- 1National Marine Science Centre, Faculty of Science and Engineering, Southern Cross University, Coffs Harbour, NSW, Australia
- 2Southern Shark Ecology Group, College of Science and Engineering, Flinders University, Bedford Park, SA, Australia
- 3New South Wales (NSW) Department of Primary Industries, Wollongbar Primary Industries Institute, Wollongbar, NSW, Australia
- 4New South Wales (NSW) Department of Primary Industries, National Marine Science Centre, Coffs Harbour, NSW, Australia
White sharks (Carcharodon carcharias) play a crucial ecological role, shaping ecosystems through direct predation and risk effects. On the east coast of Australia, immature white sharks are broadly distributed, inhabiting a wide range of habitats and ecosystems from temperate Tasmania to tropical North Queensland. Using stable isotopes and fatty acids of muscle and plasma, we examined the diet and habitat use of 136 immature white sharks (152–388 cm total length) captured on SMART drumlines on the East Australian coast. This facilitated the temporal assessment of white shark trophic ecology from a few weeks to approximately a year. Biochemistry of muscle samples showed that white sharks predominantly feed on low trophic level prey from coastal environments. A seasonal shift in diet was evident, with the increasing proportions of essential fatty acids in muscle tissues during spring and summer suggesting a greater consumption of high-nutrition preys during those months compared to autumn and winter. By combining stable isotope and fatty acid analysis, we gain a comprehensive understanding of immature white shark diet in eastern Australia. Our results confirm that white sharks are generalist predators that exhibit seasonal shifts in their diet. Their high use of coastal habitats reinforces the importance of these areas for foraging, which is crucial for growth and development during this critical life stage.
1 Introduction
Highly mobile marine predators play crucial ecological roles by connecting spatially separated food webs and shaping ecosystems through diet and habitat use (Heithaus and Dill, 2002; Heithaus et al., 2010; Williams et al., 2018). In marine ecosystems, diet and energetic requirements impact an individual’s ability to execute intrinsic biological processes necessary for migration, reproduction, and survival (de Sousa Rangel et al., 2021a). Such energetic demands vary throughout an animal’s life stages, influencing trophic role, foraging preferences and capabilities throughout ontogeny (Kohl et al., 2015; Chaguaceda et al., 2020). The trophic ecology of many marine predators, such as sharks, is well documented (Hussey et al., 2015) but often focuses on large mature individuals (Heithaus and Dill, 2002; Huveneers et al., 2018). Understanding the diet of marine predators across all life stages is essential, as resource availability and feeding preferences influence shark habitat selection, particularly in early life stages (Krausman, 1999; Heithaus, 2007).
Insight into the diet of marine predators is often limited to brief snapshots of recently consumed prey from direct observations of predation, stomach content analysis, or, more recently, genetic analyses of faecal material (Munroe et al., 2018; Clark et al., 2023). However, relying solely on snapshots of recently ingested meals may lead to inaccurate assessments of diet and foraging patterns because most recent meals might not represent a species’ diet comprehensively. Alternative indirect measures, e.g., biochemical tracers, provide valuable information over broader and more ecologically relevant spatial and temporal scales and are used to quantify and estimate resource use over weeks to years (Hussey et al., 2011; Pethybridge et al., 2014; Raoult et al., 2019; Meyer et al., 2021). Based on the principle ‘you are what you eat’, elements and compounds within prey items are assimilated into consumer tissue with minimal or predictable modification and can be traced upwards from the base of the food chain (Iverson et al., 2004; Budge et al., 2006; Munroe et al., 2018). Biochemical tracers can reveal diet, feeding patterns, and habitat use (Carlisle et al., 2021; Meyer et al., 2021), with short-term information (~30 days) gained from metabolically active tissues like plasma and liver (Hussey et al., 2012a), while less active tissue like muscle provides longer-term (months to years) information (Kim et al., 2012a).
Stable isotopes of carbon and nitrogen are widely used biochemical tracers owing to their ability to quantify basal carbon sources and trophic levels, respectively. This enables stable isotope analyses to elucidate a predator’s dietary composition and specialisation, ontogenetic changes, variations in habitat use, and ecological dynamics within a community (Peterson and Fry, 1987; Carlisle et al., 2012; Pethybridge et al., 2018). Additionally, the use of lipids and fatty acids can be used to infer the diet, habitat use, nutritional condition, and physiology of elasmobranchs (Pethybridge et al., 2011; Gallagher et al., 2017; Meyer et al., 2017; Munroe et al., 2018). Fatty acids are critical for physiological functions, including growth, development, reproduction, and cellular maintenance (Sargent et al., 1995; Tocher, 2003). However, elasmobranchs have limited capacity for lipid oxidation in extrahepatic tissues (Zammit and Newsholme, 1979; Ballantyne, 1997), and essential fatty acids, including arachidonic (ARA), eicosapentaenoic (EPA), and docosahexaenoic (DHA), cannot be synthesised de novo (Sargent et al., 1995). Therefore, fatty acids must be obtained through diet, and many remain relatively unchanged, enabling their use as dietary biomarkers (Dalsgaard et al., 2003; Iverson, 2009). Fatty acid incorporation in tissues is substantially quicker than stable isotopes, with shifts in consumer nutrition reflected in muscle and blood fatty acid profiles within weeks rather than months or years (Beckmann et al., 2013). Subsequently, fatty acids are useful biomarkers, providing a fine-scale assessment of spatiotemporal changes in diet and nutrition (Rohner et al., 2013; Alderete-Macal et al., 2020; Meyer et al., 2021).
On the east coast of Australia, juvenile and sub-adult white sharks (hereafter referred to as immature white sharks) are broadly distributed, inhabiting a wide range of coastal habitats and ecosystems from temperate Tasmania and Bass Strait (Victoria) in summer to tropical regions as far north as the southern Great Barrier Reef (Queensland) in winter (Bruce and Bradford, 2012; Bruce et al., 2019). Telemetry studies reveal that immature white sharks undertake seasonal north-south migrations, spending increased time in southern Queensland and northern New South Wales from September, before moving to southern New South Wales and Victorian waters in March (Spaet et al., 2020b). Similar patterns of seasonal movement have been reported in the North Atlantic (Skomal et al., 2017; Franks et al., 2021), South Africa (Towner et al., 2013), and the north-east Pacific (Weng et al., 2007). Although highly migratory, immature white sharks spend more time in so-called ‘nursery’ areas between Lake Macquarie (33.3°S) and South West Rocks (30.8°S), returning in consecutive years (Bruce et al., 2019).
Previous studies using stomach contents and fatty acids, and more recently environmental DNA (eDNA) metabarcoding, indicate the diet of these white sharks comprises primarily of finned fishes, benthic batoids, squid and marine mammals (Pethybridge et al., 2014; Grainger et al., 2020; Clark et al., 2023). However, inconsistencies between these studies are evident. Stomach contents evaluated by Grainger et al. (2020) found Australian salmon (Arripis trutta) had high occurrence, compared to sea mullet (Mugil cephalus) using eDNA metabarcoding by Clark et al. (2023). These discrepancies highlight the variability in white shark diet likely attributed to spatial and temporal differences within the sampling regime. Earlier studies (Pethybridge et al., 2014; Grainger et al., 2020) relied on opportunistic sampling from fisheries bycatch or shark meshing programs, with sample sizes of 21 and 52, respectively, and limited samples collected during winter, when white shark presence is high Spaet et al. (2020b). Although the novel method of environmental DNA described by Clark et al. (2023) provides a higher taxonomic resolution than biochemical tracers, a lack of reference sequence data may result in underrepresentation, while sample contamination from the surrounding environment may lead to false positives (Beng and Corlett, 2020). These studies have provided valuable insight into the diet of east coast white sharks, but temporal changes in the diet of immature white sharks in this region are yet to be described. Seasonal fluctuations in prey abundance are persistent on the east coast of Australia (Brodie et al., 2017), and the dynamic oceanographic conditions influence prey distribution (Hobday et al., 2011).
The East Australian Current (EAC) is a western boundary current that flows from the Coral Sea, southward into New South Wales (Ridgway and Godfrey, 1997). Seasonal north-south expansion and contraction of the East Australian Current, in addition to high mesoscale kinetic energy, facilitates the distribution of chlorophyll a along the coastline (Everett et al., 2014; Liu et al., 2022). In northern areas at latitudes between 28.5°S and 31°S, the shelf narrows, leading to upwelling of nutrient-rich water and increases in primary productivity (Everett et al., 2014). The EAC has the strongest influence on shelf waters during late spring and summer, affecting the abundance and distribution of large marine predators along the east coast (Young et al., 2010; Hobday et al., 2011; Lee et al., 2021). Therefore, predators may require plasticity in habitat use and foraging patterns to meet energy and nutritional requirements in such fluctuating environments. Adaptation and responses to ephemeral prey availability remain species-specific (Terraube et al., 2011; Munroe et al., 2014), yet vital, as predators with the capability to adapt will be able to cope better with the forecasted impacts on environments and preys in the Anthropocene.
In the present study, we detail the trophic ecology of immature white sharks along Australia’s east coast across temporal scales by using biochemical tracers and tissues reflecting diet from a few weeks up to a year. Specifically, we calculate annual and seasonal diet composition and infer foraging habitat from nitrogen and carbon stable isotopes. Fatty acid signatures of muscle and plasma were also used to investigate shifts in diet and trophic pathways. Together, these methods provide an integrated understanding of immature white shark diet and habitat use within a highly dynamic coastal ecosystem. Based on the documented seasonal movements of immature white sharks through acoustic and satellite telemetry and seasonal changes in prey distribution in this region, we hypothesised stable isotope and fatty acid values to reflect a seasonal shift in diet and nutritional condition.
2 Materials and methods
To quantify the diet and examine the foraging habitat of white sharks, 102 muscle and 39 blood samples (136 individuals; 8 sharks had both muscle and blood collected) were collected from sharks caught between Ballina (28.811° S 153.610° E) and Forster (32.180° S 152.511° E), New South Wales, Australia, from 9 August 2016 to 3 February 2022 (Figure 1). We captured sharks using SMART (Shark-Management-Alert-in-Real-Time) drumlines deployed in coastal waters as part of the New South Wales Department of Primary Industries (NSW DPI) Shark Management Program (see Lipscombe et al., 2023 and Tate et al., 2021 for gear configuration and deployment). Previous studies have shown that the time spent on the line using this capture method does not impact plasma fatty acids, enabling the confident use of plasma fatty acids to examine diet and habitat use (Gallagher et al., 2019; Tate et al., 2019). We recorded sex and size (total length [TL] to the nearest cm). Sharks ranged from 152–388 cm TL, including six young-of-the-year < 175 cm TL, 119 juveniles 176–300 cm TL, and 11 sub-adults 300–388 cm TL (Bruce and Bradford, 2012).
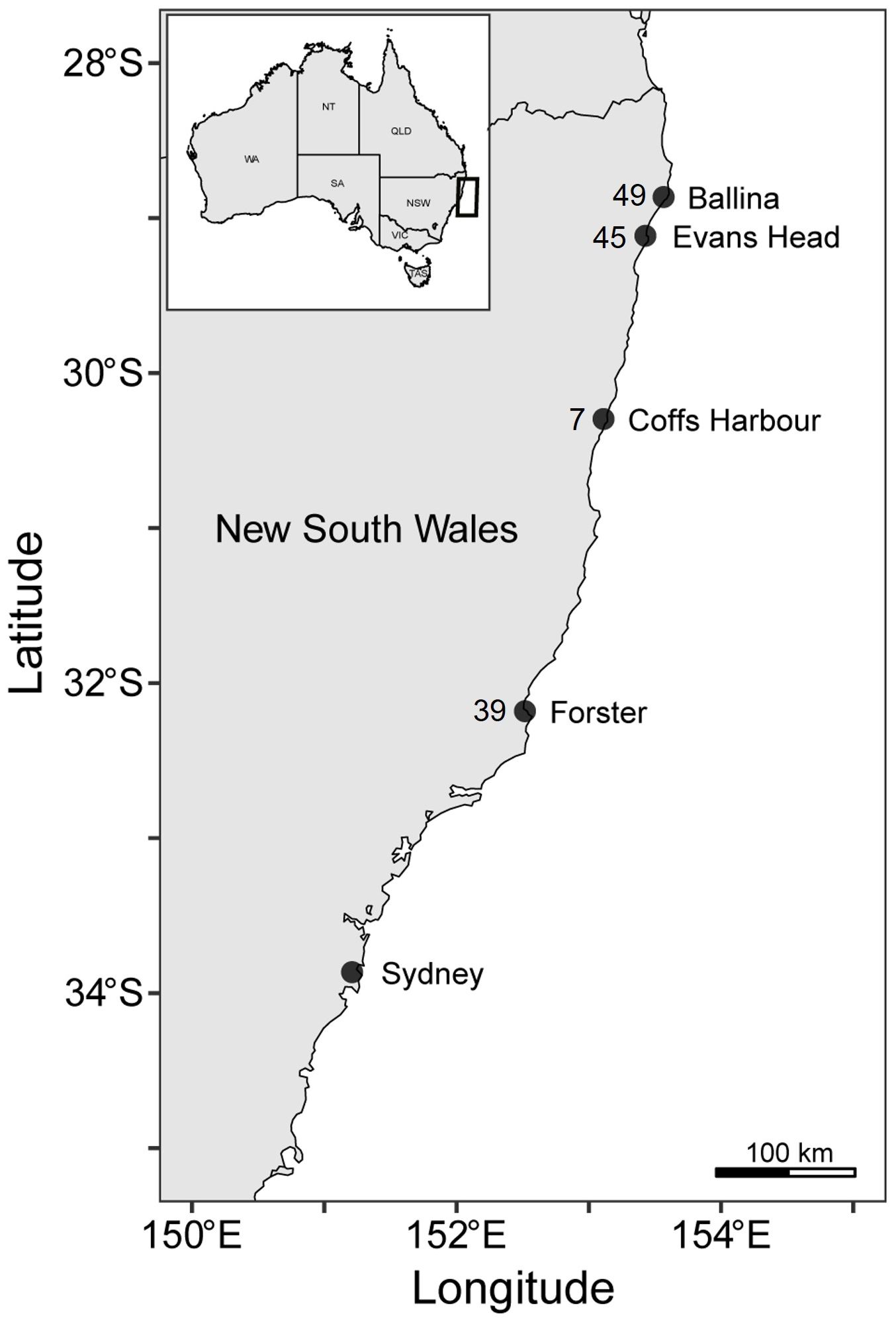
Figure 1 Sampling location and number of samples collected from white sharks in New South Wales, Australia.
2.1 Sample collection and preparation
We sampled the epaxial muscle adjacent to the dorsal fin using a hand-held 1 cm diameter stainless steel biopsy and stored on ice before transferring to a -18°C freezer. We collected blood via caudal venipuncture and placed into a lithium-heparin plasma separator vial (BD Vacutainer), centrifuged at 1534 ×g for 3 min and pipetted the plasma into 2 mL vials. We stored the plasma samples temporarily at -18°C in the field and later transferred them to a freezer at -80°C.
Lipids were extracted from samples for subsequent fatty acid analysis to ensure lipid content did not bias carbon isotopes (Newsome et al., 2010; Carlisle et al., 2017). We lyophilised muscle (0.1 ± SD 0.01 g) and plasma (1.2 ± SD 0.78 g) for 48 h (Alpha LD14 plus freeze dryer) and homogenised the samples in a TissueLyser LT (Qiagen) for 2 min at 50 Hz to facilitate optimal lipid extraction. Using a modified Bligh and Dyer (1959) method, lipids were extracted from the lyophilised tissue using 3.8 mL of dichloromethane:methanol:Milli-Q (1:2:0.8 mL) solution for approximately 18 h. We centrifuged the solution at 1534 ×g for 2 min and transferred the supernatant into a glass tube, where we separated the polar and non-polar phases using 1 mL each of dichloromethane and 0.9% NaCl saline solution and centrifuged at 1534 ×g for 2 min. The lower non-polar layer was transferred to a 2 mL vial and dried it under N2 to a constant weight. We resuspended the total lipid extract in 1.5 mL dichloromethane and stored it at -80°C for subsequent fatty acid analysis.
We air-dried the lipid-extracted tissue overnight in a fume hood to remove residual solvents. After lipid extraction, we removed urea and TMAO using three rounds of sonication in deionised water, following Kim and Koch (2012). We then oven-dried samples at 60°C for 48 h, ground them to a fine powder for 3 min at 50 Hz using a TissueLyser LT (Qiagen) and prepared them for stable isotope analysis.
2.2 Fatty acid analysis
We analysed fatty acid profiles of 102 muscle (60 female; 42 male) and 39 plasma (26 female; 13 male) tissues following a modified Bligh and Dyer trans-methylation procedure (Bligh and Dyer, 1959; described in Meyer et al., 2017). An aliquot of the total lipid extract was trans-methylated using 3 mL of dichloromethane:methanol:hydrochloric acid (10:10:1 v/v/v) for 2 h at 80°C, then allowed to cool to room temperature before adding 1 mL of Milli-Q. We extracted the resulting fatty acid methyl esters (FAME) from the methylating solvent using three washes of 1.8 ml hexane:dichloromethane (4:1 v/v), centrifuged at 1534 ×g for 5 min, collected the upper solvent layer containing the FAMES in a 2 mL glass vial, dried under N2 gas, and suspended it in 1 ml of dichloromethane. We identified and quantified individual fatty acids through gas chromatography (GC) and GC-mass spectrometry (GC-MS). We achieved peak separation using an Agilent Technologies 7890B GC (Palo Alto, California USA) with an Equity-1 fused silica capillary column (15 mm × 0.1 mm internal diameter and 0.1 mm film thickness), a flame ionisation detector, a splitless injector, and an Agilent Technologies 7683B Series auto-sampler. Samples were injected in splitless mode and carried by helium gas at an oven temperature of 120°C. The temperature was raised to 270°C at a rate of 10°C per min and then to 310°C at a rate of 5°C per min. We quantified fatty acid peaks using Agilent Technologies ChemStation software (Palo Alto, California, USA) and confirmed identities using a Finnigan Thermoquest DSQ GC-MS system. Fatty acids were converted from chromatogram peak area to percentage of total area.
2.3 Stable isotope analysis
We weighed 102 dried muscle (60 female; 42 male) and 38 plasma (25 female; 13 male) samples from white sharks (10–20 mg) into tin capsules using an automated microbalance described by Carvalho (2021) to ensure maximum accuracy. We analysed samples for δ13C and δ15N using an isotope ratio mass spectrometer (Thermo Delta V Plus) coupled to an elemental analyser (Thermo Fisher Flash EA) via an interface (Thermo Fisher Conflo IV). Isotopic ratios are expressed in delta (δ) values as the deviations from conventional standards in parts per thousand (‰) using the following formula: δ13C or δ15N = [(Rsample/Rstandard – 1)] × 1000 (‰) where Rsample is the ratio of heavy to light isotope and Rstandard is the ratio of heavy to light isotope in the reference standard. We measured Rsample against internal working standards (glycine: δ13C = -41.8, δ15N = 2.0; glucose: δ13C = -10.5; collagen: δ13C = -21.5, δ15N = 4.8), which were calibrated against international reference materials [(USGS64: δ13C = -40.8, δ15N = 1.8; USGS65: δ13C = -20.3, δ15N = 20.7; USGS64: δ13C = -0.7, δ15N = 40.8 (Schimmelmann et al., 2016)]. We reported δ13C and δ15N values relative to international reference materials V-PDB (Vienna-Pee Dee Belemnite) and atmospheric nitrogen (N2) with a precision of 0.15 ‰ (δ13C) and 0.3 ‰ (δ15N).
2.4 Stable isotopes of prey samples
We collected muscle tissue samples from 18 potential white shark prey items to calculate prey contributions to white shark diet (Supplementary Table S1). Prey items were selected based on recent studies of immature white sharks in the same region (Grainger et al., 2020; Clark et al., 2023). Muscle samples were lipid-extracted using the above protocol, and urea was removed from elasmobranch prey samples using the outlined deionised water treatment. We could not obtain samples from cownose rays (Rhinoptera neglecta), so we used values from Raoult et al. (2019), which were not lipid extracted. To account for the effects of lipids on δ13C, these values were mathematically lipid-corrected before analyses using the below equation, described in Post et al. (2007).
2.5 Statistical analysis
Of the 57 fatty acids detected, we used those with averages > 0.1% (14 muscle; 17 plasma; Table 1). We used PRIMER 7 + PERMANOVA (Permutational multivariate analysis of variance; Plymouth Routines in Multivariate Ecological Research, Anderson, 2017) to run multivariate statistical analyses, and R v4.3.1 (R Core Team, 2023) for linear and Bayesian mixing models.
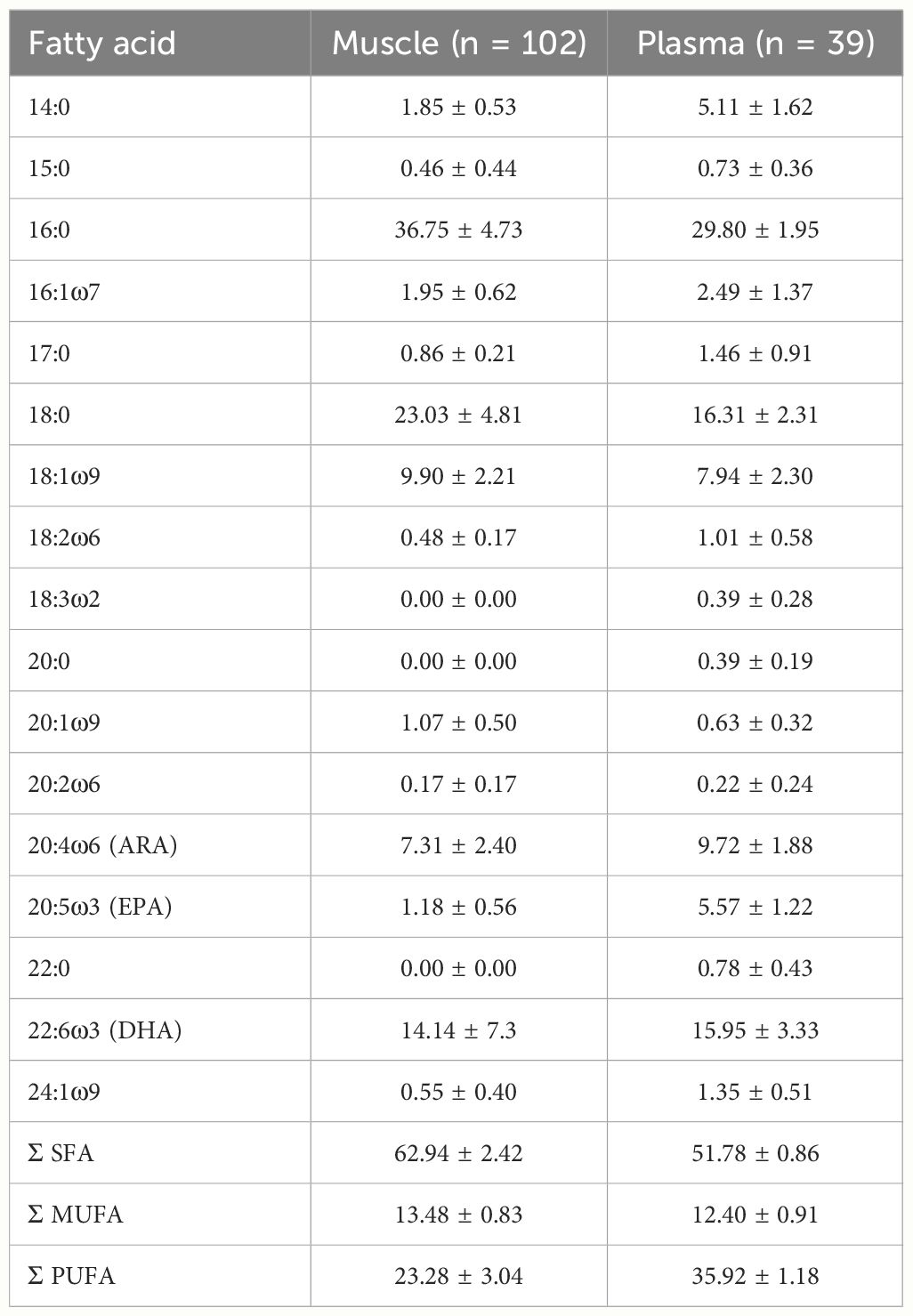
Table 1 Fatty acid relative composition (as percent of total fatty acid; mean ± SD) of white shark muscle and plasma collected in eastern Australia.
PERMANOVA analysis (Type I, based on 9999 permutations) was used to investigate differences in white shark fatty acid profiles using season and sex as fixed factors, location as a random factor, and total length as a continuous covariate. PERMANOVA analyses were completed using a Euclidean distance matrix on normalised untransformed data. Pairwise comparisons were performed if the main PERMANOVA tests showed significant differences. Principal Coordinate Ordination analysis (PCO) was used to visualise the clustering of individuals and correlation between fatty acids.
If the PERMANOVA showed significant differences in fatty acid profiles, we further explored dietary patterns by fitting univariate linear models to a subset of fatty acids selected for their ecological relevance and high correlation on the PCO. We were unable to include location as a factor in the models because of the low sample size across some locations (Supplementary Table S4). The effect of season (4 levels; fixed), sex (2 levels; fixed), and TL (continuous) on individual fatty acids (16:0, 18:0, 18:1ω9, ARA, EPA and DHA) was assessed by analysis of variance derived from each linear model. We used the same linear model to investigate changes in isotopic signatures in muscle and plasma:
Where Y is the vector of fatty acid or stable isotope values observed on each shark and X is a matrix of indicator variables with columns representing season of capture, sex and TL. The vector β contains coefficients estimated by maximum likelihood. All models were tested for normality and homogeneity in the residuals (e). The models were also used to estimate the mean response to season with 95% confidence intervals.
We used Bayesian stable isotope mixing models of the muscle samples using the simmr package (Govan et al., 2023) to calculate the overall contributions of prey sources to the diet of white sharks. We excluded plasma stable isotope values from the analysis, as the C:N after lipid extraction was greater than the threshold (3.5), introducing potential bias to the mixing model results (Post et al., 2007; Hussey et al., 2012a). Before running the mixing model, we visually assessed the isotopic signatures of consumers, ensuring they were generally within the isospace of sampled prey (Supplementary Table S1). After the application of the trophic enrichment factor, the following prey did not fall within the isospace of the consumers and were removed from the model (Supplementary Figures S2, S3): cownose ray, pilchards (Sardinops sagax), tiger shark (Galeocerdo cuvier), and bronze whaler (Carcharhinus obscurus).
We used K-means clustering; assigning observations to the group with nearest centroid so that within group variance is minimised, to aggregate the remaining prey items (n = 13; Table 2) into three groups. This was recommended by Phillips et al. (2014), whereby model accuracy is greatest when the number of sources is equal to the number of tracers + 1. Stable isotope values of prey groups were adjusted for trophic enrichment using values for lipid-extracted sand tiger shark (Carcharias taurus) muscle from Hussey et al. (2011; δ13C = 0.9 ± 0.3‰, δ15N = 2.29 ± 0.2‰). We ran the mixing model with 3600 iterations over 4 MCMC chains and deemed model convergence satisfactory through a Gelman diagnostic value of 1.
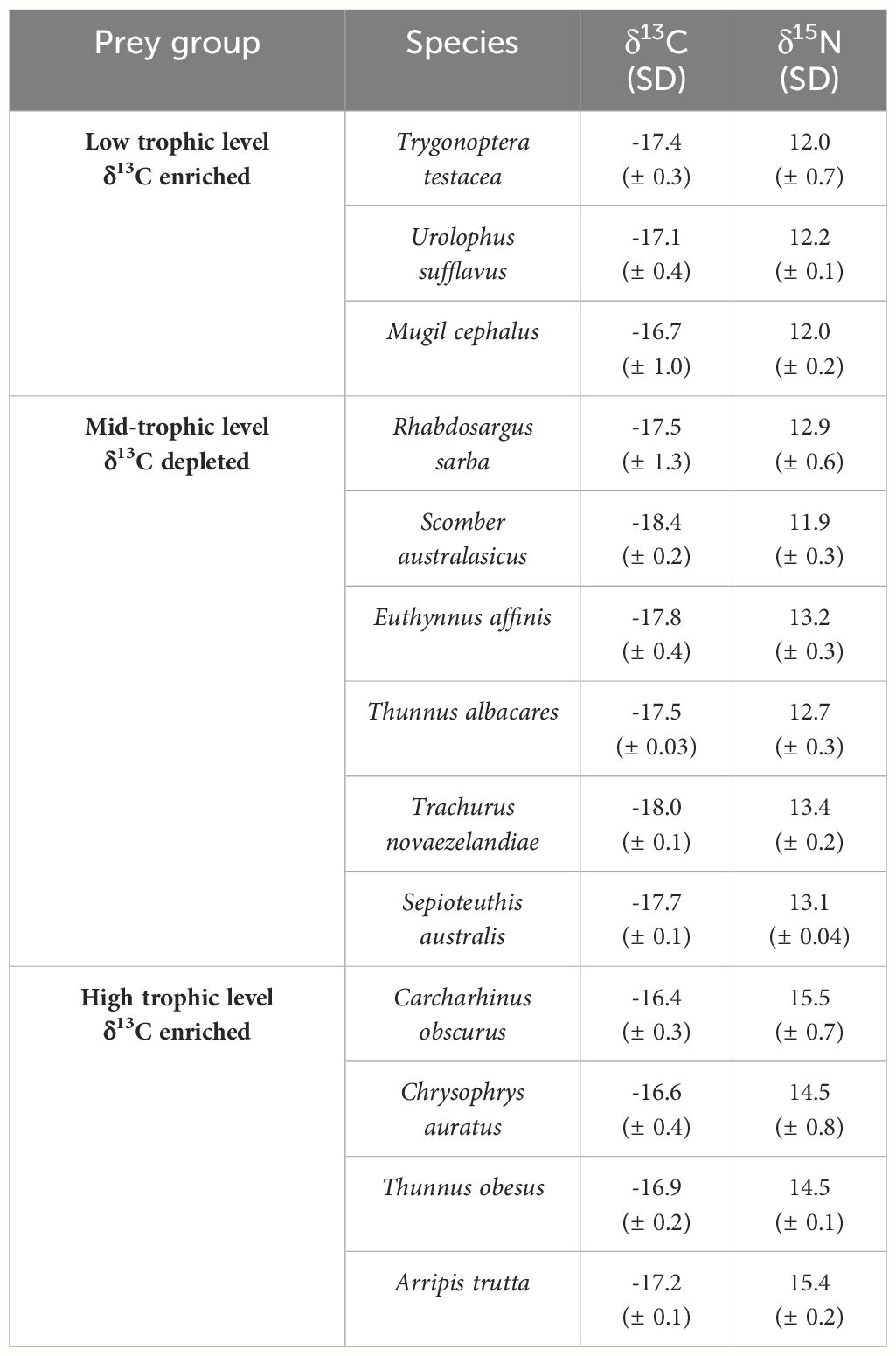
Table 2 Carbon and nitrogen isotope values (± SD) of grouped prey species used in the final Bayesian mixing model.
We ran separate Bayesian mixing models on winter, spring, and summer to examine proportional prey contribution differences among seasons. Autumn was excluded from this analysis to avoid unreliable contributions, as only four samples were collected during this season (Supplementary Table S4). Due to prey seasonality, two species, sea mullet (Mugil cephalus) and Australian salmon (Arripis trutta), were removed from the spring and summer models because their abundance is substantially reduced throughout these seasons (Lester et al., 2009; Hughes, 2012).
3 Results
3.1 Fatty acids
White shark muscle fatty acid profiles varied among seasons (PERMANOVA-pseudo-F = 4.34, p< 0.01) but were not influenced by sex or location (PERMANOVA- pseudo-F = 1.65, p > 0.05; Supplementary Table S5). Pairwise PERMANOVA revealed winter being different to both spring and summer, and autumn being different to both summer and spring (p< 0.05). Muscle samples collected in spring and summer had higher proportions of essential FA 20:4ω6 (ARA), 20:5ω3 (EPA), and 22:6ω3 (DHA) compared to samples collected in winter and autumn, which were dominated by saturated FAs 14:0, 16:0 and 18:0 (Figures 2, 3). Plasma fatty acid profiles, however, did not vary between season, sex, or location (Supplementary Table S5).
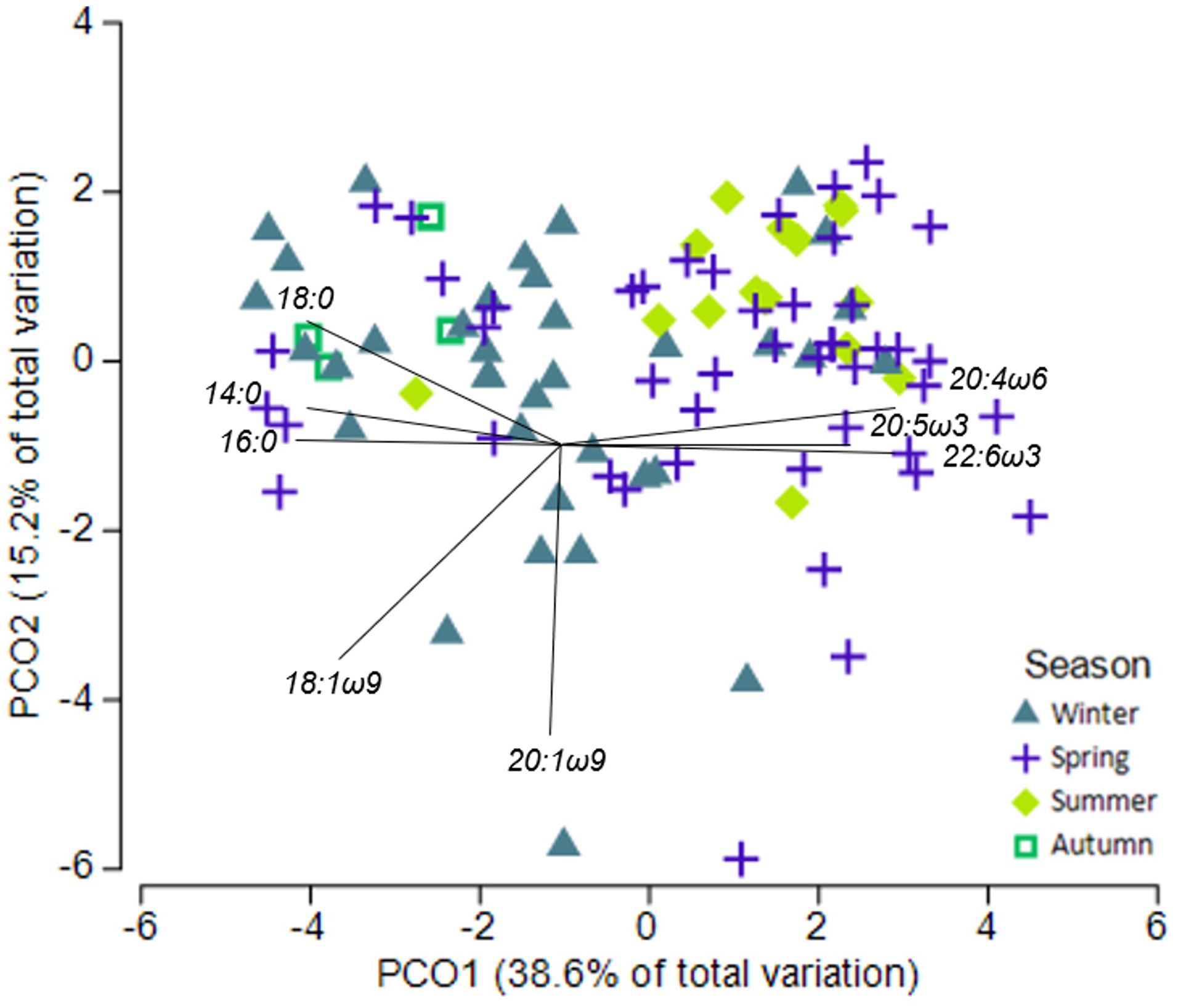
Figure 2 Principle coordinate ordination (PCO) of fatty acid profiles of white shark muscle and season. Vector overlay based on Pearson correlation with r > 0.7.
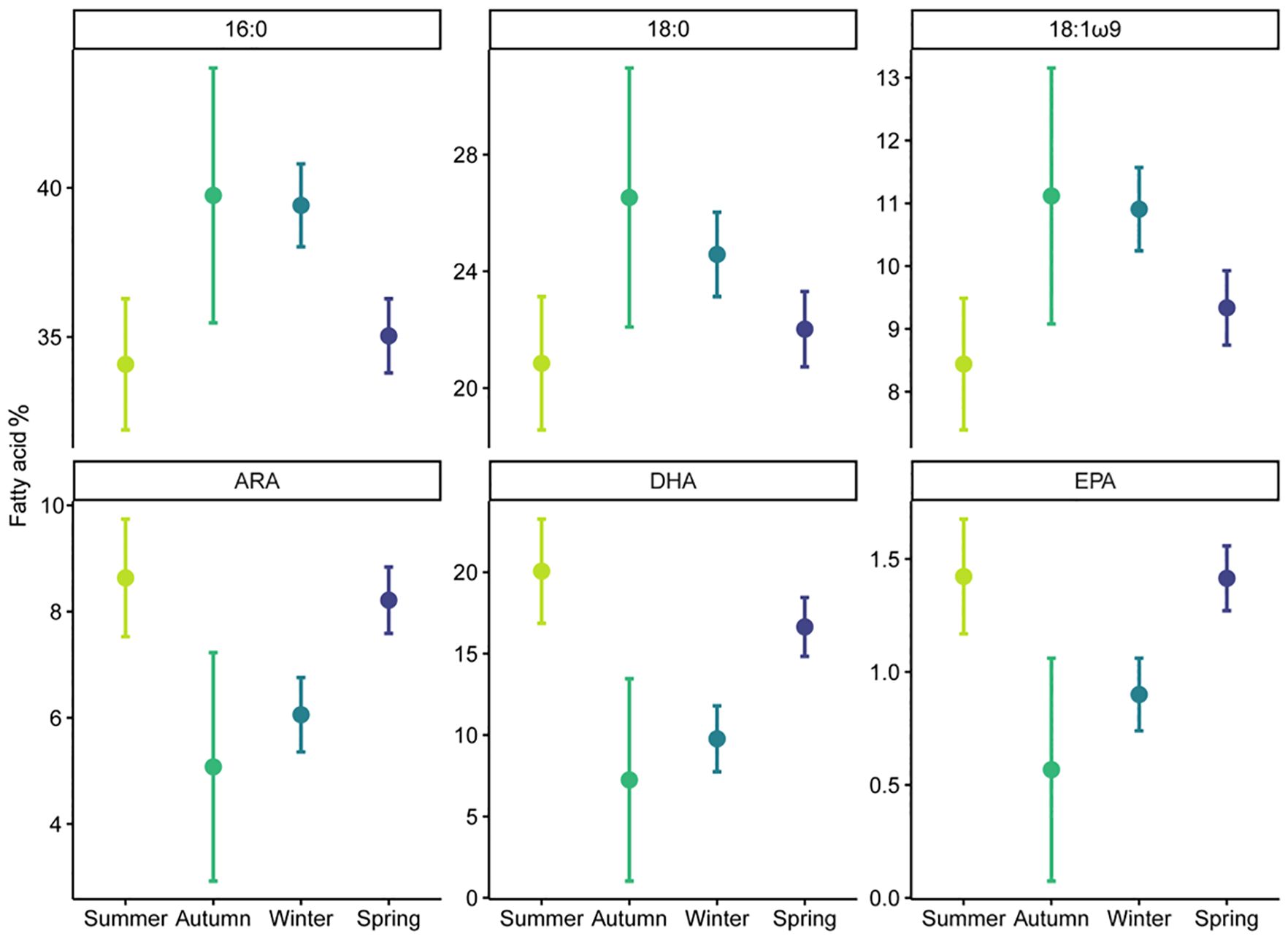
Figure 3 Seasonal variation (mean ± 95% CI) of fatty acid percentage for 16:0, 18:0, 18:1ω9, ARA (Arachidonic acid), DHA (Docosahexaenoic acid), and EPA (Eicosapentaenoic acid) in immature white shark muscle.
In muscle tissues, all six individual fatty acids were most affected by season, with autumn and winter having high 16:0, 18:0, and 18:1ω9, and low ARA, DHA, and EPA (Supplementary Tables S6, S7; Figure 3). Sex also affected muscle EPA and ARA (Supplementary Figure S8), while shark length affected muscle EPA and DHA (Supplementary Table S6; Supplementary Figure S9).
3.2 Stable isotopes
In white shark muscle tissue, δ13C ranged from -14.6 to -17.5‰ (mean ± standard deviation: -16.2 ± 0.5‰), while δ15N ranged from 14.6 to 16.4‰ (15.5 ± 0.3‰). Plasma δ13C ranged from -14.3 to -17.8‰ (-15.3 ± 0.8‰) and δ15N ranged from 12.4 to 14.9‰ (13.8 ± 0.5‰). Stable isotope values of muscle were influenced by seasons (Supplementary Table S10; Supplementary Figure S11), δ13C was lowest in winter and δ15N lowest in spring (Figure 4). Sex did not affect stable isotope values, but a weak but significant relationship existed in muscle and plasma δ15N and total length (Supplementary Figures S12, S13).
3.3 Stable isotope mixing model and diet composition
Stable isotope values of white shark tissue were within the isoscape of potential prey sources (Figure 5; Supplementary Figure S3). Low trophic level δ13C enriched prey were the primary resource used by immature white sharks and had an average contribution of 0.49 (± 0.04) to the annual diet of immature white sharks (Figure 6). High trophic level δ13C enriched prey) contributed an average of 0.37 (± 0.05), while mid-trophic level δ13C depleted prey contributed 0.14 to the diet of immature white sharks (± 0.01; Figure 6).
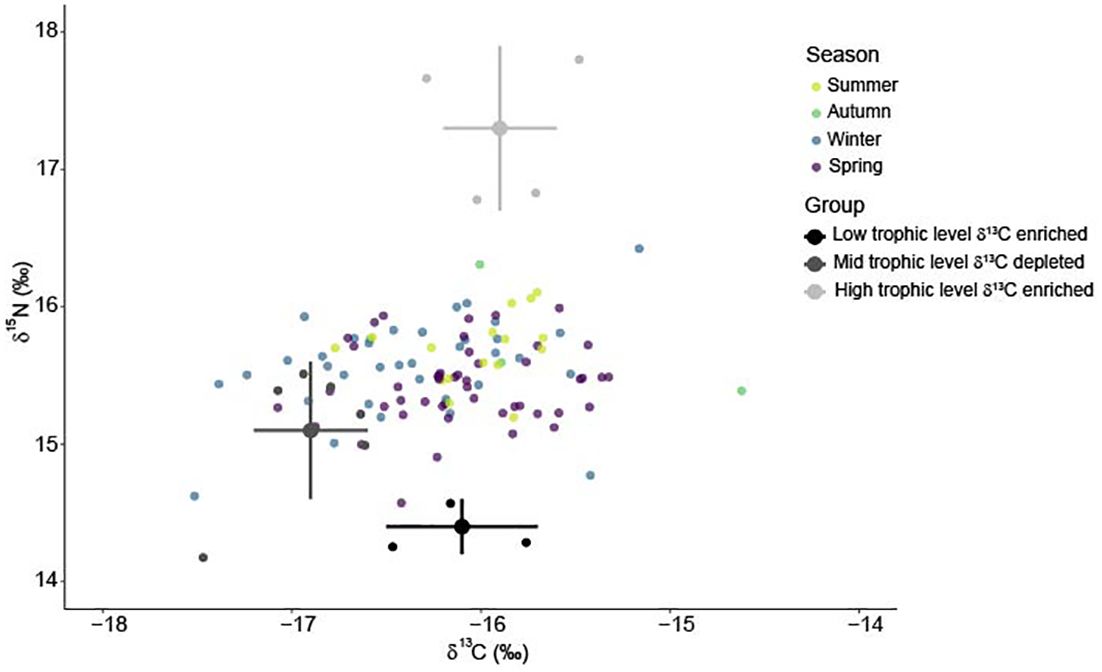
Figure 5 Biplot of white shark muscle δ13C and δ15N values (coloured by season) compared to the mean (± standard deviation) of three prey groups used in the Bayesian mixing model. Low trophic level δ13C enriched (), mid-trophic level δ13C depleted (
), high trophic level δ13C enriched (
).Prey values are corrected for trophic enrichment based on Hussey et al. (2010): + 2.3‰ δ15N values and + 0.9‰ δ13C values.
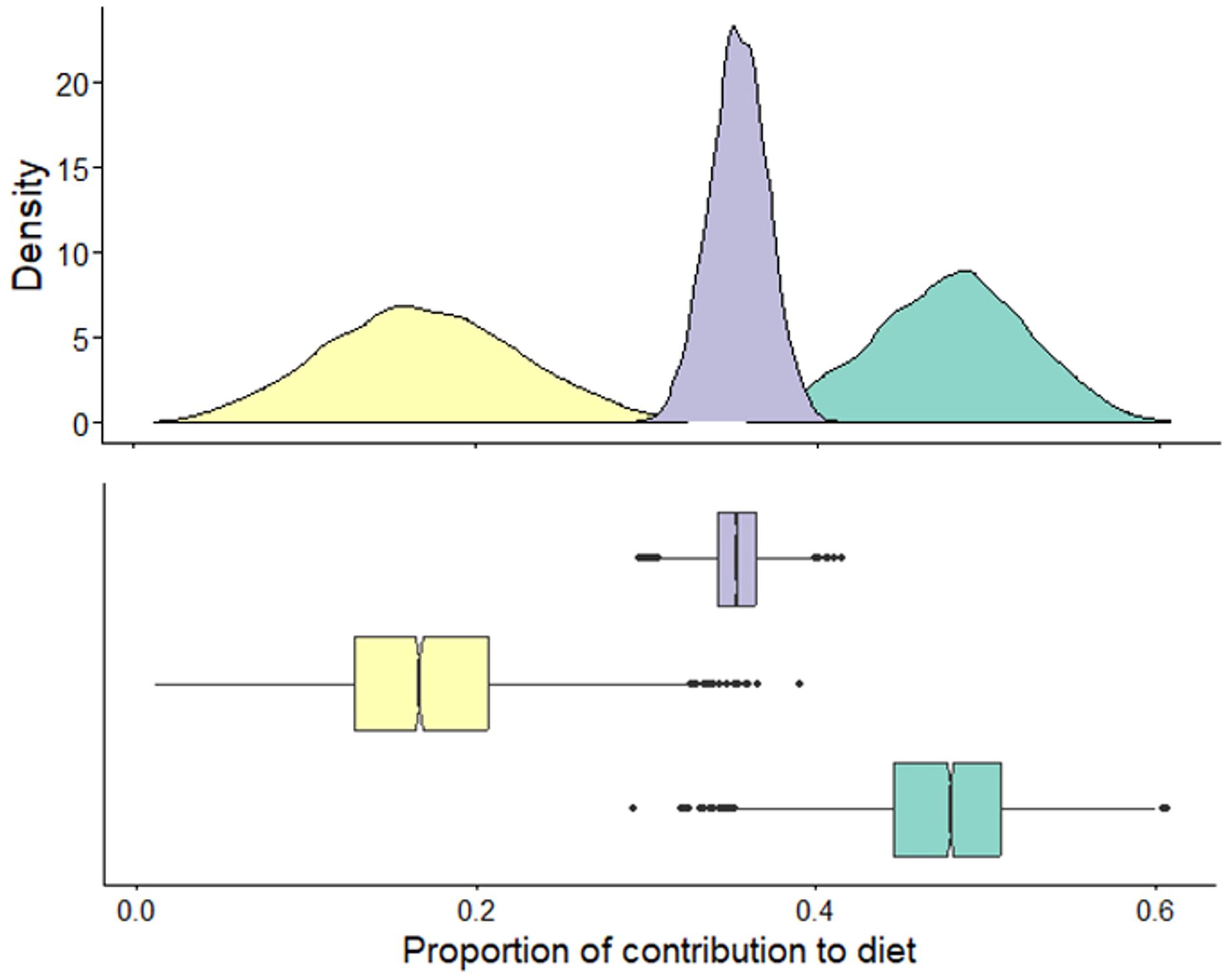
Figure 6 The proportional contribution of prey groups estimated from Bayesian mixing models for white shark muscle collected in New South Wales, Australia, between 2020-2021 ( low trophic level δ13C enriched;
mid-trophic level δ13C depleted:
high trophic level δ13C enriched).
Seasonal mixing models showed substantial variation in prey proportions. Contributions from low trophic level δ13C enriched prey) were highest in spring and summer, at 0.57 and 0.43 (± 0.04 and 0.02), respectively and decreased to 0.29 in winter (Figure 7). Mid-trophic level δ13C depleted prey (contributed minimally during spring and summer at 0.07 and 0.14 (± 0.04 and 0.09), respectively and increased to 0.40 (± 0.1) in winter (Figure 7). Proportions from high trophic level δ13C enriched prey were lowest in winter, 0.31 (± 0.03), increased during spring to 0.36 (± 0.06), and were highest in summer at 0.43 (± 0.04; Figure 7).
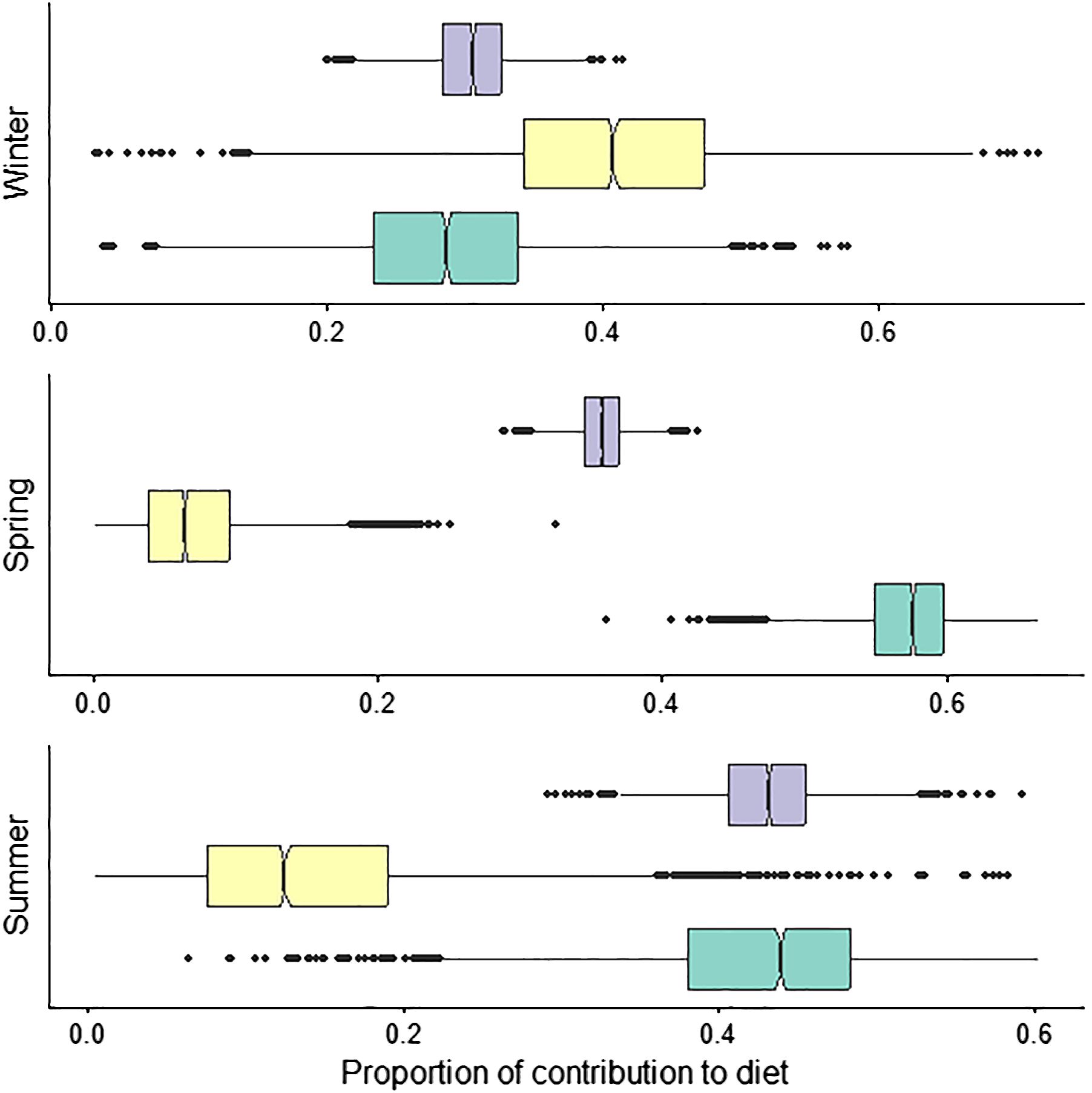
Figure 7 Seasonal Bayesian mixing models of white shark diet using muscle (autumn was excluded from these analyses due to small sample size) collected in New South Wales, Australia, between 2020-2021 ( low trophic level δ13C enriched;
mid-trophic level δ13C depleted:
high trophic level δ13C enriched). Sea mullet and Australian salmon were excluded from the spring and summer model.
4 Discussion
Short- and long-term dietary biomarkers provided a comprehensive overview of the diet and foraging habitat of immature white sharks on the east coast of Australia and revealed that they feed primarily on low-trophic prey in carbon-enriched coastal habitats. We found distinct seasonal differences in fatty acid signatures and diet composition from stable isotope mixing models. Specifically, muscle had lower proportions of essential polyunsaturated fatty acids, i.e., ARA, EPA, and DHA, during autumn and winter, suggesting that changes in prey availability linked to environmental factors, such as water temperature, influence white shark diet and foraging patterns. The increased contribution from inshore prey highlights the importance of these habitats as foraging grounds for immature white sharks, which is critical for their growth and development. Additionally, the seasonal diet shifts may influence these sharks’ nutritional condition over a longer timescale as their energetic and nutritional requirements change throughout ontogeny.
4.1 Coastal habitat use and feeding
Fatty acid and stable isotope results suggest that white sharks use coastal habitats for foraging. Muscle and plasma ARA proportions were similar to previous studies in New South Wales and South Australia (Pethybridge et al., 2014; Meyer et al., 2017; Gallagher et al., 2019) and are comparable to other coastal sharks and benthic elasmobranchs (Davidson et al., 2011; de Sousa Rangel et al., 2021c; Zhang et al., 2023). ARA derives from benthic and coastal primary producers (Sardenne et al., 2017). Therefore, animals feeding in these areas would have elevated levels of this polyunsaturated fatty acid (Caraveo-Patiño et al., 2009; Hartwich et al., 2013) compared to those feeding in offshore habitats. Over a longer-temporal scale, δ13C values support the fatty acid results of high coastal habitat use. However, the δ13C values revealed high inter-individual variation, ranging between -14.6 to -17.5‰, which encompasses habitats dominated by coastal macrophytes (-14‰) and pelagic phytoplankton (-18‰; Hobson, 1999). The variation in δ13C reported here indicate some individuals have a higher reliance on δ13C depleted prey, similar to great hammerhead sharks (Sphyrna mokarran) that forage on coastal prey in the same region (Raoult et al., 2019).
Overall proportions of ARA and DHA indicate feeding on coastal prey. These fatty acids may reflect the contribution of fish and cephalopods to the diet of white sharks (Couturier et al., 2013; Pethybridge et al., 2014), with high ARA proportions representing benthic-feeding fishes and elasmobranchs (Couturier et al., 2013). This was further supported by the stable isotope Bayesian mixing model, showing white shark diet consisted primarily of low trophic level prey from coastal habitats with a substantial contribution from species at higher trophic levels These findings are similar to previous studies reporting that immature white sharks feed on various species from benthic and pelagic ecosystems (Hussey et al., 2012b; Tamburin et al., 2020). Previous stomach content and eDNA metabarcoding analyses showed that Australian salmon (Arripis trutta) and sea mullet (Mugil cephalus) are important prey for eastern Australian immature white sharks (Grainger et al., 2020; Clark et al., 2023). Benthic and benthopelagic elasmobranchs also contribute to the diet of immature white sharks in eastern Australia (Grainger et al., 2020) and in the North-Eastern Pacific Ocean (Tamburin et al., 2020) but were rarely detected in another study using eDNA metabarcoding of cloaca swabs (Clark et al., 2023). The differences in prey composition across these studies reflect the broad range of species consumed by white sharks, likely linked to seasonal variation in prey availability or broad-scale movements exhibited by most sharks, from Queensland to Victoria Spaet et al., (2020b). Additionally, the differences in δ13C and δ15N values seen here, indicate there are substantial dietary variations among individuals which may not be reflected in the contributions from the mixing model.
4.2 Seasonal plasticity in diet
Stable isotope mixing models from muscle samples showed seasonal shifts in diet, with substantial changes from low and higher trophic level δ13C enriched prey in spring and summer to mid-trophic level δ13C depleted prey in winter. The fatty acid results further support this dietary shift. Proportions of DHA increased substantially during the spring and summer months and are similar to those found in benthic elasmobranchs (Dunstan et al., 1988; El Kebir et al., 2007) and bigeye tuna (Thunnus obesus; Peng et al., 2013). Fatty acid 18:1ω9 is a strong indicator of mesopelagic fish, such as yellowfin tuna (Thunnus albacares; Sardenne et al., 2016) and cephalopods (Phillips et al., 2001; Meyer et al., 2019), suggesting higher consumption of these prey during autumn and winter when this fatty acid increases. Seasonal variations in environmental conditions can impact the distribution and abundance of prey (Poloczanska et al., 2007; Last et al., 2011). The strengthening EAC during late spring causes persistent upwelling over shelf waters, subsequently increasing productivity (Roughan and Middleton, 2002). This coincides with white shark presence and capture in the region, which peaks during winter and spring, when water temperatures are below 21°C (Spaet et al., 2020a; Lipscombe et al., 2023). Consequently, the seasonal changes detected in our study are likely linked to the changes in prey availability associated with water temperature and season. The East Australian Current strongly influences water temperatures (Malcolm et al., 2011) and prey distribution on the east coast of Australia (Booth et al., 2007). Thus, these factors also influence shark occurrence and capture (Spaet et al., 2020a; Lipscombe et al., 2023), and habitat use by potential prey species (Gillanders et al., 2001; Zischke et al., 2012). Fatty acids are known to be influenced by sea surface temperature (Dalsgaard et al., 2003; Meyer et al., 2019); therefore, by combining these with stable isotopes, we have greater confidence in the inferences of diet. Future studies should focus on how changes in prey distribution and availability in response to the strengthening East Australian Current may impact the trophic ecology of immature white sharks.
4.3 Changes in nutritional condition among seasons
Seasonal variations in diet have the potential to impact the nutritional condition of white sharks. The higher proportions of saturated fatty acids and lower proportions of essential dietary fatty acids in autumn and winter suggest that prey consumed in these months is of lower nutritional quality or certain prey species are less abundant. Saturated fatty acids are ubiquitous in all animals and, combined with monounsaturated fatty acids, are the primary fatty acids catabolised for energy (Tocher, 2003). In contrast, polyunsaturated fatty acids are conserved for critical biological processes (Tocher, 2010). Lipid metabolism is relatively understudied in elasmobranchs, yet unlike teleost fishes, lipid storage occurs in the large liver (Zammit and Newsholme, 1979). The proportions of saturated fatty acids in muscle may suggest a metabolic adjustment to compensate for the lack of high-quality prey. Conversely, substantial increases in the proportions of the essential fatty acids, particularly DHA, in spring and summer imply a shift in diet to prey that supports cellular functions and physiological processes, such as growth, during these periods. Although the seasonal change in diet is evident, links to nutritional condition and the implications of poor nutrition in sharks are speculative and should be examined in more detail in future studies. Additionally, assessing responses and metabolic adaptations to dynamic coastal ecosystems may be invaluable for forecasting distribution changes associated with anthropogenic impacts.
4.4 Ontogenetic changes in diet
Ontogenetic diet shifts are well documented in white sharks from the Pacific (Estrada et al., 2006; Kim et al., 2012b). Due to limitations in sample availability, few (n = 8) samples were collected from sharks > 3 m; therefore, only a small increase in δ15N was detected with shark length. Furthermore, there was high variability among individuals, with a smaller shark (2.5 m) having the highest δ15N value and larger sharks exhibiting values below 15.5‰, lower than those recorded for immature sharks in Mexico and the north-east Pacific (Carlisle et al., 2012; Tamburin et al., 2020).
An animal’s energy and nutritional requirements typically increase with size (Gallagher et al., 2014). Although the relationships between fatty acid proportions and length are yet to be described in white sharks, the negative relationships seen here are vastly different from those of other shark species (de Sousa Rangel et al., 2021a; de Sousa Rangel et al., 2021c). We expected fatty acid proportions to increase with size as larger and higher quality prey were consumed. However, we observed decreasing proportions of DHA and EPA in muscle. Increases in DHA with total length were found in the plasma of tiger sharks (Galeocerdo cuvier) in the Atlantic (de Sousa Rangel et al., 2021a), where larger mature sharks exhibited higher values compared to immature sharks, which was linked to nutritional adjustments in preparation for reproduction. Similar results have been observed for mature male blacktip sharks in EPA, DHA and ARA (de Sousa Rangel et al., 2021b). Based on these results, it is plausible that our results reflect the immature life stage of white sharks, where energetic investments for reproduction are not yet required. More extensive sampling of mature white sharks across a broader spatial scale may assist in clarifying the relationships between total length and specific fatty acids when sharks are closer to maturity.
4.5 Experimental considerations
Consideration must be given to the limitations of using bulk tissue stable isotopes in trophic ecology studies. Specifically, mixing models are sensitive to trophic enrichment factors and isotopic routing, which can lead to inaccurate results (Phillips et al., Bond and Diamond, 2011). Species- and tissue-specific trophic enrichment factors are limited for large sharks, as controlled feeding experiments are impractical. Currently, no trophic enrichment factor is available for white sharks, and as a large-bodied predator occupying a high trophic level, there are several physiological (e.g., growth rate, isotope routing) and environmental (e.g., temperature) factors that may impact the discrimination of stable isotopes (Caut et al., 2009; Hussey et al., 2010; Phillips et al., 2014). Subsequently, the value provided by Hussey et al. (2010) from multiple species of sharks was deemed the best alternative. Isotopic routing remains unclear in shark tissues, yet it is well documented to cause significant issues when interpreting mixing models in teleosts, resulting in over or underestimating the contribution of sources to diet (Kelly and Martínez del Rio, 2010). We also acknowledge that while we aimed to include a range of prey items of white sharks, δ13C values indicate many of these sharks are feeding on coastal prey with values around -15‰, that were not included in the mixing model. There may also be another prey group between the low trophic and high trophic level δ13C enriched groups that these sharks are feeding on that we have been unable to sample in this study. One of the many limitations of mixing models is their inability to detect missing prey or differentiate if consumers values are the average of two separate prey groups with distinct isotopic signatures (Phillips et al., 2014; Stock et al., 2018). Additionally, the more prey included in the mixing model, the less precise the estimates of contribution (Phillips et al., 2014). Furthermore, interpretation of stable isotopes requires caution, as spatial and temporal variation of isotopes exists in marine environments and are influenced by environmental and anthropogenic factors (Pethybridge et al., 2018; Matich et al., 2021).
5 Conclusion
We examined the diet and habitat use of immature white sharks on the east coast of Australia using a combination of fatty acid and stable isotope analyses. White sharks are highly dependent on coastal resources with high contributions from low trophic level coastal prey, corroborating previous studies of white shark diet in this region. There was evidence of a seasonal shift in diet, which may be associated with changes in prey availability with varying water temperatures. These seasonal changes may influence the nutritional condition of white sharks and negatively affect physiological processes that support health and growth. This study expands on the recent dietary studies of white sharks, providing a holistic understanding of a species that is typically characterised as a generalist predator. Yet, the seasonal variations detected here reveal that their diet is more complex than previously described. Further studies investigating how ocean warming may impact prey distribution and white shark foraging would prove valuable, in addition to changes in the biochemistry and metabolism of white sharks and what implications this may have on the nutritional condition of these sharks.
Data availability statement
The raw data supporting the conclusions of this article will be made available by the authors, without undue reservation.
Ethics statement
The animal study was approved by NSW DPI and Southern Cross University Animal Care and Ethics Committee (ACEC 07/08: ARA 21/034). The study was conducted in accordance with the local legislation and institutional requirements.
Author contributions
RL: Conceptualization, Data curation, Formal analysis, Methodology, Writing – original draft, Writing – review & editing. LM: Conceptualization, Formal analysis, Writing – review & editing. PB: Conceptualization, Writing – review & editing. SM: Formal analysis, Writing – review & editing. CH: Conceptualization, Formal analysis, Writing – review & editing. AS: Conceptualization, Supervision, Writing – review & editing. PB: Conceptualization, Supervision, Writing – review & editing.
Funding
The author(s) declare financial support was received for the research, authorship, and/or publication of this article. The New South Wales Department of Primary Industries provided funding through the NSW Shark Management Program. RL is supported by an Australian Government Research and Training Program Stipend and a post-graduate scholarship from Southern Cross University. New South Wales Department of Primary Industries provided primary project funding and support. Southern Cross University provided funding to RL through a Research Training Program Scholarship.
Acknowledgments
This work was completed under NSW DPI ‘Scientific’ [Ref. P01/0059(A)], ‘Marine Parks’ (Ref. P16/0145-1.1) and ‘Animal Care and Ethics’ (ACEC 07/08: ARA 21/034) permits. This project would not have been possible without the dedicated support of contracted fishers and the NSW DPI shark research team.
Conflict of interest
The authors declare that the research was conducted in the absence of any commercial or financial relationships that could be construed as a potential conflict of interest.
The author(s) declared that they were an editorial board member of Frontiers, at the time of submission. This had no impact on the peer review process and the final decision.
Publisher’s note
All claims expressed in this article are solely those of the authors and do not necessarily represent those of their affiliated organizations, or those of the publisher, the editors and the reviewers. Any product that may be evaluated in this article, or claim that may be made by its manufacturer, is not guaranteed or endorsed by the publisher.
Supplementary material
The Supplementary Material for this article can be found online at: https://www.frontiersin.org/articles/10.3389/fmars.2024.1359785/full#supplementary-material
References
Alderete-Macal M. J., Caraveo-Patiño J., Hoyos-Padilla E. M. (2020). Ontogenetic differences in muscle fatty acid profile of white sharks Carcharodon carcharias off Guadalupe Island, México. Rev. Biol. Mar. Oceanogr 55, 37–46. doi: 10.22370/rbmo.2020.55.1.2372
Anderson M. J. (2017). “Permutational multivariate analysis of variance (PERMANOVA),” in Wiley StatsRef: Statistics Reference Online. 1-15. doi: 10.1002/9781118445112.stat07841
Ballantyne J. S. (1997). Jaws: the inside story. The metabolism of elasmobranch fishes. Comp. Biochem. Physiol. B Biochem. Mol. Biol. 118, 703–742. doi: 10.1016/S0305-0491(97)00272-1
Beckmann C. L., Mitchell J. G., Stone D. A., Huveneers C. (2013). A controlled feeding experiment investigating the effects of a dietary switch on muscle and liver fatty acid profiles in Port Jackson sharks Heterodontus portusjacksoni. J. Exp. Mar. Biol. Ecol. 448, 10–18. doi: 10.1016/j.jembe.2013.06.009
Beng K. C., Corlett R. T. (2020). Applications of environmental DNA (eDNA) in ecology and conservation: opportunities, challenges and prospects. Biol.Conserv 29, 2089–2121. doi: 10.1007/s10531-020-01980-0
Bligh E. G., Dyer W. J. (1959). A rapid method of total lipid extraction and purification. Can. J. Biochem. 37, 911–917. doi: 10.1139/o59-09
Bond A. L., Diamond A. W. (2011). Recent Bayesian stable-isotope mixing models are highly sensitive to variation in discrimination factors. Ecol. Appl. 21, 1017–1023. doi: 10.1890/09-2409.1
Booth D. J., Figueira W. F., Gregson M. A., Brown L., Beretta G. (2007). Occurrence of tropical fishes in temperate southeastern Australia: role of the East Australian Current. Estuar. Coast. Shelf Sci. 72, 102–114. doi: 10.1016/j.ecss.2006.10.003
Brodie S., Hobday A. J., Smith J. A., Spillman C. M., Hartog J. R., Everett J. D., et al. (2017). Seasonal forecasting of dolphinfish distribution in eastern Australia to aid recreational fishers and managers. Deep Sea Res. 2 Top. Stud. Oceanogr. 140, 222–229. doi: 10.1016/j.dsr2.2017.03.004
Bruce B. D., Bradford R. W. (2012). “Habitat use and spatial dynamics of juvenile white sharks, Carcharodon carcharias, in eastern Australia,” in Global perspectives on the biology and life history of the white shark. Ed. Domeier M. L. (CRC Press, Boca Raton, FL), 225–254.
Bruce B., Harasti D., Lee K., Gallen C., Bradford R. (2019). Broad-scale movements of juvenile white sharks Carcharodon carcharias in eastern Australia from acoustic and satellite telemetry. Mar. Ecol. Prog. Ser. 619, 1–15. doi: 10.3354/meps12969
Budge S. M., Iverson S. J., Koopman H. N. (2006). Studying trophic ecology in marine ecosystems using fatty acids: a primer on analysis and interpretation. Mar. mamm Sci. 22, 759–801. doi: 10.1111/j.1748-7692.2006.00079.x
Caraveo-Patiño J., Wang Y., Soto L. A., Ghebremeskel K., Lehane C., Crawford M. A. (2009). Eco-physiological repercussions of dietary arachidonic acid in cell membranes of active tissues of the Gray whale. Mar. Ecol. 30, 437–447. doi: 10.1111/j.1439-0485.2009.00289.x
Carlisle A. B., Allan E. A., Kim S. L., Meyer L., Port J., Scherrer S., et al. (2021). Integrating multiple chemical tracers to elucidate the diet and habitat of Cookiecutter Sharks. Sci. Rep. 11, 11809. doi: 10.1038/s41598-021-89903-z
Carlisle A. B., Kim S. L., Semmens B. X., Madigan D. J., Jorgensen S. J., Perle C. R., et al. (2012). Using stable isotope analysis to understand the migration and trophic ecology of north-eastern Pacific white sharks (Carcharodon carcharias). PloS One 7, e30492. doi: 10.1371/journal.pone.0030492
Carlisle A. B., Litvin S. Y., Madigan D. J., Lyons K., Bigman J. S., Ibarra M., et al. (2017). Interactive effects of urea and lipid content confound stable isotope analysis in elasmobranch fishes. Can. J. Fish Aquat Sci. 74, 419–428. doi: 10.1139/cjfas-2015-0584
Carvalho M. C. (2021). Miau, a microbalance autosampler. HardwareX 10, e00215. doi: 10.1016/j.ohx.2021.e00215
Caut S., Angulo E., Courchamp F. (2009). Variation in discrimination factors (Δ15N and Δ13C): the effect of diet isotopic values and applications for diet reconstruction. J. Appl. Ecol. 46, 443–453. doi: 10.1111/j.1365-2664.2009.01620.x
Chaguaceda F., Eklöv P., Scharnweber K. (2020). Regulation of fatty acid composition related to ontogenetic changes and niche differentiation of a common aquatic consumer. Oecologia 193, 325–336. doi: 10.1007/s00442-020-04668-y
Clark Z. S., Fish J. J., Butcher P. A., Holland O. J., Sherman C. D., Rizzari J., et al. (2023). Insights into the diet and trophic ecology of white sharks (Carcharodon carcharias) gained through DNA metabarcoding analyses of cloacal swabs. Environ. DNA. 5 (6), 1362–1377. doi: 10.1002/edn3.454
Couturier L. I., Rohner C. A., Richardson A. J., Pierce S. J., Marshall A. D., Jaine F. R., et al. (2013). Unusually high levels of n-6 polyunsaturated fatty acids in whale sharks and reef manta rays. Lipids 48, 1029–1034. doi: 10.1007/s11745-013-3829-8
Dalsgaard J., John M. S., Kattner G., Müller-Navarra D., Hagen W. (2003). Fatty acid trophic markers in the pelagic marine environment. Adv. Mar. Biol. 46, 225–340. doi: 10.1016/S0065-2881(03)46005-7
Davidson B., Sidell J., Rhodes J., Cliff G. (2011). A comparison of the heart and muscle total lipid and fatty acid profiles of nine large shark species from the east coast of South Africa. Fish Physiol. Biochem. 37, 105–112. doi: 10.1007/s10695-010-9421-8
de Sousa Rangel B., Hammerschlag N., Sulikowski J. A., Moreira R. G. (2021a). Dietary and reproductive biomarkers in a generalist apex predator reveal differences in nutritional ecology across life stages. Mar. Ecol. Prog. Ser. 664, 149–163. doi: 10.3354/meps13640
de Sousa Rangel B., Hammerschlag N., Sulikowski J. A., Moreira R. G. (2021b). Physiological markers suggest energetic and nutritional adjustments in male sharks linked to reproduction. Oecologica 196, 989–1004. doi: 10.1007/s00442-021-04999-4
de Sousa Rangel B., Moreira R. G., Niella Y. V., Sulikowski J. A., Hammerschlag N. (2021c). Metabolic and nutritional condition of juvenile tiger sharks exposed to regional differences in coastal urbanization. Sci. Total Environ. 780, 146548. doi: 10.1016/j.scitotenv.2021.146548
Dunstan G. A., Sinclair A. J., O’Dea K., Naughton J. M. (1988). The lipid content and fatty acid composition of various marine species from southern Australian coastal waters. Comp. Biochem. Physiol. B Biochem. Mol. Biol. 91, 165–169. doi: 10.1016/0305-0491(88)90130-7
El Kebir M. V.O., Barnathan G., Gaydou E. M., Siau Y., Miralles J. (2007). Fatty acids in liver, muscle and gonad of three tropical rays including non-methylene-interrupted dienoic fatty acids. Lipids 42, 525–535. doi: 10.1007/s11745-007-3040-x
Estrada J. A., Rice A. N., Natanson L. J., Skomal G. B. (2006). Use of isotopic analysis of vertebrae in reconstructing ontogenetic feeding ecology in white sharks. Ecology 87, 829–834. doi: 10.1890/0012-9658(2006)87[829:UOIAOV]2.0.CO;2
Everett J. D., Baird M. E., Roughan M., Suthers I. M., Doblin M. A. (2014). Relative impact of seasonal and oceanographic drivers on surface chlorophyll a along a Western Boundary Current. Prog. Oceanog 120, 340–351. doi: 10.1016/j.pocean.2013.10.016
Franks B. R., Tyminski J. P., Hussey N. E., Braun C. D., Newton A. L., Thorrold S. R., et al. (2021). Spatio-temporal variability in White Shark (Carcharodon carcharias) movement ecology during residency and migration phases in the Western North Atlantic. Front. Mar. Sci. 8. doi: 10.3389/fmars.2021.744202
Gallagher A. J., Meyer L., Pethybridge H. R., Huveneers C., Butcher P. A. (2019). Effects of short-term capture on the physiology of white sharks Carcharodon carcharias: amino acids and fatty acids. Endanger Species Res. 40, 297–308. doi: 10.3354/esr00997
Gallagher A. J., Shiffman D. S., Byrnes E. E., Hammerschlag-Peyer C., Hammerschlag N. (2017). Patterns of resource use and isotopic niche overlap among three species of sharks occurring within a protected subtropical estuary. Aquat Ecol. 51, 435–448. doi: 10.1007/s10452-017-9627-2
Gallagher A. J., Wagner D. N., Irschick D. J., Hammerschlag N. (2014). Body condition predicts energy stores in apex predatory sharks. Consev Physiol. 2, cou022. doi: 10.1093/conphys/cou022
Gillanders B. M., Ferrell D. J., Andrew N. L. (2001). Estimates of movement and life-history parameters of yellowtail kingfish (Seriola lalandi): how useful are data from a cooperative tagging programme? Mar. Freshw. Res. 52, 179–192. doi: 10.1071/MF99153
Govan E., Jackson A. L., Inger R., Bearhop S., Parnell A. C. (2023). simmr: A package for fitting stable isotope mixing models in R. arXiv preprint arXiv:2306.07817. doi: 10.48550/arXiv.2306.07817
Grainger R., Peddemors V. M., Raubenheimer D., Machovsky-Capuska G. E. (2020). Diet composition and nutritional niche breadth variability in juvenile white sharks (Carcharodon carcharias). Front. Mar. Sci. 7, 422. doi: 10.3389/fmars.2020.00422
Hartwich M., Martin-Creuzburg D., Wacker A. (2013). Seasonal changes in the accumulation of polyunsaturated fatty acids in zooplankton. J. Plankton Res. 35, 121–134. doi: 10.1093/plankt/fbs078
Heithaus M. R. (2007). “Nursery areas as essential shark habitats: a theoretical perspective,” in American Fisheries Society Symposium, vol. 50. (American Fisheries Society), 3.
Heithaus M. R., Dill L. M. (2002). Food availability and tiger shark predation risk influence bottlenose dolphin habitat use. Ecology 83, 480–491. doi: 10.1890/0012-9658(2002)083[0480:FAATSP]2.0.CO;2
Heithaus M. R., Frid A., Vaudo J. J., Worm B., Wirsing A. J. (2010). Unraveling the ecological importance of elasmobranchs. In Carrier C.C., Musik J. A., Heithaus M. R., (Eds.), Sharks and their relatives II (CRC Press), pp. 627–654.
Hobday A. J., Young J. W., Moeseneder C., Dambacher J. M. (2011). Defining dynamic pelagic habitats in oceanic waters off eastern Australia. Deep Sea Res. 2 Top. Stud. Oceanogr. 58, 734–745. doi: 10.1016/j.dsr2.2010.10.006
Hobson K. A. (1999). Tracing origins and migration of wildlife using stable isotopes: a review. Oecologia 120, 314–326. doi: 10.1007/s004420050865
Hughes J. (2012). The biology and population structure of eastern Australian salmon (Arripis trutta) in south-eastern Australia (Sydney, Australia: University of New South Wales).
Hussey N. E., Brush J., McCarthy I. D., Fisk A. T. (2010). δ15N and δ13C diet–tissue discrimination factors for large sharks under semi-controlled conditions. Comp. Biochem. Physiol. A Mol. Integr. Physiol. 155, 445–453. doi: 10.1016/j.cbpa.2009.09.023
Hussey N. E., Dudley S. F., Mccarthy I. D., Cliff G., Fisk A. T. (2011). Stable isotope profiles of large marine predators: viable indicators of trophic position, diet, and movement in sharks? Can. J. Fish Aquat Sci. 68, 2029–2045. doi: 10.1139/f2011-115
Hussey N. E., Macneil M. A., Olin J. A., Mcmeans B. C., Kinney M. J., Chapman D. D., et al. (2012a). Stable isotopes and elasmobranchs: tissue types, methods, applications and assumptions. J. Fish Biol. 80, 1449–1484. doi: 10.1111/j.1095-8649.2012.03251.x
Hussey N. E., MacNeil M. A., Siple M. C., Popp B. N., Dudley S. F., Fisk A. T. (2015). Expanded trophic complexity among large sharks. Food webs 4, 1–7. doi: 10.1016/j.fooweb.2015.04.002
Hussey N. E., Mccann H. M., Cliff G., Dudley S. F., Wintner S. P., Fisk A. T. (2012b). “Size-based analysis of diet and trophic position of the white shark (Carcharodon carcharias) in South African waters,” in Global perspectives on the biology and life history of the white shark. Ed. Domeier M. L. (CRC Press, Boca Raton, FL), 27–49.
Huveneers C., Apps K., Becerril-García E. E., Bruce B., Butcher P. A., Carlisle A. B., et al. (2018). Future research directions on the “elusive”white shark. Front. Mar. Sci. 5. doi: 10.3389/fmars.2018.00455
Iverson S. J. (2009). “Tracing aquatic food webs using fatty acids: from qualitative indicators to quantitative determination,” in Lipids in aquatic ecosystems (Springer New York, New York, NY), 281–308.
Iverson S. J., Field C., Don Bowen W., Blanchard W. (2004). Quantitative fatty acid signature analysis: a new method of estimating predator diets. Ecol. Monogr. 74, 211–235. doi: 10.1890/02-4105
Kelly L. J., Martínez del Rio C. (2010). The fate of carbon in growing fish: an experimental study of isotopic routing. Physiol. Biol. Zool 83, 473–480. doi: 10.1086/649628
Kim S. L., Del Rio C. M., Casper D., Koch P. L. (2012a). Isotopic incorporation rates for shark tissues from a long-term captive feeding study. J. Exp. Biol. 215, 2495–2500. doi: 10.1242/jeb.070656
Kim S. L., Koch P. L. (2012). Methods to collect, preserve, and prepare elasmobranch tissues for stable isotope analysis. Environ. Biol. Fishes 95, 53–63. doi: 10.1007/s10641-011-9860-9
Kim S. L., Tinker M. T., Estes J. A., Koch P. L. (2012b). Ontogenetic and among-individual variation in foraging strategies of northeast Pacific white sharks based on stable isotope analysis. PloS One 7, e45068. doi: 10.1371/journal.pone.0045068
Kohl K. D., Coogan S. C., Raubenheimer D. (2015). Do wild carnivores forage for prey or for nutrients? Evidence for nutrient-specific foraging in vertebrate predators. BioEssays 37, 701–709. doi: 10.1002/bies.201400171
Krausman R. P. (1999). Some basic principles of habitat use, grazing behavior of livestock and wildlife. Wildlife Range Experiment Station Bull. 70, 85–90.
Last P. R., White W. T., Gledhill D. C., Hobday A. J., Brown R., Edgar G. J., et al. (2011). Long-term shifts in abundance and distribution of a temperate fish fauna: a response to climate change and fishing practices. Glob Ecol. Biogeogr 20, 58–72. doi: 10.1111/j.1466-8238.2010.00575.x
Lee K. A., Butcher P. A., Harcourt R. G., Patterson T. A., Peddemors V. M., Roughan M., et al. (2021). Oceanographic conditions associated with white shark (Carcharodon carcharias) habitat use along eastern Australia. Mar. Ecol. Prog. Ser. 659, 143–159. doi: 10.3354/meps13572
Lester R. J. G., Rawlinson S. E., Weaver L. C. (2009). Movement of sea mullet Mugil cephalus as indicated by a parasite. Fish Res. 96, 129–132. doi: 10.1016/j.fishres.2008.10.006
Lipscombe R. S., Scott A., Morris S., Peddemors V. M., Smoothey A. F., Butcher P. A. (2023). The influence of bait position on the catch of target and non-target sharks in a SMART drumline bather protection program. Fish Res. 257, 106501. doi: 10.1016/j.fishres.2022.106501
Liu J., Zheng S., Feng M., Xie L., Feng B., Liang P., et al. (2022). Seasonal variability of eddy kinetic energy in the East Australian current region. Front. Mar. Sci. 9, 1069184. doi: 10.3389/fmars.2022.1069184
Malcolm H. A., Davies P. L., Jordan A., Smith S. D. (2011). Variation in sea temperature and the East Australian Current in the Solitary Islands region between 2001–2008. Deep Sea Res. 2 Top. Stud. Oceanogr. 58, 616–627. doi: 10.1016/j.dsr2.2010.09.030
Matich P., Shipley O. N., Weideli O. C. (2021). Quantifying spatial variation in isotopic baselines reveals size-based feeding in a model estuarine predator: implications for trophic studies in dynamic ecotones. Mar. Biol. 168, 108. doi: 10.1007/s00227-021-03920-0
Meyer L., Chambers S., Gervais C., Pethybridge H., Beckmann C., Bruce B., et al. (2021). The use of muscle lipids and fatty acids to assess shark diet and condition. J. Fish Biol. 98, 566–571. doi: 10.1111/jfb.14602
Meyer L., Pethybridge H., Nichols P. D., Beckmann C., Bruce B. D., Werry J. M., et al. (2017). Assessing the functional limitations of lipids and fatty acids for diet determination: the importance of tissue type, quantity, and quality. Front. Mar. Sci. 4. doi: 10.3389/fmars.2017.00369
Meyer L., Pethybridge H., Nichols P. D., Beckmann C., Huveneers C. (2019). Abiotic and biotic drivers of fatty acid tracers in ecology: A global analysis of chondrichthyan profiles. Funct. Ecol. 33, 1243–1255. doi: 10.1111/1365-2435.13328
Munroe S., Meyer L., Heithaus M. (2018). “Dietary biomarkers in shark foraging and movement ecology. Shark research: emerging technologies and applications for the field and laboratory,” in Shark research: emerging technologies and applications for the field and laboratory. Eds. Carrier J. C., Heithaus M. R., Simpfendorfer C. A. (CRC Press, Boca Raton, FL), 1–24.
Munroe S. E. M., Simpfendorfer C. A., Heupel M. R. (2014). Defining shark ecological specialisation: concepts, context, and examples. Rev. Fish Biol. Fishes 24, 317–331. doi: 10.1007/s11160-013-9333-7
Newsome S. D., Clementz M. T., Koch P. L. (2010). Using stable isotope biogeochemistry to study marine mammal ecology. Mar. Mamm Sci. 26, 509–572. doi: 10.1111/j.1748-7692.2009.00354.x
Peng S., Chen C., Shi Z., Wang L. (2013). Amino acid and fatty acid composition of the muscle tissue of yellowfin tuna (Thunnus albacares) and bigeye tuna (Thunnus obesus). J. Food Sci. Nutr. Res. 1, 42–45. doi: 10.12691/jfnr-1-4-2
Peterson B. J., Fry B. (1987). Stable isotopes in ecosystem studies. Annu. Rev. Ecol. Evol. Syst. 18, 293–320. doi: 10.1146/annurev.es.18.110187.001453
Pethybridge H. R., Choy C. A., Polovina J. J., Fulton E. A. (2018). Improving marine ecosystem models with biochemical tracers. Ann. Rev. Mar. Sci. 10, 199–228. doi: 10.1146/annurev-marine-121916-063256
Pethybridge H., Daley R. K., Nichols P. D. (2011). Diet of demersal sharks and chimaeras inferred by fatty acid profiles and stomach content analysis. J. Exp. Mar. Biol. Ecol. 409, 290–299. doi: 10.1016/j.jembe.2011.09.009
Pethybridge H. R., Parrish C. C., Bruce B. D., Young J. W., Nichols P. D. (2014). Lipid, fatty acid and energy density profiles of white sharks: insights into the feeding ecology and ecophysiology of a complex top predator. PloS One 9, e97877. doi: 10.1371/journal.pone.0097877
Phillips D. L., Inger R., Bearhop S., Jackson A. L., Moore J. W., Parnell A. C., et al. (2014). Best practices for use of stable isotope mixing models in food-web studies. Can. J. Zool. 92, 823–835. doi: 10.1139/cjz-2014-0127
Phillips K. L., Jackson G. D., Nichols P. D. (2001). Predation on myctophids by the squid Moroteuthis ingens around Macquarie and Heard Islands: stomach contents and fatty acid analyses. Mar. Prog. Ecol. Ser. 215, 179–189. doi: 10.3354/meps215179
Poloczanska E. S., Babcock R. C., Butler A., Hobday A. J., Hoegh-Guldberg O., Kunz T., et al. (2007). Climate change and Australian marine life. Oceanog Mar. Biol. 45, 407.
Post D. M., Layman C. A., Arrington D. A., Takimoto G., Quattrochi J., Montana C. G. (2007). Getting to the fat of the matter: models, methods and assumptions for dealing with lipids in stable isotope analyses. Oecologia 152, 179–189. doi: 10.1007/s00442-006-0630-x
Raoult V., Broadhurst M. K., Peddemors V. M., Williamson J. E., Gaston T. F. (2019). Resource use of great hammerhead sharks (Sphyrna mokarran) off eastern Australia. J. Fish Biol. 95, 1430–1440. doi: 10.1111/jfb.14160
R Core Team. (2023). _R: A Language and Environment for Statistical Computing (Vienna Austria: R Foundation for Statisical Computing). Available at: https://www.R-project.org.
Ridgway K. R., Godfrey J. S. (1997). Seasonal cycle of the East Australian current. J. Geophys. Res. Oceans 102, 22921–22936. doi: 10.1029/97JC00227
Rohner C. A., Couturier L. I., Richardson A. J., Pierce S. J., Prebble C. E., Gibbons M. J., et al. (2013). Diet of whale sharks Rhincodon typus inferred from stomach content and signature fatty acid analyses. Mar. Ecol. Prog. Ser. 493, 219–235. doi: 10.3354/meps10500
Roughan M., Middleton J. H. (2002). A comparison of observed upwelling mechanisms off the east coast of Australia. Cont Shelf Res. 22, 2551–2572. doi: 10.1016/S0278-4343(02)00101-2
Sardenne F., Bodin N., Chassot E., Amiel A., Fouché E., Degroote M., et al. (2016). Trophic niches of sympatric tropical tuna in the Western Indian Ocean inferred by stable isotopes and neutral fatty acids. Prog. Oceanogr. 146, 75–88. doi: 10.1016/j.pocean.2016.06.001
Sardenne F., Hollanda S., Lawrence S., Albert-Arrisol R., Degroote M., Bodin N. (2017). Trophic structures in tropical marine ecosystems: a comparative investigation using three different ecological tracers. Ecol. Indic. 81, 315–324. doi: 10.1016/j.ecolind.2017.06.001
Sargent J., Bell J., Bell M., Henderson R., Tocher D. (1995). Requirement criteria for essential fatty acids. J. Appl. Ichthyol. 11, 183–198. doi: 10.1111/j.1439-0426.1995.tb00018.x
Schimmelmann A., Qi H., Coplen T. B., Brand W. A., Fong J., Meier-Augenstein W., et al. (2016). Organic reference materials for hydrogen, carbon, and nitrogen stable isotope-ratio measurements: caffeines, n-alkanes, fatty acid methyl esters, glycines, L-valines, polyethylenes, and oils. Anal. Chem. 88, 4294–4302. doi: 10.1021/acs.analchem.5b04392
Skomal G. B., Braun C. D., Chisholm J. H., Thorrold S. R. (2017). Movements of the white shark Carcharodon carcharias in the North Atlantic Ocean. Mar. Ecol. Prog. Ser. 580, 1–16. doi: 10.3354/meps12306
Spaet J. L., Manica A., Brand C. P., Gallen C., Butcher P. A. (2020a). Environmental conditions are poor predictors of immature white shark (Carcharodon carcharias) occurrences on coastal beaches of eastern Australia. Mar. Ecol. Prog. Ser. 653, 167–179. doi: 10.3354/meps13488
Spaet J. L., Patterson T. A., Bradford R. W., Butcher P. A. (2020b). Spatiotemporal distribution patterns of immature Australasian white sharks (Carcharodon carcharias). Sci. Reps. 10, 1–13. doi: 10.1038/s41598-020-66876-z
Stock B. C., Jackson A. L., Ward E. J., Parnell A. C., Phillips D. L., Semmens B. X. (2018). Analyzing mixing systems using a new generation of Bayesian tracer mixing models. PeerJ 6, e5096. doi: 10.7717/peerj.5096
Tamburin E., Elorriaga-Verplancken F. R., Estupiñan-Montaño C., Madigan D. J., Sánchez-González A., Padilla M. H., et al. (2020). New insights into the trophic ecology of young white sharks (Carcharodon carcharias) in waters off the Baja California Peninsula, Mexico. Mar. Biol. 167, 1–14. doi: 10.1007/s00227-020-3660-8
Tate R. D., Cullis B. R., Smith S. D., Kelaher B. P., Brand C. P., Gallen C. R., et al. (2019). The acute physiological status of white sharks (Carcharodon carcharias) exhibits minimal variation after capture on SMART drumlines. Conserv. Physiol. 7, coz042. doi: 10.1093/conphys/coz090
Tate R. D., Kelaher B. P., Brand C. P., Cullis B. R., Gallen C. R., Smith S. D., et al. (2021). The effectiveness of Shark-Management-Alert-in-Real-Time (SMART) drumlines as a tool for catching white sharks, Carcharodon carcharias, off coastal New South Wales, Australia. Fish Manag Ecol. 28, 496–506. doi: 10.1111/fme.12489
Terraube J., Arroyo B., Madders M., Mougeot F. (2011). Diet specialisation and foraging efficiency under fluctuating vole abundance: a comparison between generalist and specialist avian predators. Oikos 120, 234–244. doi: 10.1111/j.1600-0706.2010.18554.x
Tocher D. R. (2003). Metabolism and functions of lipids and fatty acids in teleost fish. Rev. Fish Sci. 11, 107–184. doi: 10.1080/713610925
Tocher D. R. (2010). Fatty acid requirements in ontogeny of marine and freshwater fish. Aquac Res. 41, 717–732. doi: 10.1111/are.2010.41.issue-5
Towner A. V., Underhill L. G., Jewell O. J., Smale M. J. (2013). Environmental influences on the abundance and sexual composition of white sharks Carcharodon carcharias in Gansbaai, South Africa. PloS One 8, e71197. doi: 10.1371/journal.pone.0071197
Weng K. C., Boustany A. M., Pyle P., Anderson S. D., Brown A., Block B. A. (2007). Migration and habitat of white sharks (Carcharodon carcharias) in the eastern Pacific Ocean. Mar. Biol. 152, 877–894. doi: 10.1007/s00227-007-0739-4
Williams J. J., Papastamatiou Y. P., Caselle J. E., Bradley D., Jacoby D. M. (2018). Mobile marine predators: an understudied source of nutrients to coral reefs in an unfished atoll. Proc. Biol. Sci. 285, 20172456. doi: 10.1098/rspb.2017.2456
Young J. W., Lansdell M. J., Campbell R. A., Cooper S. P., Juanes F., Guest M. A. (2010). Feeding ecology and niche segregation in oceanic top predators off eastern Australia. Mar. Biol. 157, 2347–2368. doi: 10.1007/s00227-010-1500-y
Zammit V. A., Newsholme E. A. (1979). Activities of enzymes of fat and ketone-body metabolism and effects of starvation on blood concentrations of glucose and fat fuels in teleost and elasmobranch fish. Biochem. J. 184, 313–322. doi: 10.1042/bj1840313
Zhang B., Pethybridge H., Virtue P., Nichols P. D. (2023). Lipid dynamics in the southern hemisphere: a 30-year meta-analysis of marine consumers. Mar. Ecol. Prog. Ser. 710, 1–14. doi: 10.3354/meps14295
Keywords: Stable isotopes, fatty acids, trophic ecology, biomarkers, marine predator
Citation: Lipscombe RS, Meyer L, Butcherine P, Morris S, Huveneers C, Scott A and Butcher PA (2024) A taste of youth: Seasonal changes in the diet of immature white sharks in eastern Australia. Front. Mar. Sci. 11:1359785. doi: 10.3389/fmars.2024.1359785
Received: 22 December 2023; Accepted: 17 June 2024;
Published: 16 July 2024.
Edited by:
George Jackson, Loma Linda University, United StatesReviewed by:
Emigdio Marín-Enríquez, National Council of Science and Technology (CONACYT), MexicoFelipe Galván-Magaña, Centro Interdisciplinario de Ciencias Marinas (IPN), Mexico
Copyright © 2024 Lipscombe, Meyer, Butcherine, Morris, Huveneers, Scott and Butcher. This is an open-access article distributed under the terms of the Creative Commons Attribution License (CC BY). The use, distribution or reproduction in other forums is permitted, provided the original author(s) and the copyright owner(s) are credited and that the original publication in this journal is cited, in accordance with accepted academic practice. No use, distribution or reproduction is permitted which does not comply with these terms.
*Correspondence: Rebecca S. Lipscombe, ci5saXBzY29tYmU4M0Bob3RtYWlsLmNvbQ==