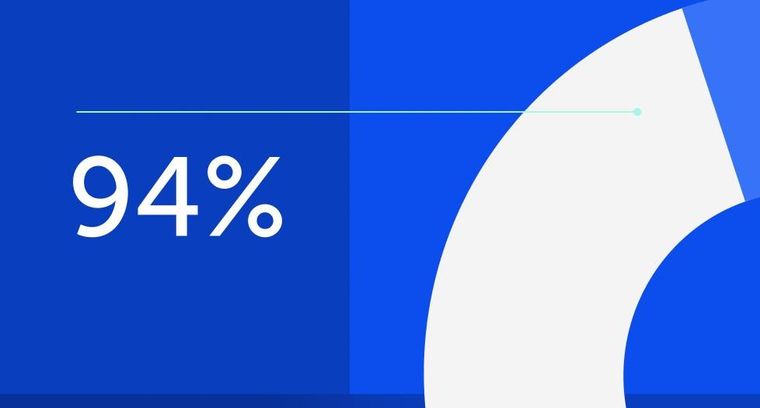
94% of researchers rate our articles as excellent or good
Learn more about the work of our research integrity team to safeguard the quality of each article we publish.
Find out more
BRIEF RESEARCH REPORT article
Front. Mar. Sci., 24 May 2024
Sec. Marine Fisheries, Aquaculture and Living Resources
Volume 11 - 2024 | https://doi.org/10.3389/fmars.2024.1361157
This article is part of the Research TopicBlue Foods Security and SustainabilityView all 15 articles
The potential of diatoms as aquatic bait, attribute to their abundance in highly unsaturated fatty acids, has been extensively studied. Temperature plays a crucial role in the synthesis of these fatty acids. This study specifically investigated the impact of temperature on the growth, total lipid content, and fatty acid composition of Skeletonema dohrnii, a planktonic diatom commonly associated with red tides and water blooms in China. The aim is to evaluate its suitability as an aquatic bait and provide insights for large-scale factory farming. Results indicated that the highest biomass and maximum growth rate occurred at 28°C, with no significant deviation from the control group at 25°C. At 28°C and 15°C, there was a significant increase in the total lipid content and the total fatty acid content, with a more pronounced effect at 15°C. At 28°C, EPA and DHA content measured at 0.97 ± 0.01 mg.DW.L-1 and 0.264 ± 0.01 mg.DW.L-1 respectively, surpass those at 15°C due to lower biomass. Conversely, at 15°C, substantial synthesis of long-chain polyunsaturated fatty acids, with EPA constituting up to 32.24 ± 0.24% of the total fatty acids, is observed. Modulating the temperature could optimize the utilization of S. dohrnii as an aquatic feed source. These findings underscore the potential of S. dohrnii as a high-quality aquafeed and lay the groundwork for its success in ocean warming scenarios.
Diatoms, a class of photosynthetic autotrophic unicellular microalgae eukaryotes, are widely distributed in the ocean, fresh water and wet soil. As the main group of phytoplankton, diatoms contribute to approximately 20% of global primary production and nearly 40% of marine primary production (Field et al., 1998). They play an important role in the material and energy circulation within marine ecosystem, forming the foundation of the marine nutrient network (Falkowski et al., 1998; Armbrust, 2009; Sepúlveda and Cantarero, 2022). Diatoms are rich in polyunsaturated fatty acids (PUFAs), including eicosapentaenoic acid (EPA), docosapentaenoic acid (DHA), myristic acid, palmitic acid, and palmitoleic acid. These compounds have been associated with protective effects against cardiovascular disease, the promotion of healthy vision, and assistance in the prevention of psychiatric disorders (Yi et al., 2017; Marella and Tiwari, 2020). The presence of PUFAs, particularly EPA, renders diatoms a valuable source of nutrition for marine mussels, crustacean larvae, and fish larvae (Reitan et al., 1997; Renaud et al., 2002; Mishra and Tiwari, 2021). Not only can this synthesis effectively reduce production costs (Valenzuela-Espinoza et al., 1999), but the cultivation of marine diatoms for the replacement of fishmeal and fish oil made from harvesting of wild-caught fish, promote ecological sustainability (Sarker, 2023).
The metabolism of diatom fatty acids is influenced by various factors, including temperature, light, nutrients, and pH. Notably, temperature is an important one, compared to other factors, it exerts a substantial impact on the physiological processes of algae due to its involvement in numerous metabolic processes, enzymatic, and photosynthetic processes, directly influencing the synthesis and transformation of diatom fatty acids (Davison, 1991; Duarte, 2007; Converti et al., 2009; Boyd et al., 2013; Sepúlveda and Cantarero, 2022). Lower temperatures have been observed to prompt Phaeodactylum tricornutum and Skeletonema menzelli to synthesize and accumulate higher levels of essential fatty acids (EFAs) and PUFAs (Jiang and Gao, 2004; Gao et al., 2014). Additionally, Nitzschia frustulum, in colder conditions, has shown increased synthesis and storage of unsaturated fatty acids (USFAs), aiding in maintaining cellular membrane fluidity and permeability (Harwood, 1988; Renaud et al., 1995). Conversely, elevated temperatures have been noted to diminish the content of EFAs and DHA in Chaetoceros sp. while increasing the amount of saturated fatty acids (SFAs) (Renaud et al., 2002). This transition from USFAs to SFAs is believed to aid in preserving the fluidity and stability of the cell membrane (Quinn, 1981; Murata and Los, 1997; Sepúlveda and Cantarero, 2022). Maia et al. (2022) found that Skeletonema costatum shows greater temperature tolerance compared to P. tricornutum, likely due to S. costatum’s capacity to accumulate more USFAs and proteins when exposed to higher temperatures. Various species of microalgae exhibit distinct responses in their fatty acid profiles to changes in temperature. Hence, identifying the optimal temperature for PUFAs accumulation is crucial for reducing production costs.
The present research explores Skeletonema dohrnii, a member of the Skeletonema genus, among the most prevalent marine planktonic diatoms in the temperate zones of the World Ocean (Shevchenko et al., 2019). S. dohrnii often contributes to the occurrence of red tides or water blooms in China, which can exert a significant and detrimental impact on the environment (Gu et al., 2012). Its rapid growth, robust reproductive capacity, and ability to thrive make it an ideal subject for study, development, and exploitation. Currently, there is a scarcity of research on S. dohrnii, with the predominant focus of studies concentrated on its ecological and physiological characteristics, while only a minority delve into its potential applications (Yamada et al., 2010; Ogura et al., 2018; Thangaraj et al., 2019; Thangaraj and Sun, 2020). Marine diatoms are often thriving under adverse marine environmental conditions (Kooistra et al., 2007; Bowler et al., 2010; Thangaraj et al., 2019). Studies have indicated that diatoms have a range of adaptive mechanisms in response to seawater warming and ocean acidification, including the adjustment of saturated fatty acid ratios to mitigate adverse effects (Jin and Agustí, 2018; Thangaraj and Sun, 2020). As such, marine diatoms possess the potential to adapt to climate change. Exploring changes in their fatty acid content and composition in response to temperature, as alongside the investigation of the nutritional value, development, and utilization of S. dohrnii, could provide insight into its lipid accumulation rate. This, in turn, could serve as a foundation for fish feed and biodiesel production, supporting the sustainable development of marine resources.
The marine diatom S. dohrnii, was isolated from the Yellow Sea and cultivated in the laboratory using the f/2 medium (25°C 90–120 µmol photons m-2·s-1, with 12:12 light-dark cycle).
Artificial seawater media (ASW) supplemented with a silicate stock solution (28.40 g·L−1) was utilized in this experiment (Sunda et al., 2005). After being cultured and adapted in ASW, S. dohrnii was subjected to three different temperatures: 15°C (the low-temperature group), 25°C (the control group), and 28°C (the high-temperature group), for a single expansion culture. The initial cell concentration was set at 1×105 cell/mL. The cells were collected in the stationary growth phase, with triplicate samples established within each temperature group (n=3).
Throughout the period from inoculation to collection, the algal solution was gently agitated and blended daily. Subsequently, 100 μl of the solution was carefully transferred to a cell counting plate using a pipette and examined under a microscope. The cell density of the sample was determined by calculating the average of the three counts (unit: cells/mL).
The specific growth rate was calculated using the following formula:
Where Nt represents the number of cells after t days of cultivation, and t denotes the duration of the culture, Nt-1 is the cell concentration on the dayt-1.
Once the algal cells completed their exponential growth phase, the liquid was collected via centrifugation (4000r/min, 4°C). The resulting freeze-dried algal powder was then stored at -20°C, and the total lipid was determined following the guidelines of GB 5009.6. To initiate the process, 500 mg of the algal powder was weighed into a Maoshi bottle, and 4 mL of water along with 5 mL of 2M HCl were added. The mixture was then heated in a water bath at 75°C for 50 min. After cooling, 5 mL of ethanol was gradually introduced and mixed, after which 25 mL of ether was added. The bottle was shaken and allowed to settle for 30 min. The upper organic layer was carefully poured out, and the extraction process was repeated thrice. The resulting extract was gathered into a conical flask of constant weight, and the solvent was evaporated in a water bath. The remaining material was dried in an oven at 100°C for 1 h, cooled for 30 min, and then weighed. The obtained test data was presented as the mean ± standard deviation. The statistical software SPSS 26.0 was used for data analysis, while Excel 2019 and SPSS 26.0 were utilized for chart creation.
Weigh 30 mg of freeze-dried algae powder and combine it with 10 mL of 4M HCl, then place the mixture in a boiling water bath for 10 min. Immediately afterwards, transfer it to a -20°C refrigerator for 15 min. Upon removal from the refrigerator, add 10 mL of chloroform and 5 mL of methanol, allowing the solution to rest at room temperature for 2 h. The lower chloroform phase is then collected via centrifugation and dried with nitrogen to obtain the total lipid. For further analysis, introduce 1 mL of n-hexane and 200 μL of potassium hydroxide-methanol solution. Vigorously shake the solution for 30 seconds and let it settle for layering. Transfer the upper n-hexane phase to a sample bottle for analysis. The percentage content of each fatty acid was determined using a gas chromatograph (GC-2014, Shimadzu Manufacturing Institute) following the internal standard method outlined in GB 5009.168.
Additionally, the area normalization method was employed to calculate the percentage content of each fatty acid. This method involves summing the peak areas of all components, which collectively represent 100% of the total peak area, and then calculating the percentage of each individual peak area relative to the total peak area. The calculation formula utilized was as follows:
where Ci% represents the percentage content of the measured component i. fi′ is the relative mass correction factor of the measured component i. Ai denotes the peak area of the measured component i. m is the mass of the measured sample. And mi represents the mass of the tested component i.
The growth of S. dohrnii under cultivation at three different temperatures was measured and is visually in Figure 1. All inoculated S. dohrnii samples exhibited growth, with the shortest growth cycle occurring at the highest temperature, spanning a period of 3 days. The maximum growth rate was observed to be the lowest at 15°C, with a value of 0.62 ± 0.09 (p<0.005), which was significantly different from the other two temperatures. Additionally, the maximum death rate was recorded at 15°C, with a value of -0.35 ± 0.03, which was statistically different from the rated observed at the other temperatures (Table 1). However, the highest growth rates were observed at 28°C (0.89 ± 0.02), followed by 25°C (0.8 ± 0.01). The results of the experiment indicated that the highest cell density of S. dohrnii was 6.5 × 106 cells/mL achieved at 25°C, while density at 28°C and 15°C were 6.35 × 106 cells/mL and 5.80 × 106 cells/mL, respectively. Likewise, the cultures initiated with a biomass concentration of 0.006 ± 0.001 g DW L-1, and eventually reached their maximum concentrations at 0.046 ± 0.002 (15°C), 0.120± 0.001 (25°C), and 0.122 ± 0.001 (28°C) g DW L-1 for S. dohrnii, respectively. Notably, there was no significant difference (p<0.05) observed between the biomass at 25°C and 28°C (Table 1).
Table 1 Specific growth rate, biomass and total lipid content of S. dohrnii under three temperature conditions.
The total lipid and fatty acid profile of S. dohrnii at different temperatures.
In the three experimental groups, temperature exhibited a significant impact on the total lipid content of the algae. As the temperature increased, there was a corresponding decrease in the total lipid content of the algae. The highest total lipid content was observed at 15°C (39.23 ± 0.32 mg/g), followed by the high-temperature group at 28°C (37.43 ± 0.31 mg/g), and the lowest content was in the control group at 25°C (30.97 ± 0.06 mg/g). These variations between the groups were statistically significant (p<0.05) (Table 2). A total of 23 kinds of fatty acids were detected in this experiment, albeit some species were only detected at specific temperatures. About the fatty acids composition, the analysis revealed 11 types of SFAs, 12 types of USFAs, 5 types of monounsaturated fatty acids (MUFAs), and 7 types of polyunsaturated fatty acids (PUFAs).
The fatty acid composition of S. dohrnii primarily consists of C14:0, C18:1:ω9, and C20:5:ω3, collectively representing nearly 60% of the total fatty acid (TFA) content. In order to facilitate comparisons of fatty acid compositions, the measured data have been converted here to percentage occupancy. Given its proximity to the natural habitat temperature of S. dohrnii, the fatty acid content at 25°C was utilized as a reference point. Notably, C14:0 and C20:5:ω3 exhibited elevated concentrations, accounting for 23.45 ± 0.25% and 25.79 ± 0.11%, respectively, whereas C18 and C22 of USFAs displayed lower levels. These observed characteristics align with the typical profile of Bacillariophyta (Zhukova and Aizdaicher, 1995; Chen, 2012). For further details, please refer to Table 2.
In comparison to the control group (25°C), at 15°C, the USFAs content in S. dohrnii exhibited a significant increase from 10.21 ± 0.01 mg/g to 27.09 ± 0.10 mg/g, while the PUFAs content showed a significant increase from 5.9 ± 0.07 mg/g to 18.31 ± 0.19 mg/g. Among the PUFAs, EPA was the most predominant, ranging from 4.08 ± 0.01 mg/g to 12.03 ± 0.03 mg/g, followed by DHA at 0.89 ± 0.03 mg/g to 2.62 ± 0.19 mg/g. Conversely, with a rise in temperature compared to the control group, at 28°C, both USFAs and PUFAs, as well as EPA and DHA, exhibited increases in content, with values of 22.55 ± 0.2 mg/g, 11.36 ± 0.13 mg/g, 7.95 ± 0.01 mg/g, and 2.16 ± 0.03 mg/g respectively (Table 2). The TFA content varied across experimental conditions, with the control group displaying the lowest level (15.77 ± 0.01 mg/g) and the low-temperature group exhibiting the highest (36.99 ± 0.05 mg/g), followed by the high-temperature group (35.02 ± 0.17 mg/g). Fatty acid compositions shifted in response to temperature variations, as depicted in Figure 2. The percentages of PUFAs decreased with increasing temperatures, registering at 49.15 ± 0.04% at 15°C, 37.17 ± 0.09% at 25°C, and 30.59 ± 0.19% at 28°C. Similarly, the proportions of EPA declined with rising temperatures, measuring 32.24 ± 0.24% at 15°C, 25.79 ± 0.11% at 25°C, and 21.73 ± 0.12% at 28°C. Conversely, the proportions of SFAs and MUFAs exhibited an upward trend with elevated temperatures. SFAs increased from 26.59 ± 0.06% at 15°C to 35.64 ± 0.20% at 28°C, while MUFAs rose from 24.26 ± 0.10% at 15°C to 32.77 ± 0.09% at 28°C (Figure 2). DHA exhibited minimal changes, showing a decrease, albeit not statistically significant, between 25°C and 28°C (Figures 2B, C). However, the percentage of both EPA and DHA in the PUFAs increased significantly with temperature, but there was little change in the data (Figure 2).
Figure 2 The proportion of the main fatty acids of S. dohrnii at 15°C (A), 25°C (B) and 28°C (C) according to the area normalization method results.
The analysis of fatty acid carbon chain length revealed that short and medium-chain fatty acids predominantly constituted the SAFs, exhibiting consistent distribution across different temperatures. However, the distribution of long-chain fatty acids exhibited significant variability based on the temperature conditions. The long-chain fatty acid content in both SFA and MUFA was positively and significantly different from the temperature change. Specifically, at 28°C, the highest count of saturated fatty acid species (10) and very long-chain unsaturated fatty acid species (3) were observed, while at 15°C, the highest count of unsaturated fatty acid species (11) and very long-chain unsaturated fatty acid species (2) was identified. Moreover, the highest content of long-chain unsaturated fatty acids (LC-USAFs) was observed at 15°C, reaching up to 24 mg/g, whereas the highest content of long-chain saturated fatty acids (LC-SAFs) was recorded at 28°C, approximately 10 mg/g.
The Intergovernmental Panel on Climate Change (IPCC, 2018) has projected that by the end of the century, global marine warming will lead to a temperature increase of 1–3°C in sea surface temperatures. This climatic shift is anticipated to trigger various impacts, including physiological alternations in marine phytoplankton, ocean stratification, a reduction in the depth of the upper mixed layer, and diminished nutrient supply to the upper layer of seawater. Consequently, these changes may result in nutrient limitation and a decline in primary productivity (Laufkötter et al., 2015; Jin and Agustí, 2018). Temperature has been identified as a major factor influencing the metabolic processes, enzymatic reactions, and photosynthesis of diatoms and other microalgae (Depauw et al., 2012; Bermudez et al., 2015; Dong et al., 2016; Liang et al., 2019; Thangaraj et al., 2020).
In this study, results showed that the highest biomass and maximum growth rate were observed at 28°C, with no statistically significant difference compared to the control group. Notably, at 28°C, the TFA content (36.99 ± 0.05 mg/g), EPA content (7.95 ± 0.01 mg/g), and DHA content (2.16 ± 0.03 mg/g) were significantly higher than those recorded in the control group. Maia et al. (2022) found that S. costatum exhibited enhanced biomass and lipid production, particularly PUFAs, at elevated temperatures in comparison to P. tricornutum. This suggests that S. costatum may possess a higher degree of tolerance to temperature variations and adaptive capabilities in anticipation of future ocean warming. This suggests the potential adaptation of S.dorhnii to future ocean warming. Numerous prior studies have demonstrated the superiority of diatoms surviving in high temperature conditions. Research indicates that S. dorhnii has the potential to adapt to ocean warming by readjusting energy metabolism within cells to better suit to warmer waters (Cheng et al., 2022). Under stress conditions, S.dorhnii tends to synthesizes lipids, mainly in the form of TAGs, rather than serve as a carbon sink (Zulu et al., 2018). Proteomics analysis revealed that the lipid synthesis proteins in S.dorhnii were upregulated at high temperature (Thangaraj et al., 2020). Studies utilizing the transcriptomic approach have revealed that the preservation of protein processing machinery and membrane structure constitutes crucial short-term physiological mechanisms employed to counteract temperature variations. These mechanisms are identified as key components associated with the adaptation to different growth temperatures (Liang et al., 2019). Upon reaching the 700th generation, the lipid metabolism of S.dorhnii assumes prominence as a pivotal element in its sustained adaptation to ocean warming (Cheng et al., 2022). However, it is noteworthy that in the high-temperature environment, there was a notable increase in the proportion of SFAs and a concurrent decrease in the proportion of USFAs. The SAFs content of S.dorhnii was significant higher than that of the control group. A greater diversity and higher content of LC-SAFs were observed at 28°C and the content reached up to 10 mg/g. The increase in SFAs is believed to contribute to the maintenance of cell membrane fluidity and stability (Renaud et al., 2002; Sepúlveda and Cantarero, 2022). Moreover, elevated LC-SAFs can increase intracellular energy expenditure, reduce intracellular energy metabolism, and sustain healthy and normal metabolic activity (Giudetti et al., 2016).
In addition to alterations in fatty acid saturation under temperature stress conditions, diatoms may employ a rapid adaptation strategy to tackle higher temperatures (O'Donnell et al., 2019). This strategy involves adjusting the optimum temperature, enhancing the maximum specific growth rate, improving light-use efficiency, diminishing photosynthetic intensity, and achieving saturation in photosynthesis at an earlier stage (Jin and Agustí, 2018; Cheng et al., 2022). For example, Chaetoceros tenuissimus was observed to enhance its high-temperatures tolerance by modifying its optimum temperature, elevating its maximum specific growth rate, optimizing light-use efficiency, reducing photosynthetic intensity, and reaching saturation in photosynthesis earlier (Jin and Agustí, 2018). In our study, S. dohrnii was found to have a higher specific growth rate at 28°C, with a maximum biomass exceeding the optimum temperature. This suggests the likelihood of employing similar adaptive mechanisms to adapt to future ocean warming.
Microalgae play an important role in promoting the sustainable development of aquaculture feeds by reducing the pressure on fish stocks and fertile soils provoked by traditional feed production methods. At present, the aquatic species nourished by microalgae boast high survival rates and exceptional nutritional value (Sarker et al., 2016; Velasquez et al., 2016; Sales et al., 2021), driving the significant momentum behind the industrial-scale integration of microalgae in aquaculture feeds (Beal et al., 2018; Shah et al., 2018; Sarker et al., 2020).
The primary obstacle hindering the widespread application of microalgae as aquaculture feeds is the high production cost. To mitigate this, the selection of high-quality microalgae species and the implementation of large-scale production processes serve as effective means to reduce expenses. The selection of appropriate microalgae for aquafeed must consider various factors, such as cell size, cell wall thickness, digestibility, pigment content, and growth rate (Gladue and Maxey, 1994; Renaud et al., 2002). Among these factors, the growth rate of algae assumes particular importance in large-scale production of microalgae as aquaculture feeds. In our study, S.dorhnii exhibited rapid growth and reproduction, enabling the cultivation of numerous algal strains in a short period of time. Its substantial accumulation of fatty acids aligns well with the requisites for large-scale production, rendering it a good candidate for the development of aquafeeds.
In addition to the factors mentioned above, nutritional value is a crucial consideration, particularly for the fatty acids, especially PUFAs, play a vital role in the growth and development of aquaculture animals. Since the 1940s, over 100 fatty acids from microalgae have been identified and applied in the development and production of aquafeeds (Roy and Pal, 2015). Spirulina platensis is recognized as an important biological source of γ-linolenic acid, constituting 20%-30% of its fatty acid composition (Becker, 2003). Cyanobacteria typically contain 25–60% PUFA and exhibit richness in fatty acids such as linoleic acid (18:2) and linolenic acid (18:3), as well as EPA (20:5) and arachidonic acid (20:4) (Borowitzka and Borowitzka, 1988). Notably, the red alga P. cruentum stands out for its high arachidonic acid content, comprising roughly 36% of the TFA content, highlighting microalgae as a reliable source of n-3 fatty acids, a significant component of high-quality fish oils (Ahern et al., 1983). These specific fatty acids are primary metabolites that accumulate in large quantities with cell growth, forming the basis for their industrial production. Studies have demonstrated that the intake of n-3 fatty acids by cultured animals can significantly reduce the risk of stress responses and chronic diseases (Zuo et al., 2012). S. costatum abundant in highly unsaturated fatty acids (HUFAs) (EPA and DHA, both n-3 fatty acids), essential amino acids, and other nutrients necessary for the growth and development of marine larvae, is widely used as an additive in aquaculture feeds (Herawati et al., 2014; Lestari et al., 2014; van Houcke et al., 2017). EPA play a crucial role in the growth and development of marine organisms, with diatoms, including S. dohrnii, particularly abundant in EPA compared to other marine microalgae (Ratnayake and Galli, 2009; Nodumo et al., 2018). The diatom Odontella aurita has been successfully cultured and marketed as a dietary supplement high in omega-3 fatty acids for several years. In cold environmental conditions, O. aurita contains 45% PUFAs and is rich in EPA, constituting up to 39% of its composition, similar to S. dohrnii (Pasquet et al., 2014). Under such conditions, biochemical processes are activated, stimulating the production of EPA and DHA. These fatty acids are synthesized through a series of desaturation and elongation reactions, as evidenced by previous studies on other diatoms and microalgae (Mimouni et al., 2003; Guihéneuf et al., 2013).
Furthermore, fish metabolize fatty acids with the highest efficiency in the order of PUFAs, followed by MUFAs and SAFs. As the chain length of MUFAs and SAFs increases, their absorption efficiency decreases (Xu et al., 2020). Our research revealed that S. dohrnii exhibited a substantial percentage of long-chain polyunsaturated fatty acids (LC-PUFAs, >C18) at 49.15± 0.04% and a notable percentage of USFAs at 73.41± 0.06% in cold temperatures. Long-chain fatty acids were also lowest in both SFAs and MUFAs in cold temperatures. Despite variations in temperature conditions, the proportion of PUFAs remained consistently high, suggesting its suitability as fish bait, especially for marine species.
Nevertheless, sustaining controlled low temperatures for large-scale cultivation in factories proves financially burdensome. Additionally, at low temperatures, the biomass of algal cells experiences a significant reduction, negatively impacting the overall yield of algal lipids, particularly EPA and DHA. Our research indicated that temperature stress induces an elevation in total lipid content, especially TFA content, regardless of whether it is high or low temperature stress. This phenomenon occurs due to diatoms redirecting their carbon flux from polysaccharide chrysolaminarin (β-1,3-gluvan) utilization, a typical carbon sink under normal conditions (Kroth et al., 2008), toward lipid synthesis under stress conditions, storing them as TAG (Zulu et al., 2018). Our data indicated that under the culture conditions, EPA and DHA production in the low-temperature group amounted to 0.553 ± 0.01 mg.DW.L-1 and 0.121 ± 0.01 mg.DW.L-1, respectively. Conversely, in the high-temperature group, EPA content measured at 0.97 ± 0.01 mg.DW.L-1 and DHA content at 0.264 ± 0.01 mg.DW.L-1, both surpassing those in the low-temperature group. Moreover, water temperature significantly influences the normal reproduction and growth of bait organisms during high summer temperatures (Bao and You, 2004; Pasquet et al., 2014). Many aquatic seedling nursery processes experience the high-temperature period in summer, such as the seedlings of Urechis unicinctus. Consequently, the demand for live microalgae at this stage is high. However, the microalgae utilized struggle to survive under high-temperature and intense light conditions, leading to their decay. This results in an inadequate and untimely supply of microalgae, thereby causing potential problems in aquatic seeding production (Pasquet et al., 2014; Zhao et al., 2021). Hence, there’s an urgent need to identify bait microalgae capable of stable growth at temperatures of 28°C and above, ensuring a reliable supply of microalgae during summer. Coupled with the aforementioned adaptive mechanism, it was found that S.dorhnii has the potential to adapt to high temperatures. With certain experimental conditions of stress, there may be a possibility to enhance the optimal temperature and tolerance without resorting gene editing. Through rapid evolutionary strategies, S.dorhnii might evolve into a source of high-temperature-tolerant aquatic microalgae during the summer period.
However, each of these feed microalgae has certain limitations (Chauton et al., 2015). At present, to enable large-scale commercial production of S. dorhnii, it is necessary to further enhance its fatty acid production capacity. A negative correlation between the optimal growth rate and lipid accumulation was observed at temperatures below the optimal range. To address this issue, a two-step culture method, in conjunction with the study of Roleda et al. (2013), can be employed.
Currently, the amount of lipids in S. dohrnii cultivated in the laboratory is not on par with that of other extensively produced aquatic microalgae. However, the increased proportion of EPA and DHA at lower temperatures suggests that temperature has a considerable influence on fatty acid concentration. Through additional exploration of various environmental factors, its potential as a source of aquatic microalgae could be further enhanced. In the future, the development of aquatic microalgae should involve considering the combination of different microalgae to maximize their individual benefits.
The original contributions presented in the study are included in the article/supplementary material. Further inquiries can be directed to the corresponding authors.
XS: Writing – review & editing, Writing – original draft, Visualization, Supervision, Resources, Project administration, Methodology, Investigation, Conceptualization. YY: Writing – original draft, Investigation, Data curation. ZS: Writing – original draft, Investigation, Data curation. YZ: Writing – original draft, Investigation, Data curation. ZL: Writing – original draft, Methodology, Investigation. JS: Supervision, Writing – review & editing, Resources, Conceptualization.
The author(s) declare financial support was received for the research, authorship, and/or publication of this article. This work was supported by the Natural Science Foundation of China (No. 42206087) and the National Key Research and Development Project of China (No. 2019YFC1407800).
Author ZL was employed by the company Tianjin Chenhui Modern Technology Group Co., Ltd.
The remaining authors declare that the research was conducted in the absence of any commercial or financial relationships that could be construed as a potential conflict of interest.
The author(s) declared that they were an editorial board member of Frontiers, at the time of submission. This had no impact on the peer review process and the final decision.
All claims expressed in this article are solely those of the authors and do not necessarily represent those of their affiliated organizations, or those of the publisher, the editors and the reviewers. Any product that may be evaluated in this article, or claim that may be made by its manufacturer, is not guaranteed or endorsed by the publisher.
Ahern T. J., Katoh S., Sada E. (1983). Arachidonic acid production by the red alga Porphyridium cruentum. Biotechnol. Bioeng. 25, 1057–1070. doi: 10.1002/bit.260250414
Armbrust E. V. (2009). The life of diatoms in the world’s oceans. Nature 459, 185–192. doi: 10.1038/nature08057
Bao Y. B., You Z. J. (2004). Influences of several environmental factors on growth in marine shellfish larvae. Fish. Sci. 12, 39–41. doi: 10.16378/j.cnki.1003–1111.2004.12.012
Beal C. M., Gerber L. N., Thongrod S., Phromkunthong W., Kiron V., Granados J., et al. (2018). Marine microalgae commercial production improves sustainability of global fisheries and aquaculture. Sci. Rep. 8, 15064. doi: 10.1038/s41598–018-33504-w
Becker E. W. (2003). “Microalgae in human and animal nutrition,” in Hand book of microalgal culture. Ed. Richmond A. (Oxford, Blackwell), 312–351. doi: 10.1002/9780470995280.ch18
Bermudez R., Feng Y. Y., Roleda M. Y., Tatters A. O., Hutchins D. A., Larsen T., et al. (2015). Long-term conditioning to elevated pCO2 and warming influences the fatty and amino acid composition of the diatom Cylindrotheca fusiformis. PloS One 10, e0123945. doi: 10.1371/journal.pone.0123945
Borowitzka M. A., Borowitzka L. J. (1988). “Limits to growth and carotenogenesis in laboratory and large-scale outdoor cultures of Dunaliella salina,” in Algal Biotechnology. Ed. Stadler T. (Elsevier Applied Science, Essex, UK), 371–381.
Bowler C., Vardi A., Allen A. E. (2010). Oceanographic and biogeochemical insights from diatom genomes. Annu. Rev. Mar. Sci. 2, 333–365. doi: 10.1146/annurev-marine-120308-081051
Boyd P. W., Rynearson T. A., Armstrong E. A., Fu F. X., Hayashi K., Hu Z. X., et al. (2013). Marine phytoplankton temperature versus growth responses from polar to tropical waters-Outcome of a scientific community-wide study. PloS One 8, e63091. doi: 10.1371/journal.pone.0063091
Chauton M. S., Reitan K. I., Norsker N. H., Tveterås R., Kleivdal H. T. (2015). A techno-economic analysis of industrial production of marine microalgae as a source of EPA and DHA-rich raw material for aquafeed: Research challenges and possibilities. Aquaculture 436, 95–103. doi: 10.1016/j.aquaculture.2014.10.038
Chen Y. C. (2012). The biomass and total lipid content and composition of twelve species of marine diatoms cultured under various environments. Food Chem. 131, 211–219. doi: 10.1016/j.foodchem.2011.08.062
Cheng L. M., Zhang S. F., Xie Z. X., Li D. X., Lin L., Wang M. H., et al. (2022). Metabolic adaptation of a globally important diatom following 700 generations of selection under a warmer temperature. Environ. Sci. Technol. 56, 5247–5255. doi: 10.1021/acs.est.1c08584
Converti A., Casazza A. A., Ortiz E. Y., Perego P., Del Borghi M. (2009). Effect of temperature and nitrogen concentration on the growth and lipid content of Nannochloropsis oculata and Chlorella vulgaris for biodiesel production. Chem. Eng. Process.: Process Intensification 48, 1146–1151. doi: 10.1016/j.cep.2009.03.006
Davison I. R. (1991). Environmental effects on algal photosynthesis: temperature. J. Phycol. 27, 2–8. doi: 10.1111/j.0022-3646.1991.00002.x
Depauw F. A., Rogato A., d’Alcalá M. R., Falciatore A. (2012). Exploring the molecular basis of responses to light in marine diatoms. J. Exp. Bot. 63, 1575–1591. doi: 10.1093/jxb/ers005
Dong H. P., Dong Y. L., Cui L., Balamurugan S., Gao J., Lu S. H., et al. (2016). High light stress triggers distinct proteomic responses in the marine diatom Thalassiosira pseudonana. BMC Genomics 17, 994. doi: 10.1186/s12864-016-3335-5
Duarte C. M. (2007). Marine ecology warms up to theory. Trends Ecol. Evol. 22, 331–333. doi: 10.1016/j.tree.2007.04.001
Falkowski P. G., Barber R. T., Smetacek V. (1998). Biogeochemical controls and feedbacks on ocean primary production. Science 281, 200–206. doi: 10.1126/science.281.5374.200
Field C. B., Behrenfeld M. J., James T. R., Falkowski P. (1998). Primary production of the biosphere: Integrating terrestrial and oceanic components. Science 281, 237–240. doi: 10.1126/science.281.5374.237
Gao X. Z., Jiang X. M., Ye L. (2014). Effects of temperature, light intensity and salinity on the growth and fatty acid composition of Skeletlnema munzelii SM-1 and SM-2. J. Biol. 31, 64–70. doi: 10.3390/ijms17060817
Giudetti A. M., Eleonora S., Luisa S., Gabriele V. G., Fabrizio D. (2016). Nutritional and hormonal regulation of citrate and carnitine/acylcarnitine transporters: Two mitochondrial carriers involved in fatty acid metabolism. International Journal of Molecular Sciences 17 (6), 817. doi: 10.3390/ijms17060817
Gladue R. M., Maxey J. E. (1994). Microalgal feeds for aquaculture. J. Appl. Phycol. 6, 131–141. doi: 10.1007/BF02186067
Gu H., Zhang X., Sun J., Luo Z. (2012). Diversity and seasonal occurrence of Skeletonema (Bacillariophyta) species in Xiamen Harbour and surrounding seas, China. Cryptogamie Algologie 33, 245–263. doi: 10.7872/crya.v33.iss3.2012.245
Guihéneuf F., Ulmann L., Mimouni V., Tremblin G. (2013). Use of radiolabeled substrates to determine the desaturase and elongase activities involved in eicosapentaenoic acid and docosahexaenoic acid biosynthesis in the marine microalga Pavlova lutheri. Phytochemistry 90, 43–49. doi: 10.1016/j.phytochem.2013.02.014
Harwood J. L. (1988). Fatty acid metabolism. Annu. Rev. Plant Physiol. Plant Mol. Biol. 39, 101–138. doi: 10.1146/annurev.pp.39.060188.000533
Herawati V. E., Hutabarat J., Radjasa O. K. (2014). Nutritional Content of Artemia sp. Fed with Chaetoceros calcitrans and Skeletonema costatum. HAYATI Journal of Biosciences. 21, 4, 166–172. doi: 10.4308/hjb.21.4.166
IPCC (2018). IPCC Special Report on Global warming of 1.5°C. Eds. Allen M., Babiker M., Chen Y., de Coninck H., Connors S., van Diemen R., Dube O., Ebi K., Engelbrecht F., Ferrat M., Ford J., Forster P., Fuss S., Guillén Bolaños T., Harold J., Hoegh-Guldberg O., Hourcade J. C., Huppmann D., Zickfeld K. (Geneva: IPCC).
Jiang H., Gao K. (2004). Effects of lowering temperature during culture on the production of polyunsaturated fatty acids in the marine diatom Phaeodactylum tricornutum (Bacillariophyceae). J. Phycol. 40, 651–654. doi: 10.1111/j.1529-8817.2004.03112.x
Jin P., Agustí S. (2018). Fast adaptation of tropical diatoms to increased warming with trade-offs. Sci. Rep. 8, 17771. doi: 10.1038/s41598–018-36091-y
Kooistra W., Gersonde R., Medlin I., Mann D. (2007). The origin and evolution of the diatoms: Their adaptation to a planktonic existence. Evol. Primary Producers Sea. 58 (11), 207–249. doi: 10.1016/B978-012370518-1/50012-6
Kroth P. G., Chiovitti A., Gruber A., Martin-Jezequel V., Mock T., Parker M. S., et al. (2008). A model for carbohydrate metabolism in the diatom Phaeodactylum tricornutum deduced from comparative whole genome analysis. PloS One 3, e1426. doi: 10.1371/journal.pone.0001426
Laufkötter C., Vogt M., Gruber N., Aita-Noguchi M., Aumont O., Bopp L., et al. (2015). Drivers and uncertainties of future global marine primary production in marine ecosystem models. Biogeosciences 12, 6955–6984. doi: 10.5194/bg-12-6955-2015
Lestari D. P., Ekawati A. W., Maftuch M. (2014). Dried Skeletonema costatum in feed formulation for the growth of Vaname Shrimp (Litopenaeus vannamei). J. Exp. Life Sci. 4, 45–49. doi: 10.21776/ub.jels.2014.004.02.04
Liang Y., Koester J. A., Liefer J. D., Irwin A. J., Finkel Z. V. (2019). Molecular mechanisms of temperature acclimation and adaptation in marine diatoms. ISME J. 13, 2415–2425. doi: 10.1038/s41396-019-0441-9
Maia I. B., Carneiro M., Magina T., Malcata F. X., Otero A., Navalho J., et al. (2022). Diel biochemical and photosynthetic monitorization of Skeletonema costatum and Phaeodactylum tricornutum grown in outdoor pilot-scale flat panel photobioreactors. J. Biotechnol. 343, 110–119. doi: 10.1016/j.jbiotec.2021.11.008
Marella T. K., Tiwari A. (2020). Marine diatom Thalassiosira weissflogii based biorefinery for co-production of eicosapentaenoic acid and fucoxanthin. Bioresour. Technol. 307, 123245. doi: 10.1016/j.biortech.2020.123245
Mimouni V., Ulmann L., Tremblin G., Robert J. M. (2003). Desaturation of linoleic acid in the marine diatom Haslea ostrearia Simonsen (Bacillariophyceae). Cryptogamie Algol. 24, 269–276.
Mishra B., Tiwari A. (2021). Cultivation of Anabena variabilis, Synechococcus elongatus, Spirulina platensis for the production of C-Phycocyanin, C-Phycoerythrin and Thalassiosira, Skeletonema, Chaetoceros for fucoxanthin. Syst. Microbiol. Biomanuf. 1, 356–361. doi: 10.1007/s43393-020-00020-w
Murata N., Los D. (1997). Membrane fluidity and temperature perception. Plant Physiol. 115, 875–879. doi: 10.1104/pp.115.3.875
Nodumo N. Z., Krzysztof Z., Katharina V., Ivo F. (2018). Current trends to comprehend lipid metabolism in diatoms. Prog. Lipid Res. 70, 1–16. doi: 10.1016/j.plipres.2018.03.001
O'Donnell D. R., Du Z. Y., Litchman E. (2019). Experimental evolution of phytoplankton fatty acid thermal reaction norms. Evol. Appl. 12, 1201–1211. doi: 10.1111/eva.12798
Ogura A., Akizuki Y., Imoda H., Mineta K., Gojobori T., Nagai S. (2018). Comparative genome and transcriptome analysis of diatom, Skeletonema costatum, reveals evolution of genes for harmful algal bloom. BMC Genomics 19, 765. doi: 10.1186/s12864-018-5144-5
Pasquet V., Ulmann L., Mimouni V., Guihéneuf F., Jacquette B., Morant-Manceau A., et al. (2014). Fatty acids profile and temperature in the cultured marine diatom Odontella aurita. J. Appl. Phycol. 26, 2265–2271. doi: 10.1007/s10811-014-0252-3
Quinn P. J. (1981). The fluidity of cell membranes and its regulation. Prog. Biophys. Mol. Biol. 38, 1–104. doi: 10.1016/0079-6107(81)90011-0
Ratnayake W. M. N., Galli C. (2009). Fat and fatty acid terminology, methods of analysis and fat digestion and metabolism: a background review paper. Ann. Of Nutr. And Metab. 55, 8–43. doi: 10.1159/000228994
Reitan K. I., Rainuzzo J. R., Øie G., Olsen Y. (1997). A review of the nutritional effects of algae in marine fish larvae. Aquaculture 155, 207–221. doi: 10.1016/S0044-8486(97)00118-X
Renaud S. M., Thinh L. V., Lambrinidis G., Parry D. L. (2002). Effect of temperature on growth, chemical composition and fatty acid composition of tropical Australian microalgae grown in batch cultures. Aquaculture 211, 195–214. doi: 10.1016/S0044-8486(01)00875-4
Renaud S. M., Zhou H. C., Parry D. L., Thinh L.-V., Woo K. C. (1995). Effect of temperature on the growth, total lipid content and fatty acid composition of recently isolated tropical microalgae Isochrysis sp., Nitzschia closterium, Nitzschia paleacea, and commercial species Isochrysis sp. (clone T.ISO). J. Appl. Phycol. 7, 595–602. doi: 10.1007/BF00003948
Roleda M. Y., Slocombe S. P., Leakey R. J. G., Day J. G., Bell E. M., Stanley M. S. (2013). Effects of temperature and nutrient regimes on biomass and lipid production by six oleaginous microalgae in batch culture employing a two-phase cultivation strategy. Bioresour. Technol. 129, 439–449. doi: 10.1016/j.biortech.2012.11.043
Roy S. S., Pal R. (2015). Microalgae in aquaculture: A review with special references to nutritional value and fish dietetics. Proc. Zool. Soc. 68, 1–8. doi: 10.1007/s12595-013-0089-9
Sales R., Galafat A., Vizcaíno A. J., Sáez M. I., Martínez T. F., Cerón-García M. C., et al. (2021). Effects of dietary use of two lipid extracts from the microalga Nannochloropsis gaditana (Lubián 1982) alone and in combination on growth and muscle composition in juvenile gilthead seabream, Sparus aurata. Algal Res. 53, 102–162. doi: 10.1016/j.algal.2020.102162
Sarker P. K. (2023). Microorganisms in fish feeds, technological innovations, and key strategies for sustainable aquaculture. Microorganisms 11, 439. doi: 10.3390/microorganisms11020439
Sarker P. K., Kapuscinski A. R., Lanois A. J., Livesey E. D., Bernhard K. P., Coley M. L. (2016). Towards sustainable aquafeeds: Complete substitution of fish oil with marine microalga Schizochytrium sp. improves growth and fatty acid deposition in Juvenile Nile Tilapia (Oreochromis niloticus). PloS One 11, e0156684. doi: 10.1371/journal.pone.0156684
Sarker P. K., Kapuscinski A. R., Vandenberg G. W., Proulx E., Sitek A. J. (2020). Towards sustainable and ocean-friendly aquafeeds: Evaluating a fish-free feed for rainbow trout (Oncorhynchus mykiss) using three marine microalgae species. Elementa: Sci. Anthropocene 8, 5. doi: 10.1525/elementa.404
Sepúlveda J., Cantarero S. I. (2022). Phytoplankton response to a warming ocean. Science 376, 1378–1379. doi: 10.1126/science.abo5235
Shah M. R., Lutzu G. A., Alam A., Sarker P., Chowdhury M. A. K., Parsaeimehr A., et al. (2018). Microalgae in aquafeeds for a sustainable aquaculture industry. J. Appl. Phycol. 30, 197–213. doi: 10.1007/s10811-017-1234-z
Shevchenko O. G., Ponomareva A. A., Turanov S., Dutova D. I. (2019). Morphological and genetic variability of Skeletonema dohrnii and Skeletonema japonicum (Bacillariophyta) from the northwestern Sea of Japan. Phycologia. 58 (1), 1–13. doi: 10.1080/00318884.2018.1517540
Sunda W. G., Price N. M., Morel F. M. (2005). Trace metal ion buffers and their use in culture studies. Algal Culturing Techniques 4, 35–63. doi: 10.1016/b978-012088426-1/50005-6
Thangaraj S., Giordano M., Sun J. (2020). Comparative proteomic analysis reveals new insights into the common and specific metabolic regulation of the diatom. Front. Plant Sci. 11, 578915. doi: 10.3389/fpls.2020.578915
Thangaraj S., Shang X. M., Sun J., Liu H. J. (2019). Quantitative proteomic analysis reveals novel insights into intracellular silicate stress-responsive mechanisms in the diatom Skeletonema dohrnii. Int. J. Mol. Sci. 20, 2540. doi: 10.3390/ijms20102540
Thangaraj S., Sun J. (2020). The biotechnological potential of the marine diatom Skeletonema dohrnii to the elevated temperature and pCO2. Mar. Drugs 18, 259. doi: 10.3390/md18050259
Valenzuela-Espinoza E., Millán-Núñez R., Núñez-Cebrero F. (1999). Biomass production and nutrient uptake by Isochrysis aff. galbana (Clone T-ISO) cultured with a low cost alternative to the f/2 medium. Aquacultural Engineering 20 (3), 145–147. doi: 10.1016/S0144-8609(99)00011-4
van Houcke J., Medina I., Maehre H. K., Cornet J., Cardinal M., Linssen J., et al. (2017). The effect of algae diets (Skeletonema costatum and Rhodomonas baltica) on the biochemical composition and sensory characteristics of Pacific cupped oysters (Crassostrea gigas) during land-based refinement. Food Res. Int. 100, 151–160. doi: 10.1016/j.foodres.2017.06.041
Velasquez S. F., Chan M. A., Abisado R. G., Traifalgar R. F. M., Tayamen M. M., Maliwat G. C. F., et al. (2016). Dietary Spirulina (Arthrospira platensis) replacement enhances performance of Juvenile Nile tilapia (Oreochromis niloticus). J. Appl. Phycol. 28, 1023–1030. doi: 10.1007/s10811-015-0661-y
Xu H. G., Giovanni M. T., David S. F., Liang M. Q., Thomas S. M., Artur R., et al. (2020). Are fish what they eat? A fatty acid’s perspective. Prog. Lipid Res. 80, 101064. doi: 10.1016/j.plipres.2020.101064
Yamada M., Katsuki E., Otsubo M., Kawaguchi M., Ichimi K., Kaeriyama H., et al. (2010). ). Species diversity of the genus Skeletonema (Bacillariophyceae) in the industrial harbor Dokai Bay, Japan. J. Oceanogr. 66, 755–771. doi: 10.1007/s10872-010-0062-4
Yi Z., Xu M., Di X., Brynjolfsson S., Fu W. (2017). Exploring valuable lipids in diatoms. Front. Mar. Sci. 4. doi: 10.3389/fmars.2017.00017
Zhao D. H., Zhao L. D., You H., Qin S., Wang Y. S., Jiao X. D. (2021). Breeding and feeding characteristics of high-temperature-resistant strains of Isochrysis galbana. Oceanol Limnol Sin. 52, 206–212. doi: 10.11693/hyhz20200300091
Zhukova N. V., Aizdaicher N. A. (1995). Fatty acid composition of 15 species of marine microalgae. Phytochemistry 39, 351–356. doi: 10.1016/0031-9422(94)00913-E
Zulu N. N., Zienkiewicz K., Vollheyde K., Feussner I. (2018). Current trends to comprehend lipid metabolism in diatoms. Prog. Lipid Res. 70, 1–16. doi: 10.1016/j.plipres.2018.03.001
Zuo R., Ai Q., Mai K., Xu W., Wang J., Xu H., et al. (2012). Effects of dietary n-3 highly unsaturated fatty acids on growth, nonspecific immunity, expression of some immune related genes and disease resistance of large yellow croaker (Larmichthys crocea) following natural infestation of parasites (Cryptocaryon irritans). Fish Shellfish Immunol. 32, 249–258. doi: 10.1016/j.fsi.2011.11.005
Keywords: Skeletonema dohrnii, unsaturated fatty acids, temperature, aquafeed, ocean warming
Citation: Shang X, Yang Y, Zan Y, Sun Z, Lu Z and Sun J (2024) Effects of temperature on the growth, total lipid content and fatty acid composition of Skeletonema dohrnii. Front. Mar. Sci. 11:1361157. doi: 10.3389/fmars.2024.1361157
Received: 25 December 2023; Accepted: 07 May 2024;
Published: 24 May 2024.
Edited by:
Jingzhen Wang, Beibu Gulf University, ChinaReviewed by:
Dinesh Kumar Sundarraj, Central Salt & Marine Chemicals Research Institute (CSIR), IndiaCopyright © 2024 Shang, Yang, Zan, Sun, Lu and Sun. This is an open-access article distributed under the terms of the Creative Commons Attribution License (CC BY). The use, distribution or reproduction in other forums is permitted, provided the original author(s) and the copyright owner(s) are credited and that the original publication in this journal is cited, in accordance with accepted academic practice. No use, distribution or reproduction is permitted which does not comply with these terms.
*Correspondence: Xiaomei Shang, c2hhbmd4aWFvbWVpQHR1c3QuZWR1LmNu; Jun Sun, cGh5dG9wbGFua3RvbkAxNjMuY29t
Disclaimer: All claims expressed in this article are solely those of the authors and do not necessarily represent those of their affiliated organizations, or those of the publisher, the editors and the reviewers. Any product that may be evaluated in this article or claim that may be made by its manufacturer is not guaranteed or endorsed by the publisher.
Research integrity at Frontiers
Learn more about the work of our research integrity team to safeguard the quality of each article we publish.