- 1Fishery College, Jimei University, Xiamen, Fujian, China
- 2Coastal Protection Department, Shenzhen Mangrove Wetland Conservation Foundation, Shenzhen, Guangdong, China
- 3Quanzhou Bay Estuary Wetland Nature Reserve Development Center, Quanzhou, Fujian, China
Coastal wetlands are characterized by high production and thus play an important role in global climate change. In past decades, the invasion of Spartina alterniflora has caused many problems of coastal wetlands in southeastern China, and the restoration of such areas was mainly conducted by replacing Spartina alterniflora with mangrove plants. This may impact the carbon storage dynamics in such areas. In this study, stable isotopes (δ13C and δ15N) and molecular analysis were used to reveal the impact of artificial restoration on the carbon storage of Quanzhou Bay Estuary Wetland Natural Reserve. The major results are as follows: (1) the change in dominant plants results in a changing major source of soil organic matter, from external sources to mangrove plants; (2) the decrease in soil organic matter following the removal of Spartina alterniflora may be primarily caused by the loss of external organic matter, while the production of mangroves may offset such loss and enhance the content and stability of carbon storage over the long term; (3) microbial CO2 assimilation may serve as an alternative source of bioavailable carbon and thus support the activity of benthic community. Our results revealed the long-term benefits of such restoration on the carbon storage function of wetlands invaded by Spartina alterniflora. Furthermore, the integrating of isotopic tracers and molecular technology may provide new insights in understanding the response of the carbon storage in coastal areas to human activity.
1 Introduction
As one of the most productive ecosystems, coastal wetlands contribute greatly to blue carbon and serve as crucial habitats for numerous species, thus playing significant roles in global climate change and coastal biodiversity conservation (Pendleton et al., 2012; Alongi, 2014). However, in the past two decades, the invasion of Spartina alterniflora (S. alterniflora) has caused many problems in coastal wetlands, such as native plant degradation, reduced biological diversity and increased methane emissions (Liu et al., 2021; Qi and Chmura, 2023; Zheng et al., 2023). This highlights the urgent need for the protection of such impaired wetlands. To date, the most widely used path for the restoration of the invaded area is to replace the invasive S.alterniflora with mangrove plants (Feng et al., 2017; Cui et al., 2023). Nevertheless, this may produce profound effects on carbon storage of coastal regions, and subsequently impact coastal biomass and biodiversity, which largely rely on the bioavailability of organic matter (Kristensen et al., 2008).
As most of the CO2 fixed by mangrove plants is stored in soil (Kristensen et al., 2008; Fourqurean et al., 2012), the replacement of the dominant plant, from S. alterniflora to mangrove plants, may produce significant effects on the content and the stability of soil organic matter (SOM). This can be attributed to (1) the differences in CO2 fixation capacity and allocation mechanisms between mangrove plants and S.alterniflora (Regnier et al., 2013; Kuwae et al., 2016; Osland et al., 2018), and (2) the different C: N ratio of photosynthetic products derived from mangrove plants and S.alterniflora (Xia et al., 2021; Li et al., 2023). Currently, many attentions have been paid to the impact of S.alterniflora on SOM (Graça et al., 2000; Feng et al., 2017; Qi and Chmura, 2023). As one of the most productive species in coastal areas, S. alterniflora has been reported to have obvious contribution to SOM (Zhang et al., 2010; Qi and Chmura, 2023). In addition, the photosynthetic products derived from S.alterniflora is characterized by lower C: N ratio and tannin contents than that derived from mangrove plants (Feng et al., 2015; Wu, 2018; Gao, 2019), thus has higher bioavailability. Therefore, it remains unclear whether the restoration by replacing S.alterniflora with mangrove plants would benefit the carbon storage function and the biodiversity in coastal areas over long-term period. Apart from dominant plants, it is also necessary to consider the influence of microbial CO2 assimilation in coastal carbon storage dynamics. Microbial CO2 assimilation has been reported in many areas, such as forest soils (Spohn et al., 2019), agricultural soils (Miltner et al., 2005; Wu et al., 2015), paddy soils (Yuan et al., 2012; Wu et al., 2014; Ge et al., 2016), and wetland soils (Beulig et al., 2015; Nowak et al., 2015; Lynn et al., 2017), contributing 0.9 and 5.4 Pg C yr−1 globally (Yuan et al., 2012). However, the simultaneous research of the CO2 fixation by dominant plants and benthic microbes in coastal wetlands is scarce.
To assess the impact of the artificial restoration on coastal carbon storage and benthic communities, a comprehensive study was conducted in Quanzhou Bay wetlands, a region invaded by S.alterniflora for many years. Since 2022, the S.alterniflora here was removed and replaced by mangrove plants, this may provide an ideal site for assessing the effects of wetland restoration. During our investigation, the stable isotopes (δ13C, δ15N) were used to determine the potential changes in the source of SOM (Phillips and Gregg, 2003; Philp, 2007; Xia et al., 2021). DNA sequencing and quantitative real-time PCR (qRT-PCR) technologies were used to analyze benthic biomass and composition, as well as the activity of functional genes for benthic microbial CO2 fixation. Additionally, considering the active nitrogen cycle processes reported in coastal wetlands (Reis et al., 2019; Wang et al., 2022), the activity of functional genes associated with benthic nitrogen cycle were also examined as indicators for the microbial response to the restoration. The aims of this study are: 1) to observe the variations in mangrove’s contribution versus S. alterniflora contribution to total SOM, 2) to reveal the long-term effects of the restoration on carbon storage and its subsequent impacts on benthic communities, and 3) to identify the role of microbial CO2 fixation during restoration. Our results will build upon previous knowledge of coastal carbon storage and provide new insights in understanding the response of coastal areas to human activity.
2 Materials and methods
2.1 Study area
Quanzhou Bay is an open bay in Fujian Province, southeastern China. It is fringed by a large area of wetlands and was designated as the Quanzhou Bay Estuary Wetland Natural Reserve in 2003. Since 1984, the invasion of S.alterniflora has been observed here, which caused many problems in the following decades, such as the degradation of native mangrove community, the decrease in benthic biomass and the loss of bird habitat (Wang et al., 2008; Cui et al., 2023; Yang et al., 2014). To prevent the further degradation of native community, the restoration by replacing the invasive S.alterniflora with mangrove plants has been carried out since 2022. S.atlerniflora in the study area was removed by mowing and plowing in later September 2022 and replaced by mangrove plants in early November 2022 (Figure 1), the details of mangrove plants are shown in Supplementary Table S1.
2.2 Sample collection
Field samplings were carried out in September 2022 (Sep 2022), October 2022 (Oct 2022), December 2022 (Dec 2022), March 2023 (Mar 2023), June 2023 (Jun 2023) and November 2023 (Nov 2023). The samplings in Oct 2022 and Dec 2022 were carried out about 10 d after the clear of S.alterniflora and the plants of mangrove seedlings. The sampled stations are shown in Figure 1, among with the control station was located in mudflat and was without any plants throughout the investigation. In order to avoid the residual signal of the S.alterniflora burned deeply in situ, surface soil (~5 cm) were collected with a sampling tube (2.9 cm inner diameter) to detect the content and the δ13C and δ15N of SOM (δ13CSOM, δ15NSOM). Samples of the neighbored seawater and plants (S.alterniflora and Kandelia candai) were also collected for the detecting of δ13C and δ15N. The above samples were all kept at −20 °C before analysis. Another three replicates of 30 g surface soil were collected and homogenized under anoxic conditions. Subsamples of 5 g soil were transferred to 50 mL tubes, cooled with dry ice and transported under an Ar atmosphere to the laboratory for molecular analyses.
2.3 Content and isotopic values of organic matter
Triplicate soil samples were collected in a 1 m × 1 m square at each station and mixed evenly before analysis. Suspend organic matters in water samples were collected by filtering 4 L of water through GF-75 filters (47mm, 0.3 μm pore size). The filters were previously combusted for 4 h at 450°C to remove organic matter. All water samples were then filtered through a 200 μm mesh sieve to remove large detritus. In the laboratory, the collected soil, plants and membrane samples were treated with HCl vapor (48 h) to remove inorganic carbon and then dried at 60°C. The dried samples were then fully pulverized, the contents of total organic carbon (TOC). total nitrogen (TN), and the δ13C and δ15N values of the samples were then measured using a Finnigan Delta V Advantage isotope ratio mass spectrometer interfaced with a Carlo Erba NC 2500 elemental analyzer, with an analytical precision <0.2‰.
2.4 DNA sampling, extraction, amplification and sequencing
The biomass and the composition of benthic microbes, and the abundance functional genes for microbial CO2 fixation (cbbM and cbbL) were observed in Nov 2023. Generally, soil genomic DNA was extracted using E.Z.N.A. Soil DNA Kit (Omega Bio-tek, Inc., USA), the concentration and quality of the genomic DNA were checked by NanoDrop 2000 spectrophotometer (Thermo Scientific Inc., USA). The V3-4 hypervariable region of bacterial 16S rRNA gene were amplified with the universal primer 338F (5’-ACTCCTACGGGAGGCAGCAG-3’) and 806R (5’-GGACTACNNGGGTATCTAAT-3’). For each sample, 8-digit barcode sequence was added to the 5’ end of the forward and reverse primers (Allwegene Company, Beijing). The PCR was carried out on a ABI 9700 PCR instrument (Applied Biosystems, USA) using 25 μL reaction volumes, containing 12.5 μL 2×Taq PCR MasterMix (Vazyme Biotech Co.,Ltd, China), 3 °C μL (2ng μL-1), 1 μL Forward Primer(5 μM)、1 μL Reverse Primer (5 μM), 2 μL template DNA, and 5.5 μL ddH2O. Cycling parameters were 95 °C for 5 min, followed by 28 cycles of 95 °C for 45 s, 55 °C for 50 s and 72 °C for 45 s with a final extension at 72 °C for 10 min. The PCR products were purified using a Agencourt AMPure XP Kit (Beckman Coulter, Inc., USA). Sequencing libraries were generated using NEB Next Ultra II DNA Library Prep Kit (New England Biolabs, Inc., USA) following the manufacturer’s recommendations. The library quality was assessed by Nanodrop 2000 (ThermoFisher Scientific, Inc., USA), Agilent 2100 Bioanalyzer (Agilent Technologies, Inc., USA), and ABI StepOnePlus Real Time PCR System (Applied Biosystems, Inc., USA), successively. Deep sequencing was performed on Illumina Miseq/Nextseq 2000/Novaseq 6000 (Illumina, Inc., USA) platform at Beijing Allwegene Technology Co., Ltd. After the run, image analysis, base calling and error estimation were performed using Illumina Analysis Pipeline Version 2.6 (Illumina, Inc., USA).
Qualified sequences were clustered into operational taxonomic units (OTUs) at a similarity threshold of 97% use Uparse algorithm of Vsearch (v2.7.1) software. The BLAST tool was used to classify all OTU representative sequences into different taxonomic groups against Silva138 and GenBank non redundant nucleus database(nt) Database, and e-value threshold was set to1e-5. QIIME (v1.8.0) was used to generate rarefaction curves and to calculate the richness and diversity indices based on the OTU information. Based on the results of taxonomic annotation and relative abundance, R (v3.6.0) was used for bar-plot diagram analysis.
2.5 The expression of functional genes
The expression of functional genes for microbial CO2 fixation (cbbM, cbbL) and nitrogen cycle (nxrA, nxrB, AOA-amoA, AOB-amoA, nirS, nirK, nosZ, nrfA, narG, norB and nifH) was analyzed by quantitative real-time PCR (qRT-PCR). Generally, the total RNA samples were isolated by TRIzol reagent (TIANGEN BIOTECH, Beijing). Then the RNA purity and concentration was measured by using the NanoPhotometer spectrophotometer (IMPLEN, CA, USA). After detecting, cDNA was synthesized using 2 μg RNA using the PrimeScriptTM RT reagent Kit with gDNA Eraser (TaKaRa). The specific primer for function genes were designed using Primer 5.0 by Allwegene Technology (Allwegene Technology Co., Ltd. Beijing, China), the details are shown in Supplementary Table S2. The 16S rRNA was used as internal reference gene. qRT-PCR reaction was performed using SYBR® Premix Ex TaqTM II (Tli RNaseH Plus) and was conducted on ABI 7500 Real-time Detection System (Thermo Fisher Scientific, USA). The PCR reaction was carried out with the following reaction conditions: 95°C for 30 s; followed by 45 cycles of 95°C for 5 s, 60°C for 40 s. Samples for qRT-PCR were run in 3 biological replicates with 3 technical replicates and the data were represented as the mean ± SD (n = 3) for Student’s t-test analysis. The relative gene expression was calculated using the 2-△△CT algorithm (Pfaffl, 2001).
2.6 Statistical analysis
Pearson’s correlations analysis and ANOVA test were conducted using the Statistical Package for Social Sciences program (version 19.0) and R (v3.6.0). The source analysis of organic matters based on δ13C, δ15N values were conducted by IsoSource (version 1.3), with the increment of 1% and the tolerance of 0.1% (Phillips and Gregg, 2003). The cluster analysis of OTUs and the diversity analysis of benthic community were completed by Mothur(v1.48.0) and R(v3.6.0).
3 Results
3.1 The content and isotopic values of soil organic matter
The geo-chemical soil parameters are shown in Supplementary Table S3. The contents of the total organic carbon (TOC) and nitrogen (TN) in soil ranged from 0.17 ~ 1.89% (0.64 ± 0.27%, n = 36) and 0.02 ~ 0.15% (0.08 ± 0.03%, n = 36) during the investigation. Except for the maximum observed at A (1.89%) in Dec 2022, the contents of TOC at each stations decreased from Sep 2022 to Dec 2022 and recovered from Dec 2022 to Nov 2023, with less spatial variation (Figure 2A). Higher TN were also observed in Sep 2022, which decreased rapidly following the removal of S. alterniflora and slightly varied in the following months (Figure 2B). The C: NSOM ranged from 5.3 ~ 38.8 (10.4 ± 5.3, n = 36), which increased rapidly in Oct 2022, and kept high in the following investigation. The carbon and nitrogen storage in the surface 5 cm soil were observed by multiply the contents of TOC and TN with the bulk density (Supplementary Table S3). The surface carbon storage in the study area ranged from 106.1 ~ 656.9 t ha-1 (292.4 ± 120.1 t ha-1, n = 36), with similar temporal variation with TOC (Figure 2C). The surface nitrogen storage ranged from 2.3 ~ 13.6 t ha-1 (7.0 ± 2.8 t ha-1, n = 36), which decreased rapidly after the removal of S.alterniflora, and slightly increased in the following mouths (Figure 2D). Generally, the spatial variation of SOM content was not obvious throughout the investigation.
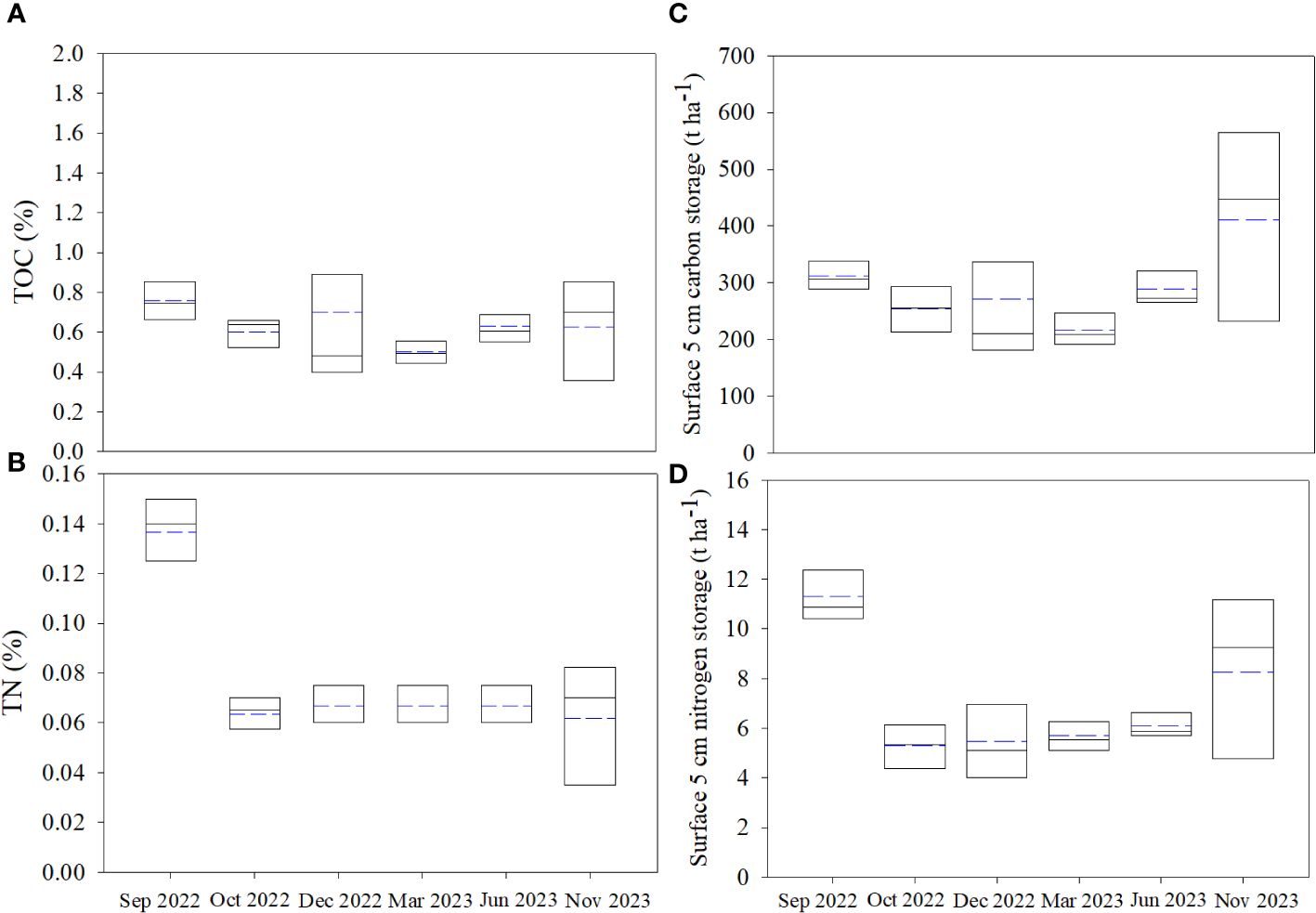
Figure 2 The variation of the carbon and nitrogen storage in the study area: (A) total organic carbon (TOC), (B) total nitrogen (TN), (C) surface carbon storage and (D) surface nitrogen storage. The solid and the dotted lines represent the median and mean respectively.
The δ13CSOM and δ15NSOM in the study area ranged from −28.60‰ ~ −20.96‰ (−23.15 ± 1.64‰, n = 36) and 4.03‰ ~ 14.42‰ (6.41 ± 2.57‰, n = 36) respectively. δ13CSOM varied slightly during the investigation (Figure 3), except for the minimum observed at A in Dec 2022 (−28.60‰). Whereas the δ15NSOM in Jun 2023 was obviously higher (Figure 3) than that in other seasons.
3.2 Benthic microbial biomass and composition
The absolute abundance of 16S rRNA, cbbM and cbbL ranged from 1.3×107 ~ 3.7×107 Copy g-1, 2.6×108 ~ 6.2×108 Copy g-1 and 8.9×106 ~ 1.1×107 Copy g-1 respectively. The abundance of 16S rRNA and cbbL has less spatial variation, while the abundance of cbbM at C, D and the control station are obviously higher than that at the other stations (Figure 4).
The composition of benthic microbes at phylum, class, order, family, genus and species level are shown in Figure 5. Most of the benthic microbes in the study area belonged to proteobacteria (Figure 5A), among which Gammaproteobacteria was the major one, accounting 22.7% ~ 29.7% (26.3 ± 2.8%, n=6) of the total biomass (Figure 5B). At the species level, most of the observed microbes were uncultured species, accounting more than 60% of the total biomass at all stations (Figure 5F). Cluster analysis of the observed OTUs indicates that the microbial composition at A and B are similar with each other, while those at D and E are more similar to that at the control station (Figure 5). However, the microbial diversity at the sampled stations has no obvious difference (Supplementary Table S4).
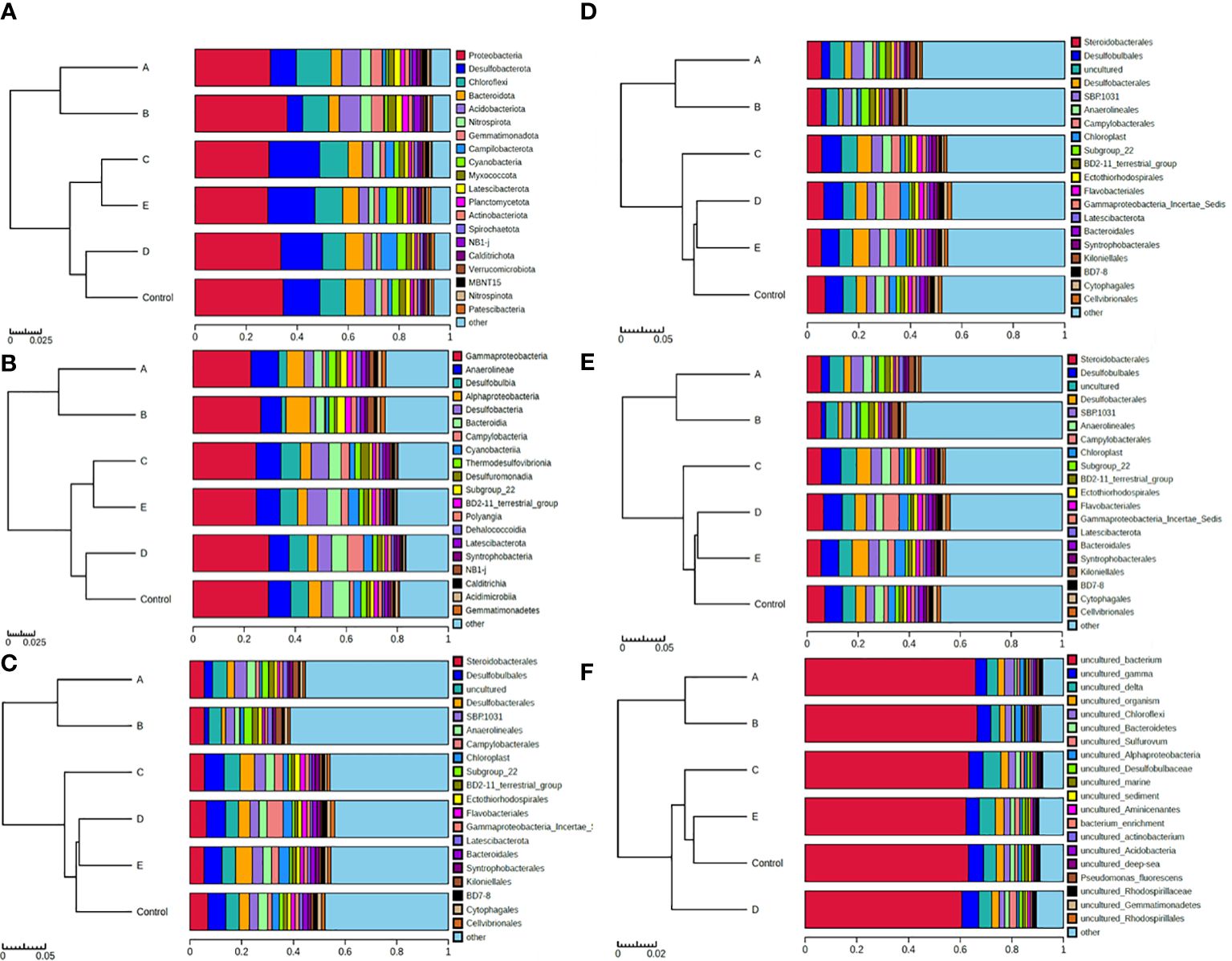
Figure 5 The composition of benthic microbes. (A-F) represents the result at phylum, class, order, family, genus and species level respectively.
3.3 The expression of functional genes for microbial CO2 fixation and nitrogen cycles
The relative expression of cbbL, cbbM, and functional genes for nitrogen cycle (nxrA, nxrB, AOA-amoA, AOB-amoA, nirS, nirK, nosZ, nrfA, narG, norB and nifH) was calculated by 2-△△CT algorithm. The ratio of the 2-△△CT observed at A ~ E to that at the control stations were used to indicate the spatial variation of the expression of the functional genes in the study area. The results are shown in Figure 6. Generally, the expression of cbbL at A ~ E were lower than that at the control station, while the expression of cbbM at A, B and E were higher (Figure 6A). The expression of functional genes for ammonia oxidation at A ~ E were lower than that at the control station (Figure 6B), while that for denitrification were obviously higher (Figure 6C). The maximum expression of nitrification and nitrogen fixation were observed at A, while that at the other stations were lower or similar to the control station (Figure 6D).
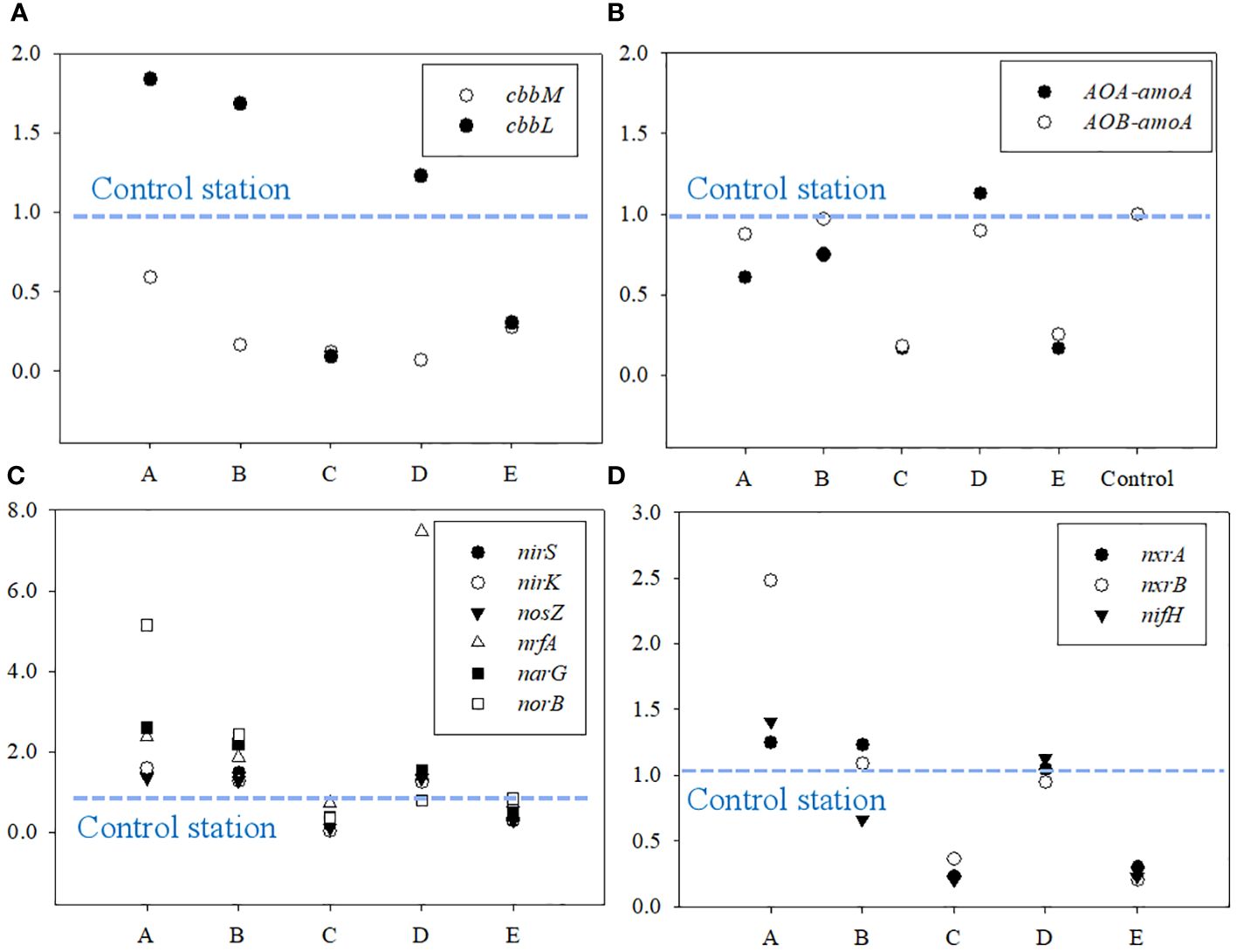
Figure 6 The relative expression activity of functional genes for (A) microbial CO2 fixation, (B) ammonia oxidation, (C) denitrification, and (D) nitrification and nitrogen fixation.
4 Discussion
4.1 The long-term benefits of artificial restoration on carbon storage
During our investigation, the carbon and nitrogen storage in the surface soil (5 cm) exhibited a rapid decrease following the removal of S.alterniflora, but gradual increased since the plant of mangroves (Figures 2C, D). At most of the sampled stations, the carbon storage observed in Nov 2023 were higher than that in Sep 2022. This indicate that mangrove plants may have greater contribution to SOM than S.alterniflora. Previous studies have reported that the production of C3 plants tend to exhibit greater isotopic fractionation between 12C and 13C (Reibach and Benedict, 1977; Roeske and O’Leary, 1984; Drake, 2014). Hence the photosynthetic products derived from C3 plants are usually characterized by lower δ13C than that derived from C4 plants. In this study, δ13CSOM was found to be negatively correlated with TOC (Figure 7). This also suggests that mangrove plants (C3 plants) may have replaced S.alterniflora (C4 plants) to be the dominant source of SOM in the study area. To test this hypothesis, δ13CSOM and δ15NSOM were used as indicators for tracing the source of SOM (Phillips and Gregg, 2003).
Apart from S. alterniflora and mangrove plants, the production of benthic algae and the imports of external organic matter (by seawater or freshwater) may also have great contribution to SOM (Zhang et al., 2010; Alongi, 2014; Gao, 2019). In addition, the study area was located in a region with a dense human population, the imports of anthropogenic sewage may also be considered (Bianchi, 2011; Li et al., 2023). The contribution of the above sources to total SOM was observed in three steps. First, the sources at A ~ E were divided into S.alterniflora, mangrove plants, and “other source”. The control station without S.alterniflora and any mangrove plants was set to observe the δ13C and δ15N of “other source” (Figure 1). Based on multiterminal element mixing method (two-terminal elements in Sep 2022 and Oct 2022), the contributions of the above sources to total SOM at A ~ E were observed. Then, the composition of the “other source”, which was mainly composed by benthic algae, seawater, freshwater and sewage, was analyzed by a four-terminal element mixing method. Finally, by synthesizing the results of the above steps, the contributions of different sources to total SOM were observed. The δ13C and δ15N of all end-elements are shown in Table 1, the contribution of mangroves plants was observed by summing the contribution of all species at each station (Supplementary Table S1).
The change in the major source of the SOM in the study area was as follows (Figure 8): (1) in Sep 2022, most of the SOM was derived from external sources, accounting for >50% of total SOM. Benthic algae was another important source, with a higher contribution (26.1 ~ 32.2%) than the dominant S. alterniflora (5.0 ~ 22.9%). (2) In Oct 2022, after the clearing of S. alterniflora, a simultaneous decrease in the contributions of S.alterniflora and seawater was observed, from 3.4 ~ 21.3% to 0.2 ~ 9.9% and from 21.7 ~ 26.3% to 16.6 ~ 18.4%, respectively. A considerable increasing contribution of sewage was also observed, from 3.1 ~ 4.6% to 30.9 ~ 34.2%. (3) From Dec 2022 to Jun 2023, a constantly increasing contribution of mangroves was observed. In Dec 2022, approximately 81.2% of total SOM at A was derived from mangrove plants. In addition, the contents of TOC (r=0.765, p<0.05, n=15) and TN (r=519, p<0.05, n=15) derived from mangrove plants were positively correlated with the individual numbers of mangrove plants (Supplementary Table S1). (4) In Nov 2023, mangrove plants was still the major source of SOM at all stations. Another point to note is that the contribution of S.alterniflora increased, which was similar with the contribution of mangroves at E. The signal of S.alterniflora was observed throughout the investigation. We propose that the relatively weaker signal observed in Oct 2022 may be derived from the residues of S.alterniflora buried in situ, while the stronger signal starting in Dec 2022 may indicate the possible recurrence of S. alterniflora, which was indeed observed in Jun 2023 and Nov 2023.
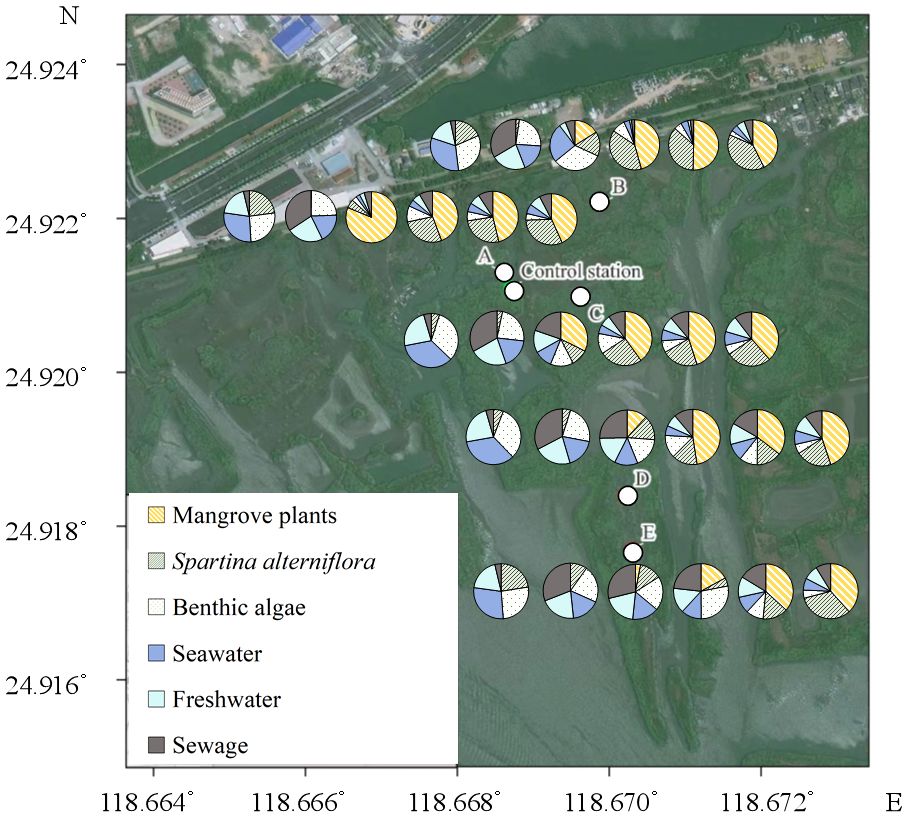
Figure 8 The contributions of different source to the total soil organic matter. The pies from left to right represent Sep 2022, Oct 2022, Dec 2022, Mar 2023, Jun 2023 and Nov 2023 respectively.
The increasing content of SOM indicates that the restoration by replacing the invasive S.alterniflora with mangrove plants may be beneficial for the carbon storage function in the study area. Though the signal of S.alterniflora can be observed throughout the investigation, its contribution to total SOM was not as high as except, even in Sep 2022, while the strong signal of mangrove plants was observed at all stations since Dec 2022 (Figure 8). This indicates that the direct contribution of mangrove plants to total SOM may be stronger than S.alterniflora. The most possible reason for this is the different carbon allocation of mangrove plants and S.alterniflora. It has been reported that more than half of the carbon fixed by mangrove plants may be conserved in soil (Alongi, 2014; Kristensen et al., 2008). However, most of the carbon fixed by S.alterniflora may be converted to its vegetation biomass, rather than being conserved in soil (Hemminga et al., 1996; Castañeda-Moya et al., 2013; Zheng et al., 2023). Even so, the potential contribution of S.alterniflora can not be ignored. In this study, the contribution of seawater decreased following the removal of S.alterniflora (Figure 8), suggesting the strong accumulation of external organic matters by the roots of S.alterniflora (Castañeda-Moya et al., 2013; Zheng et al., 2023). Hence, we propose that the decrease in the carbon and nitrogen storage after the removal of S. alterniflora from Sep 2022 to Oct 2022 may be mainly caused by the loss of external organic matters derived from seawater (Figure 8). However, such loss can be offset with the growth of mangrove plants, as the accumulation of external organic matters by the complex roots of mature mangrove plants may be stronger than that of S.alterniflora (Alongi, 2014; Kristensen et al., 2008). Another reason for the increasing carbon storage in the study area may be the different C:N ratio of organic matters derived from different source. It has been reported that organic matter derived from S.alterniflora and seawater has lower C:N ratio and tannin content than that derived from mangrove plants (Feng et al., 2015; Wu, 2018; Gao, 2019). This can be further confirmed by the increasing contribution of mangrove to SOM, and the correlations between the C: NSOM, TOC and δ13CSOM (Figure 7). Due to the biological preference for N during the utilization of organic matter, SOM with higher C:N ratio is usually characterized by lower bioavailability and higher stability (Dauwe and Middelburg, 1998; Jílková et al., 2020). Thus, the replacement of S.alterniflora by mangroves may simultaneously increase the content and the stability of SOM, and hence promote the carbon storage function of the study area in long term (Figure 9).
Generally, our results reveal the benefits of the restoration for the carbon storage function of coastal areas. However, the persistent signal of S.alterniflora reveals its long-term effect, highlighting the need for the long-term monitoring of the study area. Our results also indicate that δ13C and δ15N may be effective in the early warning of S.alterniflora invasion and sewage discharge, and may be helpful in the future restoration and monitoring of coastal wetlands.
4.2 Microbial CO2 fixation as an alternative source of bioavailable carbon
During our investigation, the negative correlation between the abundance of 16S rRNA and C: NSOM was observed in Nov 2023 (r=−0.950, p<0.01, n=6), suggesting that C: NSOM may be efficient in indicating the bioavailability of SOM. The difference in C: NSOM between A ~ E and the control station increased with the contribution of mangroves (r=0.472, p<0.01, n=30), indicating the significant contribution of organic matters with higher C: N ratio and tannin contents by mangrove plants. Therefore, we propose that higher abundance of 16S rRNA may be observed at stations with lower contribution of mangroves and higher contribution of seawater, algae or S.alterniflora. However, there was no statistically significant correlation between 16S rRNA abundance and the contribution ratio of the present source of SOM in 4.1. This suggests the existence of other potential sources of bioavailable organic matter for the growth and the activity of the benthic microbes in the study area, which mainly composed by heterotrophic proteobacteria (Figure 5). Microbial assimilation of CO2 may be the major one.
Microbial assimilation of CO2 is a ubiquitous process in soils (Berg, 2011; Wood et al., 1941), which has been widely reported (Beulig et al., 2015; Yuan et al., 2012; Nowak et al., 2015; Ge et al., 2016; Spohn et al., 2019). RubisCo is the key carboxylating enzyme for the CO2 fixation based on Calvin-Benson-Bassham cycles, and has been reported to be highly abundant in agricultural, forest, and wetlands (Nanba et al., 2004; Tolli and King, 2005; Nowak et al., 2015). Hence, the abundance of cbbL and cbbM, the functional genes for the form of RubisCO, was widely used to indicate the activity of benthic microbial CO2 fixation (Nanba et al., 2004; Tolli and King, 2005; Nowak et al., 2015). During our investigation, the activity of benthic nitrogen cycle were also observed by detecting the relative expression of the associated functional genes (Figure 6). The obvious sewage contribution to SOM (Figure 8) and the higher δ15NSOM observed in the study area suggests the potential active denitrification and ammonia oxidation in the study area (Craine et al., 2015; Denk et al., 2017), which have been widely reported in coastal areas under the effects of sewage (Reis et al., 2019; Wang et al., 2022). As most of the microbes associated with benthic nitrogen cycle are heterotrophic (Reis et al., 2019), its activity may largely reflect the bioavailability of organic matters in the study area. During our investigation, the abundance of cbbM was about 30 to 60 times higher than that of cbbL (Figure 4). When comparing the relative expression, the maximum expression of cbbL was observed at the control station, while that of cbbM was observed at A (Figure 6). Though there was no statistical correlation between 16S rRNA, cbbM and cbbL abundance, the significant correlations between the expression of cbbM and the activity of the functional genes for nitrogen cycle were observed (Figure 10). This suggests that microbial assimilation of CO2 may facilitate the benthic nitrogen cycle processes, thus may serve as an important source of bioavailable carbon for the benthic microbes in the study area. Therefore, we propose that though the removal of S.alterniflora may reduce the bioavailability of SOM, the CO2 fixed by benthic microbes may serve as an alternative source of bioavailable carbon and hence support the benthic community in the study area (Figure 9). However, the effects of microbial utilization of CO2 on the isotopic composition of SOM remains unclear at present, which need to be considered in future investigation to understand the contribution of microbial CO2 fixation to the carbon storage in the study area.
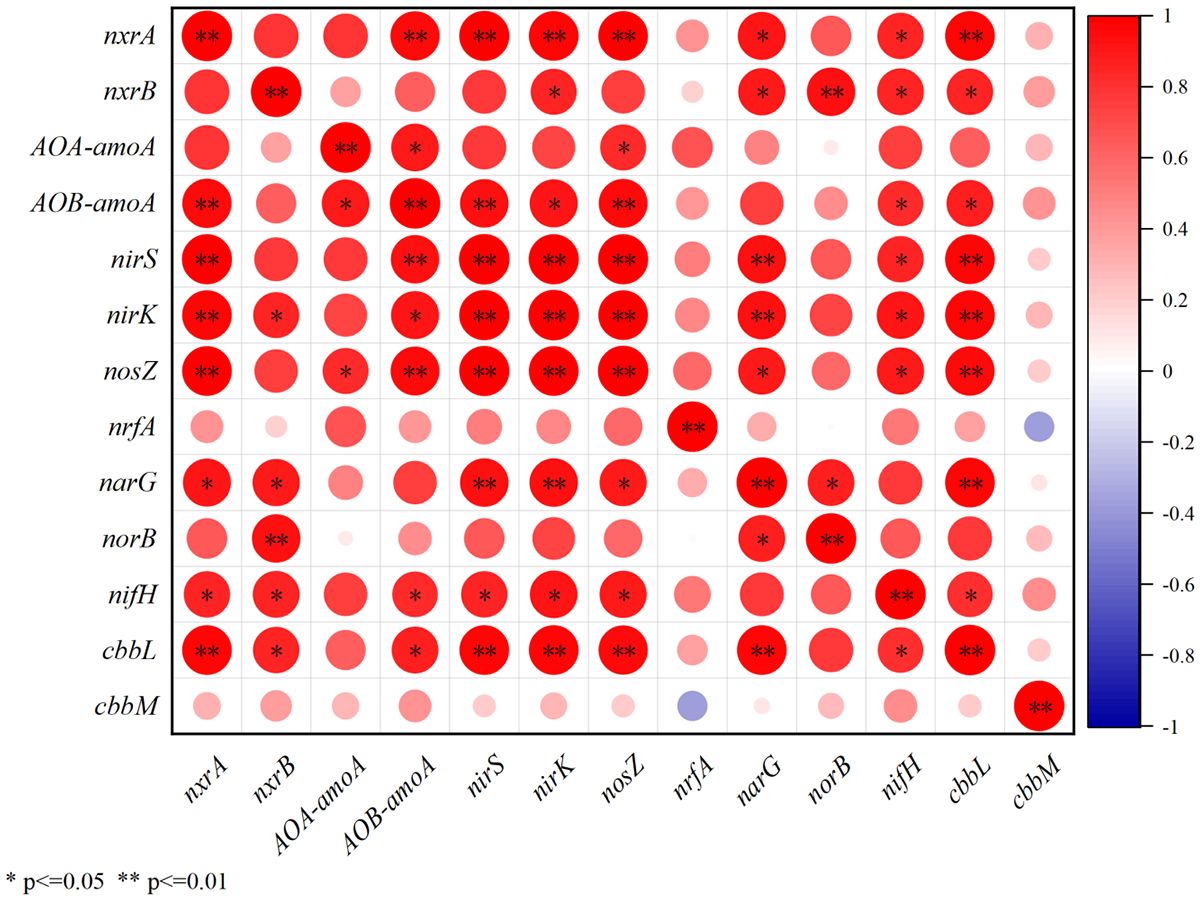
Figure 10 The correlations between the relative expression of cbbM, cbbL and functional genes for denitrification, ammonia oxidation, nitrification and nitrogen fixation.
5 Conclusion
In summary, our study revealed the response of the carbon storage to artificial restoration in an impaired wetland invaded by S.alterniflora. The change of the dominant plants, from S.alterniflora to mangrove plants, may decrease the content of the carbon storage in short time, mostly due to the loss of external organic matters. However, in the long term, mangroves plants may simultaneously enhance the content and the stability of the carbon storage in the study area, indicating the benefits of the restoration on the carbon storage function of such impaired wetlands. Our results also revealed the significant role of microbial CO2 assimilation, which serves as an alternative source of bioavailable carbon and thus support the activity of benthic community. This cutting-edge study indicated the efficiency of the coupling use of isotopic tracer and molecular analysis in revealing the response of coastal carbon storage to human activity, which may provide new insights in the future protection and restoration of coastal wetlands.
Data availability statement
The original contributions presented in the study are included in the article/Supplementary Material. Further inquiries can be directed to the corresponding author.
Author contributions
TC: Data curation, Investigation, Writing – original draft. DL: Conceptualization, Data curation, Formal Analysis, Funding acquisition, Investigation, Methodology, Project administration, Resources, Writing – original draft, Writing – review & editing. Y-JS: Funding acquisition, Investigation, Writing – review & editing. YG: Investigation, Resources, Writing – review & editing. KL: Investigation, Writing – review & editing. FJ: Investigation, Writing – review & editing. JL: Software, Writing – review & editing. YY: Resources, Writing – review & editing. RC: Resources, Writing – review & editing.
Funding
The author(s) declare that financial support was received for the research, authorship, and/or publication of this article. This work was supported by the National Natural Science Foundation of China (42306053), the Natural Science Foundation of Fujian Province, China (2021J05156), the Open fund of the Key Laboratory of Global Change and Marine-Atmospheric Chemistry (GCMAC2310), and Shenzhen Mangrove Wetlands Conservation Foundation (S22251).
Acknowledgments
We are grateful to Shenzhen Mangrove Wetland Conservation Foundation and Quanzhou Bay estuary wetland nature reserve development center for the help while sampling and the sharing of background information.
Conflict of interest
The authors declare that the research was conducted in the absence of any commercial or financial relationships that could be construed as a potential conflict of interest.
Publisher’s note
All claims expressed in this article are solely those of the authors and do not necessarily represent those of their affiliated organizations, or those of the publisher, the editors and the reviewers. Any product that may be evaluated in this article, or claim that may be made by its manufacturer, is not guaranteed or endorsed by the publisher.
Supplementary material
The Supplementary Material for this article can be found online at: https://www.frontiersin.org/articles/10.3389/fmars.2024.1364412/full#supplementary-material
References
Alongi D. M. (2014). Carbon cycling and storage in mangrove forests. Annu. Rev. Mar. Sci. 6, 195–219. doi: 10.1146/annurev-marine-010213-135020
Berg I. (2011). Ecological aspects of the distribution of different autotrophic CO2 fixation pathways. Appl. Environ. Microbiol. 77 (6), 1925–1936. doi: 10.1128/AEM.02473-10
Beulig F., Heuer V. B., Akob D. M., Viehweger B., Elvert M., Herrmann M., et al. (2015). Carbon flow from volcanic CO2 into soil microbial communities of a wetland mofette. ISME J. 9, 746–759. doi: 10.1038/ismej.2014.148
Bianchi T. S. (2011). The role of terrestrially derived organic carbon in the coastal ocean: a changing paradigm and the priming effect. Proc. Natl. Acad. Sci. U.S.A. 108, 19473–19481. doi: 10.1073/pnas.1017982108
Castañeda-Moya E., Twilley R. R., Rivera-Monroy V. H. (2013). Allocation of biomass and net primary productivity of mangrove forests along environmental gradients in the Florida Coastal Everglades, USA. Forest. Ecol. Manage. 307, 226–241. doi: 10.1016/j.foreco.2013.07.011
Craine J. M., Brookshire E. N. J., Cramer M. D., Hasselquist Niles J., Koba K., Marin-Spiotta E., et al. (2015). Ecological interpretations of nitrogen isotope ratios of terrestrial plants and soils. Plant Soil. 396, 1–26. doi: 10.1007/s11104-015-2542-1
Cui L., Berger U., Cao M., Zhang Y., He J., Pan L., et al. (2023). Conservation and restoration of mangroves in response to invasion of Spartina alterniflora based on the MaxEnt model: a case study in China. Forests. 14, 1220. doi: 10.3390/f14061220
Dauwe B., Middelburg J. J. (1998). Amino acids and hexosamines as indicators of organic matter degradation state in North Sea sediments. Limnol. Oceanogr. 43, 782–798. doi: 10.4319/lo.1998.43.5.0782
Denk T. R. A., Mohn J., Decock C., Lewicka-Szczebak D., Harris E., Butterbach-Bahl K., et al. (2017). The nitrogen cycle: a review of isotope effects and isotope modeling approaches. Soil Biol. Biochem. 105, 121–137. doi: 10.1016/j.soilbio.2016.11.015
Drake B. L. (2014). Using models of carbon isotope fractionation during photosynthesis to understand the natural fractionation ratio. Radiocarbon. 56, 29–38. doi: 10.2458/56.16155
Feng J., Huang Q., Qi F., Guo J., Lin G. (2015). Utilization of exotic Spartina alterniflora by fish community in the mangrove ecosystem of Zhangjiang Estuary: Evidence from stable isotope analyses. Biol. Invasions 17, 2113–2121. doi: 10.1007/s10530-015-0864-9
Feng J., Zhou J., Wang L., Cui. X., Ning C., Wu H., et al. (2017). Effects of short-term invasion of Spartina alterniflora and the subsequent restoration of native mangroves on the soil organic carbon, nitrogen and phosphorus stock. Chemosphere. 184, 774–783. doi: 10.1016/j.chemosphere.2017.06.060
Fourqurean J. W., Duarte C. M., Kennedy H., Marbà N., Holmer M., Mateo M. A., et al. (2012). Seagrass ecosystems as a globally significant carbon stock. Nat. Geosci. 5, 505–509. doi: 10.1038/ngeo1477
Gao Y. (2019). Studies on distribution patterns of and controlling factors for soil carbon pools of selected mangrove wetlands in China. Tsinghua University, Beijing.
Ge T., Wu X., Liu Q., Zhu Z., Yuan H., Wang W., et al. (2016). Effect of simulated tillage on microbial autotrophic CO2 fixation in paddy and upland soils. Sci. Rep-UK. 6, 1–9. doi: 10.1038/srep19784
Graça M. A., Newell S. Y., Kneib R. T. (2000). Grazing rates of organic matter and living fungal biomass of decaying Spartina alterniflora by three species of salt-marsh invertebrates. Mar. Biol. 136, 281–289. doi: 10.1007/s002270050686
Guo M. (2012). Studies on food sources of key macro-benthic fauna in mangrove wetlands of Zhangjiang Estuary and their implications. Xiamen University, Xiamen.
Hemminga M. A., Huiskes A. H. L., Steegstra M., Van-Soelen J. (1996). Assessment of carbon allocation and biomass production in a natural stand of the salt marsh plant Spartina anglica using 13C. Mar. Ecol. Prog. Ser. 130, 169–178. doi: 10.3354/meps130169
Jílková V., Straková P., Frouz J. (2020). Foliage C:N ratio, stage of organic matter decomposition and interaction with soil affect microbial respiration and its response to C and N addition more than C:N changes during decomposition. Appl. Soil. Ecol. 152, 103568. doi: 10.1016/j.apsoil.2020.103568
Kristensen E., Bouillon S., Dittmar T., Marchand C. (2008). Organic carbon dynamics in mangrove ecosystems: a review. Aqua. Bot. 89, 201–219. doi: 10.1016/j.aquabot.2007.12.005
Kuwae T., Kanda J., Kubo A., Nakajima F., Ogawa H., Sohma A., et al. (2016). Blue carbon in human-dominated estuarine and shallow coastal systems. Ambio. 45, 290–301. doi: 10.1007/s13280-015-0725-x
Li D., Yan J., Lu Z., Chu T. S., Li J., Chu T.-J. (2023). Use of δ13C and δ15N as indicators to evaluate the influence of sewage on organic matter in the Zhangjiang mangrove–estuary ecosystem, southeastern China. Water. 15, 3660. doi: 10.3390/w15203660
Liu J. Q., Wang W. Q., Shen L. D., Yang Y. L., Xu J. B., Tian M. H., et al. (2021). Response of methanotrophic activity and community structure to plant invasion in China’s coastal wetlands. Geoderma. 407, 115569. doi: 10.1016/j.geoderma.2021.115569
Lynn T. M., Ge T., Yuan H., Wei X., Wu X., Xiao K., et al. (2017). Soil carbon-fixation rates and associated bacterial diversity and abundance in three natural ecosystems. Microb. Ecol. 73, 645–657. doi: 10.1007/s00248-016-0890-x
Miltner A., Kopinke F. D., Kindler R., Selesi D., Hartmann A., Kästner M. (2005). Nonphototrophic CO2 fixation by soil microorganisms. Plant Soil. 269, 193–203. doi: 10.1007/s11104-004-0483-1
Mo B., Qin C., Chen P., Li X., Yuan H. (2017). Feeding habits of the purple sea urchin Heliocidaris crassispina based on stable carbon and nitrogen isotope analysis. J. Fish. Sci. China. 24, 566–575. doi: 10.3724/SP.J.1118.2017.16278
Nanba K., King G. M., Dunfield K. (2004). Analysis of facultative lithotroph distribution and diversity on volcanic deposits by use of the large subunit of ribulose 1,5-bisphosphate carboxylase/oxygenase, Appl. Environ. Microb. 70, 2245–2253. doi: 10.1128/AEM.70.4.2245-2253.2004
Nowak M. E., Beulig F., Fischer J. V., Muhr J., Küsel K., Trumbore S. E. (2015). Autotrophic fixation of geogenic CO2 by microorganisms contributes to soil organic matter formation and alters isotope signatures in a wetland mofette. Biogeosciences. 12, 7169–7183. doi: 10.5194/bg-12-7169-2015
Osland M. J., Gabler C., Grace J. B., Day R. H., McCoy M. L., McLeod J. L., et al. (2018). Climate and plant controls on soil organic matter in coastal wetlands. Global Change Biol. 24, 5361–5379. doi: 10.1111/gcb.14376
Pendleton L., Donato D. C., Murray B. C., Crooks S., Jenkins W. A., Sifleet S., et al. (2012). Estimating global ‘‘blue carbon’’ emissions from conversion and degradation of vegetated coastal ecosystems. PloS One. 7, e43542. doi: 10.1371/journal.pone.0043542
Pfaffl M. W. (2001). A new mathematical model for relative quantification in real-time RT-PCR. Nucleic Acids Res. 29, e45. doi: 10.1093/nar/29.9.e45
Phillips D. L., Gregg J. W. (2003). Source partitioning using stable isotopes: coping with too many sources. Oecologia. 136, 261–269. doi: 10.1007/s00442-003-1218-3
Philp P. (2007). The emergence of stable isotopes in environmental and forensic geochemistry studies. Environ. Chem. Lett. 5, 57–66. doi: 10.1007/s10311-006-0081-y
Qi X., Chmura G. L. (2023). Invasive Spartina alterniflora marshes in China: a blue carbon sink at the expense of other ecosystem services. Front. Ecol. Environ. 21, 182–190. doi: 10.1002/fee.2611
Ramírez-Álvarez N., Macías-Zamora J. V., Burke R. A., Rodriguez-Villanueva L. (2007). Use of δ13C, δ15N, and carbon to nitrogen ratios to evaluate the impact of sewage-derived particulate organic matter on the benthic communities of the Southern California Bight. Environ. Toxicol. Chem. 26, 2332–2338. doi: 10.1897/06-651
Regnier P. A. G., Friedlingstein P., Ciais P., Mackenzie F. T., Gruber N., Janssens I. A., et al. (2013). Anthropogenic perturbation of the carbon fluxes from land to ocean. Nat. Geosci. 6, 597–607. doi: 10.1038/ngeo1830
Reibach P. H., Benedict C. R. (1977). Fractionation of stable carbon isotopes by phosphoenolpyruvate carboxylase from C4 plants. Plant Physiol. 59, 564–568. doi: 10.1104/pp.59.4.564
Reis C. R. G., Reed S. C., Oliveira R. S., Nardoto G. B. (2019). Isotopic evidence that nitrogen enrichment intensifies nitrogen losses to the atmosphere from subtropical mangroves. Ecosystems. 22, 1126–1144. doi: 10.1007/s10021-018-0327-0
Roeske C. A., O’Leary M. H. (1984). Carbon isotope effects on enzyme catalyzed carboxylation of ribulose bisphosphate. Biochemistry. 23, 6275–6284. doi: 10.1021/bi00320a058
Spohn M., Müller K., Höschen C., Mueller C. W., Marhan S. (2019). Dark microbial CO2 fixation in temperate forest soils increases with CO2 concentration. Global Change Biol. 26, 1926–1935. doi: 10.1111/gcb.14937
Tolli J., King G. M. (2005). Diversity and structure of bacterial chemolithotrophic communities in pine forest and agroecosystem soils, Appl. Environ. Microb. 71, 8411–8418. doi: 10.1128/AEM.71.12.8411-8418.2005
Wang A., Chen J., Li D. (2008). Impact of Spartina alterniflora on sedimentary environment of coastal wetlands of the Quanzhou Bay. Ocean Eng. 26, 60–69. doi: 10.3969/j.issn.1005-9865.2008.04.010
Wang F. F., Xiao K., Santos I. R., Lu Z. Y., Tamborski J., Wang Y., et al. (2022). Porewater exchange drives nutrient cycling and export in a mangrove-salt marsh ecotone. J. Hydrol. 606, 127401. doi: 10.1016/j.jhydrol.2021.127401
Wood H., Werkman C., Hemingway A., Nier A. (1941). Heavy carbon as a tracer in heterotrophic carbon dioxide assimilation. J. Biol. Chem. 139, 365–376. doi: 10.1016/S0021-9258(19)51392-8
Wu G. (2018). Organic carbon sources and microbial carbon assimilation in mangrove ecosystems. Xiamen University, Xiamen.
Wu X., Ge T., Wang W., Yuan H., Wegner C. E., Zhu Z., et al. (2015). Cropping systems modulate the rate and magnitude of soil microbial autotrophic CO2 fixation in soil. Front. Microbiol. 6. doi: 10.3389/fmicb.2015.00379
Wu X., Ge T., Yuan H., Li B., Zhu H., Zhou P., et al. (2014). Changes in bacterial CO2 fixation with depth in agricultural soils. Appl. Microbiol. Biot. 98, 2309–2319. doi: 10.1007/s00253-013-5179-0
Xia S., Song Z., Li Q., Guo L. D., Yu C. X., Singh B. P., et al. (2021). Distribution, sources, and decomposition of soil organic matter along a salinity gradient in estuarine wetlands characterized by C:N ratio, δ13C-δ15N and lignin biomarker. Global Change Biol. 27, 417–434. doi: 10.1111/gcb.15403
Yang J., Gao J., Liu B., Zhang W. (2014). Sediment deposits and organic carbon sequestration along mangrove coasts of the Leizhou Peninsula, southern China. Estuar. Coast. Shelf Sci. 136, 3–10. doi: 10.1016/j.ecss.2013.11.020
Yuan H., Ge T., Chen C., O’Donnell A. G., Wu J. (2012). Significant role for microbial autotrophy in the sequestration of soil carbon. Appl. Environ. Microb. 78, 2328–2336. doi: 10.1128/AEM.06881-11
Zhang J. (2008). Influence of habitat heterogeneity of mangroves on crabs’ species, biomass and food sources. Xiamen University, Xiamen.
Zhang Y., Ding W., Luo J., Donnison A. (2010). Changes in soil organic carbon dynamics in an eastern Chinese coastal wetland following invasion by a C4 plant Spartina alterniflora. Soil Biol. Biochem. 42, 1712–1720. doi: 10.1016/j.soilbio.2010.06.006
Keywords: stable isotopes, microbial CO2 assimilation, carbon storage, Spartina alterniflora, mangrove
Citation: Chu T, Li D, Shih Y-J, Guo Y, Liu K, Ji F, Li J, Yin Y and Chen R (2024) Coupling use of stable isotopes and functional genes as indicators for the impacts of artificial restoration on the carbon storage of a coastal wetland invaded by Spartina alterniflora, southeastern China. Front. Mar. Sci. 11:1364412. doi: 10.3389/fmars.2024.1364412
Received: 02 January 2024; Accepted: 21 March 2024;
Published: 05 April 2024.
Edited by:
Jianxiang Feng, Sun Yat-sen University, ChinaCopyright © 2024 Chu, Li, Shih, Guo, Liu, Ji, Li, Yin and Chen. This is an open-access article distributed under the terms of the Creative Commons Attribution License (CC BY). The use, distribution or reproduction in other forums is permitted, provided the original author(s) and the copyright owner(s) are credited and that the original publication in this journal is cited, in accordance with accepted academic practice. No use, distribution or reproduction is permitted which does not comply with these terms.
*Correspondence: Danyang Li, bGlkYW55YW5nQGptdS5lZHUuY24=