- Institute of Oceanology Polish Academy of Sciences, Sopot, Poland
This pilot study investigated the bioavailability and remineralization kinetics of the sediment-derived dissolved organic carbon (DOC) from the Gdańsk Deep, a depositional area in the Baltic Sea. This was assessed in the long-lasting (126 d) incubation experiment, in which the mixture of DOC from sediment pore water and bottom water was exposed to oxic microbial respiration with incubation of bottom water as a control run. The obtained decay curves allowed us to distinguish three DOC fractions: labile (DOCL), semi-labile (DOCSL), and refractory (DOCR). In bottom water, the refractory fraction was predominant and amounted to almost 85% of total DOC, whereas about 15% of DOC was bioavailable: 6% labile and 9% semi-labile. In contrast, DOC from pore water was much more bioavailable DOC (~55% of total DOC) and contained 11% DOCL and 44% DOCSL. The remineralization rate constants recalculated to the in situ temperature of 6°C for labile and semi-labile DOC in pore water were 0.025 d−1 and 0.002 d−1, respectively, whereas, in bottom water, 0.026 d−1 and 0.004 d−1. The half-life times for DOCL were comparable for both bottom water and pore water and amounted to 26.2 d and 27.6 d, respectively. For DOCSL, the half-life time was shorter for bottom water (165.5 d) than for pore water (322.9 d).
1 Introduction
Dissolved organic matter (DOM) is an important constituent of seawater, especially in the marginal seas, where its concentrations are usually higher than those observed in the open ocean (Hedges, 2002; Carlson and Hansell, 2015). This is mainly due to the often high productivity of coastal waters and significant input of terrigenous organic material. These features make DOM an unneglectable component for many biogeochemical studies, from small-scale process-oriented research to those aiming at a holistic understanding of coastal ecosystem functioning (Ridgwell and Arndt, 2015).
In general, DOM is a heterogeneous mixture of organic compounds having a variety of properties, such as contributing to the seawater color, fueling the microbial loop, contributing to the transport of macronutrients and heavy metals, or influencing the pH of seawater. Yet, the chemical characterization and quantification of individual DOM species still remain a challenge (Repeta, 2015). Thus, DOM fluxes are often assessed by quantifying dissolved organic carbon (DOC), a major constituent of DOM. This bulk approximation through DOC also allows us to link quantitatively DOM with the marine carbon cycle and incorporate it into the biogeochemical ecosystem models (e.g., Neumann et al., 2022). Typically, DOC in models is an important link between particulate organic carbon and CO2 pools. However, the heterogeneity of compounds that make up DOC makes the parametrization of remineralization processes a challenging task, as the DOC originating from different sources may differ significantly in terms of its reactivity or bioavailability for microorganisms.
Generally, DOC can be divided into labile and recalcitrant pools with a few more subgroups distinguishable in each of these fractions, differing in their stabilities over time (Hansell, 2013). Autochthonous marine DOC is commonly reported to be more bioavailable than the terrigenous fraction. The latter, in addition to its generally relatively low reactivity, undergoes continuous remineralization on its way, which contributes to the overall recalcitrance increase of the remaining fraction entering the sea (Raymond et al., 2015). Although the bioavailability of DOC is widely studied in surface waters and along the land-ocean continuum, little is known about the reactivity of DOC released from marine sediments. This is the case even though there is growing evidence that sediments in coastal organic matter (OM)–rich regions are an important source of DOC [e.g., Alperin et al. (1999), Loginova et al. (2020), and Lengier et al. (2021)]. In the literature, there is a broad spectrum of opinions about the bioavailability of sediment-derived DOC, which simultaneously indicates that regional differences may occur. Some reports [e.g., Burdige and Komada (2015) and Chen et al. (2016)] suggest that sediments are an important source of bio-refractory DOC to the water column, while others indicate that DOC released from sediments drives microbial respiration [e.g., Burdige et al. (2016) and Komada et al. (2016)].
Taking into account this ambiguity, we conducted the first pilot study in the Gdańsk Deep, one of the depositional areas of the Baltic Sea, intending to assess the bioavailability of DOC released from sediments through incubation experiments. The Baltic Sea waters have three to four times higher DOC concentrations than commonly observed in the open ocean [e.g., Kuliński et al. (2011)]. It was shown that the Baltic DOC is a mixture of both marine (eutrophication-related) and terrestrial DOC, the latter spreading widely also in the central basins and deep water layers [e.g., Alling et al. (2008)]. However, the available reports indicate that also the Baltic Sea sediments rich in OM are an important source of DOC to the water column (Reader et al., 2019; Lengier et al., 2021). Yet, the fate of sediment-derived DOC is still highly understudied here. This was the motivation for this study for which two pivotal goals were set: (i) to estimate the share of bioavailable and recalcitrant sediment-derived DOC fractions (Figure 1) and (ii) to determine the remineralization rate constants and half-life times of the identified bioavailable DOC fractions by investigating the DOC remineralization kinetics. This was done through the incubation experiment in which we tried to mimic how quickly and to what extent sedimentary DOC can be remineralized after it is released (diffusive fluxes) to overlying bottom waters. We point out that the results will contribute to the scarce global dataset about the reactivity of sediment-derived DOC. Simultaneously, this initial research being a reference point for future investigations in the region can provide an important and presently missing parametrization for biogeochemical ecosystem models aiming at deciphering the carbon cycle in the Baltic.
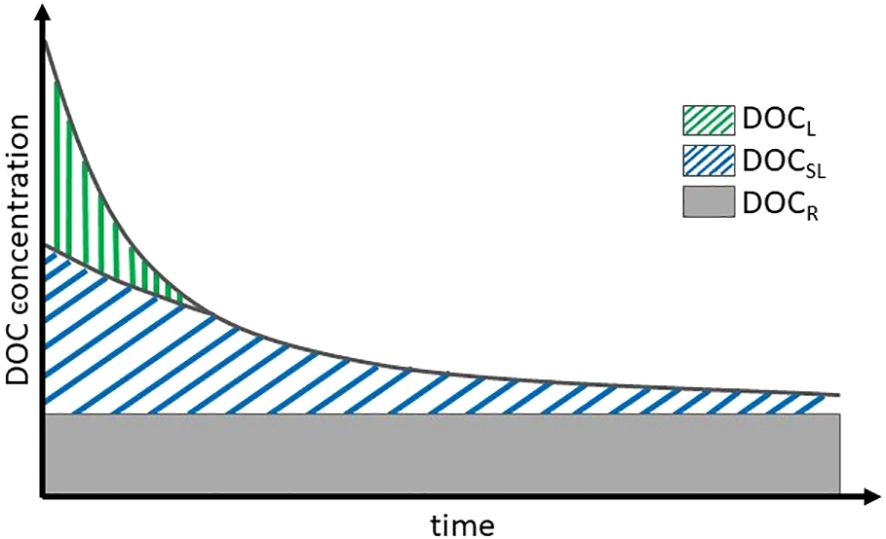
Figure 1 Conceptual scheme of DOC decay over time, including labile (DOCL), semi-labile (DOCSL), and refractory (DOCR) fractions of DOC.
2 Methods
2.1 Study area
The Gdańsk Deep is located in the southern Baltic Proper and has a maximum depth of 118 m. Permanent halocline located between 60 and 80 m depth causes a limited vertical mixing of water masses, and, consequently, temporal oxygen depletion in the near-bottom waters and anoxic sediments (Hansson and Viktorsson, 2023). This depositional basin is characterized by muddy sediments enriched in OM (organic carbon concentration range from 6% to 8%; Kuliński and Pempkowiak, 2011; Winogradow and Pempkowiak, 2014) and poorly developed or even absent macrofaunal benthic communities. Muddy sediments tend to experience strictly diffusive conditions (Huettel et al., 1998). The Gdańsk Deep region has been suggested as a significant source of DOM to the water column (Reader et al., 2019). Winogradow and Pempkowiak (2014) estimated that the diffusive flux of DOC out of sediments ranges between 0.8g C m−2 yr−1 and 1.4g C m−2 yr−1. Additionally, the studied region is under the high influence of the Vistula River (Voss et al., 2006), which is a significant source of terrigenous OM and nutrients that fuel the primary production and OM sedimentation in the region (Łysiak-Pastuszak et al., 2004; Voss et al., 2006; Reader et al., 2019).
2.2 Sampling
Seawater and sediment cores were collected at station P1 (54˚44.730′N and 19˚08.531′E) during the r/v Oceania cruise in March 2021 (Supplementary Figure 1). The bottom water was sampled using a pre-cleaned 10-L Niskin bottle about 2 m above the sediments. To identify the in situ properties of investigated water, samples for DOC, temperature, salinity, and oxygen concentration were collected. Surface sediments (0 to 5 cm) were collected using Gemax gravity corer, a total of eight cores were taken and mixed to obtain enough material for pore water extraction. First, a subsample was collected to determine the water content (drying at 60°C, 24 h), organic carbon concentrations (OC), and the stable isotopic composition of OC (δ13OC). Immediately after sampling, the rest of the sediment was centrifuged (3,000 rpm, 10 min) to extract pore water. Obtained pore water and bottom water were filtered through pre-combusted glass fibre filters (pore size, 0.4 µm; MN GF5) and stored in the dark at 4°C until the beginning of the incubation experiment that started already during the cruise after 12 h after extracting the sediment cores. All laboratory glassware and glass fibre filters used during the experiment were previously pre-combusted (450°C, 6 h) to reduce the possibility of sample contamination.
2.3 Incubation setup
The first step of the incubation setup included the preparation of the bottom and pore water mixture in a volume ratio of 4:1. This was done to reflect the return flux of DOC from sediments to the overlying waters and to observe DOC changes over time during the incubation experiment. Parallel to the bottom-pore water mixture, untreated bottom water was prepared as a control run. The effects observed within the control samples were subtracted proportionally in further calculations from the results obtained for the mixture to obtain the decay curve for the sediment-derived DOC only. To ensure oxic conditions in the mixture and the control run throughout the experiment, both solutions were bubbled before the experiment with ambient air to reach ~100% O2 air saturation. Such prepared solutions were poured into pre-cleaned 40 -ml glass vials and stored in the dark open to the atmosphere (protected from contamination only with the hydrophobic polytetrafluorethylene (PTFE) filter with a pore size of 0.22 µm). The dissolved oxygen concentration has been monitored daily using an Oxygen Dipping Probe with a PreSens Fibox 4 oxygen meter. Additionally, to maintain oxic conditions throughout the experiment, samples were continuously mixed using an orbital laboratory shaker (type WL-972, JW Electronic). At the beginning (t = 0) and after 1 d, 2 d, 6 d, 18 d, 35 d, 73 d, and 126 d of incubation, individual samples (three replicates for time steps t = 0, t = 18, and t = 126) were again filtered, acidified with 50 µl of concentrated HCl to remove carbonates and stop biological processes, and stored in dark until DOC concentration analysis. As DOC sampling and determination are prone to contamination, blank samples (MiliQ water treated the same as samples) were additionally collected at various stages of preparation (before and after centrifugation) and incubation experiment (time steps t = 0, t = 18, and t = 126). Measurements did not indicate contamination of the samples at any stage of the incubation experiment (the absolute blank value sample ranged between 5.7 µmol L− 1 and 9.2 µmol L−1 and the percentage to relative pore water values from 0.4 to 0.7; Supplementary Table 1). The incubation was carried out at 20°C ± 0.1°C for 126 d. Although this temperature does not occur in the bottom waters of the Baltic Sea, it is frequently observed in the surface waters during summer (Meier et al., 2022). Furthermore, conducting the incubation experiments at room temperature is a commonly used approach, also for the Arctic regions (Vonk et al., 2015), as it allows for obtaining quicker DOC changes over time (the remineralization constant rate is temperature-dependent; Bendtsen et al., 2015; Lønborg et al., 2018) and thus for better quantifying the DOC remineralization dynamics.
2.4 Analytical procedures
Temperature, salinity, and oxygen saturation were measured using a multimeter (Hach-Lange, HQ40D). The precision of the measurements was 0.01°C, 0.01 unit, and ±1–2%, respectively.
The analyses of OC and δ13C were performed in an Elemental Analyzer Flash EA 1112 Series combined with an Isotopic Ratio Mass Spectrometer (Thermo Electron Corp., Germany). The OC concentration was calibrated against certified reference materials (marine sediments; Fluβsediment) provided by HEKAtech GmbH. δ13C was calculated using the laboratory working pure reference gases (CO2) calibrated with IAEA standards (CO-8 and USGS40).
DOC was analyzed using the automated total organic analyzer TOC-L (Shimadzu) equipped with an NDIR CO2 detector. The samples were oxidized with a high-temperature (680°C) method and a platinum catalyst. Quality control consisted of the regular analysis of blanks, as well as accuracy and precision checks based on comparisons with the reference material, which was North Atlantic water obtained from the Hansell Laboratory (recovery, 98.9%; precision characterized by relative standard deviation, 0.9%).
2.5 Calculations
To quantify DOC remineralization in the incubation experiment, we used the first-order reaction kinetics (Arndt et al., 2013; LaBrie et al., 2020), which has been successfully applied to surface waters in the Baltic Sea in the past [e.g., Kuliński et al. (2016)]. In short (detailed description in Supplementary Material), it assumes that changes in DOC concentration over time depend on the initial DOC concentration (DOCt=0) and remineralization rate constant (k). In this study, we used continuous full oxic conditions not to influence the DOC decay through the microorganisms’ community structure changes over time. To reflect differences in the DOC reactivity, we distinguished three major DOC fractions: labile (DOCL), semi-labile (DOCSL), and refractory (DOCR) following the approach that the decay of the labile fractions of DOC causes an increase in the relative contribution of more refractory ones (LaBrie et al., 2020; Figure 1). Hence, the bulk DOC change over time was described by Equation 1:
where . d are remineralization rate constants for DOCL and DOCSL, respectively.
Based on existing literature (Benner, 2002; Kuliński et al., 2016), it is expected that, after about 20 d, there will be no more DOCL in the samples. Thus, the integrated form of Equation 1 in the later stage of incubation (>20 d) can be given by Equation 2:
DOCR has been found as the value guaranteeing the highest linearity of Equation 2, whereas the kSL was determined from the slope of Equation 2. Then, resolved Equation 2 allowed finding the DOCL(t=0) and kL from Equation 1. Additionally, the half-life times (t1/2) for each of the bioavailable DOC fractions (DOCL and DOCSL) were established (Equation 3):
To estimate the influence of higher temperature during the incubation experiment, we implemented the Q10 factor (Equation 4). It describes the increase of the rate constant of any biochemical reaction at an increase of the temperature by 10°C. We used the Q10 factor of 2.2 previously reported for seawater (Lønborg and Álvarez-Saldago, 2012) because there are no data for pore waters available.
where k1 and k2 are the rates at which products of a reaction are produced (mmol s−1) at two different corresponding temperatures: T1 and T2, in which the reaction occurred.
3 Results
The physicochemical parameters of the bottom water were within the range previously reported for the Gdańsk Deep (Szymczycha et al., 2017) and amounted to a temperature of 6.1°C, salinity of 10.2, oxygen concentration of 2.1 mg L−1 (18.1% air saturation (a.s.)). The organic carbon content in surface sediments was 5.74% ± 0.06%, whereas its isotopic composition expressed as δ13C was −26.5‰ ± 0.1‰, indicating a relatively high OM content (a characteristic feature of depositional areas; Winogradow and Pempkowiak, 2014), as well as a mixed contribution of marine and terrigenous OM (medium-high δ13C values).
For both sets of incubated samples (incubation mixture of pore and bottom waters and control run of bottom water), a continuous decrease in DOC concentration was observed (Figure 2A). In both cases, the highest DOC decrease occurred in the first 18 d, after which the decay curve flattened with a significantly smaller DOC decrease by the end of the incubation. Relative standard deviations of DOC concentration for repeated time steps (t = 0, t = 18, and t = 126) did not exceed 5%. To determine the DOC decay in the pore water itself, the results obtained for the incubation mixture have been corrected by subtracting the DOC results from the control run (bottom water) at a given time (Figure 2B). The initial DOC concentrations (DOCt=0) were 304 µmol L−1 and 1408 µmol L−1, for bottom and pore water, respectively. During the whole incubation period, the DOC loss was about 14% in the bottom water (from 304 µmol L−1 to 261 µmol L−1; Figure 2C) and about 40% in the pore water (from 1,408 µmol L−1 to 850 µmol L−1; Figure 2D). The exponential character of the decay curves justified the use of first-order kinetics to quantify the remineralization dynamics of DOC. All the results of the calculations (linear equations and determination coefficients, remineralization rate constants, and half-life times for DOCL and DOCSL fractions) are presented in Table 1 and in Supplementary Figure 2.
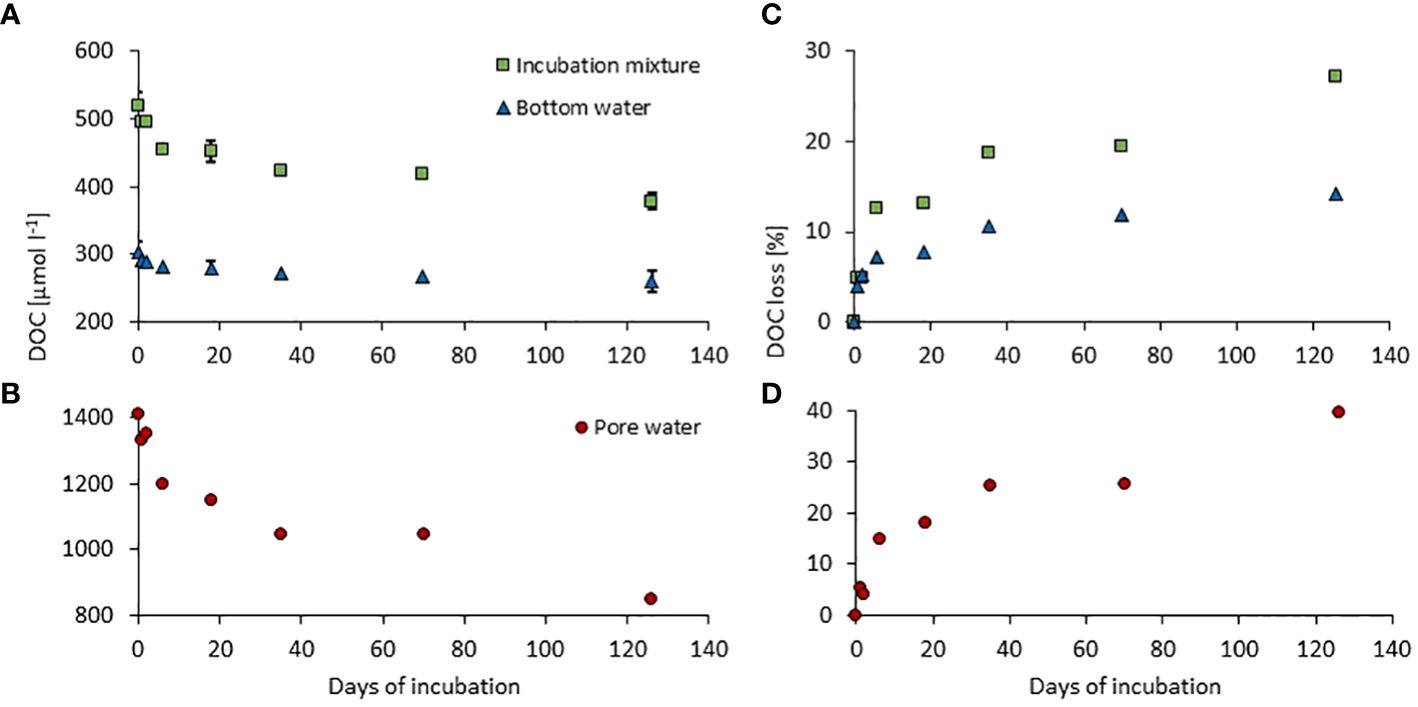
Figure 2 DOC decay during incubation of the bottom and pore waters mixture (green; A, bottom water (blue; A) and pore water (red; B), and cumulative DOC loss presented as a percentage of initial concentrations (C, D).
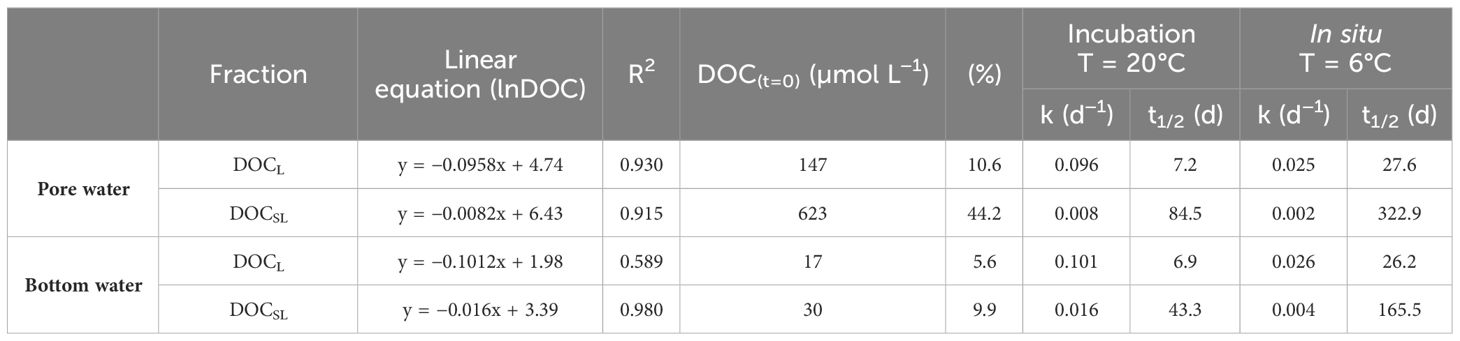
Table 1 Qualitative and quantitative characteristics of labile (DOCL), semi-labile (DOCSL), and refractory (DOCR) DOC for incubation temperature (20°C) and in situ temperature (6°C).
4 Discussion
4.1 Bioavailability of DOC released from sediments
In the bottom water, the refractory DOC fraction was predominant and amounted to 257 µmol L−1, corresponding to almost 85% of total DOC. Only 15% of total DOC in bottom water was bioavailable: 6% of DOCL and 9% of DOCSL (Figure 3). Quantitatively, the refractory DOC was also predominant in the pore water and amounted to 638 µmol L−1; however, it constituted a much smaller share of total DOC, namely about 45%. Consequently, there was approximately five times more bioavailable DOC in the pore water than in the bottom water: DOCL and DOCSL in pore water amounted to 147 µmol L−1 and 623 µmol L−1, respectively, and combined as bioavailable fractions they added up to about 55% of total DOC. The difference in the share of bioavailable DOC may be explained by different compositions and provenience of OM in sediments and bottom water. Refractory compounds of DOC, such as humic and fulvic acids and structural carbohydrates, are transported from the land, reach the bottom waters, and then accumulate in marine sediments (Asmala et al., 2014; Winogradow and Pempkowiak, 2018). Fresh and labile OM of planktonic origin also reach the sediments but then are quickly remineralized (Miltner et al., 2005; Asmala et al., 2013, 2014). It was previously stated that surface-most layers of sediments within the depositional areas of the Baltic Sea contain a large proportion of autochtonous sedimentary organic carbon; however, this contribution decreases substantially in deeper layers of sediments (Winogradow and Pempkowiak, 2018). These findings are consistent with our observations, which suggest that a lot of DOC in pore waters mostly originates from fresh OM and, therefore, contains a significant fraction of labile and semi-labile DOC. Additionally, the diffusion of DOC from deeper sediment layers to the surface should be taken into account, which may lead to an increase in DOC concentration in the surface. However, this is a fraction deposited to sediments some time ago and exposed for a longer time to remineralization processes, and, thus, it probably contributes to the refractory DOC budget. In the case of bottom water, the high contribution of refractory DOC may be due to their long residence time. Bottom water in the Baltic Sea is formed through the mixing of the saline and dense water inflowing from the North Sea with the brackish Baltic Sea surface water (Elken and Matthäus, 2008) both containing some DOC accumulated from production in surface waters (Kuliński et al., 2011; Kuliński et al., 2022). After sinking to the deep layers, it is further transported deep into the basin, and the DOC that it contains can be readily remineralized leaving the majority of refractory DOC, resistant to biochemical oxidation. On the other hand, a continuous efflux of DOC from sediments, which we have identified as highly bioavailable, contributes to the composition and reactivity of the bottom water DOC pool. This is in line with the findings of Komada et al. (2013), who reported that a significant DOC fraction in anaerobic surface-most sediments of the Santa Monica Basin (California Borderland) is 14C-young and labile, but escaping the sediments is very quickly remineralized in the water column. However, to precisely track the fate of DOC released from sediments in the Baltic Sea, future studies should include linking OM bioavailability with its chemical composition and qualitative properties, such as humic-like fluorescence, aromaticity, and molecular weight as smaller, less humic-like compounds are favorable to degradation than large, humic-like constituents (Asmala et al., 2014).
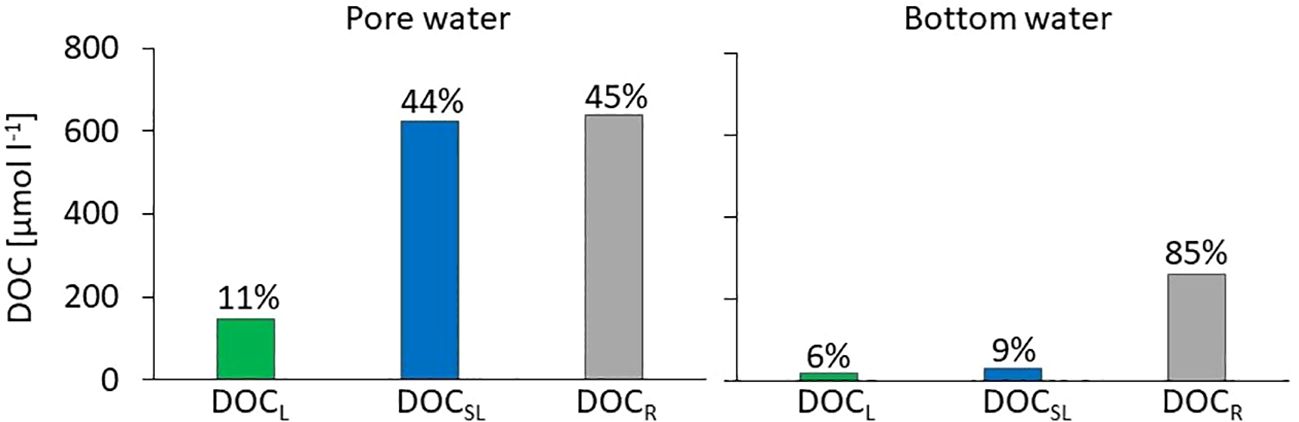
Figure 3 Concentrations and relative contributions (value above the bars) of labile (DOCL), semi-labile (DOCSL), and refractory (DOCR) DOC fractions in pore and bottom water.
Interestingly, such a high bioavailability as we have identified for sediment-derived DOC (55%) has not been previously observed in the Baltic Sea area. Our results are much higher than the values reported for DOC in surface waters, which ranged from 0% to 12% in open waters of the Gulf of Finland and the Bothnian Sea (Hoikkala et al., 2015; Rowe et al., 2018) to 30% in the southern Baltic Sea and 34% in the Mecklenburg Bight (Kuliński et al., 2016; Rowe et al., 2018). The latter regions are under high anthropogenic pressure and a high inflow of DOM and nutrients carried by river discharge from Oder and Vistula Rivers, in which bioavailable DOC amounted to 16% and 19%, respectively (Kuliński et al., 2016).
4.2 Remineralization rate constants and half-life times of DOC
The incubation experiments were conducted at a room temperature (20°C) to have visible DOC decay in time, as with increasing temperature, the rate of metabolic processes increases, and, thus, also the remineralization rate constant is higher and half-life time is smaller (Table 1). To recalculate the above-mentioned parameters to in situ temperature (6°C), the Q10 factor was used, which represents the increase of the rate constant of any biochemical reaction at an increase of the temperature by 10°C (see details in Section 2.5). The highest remineralization rate constant was found for the labile DOC fraction in the pore water (in situ temperature) and amounted to 0.025 d−1 (t1/2 = 27.6 d); these values were very close to those obtained for DOCL fraction in bottom water (k=0.026 d−1 and t1/2 = 26.2 d). The relative contribution of the most bioavailable DOC fraction is the smallest, both in the pore and above-bottom waters (~11% and 6%, respectively), but it undergoes the fastest remineralization. Similar results of kL and t1/2 for bottom and pore waters may indicate sediments as the source of labile DOC to bottom waters, but, to confirm this, it would be necessary to qualitatively characterize DOC. A promising tool for determining the reactivity of DOC is the molecular size of organic compounds and the total size distribution of molecules of DOM (Reader et al., 2019; Loginova et al., 2020), as high molecular weight DOM (HMW; mainly carbohydrates, but and also amino acids from phytoplankton exudation and cell lysis) will be more bioreactive and readily assimilated by microbial communities than low molecular weight DOM (LMW; Benner and Amon, 2015). Moreover, study published by Burdige and Gardner (1998) also stated that the vast majority of the DOC accumulated with depth in the sediment pore waters in Chesapeake Bay was LMW-DOC, which confirms that most of the HMW-DOC is preferentially remineralized in the surface sediment layers. In the case of semi-labile DOC, the results differ significantly between pore water and bottom water. In the first case, its concentration and relative contribution (623 µmol L−1 and 44%, respectively) is much higher, but it undergoes two times slower remineralization rate (k = 0.002 d−1 and t1/2 = 323 d) compared to bottom water (k = 0.004 d−1 and t1/2 = 165 d). Significantly slower remineralization of DOC in pore waters is probably caused by the age of OM. In sediments, DOC may be pre-aged, as it may originate from OM deposited several decades ago. In deeper sediments, DOC production usually exceeds consumption, causing higher DOC concentrations and, consequently, through diffusion, the transport of older DOC to the surface layer of sediments (Burdige and Komada, 2015; Burdige et al., 2016).
4.3 Importance of bioavailable DOC released from sediments
Nilsson et al. (2019) indicated that approximately 96% of POC that is deposited in the sediments returns to the water column in the form of dissolved fractions, either as a product of remineralization (dissolved inorganic carbon) or decomposition/hydrolysis (DOC). The return flux of DOC can be measured using different approaches, and, usually, benthic incubations show higher DOC release (Niemisto and Lund-Hansen, 2019; Nilsson et al., 2019; Silberberger et al., 2022) compared to diffusion models (Brodecka-Goluch and Łukawska-Matuszewska, 2018; Lengier et al., 2021). In the Baltic Sea, the DOC return flux varies between regions and amounts to 0.44 mmol m−2 d−1 in the Baltic Proper (Lengier et al., 2021), 10 mmol m−2 d−1 in the Puck Bay (Silberberger et al., 2022), and 61 mmol m−2 d−1 in the Gulf of Finland (Niemisto and Lund-Hansen, 2019). Taking into account the DOC return flux for the Baltic Proper (0.44mmol m−2 d−1; Lengier et al., 2021) as being the most representative of the Gdańsk Deep region, and the DOC bioavailability determined in our study (55%), DOC of about 0.24 mmol m−2 d−1 released from sediments is incorporated into the microbial loop leading to the release of CO2. As the labile DOC fraction is highly reactive (short half-life time), the effects of its remineralization may likely be observed next to the source in sediments. However, the experiment showed that a significant fraction of bioavailable DOC is semi-labile with a half-life time measured in months, which suggests that its release from sediments in one spot and further remineralization may also affect remote locations. To fully unravel these features, however, it is necessary to expand the experimental work on the reactivity of sedimentary DOC to other Baltic Sea regions and also to hypoxic and anoxic conditions where other bacteria communities and other biogeochemical processes play a role. Although our study focused only on the oxic conditions, still, the obtained results may give an important approximation of the sedimentary DOC reactivity in general. The research performed in other aquatic environments (Bastviken et al., 2004) suggests that, while in a short period of time, the reactivity of DOC is comparable in aerobic and anaerobic conditions, greater bioavailability of DOC may be observed in aerobic conditions when experiments are conducted for a longer period of time, allowing for remineralization of the semi-labile fraction. Moreover, as the DOC released from sediments enters, the complex soup of organic compounds dissolved in the water column, in which terrestrial fraction constitutes a significant fraction; also, in the central basins (Alling et al., 2008), it would be interesting to verify how this addition of relatively highly reactive (or bioavailable) sedimentary DOC may change the bioavailability of more recalcitrant DOC fraction already present in the Baltic waters. We hope this pilot study on the bioavailability of sedimentary-derived DOC, together with all the literature in the field published so far, will become a trigger for conducting full-scale and basin-wide research that would combine the characterization of chemical composition, bioavailability/reactivity, and provenience (including sediments) of DOC and finally incorporate these processes in 3D ecosystem models to complete the carbon budget for the Baltic Sea. Furthermore, the obtained results, although focusing on DOC only, approximate the bioavailability of DOM in general (DOC is a measure of DOM), which implies a need to include in future research other environmentally essential components of which DOM is a carrier, such as nitrogen and phosphorus as well as heavy metals.
5 Conclusions
Our pilot research indicated that up to 55% of DOC released from the Gdańsk Deep sediments is bioavailable under oxic conditions. One-fifth of this reactive DOC is labile and undergoes remineralization during days/weeks (half-life time of about 27 d at 6°C). However, most of the bioavailable DOC has been classified as a semi-labile fraction being remineralized during weeks/months (half-life time of about 323 d at 6°C). This gives us an important hint for conceptualizing future experiments including their time frame. The present study can be considered as a reference point for further extensive research in other areas of the Baltic but also under different oxygen conditions and including other DOM components. This will help to scale up via biogeochemical models the fate of sedimentary DOM in the Baltic Sea and to better understand the cycling of carbon, but also nitrogen and phosphorus.
Data availability statement
Results can be made available after contacting the corresponding author.
Author contributions
ML: Conceptualization, Investigation, Methodology, Visualization, Writing – original draft. KM: Visualization, Writing – review & editing. BS: Conceptualization, Writing – review & editing. KK: Conceptualization, Writing – review & editing.
Funding
The author(s) declare that financial support was received for the research, authorship, and/or publication of this article. This study was conducted within the framework of the project number 2019/33/N/ST10/00161 funded by the National Science Centre and statutory activities of IOPAN.
Conflict of interest
The authors declare that the research was conducted in the absence of any commercial or financial relationships that could be construed as a potential conflict of interest.
Publisher’s note
All claims expressed in this article are solely those of the authors and do not necessarily represent those of their affiliated organizations, or those of the publisher, the editors and the reviewers. Any product that may be evaluated in this article, or claim that may be made by its manufacturer, is not guaranteed or endorsed by the publisher.
Supplementary material
The Supplementary Material for this article can be found online at: https://www.frontiersin.org/articles/10.3389/fmars.2024.1359563/full#supplementary-material
References
Alling V., Humborg C., Mörth C.-M., Rahm L., Pollehne F. (2008). Tracing terrestrial organic matter by d34S and d13C signatures in a subarctic estuary. Limnol. Oceanogr. 53, 2594–2602. doi: 10.4319/lo.2008.53.6.2594
Alperin M. J., Martens C. S., Albert D. B., Suayah I. B., Benninger L. K., Blair N. E., et al. (1999). Benthic fluxes and porewater concentration profiles of dissolved organic carbon in sediments from the North Carolina continental slope. Geochim Cosmochim Acta 63 (3/4), 427–448. doi: 10.1016/S0016-7037(99)00032-0
Arndt S., Jørgensen B. B., LaRowe D. E., Middelburg J. J., Pancost R. D., Regnier P. (2013). Quantifying the degradation of organic matter in marine sediments: A review and synthesis. Earth Science-review 123, 53–86. doi: 10.1016/j.earscirev.2013.02.008
Asmala E., Autio R., Kaartokallio H. (2014). Processing of humic-rich riverine dissolved organic matter by estuarine bacteria: effects of predegradation and organic nutrients. Aquat. Sci. 76, 451e463. doi: 10.1007/s00027-014-0346-7
Asmala E., Autio R., Kaartokallio H., Pitkänen L., Stedmon C. A., Thomas D. N. (2013). Bioavailability of riverine dissolved organic matter in three Baltic Sea estuaries and the effect of catchment land use. Biogeosciences 10, 6969–6986. doi: 10.5194/bg-10-6969-2013
Bastviken D., Persson L., Odham G., Tranvik L. (2004). Degradation of dissolved organic matter in oxic and anoxic lake water. Limnol. Oceanogr. 49, 109–116. doi: 10.4319/lo.2004.49.1.0109
Bendtsen J., Hilligsøe K. M., Hansen J. L. S., Richardson K. (2015). Analysis of remineralisation, lability, temperature sensitivity and structural composition of organic matter from the upper ocean. Prog. Oceanography 130, 125–145. doi: 10.1016/j.pocean.2014.10.009
Benner R. (2002). “Chapter 3 — chemical composition and reactivity,” in Biogeochemistry of Marine Dissolved Organic Matter. Eds. Dennis A. H., Craig A. C. (Academic Press, San Diego), 59–90.
Benner R., Amon R. M. W. (2015). The size-reactivity continuum of major bioelements in the Ocean. Ann. Rev. Mar. Sci. 7, 185–205. doi: 10.1146/annurev-marine-010213-135126
Brodecka-Goluch A., Łukawska-Matuszewska K. (2018). Pore water dissolved organic and inorganic carbon in relation to methane occurrence in sediments of the Gdansk basin (southern Baltic Sea). Cont. Shelf Res. 167, 11–20. doi: 10.1016/j.csr.2018.08.008
Burdige D. J., Gardner K. G. (1998). Molecular weight distribution of dissolved organic carbon in marine sediment pore waters. Mar. Chem. 62, 45–64. doi: 10.1016/S0304-4203(98)00035-8
Burdige D. J., Komada T. (2015). “Sediment pore waters,” in Biogeochemistry of Marine Dissolved Organic Matter (Second Edition). Eds. Hansell D. A., Carlson C. A. (Academic Press, Boston), 535–577. second edition edn. doi: 10.1016/C2012-0-02714-7
Burdige D. J., Komada T., Magen C., Chanton J. P. (2016). Modeling studies of dissolved organic matter cycling in Santa Barbara Basin (CA, USA) sediments. Geochim Cosmochim Acta 195, 100–119. doi: 10.1016/j.gca.2016.09.007
Carlson C. A., Hansell D. A. (2015). “DOM sources, sinks, reactivity, and budgets,” in Biogeochemistry of Marine Dissolved Organic Matter (Second Edition). Eds. Hansell D. A., Carlson C. A. (Academic Press, Boston), 509–533. doi: 10.1016/B978-0-12-405940-5.00003-0
Chen M., Kim J. H., Nam S., Niessen F., Hong W. L., Kang M. H., et al. (2016). Production of fluorescent dissolved organic matter in Arctic Ocean sediments. Sci. Rep. 6, 39213. doi: 10.1038/srep39213
Elken J., Matthäus W. (2008). “Baltic sea oceanography,” in Assessment of Climate Change for the Baltic Sea Basin (The BACC Author Team, Springer-Verlag, Berlin), 379–386, ISBN: ISBN 978-3-540-72786-6.
Hansell D. A. (2013). Recalcitrant dissolved organic carbon fractions. Annu. Rev. Mar. Sci. 5, 1. doi: 10.1146/annurev-marine-120710-100757
Hansson M., Viktorsson L. (2023). Oxygen Survey in the Baltic Sea 2022 -Extent of Anoxia and Hypoxia 1960-2022. Report Oceanography No. 74 (Göteborg, Sweden: Swedish Meteorological and Hydrological Institute - SMHI Oceanographic Unit), 92.
Hedges J. I. (2002). “Why dissolved organic matter?,” in Biogeochemistry of Marine Dissolved Organic Matter. Eds. Hansell D. A., Carlson C. A. (Academic Press, San Diego, California).
Hoikkala L., Kortelainen P., Soinne H., Kuosa H. (2015). Dissolved organic matter in the Baltic Sea. J. Mar. Syst. 142, 47–61. doi: 10.1016/j.jmarsys.2014.10.005
Huettel M. A., Ziebis A. S., Forster A. S., Luther III B.G.W. (1998). Advective transport affecting metal and nutrient distributions and interfacial fluxes in permeable sediments. Geochimica Cosmochimica Acta 62, 613–631. doi: 10.1016/S0016-7037(97)00371-2
Komada T., Burdige D. J., Crispo S. M., Druffel E. R. M., Griffin S., Johnson L., et al. (2013). Dissolved organic carbon dynamics in anaerobic sediments of the Santa Monica Basin. Geochimica Cosmochimica Acta 110, 253–273. doi: 10.1016/j.gca.2013.02.017
Komada T., Burdige D. J., Li H. L., Magen C., Chanton J. P., Cada A. K. (2016). Organic matter cycling across the sulfate-methane transition zone of the Santa Barbara Basin, California Borderland. Geochim Cosmochim Acta 176, 259–278. doi: 10.1016/j.gca.2015.12.022
Kuliński K., She J., Pempkowiak J. (2011). Short and medium term dynamics of the carbon exchange between the Baltic Sea and the North Sea. Continental Shelf Res. 31, 1611–1619. doi: 10.1016/j.csr.2011.07.001
Kuliński K., Hammer K., Schneider B., Schulz-Bull D. (2016). Remineralization of terrestrial dissolved organic carbon in the Baltic Sea. Mar. Chem. 181, 10–17. doi: 10.1016/j.marchem.2016.03.002
Kuliński K., Pempkowiak J. (2011). The carbon budget of the Baltic Sea. Biogeosciences 8, 3219–3230. doi: 10.5194/bg-8-3219-2011
Kuliński K., Rehder G., Asmala E., Bartosova A., Carstensen J., Gustafsson B., et al. (2022). Biogeochemical functioning of the baltic sea. Earth Syst. Dynam. 13, 633–685. doi: 10.5194/esd-13-633-2022
LaBrie R., Lapierre J. F., Maranger R. (2020). Contrasting patterns of labile and semilabile dissolved organic carbon from continental waters to the open ocean. J. Geophysical Research: Biogeosciences 125, e2019JG005300. doi: 10.1029/2019JG005300
Lengier M., Szymczycha B., Brodecka-Goluch A., Kłostowska Ż., Kuliński K. (2021). Benthic diffusive fluxes of organic carbon, ammonium and phosphates from deep water sediments of the Baltic Sea. Oceanologia 63, 370–384. doi: 10.1016/j.oceano.2021.04.002
Loginova A. N., Dale A. W., Le Moigne F. A. C., Thomsen S., Sommer S., Clemens D., et al. (2020). Sediment release of dissolved organic matter to the oxygen minimum zone off Peru. Biogeosciences 17, 4663–4679. doi: 10.5194/bg-17-4663-2020
Lønborg C., Álvarez-Saldago X. A. (2012). Recycling versus export of bioavailable dissolved organic matter in the coastal ocean and efficiency of the continental shelf pump. Global Biogeochemical Cycles 26, GB3018. doi: 10.1029/2012GB004353
Lønborg C., Álvarez–Salgado X. A., Letscher R. T., Hansell D. A. (2018). Large stimulation of recalcitrant dissolved organic carbon degradation by increasing ocean temperatures. Front. Mar. Sci. 4. doi: 10.3389/fmars.2017.00436
Łysiak-Pastuszak E., Drgas N., Piątkowska Z. (2004). Eutrophication in the Polish coastal zone: the past, present status and future scenarios. Mar. pollut. Bull. 49, 186–195. doi: 10.1016/j.marpolbul.2004.02.007
Meier M., Kniebusch M., Dieterich C., Gröger M., Zorita E., Elmgren R., et al. (2022). Climate change in the Baltic Sea region: a summary. Earth Syst. Dynam. 13, 457–593. doi: 10.5194/esd-13-457-2022
Miltner A., Emeis K. C., Struck U., Leipe T., Voss M. (2005). Terrigenous organic matter in Holocene sediments from the central Baltic Sea, NW Europe. Chem. Geol. 216, 313–328. doi: 10.1016/j.chemgeo.2004.11.016
Neumann T., Radtke H., Cahill B., Schmidt M., Rehder G. (2022). Non-Redfieldian carbon model for the Baltic Sea (ERGOM version 1.2) – implementation and budget estimates. Geosci. Model. Dev. 15, 8473–8540. doi: 10.5194/gmd-15-8473-2022
Niemisto J., Lund-Hansen L. C. (2019). Instantaneous effects of sediment resuspension on inorganic and organic benthic nutrient fluxes at a shallow water coastal site in the gulf of Finland, baltic sea. Estuaries Coasts 42, 2054–2071. doi: 10.1007/s12237-019-00648-5
Nilsson M. M., Kononets N. M., Ekeroth N., Viktorsson L., Hylén A., Sommer S., et al. (2019). Organic carbon recycling in Baltic Sea sediments – An integrated estimate on the system scale based on in situ measurements. Mar. Chem. 209, 81–93. doi: 10.1016/j.marchem.2018.11.004
Raymond P. A., Spencer R. G. M., Riverine D. O. M. (2015). Biogeochemistry of Marine Dissolved Organic Matter (Second Edition). Eds. Hansell D. A., Carlson C. A. (Boston: Academic Press), 509–533. doi: 10.1016/B978-0-12-405940-5.00011-X
Reader H. E., Thoms F., Voss M., Stedmon C. A. (2019). The influence of sediment-derived dissolved organic matter in the vistula river estuary/gulf of gdańsk. JGR Biogeosciences 124, 115–126. doi: 10.1029/2018JG004658
Repeta D. J. (2015). “Chemical characterization and cycling of dissolved organic matter,” in Biogeochemistry of Marine Dissolved Organic Matter (Second Edition). Eds. Hansell D. A., Carlson C. A. (Academic Press, Boston), 21–63. doi: 10.1016/B978-0-12-405940-5.00002-9
Ridgwell A., Arndt S. (2015). “Why dissolved organics matter: DOC in ancient oceans and past climate change,” in Biogeochemistry of Marine Dissolved Organic Matter (Second Edition). Eds. Hansell D. A., Carlson C. A. (Academic Press, Boston), 509–533. doi: 10.1016/B978-0-12-405940-5.00001-7
Rowe O. F., Dinasquet J., Paczkowska J., Figueroa D., Riemann L., Andersson A. (2018). Major differences in dissolved organic matter characteristics and bacterial processing over an extensive brackish water gradient, the Baltic Sea. Mar. Chem. 202, 27–36. doi: 10.1016/j.marchem.2018.01.010
Silberberger M. J., Koziorowska-Makuch K., Borawska Z., Szczepanek M., Kędra M. (2022). Disentangling the drivers of benthic oxygen and dissolved carbon fluxes in the coastal zone of the southern baltic sea. Estuaries Coasts 45, 2450–2471. doi: 10.1007/s12237-022-01074-w
Szymczycha B., Winogradow A., Kuliński K., Koziorowska K., Pempkowiak P. (2017). Diurnal and seasonal DOC and POC variability in the land-locked sea. Oceanologia 59, 379–388. doi: 10.1016/j.oceano.2017.03.008
Vonk J. E., Tank S. E., Mann P. J., Spencer R. G. M., Treat C. C., Striegl R. G., et al. (2015). Biodegradability of dissolved organic carbon in permafrost soils and aquatic systems: a meta-analysis. Biogeosciences 12, 6915–6930. doi: 10.5194/bg-12-6915-2015
Voss M., Deutsch B., Elmgren R., Humborg C., Kuuppo P., Pastuszak M., et al. (2006). Source identification of nitrate by means of isotopic tracers in the Baltic Sea catchments. Biogeosciences 3, 663–676. doi: 10.5194/bg-3-663-2006
Winogradow A., Pempkowiak J. (2014). Organic carbon burial rates in the Baltic Sea sediments. Estuarine Coast. Shelf Sci. 138, 27–36. doi: 10.1016/j.ecss.2013.12.001
Keywords: DOC, bioavailability, pore water, remineralization rate constant, half-life, Gdańsk Basin
Citation: Lengier M, Koziorowska-Makuch K, Szymczycha B and Kuliński K (2024) Bioavailability and remineralization rates of sediment-derived dissolved organic carbon from a Baltic Sea depositional area. Front. Mar. Sci. 11:1359563. doi: 10.3389/fmars.2024.1359563
Received: 21 December 2023; Accepted: 20 March 2024;
Published: 08 April 2024.
Edited by:
Selvaraj Kandasamy, Central University of Tamil Nadu, IndiaReviewed by:
Per O.J. Hall, University of Gothenburg, SwedenSolomon Dan, Beibu Gulf University, China
Copyright © 2024 Lengier, Koziorowska-Makuch, Szymczycha and Kuliński. This is an open-access article distributed under the terms of the Creative Commons Attribution License (CC BY). The use, distribution or reproduction in other forums is permitted, provided the original author(s) and the copyright owner(s) are credited and that the original publication in this journal is cited, in accordance with accepted academic practice. No use, distribution or reproduction is permitted which does not comply with these terms.
*Correspondence: Katarzyna Koziorowska-Makuch, a2tvemlvQGlvcGFuLnBs