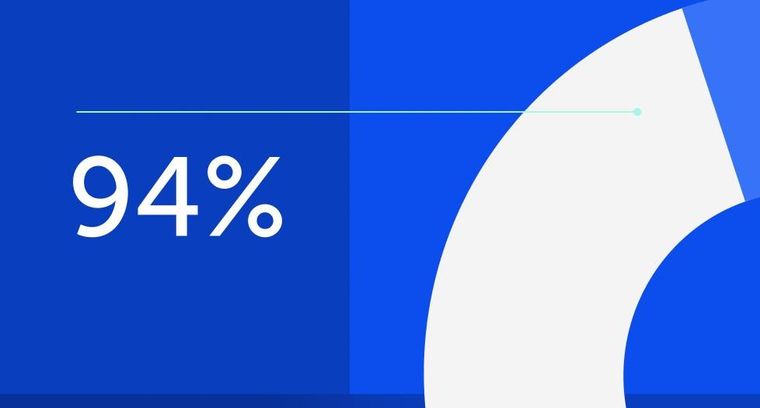
94% of researchers rate our articles as excellent or good
Learn more about the work of our research integrity team to safeguard the quality of each article we publish.
Find out more
ORIGINAL RESEARCH article
Front. Mar. Sci., 06 March 2024
Sec. Coastal Ocean Processes
Volume 11 - 2024 | https://doi.org/10.3389/fmars.2024.1359779
This article is part of the Research TopicCarbon Cycle Vulnerability Across Coastal and Forested Wetlands in Response to Anthropogenic PerturbationsView all 7 articles
Blue carbon is fast garnering international interest for its disproportionate contribution to global carbon stocks. However, our understanding of the size of these blue carbon stocks, as well as the provenance of carbon that is stored within them, is still poor. This is especially pertinent for many small-island nations that may have substantial blue carbon ecosystems that are poorly studied. Here, we present a preliminary assessment of blue carbon from three islands in the Maldives. The higher purpose of this research was to assess the feasibility of using blue carbon to help offset carbon emissions associated with Maldivian tourism, the largest Maldivian industry with one of the highest destination-based carbon footprints, globally. We used stable isotope mixing models to identify how habitats contributed to carbon found in sediments, and Loss on Ignition (LoI) to determine carbon content. We found that for the three surveyed islands, seagrasses (Thalassia hemprichii, Thalassodendron ciliatum, Halodule pinofilia, Syringodium isoetifolium, and Cymodocea rotundata) were the main contributors to sediment blue carbon (55 – 72%) while mangroves had the lowest contribution (9 – 44%). Surprisingly, screw pine (Pandanus spp.), a relative of palm trees found across many of these islands, contributed over a quarter of the carbon found in sediments. Organic carbon content (‘blue carbon’) was 6.8 ± 0.3 SE % and 393 ± 29 tonnes ha-1 for mangrove soils, and 2.5 ± 0.2% and 167 ± 20 tonnes ha-1 for seagrasses, which is slightly higher than global averages. While preliminary, our results highlight the importance of seagrasses as carbon sources in Maldivian blue carbon ecosystems, and the possible role that palms such as screw pines may have in supplementing this. Further research on Maldivian blue carbon ecosystems is needed to: 1) map current ecosystem extent and opportunities for additionality through conservation and restoration; 2) determine carbon sequestration rates; and 3) investigate options and feasibility for tourism-related blue carbon crediting. Overall, the opportunity for blue carbon in the Maldives is promising, but the state of knowledge is very limited.
The carbon footprint of the tourism industry is significant, accounting for ~8% of the world’s carbon emissions and is expected to grow by 4% annually into the future (Lenzen et al., 2018). Small-island destinations such as the Maldives, Seychelles, Cyprus, and Mauritius have especially high carbon footprints due to international, high-income visitors (Cowburn et al., 2018). In 2017, tourism accounted for roughly 30% of the Maldives’ GDP with approximately 1.4 million tourists visiting the Archipelago (Mohamed, 2016). Out of 160 countries assessed, the Maldives has the highest visitor carbon footprint in the world (Lenzen et al., 2018), and the main contributors to traveler carbon footprints are transport, shipping and food (Sun, 2014). Since these three activities cannot be eliminated, and with tourism increasing globally, there is growing need to investigate ways to decarbonize or offset emissions from island tourism (Barney et al., 2021). The most effective way to achieve this is through higher energy efficiency, incorporation of renewable energy sources, and sustainable food provisioning, with the remainder of emissions offset through nature-based initiatives (van Vuuren et al., 2018; Lewis et al., 2019).
As tourism in these remote islands is closely connected to coastal ecosystems, there is an opportunity for tourism operators and guests to reduce their emissions through the protection and restoration of blue carbon ecosystems, including seagrass meadows, macroalgae and mangrove forests (Arina et al., 2020; Macreadie et al., 2022). Ecotourism operators in the Maldives often play an active role in marine conservation initiatives but the growing interest in ecotourism is leading to increasing scrutiny from tourists over offsetting environmental impacts (Xu et al., 2023). Blue carbon ecosystems are among the world’s most efficient and long-term carbon sinks and provide a range of co-benefits, such as providing coastal protection and supporting healthy fisheries, to the surrounding local communities (Macreadie et al., 2021). Ideally, tourists and providers (e.g. resorts) could account for their carbon emissions through blue carbon projects and be connected to those projects as part of the tourism experience (Fakfare and Wattanacharoensil, 2023). For example, guest visits to nearby mangrove restoration projects could be delivered by local communities where tourists can see carbon offsets in action. In addition to better connecting tourists with their carbon footprint, there would likely be further ecological, economic, and social benefits to local communities (Su et al., 2021). These benefits are especially pertinent in small, remote island communities with little infrastructure or funding for renewable development to lower their emissions, and where these sustainability goals must also counter the pressures of developing coastal habitats for tourism (Connell, 2018).
Our overarching goal was to investigate opportunities for using blue carbon as a climate mitigation and emission reduction strategy for the Maldivian tourism sector, while delivering a range of additional ecological and social benefits for the country. As there were no published data on blue carbon in the Maldives at the time of this study, we sought to sample blue carbon at several islands to get a sense for whether levels of blue carbon are comparable to global averages, and to determine the provenance (source) of that blue carbon (Sreelekshmi et al., 2022). Determining the source is important for any blue carbon strategy as it helps understand if a given blue carbon ecosystem is generating its own organic carbon (‘autochthonous’ carbon), or whether it is reliant on carbon being produced elsewhere and being trapped by a blue carbon ecosystem (‘allochthonous’ carbon). These are necessary steps to correctly prioritize habitats for protection and carbon sequestration, and our results will aid in determining which primary producers drive carbon sequestration in Maldivian islands.
Sampling sites were chosen in Laamu Atoll (also known as Haddhunmathi) in the Southern Central Maldives, specifically the islands of L.Hithadhoo, L.Maabaidhoo and L.Gaadhoo (Figure 1). These three sites consisted of sandy areas adjacent to residential housing development and were characterized by mangroves on the high ground and seagrasses close to the shoreline. Other sites within the atoll have similar characteristics but these were chosen given previous cooperation with the local communities that facilitated sampling and ease of access. Gaadhoo was chosen specifically because its mangrove ecosystem is enclosed in the center of the island, whereas the other systems are open and intertidal. Mangrove habitats consisted of Red Rhizophora mucronata, yellow Ceriops tagal, black Lumnitzera racemosa mangroves, and ironwood Pemphis acidula. Seagrass habitats were made up of diverse species assemblages including Thalassia hemprichii, Thalassodendron ciliatum, Halodule pinofilia, Syringodium isoetifolium, and Cymodocea rotundata.
Figure 1 The sampling campaign involved taking soil cores from mangrove forests and seagrass meadows in the Maldives (red shaded area in top left) at three islands across Laamu Atoll (bottom left);, Maabaidhoo, Gaadhoo, and Hithadhoo (locations indicated by red arrows).Red stars indicate sampling locations. Imagery credit Google Earth 2023.
In 2019, 18 sediment cores were collected from both mangrove (total of 9; Figure 1) and seagrass (total of 9; Figure 1) ecosystems across all chosen sites, to quantify the soil carbon parameters for the first time. At each site, we collected a minimum of three cores from each of the two ecosystems, at a minimum of 1 m apart, parallel to the coastline. At each of the sampling points, a coring device made from PVC pipe (100 cm length, 5 cm internal diameter) was hammered into the sediment to a depth of 1 m below the sediment surface or until hard bedrock sediments were reached. Core compaction, estimated by measuring the distance between the sediment surface within the core and the outer sediment surface, was recorded in the field for all PVC cores before dislodging from the seafloor. Once dislodged, the sediments were transported to the lab and extruded in situ.
To stabilize sediments for transport and storage, the cores were filled with foam material and sealed with tape. While we strived for 1 m cores (and this was achievable at some locations – e.g. 1134 cm at Gaadhoo Island seagrass meadows), there were many instances where we could not achieve such depths. Figure 2 shows photos of extruded cores, illustrating the varying lengths of achievable cores (in this case cores of ~40-60 cm are shown) and sediment types. Therefore, we extruded and divided sediments into the following sections that were common to all cores to enable between-site comparisons: 0- 2, 10-12 and 28-30 from the surface of the core. Plant belowground biomass was not separated from sediment due to its interwoven nature. Dry samples were sent to the UHH Analytical Laboratory, University of Hawai’i at Hilo, to estimate the organic matter content via Loss on ignition (LOI) method. LOI is a measure of the mass of a sample lost when heated to high temperatures of 450°C for 4–8 hours (Heiri et al., 2001).
Figure 2 (A) The field team from Six Senses Laamu, the Maldives Underwater Initiative, and the Blue Carbon Lab with an extruded core from a seagrass meadow; (B) cores from Gaadhoo Island showing the high variability in sediment types (carbonate-rich sands at the top, organic-rich soils at the bottom); and (C) a close up of a slice from a core showing the peaty material with high amounts of organic matter.
Subsequently, we used Kauffman and Donato (2012) and Fourqurean et al. (2012b) to establish the relationship between LOI% and organic carbon (OC%) for mangroves and seagrass samples. While LOI is known to drift from a direct relationship with percent organic carbon, this drift mainly occurs when LOI values are high [> 20% (Santisteban et al., 2004)], which was not the case for any of our samples. LOI can also produce more error for organic carbon measurements where soils contain calcium carbonate, but this error is usually < 0.25% (Li et al., 2020). Since this study was exploratory in nature, the use of LOI to obtain organic carbon (OC%) was deemed appropriate. Soil carbon density and carbon stock were calculated according to (Howard et al., 2014). The 28-30 cm section was used to estimate the carbon parameters for the remainder of the sediment core (20-50 cm). Visual analysis of the core showed that upper layers were more variable than lower, while lower layers tended to be homogenous; therefore, the lowest layer of 28-30 cm was used to extrapolate estimates for the deeper layers, and data from deeper parts of the core (> 30cm) were not used.
Due to methodological issues that arose during sample processing, soil bulk densities from Laamu atoll could not be used for its intended purpose. Since this data is necessary to calculate carbon stocks, and no such data is available for the Maldives, instead we have used surrogate values from cores obtained in the same fashion from the next atoll directly south from Laamu, Huvadhu Atoll. Preliminary analysis from seagrass sediments around H.Fares-Maathodaa and H.Hoandedhdhoo islands provided organic carbon values (Helber et al., unpublished data) that were within the same range of the current study. As sediment organic carbon was the most important variable in models to successfully predict Dry Bulk Density (DBD) of soils (Katuwal et al., 2020; Alemu I et al., 2022) and raw DBD data are rarely provided in other seagrass studies [see Piñeiro-Juncal et al. (2022)], we feel confident to use the DBD values obtained from H.Fares-Maathodaa and H.Hoandedhdhoo in our study. Dry bulk density values from mangroves were not available from this atoll, so instead mean values from Sri Lankan sites (the nearest available data) were used (Perera and Amarasinghe, 2019).
Twelve seagrass sediment cores were sampled in November and December 2022 around Fares-Maathodaa and Hoandedhdhoo and sectioned into 2 cm slices until 20cm depth. From below 20 cm depth, 2 cm sediment slices were taken every 5 cm, and from 50 cm onwards every 10 cm. Sediment slices were transferred into ziplock bags and frozen at -20°C until further analysis. For the determination of Dry Bulk Density (DBD), sediment sections were oven-dried at 60°C until there were no further changes in dry weight and their final weight recorded.
Sediments often contain inorganic carbon that could lead mixing models to produce inaccurate results for δ13C values. As a result, sediments were acidified using an HCl bath until they stopped bubbling. Since this acidification can inadvertently impact δ15N values, a separate un-acidified sample would also be processed to obtain accurate δ15N values. One set of sediment samples from Gaadhoo had extremely high %C and %N indicative of low or no inorganic carbon, and so these were not acidified for either δ15N or δ13C value measurements.
Samples of sediment and possible sediment sources were processed at the UHH Analytical Laboratory at the University of Hawai’i. Stable isotopes of 15N and 13C were measured as well as %C and %N. Isotope ratios were measured relative to standards USGS 40 and 41 (atmospheric air for δ15N values and Vienna Pee Dee Belemnite for δ13C values. Elemental precision relative to the quality control samples of similar elemental composition run alongside samples (Buffalo river sediment for sediments and peach leaf tissue for plant tissues) with known stable isotope ratios was < 0.2‰ for both δ13C and δ15N values.
Provenance of sediment organic matter was determined using Bayesian stable isotope mixing models through package MixSIAR in R V 4.0.0 (Stock et al., 2018). A key assumption with these mixing models is that all sources need to differ (Phillips et al., 2014). As a result, sources that had standard deviations that overlapped were grouped a posteriori (Mangrove and Screw Pine at Gaadhoo). In all cases, the final number of sources was less than the recommended maximum of n + 1 where n is equal to the number of tracers (Parnell et al., 2010). Since the number of sources differed between locations, each island had a separate mixing model run. No trophic enrichment factors were used given there should be no fractionation of sediments, but standard deviations were set to account for elemental precision (± 0.2‰). Although there is some evidence that decomposition can impact stable isotope fractionation, these effects are unpredictable (Kelleway et al., 2022), and the effect of decomposition is generally < 3 ‰ so will not significantly change the mixing polygon. As a result, fractionation effects from decomposition were not included here. Concentration dependencies were used given the differences in %C and %N between some of the sources present (Phillips and Koch, 2002). Model runs were adjusted to either “long” or “very long” run lengths to meet Geweke and Gelman-Rubin diagnostic criteria (Stock et al., 2018).
Across all sites, loss on ignition results indicated sediments cores contained 7.75 ± 3.43% organic matter (mean ± SD), with mangrove cores containing higher concentrations (9.31 ± 3.51%) than seagrasses (6.68 ± 2.98%), and there was no significant difference between sites for mangroves (df = 2, F = 2.02, p > 0.05) or seagrasses (df = 2, F = 0.09, p > 0.05). Percent organic carbon from ashed sediments was 6.54 ± 12.90%OC, with the high standard deviation reflecting the variability across sites and sediment cores, whereby mangrove sites at Gaadhoo had very high %OC (33.56 ± 5.56). Excluding these sites at Gaadhoo with very high concentrations of organic carbon, sediments cores contained 0.73 ± 0.50%OC. Mass spectrometry indicated un-acidified sediment samples were 11.13 ± 0.65%C (mean ± SD), whereas acidified samples without inorganic carbon were 26.72 ± 6.42%C, suggesting roughly a quarter of organic matter was carbon.
Soil carbon density in the top 30 cm of the soil profile in mangrove and seagrasses ecosystems was on average 0.09 ± 0.01 g cm-3 (± SE, n= 18) and 1.22 ± 0.03 g cm-3 (± SE, n= 144, Figure 3), respectively. The average dry bulk density in the mangroves and seagrasses was 1.28 ± 0 g cm-3 and 1.32 ± 0.03 g cm-3, respectively (Figure 3). Organic carbon content was on average 2.7 times higher in the mangrove soils 6.81 ± 0.3% compared to seagrass soil at 2.52 ± 0.2% (Figure 3).
Figure 3 Distribution of three soil estimates: carbon density, dry bulk density, carbon content, in two coastal ecosystems: mangrove and seagrasses sampled across Laamu Atoll, Maldives. Soil samples were collected from the top 30 cm of sediment cores. Bulk density was incorrectly processed, so seagrass core bulk densities from sites in the next atoll Huvadhu (Faresmaathodaa and Hoandedhdhoo) were used here. No mangrove bulk density is available for this area, so bulk density values were taken from Sri Lankan mangroves (Perera and Amarasinghe, 2019).
Soil carbon density in the top 2 cm of the soil profile in mangrove and seagrasses ecosystems was on average 0.07 ± 0.01 SE (mg cm-3, n = 6) and 1.01 ± 0.07 SE (mg cm-3, n = 12, Figure 3), respectively. The 10-12 cm depth range recorded an average carbon density of 0.1 ± 0.01 SE (g cm-3, n = 6) for mangroves and 1.16 ± 0.10 SE (g cm-3, n = 12) for seagrasses. At depths of 28-30 cm and 29-31 cm, carbon densities were 0.09 ± 0.01 SE (g cm-3, n = 6) and 1.59 ± 0.10 SE (g cm-3, n = 10), respectively (Figure 2). The average dry bulk density was the highest in the bottom part of the soil profile at the 28-30 cm depth, with mangroves at 1.35 ± 0.09 SE (g cm-3) and, at 29-31 cm depth, seagrasses at 1.59 ± 0.10 SE (g cm-3). Organic carbon content was highest at 10-12 cm depth (7.55 ± 0.6%) under mangroves and at 0-2 cm depth under seagrasses (2.99 ± 0.6%).
The carbon stock recorded in the mangrove ecosystem soil was on average 393 ± 29 tonnes ha-1 (± SE) and 167 ± 20 tonnes ha-1 in the seagrass ecosystem (± SE, Figure 4).
Figure 4 Distribution of soil organic carbon stock estimates (mean ± S.D.) in two coastal ecosystems: mangrove and seagrasses sampled across Laamu Atoll, Maldives. Seagrass soil bulk densities used for these calculations were taken from nearby sites in the next atoll, while mangrove soil bulk densities were taken from Sri Lanka (Perera and Amarasinghe, 2019). Gaadhoo mangrove stock was not included due to very high carbon content.
A total of 38 plant samples and 55 sediment samples were analyzed. These were from a mix of species that were not always present across sites, including: Red Rhizophora mucronata, yellow Ceriops tagal, black Lumnitzera racemosa mangroves, and ironwood Pemphis acidula, all of which were classified as ‘mangrove’. Species of seagrass included Thalassia hemprichii, Thalassodendron ciliatum, Halodule pinofilia, Syringodium isoetifolium and Cymodocea rotundata. Sediment stable isotope values were generally bound within the polygon produced by the likely sediment sources (mangrove, screw pine and seagrass, Figure 5). Depending on the island sampled, sediment stable isotope values were either grouped closely to seagrass or mangrove/screw pine values (e.g. Gaadhoo), or more broadly distributed between the available sources (e.g. Maabaidhoo).
Mangroves consistently provided the smallest proportion of organic matter to sediments, with screw pine contributing slightly more (0.23 ± 0.12, mean ± SD; Figure 6). Across all islands, seagrasses contributed the most to sediment organic content, with the highest contribution from seagrasses ranging to 0.72 ± 0.05 (SD) in Maabaidoo and lowest mean contribution of 0.55 ± 0.10 (SD) in Gaadhoo. In some cases, some of the distributions of modelled contributions to sediments were broad for sources, ranging from 0.25 to 0.75 for mangroves in Gaadhoo, for example.
Figure 5 Isospace plots of sediments cores and possible sediment sources (Mangroves, screw pine, seagrasses) from the three islands surveyed in Laamu Atoll, Maldives. Note that since we expect no isotopic enrichment of sources, no corrections to source or consumer values were made.
Figure 6 Boxplots of modelled outputs from Bayesian stable isotope mixing models of contribution to sediment of available sources (Mangroves, screw pine, seagrasses) in Hithadhoo, Gaadhoo, and Maabaidhoo, Laamu Atoll, Maldives. In all cases, seagrasses contributed the most to the organic components of sediment cores.
Across the three Maldivian islands surveyed, seagrasses were always the dominant contributor to sediment organic material. Nearly a quarter of organic material found in sediments was carbon, but just 11% of total sediment was carbon. These results underline that these Maldivian habitats likely sequester large amounts of carbon, and that local communities could design tourism carbon offset programs around these blue carbon ecosystems if opportunities for abatement exist.
Seagrasses were the primary contributor of organic material in sediments of three islands of the Maldives, while mangroves generally contributed less organic material to sediments but contained higher amounts of carbon. This is not entirely surprising given the greater primary production of seagrasses and their role in sustaining mangrove sediments elsewhere, where mangroves function as sediment traps rather than carbon sequesters (Walton et al., 2014). The purported mechanism for this transfer is transport and capture of seagrass detritus or wrack. Despite being within a few kilometers of each other, however, the three sites had a broad range of mangrove contributions to sediment (9 – 45%). The relatively greater mangrove contribution in Gaadhoo could be because it is a more enclosed wetland with less possibility for tidal flushing than the other sites, and thus it is likely that site-specific processes will impact overall assessments of carbon sequestration. Together, these results suggest that carbon in these Maldivian habitats is sequestered by seagrasses and stored in nearby mangrove habitats, and that both should be protected to continue sequestering carbon. In areas where seagrasses are present without adjacent mangrove habitats, there may be opportunities to capture seagrass-sourced carbon if nearby mangrove sites could be rehabilitated.
Screw pines, or similar palm trees, appeared to provide a non-negligible proportion of the organic material found in sediments across the islands. In the one system where stable isotope values of screw pine were isotopically distinct from mangroves, the screw pine provided nearly a quarter of the organic material. This would suggest that in systems with screw pine and mangrove that do not have independent stable isotope values, screw pines are likely responsible for most of the contribution to the organic material, though stable isotopes may not be able to distinguish this. Screw pines are C3 plants closely related to other palm trees, so it is likely that in systems where screw pines are not found alongside mangroves, such as Maabaidhoo, that the apparent higher contribution of mangroves in that system is driven by production from nearby palms. This result is not surprising given palm trees can cover vast swathes of coastal areas and sequester large amounts of aboveground (Dey et al., 2014) and soil (Saha et al., 2010) carbon, though data on their contribution to soil carbon are scarce (Lorenz and Lal, 2014). Palms shed large fronds that lose ~70% of their mass within a year (Moradi et al., 2014; Pulunggono et al., 2019), possibly to nearby sediments. These results highlight that palms like screw pines may be important contributors to carbon sequestration and future research should scrutinize their role in other ecosystems where they occur.
Our results indicate that mangroves and seagrass environments in the Maldives have carbon sequestration potential higher than the global average. Carbon stock in mangrove soils recorded in this study (up to 393 tonnes ha -1) was higher than the global average as estimated by Atwood et al. (2017) at 283 ± 193 tonnes ha-1, while seagrasses also contained an average of 167 tonnes ha -1, which was more than the global median carbon stock determined by Fourqurean et al. (2012a) at 139.7 tonnes ha-1. The median carbon storage value for the Indo-Pacific region calculated in Fourqurean et al. (2012a) was only 23.6 ± 8.3 tonnes ha-1, therefore the average carbon stock in the seagrass ecosystem soils in the Maldives was considerably higher than the regional average. The surprisingly high blue carbon storage capacity of seagrasses in the Maldives makes them a valuable contributor for future blue carbon crediting schemes, and further argues for their protection where they are often seen as nuisance habitats and removed by resorts (ކކކޮންސަލްޓިންގުސީޑީއީ and Consulting, 2017). Future studies should aim to assess which of the identified mangrove species sequester more carbon in soils to direct management efforts to enhance carbon sequestration potential.
Sediments contained variable concentrations of organic carbon, in some cases much higher than those typically recorded for these habitats. For example, the highest concentration of organic carbon in widescale seagrass restoration projects was < 0.65% (Greiner et al., 2013), while seagrasses in a macro-tidal estuary had values < 1.8% (Ricart et al., 2015). In contrast, some of the mangrove sites surveyed in the present study had %OC concentrations greater than 30%. This variability reflects the diversity of habitats within the Maldives: any attempt to develop blue carbon offset programs will have to target specific sites with greater sequestration potential (e.g. depositional environments with stable seagrass meadows and mangroves forests). It also highlights that some smaller, more productive areas such as the mangroves in Gaadhoo can have disproportionate sequestration potential.
One of the key assumptions in Bayesian stable isotope mixing models is that all sources have been included in the mixing model (Phillips et al., 2014). This typically results in a mixing polygon that encompasses all the consumers within the model (Smith et al., 2013). While these requirements appeared to be met for Gaadhoo and Hithadhoo, in Maabaidhoo the stable isotope values of the sediments appeared high relative to the closest available source (Mangroves). This placed them at the outer limits of what is feasible given the mixing polygon. While not able to be confirmed, it is possible that this high δ15N value is driven by sewage or wastewater inputs that are known to have this effect on stable isotope values (Gaston et al., 2004; Page et al., 2023). The very high values could also indicate that, while we attempted to sample every likely source in the system, a sediment source with stable isotope values like those of screw pines in the other islands was not included in this model. This means that the Maabaidhoo model likely overestimates the contribution of mangroves to sediments.
We recorded differences in measurements of organic carbon content between mass spectrometry used for stable isotope analysis and more traditional ignition techniques. Mass spectrometry recorded higher percentages of organic carbon (25%) than ashed sediments (6.5%). Loss on ignition approaches are widely accepted as the industry standard, however, the mineral composition of sediments can result in measurement errors (Santisteban et al., 2004), and refining ignition temperatures can reduce error (Wang et al., 2011), but was not possible in this instance. Conversely, acid-treated samples can overestimate carbon content due to unreacted carbonate carbon (Kennedy et al., 2005). The higher variability of organic carbon measurements from mass spectrometry in our results suggest some unreacted carbonate carbon remained in some of the samples, meaning loss-on-ignition measurements were likely more accurate. Thus, while mass spectrometry is a more precise method, it may be more prone to measurement error from contamination. Future studies should investigate how to reduce this error so that carbon sediment assessments can benefit from the greater precision of mass spectrometry and interpret our results in the context of these uncertainties.
In conclusion, our study sheds light on the blue carbon dynamics of coastal vegetated ecosystems of the Maldives. Our findings indicate that the carbon sequestration potential of Maldivian mangroves and seagrasses surpasses global averages. Seagrasses emerged as consistent champions in contributing organic material to sediments across the surveyed islands, highlighting their pivotal role in carbon sequestration. Mangroves, while generally providing less organic material, exhibited higher carbon concentrations, underlining their significance in storing carbon in these ecosystems. The variable contribution of mangroves to sediment, influenced by local conditions, emphasizes the need for site-specific conservation strategies. Surprisingly, screw pines, previously not reported in blue carbon assessments, were found to be non-negligible contributors to sediment organic material. The distinct stable isotope values of screw pines in some instances suggested their substantial role, raising questions about the potential carbon sequestration capacity of palm trees in coastal areas. Future research should explore the contribution of palms, like screw pines, in other ecosystems to comprehensively understand their impact on carbon sequestration. This research is timely given the lack of previous information on Maldivian blue carbon, and the growing interest from the tourism sector in ‘blue carbon offsets’ for travelers. However, we wish to stress that due to limited resources, this study was only able sample a small fraction of the number of islands in the Maldives. We recommend further investment in a national sampling campaign to reduce data uncertainty, in combination with a spatial assessment of blue carbon opportunities via conservation and restoration. Conservation and restoration of Maldivian blue carbon ecosystems will have benefits beyond carbon, including: coastal protection, shoreline stabilization, coastal resilience (helping islands keep pace with sea level rise through accretion), supporting fish and fisheries, maintaining biodiversity, pollution removal (plastics, nutrients, pathogens), and maintaining water quality.
The original contributions presented in the study are included in the article/Supplementary Material. Further inquiries can be directed to the corresponding author.
PM: Conceptualization, Funding acquisition, Methodology, Project administration, Resources, Supervision, Writing – original draft, Writing – review & editing. MW: Conceptualization, Data curation, Investigation, Methodology, Project administration, Supervision, Writing – original draft, Writing – review & editing. PR: Data curation, Formal analysis, Methodology, Resources, Supervision, Writing – original draft, Writing – review & editing. JH: Funding acquisition, Methodology, Resources, Supervision, Writing – original draft, Writing – review & editing. SH: Conceptualization, Data curation, Formal analysis, Methodology, Resources, Writing – original draft, Writing – review & editing. PW: Data curation, Formal analysis, Methodology, Visualization, Writing – original draft, Writing – review & editing. VR: Formal analysis, Methodology, Visualization, Writing – original draft, Writing – review & editing.
The author(s) declare that no financial support was received for the research, authorship, and/or publication of this article.
Many thanks to all the volunteers that assisted with fieldwork. In particular, we want to acknowledge those from Six Senses Laamu: Kaia Mohammad - Fieldwork & sample processing, Humaam Nihad - Fieldwork & sample processing, and Ali Shareef – Fieldwork. The island councils of Maabaidhoo and Hithadhoo, the ex-residents of Gaadhoo, and Laamu Atoll Council for granting us access. Laamu Atoll Council who supported the research permit application for our study. Generation of DBD data was funded by a Leverhulme Trust Research Project Grant (RPG-2021-417) to HK East.
The authors declare that the research was conducted in the absence of any commercial or financial relationships that could be construed as a potential conflict of interest.
All claims expressed in this article are solely those of the authors and do not necessarily represent those of their affiliated organizations, or those of the publisher, the editors and the reviewers. Any product that may be evaluated in this article, or claim that may be made by its manufacturer, is not guaranteed or endorsed by the publisher.
The Supplementary Material for this article can be found online at: https://www.frontiersin.org/articles/10.3389/fmars.2024.1359779/full#supplementary-material
ކޮންސަލްޓިންގުސީޑީއީ, C.D.E. Consulting (2017). Environmental impact assessment for the proposed seagrass removal at Maafaru Island, Noonu Atoll.
Alemu I J. B., Yaakub S. M., Yando E. S., Lau R. Y. S., Lim C. C., Puah J. Y., et al. (2022). Geomorphic gradients in shallow seagrass carbon stocks. Estuar. Coast. Shelf Sci. 265, 107681. doi: 10.1016/j.ecss.2021.107681
Arina N., Raynusha C., Hidayah N., Zainee N. F. A., Prathep A., Rozaimi M. (2020). Coralline macroalgae contribution to ecological services of carbon storage in a disturbed seagrass meadow. Mar. Environ. Res. 162, 105156. doi: 10.1016/j.marenvres.2020.105156
Atwood T. B., Connolly R. M., Almahasheer H., Carnell P. E., Duarte C. M., Ewers Lewis C. J., et al. (2017). Global patterns in mangrove soil carbon stocks and losses. Nat. Clim. Change 7, 523–528. doi: 10.1038/nclimate3326
Barney A., Polatidis H., Jelić M., Tomašević N., Pillai G., Haralambopoulos D. (2021). Transition towards decarbonisation for islands: Development of an integrated energy planning platform and application. Sustain. Energy Technol. Assess. 47, 101501. doi: 10.1016/j.seta.2021.101501
Connell J. (2018). Islands: balancing development and sustainability? Environ. Conserv. 45, 111–124. doi: 10.1017/S0376892918000036
Cowburn B., Moritz C., Birrell C., Grimsditch G., Abdulla A. (2018). Can luxury and environmental sustainability co-exist? Assessing the environmental impact of resort tourism on coral reefs in the Maldives. Ocean Coast. Manage. 158, 120–127. doi: 10.1016/j.ocecoaman.2018.03.025
Dey A., Islam M., Masum K. M. (2014). Above ground carbon stock through palm tree in the Homegarden of Sylhet City in Bangladesh. J. For. Environ. Sci. 30, 293–300. doi: 10.7747/JFS.2014.30.3.293
Fakfare P., Wattanacharoensil W. (2023). Low-carbon tourism for island destinations: A crucial alternative for sustainable development. Sustain. Dev. 31, 180–197. doi: 10.1002/sd.2382
Fourqurean J. W., Duarte C. M., Kennedy H., Marbà N., Holmer M., Mateo M. A., et al. (2012a). Seagrass ecosystems as a globally significant carbon stock. Nat. Geosci. 5, 505–509. doi: 10.1038/ngeo1477
Fourqurean J. W., Kendrick G. A., Collins L. S., Chambers R. M., Vanderklift M. A., Fourqurean J. W., et al. (2012b). Carbon, nitrogen and phosphorus storage in subtropical seagrass meadows: examples from Florida Bay and Shark Bay. Mar. Freshw. Res. 63, 967–983. doi: 10.1071/MF12101
Gaston T. F., Kostoglidis A., Suthers I. M. (2004). The 13C, 15N and 34S signatures of a rocky reef planktivorous fish indicate different coastal discharges of sewage. Mar. Freshw. Res. 55, 689–699. doi: 10.1071/MF03142
Greiner J. T., McGlathery K. J., Gunnell J., McKee B. A. (2013). Seagrass restoration enhances “Blue Carbon” sequestration in coastal waters. PloS One 8, e72469. doi: 10.1371/journal.pone.0072469
Heiri O., Lotter A. F., Lemcke G. (2001). Loss on ignition as a method for estimating organic and carbonate content in sediments: reproducibility and comparability of results. J. Paleolimnol. 25, 101–110. doi: 10.1023/A:1008119611481
Howard J., Hoyt S., Isensee K., Telszewski M., Pidgeon E. (2014). Coastal blue carbon: methods for assessing carbon stocks and emissions factors in mangroves, tidal salt marshes, and seagrasses.
Katuwal S., Knadel M., Norgaard T., Moldrup P., Greve M. H., de Jonge L. W. (2020). Predicting the dry bulk density of soils across Denmark: Comparison of single-parameter, multi-parameter, and vis–NIR based models. Geoderma 361, 114080. doi: 10.1016/j.geoderma.2019.114080
Kauffman J. B., Donato D. (2012). Protocols for the measurement, monitoring and reporting of structure, biomass and carbon stocks in mangrove forests (Bogor, Indonesia: Working Paper 86. CIFOR). doi: 10.17528/cifor/003749
Kelleway J. J., Trevathan-Tackett S. M., Baldock J., Critchley L. P. (2022). Plant litter composition and stable isotope signatures vary during decomposition in blue carbon ecosystems. Biogeochemistry 158, 147–165. doi: 10.1007/s10533-022-00890-3
Kennedy P., Kennedy H., Papadimitriou S. (2005). The effect of acidification on the determination of organic carbon, total nitrogen and their stable isotopic composition in algae and marine sediment. Rapid Commun. Mass Spectrom. 19, 1063–1068. doi: 10.1002/rcm.1889
Lenzen M., Sun Y.-Y., Faturay F., Ting Y.-P., Geschke A., Malik A. (2018). The carbon footprint of global tourism. Nat. Clim. Change 8, 522–528. doi: 10.1038/s41558-018-0141-x
Lewis S. L., Wheeler C. E., Mitchard E. T. A., Koch A. (2019). Restoring natural forests is the best way to remove atmospheric carbon. Nature 568, 25–28. doi: 10.1038/d41586-019-01026-8
Li N., Sack D., Sun J., Liu S., Liu B., Wang J., et al. (2020). Quantifying the carbon content of aeolian sediments: Which method should we use? CATENA 185, 104276. doi: 10.1016/j.catena.2019.104276
Lorenz K., Lal R. (2014). Soil organic carbon sequestration in agroforestry systems. A review Agron. Sustain. Dev. 34, 443–454. doi: 10.1007/s13593-014-0212-y
Macreadie P. I., Costa M. D. P., Atwood T. B., Friess D. A., Kelleway J. J., Kennedy H., et al. (2021). Blue carbon as a natural climate solution. Nat. Rev. Earth Environ. 2, 826–839. doi: 10.1038/s43017-021-00224-1
Macreadie P. I., Robertson A. I., Spinks B., Adams M. P., Atchison J. M., Bell-James J., et al. (2022). Operationalizing marketable blue carbon. One Earth 5, 485–492. doi: 10.1016/j.oneear.2022.04.005
Mohamed M. (2016). State of the Environment (Maafanno, Male, Maldives: Ministry of Environment and Energy).
Moradi A., Teh C. B. S., Goh K. J., Husni M. H. A., Ishak C. F. (2014). Decomposition and nutrient release temporal pattern of oil palm residues. Ann. Appl. Biol. 164, 208–219. doi: 10.1111/aab.12094
Page C. E., Ainsworth T. D., Leggat W., Egan S., Gupta A. S., Raoult V., et al. (2023). Localising terrestrially derived pollution inputs to threatened near-shore coral reefs through stable isotope, water quality and oceanographic analysis. Mar. pollut. Bull. 193, 115193. doi: 10.1016/j.marpolbul.2023.115193
Parnell A. C., Inger R., Bearhop S., Jackson A. L. (2010). Source partitioning using stable isotopes: coping with too much variation. PloS One 5, e9672. doi: 10.1371/journal.pone.0009672
Perera K. A. R. S., Amarasinghe M. D. (2019). Carbon sequestration capacity of mangrove soils in micro tidal estuaries and lagoons: A case study from Sri Lanka. Geoderma 347, 80–89. doi: 10.1016/j.geoderma.2019.03.041
Phillips D. L., Inger R., Bearhop S., Jackson A. L., Moore J. W., Parnell A. C., et al. (2014). Best practices for use of stable isotope mixing models in food-web studies. Can. J. Zool. 92, 823–835. doi: 10.1139/cjz-2014-0127
Phillips D. L., Koch P. L. (2002). Incorporating concentration dependence in stable isotope mixing models. Oecologia 130, 114–125. doi: 10.1007/s004420100786
Piñeiro-Juncal N., Serrano O., Mateo M. Á., Diaz-Almela E., Leiva-Dueñas C., Martinez-Cortizas A. (2022). Review of the physical and chemical properties of seagrass soils. Geoderma 428, 116219. doi: 10.1016/j.geoderma.2022.116219
Pulunggono H. B., Anwar S., Mulyanto B., Sabiham S. (2019). Decomposition of oil palm frond and leaflet residues. AGRIVITA J. Agric. Sci. 41, 524–536. doi: 10.17503/agrivita.v41i3.2062
Ricart A. M., York P. H., Rasheed M. A., Pérez M., Romero J., Bryant C. V., et al. (2015). Variability of sedimentary organic carbon in patchy seagrass landscapes. Mar. pollut. Bull. 100, 476–482. doi: 10.1016/j.marpolbul.2015.09.032
Saha S. K., Ramachandran Nair P. K., Nair V. D., Mohan Kumar B. (2010). Carbon storage in relation to soil size-fractions under tropical tree-based land-use systems. Plant Soil 328, 433–446. doi: 10.1007/s11104-009-0123-x
Santisteban J. I., Mediavilla R., López-Pamo E., Dabrio C. J., Zapata M. B. R., García M. J. G., et al. (2004). Loss on ignition: a qualitative or quantitative method for organic matter and carbonate mineral content in sediments? J. Paleolimnol. 32, 287–299. doi: 10.1023/B:JOPL.0000042999.30131.5b
Smith J. A., Mazumder D., Suthers I. M., Taylor M. D. (2013). To fit or not to fit: evaluating stable isotope mixing models using simulated mixing polygons. Methods Ecol. Evol. 4, 612–618. doi: 10.1111/2041-210X.12048
Sreelekshmi S., Harikrishnan M., Nandan S. B., Kaimal V. S., Hershey N. R. (2022). Ecosystem carbon stock and stable isotopic signatures of soil organic carbon sources across the mangrove ecosystems of Kerala, Southern India. Wetlands 42, 29. doi: 10.1007/s13157-022-01540-y
Stock B. C., Jackson A. L., Ward E. J., Parnell A. C., Phillips D. L., Semmens B. X. (2018). Analyzing mixing systems using a new generation of Bayesian tracer mixing models. PeerJ 6, e5096. doi: 10.7717/peerj.5096
Su J., Friess D. A., Gasparatos A. (2021). A meta-analysis of the ecological and economic outcomes of mangrove restoration. Nat. Commun. 12, 5050. doi: 10.1038/s41467-021-25349-1
Sun Y.-Y. (2014). A framework to account for the tourism carbon footprint at island destinations. Tour. Manage. 45, 16–27. doi: 10.1016/j.tourman.2014.03.015
van Vuuren D. P., Stehfest E., Gernaat D. E. H. J., van den Berg M., Bijl D. L., de Boer H. S., et al. (2018). Alternative pathways to the 1.5°C target reduce the need for negative emission technologies. Nat. Clim. Change 8, 391–397. doi: 10.1038/s41558-018-0119-8
Walton M. E. M., Al-Maslamani I., Skov M. W., Al-Shaikh I., Al-Ansari I. S., Kennedy H. A., et al. (2014). Outwelling from arid mangrove systems is sustained by inwelling of seagrass productivity. Mar. Ecol. Prog. Ser. 507, 125–137. doi: 10.3354/meps10827
Wang Q., Li Y., Wang Y. (2011). Optimizing the weight loss-on-ignition methodology to quantify organic and carbonate carbon of sediments from diverse sources. Environ. Monit. Assess. 174, 241–257. doi: 10.1007/s10661-010-1454-z
Keywords: blue carbon, stable isotopes, Maldives, sequestration, seagrass, mangrove, nature-based solutions, organic carbon
Citation: Macreadie PI, Wartman M, Roe P, Hodge JM, Helber SB, Waryszak P and Raoult V (2024) Seagrasses produce most of the soil blue carbon in three Maldivian islands. Front. Mar. Sci. 11:1359779. doi: 10.3389/fmars.2024.1359779
Received: 21 December 2023; Accepted: 26 February 2024;
Published: 06 March 2024.
Edited by:
Derrick Y. F. Lai, The Chinese University of Hong Kong, ChinaReviewed by:
Amrit Kumar Mishra, James Cook University, AustraliaCopyright © 2024 Macreadie, Wartman, Roe, Hodge, Helber, Waryszak and Raoult. This is an open-access article distributed under the terms of the Creative Commons Attribution License (CC BY). The use, distribution or reproduction in other forums is permitted, provided the original author(s) and the copyright owner(s) are credited and that the original publication in this journal is cited, in accordance with accepted academic practice. No use, distribution or reproduction is permitted which does not comply with these terms.
*Correspondence: Vincent Raoult, dmluY2VudC5yYW91bHRAZGVha2luLmVkdS5hdQ==
Disclaimer: All claims expressed in this article are solely those of the authors and do not necessarily represent those of their affiliated organizations, or those of the publisher, the editors and the reviewers. Any product that may be evaluated in this article or claim that may be made by its manufacturer is not guaranteed or endorsed by the publisher.
Research integrity at Frontiers
Learn more about the work of our research integrity team to safeguard the quality of each article we publish.