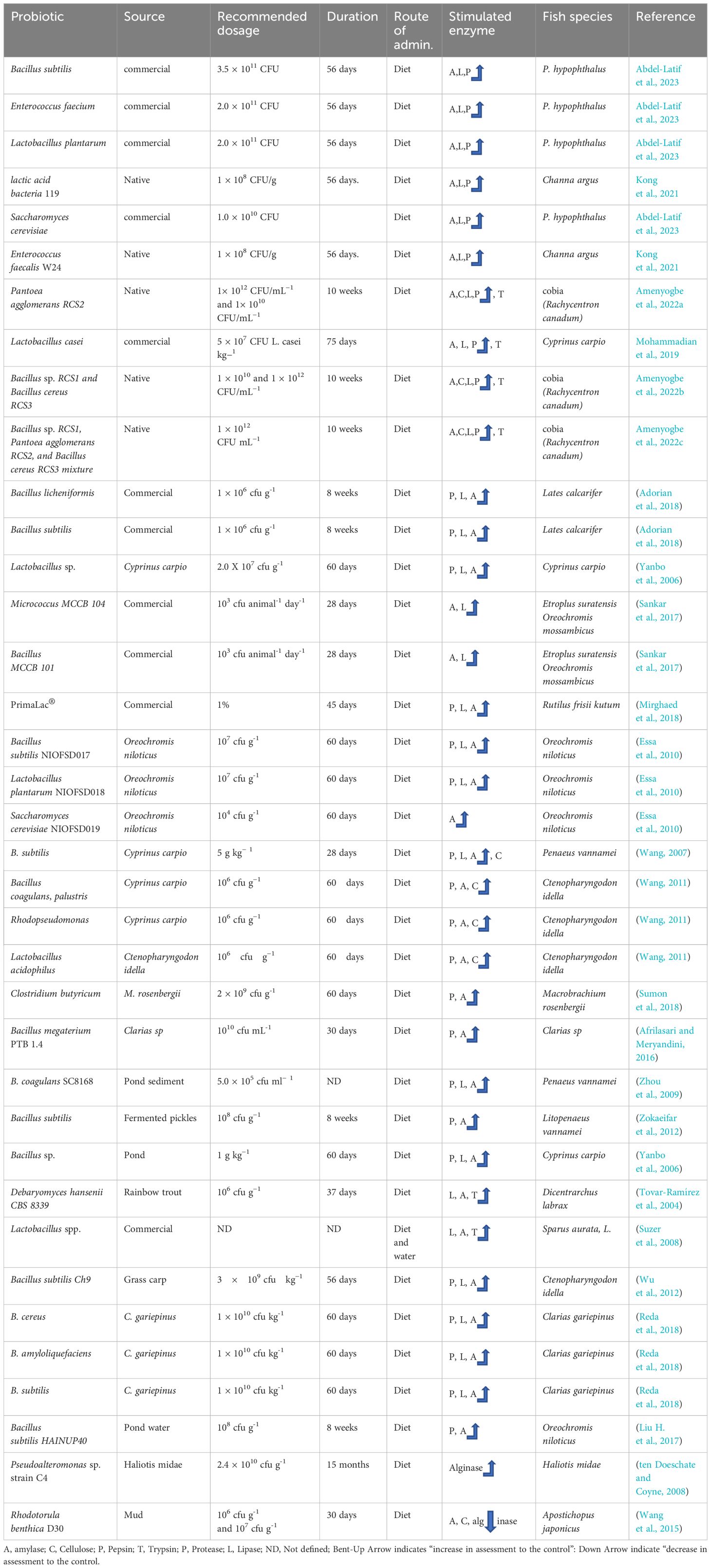
Table 1 An overview of the application of probiotics in regulating digestive enzymes in cultured fish.
As this study probe into the existing body of literature, it becomes evident that while certain aspects of these interactions have been elucidated, a nuanced and comprehensive understanding still needs to be discovered. The variations in experimental design, species-specific responses, and the lack of standardized methodologies contribute to the complexity of the field. Consequently, this review will critically evaluate the methodologies employed in existing studies and propose standardized approaches to facilitate cross-study comparisons and data extrapolation. Looking ahead, it is imperative to outline a roadmap for future investigations. Identifying specific strains, doses, and application methods of probiotics, prebiotics, and synbiotics that yield optimal outcomes in different fish species represents a critical avenue for further exploration. Moreover, the molecular mechanisms underlying the observed effects still need to be more understood and warrant in-depth investigation. By addressing these research gaps, the study aim to contribute to the sustainable advancement of aquaculture practices, fostering a balance between increased production efficiency and preserving fish health and welfare.
2 Data availability, materials and methods
Following the methods used by Amenyogbe (2023), in brief, detecting or identifying papers was conducted using Web of Science, Google Scholar, and subscribed journals as Core Collections. In order to retrieve papers published up to 2023, several keywords were initially used as hunt criteria. According to the preliminary search results, studies related to fish probiotics, prebiotics, and synbiotics were distinguished. A total of 233 publications describing aquaculture probiotics, prebiotics, and synbiotics have been obtained in full text.
3 Overview of the importance of digestive health and the role of oxidative stress and antioxidant defense in fish health
A group of enzymes present in the digestive tracts of animals are known as digestive enzymes, a, play a crucial role in breaking down macromolecules into their tiniest sizes for efficient absorption via the body, energy metabolism, and overall health (Watling, 2015; Mata-Sotres et al., 2016; Xiong et al., 2019; Assan et al., 2022; Gao et al., 2022; Amenyogbe et al., 2022a; Amenyogbe et al., 2022b; Amenyogbe et al., 2022c). Understanding the complexities of fish digestive physiology is essential for optimizing aquaculture practices, preserving wild populations, and ensuring sustainable fisheries (Xiong et al., 2019). While data from fish indicates qualitative similarities with digestive enzymes in other vertebrates, the understanding of fish digestive processes lags behind that of mammals. This disparity arises from different methodologies used in analyzing data, particularly in the collection of digestive tract secretions from other vertebrates (Assan et al., 2022). Examining the presence and activity levels of digestive enzymes provides valuable insights into food acceptance, the rate of fish larvae development, digestive efficiency, and ultimately, survival rates (Suzer et al., 2008). Research, such as that undertaken by Solovyev et al. (2014), demonstrates that analyzing the activity of digestive enzymes in fish offers valuable understanding of their role in ecosystems and feeding habits under natural conditions. Mata-Sotres et al. (2016) noted that impressive growth rates in larval fish result from effective food processing facilitated by high ingestion rates and the presence of highly active digestive enzymes. Fish need proteases to break down proteins from their diet into amino acids (Huang et al., 2021). Amino acids are essential for various physiological functions, including growth, tissue repair, and the synthesis of enzymes and hormones. Lipases help in the digestion of lipids (fats) (Calado et al., 2007; Rivera-Pérez et al., 2010; Gao et al., 2022). Fatty acids derived from lipid digestion are crucial for energy production, cell membrane formation, and the synthesis of hormones and signaling molecules. Carbohydrases are responsible for breaking down complex carbohydrates into simple sugars. These sugars serve as a direct energy source for fish and are essential for maintaining metabolic processes. The efficiency of digestion directly impacts the fish’s growth, development, and overall health.
In literature, the participation of cellulolytic, lipolytic, proteolytic, and amylolytic, enzymes in breaking down carbohydrates, proteins, lipids, and cellulose during fish digestion is emphasized (Falc´on-Hidalgo et al., 2011; Ray et al., 2012; Nedaei et al., 2019; Gao et al., 2022). These enzymes play a crucial role in stimulating the development and growth of aquaculture fish species (Kavitha et al., 2018). The rate of digestion in fish is established to impact nutrient uptake, consequently influencing the overall growth and development of the organism (Pittman et al., 2013). In aquaculture, the growth and production costs of fish are heavily influenced by the digestibility of their feed, which can be improved through the augmentation of digestive enzyme activityThe presence of intestinal bacteria aids in food digestion, while the introduction of probiotic bacteria enhances nutrient absorption and promotes more efficient food digestion (Afrilasari and Meryandini, 2016). Probiotic bacteria have been shown to increase digestive enzyme activities, leading to enhanced growth in various species, including white shrimp Litopenaeus vannamei, Indian white shrimp Fenneropenaeus indicus, abalone Haliotis asinina, and humpback grouper Cromileptes altivelis (Takami et al., 2006; Zokaeifar et al., 2012; Marlida et al., 2014; Faturrahman et al., 2015). Probiotics, as beneficial microbes, are recognized for improving the overall health and nutrition of host organisms (Assan et al., 2022). Research has shown that probiotics enhance the activity of digestive enzymes in fish by producing their own enzymes. These enzymes are released into the intestinal tract, increasing the overall pool of enzymes. As a result, they break down food nutrients, making them more accessible to the host fish through the resulting metabolites they secrete (Wang, 2007; Suzer et al., 2008; Zhou et al., 2009). Research on fish digestive health has substantially contributed to understanding nutrient digestion, absorption, and utilization (Table 1) (Burns et al., 2016; Yan et al., 2016; Nie et al., 2017; Smith et al., 2017). Studies have elucidated the enzymatic processes involved in breaking down complex nutrients, such as proteins, lipids, and carbohydrates. Moreover, advancements in molecular biology have allowed researchers to explore the microbiota residing in the fish gut, shedding light on its role in nutrient metabolism and host immunity (Liu et al., 2017; Nie et al., 2017).
Elevated levels of reactive oxygen species (ROS) within cells, associated with oxidative stress, can lead to detrimental effects on lipids, proteins, and DNA (Schieber and Chande, 2014; Hoseinifar et al., 2021). Oxidative stress occurs when there is an imbalance between the production of reactive oxygen species (ROS) and the ability of the fish’s antioxidant defense system to neutralize them. The imbalance between ROS production and antioxidant defense, termed oxidative stress, can result in DNA hydroxylation, protein denaturation, lipid peroxidation, apoptosis, and ultimately cell damage (Hoseinifar et al., 2021). Hydroxyl radicals and hydrogen peroxides are key oxygen radicals contributing to ROS. To counteract the negative impacts of naturally occurring ROS, living organisms have developed an antioxidant defense system, comprising enzymatic antioxidants (e.g., superoxide dismutase, glutathione peroxidase, glutathione reductase, and catalase) and non-enzyme antioxidants (e.g., glutathione, thioredoxin, vitamin C, and vitamin E) (Hoseinifar et al., 2021). ROS, such as superoxide radicals and hydrogen peroxide, can cause damage to lipids, proteins, and DNA within the fish’s cells (Schieber and Chande, 2014; Hoseinifar et al., 2021). This antioxidant defense system maintains the balance between ROS production and removal under normal physiological conditions (Li et al., 2016; Dong et al., 2017), playing a crucial role in preserving homeostasis and preventing oxidative stress-related damage (Li et al., 2016; Dong et al., 2017). Fish produce ROS as byproducts during normal metabolic processes. Factors like pollution, temperature changes, and poor water quality can increase ROS production (Cadenas, 1995; Schieber and Chande, 2014; Halliwell and Gutteridge, 2015). Infections and diseases can trigger ROS production as part of the fish’s immune response. ROS can damage cell membranes, proteins, and DNA, leading to impaired cellular function (Halliwell and Gutteridge, 2015; Li et al., 2016; Dong et al., 2017). Fish experiencing oxidative stress are more susceptible to diseases (Jena, 2012; Birnie-Gauvin et al., 2017; Prego-Faraldo et al., 2017; Biller and Takahashi, 2018; Ji et al., 2018; Hematyar et al., 2019). Oxidative stress can hinder growth rates in fish.
The physiological status of an organism is closely linked to its antioxidant defense, with increased efficiency and levels of antioxidant defense providing various benefits to the host (Halliwell and Gutteridge, 2015; Li et al., 2016; Yang et al., 2018). Consequently, there has been considerable research focused on enhancing the activities of antioxidant enzymes in fish and shellfish. While synthetic antioxidants such as butylated hydroxyanisole and butylated hydroxytoluene have been traditionally employed to boost antioxidant defense and inhibit lipid peroxidation, their use is becoming restricted due to environmental and health concerns, including liver damage and cancer (Halliwell and Gutteridge, 2015; Li et al., 2016; Yang et al., 2018). In recent years, efforts have been directed toward identifying novel and safe natural antioxidants as alternatives to synthetic compounds (Hoseinifar et al., 2021).
Fish have evolved elaborate antioxidant defense mechanisms to counteract oxidative stress and maintain cellular homeostasis (Halliwell and Gutteridge, 2015; Li et al., 2016; Yang et al., 2018; Hoseinifar et al., 2021). These defense mechanisms include enzymatic and non-enzymatic antioxidants. Converts superoxide radicals into hydrogen peroxide. Converts hydrogen peroxide into water and oxygen (Yang et al., 2018; Gao et al., 2022). Neutralizes hydrogen peroxide and lipid peroxides. Non-enzymatic Antioxidants: Vitamins C and E Act as scavengers of free radicals. Glutathione acts as a crucial cellular antioxidant. Carotenoids have antioxidant properties and contribute to fish coloration (Malhotra and Kaufman, 2007; Birnie-Gauvin et al., 2017; Xie et al., 2019). In the context of aquaculture, where oxidative stress is particularly relevant, especially in intensive and stressful modern culture systems, researchers are exploring the potential of enhancing the antioxidant defense of cultured organisms using beneficial microbes. Previous studies in aquaculture have demonstrated promising antioxidative effects of beneficial additives such as probiotics, prebiotics, and synbiotics across various fish species (Dawood et al., 2018; Van Doan et al., 2020; Hoseinifar et al., 2021). This highlights the importance of investigating the use of beneficial microorganisms to ameliorate antioxidant defense in aquaculture practices.
Despite these advancements, several gaps persist in our knowledge of fish digestive health and the role of oxidative stress and antioxidant defense in fish health. First, there is a need for more comprehensive studies on the diversity and function of the fish gut microbiome across species and environmental conditions (Burns et al., 2016; Yan et al., 2016; He et al., 2017; Nie et al., 2017; Smith et al., 2017). This includes understanding how external factors, such as diet, water quality, and stress, impact the composition and activity of the gut microbiota. Second, research on the ontogeny of the fish digestive system is limited, hindering our understanding of how digestive processes change during different life stages. Third, the influence of environmental pollutants on fish digestive health remains an underexplored area, with potential implications for both wild and farmed fish populations (Sullam et al., 2012; Schmidt et al., 2015; Yan et al., 2016; Nie et al., 2017).
Addressing these research gaps requires a multidisciplinary approach. Future studies should integrate molecular biology, ecology, and aquaculture science to provide a holistic understanding of fish digestive health. Investigating the impact of environmental stressors, such as climate change and pollution, on fish digestive physiology is crucial for predicting and mitigating potential adverse effects. Additionally, continue advancements in omics technologies can facilitate in-depth analyses of the fish gut microbiome, enabling researchers to uncover novel interactions between the microbiota and host physiology (Sullam et al., 2012). Collaboration between researchers, industry stakeholders, and policymakers is essential for translating research findings into practical applications. Developing strategies to enhance digestive health in aquaculture settings can improve fish growth rates, feed efficiency, and disease resistance. Furthermore, understanding the role of the fish gut in nutrient cycling within aquatic ecosystems is vital for managing and conserving wild fish populations. By addressing these gaps and adopting a holistic approach that considers the diverse factors influencing digestive physiology, researchers can contribute to developing sustainable aquaculture practices and conserving wild fish populations. The integration of emerging technologies and collaboration across disciplines will be instrumental in advancing our knowledge of this complex and vital aspect of fish biology.
3.1 Overview of probiotics, prebiotics, and synbiotics: advantages in the context of fish farming
Probiotics are live microorganisms that confer health benefits to the host when administered in adequate amounts (Fuller, 1989; FAO/WHO, 2001; FAO, 2011; Ferreira, 2014; Newaj-Fyzul et al., 2014; Jesus et al., 2016; FAO, 2016). In the case of fish, these microorganisms are usually bacteria or yeast strains that promote a balanced and beneficial microbial community in the fish gut (Nayak, 2010; Iribarren et al., 2012; Ferreira, 2014; Newaj-Fyzul et al., 2014). The gut microbiota plays a crucial role in nutrient absorption, immune system regulation, and overall health (Figures 1–3). Probiotics for fish farming are commonly chosen from Lactic acid bacteria (LAB) such as Lactobacillus and Bacillus species (Balcázar et al., 2006; Dotta et al., 2011; Ferreira, 2014; Torres, 2014; Paixão et al., 2017; Yu et al., 2017; Zhai et al., 2017; Didinen et al., 2018; Amenyogbe et al., 2021; Amenyogbe et al., 2022a; Amenyogbe et al., 2022b; Amenyogbe et al., 2022c). Probiotics are recognized for their capacity to produce beneficial enzymes that aid in digestion and protect the gastrointestinal tract (GIT) (Sumon et al., 2018). The proper use of probiotics can enhance the balance of intestinal microorganisms, leading to improved activity of digestive enzymes, enhanced food absorption, and reduced pathogenic issues in the GIT (Figure 3) (Balcazar et al., 2007; Dotta et al., 2011; Muñoz-Atienza et al., 2013; Ferreira, 2014; Torres, 2014; Azevedo et al., 2016; Paixão et al., 2017; Yu et al., 2017; Zhai et al., 2017; Didinen et al., 2018; Linh et al., 2018; Chen et al., 2019; Amenyogbe et al., 2021; Ringø et al., 2022; Amenyogbe et al., 2022a). Scientists suggest that a key way probiotics benefit organisms in cultivation is by enhancing their nutrition through the generation of extra digestive enzymes. This leads to improved feed efficiency, accelerated growth, and the mitigation of intestinal disorders and antinutritional factors (Verschuere et al., 2000; Suzer et al., 2008). Following transit through the stomach, probiotics multiply within the intestine and utilize diverse carbohydrates to support their growth, while also producing vital digestive enzymes including lipase amylase, and protease (Suzer et al., 2008), by adding additional enzymes to those naturally produced by the host, the overall levels of digestive enzymes in the host’s gut are increased.
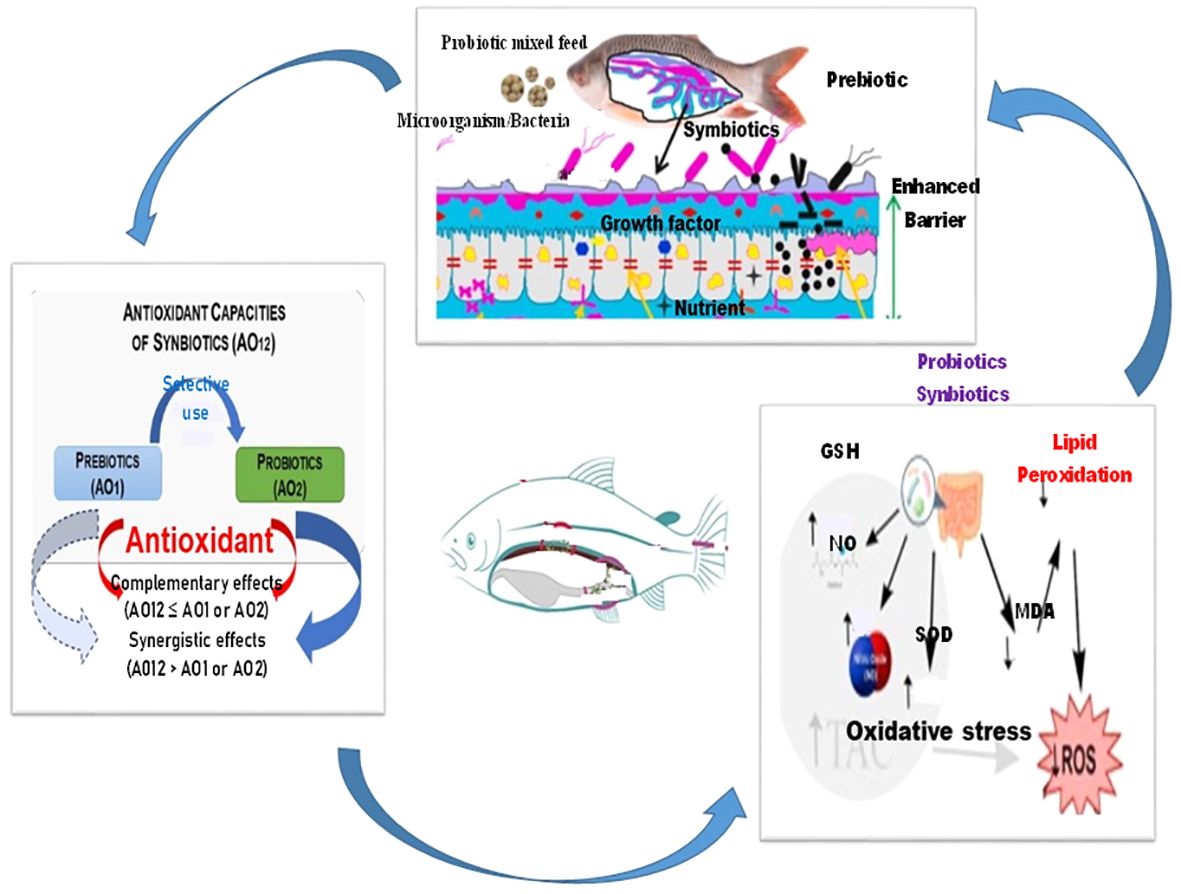
Figure 1 The mechanism by which probiotics, prebiotics and synbiotics act in the guts of host animals. Probiotics, Prebiotics, and Synbiotics plays a crucial role in nutrient absorption, immune system regulation, decreased oxidative stress and overall health, indicating that these microorganisms may have a significant protective effect via enhancing enzymatic and non-enzymatic antioxidant ability, non-specific immune response and reduced oxidative stress effect (Heshmati et al., 2018; Lin et al., 2019; Hosain and Liangyi, 2020; Rohani et al., 2021; Mounir et al., 2022). Note: The arrow indicates the various functions of probiotics, prebiotics and synbiotics in protecting aquatic species especially fish.
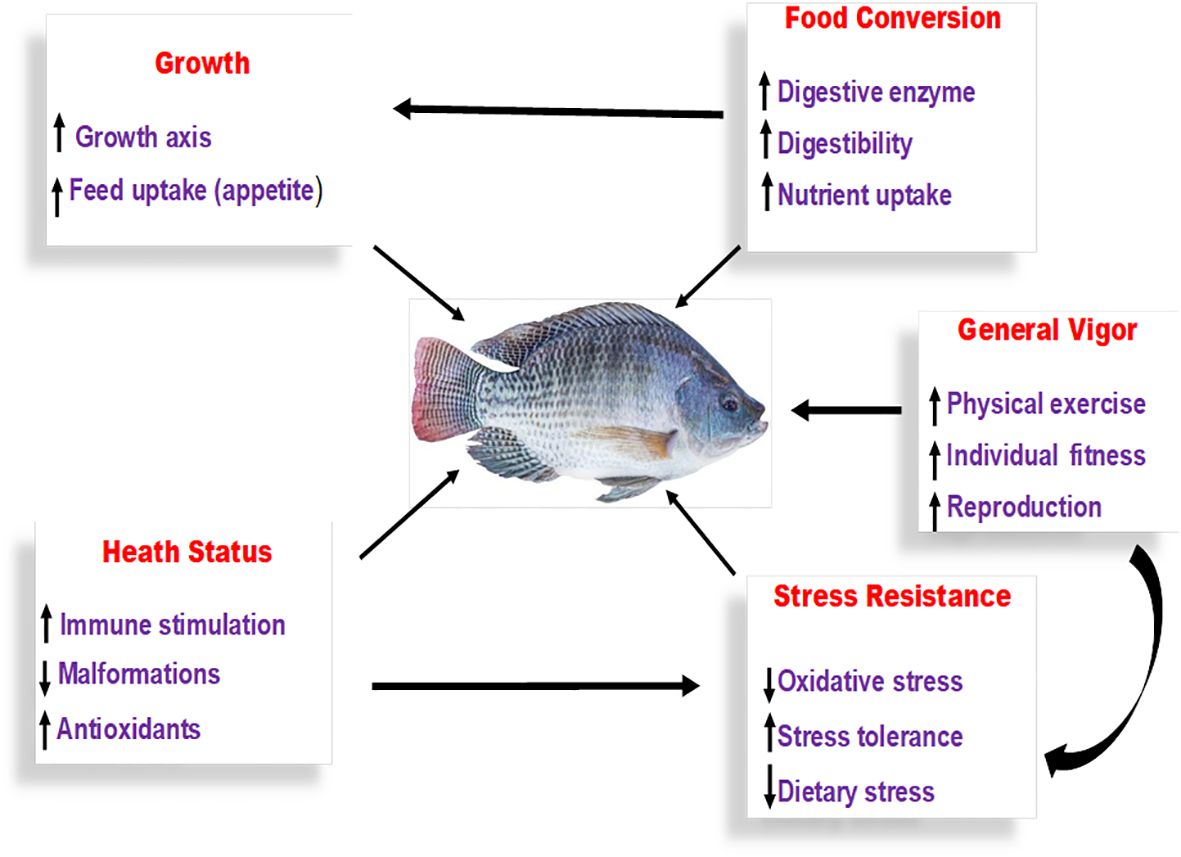
Figure 2 Method of exploit of probiotics, prebiotics and synbiotics. Probiotics function through various mechanisms to benefit fish, such as promoting growth and appetite, optimizing feed conversion through the secretion of microbial enzymes for digestion, enhancing the nutritional value of feed by augmenting both macro and micronutrient absorption, boosting stress resilience, and bolstering overall health by activating the fish’s immune response and enrichment of overall vigor (Wuertz et al., 2021).
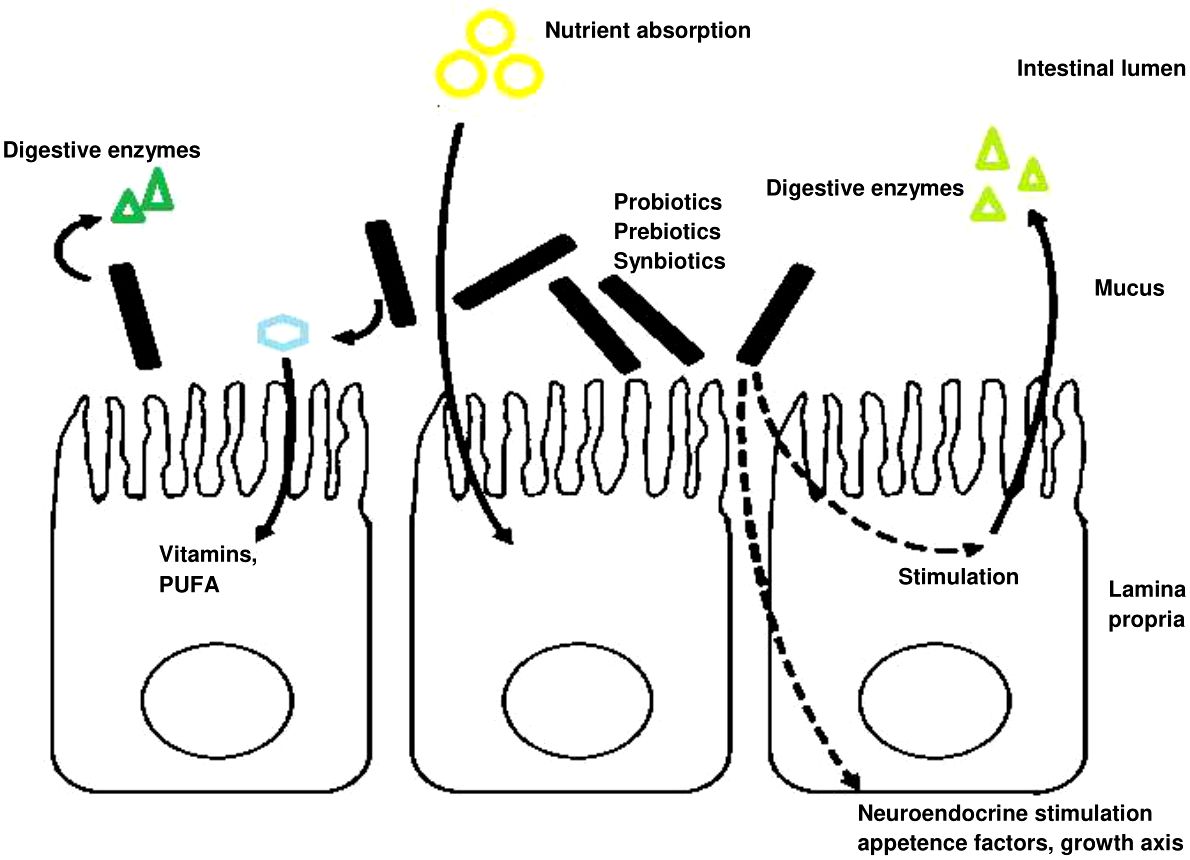
Figure 3 Nutrition- and growth-related effects (modes of action) of probiotics in the gastrointestinal tract (GIT). Probiotics exert various effects within the gastrointestinal tract (GIT) that influence nutrition and growth. These effects encompass direct actions, such as the release of digestive enzymes and the facilitation of (micro) nutrient absorption, including cofactors, vitamins, and polyunsaturated fatty acids. Additionally, probiotics indirectly enhance nutrient uptake and absorption while stimulating enzyme secretion. Furthermore, they contribute to neuroendocrine modulation, promoting appetite and fostering growth (Wuertz et al., 2021).
Amylases are enzymes that break down starch into smaller carbohydrates like glucose, maltose, and maltotriose, making them available for absorption by the host (Mardani et al., 2018). These enzymes are found throughout the intestinal tract of fish, with their activity varying depending on the fish species. Research indicates that the modulation of amylase activity is influenced by the type of food and supplements, as well as the administration of probiotics (Murashita et al., 2018). Studies have shown that dietary supplementation with probiotics, such as PrimaLac®, can significantly increase (5.45% compared to the control) amylase activity in Caspian white fish (Rutilus frisii kutum), leading to improved feed utilization and growth performance (Mirghaed et al., 2018). Probiotics, whether used individually or in conjunction with other bacterial strains, have been shown to increase the levels of amylase activity in various types of fish. For example, tilapia (Oreochromis niloticus) fed with a diet supplemented with B. subtilis HAINUP40 exhibited significantly higher (185.71%) amylase activity in the digestive tract (Liu et al., 2017). Similar positive effects on amylase activity and growth were reported in catfish and shrimp when supplemented with specific probiotics. The positive impact of probiotics on amylase activity extends to various fish species, including Nile tilapia (16.46%, 44.02%, and 37.03% respectively when fed with supplemented with Bacillus subtilis NIOFSD017, Lactobacillus plantarum NIOFSD018, and S. cerevisiae) (Essa et al., 2010), catfish (Clarias sp.) (63.64% increased when supplemented with Bacillus megaterium PTB 1.4) (Afrilasari and Meryandini, 2016), shrimp (about 44.74% increase when fed with supplanted B. subtilis) (Wang, 2007). Additionally, the use of specific probiotics like Bacillus amyloliquefaciens, B. cereus, and B. subtilis has been associated with substantial increases (approximately 170.00%, 265.00%, and 62.50%) in amylase activity in catfish. In prawn Macrobrachium rosenbergii, the supplementation of Clostridium butyricum in the diet led to a significant increase (55.56%) in digestive amylase activities (Sumon et al., 2018).
Furthermore, a combination of photosynthetic bacteria and Bacillus sp. was found to enhance (approximately 44.74%) amylase activity in shrimp (Penaeus vannamei). Probiotic supplementation in a study by Sankar et al. (2017), using Micrococcus MCCB 104 and Bacillus MCCB 101, was linked to increased (approximately 9.41% and 61.18% correspondingly) intestinal amylase in Mozambique tilapia (Oreochromis mossambicus). Carnivorous fish, specifically snakehead (Channa striata) fingerlings, showed higher amylase specific activities when fed with Lactobacillus acidophilus-supplemented diets compared to prebiotic-supplemented diets (Munir et al., 2016). Interestingly, there were instances where amylase activities increased in fish infected with Aphanomyces invadans when fed with Saccharomyces cerevisiae (Devi et al., 2019). European seabass (Dicentrarchus labrax) also exhibited increased amylase activities when fed with a diet containing Bacillus subtilis, Lactococcus lactis, and Saccharomyces cerevisiae (Tovar et al., 2002). However, the supplementation of Debaryomyces hansenii CBS 8339 led to a reduction in amylase activity in European seabass compared to the control diet (Tovar-Ramírez et al., 2004). These findings collectively highlight the significant role of various factors, including probiotics and specific bacteria, in modulating amylase activity and influencing the digestive performance of different fish species.
The significance of proteases in digestion lies in their ability to break down protein in food by hydrolyzing peptide bonds, releasing essential amino acids for the body (Naidu, 2011). The addition of probiotics has been demonstrated to boost the digestibility of proteins in fish, leading to enhanced performance in those fed with probiotic-included diets (Allameh et al., 2017). Studies have reported increased protease activity in various fish species when fed diets supplemented with specific probiotics. For instance, Wang (2007) found a significant 78.38% increase in the protease activity of shrimp (Penaeus vannamei) when fed a diet supplemented with Bacillus sp. compared to the control. Similarly, Wang (2011) observed an 18.18% increase in protease activity in grass carp (Ctenopharyngodon idella) when supplemented with Bacillus coagulans. Essa et al. (2010) documented elevated digestive protease activity in Nile tilapia fed probiotics Lactobacillus plantarum NIOFSD018 (76.92% increase) and Bacillus subtilis NIOFSD017 (73.08% increase). Bacillus megaterium PTB 1.4 was reported to boost catfish protease activity by 245.00% (Afrilasari and Meryandini, 2016), and Mirghaed et al. (2018) observed a similar effect in Caspian white fish with PrimaLac® supplementation.
In Nile tilapia, Liu et al. (2017) noted a 133.33% rise in protease activity within the digestive tract following an 8-week regimen of a diet enriched with B. subtilis HAINUP40. Prawn (Macrobrachium rosenbergii) juveniles treated with the probiotic Clostridium butyricum demonstrated an 86.67% increase in digestive protease activity compared to control groups (Sumon et al., 2018). Similarly, Wang and Xu (2006) observed heightened digestive protease activities in C. carpio juveniles upon supplementation with a Bacillus sp. probiotic diet. Experiments conducted by Zhou et al. (2009) revealed that the probiotic B. coagulans SC8168, when introduced as a water additive, significantly elevated protease activities in shrimp larvae. B. subtilis consistently elicited increased digestive protease activity in shrimp, as evidenced by studies from Liu et al. (2009), Wang (2007), and Zokaeifar et al. (2012). Reda et al. (2018) noted a significant enhancement in protease activity in Clarias gariepinus when their diets were enriched with native strains of B. amyloliquefaciens, B. cereus, and B. subtilis. In contrast, Wang et al. (2015) documented a decrease of 0.78% in protease activity in sea cucumber (Apostichopus japonicus) following treatment with probiotic Rhodotorula benthica D30.
Lipases play a crucial role in catalyzing various biochemical reactions, including the hydrolysis of triglycerides into glycerol and free fatty acids, as well as the hydrolysis, transesterification, and synthesis of esters (Thakur, 2012). These enzymes exhibit enantioselective properties. Widely distributed in bacteria, plant, and animal tissues, lipases are renowned for their involvement in fat digestion in the small intestine, acting as a class of digestive enzymes (Bruno, 2010). Numerous studies have explored the impact of probiotics on the lipase activity of various fish species. For instance, Tovar-Ramírez et al. (2004) investigated the modulation of digestive enzymes in European sea bass larvae using the live yeast Debaryomyces hansenii, observing increased survival, growth parameters, and a substantial rise in lipase activity (approximately 833.33% higher than the control). In juvenile C. carpio, Yanbo et al. (2006) observed a notable increase in lipase activity after the application of Bacillus sp. Likewise, the incorporation of Lactobacillus spp. into the diet of gilthead sea bream larvae resulted in elevated lipase activity, approximately 1.69% higher than the control group (Suzer et al., 2008). Additionally, in shrimp larvae, the presence of B. coagulans SC8168 not only provided various benefits but also led to a significant increase in lipase activity, approximately 20.00% higher (Zhou et al., 2009).
In addition, Sankar and colleagues (2017) observed elevated levels of intestinal lipase enzymes in O. mossambicus following dietary supplementation with Micrococcus MCCB 104 and Bacillus MCCB 101, showing approximately a 26.32% and 7.02% increase, respectively. Similarly, Wang (2011) demonstrated a significant enhancement in lipase activity in Labeo rohita when the diet was enriched with Bacillus coagulans. Furthermore, Essa et al. (2010) documented increased digestive lipase activity in Nile tilapia after administration of the probiotics Bacillus subtilis NIOFSD017 and Lactobacillus plantarum NIOFSD018. Additionally, Wu et al. (2012) observed a substantial increase (approximately 65.22%) in lipase activity in grass carp (Ctenopharyngodon idella) following dietary administration of Bacillus subtilis compared to the control treatment. In summary, probiotics exhibit positive effects on the activity of digestive enzymes, including amylases, proteases, and lipases, in cultured fish. Fish are poikilothermic animals, meaning their body temperature fluctuates with the environment (Frick et al., 2018). Optimal digestive function is critical for their metabolic processes, especially in varied environmental conditions. These effects contribute to enhanced nutrient absorption, feed efficiency, and overall growth performance. However, outcomes may vary depending on the specific probiotic strains and fish species involved. A healthy gut microbiota contributes to optimal growth performance in fish. Probiotics have been shown to mitigate the adverse effects of stress on fish by promoting a stable gut environment (Amenyogbe et al., 2022c). A balanced microbial community in the fish gut can contribute to the breakdown of organic matter, helping to maintain good water quality in aquaculture systems. In fact, using probiotics, in fish farming is a promising strategy to enhance the health and productivity of farmed fish (Amenyogbe, 2023). It involves manipulating the gut microbiota to create a favorable environment that supports the well-being of the fish and minimizes the risk of diseases. Probiotics can produce substances like organic acids, bacteriocins, and hydrogen peroxide, inhibiting harmful bacteria growth (Tremaroli and Bäckhed, 2012; Falcinelli et al., 2018; Liu et al., 2021). Probiotics can improve nutrient absorption by enhancing the intestinal mucosa’s surface area (Figure 1) (Heshmati et al., 2018; Lin et al., 2019; Hosain and Liangyi, 2020; Rohani et al., 2021; Mounir et al., 2022). The choice of probiotic strains is critical and should be species-specific. Strains should be resistant to environmental stressors, bile salts, and gastric acid to survive the passage through the digestive tract (Amenyogbe et al., 2021). Encapsulation of probiotics in feed helps protect them from environmental conditions, ensuring their viability until they reach the intestines (Simo´n et al., 2021). Studies have shown that fish supplemented with probiotics exhibit improved growth rates, feed conversion efficiency, and biomass production (Table 1) (Panigrahi and Azad, 2007; Muñoz-Atienza et al., 2013; Chen et al., 2019; Assan et al., 2022). Probiotics contribute to enhanced nutrient utilization, leading to better growth outcomes. Probiotics bolster the immune system by stimulating the production of immune-related molecules (Zapata and Lara-Flores, 2012; Xu et al., 2013; Amenyogbe et al., 2020; Amenyogbe, 2023). Probiotics can contribute to the sustainability of aquaculture by reducing the need for antibiotics and other chemical treatments (Amenyogbe et al., 2021). Challenges include the need for strain-specific research, optimizing delivery methods, and understanding the interactions between probiotics and the fish microbiome (Amenyogbe, 2023). The use of probiotics in fish digestive health is a promising avenue in aquaculture. Through their diverse mechanisms of action, probiotics contribute to improved digestion and growth, disease prevention, and environmental sustainability. Continued research will provide further insights into probiotics’ specific applications and benefits in different fish species and aquaculture systems. As research in this field continues (Supplementary 4), further insights into specific strains, dosages, and application methods will likely lead to more efficient and sustainable aquaculture practices.
Prebiotics, on the other hand, are non-digestible compounds that stimulate the growth and activity of beneficial microorganisms, such as probiotics, in the gastrointestinal tract. In fish farming, prebiotics are often carbohydrates like oligosaccharides and fructooligosaccharides (Assan et al., 2022). Over the last three decades, the conventional use of antibiotics in aquaculture has faced criticism due to concerns about the emergence of antibiotic-resistant bacteria, the presence of antibiotic residues in aquatic food, disruption of microbial ecosystems in aquacultural environments, and the potential suppression of the immune systems in aquatic animals (Ringø et al., 2010). As an alternative approach to antibiotics, prebiotics have garnered significant attention in the field of aquaculture. Ingredients rich in dietary fiber, such as plant-based materials (cellulose, hemicellulose), can serve as prebiotics for fish. Fiber promotes the growth of fiber-degrading bacteria in the gut, enhancing the overall microbial diversity. Oligosaccharides, including fructooligosaccharides (FOS) and inulin, are commonly used prebiotics in fish diets (Ganguly et al., 2013; Ringø et al., 2014; Di Gioia and Biavati, 2018). These compounds resist digestion in the upper gastrointestinal tract and reach the lower intestine, where they selectively stimulate the growth of beneficial bacteria (Ganguly et al., 2013; Ringø et al., 2014). Mannan-Oligosaccharides (MOS), derived from yeast cell walls, have been recognized for their prebiotic properties in fish (Song et al., 2014; Akhter et al., 2015; Di Gioia and Biavati, 2018). They can bind to pathogenic bacteria, preventing their attachment to the gut epithelium and facilitating their removal from the digestive system.Mannan oligosaccharides are structurally complex carbohydrates derived from yeast cell walls, primarily composed of mannose (Mata-Sotres et al., 2016). The addition of prebiotics, particularly mannan, to sturgeon feeds emerges as a viable alternative for enhancing feed efficiency and promoting sturgeon health (Pryor et al., 2003; Akrami et al., 2009). The effects of mannan prebiotics have been extensively studied in various aquatic species, including tilapia fingerlings (Hisano et al., 2007), channel catfish (Welker et al., 2007), juvenile Nile tilapia (Sado et al., 2008), rohu (Andrews et al., 2009), Japanese flounder (Ye et al., 2011), and grass carp (Shaker Khoshroudi, 2011). These compounds serve as a food source for beneficial bacteria, promoting their proliferation and enhancing their positive effects on the host fish. By fostering the growth of beneficial bacteria, prebiotics contribute to a more stable and resilient gut microbiota in fish Assan et al., 2022). This, in turn, can improve the fish’s overall health and disease resistance (Mata-Sotres et al., 2016; Assan et al., 2022).
The role of prebiotics in fish digestive health is a subject of growing interest in aquaculture research. Prebiotics are non-digestible compounds that selectively promote the growth and activity of beneficial microorganisms in the gastrointestinal tract, ultimately improving the overall health and performance of the host organism (Gatesoupe, 2010; Xu et al., 2022). In the context of fish, the digestive system is a crucial aspect of their well-being, as it directly influences nutrient absorption, disease resistance, and growth. In this study, we explore the importance of prebiotics in fish digestive health, their sources, mechanisms of action, and potential applications in aquaculture. Fish require a balanced intake of proteins, fats, carbohydrates, vitamins, and minerals for growth and development (Roberfroid, 2007). Prebiotics play a crucial role in enhancing nutrient absorption by promoting the growth of beneficial bacteria that aid digestion and breakdown of complex nutrients (Hanley et al., 1995; Geraylou et al., 2013). Polysaccharides, synonymous with prebiotics, refer to non-digestible feed ingredients that stimulate the growth of beneficial microbiota in the gastrointestinal tract (Song et al., 2014). Numerous studies in aquaculture have illustrated this phenomenon. For instance, Astragalus polysaccharides were shown to enhance the activity of digestive enzymes in O. niloticus (Yu et al., 2022). Similarly, Sparus aurata exhibited increased digestive enzyme activity when fed fructooligosaccharides (Guerreiro et al., 2016). In the case of Channa striata, feeding with β-glucan, galactose-oligosaccharide, and mannaoligosaccharide demonstrated a similar positive effect (Munir et al., 2018). Additionally, Diplodus sargus displayed improved digestive enzyme activity when fed a diet supplemented with xylooligosaccharides, small chain fructooligosaccharides, and galactoseoligosaccharides, respectively (Guerreiro et al., 2016).
A well-balanced microbial community helps in preventing the colonization of harmful pathogens (Landers et al., 2012; Tzivara et al., 2013; Amenyogbe et al., 2021; Amenyogbe et al., 2022c). Aquaculture environments can expose fish to various stressors, such as water quality, handling, or transportation changes (Hoseinifar et al., 2010; Daniels and Hoseinifar, 2014). Stress can negatively impact the digestive system. Prebiotics have been shown to mitigate the effects of stress on fish by stabilizing the gut microbiota and promoting the production of bioactive compounds that positively influence stress response (Gatesoupe, 2010; Di Gioia and Biavati, 2018; Xu et al., 2022). The selective stimulation of prebiotics helps in maintaining a balanced microbial community, contributing to a healthier gut environment (Gatesoupe, 2010; Xu et al., 2022). Prebiotics promote competitive exclusion by providing a substrate for beneficial bacteria to outcompete pathogenic microorganisms for resources and attachment sites in the gut (Xu et al., 2022).
Studies have demonstrated that the inclusion of prebiotics in fish diets can lead to improved growth rates, feed conversion efficiency, and overall performance (Hoseinifar et al., 2011a, Hoseinifar et al., 2011b; Geraylou et al., 2013; Xu et al., 2022; Gatesoupe, 2010; Ta’ati et al., 2011; Ringø et al., 2010; Mohajer Esterabadi et al., 2010);. Prebiotics have shown promise in preventing and controlling fish diseases by enhancing the immune response and inhibiting the growth of pathogenic bacteria. By improving nutrient utilization and reducing the environmental impact of aquaculture operations, prebiotics contribute to the sustainability of fish farming practices (Geraylou et al., 2013; Xu et al., 2022).The effectiveness of prebiotics can vary among fish species, highlighting the need for species-specific research to optimize prebiotic supplementation in different aquaculture systems. Determining the optimal dosage and formulation of prebiotics in fish diets is a complex task, as it depends on factors such as fish species, age, and environmental conditions (Gatesoupe, 2010; Di Gioia and Biavati, 2018; Xu et al., 2022). The interactions between prebiotics and other dietary components need further exploration to ensure a balanced and effective nutritional profile for fish.
As aquaculture continues to evolve, optimizing the use of prebiotics offers a promising avenue for enhancing the sustainability and productivity of fish farming operations. Further research in this field will further refine our understanding of the specific requirements for different fish species and contribute to developing tailored aquaculture prebiotic strategies.
Synbiotics refer to the combination of probiotics and prebiotics, creating a synergistic effect to enhance the health-promoting benefits in the host organism (Mohapatra et al., 2013). In fish farming, the use of synbiotics aims to maximize the colonization and activity of probiotics in the gut by providing a suitable nutritional environment through prebiotics. The combination of probiotics and prebiotics can have several advantages, such as improved survival rates of probiotics during storage and transportation, increased adherence of probiotics to the gut lining, and sustained activity of beneficial microorganisms in the fish gastrointestinal tract.
4 Interconnection of digestive enzymes, oxidative stress, and significance of antioxidant defense in maintaining fish health
All aerobic organisms, including those in aquatic environments, require molecular oxygen (O2) for essential metabolic processes and energy production (Hoseinifar et al., 2021). However, the oxygen dependence poses a risk of oxidative stress, creating a paradox known as the ‘aerobic-life paradox’ or ‘oxygen paradox’ (Ahmad, 1995; Davies, 1995; Hoseinifar et al., 2021). Oxygen can exist in atomic form (O), a free radical, a free bi-radical (Davies, 1995). Reactive oxygen species (ROS) are generated during partial oxygen reduction, with superoxide anion (O2-), hydroxyl radical (OH), and hydrogen peroxide (H2O2) being the most physiologically active (Schieber and Chande, 2014; Halliwell and Gutteridge, 2015; Hoseinifar et al., 2021).
Superoxide dismutase (SOD) eliminates superoxide by converting it into oxygen and hydrogen peroxide. Hydroxyl radicals have a short half-life and are highly reactive, causing significant harm. Hydrogen peroxide, produced during O2 reduction, can be partially eliminated by enzymes like catalase (CAT) and glutathione peroxidase (GPx) (Halliwell and Gutteridge, 2015; Li et al., 2016; Hoseinifar et al., 2021). ROS, produced through normal metabolism, play essential roles in physiological functions, but excessive concentrations lead to cellular damage, including lipid peroxidation, protein modifications, and DNA alterations (Schieber and Chande, 2014; Dong et al., 2017).
To maintain balance, organisms utilize antioxidant mechanisms from endogenous and exogenous sources (Wang et al., 2013; Franco and Martınez-Pinilla, 2017; Hoseinifar et al., 2021). Fish and shellfish possess adapted enzymatic systems, including SOD, CAT, and GPx, along with lower-molecular-weight antioxidants such as vitamins like vitamin E, K and C, carotenoids, amino acids, and peptides (Birnie-Gauvin et al., 2017; Prego-Faraldo et al., 2017; Biller and Takahashi, 2018; Ji et al., 2018; Parolini et al., 2019; Hoseinifar et al., 2021). The antioxidant system helps prevent or repair oxidative stress effects (Li et al., 2016; Cao et al., 2019; Devi et al., 2019; Parolini et al., 2019). Enzymes like SOD and CAT play crucial roles in eliminating ROS, while non-enzymatic antioxidants like vitamin C and vitamin E act as powerful scavengers and reducers, contributing to the overall antioxidant defense system in fish and shellfish (Narra et al., 2015; Cheng et al., 2018; Hossain et al., 2018. The intricate balance between ROS formation and elimination is crucial for maintaining cellular functions and preventing oxidative stress-related damage.
Proper digestion ensures optimal nutrient absorption, providing essential building blocks for antioxidant synthesis. Efficient lipid and carbohydrate digestion contribute to energy production, which is essential for antioxidant defense mechanisms. Environmental stressors affecting digestion can contribute to oxidative stress. Antioxidant defense helps mitigate the adverse effects of stress (Halliwell and Gutteridge, 2015; Li et al., 2016; Yang et al., 2018; Hoseinifar et al., 2021). Antioxidants play a role in immune function, aiding the defense against pathogens and reducing the likelihood of oxidative damage during infections. The interplay between digestive enzymes, oxidative stress, and antioxidant defense is intricate and vital for fish health (Yang et al., 2018). A balanced diet, optimal digestion, and a robust antioxidant defense system contribute to fish’s overall well-being and resilience in both natural and aquaculture settings.
5 Evidence of prebiotics influencing digestive enzyme activity in fish
The influence of prebiotics on digestive enzyme activity in fish has been a subject of increasing interest in aquaculture research. In the context of fish nutrition, understanding how prebiotics impact digestive enzyme activity is crucial for optimizing feed formulations and promoting efficient nutrient utilization. The gastrointestinal tract of fish contains various enzymes such as proteases, lipases, and carbohydrases, which are responsible for the digestion of proteins, lipids, and carbohydrates, respectively (Assan et al., 2022). Prebiotics, acting as substrates for beneficial gut bacteria, have been shown to influence the gut environment and subsequently impact the activity of these digestive enzymes (Ta’ati et al., 2011; Hoseinifar et al., 2011a, Hoseinifar et al., 2011b; Geraylou et al., 2013; Munir et al., 2016; Xu et al., 2022).
Prebiotics, often in the form of non-digestible carbohydrates like fructooligosaccharides (FOS) and inulin, serve as substrates for beneficial gut bacteria (Bartlett, 1937). Prebiotics contribute to the maintenance of a balanced microbial community in the gut. A well-balanced microbiota is essential for the production of certain enzymes that aid in the digestion and absorption of nutrients (Amenyogbe et al., 2022a, Amenyogbe et al., 2022b, Amenyogbe et al., 2022c). The presence of beneficial bacteria can positively influence the expression and activity of digestive enzymes through complex interactions within the gut microbiome (Amenyogbe et al., 2022a, Amenyogbe et al., 2022b, Amenyogbe et al., 2022c). Several studies have provided evidence supporting the influence of prebiotics on digestive enzyme activity in fish: Research conducted on various fish species, including tilapia and rainbow trout, has shown that dietary supplementation with prebiotics can enhance lipase activity in the intestinal tract (Hoseinifar et al., 2011a; Mehrabi et al., 2012; Geraylou et al., 2013; Munir et al., 2016; Xu et al., 2022; Rodriguez-Estrada et al., 2009; Ta’ati et al., 2011). The increased lipase activity is attributed to the modulation of gut microbiota and the production of microbial metabolites that positively affect enzyme secretion. Studies on carnivorous fish, such as salmon, have demonstrated that prebiotic supplementation can influence protease activity in the stomach and intestine (Jeney and Jeney, 2002; Mahious et al., 2006; Aly et al., 2008; Rodriguez-Estrada et al., 2009; Mohajer Esterabadi et al., 2010; Ai et al., 2011; Geng et al., 2011; Ta’ati et al., 2011; Ye et al., 2011; Hoseinifar et al., 2011a, Hoseinifar et al., 2011b; Mehrabi et al., 2012; Abid et al., 2013; Cerezuela et al., 2013; Munir et al., 2016). The stimulation of protease activity is often associated with changes in the gut microbiota composition and the release of microbial enzymes (Munir et al., 2016). Prebiotics, particularly those with a carbohydrate-based structure, have been linked to enhanced carbohydrase activity in fish. This effect is often observed in herbivorous and omnivorous species (Abdel-Tawwab et al., 2008; Munir et al., 2016). Improved carbohydrase activity is thought to result from the fermentation of prebiotics, leading to the production of SCFAs that stimulate the secretion of digestive enzymes (Dhanasiri et al., 2023).
Understanding the impact of prebiotics on digestive enzyme activity in fish has practical implications for aquaculture. Enhanced digestive enzyme activity in farmed fish, facilitated by prebiotics, can improve nutrient utilization, leading to better growth and feed conversion ratios while promoting a balanced gut microbiota and disease resistance. This contributes to sustainable aquaculture by reducing nutrient excretion into the water. Prebiotics influence enzyme activity through microbial fermentation and gut microbiota modulation, offering potential for optimized diets to enhance growth, disease resistance, and environmental sustainability in aquaculture. Further research is required to refine recommendations for different fish species.
6 Probiotics and synbiotics effects on digestive enzymes in fish
Probiotics are commonly associated with promoting gut health by modulating the composition and function of the gut microbiota (Yanbo et al., 2006; Sumon et al., 2018). Probiotics can influence digestive enzymes through various mechanisms (Verschuere et al., 2000; Suzer et al., 2008; Assan et al., 2022). Probiotic strains such as Lactobacillus and Bifidobacterium can enhance lactase activity. Lactase is the enzyme responsible for breaking down lactose, the sugar found in milk (Mardani et al., 2018). Increased lactase activity can be beneficial for individuals with lactose intolerance, allowing for better digestion of dairy products. Some probiotics produce proteases, enzymes that break down proteins (Naidu, 2011). These proteases may complement the host’s digestive enzymes, aiding in the breakdown of dietary proteins into smaller peptides and amino acids (Afrilasari and Meryandini, 2016; Allameh et al., 2017; Mirghaed et al., 2018). Probiotics may influence amylase activity, which is responsible for breaking down carbohydrates into simpler sugars (Murashita et al., 2015; Mardani et al., 2018; Murashita et al., 2018). By modulating carbohydrate metabolism, probiotics can impact the availability of nutrients for both the host and the gut microbiota. Probiotics, such as certain strains of Lactobacillus and Bifidobacterium, may possess bile salt hydrolase activity (Liu et al., 2017). This enzyme plays a role in bile salt metabolism and can impact the digestion and absorption of dietary fats.
While prebiotics are not enzymes themselves, prebiotics indirectly influence digestive enzymes through their effects on the gut microbiota SCFAs, can influence the activity of various digestive enzymes, including amylases and proteases (Akrami et al., 2015). The fermentation of prebiotics by gut bacteria can alter the pH of the gut environment (Ganguly et al., 2013; Hoseinifar et al., 2014; Song et al., 2014; Dawood and Koshio, 2016). Changes in pH can influence the activity of digestive enzymes, creating an environment that may be more or less conducive to their function (Merrifield et al., 2014; Feckaninova et al., 2017; Ringø et al., 2018).
The interaction between probiotics and prebiotics can profoundly impact digestive enzymes; Prebiotics can serve as a source of energy for probiotics, promoting their growth and survival in the gut (Dawood and Koshio, 2016). This can enhance the overall impact of probiotics on digestive enzyme activities. Synbiotics work together to stimulate the growth of beneficial bacteria selectively (Dawood and Koshio, 2016). This selective stimulation can influence the overall balance of the gut microbiota, potentially affecting the production and activity of various digestive enzymes. Probiotics, prebiotics, and synbiotics can exert a multifaceted influence on digestive enzymes (Ganguly et al., 2013; Hoseinifar et al., 2014; Song et al., 2014; Dehaghani et al., 2015; Dawood and Koshio, 2016). These interactions contribute to the overall maintenance of gut health and highlight the intricate relationship between the microbiota and host digestive processes. The field of research in this area continues to evolve, providing deeper insights into the specific mechanisms by which these components modulate digestive enzyme activities.
Different fish species have unique digestive systems and nutritional requirements. Tailoring synbiotic formulations to specific species ensures optimal results. Synbiotics contribute to maintaining a healthy microbial balance in the gut and surrounding water, thus positively influencing overall water quality (Di Gioia and Biavati, 2018). Identifying the most effective probiotic strains for different fish species remains a challenge. Continued research is needed to understand the specific requirements of diverse aquatic organisms. Various environmental factors, such as temperature and water quality, influence aquaculture systems. Future developments in synbiotic applications should consider these factors for consistent and reliable outcomes. Synbiotics represent a promising avenue for promoting digestive health in fish. By combining the strengths of probiotics and prebiotics, aquaculturists can optimize the gut microbiota, leading to improved nutrient utilization, growth, and overall resilience against diseases. Further research and development in this field will uncover new insights and refine the application of synbiotics in the dynamic context of fish farming.
7 Probiotics, prebiotics, and synbiotics impact on oxidative stress in fish
Studies have shown that probiotics can positively influence the antioxidant defense system in fish (Van Doan et al., 2020; Hoseinifar et al., 2021). These microorganisms can enhance the activity of antioxidant enzymes like superoxide dismutase (SOD), catalase (CAT), and glutathione peroxidase (GPx) (Supplementary Table 1; Figure 1) (Lin et al., 2019; Hosain and Liangyi, 2020; Hoseinifar et al., 2021; Rohani et al., 2021; Mounir et al., 2022; Abdel-Latif et al., 2023; Wang et al., 2019; Dawood et al., 2018; Heshmati et al., 2018). By doing so, probiotics contribute to the elimination of reactive oxygen species (ROS) and reduce oxidative stress in fish tissues (Dawood et al., 2018; Ringø et al., 2018; Hoseinifar et al., 2021). Additionally, probiotics may help maintain the balance of gut microbiota, promoting a healthy gut environment. A well-balanced gut microbiota is crucial for the absorption of nutrients and can indirectly impact the antioxidant status of the fish. Prebiotics indirectly affect oxidative stress by supporting the growth of probiotics (Dawood et al., 2018; Abdel-Latif et al., 2023). By providing a favorable environment for beneficial bacteria in the gut, prebiotics contribute to the maintenance of a healthy gut microbiota. This, in turn, helps in the prevention of oxidative stress by ensuring optimal nutrient absorption and gut function.
The combination of probiotics and prebiotics in synbiotics often results in enhanced effects on oxidative stress compared to individual administration (Supplementary Table 2) (Hoseinifar et al., 2021; Mohammadi et al., 2021; Puvanasundram et al., 2021; Wang et al., 2019; Dawood et al., 2018; Ringø et al., 2018). Synbiotics can promote the survival and activity of probiotics in the gut, creating a more resilient and diverse microbial community (Lin and Yen, 1999). This enhanced gut health can lead to improved antioxidant defense mechanisms and a reduced risk of oxidative stress in fish (Dawood et al., 2018; Ringø et al., 2018; Hoseinifar et al., 2021; Abdel-Latif et al., 2023).
Different strains of probiotics may have varying effects on oxidative stress (Dawood et al., 2018; Ringø et al., 2018). Understanding the specific strains and their interactions is crucial for designing effective probiotic formulations. The efficacy of probiotics, prebiotics, and synbiotics in mitigating oxidative stress can be influenced by the dosage and duration of administration (Ahire et al., 2013). Optimal levels need to be determined through careful experimentation. Responses to probiotics and prebiotics can vary among fish species (Ringø et al., 2016; Dawood et al., 2016a; Li et al., 2019; Van Doan et al., 2020). Research should consider the specific requirements and characteristics of the target species. Water quality, temperature, and other environmental factors can influence the effectiveness of these supplements. A holistic approach that considers both biological and environmental factors is essential. Probiotics, prebiotics, and synbiotics play pivotal roles in influencing oxidative stress in fish through their effects on gut health and the antioxidant defense system (Supplementary Table 2). Ongoing research in this field is vital for optimizing formulations and application strategies to enhance the health and well-being of cultured fish species.
8 The relationship between probiotics, prebiotics, synbiotics, and antioxidant defenses in fish
The relationship between probiotics, prebiotics, synbiotics, and antioxidant defenses in fish is a complex and fascinating area of research. In this review, we broke down each component and discussed their roles in enhancing antioxidant defenses in fish. Several studies suggest that probiotics positively influence antioxidant defenses in fish through various mechanisms (Supplementary Tables 1–3) (Wang et al., 2019; Dawood et al., 2018; Gobi et al., 2018; Yi et al., 2018). Probiotics have been shown to possess free radical scavenging abilities, helping to neutralize reactive oxygen species (ROS) in fish (Hussain et al., 2003; Brzozowski et al., 2006; Gomez-Guzman et al., 2015; Trinder et al., 2015; Salem et al., 2018). This reduces oxidative stress, a critical factor in cellular damage. The immune system and antioxidant defenses are closely linked. Probiotics can modulate the immune response in fish, leading to a more efficient defense against oxidative stress.
The connection between prebiotics and antioxidant defenses in fish can be understood through the following points; by promoting the growth of beneficial gut bacteria, prebiotics indirectly contribute to antioxidant defenses (Gibson, 2004; Guerreiro et al., 2016). The enhanced microbial activity can lead to the production of bioactive compounds that have antioxidant properties (Hoseinifar et al., 2017a, b). Prebiotics often exhibit anti-inflammatory effects, which can reduce oxidative stress (Gibson, 2004; Zhang et al., 2014; Guerreiro et al., 2016). Inflammation is closely linked to the generation of free radicals, and by mitigating inflammation, prebiotics contribute to improved antioxidant status.
Synbiotics refer to a combination of probiotics and prebiotics, where prebiotics serve as the fuel for probiotic microorganisms (Huynh et al., 2017; Abdel-Latif et al., 2023; Hoseinifar et al., 2015a, b). The synergistic effects of probiotics and prebiotics provide additional advantages for antioxidant defenses in fish; Prebiotics act as a substrate for probiotics, promoting their growth and survival in the gut (Rurangwa et al., 2009; Zhang et al., 2013; Llewellyn et al., 2014). This ensures a sustained and effective presence of probiotics, leading to prolonged antioxidant benefits. The combination of probiotics and prebiotics often results in a more comprehensive approach to enhancing antioxidant defenses (Kumar et al., 2018; Devi et al., 2019; Ashouri et al., 2020; Abdel-Latif et al., 2023; Hoseinifar et al., 2017a, b; Zhang et al., 2014). This can include both direct antioxidant activity and indirect effects through modulation of the gut microbiota and immune system (Kumar et al., 2018; Devi et al., 2019; Ashouri et al., 2020).
In fact, probiotics, prebiotics, and synbiotics play pivotal roles in enhancing antioxidant defenses in fish through various mechanisms. These include direct scavenging of free radicals, stimulating antioxidant enzyme production, modulation of immune responses, and promoting beneficial gut microbiota. Integrating these components in aquaculture practices holds promise for improving fish’s overall health and stress resistance, contributing to sustainable and efficient aquaculture systems. However, it is important to note that the specific effects can vary depending on the fish species, environmental conditions, and the particular strains of probiotics used. Ongoing research in this field will likely provide more insights and refine the application of these strategies in aquaculture.
9 Factors such as fish species, diet, environmental conditions, and dosage affect the efficacy of probiotics, prebiotics, and synbiotics
The efficacy of probiotics, prebiotics, and synbiotics in aquaculture is influenced by a variety of factors, including fish species, diet, environmental conditions, and dosage (Cerezuela et al., 2013; Zoumpopoulou et al., 2018; Cavalcante et al., 2020). The activity of digestive enzymes like amylase, protease, and lipase in fish is influenced by factors such as fish species, age, dietary enzymes, and food habits (Falc´on-Hidalgo et al., 2011; Assan et al., 2022). Host-produced digestive enzymes are regulated in response to nutrient intake (Gisbert et al., 2018). For example, feeding rainbow trout (Oncorhynchus mykiss) diets deficient in fish oil led to decreased lipase activity (Ducasse-Cabanot et al., 2007). High-protein diets activated trypsin and chymotrypsin activity in rainbow trout (Rungruangsak-Torrissen et al., 2009), while red seabream (Pagrus major) on soybean meal diets exhibited decreased hepatopancreatic enzymes and protease activity compared to fishmeal-based diets (Murashita et al., 2018). This difference in digestive enzyme activities may be attributed to antinutritional factors in soybean, not necessarily reduced nutrient intake (Yasothai, 2016; Assan et al., 2022).
A comparative study of amylase and proteolytic activities in six fish species revealed that omnivorous species, like gilthead seabream, had higher amylase activity, whereas carnivorous species exhibited greater proteolytic enzyme activity (Hidalgo et al., 1999). In larval Yellow catfish (Pelteobagrus fulvidraco), pepsin and trypsin activity increased initially and then decreased with age (Wang et al., 2006). Older abalone (Haliotis laevigata) showed a decrease in trypsin and amylase activity compared to younger ones (Bansemer et al., 2016), indicating age-dependent variations in digestive enzyme activity (Infante and Cahu, 2001).
Stocking density also indirectly affects digestive enzyme activity; higher density results in decreased lipase, amylase, and trypsin activities in fish and shrimp (Liu et al., 2017; Dong et al., 2018; Liu et al., 2018), likely due to reduced appetite and stress. Intestinal brush border enzyme development, seasonal changes, diet variations, probiotic supplementation, and rearing environment impact gastrointestinal microbial communities, with unfavorable conditions leading to stress, decreased appetite, and subsequently lower digestive enzyme activity. Rearing temperature influences digestive enzymes, with decreased activity observed at lower temperatures (Bansemer et al., 2016). It is crucial to consider these factors in dietary formulation for fish, as decreased digestive capacity can lead to reduced growth (Iqbal et al., 2016; Zeng et al., 2016). Despite the positive effects of probiotics in modulating digestive enzyme activities, careful consideration of the mentioned factors is necessary for optimizing probiotics’ digestive enzyme modulation capacity.
In the realm of digestive enzymes, beyond the primary categories like amylase, protease, and lipase, there are noteworthy subclasses such as cellulase (related to amylase) and alginase, both influenced by probiotics. These enzymes hold significance, particularly for omnivorous and herbivorous fish, which predominantly consume plants containing cellulose and algin. Some fish species lack the synthesis of cellulase and alginase or produce them in insufficient quantities, necessitating supplementation (Clements, 1997; Assan et al., 2022).
While literature on the modulation of alginase by probiotics is limited, available studies confirm increased alginase activities in South African abalone (Haliotis midae) (approximately 58.33%) (ten Doeschate and Coyne, 2008) and juvenile sea cucumber (Apostichopus japonicus) (97.47%) (Wang et al., 2015) associated with probiotic diet supplementation. Other research indicates the production of alginase by probiotic bacteria (Huddy and Coyne, 2014; Escamilla-Montes et al., 2015).
It is noteworthy that some fish generally lack cellulose due to its plant origin, and the presence of cellulase enzymes in fish intestines is likely a result of ingested microbial flora. Inclusion of probiotic bacteria in fish diet becomes essential for cellulase activity. Although cellulose is strictly derived from plants, herbivorous fish exhibit a higher population of cellulase-producing bacterial strains due to the presence of microbial flora in their digestive tracts (Kar and Ghosh, 2008). Yet, only a few studies have touched upon the modulation of cellulase in fish concerning probiotic activities. For instance, Bairagi et al. (2004) reported exogenous and extracellular digestive cellulose production by certain Bacillus spp. strains in rohu (Labeo rohita) fingerlings. Similarly, probiotic supplementation was found to elevate the activities of digestive cellulase enzymes in Mori (Cirrhinus mrigala) and shrimp (Penaeus vannamei) (Ullah et al., 2018). Table 1 provides a summary of the source, dose, and duration of probiotics used to modulate digestive enzymes in cultured fish.
Different fish species have varying physiological and immunological characteristics. Therefore, the response to probiotics, prebiotics, and synbiotics can be species-specific (Ringø and Song, 2016; Huynh et al., 2017). The microbial communities in the gut, which interact with these supplements, may vary among species. Understanding the specific requirements of the target species is crucial for the successful application of these supplements (Dawood and Koshio, 2016; Huynh et al., 2017; Cavalcante et al., 2020). The fish diet plays a significant role in the efficacy of probiotics, prebiotics, and synbiotics. The composition and nutritional content of the feed can impact the survival and proliferation of beneficial microorganisms (Akhter et al., 2015). Some probiotics may require specific nutrients for growth, and prebiotics can serve as a substrate for beneficial bacteria. The diet’s balance between probiotics and prebiotics is essential for optimal performance.
Environmental factors, such as water quality, temperature, and salinity, influence the gut microbiota of fish (Hura et al., 2018; Hlordzi et al., 2020; Amenyogbe et al., 2021). Probiotics and prebiotics may be sensitive to environmental conditions, affecting their viability and functionality (Kuebutornye et al., 2019). High temperatures or abrupt changes in water quality can stress fish, compromising their immune system and making them more susceptible to diseases (Hoseinifar et al., 2018; Ringø et al., 2018; Kuebutornye et al., 2019; Hlordzi et al., 2020; Van Doan et al., 2020). Therefore, maintaining stable and suitable environmental conditions is crucial for the effectiveness of these supplements. The dosage and method of administration significantly impact the efficacy of probiotics, prebiotics, and synbiotics (Cerezuela et al., 2013; Kumar et al., 2018; Zoumpopoulou et al., 2018; Cavalcante et al., 2020). The optimal dosage can vary based on factors like fish size, age, and health status. Overdosing may lead to competition among microorganisms for resources, while underdosing may not provide the desired benefits. The method of administration, whether through the feed or water, also affects the contact time between the supplements and the gut microbiota.
Interaction with other feed additives, such as antibiotics or immunostimulants, can influence the efficacy of probiotics, prebiotics, and synbiotics (Banerjee et al., 2013; Simo´n et al., 2021). Some additives may enhance or inhibit the activity of beneficial microorganisms. Understanding these interactions is crucial for formulating a balanced and effective feed regimen (Hura et al., 2018; Hlordzi et al., 2020). The health status of fish is a critical factor affecting the success of probiotics and prebiotics. In stressed or diseased fish, the gut microbiota may be altered, making it challenging for probiotics to establish themselves (Hoseinifar et al., 2018; Ringø et al., 2018; Kuebutornye et al., 2019; Hlordzi et al., 2020; Van Doan et al., 2020). Addressing the underlying health issues before introducing these supplements is essential in such cases.
The choice of probiotic strains is paramount. Different strains exhibit distinct properties, including resistance to environmental conditions, adhesion to the gut epithelium, and production of bioactive compounds (Cerezuela et al., 2013; Zoumpopoulou et al., 2018; Cavalcante et al., 2020). Selecting strains with proven benefits for the target species under specific conditions is crucial for achieving the desired effects (Akhter et al., 2015; Dawood and Koshio, 2016; Huynh et al., 2017; Cavalcante et al., 2020). The duration of administering probiotics, prebiotics, or synbiotics is an important consideration. Some benefits may take time to manifest, and prolonged administration might be necessary for sustained effects (Amenyogbe, 2023). Conversely, continuous use without periodic evaluation may lead to a decline in efficacy.
Therefore, a holistic approach that considers the interplay of fish species, diet, environmental conditions, dosage, interactions with other feed additives, health status, strain selection, and duration of administration is essential for optimizing the efficacy of probiotics, prebiotics, and synbiotics in aquaculture. Researchers and aquaculturists need to tailor their strategies based on a thorough understanding of these factors to promote the health and performance of fish populations.
10 Current challenges and future directions in implementing probiotics, prebiotics, and synbiotics in fish farming
Different fish species have unique gut microbiomes and nutritional requirements. Identifying probiotic strains that are effective across a range of species is challenging. Tailoring probiotic formulations to specific fish species is crucial for optimal performance (Amenyogbe, 2023). Probiotics need to survive and remain viable during the manufacturing process, storage, and administration to the fish. Harsh environmental conditions can reduce their effectiveness. Improving the encapsulation techniques and storage conditions is essential for enhancing probiotic viability (Hoseinifar et al., 2018; Ringø et al., 2018; Kuebutornye et al., 2019; Hlordzi et al., 2020; Van Doan et al., 2020). Determining the optimal dosage and duration of probiotic supplementation is complex. Too little may be ineffective, while excessive amounts can be wasteful and may lead to unintended consequences (Akhter et al., 2015; Dawood and Koshio, 2016; Huynh et al., 2017; Cavalcante et al., 2020). Conducting long-term studies to understand the sustained effects of probiotics on fish health and growth is necessary (Amenyogbe, 2023).
The interaction of probiotics with different feed components and additives needs to be better understood. Some compounds in feed may inhibit or enhance the efficacy of probiotics. Investigating the compatibility of probiotics with various feed formulations is critical for successful integration (Kuebutornye et al., 2019; Amenyogbe et al., 2021). Environmental conditions in aquaculture systems, such as temperature, pH, and water quality, can impact the performance of probiotics (Kuebutornye et al., 2019; Amenyogbe et al., 2021). Adapting probiotic formulations to diverse environmental conditions is a challenge. Research on developing robust probiotic strains that can withstand a range of environmental variables is needed. The need for standardized regulations for probiotic use in aquaculture poses a challenge (Amenyogbe et al., 2020). Obtaining regulatory approval for probiotics is crucial for their widespread adoption. Establishing international standards and guidelines for probiotics in aquaculture is essential for ensuring product safety and efficacy.
Understanding the host-microbiome interactions to develop species-specific probiotic interventions is necessary (Kuebutornye et al., 2019; Amenyogbe et al., 2021). Research on innovative encapsulation methods to enhance the survival and targeted delivery of probiotics to the fish gut is needed. Exploring nanotechnology and other advanced delivery systems for efficient probiotic administration are areas that can be looked at. Conducting dose-response studies to determine the optimal concentration of probiotics for different fish species and life stages are necessary. Investigating the potential for developing time-release formulations for sustained probiotic delivery is very much needed. Systematic analysis of the interaction between probiotics and various feed components to optimize feed formulations. Identifying feed additives that synergistically enhance the efficacy of probiotics is an area that researchers can look at. Assessing the ecological impact of probiotics on the overall aquatic environment, including non-target species and microbial communities, is important. Investigating the potential for developing eco-friendly probiotic formulations is a way to go.
Long-term studies to evaluate the extended effects of probiotic supplementation on fish health, disease resistance, and overall performance are very much needed attention. Exploring the potential for probiotics to mitigate the impact of stressors in aquaculture systems is also needs to be fully understood and needs much research. Collaborative efforts among researchers, industry stakeholders, and regulatory bodies to establish standardized guidelines for probiotic use in fish farming are the uttermost important. Addressing the current challenges and exploring these suggested research directions can contribute to the successful implementation of probiotics, prebiotics, and synbiotics in fish farming, ultimately improving the sustainability and efficiency of aquaculture practices.
11 Our viewpoints on methodological evaluation and standardization in probiotic, prebiotic, and synbiotic studies in fish farming
Research on probiotic, prebiotic, and synbiotic interactions in fish farming is a complex and evolving field with essential implications for aquaculture sustainability. Existing methodologies, however, show variations that hinder cross-study comparisons and data extrapolation. The selection and characterization of probiotics, prebiotics, and synbiotics in existing studies is often inconsistent. It is important to note that strain sources, identification methods, and strain-specific traits can significantly impact results. The reproducibility of strain isolation, identification (e.g., molecular techniques), and characterization (e.g., viability, adhesion capacity) must be ensured using standard methods. Identifying dose-response relationships can be challenging because dosing strategies vary widely among studies. Considering fish species, weight, and environmental conditions, standardized dosing protocols are crucial. Transparent reporting of administration routes (e.g., feed, water) and frequency will facilitate the replication of experiments. Existing studies have a variety of experimental designs, durations, and environmental conditions that make comparisons difficult. The framework for an investigation must be standardized, with appropriate controls and statistical analyses. The long-term effects of probiotics, prebiotics, and synbiotics would be better understood through longitudinal studies with consistent environmental parameters.
Variability in methodologies for assessing digestive enzymes complicates result interpretation. Standardized assays for specific enzymes relevant to fish digestion should be adopted, considering substrate concentration and reaction time factors. Enzyme activity should be expressed consistently, allowing for meaningful comparisons. Inconsistencies in oxidative stress and antioxidant defense measurements undermine the result’s reliability. Standardized assays (e.g., lipid peroxidation, antioxidant enzyme activities) with validated protocols must be employed. Additionally, reporting basal and induced stress conditions will enhance understanding of the system’s resilience.
Implement internationally recognized protocols for isolating, identifying, and characterizing probiotic, prebiotic, and synbiotic strains to ensure uniformity across studies. Develop standardized dosing guidelines based on fish species, weight, and environmental conditions. Researchers should clearly document administration routes and frequency to facilitate replication. Establish a standardized experimental framework, including appropriate controls and statistical analyses. Encourage longitudinal studies with consistent environmental parameters to assess long-term effects.
Researchers should adopt standardized assays for digestive enzyme analysis, accounting for factors like substrate concentration and reaction time. Enzyme activity reporting should be consistent for meaningful cross-study comparisons. Developing and endorsing standardized assays with validated protocols for oxidative stress and antioxidant defense assessments is essential. Researchers are encouraged to report basal and induced stress conditions to capture the system’s resilience. Standardizing methodologies in fish farming in probiotic, prebiotic, and synbiotic studies is imperative for advancing the field and facilitating cross-study comparisons. Implementing uniform strain characterization, dosing guidelines, experimental protocols, and measurement techniques will enhance the findings’ reliability and applicability, contributing to aquaculture’s sustainable development. Researchers, industry stakeholders, and regulatory bodies must collaborate to establish and endorse these standardized approaches for the benefit of the aquaculture sector.
12 Investigation gaps and approaching perspectives
Despite significant progress, there still needs to be more in our understanding of the mechanisms through which probiotics, prebiotics, and synbiotics exert their effects on digestive enzymes, oxidative stress, and antioxidant defense in fish. Specific gaps include a need for standardized protocols for evaluating the efficacy of these supplements, a need for standardized information on optimal dosages and administration methods, and long-term studies to assess sustained effects. While there is substantial literature and research on the impact of microbial feed additives on factors such as growth performance, immune response, and disease resistance in fish, there is a noticeable lack of studies specifically addressing the modulation of antioxidant defense through the use of functional feed additives. Exploring the significance of these environmentally friendly feed additives on antioxidant defense represents a clear avenue for future research. Additionally, there are proposed mechanisms of action related to the modification of antioxidant defense activities after treatment with functional feed additives, and it is essential to conduct thorough investigations to validate and confirm these suggested modes of action in fish. The interaction between host genetics, diet, and the gut microbiome in response to these supplements requires further exploration. More research is needed to identify suitable biomarkers that accurately reflect the health status of fish in response to probiotics, prebiotics, and synbiotics. Research should address the species-specific responses to these supplements, as different fish species may exhibit variations in their gut microbiota and physiological responses. Future research should explore the environmental impact of probiotics, prebiotics, and synbiotics in aquaculture, considering sustainability and potential ecological consequences. While existing research provides valuable insights into the positive effects of probiotics, prebiotics, and synbiotics on digestive enzymes, oxidative stress, and antioxidant defense in fish farming, further investigation is necessary to address species-specific variations, and long-term impacts for sustainable aquaculture practices. Researchers should focus on filling these gaps to optimize the effective use of these supplements in fish farming.
13 Conclusion
In conclusion, the application of probiotics, prebiotics, and synbiotics in the aquaculture industry holds great promise for improving digestive health and reducing oxidative stress in fish populations. These interventions offer multifaceted benefits, highlighting their potential as effective tools for sustainable aquaculture practices. Probiotics, particularly beneficial bacteria such as Lactobacillus and Bacillus species, have shown significant improvements in gut microbiota composition and function. These microorganisms play crucial roles in aiding nutrient absorption, inhibiting pathogenic bacteria, and modulating immune responses. By establishing a balanced microbial community within the gastrointestinal tract, probiotics promote optimal digestion and nutrient utilization, contributing to the overall resilience of fish. Complementing the effects of probiotics, prebiotics are non-digestible compounds that selectively stimulate the growth and activity of beneficial microorganisms. Prebiotics such as fructo-oligosaccharides (FOS) and inulin support the establishment of a robust gut microbiome, facilitating nutrient assimilation and bolstering the immune system. When combined with probiotics, these prebiotics create synbiotics, offering a holistic approach to promoting digestive health in fish. This cooperative strategy reinforces positive outcomes in growth performance, disease resistance, and stress tolerance. Furthermore, probiotics, prebiotics, and synbiotics play a crucial role in managing oxidative stress in fish. Oxidative stress, resulting from an imbalance between the productions of reactive oxygen species (ROS) and antioxidant defense mechanisms, can have detrimental effects on fish health. These bioactive compounds enhance antioxidant enzyme activities, scavenge free radicals, and reduce lipid peroxidation, serving as effective mitigating agents against oxidative stress.
As the aquaculture industry continues to evolve, integrating probiotics, prebiotics, and synbiotics into fish diets represents a proactive approach toward sustainable and responsible farming practices. However, it is essential to acknowledge that their efficacy can be species-specific and influenced by various environmental factors. Therefore, ongoing research and tailored approaches are crucial to optimizing their application for different fish species and production systems. The utilization of probiotics, prebiotics, and synbiotics offers a promising avenue for enhancing digestive health and managing oxidative stress in fish. These interventions contribute to the overall well-being of fish populations and align with the broader goals of sustainable aquaculture by reducing reliance on antibiotics and promoting ecological balance within aquatic ecosystems.
Author contributions
EA: Conceptualization, Data curation, Formal analysis, Investigation, Methodology, Project administration, Resources, Supervision, Validation, Writing – original draft, Writing – review & editing. ED: Data curation, Formal analysis, Investigation, Writing – review & editing. CL: Formal analysis, Investigation, Methodology, Writing – review & editing. GB: Formal analysis, Investigation, Methodology, Writing – review & editing. RD: Data curation, Formal analysis, Investigation, Methodology, Writing – review & editing. EA: Data curation, Investigation, Methodology, Writing – review & editing. JH: Data curation, Formal analysis, Funding acquisition, Investigation, Methodology, Resources, Supervision, Validation, Writing – review & editing.
Funding
The author(s) declare financial support was received for the research, authorship, and/or publication of this article. This work was supported by grants from the National Key R&D Program of China (2022YFD2401203), the Zhanjiang Marine youth talent innovation project in 2021 (2021E05026); Key scientific research platforms and projects of ordinary universities in Guangdong Province in 2022 (2022KCXTD013).
Acknowledgments
We acknowledge all funders of this work.
Conflict of interest
The authors declare that the research was conducted in the absence of any commercial or financial relationships that could be construed as a potential conflict of interest.
Publisher’s note
All claims expressed in this article are solely those of the authors and do not necessarily represent those of their affiliated organizations, or those of the publisher, the editors and the reviewers. Any product that may be evaluated in this article, or claim that may be made by its manufacturer, is not guaranteed or endorsed by the publisher.
Supplementary material
The Supplementary Material for this article can be found online at: https://www.frontiersin.org/articles/10.3389/fmars.2024.1368436/full#supplementary-material
References
Abdel-Latif H. M., Chaklader M. R., Shukry M., Ahmed H. A., Khallaf M. A. (2023). A multispecies probiotic modulates growth, digestive enzymes, immunity, hepatic antioxidant activity, and disease resistance of Pangasianodon hypophthalmus fingerlings. Aquaculture 563, 738948. doi: 10.1016/j.aquaculture.2022.738948
Abdel-Tawwab M., Abdel-Rahman A. M., Ismael N. E. (2008). Evaluation of commercial live bakers’ yeast, Saccharomyces cerevisiae as a growth and immunity promoter for Fry Nile tilapia, Oreochromis niloticus (L.) challenged in situ with Aeromonas hydrophila. Aquaculture 280, 185–189. doi: 10.1016/j.aquaculture.2008.03.055
Abid A., Davies S. J., Waines P., Emery M., Castex M., Gioacchini G., et al. (2013). Dietary synbiotic application modulates Atlantic salmon (Salmo salar) intestinal microbial communities and intestinal immunity. Fish Shellfish Immunol. 35, 1948–1956. doi: 10.1016/j.fsi.2013.09.039
Adorian T. J., Jamali H., Farsani H. G., Darvishi P., Hasanpour S., Bagheri T., et al. (2018). Effects of probiotic bacteria Bacillus on growth performance, digestive enzyme activity, and hematological parameters of Asian Sea bass, Lates calcarifer (Bloch). Probiotics Antimicrob. Proteins. 11, 248–255. doi: 10.1007/s12602-018-9393-z
Afrilasari W., Meryandini A. (2016). Effect of Probiotic Bacillus megaterium PTB 1.4 on the Population of Intestinal Microflora, Digestive Enzyme Activity and the Growth of Catfish (Clarias sp.). HAYATI J. Biosci. 23, 168–172. doi: 10.1016/j.hjb.2016.12.005
Ahire J. J., Mokashe N. U., Patil H. J., Chaudhari B. L. (2013). Antioxidative potential of folate producing probiotic Lactobacillus helveticus CD6. J. Food Sci. Technol. 50, 26–34. doi: 10.1007/s13197-011-0244-0
Ahmad S. (1995). “Preface,” in Oxidative stress and antioxidant defenses in biology. Ed. Ahmad S. (Chapman & Hall, New York), 11–17.
Ai Q., Xu H., Mai K., Xu W., Wang J., Zhang W. (2011). Effects of dietary supplementation of Bacillus subtilis and fructooligosaccharide on growth performance, survival, non-specific immune response and disease resistance of juvenile large yellow croaker, Larimichthys crocea. Aquaculture 317, 155–161. doi: 10.1016/j.aquaculture.2011.04.036
Akhter N., Wu B., Memon A. M., Mohsin M. (2015). Probiotics and prebiotics associated with aquaculture: A review. Fish Shellfish Immunol. 45, 733–741. doi: 10.1016/j.fsi.2015.05.038
Akrami R., Hajimoradloo A., Matinfar A., Abedian Kinari A. (2009). Effect of dietary prebiotic inulin on growth performance, intestinal microflora, body composition and hematological parameters of juvenile beluga, huso huso (Linnaeu). J. World Aquacult. Soc 40 (6), 771–779. doi: 10.1111/j.1749-7345.2009.00297.x
Akrami R., Nasri-Tajan M., Jahedi A., Jahedi M., Mansour M. R., Jafarpour S. A. (2015). Effects of dietary synbiotic on growth, survival, lactobacillus bacterial count, blood indices and immunity of beluga (Huso huso Linnaeus 1754) juvenile. Aquaculture Nutr. 21, 952–959. doi: 10.1111/anu.12219
Allameh S. K., Noaman V., Nahavandi R. (2017). Effects of probiotic bacteria on fish performance. Adv. Tech. Clin. Microbiol. 1, 1–5.
Aly S. M., Abdel-Galil Ahmed Y., Abdel-Aziz Ghareeb A., Mohamed M. F. (2008). Studies on Bacillus subtilis and Lactobacillus acidophilus, as potential probiotics, on the immune response and resistance of Tilapia nilotica (Oreochromis niloticus) to challenge infections. Fish Shellfish Immunol. 25, 128–136. doi: 10.1016/j.fsi.2008.03.013
Amenyogbe E. (2023). Application of probiotics for sustainable and environment-friendly aquaculture management - A review. Cogent Food Agric. 9 (1), 2226425. doi: 10.1080/23311932.2023.2226425
Amenyogbe E., Chen G., Wang Z., Huang J., Huang B., Li H. (2020). The exploitation of probiotics, prebiotics, and synbiotics in aquaculture: present study, limitations and future directions. : a review. Aquaculture Int. 28, 1017–1041. doi: 10.1007/s10499-020-00509-0
Amenyogbe E., Huang J.-S., Chen G., Wang W.-Z. (2021). Probiotic Potential of Indigenous (Bacillus sp. RCS1, Pantoea agglomerans RCS2, and Bacillus cereus strain RCS3) Isolated from Cobia Fish (Rachycentron canadum) and Their Antagonistic Effects on the Growth of Pathogenic Vibrio alginolyticus, Vibrio harveyi, Streptococcus iniae, and Streptococcus agalactiae. Front. Mar. Sci. 8. doi: 10.3389/fmars.2021.672213
Amenyogbe E., Luo J., Fu W.-J., Abarike E. D., Wang Z.-L., Huang J.-S., et al. (2022c). Effects of autochthonous strains mixture on gut microbiota and metabolic profile in cobia (Rachycentron canadum). Sci. Rep. 12, 17410. doi: 10.1038/s41598-022-19663-x
Amenyogbe E., Yang E. J., Xie R. T., Huang J. S., Chen G. (2022a). Influences of indigenous isolates Pantoea agglomerans RCS2 on growth, proximate analysis, haematological parameters, digestive enzyme activities, serum biochemical parameters, antioxidants activities, intestinal morphology, disease resistance, and molecular immune response in juvenile’s cobia fish (Rachycentron canadum). Aquaculture 551, 737942. doi: 10.1016/j.aquaculture.2022.737942
Amenyogbe E., Zhang J. D., Huang J. S., Chen G. (2022b). The efficiency of indigenous isolates Bacillus sp. RCS1 and Bacillus cereus RCS3 on growth performance, blood biochemical indices and resistance against Vibrio harveyi in cobia fish (Rachycentron canadum) juveniles. Aquaculture Rep. 25, 101241. doi: 10.1016/j.aqrep.2022.101241
Andrews S. R., Sahu N. P., Pal A. K., Kumar S. (2009). Haematological modulation and growth of labeo rohita fingerlings: Effect of dietary mannan oligosaccharide, yeast extract, protein hydrolysate and chlorella. Aquaculture Res. 41 (1), 61–69. doi: 10.1111/j.1365-2109.2009.02304.x
Ashouri G., Mahboobi Soofiani N., Hoseinifar S. H., Jalali S. A. H., Morshedi V., Valinassab T., et al. (2020). Influence of dietary sodium alginate and Pediococcus acidilactici on liver antioxidant status, intestinal lysozyme gene expression, histomorphology, microbiota, and digestive enzymes activity, in Asian sea bass (Lates calcalifer) juveniles. Aquacult 518, 734638. doi: 10.1016/j.aquaculture.2019.734638
Assan D., Kuebutornye F. K. A., Hlordzi V., Chen H., Mraz J., Mustapha U. F., et al. (2022). Effects of probiotics on digestive enzymes of fish (finfish and shellfish); status and prospects: A mini review. Comp. Biochem. Physiol. Part B: Biochem. Mol. Biol. 257, 110653. doi: 10.1016/j.cbpb.2021.110653
Azevedo R. V., Filho J. C. F., Pereira S. L., Cardoso L. D., Júnior M. V. V., Andrade D. R. (2016). Suplementação com prebiótico, probiótico e simbiótico para juvenis de tambaqui a duas densidades de estocagem. Pesq. agropec. bras. Brasília. 51, 9–16. doi: 10.1590/S0100-204X2016000100002
Bairagi A., Sarkar Ghosh K., Sen S. K., Ray A. K. (2004). Evaluation of the nutritive value of Leucaena leucocephala leaf meal, inoculated with fish intestinal bacteria Bacillus subtilis and Bacillus circulans in formulated diets for rohu, Labeo rohita (Hamilton) fingerlings. Aquac. Res. 35, 436–446. doi: 10.1111/j.1365-2109.2004.01028.x
Balcázar J. L., Blas I. D., Ruiz-Zarzuela I., Cunningham D., Vendrell D., Múzquiz J. L. (2006). The role of probiotics in aquaculture. Veterinary Microbiol. 114, 173–186. doi: 10.1016/j.vetmic.2006.01.009
Balcazar J. L., de Blas I., Ruiz-Zarzuela I., Vendrell D., Gironés O., Muzquiz J. L. (2007). Enhancement of the immune response and protection induced by probiotic lactic acid bacteria against furunculosis in rainbow trout (Oncorhynchus mykiss). FEMS Immunol. Med. Microbiol. 51 (1), 185–193. doi: 10.1111/j.1574-695x.2007.00294.x
Banerjee S. P., Dora K. C., Chowdhury S. (2013). Detection, partial purification and characterisation of bacteriocin produced by Lactobacillus brevis FPTLB3 isolated from freshwater fish. J. Food Sci. Technol. 50, 17–25. doi: 10.1007/s13197-011-0240-4
Bansemer M. S., Qin J. G., Harris J. O., Schaefer E. N., Wang H., Mercer G. J., et al. (2016). Age-dependent response of digestive enzyme activities to dietary protein level and water temperature in greenlip abalone (Haliotis laevigata). Aquaculture 451, 451–456. doi: 10.1016/j.aquaculture.2015.10.013
Bartlett M. S. (1937). Properties of sufficiency and statistical tests. Proc. R. Soc. London Ser. a- Math. Phys. Sci. 160, 268–282.
Beck B. R., Kim D., Jeon J., Lee S., Kim H. K., Kim O., et al. (2014). The effects of combined dietary probiotics Lactococcus lactis BFE920 and Lactobacillus plantarum FGL0001 on innate immunity and disease resistance in olive flounder (Paralichthys olivaceus). Fish Shellfish Immunol. 42 (1), 177–183. doi: 10.1016/j.fsi.2014.10.035
Biller J. D., Takahashi L. S. (2018). Oxidative stress and fish immune system: phagocytosis and leukocyte respiratory burst activity. Acad. Bras. Cienc. 90, 3403–3414. doi: 10.1590/0001-3765201820170730
Birnie-Gauvin K., Costantini D., Cooke S. J., Willmore W. G. (2017). Comparative and evolutionary approach to oxidative stress in fish: a review. Fish Fish. 18, 928–942. doi: 10.1111/faf.12215
Brzozowski T., Konturek P. C., Mierzwa M., Drozdowicz D., Bielanski W., Kwiecien S., et al. (2006). Effect of probiotics and triple eradication therapy on the cyclooxygenase (COX)-2 expression, apoptosis, and functional gastric mucosal impairment in Helicobacter pylori-infected Mongolian gerbils. Helicobact 11, 10–20. doi: 10.1111/j.0083-8703.2006.00373.x
Burns A. R., Stephens W. Z., Stagaman K., Wong S., Rawls J. F., Guillemin K., et al. (2016). Contribution of neutral processes to the assembly of gut microbial communities in the zebrafish over host development. ISME J. 10, 655–664. doi: 10.1038/ismej.2015.142
Cadenas E. (1995). “Mechanisms of oxygen activation and reactive oxygen species detoxification,” in Oxidative stress and antioxidant defences in biology. Ed. Ahmad S. (Chapman & Hall, New York), 1–46.
Calado R., Dionísio G., Dinis M. T. (2007). Starvation resistance of early zoeal stages of marine ornamental shrimps Lysmata spp. (Decapoda: Hippolytidae) from different habitats. J. Exp. Mar. Biol. Ecol. 351, 226–233. doi: 10.1016/j.jembe.2007.06.022
Cao H., Yu R., Zhang Y., Hu B., Jian S., Wen C., et al. (2019). Effects of dietary supplementation with b-glucan and Bacillus subtilis on growth, fillet quality, immune capacity, and antioxidant status of Pengze crucian carp (Carassius auratus var. Pengze). Aquacult 508, 106–112. doi: 10.1016/j.aquaculture.2019.04.064
Cavalcante R. B., Telli G. S., Tachibana L., Dias D. D. C., Oshiro E., Natori M. M., et al. (2020). Probiotics, Prebiotics and Synbiotics for Nile tilapia: Growth performance and protection against Aeromonas hydrophila infection. Aquaculture Rep. 17, 100343. doi: 10.1016/j.aqrep.2020.100343
Cerezuela R., Fumanal M., Tapia-Paniagua S. T., Meseguer J., Moriñigo M. A., Esteban M. A. (2013). Changes in intestinal morphology and microbiota caused by dietary administration of inulin and Bacillus subtilis in gilthead sea bream (Sparus aurata L.) specimens. Fish Shellfish Immunol. 34, 1063–1070. doi: 10.1016/j.fsi.2013.01.015
Chen D., Yao Y., Cui Z., Zhang X., Guo X., Zhou Y., et al. (2019). Comparative study of the immunoprotective effect of two grass carp-sourced Bacillus subtilis spore-based vaccines against grass carp reovirus. Aquaculture 504, 88–95. doi: 10.1016/j.aquaculture.2019.01.055
Cheng C. H., Liang H. Y., Luo S. W., Wang A. L., Ye C. X. (2018). The protective effects of vitamin C on apoptosis, DNA damage and proteome of pufferfish (Takifugu obscurus) under low temperature stress. J. Therm Biol. 71, 128–135. doi: 10.1016/j.jtherbio.2017.11.004
Clements K. D. (1997). “Fermentation and gastrointestinal microorganisms in fishes,” in Gastrointestinal microbiology. Chapman & Hall Microbiology Series. Eds. Mackie R. I., White B. A. (Boston, MA: Springer). doi: 10.1007/978-1-4615-4111-0_6
Daniels C., Hoseinifar S. H. (2014). “Prebiotic applications in shellfish,” in Aquaculture nutrition: gut health, probiotics and prebiotics. Eds. Merrifield D., Ringø E. (Wiley, Chichester), 401–418. doi: 10.1002/9781118897263.ch15
Davies K. (1995). Oxidative stress: the paradox of aerobic life. Biochem. Soc. Symp. 61, 1–31. doi: 10.1042/bss0610001
Dawood M. A. O., Koshio S. (2016). Recent advances in the role of probiotics and prebiotics in carp aquaculture: a review. Aquaculture 454, 243–251. doi: 10.1016/j.aquaculture.2015.12.033
Dawood A. O., Koshio S., Esteban M.Á. (2018). Beneficial roles of feed additives as immunostimulants in aquaculture: A review. Rev. Aquaculture 10, 950–974. doi: 10.1111/raq.12209
Dawood M. A., Koshio S., Ishikawa M., El-Sabagh M., Esteban M. A., Zaineldin A. I. (2016a). Probiotics as an environment-friendly approach to enhance red sea bream, Pagrus major growth, immune response and oxidative status. Fish Shellfish Immunol. 57, 170–178. doi: 10.1016/j.fsi.2016.08.038
Dawood M. A. O., Koshio S., Ishikawa M., Yokoyama S., El Basuini M. F., Hossain M. S., et al. (2016b). Effects of dietary supplementation of Lactobacillus rhamnosus or/and Lactococcus lactis on the growth, gut microbiota and immune responses of red sea bream, Pagrus major. Fish Shellfish Immunol. 49, 275–285. doi: 10.1016/j.fsi.2015.12.047
Dehaghani P. G., Baboli M. J., Moghadam A. T., Ziaei-Nejad S., Pourfarhadi M. (2015). Effect of synbiotic dietary supplementation on survival, growth performance and digestive enzyme activities on common carp (Cyprinus carpio) fingerlings. Czech J. Anim. Sci. 60, 224–232. doi: 10.17221/8172-CJAS
Devi G., Harikrishnan R., Paray B. A., Al-Sadoon M. K., Hoseinifar S. H., Balasundaram C. (2019). Effect of symbiotic supplemented diet on innate-adaptive immune response, cytokine gene regulation and antioxidant property in Labeo rohita against Aeromonas hydrophila. Fish Shellfish Immunol. 89, 687–700. doi: 10.1016/j.fsi.2019.04.036
Dhanasiri A. K. S., Jaramillo-Torres A., Chikwati E. M., Forberg T., Krogdahl Å., Kortner T. M. (2023). Effects of dietary supplementation with prebiotics and Pediococcus acidilactici on gut health, transcriptome, microbiota, and metabolome in Atlantic salmon (Salmo salar L.) after seawater transfer. Anim. Microbiome 11, 10. doi: 10.1186/s42523-023-00228-w
Didinen B. I., Onuk E. E., Metin S., Cayli O. (2018). Identification and characterization of lactic acid bacteria isolated from rainbow trout (Oncorhynchus mykiss, Walbaum 1792), with inhibitory activity against Vagococcus salmoninarum and Lactococcus garvieae. Aquaculture Nutr. 24 (1), 400–407. doi: 10.1111/anu.12571
Di Gioia D., Biavati B. (2018). “Probiotics and prebiotics in animal health and food safety: Conclusive remarks and future perspectives,” in Probiotics and prebiotics in animal health and food safety. Eds. Di Gioia D., Biavati B. (Springer, Cham). doi: 10.1007/978-3-319-71950-4_11
Dong M., Liang Y., Ramalingam R., Tang S. W., Shen W., Ye R., et al. (2017). Proteomic characterization of the interactions between fish serum proteins and waterborne bacteria reveals the suppression of anti-oxidative defense as a serum-mediated antimicrobial mechanism. Fish Shellfish Immunol. 62, 96–106. doi: 10.1016/j.fsi.2017.01.013
Dong J., Zhao Y.-Y., Yu Y.-H., Sun N., Li Y.-D., Wei H., et al. (2018). Effect of stocking density on growth performance, digestive enzyme activities, and nonspecific immune parameters of Palaemonetes sinensis. Fish Shellfish Immunol. 73, 37–41. doi: 10.1016/j.fsi.2017.12.006
Dotta G., Mouriño J. L. P., Jatobá A., Morán R. E. B., Pilate C., Martins M. L. (2011). Acute inflammatory response in Nile tilapia fed probiotic Lactobacillus plantarum in the diet. Acta Scientiarum. Biol. Sci. 33, 239–246. doi: 10.4025/actascibiolsci.v33i3.8011
Ducasse-Cabanot S., Zambonino-Infante J., Richard N., Medale F., Corraze G., Mambrini M., et al. (2007). Reduced lipid intake leads to changes in digestive enzymes in the intestine but has minor effects on key enzymes of hepatic intermediary metabolism in rainbow trout (Oncorhynchus mykiss). Animal 1, 1272–1282. doi: 10.1017/S1751731107000596
Escamilla-Montes R., Luna-Gonz´alez A., del Carmen Flores-Miranda M., Alvarez-Ruiz P., Fierro-Coronado J. A., S´anchez-Ortiz A. C., et al. (2015). Isolation and characterization of potential probiotic bacteria suitable for mollusk larvae cultures. Thai. J. Vet. Med. 45, 11. doi: 10.56808/2985-1130.2614
Essa M. A., El-Serafy S. S., El-Ezabi M. M., Daboor S. M., Esmael N. A., Lall S. P. (2010). Effect of different dietary probiotics on growth, feed utilization and digestive enzymes activities of nile tilapia. J. Arab. Aquac. Soc 5, 143–162.
Falc´on-Hidalgo B., Forrellat-Barrios A., Farn´es O. C., Hern´andez K. U. (2011). Digestive enzymes of two freshwater fishes (Limia vittata and Gambusia punctata) with different dietary preferences at three developmental stages. Comp. Biochem. Physiol. B Biochem. Mol. Biol. 158, 136–141. doi: 10.1016/j.cbpb.2010.10.009
Falcinelli S., Rodiles A., Hatef A., Picchietti S., Cossignani L., Merrifield D. L., et al. (2018). Influence of probiotics administration on gut microbiota core: A review on the effects on appetite control, glucose, and lipid metabolism. J. Clin. Gastroenterol. Proc. 9th Probiotics Prebiotics New Foods Nutraceuticals Botanicals Nutr. Hum. Microbiota Health Meeting held Rome Italy September 10 to 12 2017, S50–S56. doi: 10.1097/MCG.0000000000001064
FAO. (2011). Food and agriculture organization of the united nations: The state of world fisheries and aquaculture, Rome. Available at: http://www.fao.org/3/ai5555e.pdf.
FAO. (2016). Probiotics in animal nutrition. Production, impact and regulation (FAO Animal Production and Health Paper No. 179 Rome). Available at: http://www.fao.org/3/a-i5933e.pdf.
FAO/WHO. (2001). Probiotics in food. Health and nutritional properties and guidelines for evaluation. Report of a joint FAO/WHO expert consultation on evaluation of health and nutritional properties of probiotics in food including powder milk with live lactic acid bacteria (Cordoba, Argentina). Available at: http://www.who.int/foodsafety/publications/fs_-management/en/probiotics.pdf.
Faturrahman R. I.S., Sukiman D. (2015). Improved of growth rate of abalone haliotis asinine fed pudding probiotic-enriched protein. Proc. Environ. Sci. 23, 315–322. doi: 10.1016/j.proenv.2015.01.046
Feckaninova A., Koscova J., Mudronova D., Popelka P., Toropilova J. (2017). The use of probiotic bacteria against Aeromonas infections in salmonid aquaculture. Aquacult 469, 1–8. doi: 10.1016/j.aquaculture.2016.11.042
Ferreira C. M. (2014). Uso de probiótico durante o transporte de juvenis de tambaqui (Colossoma macropomum) em sistema feChado. Mestrado em Ciência Animal) – Faculdade de Agronomia e Medicina Veterinária e Zootecnia, Universidade Federal do Mato Grosso, Cuiabá.
Franco R., Martınez-Pinilla E. (2017). Chemical rules on the assessment of antioxidant potential in food and food additives aimed at reducing oxidative stress and neurodegeneration. Food Chem. 235, 318–323. doi: 10.1016/j.foodchem.2017.05.040
Frick C., Vierheilig J., Linke R., Savio D., Zornig H., Antensteiner R., et al. (2018). Poikilothermic animals as a previously unrecognized source of fecal indicator bacteria in a backwater ecosystem of a large river. Appl. Environ. Microbiol. 84 (16), e00715-18. doi: 10.1128/AEM.00715-18
Fuller R. (1989). Probiotics in manandanimals. J. Appl. bacteriology New York. 66, 356–378. doi: 10.1111/j.1365-2672.1989.tb05105.x
Ganguly S., Dora K. C., Sarkar S., Chowdhury S. (2013). Supplementation of prebiotics in fish feed: a review. Rev. Fish Biol. Fish 23, 195–199. doi: 10.1007/s11160-012-9291-5
Gao Q., Liu B., Shan F., Liu B., Gu Z., Song C., et al. (2022). Effects of oxidized fish oil on digestive enzyme activity and antioxidant system in Macrobrachium rosenbergii post-larvae. Aquaculture Rep. 23, 101062. doi: 10.1016/j.aqrep.2022.101062
Gatesoupe F. J. (2010). “Probiotics and other microbial manipulations in fish feeds: prospective health benefits,” in Bioactive foods in promoting health (Academic press, San Diego, CA). doi: 10.1016/B978-0-12-374938-3.00032-3
Geng X., Dong X.-H., Tan B.-P., Yang Q.-H., Chi S.-Y., Liu H.-Y., et al. (2011). Effects of dietary chitosan and Bacillus subtilis on the growth performance, non-specific immunity and disease resistance of cobia, Rachycentron canadum. Fish Shellfish Immunol. 31, 400–406. doi: 10.1016/j.fsi.2011.06.006
Geraylou Z., Souffreau C., Rurangwa E., Maes G. E., Spanier K. I., Courtin C. M., et al. (2013). Prebiotic effects of arabinoxylan oligosaccharides on juvenile Siberian sturgeon (Acipenser baerii) with emphasis on the modulation of the gut microbiota using 454 pyrosequencing. FEMS Microbiol. Ecol. 86, 357–371. doi: 10.1111/fem.2013.86.issue-2
Gibson G. R. (2004). Fibre and effects on probiotics (the prebiotic concept). Clin. Nutr. Supp. 1, 25–31. doi: 10.1016/j.clnu.2004.09.005
Gisbert E., Nolasco H., Solovyev M. (2018). Towards the standardization of brush border purification and intestinal alkaline phosphatase quantification in fish with notes on other digestive enzymes. Aquaculture 487, 102–108. doi: 10.1016/j.aquaculture.2018.01.004
Gobi N., Vaseeharan B., Chen J.-C., Rekha R., Vijayakumar S., Anjugam M., et al. (2018). Dietary supplementation of probiotic Bacillus licheniformis Dahb1 improves growth performance, mucus and serum immune parameters, antioxidant enzyme activity as well as resistance against Aeromonas hydrophila in tilapia Oreochromis mossambicus. Fish Shellfish Immunol. 74, 501–508. doi: 10.1016/j.fsi.2017.12.066
Gomez-Guzman M., Toral M., Romero M., Jimenez R., Galindo P., Sanchez M., et al. (2015). Antihypertensive effects of probiotics Lactobacillus strains in spontaneously hypertensive rats. Mol. Nutr. Food Res. 59, 2326–2336. doi: 10.1002/mnfr.201500290
Guerreiro I., Couto A., MaChado M., Castro C., Pous~ao- Ferreira P., Oliva-Teles A., et al. (2016). Prebiotics effect on immune and hepatic oxidative status and gut morphology of white sea bream (Diplodus sargus). Fish Shellfish Immunol. 50, 168–174. doi: 10.1016/j.fsi.2016.01.023
Halliwell B., Gutteridge J. (2015). Free radicals in biology and medicine. 5th ed (New York: Oxford University Press). doi: 10.1093/acprof:oso/9780198717478.001.0001
Hanley F., Brown H., Carbery J. (1995). “First observations on the effects of mannan oligosaccharide added to hatchery diets for warm water hybrid red tilapia,” in Poster presented at the 11th annual symposium on biotechnology in the feed industry, Lexington, KY, USA.
He S., Wang Q., Li S., Ran C., Guo X., Zhang Z., et al. (2017). Antibiotic growth promoter olaquindox increases pathogen susceptibility in fish by inducing gut microbiota dysbiosis. Sci. China Life Sci. 60, 1260–1270. doi: 10.1007/s11427-016-9072-6
Hematyar N., Rustad T., Sampels S., Kastrup Dalsgaard T. (2019). Relationship between lipid and protein oxidation in fish. Aquac Res. 50, 1393–1403. doi: 10.1111/are.14012
Heshmati J., Farsi F., Shokri F., Rezaeinejad M., Almasi-Hashiani A., Vesali S., et al. (2018). A systematic review and meta-analysis of the probiotics and synbiotics effects on oxidative stress. J. Funct. Foods 46, 66–84. doi: 10.1016/j.jff.2018.04.049
Hidalgo M. C., Urea E., Sanz A. (1999). Comparative study of digestive enzymes in fish with different nutritional habits. Proteolytic and amylase activities. Aquaculture 170, 267–283. doi: 10.1016/S0044-8486(98)00413-X
Hisano H., Barros M. M., Pezzato L. E. (2007). Levedura e zinco como pro nutrientes em racoes para tilapia-do-Nilo (Oreochromis niloticus). Aspectos hematologicos. Bol. Inst. Pesca 33, 35–42.
Hlordzi V., Kuebutornye F. K. A., Afriyie G., Abarike E. D., Lu Y., Chi S., et al. (2020). The use of Bacillus species in maintenance of water quality in aquaculture: A review. Aquaculture Rep. 18, 100503. doi: 10.1016/j.aqrep.2020.100503
Hosain M. A., Liangyi X. (2020). Impacts of probiotics on feeding technology and its application in aquaculture. J. Aquaculture Fisheries Fish Sci. 3, 174–185. doi: 10.25177/JAFFS.3.1.RA.622
Hoseinifar S. H., Ahmadi A., Khalili M., Raeisi M., Van Doan H., Caipang C. M. (2017a). The study of antioxidant enzymes and immune-related genes expression in common carp (Cyprinus carpio) fingerlings fed different prebiotics. Aquac Res. 48, 5447–5454. doi: 10.1111/are.2017.48.issue-11
Hoseinifar S. H., Esteban M. A., Cuesta A., Sun Y.-Z. (2015a). Prebiotics and fish immune response: A review of current knowledge and future perspectives. Rev. Fish Sci. Aquac. 23, 315–328. doi: 10.1080/23308249.2015.1052365
Hoseinifar S. H., Hoseini S. M., Bagheri D. (2017b). Effects of galactooligosaccharide and Pediococcus acidilactici on antioxidant defence and disease resistance of rainbow trout, Oncorhynchus mykiss. Ann. Anim. Sci. 17, 217–227. doi: 10.1515/aoas-2016-0024
Hoseinifar S. H., Hosseini M., Paknejad H., Safari R., Jafar A., Yousefi M., et al. (2019). Enhanced mucosal immune responses, immune related genes and growth performance in common carp (Cyprinus carpio) juveniles fed dietary Pediococcus acidilactici MA18/5M and raffinose. Dev. Comp. Immunol. 94, 59–65. doi: 10.1016/j.dci.2019.01.009
Hoseinifar S. H., Mirvaghefi A., Amiri B. M., Rostami H. K., Merrifield D. L. (2011b). The effects of oligofructose on growth performance, survival and autochthonous intestinal microbiota of beluga (Huso huso) juveniles. Aquaculture Nutr. 17, 498–504. doi: 10.1111/anu.2011.17.issue-5
Hoseinifar S. H., Mirvaghefi A., Amoozegar M. A., Merrifield D., Ringø E. (2015b). In vitro selection of a synbiotic and in vivo evaluation on intestinal microbiota, performance and physiological response of rainbow trout (Oncorhynchus mykiss) fingerlings. Aquacult Nutr. 23, 111–118. doi: 10.1111/anu.12373
Hoseinifar S. H., Mirvaghefi A., Merrifield D. L., Amiri B., Yelghi S., Bastami K. (2011a). The study of some haematological and serum biochemical parameters of juvenile beluga (Huso huso) fed oligofructose. Fish Physiol. Biochem. 37, 91–96. doi: 10.1007/s10695-010-9420-9
Hoseinifar S. H., Ringø E., Masouleh A. S., Esteban M. A. (2014). Probiotic, prebiotic and synbiotic supplements in sturgeon aquaculture: a review. Rev. Aquac 6, 1–14. doi: 10.1111/raq.12082
Hoseinifar S. H., Sun Y., Wang A., Zhou Z. (2018). Probiotics as means of diseases control in aquaculture, a review of current knowledge and future perspectives. Front. Microbiol. 9. doi: 10.3389/fmicb.2018.02429
Hoseinifar S. H., Yousefi S., Van Doan H., Ashouri G., Gioacchini G., Maradonna F., et al. (2021). Oxidative stress and antioxidant defense in fish: the implications of probiotic, prebiotic, and synbiotics. Rev. Fisheries Sci. Aquaculture 29 (2), 198–217. doi: 10.1080/23308249.2020.1795616
Hoseinifar S. H., Zare P., Merrifield D. L. (2010). The effects of inulin on growth factors and survival of the Indian white shrimp larvae and postlarvae (Fenneropenaeus indicus). Aquac Res. 41, e348–e352. doi: 10.1111/are.2010.41.issue-9
Hossain M. S., Koshio S., Ishikawa M., Yokoyama S., Sony N. M., Dossou S., et al. (2018). Influence of dietary inosine and vitamin C supplementation on growth, blood chemistry, oxidative stress, innate and adaptive immune responses of red sea bream, Pagrus major juvenile. Fish Shellfish Immunol. 82, 92–100. doi: 10.1016/j.fsi.2018.08.014
Huang Y., Hong Y., Yin H., Yan G., Huang Q., Li Z., et al. (2021). Imidacloprid induces locomotion impairment of the freshwater crayfish, Procambarus clarkii via neurotoxicity and oxidative stress in digestive system. Aquat. Toxicol. 238, 105913. doi: 10.1016/j.aquatox.2021.105913
Huddy R. J., Coyne V. E. (2014). Detection and Localisation of the Abalone Probiotic Vibrio midae SY9 and Its Extracellular Protease, VmproA, within the Digestive Tract of the South African Abalone, Haliotis midae. PloS One 9, e86623. doi: 10.1371/journal.pone.0086623
Hura M. U. D., Zafar T., Borana K., Prasad J. R., Iqbal J. (2018). Effect of commercial probiotic Bacillus megaterium on water quality in composite culture of major carps. The International. J. Agric. Science. 8, 268–273.
Hussain T., Gupta S., Mukhtar H. (2003). Cyclooxygenase-2 and prostate carcinogenesis. Cancer Lett. 191, 125–135. doi: 10.1016/S0304-3835(02)00524-4
Huynh T.-G., Shiu Y.-L., Nguyen T.-P., Truong Q.-P., Chen J.-C., Liu C.-H. (2017). Current applications, selection, and possible mechanisms of actions of synbiotics in improving the growth and health status in aquaculture: A review. Fish Shellfish Immunol. 64, 367–382. doi: 10.1016/j.fsi.2017.03.035
Ibrahem M. D. (2015). Evolution of probiotics in aquatic world: Potential effects, the current status in Egypt and recente prospectives. J. Advanced Res. 6, 765–791. doi: 10.1016/j.jare.2013.12.004
Infante J. L. Z., Cahu C. L. (2001). Ontogeny of the gastrointestinal tract of marine fish larvae. Comp. Biochem. Physiol. Part C Toxicol. Pharmacol. 130, 477–487. doi: 10.1016/S1532-0456(01)00274-5
Iqbal K. J., Ashraf M., Javid A., Khan N., Abbas F., Hafeez-Ur-Rehman M., et al. (2016). Effect of different plant and animal origin (fishmeal) feeds on digestive enzyme activity and haematology of juvenile Labeo rohita. Pak. J. Zool. 48, 201–207.
Iribarren D., Dagá P., Moreira M. T., Feijoo G. (2012). Potential environmental effects of probiotics used in aquaculture. Aquacult Int. 20, 779–789. doi: 10.1007/s10499-012-9502-z
Jena N. R. (2012). DNA damage by reactive species: mechanisms, mutation and repair. J. Biosci. 37, 503–517. doi: 10.1007/s12038-012-9218-2
Jeney G., Jeney Z. S. (2002). Application of immunostimulants for modulation of the non-specific defense mechanisms in sturgeon hybrid: Acipenser ruthenus 9 A. baerii. J. Appl. Icht 18, 416–419. doi: 10.1046/j.1439-0426.2002.00405.x
Jesus G. F. A., Pereira S. A., Pereira G. V., Silva. B. C., Martins M. L., Mouriño J. L. P. (2016). Probióticos na piscicultura (Tubarão, Santa Catarina: Aquaculture Brasil).
Ji Y., Qiu J. B., Xie T., McCarron P., Li A. F. (2018). Accumulation and transformation of azaspiracids in scallops (Chlamys farreri) and mussels (Mytilus galloprovincialis) fed with Azadinium poporum, and response of antioxidant enzymes. Toxicon. 143, 20–28. doi: 10.1016/j.toxicon.2017.12.040
Kar N., Ghosh K. (2008). Enzyme producing bacteria in the gastrointestinal tracts of Labeo rohita (Hamilton) and Channa punctatus (Bloch). Turk. J. Fish. Aquat. Sci. 1, 115–120.
Kavitha M., Raja M., Perumal P. (2018). Evaluation of probiotic potential of Bacillus spp. isolated from the digestive tract of freshwater fish Labeo calbasu (Hamilto). Aquac. Rep. 11, 59–69. doi: 10.1016/j.aqrep.2018.07.001
Kong Y., Li M., Chu G., Liu H., Shan X., Wang G., et al. (2021). The positive effects of single or conjoint administration of lactic acid bacteria on Channa argus: Digestive enzyme activity, antioxidant capacity, intestinal microbiota and morphology. Aquaculture 531, 735852. doi: 10.1016/j.aquaculture.2020.735852
Kuebutornye F. K. A., Abarike E. D., Lu Y. (2019). A review on the application of Bacillus as probiotics in aquaculture. Fish Shellfish Immunol. 87, 820–828. doi: 10.1016/j.fsi.2019.02.010
Kumar P., Jain K. K., Sardar P. (2018). Effects of dietary symbiotic on innate immunity, antioxidant activity and disease resistance of Cirrhinus mrigala juveniles. Fish Shellfish Immunol. 80, 124–132. doi: 10.1016/j.fsi.2018.05.045
Landers T. F., Cohen B., Wittum T. E., Larson E. L. (2012). A review of antibiotic use in food animals: perspective, policy, and potential. Public Health Rep. 127, 4–22. doi: 10.1177/003335491212700103
Li H., Jiang W., Liu Y., Jiang J., Zhang Y., Wu P., et al. (2016). The metabolites of glutamine prevent hydroxyl radical-induced apoptosis through inhibiting mitochondria and calcium ion involved pathways in fish erythrocytes. Free Radic. Biol. Med. 92, 126–140. doi: 10.1016/j.freeradbiomed.2016.01.007
Li X., Ringø E., Hoseinifar S. H., Lauzon H., Birkbeck H., Yang D. (2019). Adherence and colonisation of microorganisms in the fish gastrointestinal tract. Rev. Aquacult. 11, 603–618. doi: 10.1111/raq.12248
Lin Y., Saputra F., Chen Y., Hu S. (2019). Dietary administration of Bacillus amyloliquefaciens R8 reduces hepatic oxidative stress and enhances nutrient metabolism and immunity against Aeromonas hydrophila and Streptococcus agalactiae in zebrafish (Danio rerio). Fish Shellfish Immunol. 86, 410–419. doi: 10.1016/j.fsi.2018.11.047
Lin M.-Y., Yen C.-L. (1999). Antioxidative ability of lactic acid bacteria. J. Agric. Food Chem. 47, 1460–1466. doi: 10.1021/jf981149l
Linh N. T. H., Sakai K., Taoka Y. (2018). Screening of lactic acid bacteria isolated from fermented food as potential probiotics for aquacultured carp and amberjack. Fisheries Science. 84, 101–111. doi: 10.1007/s12562-017-1150-9
Liu C. H., Chiu C. S., Ho P. L., Wang S. W. (2009). Improvement in the growth performance of white shrimp, Litopenaeus vannamei, by a protease-producing probiotic, Bacillus subtilis E20, from natto. J. Appl. Microbiol. 107, 1031–1041. doi: 10.1111/j.1365-2672.2009.04284.x
Liu M., Tang L., Hu C., Huang Z., Sun B., Lam J. C. W., et al. (2021). Antagonistic interaction between perfluorobutanesulfonate and probiotic on lipid and glucose metabolisms in the liver of zebrafish. Aquat. Toxicol. 237, 105897. doi: 10.1016/j.aquatox.2021.105897
Liu H., Wang S., Cai Y., Guo X., Cao Z., Zhang Y., et al. (2017). Dietary administration of Bacillus subtilis HAINUP40 enhances growth, digestive enzyme activities, innate immune responses and disease resistance of tilapia, Oreochromis niloticus. Fish Shellfish Immunol. 60, 326–333. doi: 10.1016/j.fsi.2016.12.003
Liu G., Ye Z., Liu D., Zhao J., Sivaramasamy E., Deng Y., et al. (2018). Influence of stocking density on growth, digestive enzyme activities, immune responses, antioxidant of Oreochromis niloticus fingerlings in biofloc systems. Fish Shellfish Immunol. 81, 416–422. doi: 10.1016/j.fsi.2018.07.047
Liu G., Zhu S., Liu D., Guo X., Ye Z. (2017). Effects of stocking density of the white shrimp Litopenaeus vannamei (Boone) on immunities, antioxidant status, and resistance against Vibrio harveyi in a biofloc system. Fish Shellfish Immunol. 67, 19–26. doi: 10.1016/j.fsi.2017.05.038
Llewellyn M. S., Boutin S., Hoseinifar S. H., Derome N. (2014). Teleost microbiomes: the state of the art in their characterization, manipulation and importance in aquaculture and fisheries. Front. Microbiol. 5. doi: 10.3389/fmicb.2014.00207
Mahious A. S., Van Loo J., Ollevier F. (2006). “Impact of the prebiotics, inulin and oligofructose on microbial fermentation in the spiral valve of Siberian sturgeon (Acipenser baerii),” in Proceedings of the World Aquaculture Society Meeting (World Aquaculture Society, Florence, Italy), 709.
Malhotra J. D., Kaufman R. J. (2007). Endoplasmic reticulum stress and oxidative stress: a vicious cycle or a double edged sword? Antioxid. Redox Signal. 9 (12), 2277–2293. doi: 10.1089/ars.2007.1782
Mardani T., Khiabani M. S., Mokarram R. R., Hamishehkar H. (2018). Immobilization of α-amylase on chitosan-montmorillonite nanocomposite beads. Int. J. Biol. Macromol. 120 (Pt A), 354–360. doi: 10.1016/j.ijbiomac.2018.08.065
Marlida R., Suprayudi M. A., Widanarni, Harris E. (2014). Growth, digestive enzyme activity and health status of humpback grouper (Cromileptes altivelis) fed with synbiotic. Pak. J. Nutr. 13, 319–326. doi: 10.3923/pjn.2014.319.326
Mata-Sotres J. A., Moyano F. J., Martínez-Rodríguez G., Yúfera M. (2016). Daily rhythms of digestive enzyme activity and gene expression in gilthead seabream (Sparus aurata) during ontogeny. Comp. Biochem. Physiol. Part A Mol. Integr. Physiol. 197, 43–51. doi: 10.1016/j.cbpa.2016.03.010
Mehrabi Z., Firouzbakhsh F., Jafarpour A. (2012). Effects of dietary supplementation of symbiotic on growth performance, serum biochemical parameters and carcass composition in rainbow trout (Oncorhynchus mykiss) fingerlings. J. Anim. Physiol. Anim. Nutr. 96, 474–481. doi: 10.1111/j.1439-0396.2011.01167.x
Merrifield D. L., Balcazar J. L., Daniels C., Zhou Z., Carnevali O., Sun Y.-Z., et al. (2014). “Indigenous lactic acid bacteria in fish and crustaceans,” in Aquaculture Nutrition: gut health, probiotics and prebiotics, vol. 2014 . Eds. Merrifield D., Ringø E. (Wiley-Blackwell Publishing, Oxford), 128–168.
Mirghaed A. T., Yarahmadi P., Hosseinifar S. H., Tahmasebi D., Gheisvandi N., Ghaedi A. (2018). The effects singular or combined administration of fermentable fiber and probiotic on mucosal immune parameters, digestive enzyme activity, gut microbiota and growth performance of Caspian white fish (Rutilus frisii kutum) fingerlings. Fish Shellfish Immunol. 77, 194–199. doi: 10.1016/j.fsi.2018.02.007
Mohajer Esterabadi M., Vahabzadeh H., Zamani A. A., Soudagar M., Ghorbani Nasrabadi R. (2010). Effect of dietary immunogen prebiotics on growth and survival indices of giant sturgeon (Huso huso Linne 1758) juveniles. J. Fisheries 4, 610–672.
Mohammadi G., Rafiee G., Tavabe K. R., Abdel-Latif H. M., Dawood M. A. (2021). The enrichment of diet with beneficial bacteria (single- or multi- strain) in biofloc system enhanced the water quality, growth performance, immune responses, and disease resistance of Nile tilapia (Oreochromis niloticus). Aquaculture 539, 736640. doi: 10.1016/j.aquaculture.2021.736640
Mohammadian T., Nasirpour M., Tabandeh M. R., Mesbah M. (2019). Synbiotic effects of β-glucan, mannan oligosaccharide and Lactobacillus casei on growth performance, intestine enzymes activities, immune-hematological parameters and immune-related gene expression in common carp, Cyprinus carpio: An experimental infection with Aeromonas hydrophila. Aquaculture 511, 634197. doi: 10.1016/j.aquaculture.2019.06.011
Mohapatra S., Chakraborty T., Kumar V., DeBoeck V., Mohanta K. N. (2013). Aquaculture and stress management: a review of probiotic intervention. J. Anim. Physiol. Anim. Nutr. 97, 405–430. doi: 10.1111/j.1439-0396.2012.01301.x
Mounir M., Ibijbijen A., Farih K., Rabetafika H. N., Razafindralambo H. L. (2022). Synbiotics and their antioxidant properties, mechanisms, and benefits on human and animal health: A narrative review. Biomolecules 12 (10), 1443. doi: 10.3390/biom12101443
Mukherjee A., Chandra G., Ghosh K. (2019). Single or conjoint application of autochthonous Bacillus strains as potential probiotics: Effects on growth, feed utilization, immunity and disease resistance in Rohu, Labeo rohita (Hamilton). Aquaculture 512, 734302. doi: 10.1016/j.aquaculture.2019.734302
Munir M. B., Hashim R., Abdul Manaf M. S., Mohd Nor S. A. (2016). Dietary prebiotics and probiotics influence the growth performance, feed utilisation, and body indices of snakehead (Channa striata) fingerlings. Trop. Life Sci. Res. 27, 111–125. doi: 10.21315/tlsr2016.27.2.9
Munir M. B., Hashim R., Nor S. A. M., Marsh T. L. (2018). Effect of dietary prebiotics and probiotics on snakehead (Channa striata) health: Haematology and disease resistance parameters against aeromonas hydrophila. Fish Shellfish Immunol. 75, 99–108. doi: 10.1016/j.fsi.2018.02.005
Muñoz-Atienza E., Gómez-Sala B., Araújo C., Campanero C., del Campo R., Hernández P. E., et al. (2013). Antimicrobial activity, antibiotic susceptibility and virulence factors of Lactic Acid Bacteria of aquatic origin intended for use as probiotics in aquaculture. BMC Microbiol. 13, 15. doi: 10.1186/1471-2180-13-15
Murashita K., Fukasa H., Takahashi N., Hosomi N., Matsunari H., Furuita H., et al. (2015). Effect of feed ingredients on digestive enzyme secretion in fish. Bull. Fish. Res. Agen. 40, 69–74.
Murashita K., Matsunari H., Furuita H., Rønnestad I., Oku H., Yamamoto T. (2018). Effects of dietary soybean meal on the digestive physiology of red seabream Pagrus major. Aquaculture 493, 219–228. doi: 10.1016/j.aquaculture.2018.05.005
Naidu S. K. (2011). Characterization and purification of protease enzyme. J. Appl. Pharm. Sci. 1, 107–112.
Narra M. R., Rajender K., Rudra Reddy R., Rao J. V., Begum G. (2015). The role of vitamin C as antioxidant in protection of biochemical and haematological stress induced by chlorpyrifos in freshwater fish Clarias batrachus. Chemosphere 132, 172–178. doi: 10.1016/j.chemosphere.2015.03.006
Nayak S. (2010). Probiotics and immunity: A fish perspective. Fish Shellfish Immunol. 29, 2–14. doi: 10.1016/j.fsi.2010.02.017
Nedaei S., Noori A., Valipour A., Khanipour A. A., Hoseinifar S. H. (2019). Effects of dietary galactooligosaccharide enriched commercial prebiotic on growth performance, innate immune response, stress resistance, intestinal microbiota and digestive enzyme activity in Narrow clawed crayfish (Astacus leptodactylus Eschscholtz, 1823). Aquaculture. 499, 80–89. doi: 10.1016/j.aquaculture.2018.08.076
Newaj-Fyzul A., Al-Harbi A., Austin B. (2014). Review: Developments in the use of probiotics for disease control in aquaculture. Aquaculture 431, 1–11. doi: 10.1016/j.aquaculture.2013.08.026
Nie L., Zhou Q., Qiao Y., Chen J. (2017). Interplay between the gut microbiota and immune responses of ayu (Plecoglossus altivelis) during Vibrio Anguillarum infection. Fish Shellfish Immunol. 68, 479–487. doi: 10.1016/j.fsi.2017.07.054
Olmos-Soto J. (2014). Bacillus subtilis a potential probiotic bacterium to formulate functional feeds for aquaculture (HTTPS://doi.org/). J. Microb. Biochem. Technol. 6, 361–365. doi: 10.4172/1948-5948.1000169
Paixão A. E. M., Santos J. C., Pinto M. S., Pereira D. S. P., Ramos C. E. C. O., Cerqueira R. B., et al. (2017). Effect of commercial probiotics (Bacillus subtilis and Saccharomyces cerevisiae) on growth performance, body composition, hematology parameters, and disease resistance against Streptococcus agalactiae in tambaqui (Colossoma macropomum). Aquacult Int. 25, 2035–2045. doi: 10.1007/s10499-017-0173-7
Panigrahi A., Azad I. S. (2007). Microbial intervention for better fish health in aquaculture: the Indian scenario. Fish Physiol. Biochem. 33, 429–440. doi: 10.1007/s10695-007-9160-7
Parolini M., Iacobuzio R., De Felice B., Bassano B., Pennati R., Saino N. (2019). Age- and sex-dependent variation in the activity of antioxidant enzymes in the brown trout (Salmo trutta) ). Fish Physiol. Biochem. 45, 145–154. doi: 10.1007/s10695-018-0545-6
Pittman K., Yúfera M., Pavlidis M., Geffen A. J., Koven W., Ribeiro L., et al. (2013). Fantastically plastic: fish larvae equipped for a new world. Rev. Aquac. 5, S224–S267. doi: 10.1111/raq.12034
Prego-Faraldo M. V., Vieira L. R., Eirin-Lopez J. M., Mendez J., Guilhermino L. (2017). Transcriptional and biochemical analysis of antioxidant enzymes in the mussel Mytilus galloprovincialis during experimental exposures to the toxic dinoflagellate Prorocentrum lima. Mar. Environ. Res. 129, 304–315. doi: 10.1016/j.marenvres.2017.06.009
Pryor G. S., Royes J. B., Chapman F. A. (2003). Mannan oligosaccharides in fish nutrition: effects of dietary supplementation on growth and gastrointestinal villi structure in gulf of mexico sturgeon. N. Am. J. Aquacult. 5, 106–111.
Puvanasundram P., Chong C. M., Sabri S., Yusoff M. S., Karim M. (2021). Multi-strain probiotics: Functions, effectiveness and formulations for aquaculture applications. Aquaculture Rep. 21, 100905. doi: 10.1016/j.aqrep.2021.100905
Ray A. K., Ghosh K., Ringø E. (2012). Enzyme-producing bacteria isolated from fish gut: a review. Aquac. Nutr. 18, 465–492. doi: 10.1111/j.1365-2095.2012.00943.x
Reda R. M., El-Hady M., Selim K. M., El-Sayed H. M. (2018). Comparative study of three predominant gut Bacillus strains and a commercial B. Amyloliquefaciens as probiotics on the performance of Clarias gariepinus. Fish Shellfish Immunol. 80, 416–425. doi: 10.1016/j.fsi.2018.06.031
Ribas L., Piferrer F. (2014). The zebrafish (Danio rerio) as a model organism, with emphasis on applications for finfish aquaculture research. Rev. Aquaculture 6 (4), 209–240. doi: 10.1111/raq.12041
Ringø E., Dimitroglou A., Hoseinifar S. H., Davies S. J. (2014). “Prebiotics in finfish: an update,” in Aquaculture nutrition: gut health, probiotics and prebiotics (Wiley-Blackwell Scientific Publication, Hoboken, NJ).
Ringø E., Harikrishnan R., Soltani M., Ghosh K. (2022). The effect of gut microbiota and probiotics on metabolism in fish and shrimp. Anim. Open Access J. From MDPI 12 (21), 3016. doi: 10.3390/ani12213016
Ringø E., Hoseinifar S. H., Ghosh K., Doan H., Van Beck B. R., Song S. K. (2018). Lactic acid bacteria in finfish—an update. Front. Microbiol. 9. doi: 10.3389/fmicb.2018.01818
Ringø E., Olsen R. E., Gifstad T. Ø., Dalmo R. A., Amlund H., Hemre G. I., et al. (2010). Probiotics in aquaculture: a review. Aquac. Nutr. 16 (2), 117–136.
Ringø E., Song S. K. (2016). Application of dietary supplements (synbiotics and probiotics in combination with plant products and β-glucans) in aquaculture. Aquac. Nutr. 22, 4–24. doi: 10.1111/anu.12349
Ringø E., Zhou Z., Vecino L. G., Wadsworth S., Romero J., Krogdahl Å., et al. (2016). Effect of dietary components on the gut microbiota of aquatic animals. A never-ending story? Aquaculture Nutr. 22, 219–282. doi: 10.1111/anu.12346
Rivera-Pérez C., Navarrete del Toro M. d. l. Á., García-Carreño F. L. (2010). Digestive lipase activity through development and after fasting and re-feeding in the whiteleg shrimp Penaeus vannamei. Aquaculture 300, 163–168. doi: 10.1016/j.aquaculture.2009.12.030
Roberfroid M. (2007). Prebiotics: the concept revisited1. J. Nutr. 137, 830S–837S. doi: 10.1093/jn/137.3.830S
Rodriguez-Estrada U., Satoh S., Haga Y., Fushimi H., Sweetman J. (2009). Effects of single and combined supplementation of Enterococcus faecalis, mannanoligosaccharide and polyhydrobutyric acid on growth performance and immune response of rainbow trout Oncorhynchus mykiss. Aquac Sci. 57, 609–617.
Rohani M. F., Islam S. M., Hossain M. K., Ferdous Z., Siddik M. A., Nuruzzaman M., et al. (2021). Probiotics, prebiotics and synbiotics improved the functionality of aquafeed: Upgrading growth, reproduction, immunity and disease resistance in fish. Fish Shellfish Immunol. 120, 569–589. doi: 10.1016/j.fsi.2021.12.037
Rungruangsak-Torrissen K., Stien L. H., Daae B. S., Vgseth T., Thorsheim G. B., Tobin D., et al. (2009). Different dietary levels of protein to lipid ratio affected digestive efficiency, skeletal growth, and muscle protein in rainbow trout families. Sch. Res. Exch. 2009, 1–13. doi: 10.3814/2009/709529
Rurangwa E., Laranja J. L., Van Houdt R., Delaedt Y., Geraylou Z., Van de Wiele T., et al. (2009). Selected nondigestible carbohydrates and prebiotics support the growth of probiotic fish bacteria mono-cultures in vitro. J. Appl. Microbiol. 106, 932–940. doi: 10.1111/jam.2009.106.issue-3
Sado R. Y., De Almeida Bicudo Á. J., Possebon Cyrino J. E. (2008). Feeding dietary mannan oligosaccharides to juvenile nile tilapia, Oreochromis niloticus, has no effect on hematological parameters and showed decreased feed consumption. J. World Aquacult. Soc 39 (6), 821–826. doi: 10.1111/j.1749-7345.2008.00219.x
Salem R., El-Habashi N., Fadl S. E., Sakr O. A., Elbialy Z. I. (2018). Effect of probiotic supplement on aflatoxicosis and gene expression in the liver of broiler chicken. Environ. Toxicol. Pharmacol. 60, 118–127. doi: 10.1016/j.etap.2018.04.015
Sankar H., Philip B., Philip R., Singh S. B. (2017). Effect of probiotics on digestive enzyme activities and growth of cichlids, Etroplus suratensis (Pearl spot) and Oreochromis mossambicus (Tilapia). Aquaculture Nutr. 23, 852–864. doi: 10.1111/anu.2017.23.issue-4
Schieber M., Chande N. S. (2014). ROS function in redox signalling and oxidative stress. Curr. Biol. Biol. 24, R453–R462. doi: 10.1016/j.cub.2014.03.034
Schmidt V. T., Smith K. F., Melvin D. W., Amaral-Zettler L. A. (2015). Community assembly of a euryhaline fish microbiome during salinity acclimation. Mol. Ecol. 24, 2537–2550. doi: 10.1111/mec.13177
Shaker Khoshroudi M. (2011). Effect of dietary mannan oligosaccharide on growth performance, survival, some haematological and serum biochemical parameters of grass carp (Ctenopharyngodon idella) juveniles (, Babol branch, Iran: Islamic Azad University), 57. M.A. thesis.
Simo´n R., Docando F., Nuñez-Ortiz N., Tafalla C., D´ıaz-Rosales P. (2021). Mechanisms used by probiotics to confer pathogen resistance to teleost fish. Front. Immunol. 12. doi: 10.3389/fimmu.2021.653025
Smith P., Willemsen D., Popkes M., Metge F., Gandiwa E., Reichard M., et al. (2017). Regulation of life span by the gut microbiota in the short-lived african turquoise killifish. eLife. 6, e27014. doi: 10.7554/eLife.27014.040
Solovyev M. M., Kashinskaya E. N., Izvekova G. I., Gisbert E., Glupov V. V. (2014). Feeding habits and ontogenic changes in digestive enzyme patterns in five freshwater teleosts. J. Fish Biol. 85, 1395–1412. doi: 10.1111/jfb.12489
Song S. K., Beck B. R., Kim D., Park J., Kim J., Kim H. D., et al. (2014). Prebiotics as immunostimulants in aquaculture: a review. Fish Shellfish Immunol. 40, 40–48. doi: 10.1016/j.fsi.2014.06.016
Sullam K. E., Essinger S. D., Lozupone C. A., Rosen G. L., Knight R., Kilham S. S., et al. (2012). Environmental and ecological factors that shape the gut bacterial communities of fish: A meta-analysis. Mol. Ecol. 21, 3363–3378. doi: 10.1111/j.1365-294X.2012.05552.x
Sumon M. S., Ahmmed F., Khushi S. S., Ahmmed M. K., Rouf M. A., Chisty M. A. H., et al. (2018). Growth performance, digestive enzyme activity and immune response of Macrobrachium rosenbergii fed with probiotic Clostridium butyricum incorporated diets. J. King Saud. Univ. - Sci. 30, 21–28. doi: 10.1016/j.jksus.2016.11.003
Suzer C., Çoban D., Kamaci H. O., Saka S., Firat K., Otgucuo˘glu O., et al. (2008). Lactobacillus spp. bacteria as probiotics in gilthead sea bream (Sparus aurata, L.) larvae: effects on growth performance and digestive enzyme activities. Aquaculture 280, 140–145. doi: 10.1016/j.aquaculture.2008.04.020
Ta’ati R., Soltani M., Bahmani M., Zamini A. A. (2011). Effects of the prebiotics Immunoster and Immunowall on growth performance of juvenile beluga (Huso huso). J. Appl. Icht 27, 796–798. doi: 10.1111/j.1439-0426.2010.01664.x
Takami G. A., Lovett D. L., Mirvaghefi A. R., Shakouri M. (2006). The effect of bacillus spp. bacteria used as probiotics on digestive enzyme activity, survival and growth in the indian white shrimp Fenneropenaeus indicus. Aquaculture 252, 516–524. doi: 10.1016/j.aquaculture.2005.07.021
ten Doeschate K., Coyne V. (2008). Improved growth rate in farmed Haliotis midae through probiotic treatment. Aquaculture 284, 174–179. doi: 10.1016/j.aquaculture.2008.07.018
Thakur S. (2012). Lipases, its sources, properties and applications: a review. Int. J. Sci. Eng. Res. 3, 1–29.
Torres D. E. (2014). Avaliação do efeito de microrganismos probióticos sobre o desempenho zootécnico, hematológico e tolerância ao estresse da truta arco-íris (Onchorhynchus mykiss). Mestrado em Biotecnologia Industrial) – Escola de Engenharia de Lorena, Universidade de São Paulo, Lorena.
Tovar D., Zambonino J., Cahu C., Gatesoupe F. J., Vázquez-Juárez R., Lésel R. (2002). Effect of live yeast incorporation in compound diet on digestive enzyme activity in sea bass (Dicentrarchus labrax) larvae. Aquaculture 204, 113–123.
Tovar-Ramírez D., Zambonino Infante J., Cahu C., Gatesoupe F., Vázquez-Juárez R. (2004). Influence of dietary live yeast on European sea bass (Dicentrarchus labrax) larval development. Aquaculture 234, 415–427. doi: 10.1016/j.aquaculture.2004.01.028
Tremaroli V., Bäckhed F. (2012). Functional interactions between the gut microbiota and host metabolism. Nature 489, 242–249. doi: 10.1038/nature11552
Trinder M., Bisanz J., Burton J., Reid G. (2015). ). Probiotic lactobacilli: a potential prophylactic treatment for reducing pesticide absorption in humans and wildlife. Benef Microbes 6, 841–847. doi: 10.3920/BM2015.0022
Tzivara A., Papatsiros V. G., Katsoudas V., Dritsa L., Katsoulos P. D., Evaggelidou M., et al. (2013). Residues depletion study and withdrawal period determination of sulphadiazine and trimethoprim premix in pigs. Am. J. Anim. Vet. Sci. 8, 37–44. doi: 10.3844/ajavsp.2013.37.44
Ullah A., Zuberi A., Ahmad M., Shah A. B., Younus N., Ullah S., et al. (2018). Dietary administration of the commercially available probiotics enhanced the survival, growth, and innate immune responses in Mori (Cirrhinus mrigala) in a natural earthen polyculture system. Fish Shellfish Immunol. 72, 266–272. doi: 10.1016/j.fsi.2017.10.056
Van Doan H., Hoseinifar S. H., Ringø E., Esteban M. A., Dadar M., Dawood M. A. O., et al. (2020). Host-associated probiotics: a key factor in sustainable aquaculture. Rev. Fish Sci. Aquac. 28 (1), 16–42. doi: 10.1080/23308249.2019.1643288
Van Doan H., Hoseinifar S. H., Tapingkae W., Tongsiri S., Khamtavee P. (2016). Combined administration of low molecular weight sodium alginate boosted immunomodulatory, disease resistance and growth enhancing effects of Lactobacillus plantarum in Nile tilapia (Oreochromis niloticus). Fish Shellfish Immunol. 58, 678–685. doi: 10.1016/j.fsi.2016.10.013
Verschuere L., Rombaut G., Sorgeloos P., Verstraete W. (2000). Probiotic bacteria as biological control agents in aquaculture. Microbiol. Mol. Biol. Rev. 64, 655–671. doi: 10.1128/MMBR.64.4.655-671.2000.Updated
Wang Y. (2007). Effect of probiotics on growth performance and digestive enzyme activity of the shrimp Penaeus vannamei. Aquaculture 269, 259–264. doi: 10.1016/j.aquaculture.2007.05.035
Wang Y. (2011). Use of probiotics Bacillus coagulans, Rhodopseudomonas palustris and Lactobacillus acidophilus as growth promoters in grass carp (Ctenopharyngodon idella) fingerlings. Aquaculture Nutr. 17, 372–378. doi: 10.1111/anu.2011.17.issue-2
Wang Y. B., Xu Z. R. (2006). Effect of probiotics for common carp (Cyprinus carpio) based on growth performance and digestive enzyme activities. Anim. Feed Sci. Technol. 127, 283–292.
Wang P., Chen S., Wei C., Yan Q., Sun Y.-Z., Yi G., et al. (2021). Monascus purpureus M-32 improves growth performance, immune response, intestinal morphology, microbiota and disease resistance in Litopenaeus vannamei. Aquaculture 530, 735947. doi: 10.1016/j.aquaculture.2020.735947
Wang Y., Hu S., Chiu C., Liu C. (2019). Multiple-strain probiotics appear to be more effective in improving the growth performance and health status of white shrimp, Litopenaeus vannamei, than single probiotic strains. Fish Shellfish Immunol. 84, 1050–1058. doi: 10.1016/j.fsi.2018.11.01784
Wang C., Liu Y., Sun G., Li X., Liu Z. (2019). Growth, immune response, antioxidant capability, and disease resistance of juvenile Atlantic salmon (Salmo salar L.) fed Bacillus velezensis V4 and Rhodotorula mucilaginosa compound. Aquacult. 500, 65–74. doi: 10.1016/j.aquaculture.2018.09.052
Wang C., Xie S., Zhu X., Lei W., Yang Y., Liu J. (2006). Effects of age and dietary protein level on digestive enzyme activity and gene expression of Pelteobagrus fulvidraco larvae. Aquaculture 254, 554–562. doi: 10.1016/j.aquaculture.2005.11.036
Wang C., Yue X., Lu X., Liu B. (2013). The role of catalase in the immune response to oxidative stress and pathogen challenge in the clam Meretrix meretrix. Fish Shellfish Immunol. 34, 91–99. doi: 10.1016/j.fsi.2012.10.013
Wang J., Zhao L., Liu J., Wang H., Xiao S. (2015). Effect of potential probiotic Rhodotorula benthica D30 on the growth performance, digestive enzyme activity and immunity in juvenile sea cucumber Apostichopus japonicus. Fish Shellfish Immunol. 43, 330–336. doi: 10.1016/j.fsi.2014.12.028
Watling L. (2015). “Feeding and digestive system,” in Functional Morphology and Diversity Eds. Watling L., Thiel M. (New York: The Natural History of the Crustacea). doi: 10.1093/acprof:osobl/9780195398038.003.0008
Welker T. L., Lim C., Yildirim-Aksoy M., Shelby R., Klesius P. H. (2007). Immune response and resistance to stress and Edwardsiella ictaluri challenge in channel catfish, Ictalurus punctatus, fed diets containing commercial whole-cell yeast or yeast subcomponents. J. World Aquacult. Soc 38 (1), 24–35. doi: 10.1111/j.1749-7345.2006.00070.x
Wu Z. X., Feng X., Xie L. L., Peng X. Y., Yuan J., Chen X. X. (2012). Effect of probiotic Bacillus subtilis Ch9 for grass carp, Ctenopharyngodon idella (Valenciennes 1844), on grwth performance, digestive enzyme activities and intestinal microflora. J. Appl. Ichthyol. 28, 721–727. doi: 10.1111/j.1439-0426.2012.01968.x
Wuertz S., Schroeder A., Wanka K. M. (2021). Probiotics in fish nutrition—Long-standing household remedy or native nutraceuticals? Water 13, 1348. doi: 10.3390/w13101348
Xia Y., Lu M., Chen G., Cao J., Gao F., Wang M., et al. (2018). Effects of dietary Lactobacillus rhamnosus JCM1136 and Lactococcus lactis subsp. Lactis JCM5805 on the growth, intestinal microbiota, morphology, immune response and disease resistance of juvenile Nile tilapia, Oreochromis niloticus. Fish Shellfish Immunol. 76, 368–379. doi: 10.1016/j.fsi.2018.03.020
Xie L. X., Chen S. Q., Yao C. R., Li D. P., Li L., Tang R. (2019). Nitrite induces endoplasmic reticulum stress and associates apoptosis of liver cells in grass carp (Ctenopharyngodon idella). Aquacult. 507, 275–281. doi: 10.1016/j.aquaculture.2019.04.016
Xiong B., Nie L., Chen J. (2019). Current understanding on the roles of gut microbiota in fish disease and immunity. Zoological Res. 40, 70–76. doi: 10.24272/j.issn.2095-8137.2018.069
Xu W., Lutz C. G., Taylor C. M., Ortega M. C. (2022). Improvement of fish growth and metabolism by oligosaccharide prebiotic supplement. Aquaculture Nutr. 2022, 5715649. doi: 10.1155/2022/5715649
Xu H.-M., Rong Y.-J., Zhao M.-X., Song B., Chi Z.-M. (2013). Antibacterial activity of the lipopetides produced by Bacillus amyloliquefaciens M1 against multidrug-resistant Vibrio spp. isolated from diseased marine animals. Appl. Microbiol. Biotechnol. 98, 127–136. doi: 10.1007/s00253-013-5291-1
Yan Q., Li J., Yu Y., Wang J., He Z., Van Nostrand J. D., et al. (2016). Environmental filtering decreases with fish development for the assembly of gut microbiota. Environ. Microbiol. 18, 4739–4754. doi: 10.1111/1462-2920.13365
Yanbo W., Zirong X., Wang Y. B., Xu Z. R. (2006). Effect of probiotics for common carp (Cyprinus carpio) based on growth performance and digestive enzyme activities. Anim. Feed Sci. Technol. 127, 283–292. doi: 10.1016/j.anifeedsci.2005.09.003
Yang Y. Z., Zhao Y., Yang L., Yu L. P., Wang H., Ji X. S. (2018). Characterization of 2-Cys peroxiredoxin 3 and 4 in common carp and the immune response against bacterial infection. Comp. Biochem. Physiol. Part B: Biochem. Mol. Biol. 217, 60–69. doi: 10.1016/j.cbpb.2017.12.012
Yasothai R. (2016). Antinutritional factors in soybean meal and its deactivation. Int. J. Sci. Environ. Technol. 5, 3793–3797.
Ye J. D., Wang K., Li F. D., Sun Y. Z. (2011). Single or combined effects of fructo- and mannan oligosaccharide supplements and Bacillus clausii on the growth, feed utilization, body composition, digestive enzyme activity, innate immune response and lipid metabolism of the Japanese flounder Paralichthys olivaceus. Aquac Nutr. 17, e902–e911. doi: 10.1111/anu.2011.17.issue-4
Yi Y., Zhang Z., Zhao F., Liu H., Yu L., Zha J., et al. (2018). Probiotic potential of Bacillus velezensis JW: antimicrobial activity against fish pathogenic bacteria and immune enhancement effects on Carassius auratus. Fish Shellfish Immunol. 78, 322–330. doi: 10.1016/j.fsi.2018.04.055
Yu L., Zhai Q., Zhu J., Zhang C., Li T., Liu X., et al. (2017). Dietary Lactobacillus plantarum supplementation enhances growth performance and alleviates aluminum toxicity in tilapia. Ecotoxicol Environ. Saf. 143, 307–314. doi: 10.1016/j.ecoenv.2017.05.023
Yu W., Yang Y., Zhou Q., Huang X., Huang Z., Li T., et al. (2022). Effects of dietary Astragalus polysaccharides on growth, health and resistance to Vibrio harveyi of Lates calcarifer. Int. J. Biol. Macromol. 207, 850–858. doi: 10.1016/j.ijbiomac.2022.03.176
Zapata A. A., Lara-Flores M. (2012). Antimicrobial activities of lactic acid bacteria strains isolated from nile tilapia intestine (Oreochromis niloticus). J. Biol. Life Sci. 4 (1), 164–171. doi: 10.5296/jbls.v4i1.2408
Zeng Y.-Y., Jiang W.-D., Liu Y., Wu P., Zhao J., Jiang J., et al. (2016). Optimal dietary alphalinolenic acid/linoleic acid ratio improved digestive and absorptive capacities and target of rapamycin gene expression of juvenile grass carp (Ctenopharyngodon idellus). Aquac. Nutr. 22, 1251–1266. doi: 10.1111/anu.2016.22.issue-6
Zhai Q., Wang H., Tian F., Zhao J., Zhang H., Chen W. (2017). Dietary Lactobacillus plantarum supplementation decreases tissue lead accumulation and alleviates lead toxicity in Nile tilapia (Oreochromis niloticus). Aquaculture Res. 48, 5094–5103. doi: 10.1111/are.2017.48.issue-9
Zhang C.-N., Li X.-F., Xu W.-N., Jiang G.-Z., Lu K.-L., Wang L. N., et al. (2013). Combined effects of dietary fructooligosaccharide and bacillus licheniformis on innate immunity, antioxidant capability and disease resistance of triangular bream (Megalobrama terminalis). Fish Shellfish Immunol. 35 (5), 1380–1386. doi: 10.1016/j.fsi.2013.07.047
Zhang C.-N., Tian H.-Y., Li X.-F., Zhu J., Cai D.-S., Xu C., et al. (2014). The effects of fructooligosaccharide on the immune response, antioxidant capability and HSP70 and HSP90 expressions in blunt snout bream (Megalobrama amblycephala) under high heat stress. Aquacult. 433, 458–466. doi: 10.1016/j.aquaculture.2014.07.007
Zhou X., Wang Y., Li W. (2009). Effect of probiotic on larvae shrimp (Penaeus vannamei) based on water quality, survival rate and digestive enzyme activities. Aquaculture 287, 349–353. doi: 10.1016/j.aquaculture.2008.10.046
Zokaeifar H., Balcázar J. L., Saad C. R., Kamarudin M. S., Sijam K., Arshad A., et al. (2012). Effects of Bacillus subtilis on the growth performance, digestive enzymes, immune gene expression and disease resistance of white shrimp, Litopenaeus vannamei. Fish Shellfish Immunol. 33, 683–689. doi: 10.1016/j.fsi.2012.05.027
Zoumpopoulou G., Kazou M., Alexandraki V., Angelopoulou A., Papadimitriou K., Pot B., et al. (2018). “Probiotics and prebiotics: an overview on recent trends,” in Probiotics and Prebiotics in Animal Health and Food Safety (Springer International Publishing, Cham), 1–34. doi: 10.1007/978-3-319-71950-4_1
Keywords: oxidative damage, antioxidant systems, digestive health, nutritional supplements, synbiotic effects, aquaculture
Citation: Amenyogbe E, Droepenu EK, Ayisi CL, Boamah GA, Duker RQ, Abarike ED and Huang J-s (2024) Impact of probiotics, prebiotics, and synbiotics on digestive enzymes, oxidative stress, and antioxidant defense in fish farming: current insights and future perspectives. Front. Mar. Sci. 11:1368436. doi: 10.3389/fmars.2024.1368436
Received: 15 January 2024; Accepted: 24 April 2024;
Published: 16 May 2024.
Edited by:
Yafei Duan, South China Sea Fisheries Research Institute, ChinaReviewed by:
Basanta Kumar Das, Central Inland Fisheries Research Institute (ICAR), IndiaSofia Priyadarsani Das, National Taiwan Ocean University, Taiwan
Copyright © 2024 Amenyogbe, Droepenu, Ayisi, Boamah, Duker, Abarike and Huang. This is an open-access article distributed under the terms of the Creative Commons Attribution License (CC BY). The use, distribution or reproduction in other forums is permitted, provided the original author(s) and the copyright owner(s) are credited and that the original publication in this journal is cited, in accordance with accepted academic practice. No use, distribution or reproduction is permitted which does not comply with these terms.
*Correspondence: Jian-sheng Huang, aHVhbmdqc0BnZG91LmVkdS5jbg==