- Leigh Marine Laboratory, Institute of Marine Science, University of Auckland, Auckland, New Zealand
The assessment of site suitability is a crucial step for informing future successful species reintroductions. It ensures that translocated species have the highest chance of survival in their new environment while minimising ecological risks. However, it can be challenging when risk factors are unknown, especially when working with sessile species that cannot easily relocate to more favourable conditions. Under these scenarios, rapid (1-2 week-long), small-scale (< 1 m2) experimental translocations can help reduce uncertainty and improve restoration outcomes. This study conducted small-scale experimental translocations of green-lipped mussels, Perna canaliculus, to 11 shallow coastal sites spread across Tīkapa Moana/the Hauraki Gulf, Aotearoa/New Zealand to investigate the relationship between predator abundance, environmental factors, and mussel loss to help refine existing site selection criteria. The total number of known mussel predators counted from timelapse images was used as a proxy for potential predator pressure. Translocated mussel survival ranged from 10 - 99% and was best predicted by current speed, wind direction, predator abundance, water clarity, and depth (adjusted R2 = 0.505). Predator abundance was best explained by site location (p = 0.001) and had weak correlations among environmental parameters (Rho = 0.067). These results suggest that small, short-term (1-2 week) experimental translocations can help to refine site selection criteria and reduce uncertainty in the site-selection process for larger-scale shellfish reintroduction efforts with unknown and/or hard-to-control risk factors.
Introduction
Acknowledgement of Indigenous knowledge contribution
We would like to acknowledge and express our deep gratitude to members of the Ngāti Manuhiri Settlement Trust (NMST) and Ngāi Tai ki Tāmaki (NTkT) who have shared their knowledge and wisdom with us in the development of this research project. The land and water upon which we conduct our research is the traditional territory of these communities, and their cultural and spiritual connections to these entities are fundamental to their well-being and identity. As part of our commitment to ethical research practices, we have involved both groups in a process of engagement and collaboration to ensure that their perspectives, values, and priorities are reflected in our research design and implementation. Indigenous knowledge is multifaceted, diverse, and deeply rooted in cultural and historical contexts, and we are committed to treating this knowledge with the respect and sensitivity that it deserves. Since our research project has the potential to impact the communities with whom we work, we are committed to ongoing communication and engagement with them throughout the project lifecycle. A collaborative and inclusive approach to research is essential for building trust, respect, and positive relationships with all of our stakeholders, so we will continue to prioritize this approach in all of our work.
The reintroduction of habitat-forming species is rapidly gaining popularity as an active intervention tool to restore degraded marine ecosystems (Swan et al., 2016; Bayraktarov et al., 2020; Fitzsimons et al., 2020; Sievers et al., 2022). A number of these projects currently rely on the translocation of individuals from growth sites (e.g., aquaculture, nurseries, wild populations) to recipient sites with a need for restoration (e.g., degraded areas, De Paoli et al., 2015; Barton et al., 2017; Wilcox et al., 2018; Benjamin et al., 2023). The selection of restoration sites in degraded areas involves a careful evaluation of the risks associated with reintroduction activities and the suitability of the restoration site for translocated populations (Seddon et al., 2007; Elsäßer et al., 2013; Section 5, IUCN/SSC, 2013; Fitzsimons et al., 2020; Robinson et al., 2020). However, there are often many environmental unknowns that can hinder the success of translocation efforts, even after careful consideration of potential risks. As a result, translocations may fail due to unexpected factors, such as unknown criteria for suitable habitat, especially if the species is reintroduced outside of their historic range (Fischer and Lindenmayer, 2000; Stadtmann and Seddon, 2018). This is especially apparent at the beginning of restoration initiatives that work with species and sites with unknown risks (e.g., see outcomes of mussel translocations reported in De Paoli et al., 2015; Wilcox et al., 2018; Alder et al., 2020). There is mounting evidence that small (≥1.5 m2) experimental plots can be used to assess the details of site suitability of recipient environments over an extended period (e.g., 18 months, Benjamin et al., 2023). However, since this process takes time and monitoring resources, it is useful to consider additional tools that can further refine the site selection process ahead of this commitment of resources, across a shorter timeframe, and a larger number of candidate sites.
Predators in the recipient environment often pose one of the biggest limitations to the success of species translocations by consuming newly translocated individuals (hereafter founders; Fischer and Lindenmayer, 2000; Moseby et al., 2015; Robinson et al., 2020). Managing the risk of predation to founders can be difficult, but addressing this early in the translocation process can yield successful outcomes (e.g., see approaches to predation risk management in Robinson et al., 2020). To minimize founder losses and improve the success of reintroductions, translocations are often planned in areas where predator populations are naturally low or absent (e.g., Robinson et al., 2020). In terrestrial biomes, this can be achieved by reintroducing species to predator-free areas such as peninsulas (e.g., Tawharanui Regional Park, Aotearoa/New Zealand [hereafter New Zealand], Jahn et al., 2022) or islands (e.g., Tiritiri Matangi Island, New Zealand, Towns and Ballantine, 1993; Seddon et al., 2014; Moseby et al., 2015; Van Heezik and Seddon, 2018). However, in open marine environments with no control over potential predator movement or water flow, this can be difficult. This is especially evident when working with sessile, biogenic marine species that cannot easily relocate themselves, unlike what has been seen with translocated fish species (e.g., Cochran-Biederman et al., 2015). Furthermore, assessments of marine predator populations can be a complicated process due to the difficulties of obtaining in-situ observations of mobile predator species, which is also an issue for fisheries management (Cochrane, 1999).
Predation remains a pervasive factor limiting a number of shellfish restoration initiatives and can impact the resilience of reefs to future disturbances (e.g., for mussels, De Paoli et al., 2015, 2017; Wilcox and Jeffs, 2019; Alder et al., 2020; for oysters, Tedford and Castorani, 2022; for clams, Whitlow et al., 2003). Part of this issue may be related to the inevitable loss of founders during the translocation process. For example, in the days to weeks following the translocation of mussels to the seafloor, it is common to incur losses due to the stresses encountered during and immediately after the translocation process (Wilcox et al., 2018). Some of these stresses occur:
● during transport due to: excessive handling time (Nguyen et al., 2020; Delorme et al., 2021), physical crushing by harvest machinery or the mass of conspecifics (Wilcox et al., 2018), thermal shock (Delorme et al., 2021), loss of condition (Nguyen et al., 2020; Delorme et al., 2021), lack of gas exchange (Nguyen et al., 2020).
● following deployment to the seafloor due to: smothering by conspecifics (Wilcox et al., 2018), sinking into sediment (Wilcox et al., 2018), low quality and/or availability of food at restoration sites compared with initial growth environment (Wilcox et al., 2018; Alder et al., 2021).
Previous work has suggested that these losses can generate an odour plume that attracts nearby predators, which may further exacerbate mussel loss (e.g., in the lab with the Asian green mussel, Perna viridis, Shin et al., 2002; as suggested for in-situ losses of reintroduced green-lipped mussel, Perna canaliculus, Wilcox and Jeffs, 2019; at in-situ experimental plots of green-lipped mussels, Benjamin et al., 2023). Some of the mobile predator species that have so far limited shellfish reintroductions include:
● sea stars: Pātangaroa/eleven-armed sea stars (Coscinasterias muricata; New Zealand, Wilcox and Jeffs, 2019; Paul-Burke et al., 2022; Benjamin et al., 2023).
● crabs: European green crab (Carcinus maenas; Netherlands, De Paoli et al., 2015; United States, Whitlow et al., 2003; Floyd and Williams, 2004).
● gastropods: Oyster drills (Ocinebrina inornata, Urosalpinx cinerea; United States, Buhle and Ruesink, 2009).
● birds: oystercatchers (Haematopus ostralegus; Netherlands, De Paoli et al., 2015).
● fish: Tāmure/Australasian snapper (Pagrus auratus; New Zealand, Alder et al., 2020, 2022), Whai/ray spp. (New Zealand, Australia, Alder et al., 2020, 2022; Martinez-Baena et al., 2023).
Currently, the most effective predator deterrent in marine systems are physical barriers that inhibit predator access to founders (e.g., Capelle et al., 2019; Alder et al., 2020). However, the addition of barriers incurs substantial additional costs, may not be permitted by local legislation, and is often impractical at restoration scales that are ecologically meaningful (Alder et al., 2020; Schotanus et al., 2020; Alder et al., 2021). Therefore, there is a need to refine methods for understanding the presence and/or movement of mobile predator species that may limit the establishment of founders ahead of expensive, large-scale restoration efforts. There is some indication that methods and timing of translocations may limit predation and improve initial translocated mussel survival (Bertolini et al., 2018; Alder et al., 2022), however less is known about methods to assess the spatial variability of predators at desired restoration sites prior to restoration efforts. There is mounting evidence that the strategic placement of bivalve species can limit predation, such as the installation of euryhaline oyster reefs at less saline locations to limit the impact of freshwater intolerant oyster drills (Johnson and Smee, 2014; Pusack et al., 2019). These observations suggest that preliminary assessments of predator abundances could be a useful tool during initial site selection to reduce the possibility of subsequent losses. Reintroductions of small numbers of individuals can reduce costs and further clarify whether certain sites are suitable for survival of the reintroduced species and if it is worth continuing reintroduction efforts (e.g., as seen with freshwater fish, George et al., 2009).
In the south-eastern Hauraki Gulf of the North Island of New Zealand, endemic green-lipped mussel (Perna canaliculus) reefs once covered ≥ 600 km2 of the seafloor, with the densest reefs inhabiting large stretches of the Firth of Thames (Paul, 2012). Throughout the majority of the 20th century (1900-1969), these reefs were dredged to near functional extinction leading to the collapse of the dredge industry. Despite the collapse and subsequent lack of commercial bottom dredging in these areas, these reefs have failed to reestablish naturally (Paul, 2012). Over the past decade, community groups have started to restore these reefs throughout their native range. At present, mussel reef restoration currently focuses efforts at subtidal, soft-sediment areas devoid of biogenic structure and have seen variable rates of success with only a small number of pilot reefs surviving for more than 2 years. This means that it is important to gain an understanding of local conditions and the potential predators that might be attracted to the sudden introduction of both hard-bottom habitat and a novel food source. Understanding how these factors interact with mussel survival can then be used to determine which sites should be prioritised for future, longer-term site suitability trials, and eventually, large-scale restoration efforts. This study seeks to address whether rapid (week-long), small-scale (< 100 mussels m-2) experimental translocations can be used as a preliminary screening process to improve site selection in degraded areas inside and outside the historic range of green-lipped mussels throughout Tīkapa Moana/the Hauraki Gulf (hereafter the Hauraki Gulf) of the North Island of New Zealand. This study will identify whether a remote assessment of predator abundance, environmental parameters (water velocity, wind speed, wind direction, rainfall, moon phase), and site characteristics (depth, substrate type, effective fetch) correlate with short-term translocated mussel survival following placement on the seafloor.
Material and methods
To test whether field assessments of predator communities can benefit site selection, a series of experimental green-lipped mussel translocations were carried out at 11 sites across the Hauraki Gulf within a 2-month period (October-November 2022; Figure 1). Each translocation lasted one-two weeks depending on site access (e.g., as a result of inclement weather), after which all experimental materials were recovered from the site. Previous work has indicated that translocated adult green-lipped mussels can survive across a range of conditions (e.g., turbidity gradients) when held under cages (McLeod et al., 2012). However, when left unprotected at certain sites they can quickly be removed by local predators (e.g., by predatory sea stars in their historic range, Wilcox et al., 2018; Benjamin et al., 2023). One-two weeks were considered sufficient to compare the potential impacts of predators and environmental factors on mussel survival to inform site selection criteria as previous work has indicated that predators will remove newly translocated mussels within the first few days following placement on the seafloor (Alder et al., 2020, 2022).
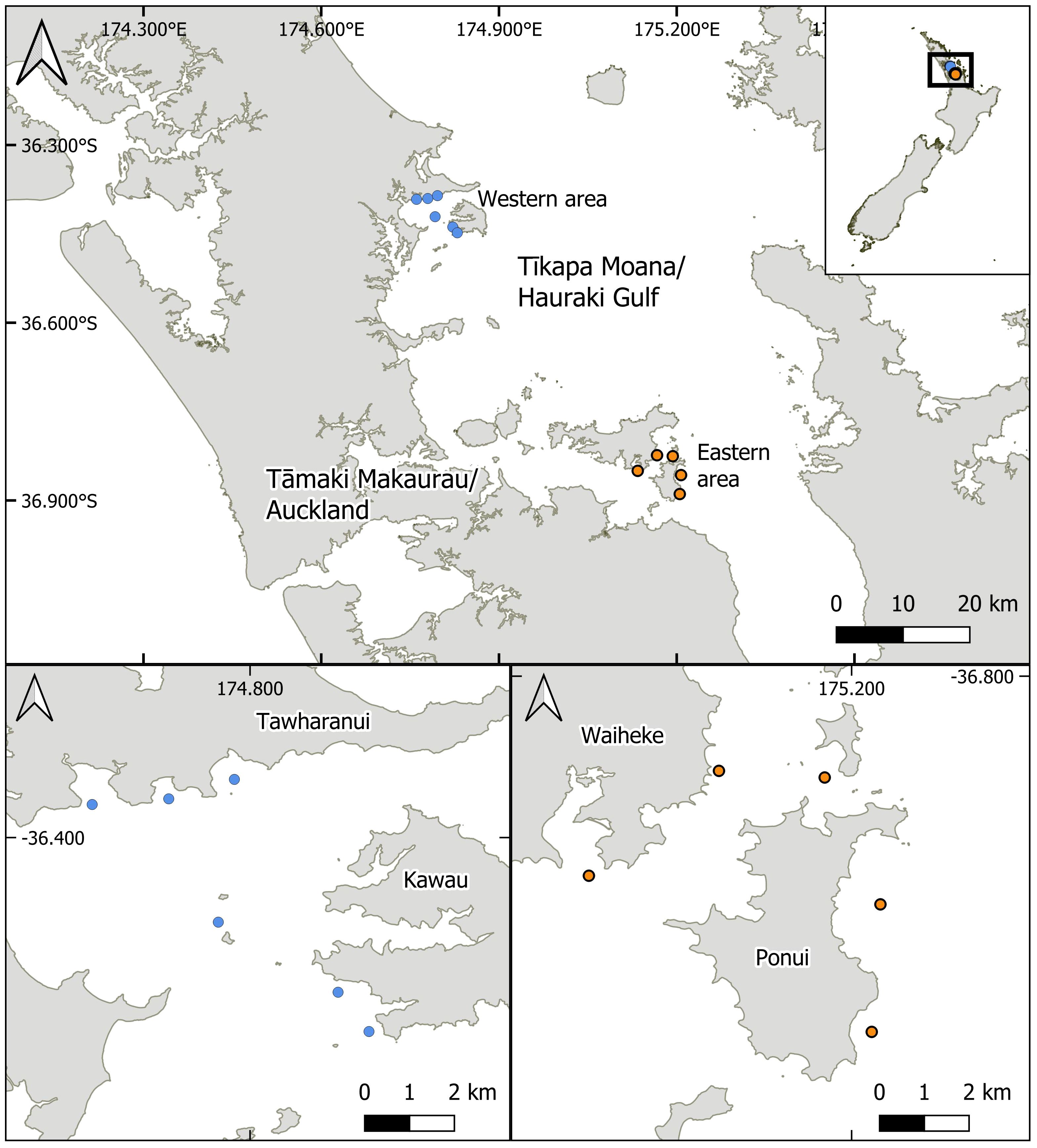
Figure 1 Map of study sites. The site selection process was made in consultation with local indigenous stakeholder groups (blue West: Ngāti Manuhiri; orange East: Ngāi Tai ki Tāmaki) who provided guidance on suitable locations for the study. All of the sites surveyed were suggested as possible locations to consider future mussel reef restoration initiatives. Sites in the eastern Te-Moananui-ā-Toi/Hauraki Gulf (orange sites) were located within the historic range of dense mussel reefs.
Site selection
Sites were selected to cover a range of locations in areas outside (western area, sites W1-W6) and inside (eastern area, sites E1-E5) the historic range of dense, green-lipped mussel reefs in the Hauraki Gulf (Figure 1). Prior to initial site surveys, a shortlist of sites was selected from a list vetted by local indigenous kaitiaki (i.e., Māori environmental stewards, Bennett et al., 2021) who provided guidance on suitable locations for the study (Western area, Ngāti Manuhiri; Eastern area, Ngāi Tai ki Tāmaki). Following the site vetting process and prior to the deployment of timelapse cameras, sites were surveyed using a remotely operated vehicle (BlueROV2) to qualify site characteristics and identify any existing habitat (e.g., shellfish or rocky reef) that may influence the outcome of the study.
Site classification and environmental parameters
To account for differences in site characteristics that can influence mussel loss and predator abundance at different sites during the study period, six site parameters were included (Supplementary Table 1):
● Tidal range and site depth: Daily tidal ranges were obtained by taking the difference in water level between high tide and low tide based on local tide tables. The depth at each site was determined by using the depth recorded at deployment by a dive computer (Shearwater Peregrine) and a correction factor based on the tidal range and the time of day. For example, if experimental groups were deployed to the seabed (8 m max depth) at high tide on a day with a tidal range of 2 m, then 1 m would be subtracted for a mean site depth of 7 m.
● Effective fetch: Effective fetch was used as a proxy for wind exposure. Since higher levels of wind exposure can lead to larger sea states which can remove mussels from the seafloor, this method was used as a proxy for the potential to dislodge mussels from the seafloor. This method calculates the distance (m) to land from the site location using a straight line for every 10° to the nearest landmass going out to a maximum of 300 km (Perry et al., 2018; Seers, 2021). The output provides an average distance for each quadrant (i.e., North, East, South, West). The value for effective fetch for each site was a total average based on quadrant averages.
● Substrate type: At each site the substrate type was qualitatively assessed via ROV footage and by divers as either mud, sand, or rhotolith dominated as no other habitats were observed.
● Water velocity (g plaster loss block-1 day-1): To gain a measure of water velocity during the course of each deployment, three blocks made from plaster of Paris (initial dry weight 328.75 ± 19.40 SD g) were attached to the centre camera with their base stake sticking into the sediment at a height similar to mussels atop sediment (Yund et al., 1991; Commito et al., 1995; Figure 2). Upon recovery, plaster blocks were dehydrated and weighed to obtain a final dry weight. The difference between initial dry weight and final dry weight was used as a proxy for water velocity over the course of each deployment. To account for differences in deployment lengths, this difference was divided by deployment length (days) to obtain estimated daily water velocity for each site.
● Turbidity (Percent of turbidity-obscured images): Since predator species like Tāmure/Australasian snapper (Hereafter Australasian snapper) are visual hunters and suspended sediment can impact mussel filtration rates, the number of turbidity-obscured images recorded for each deployment were included as a proxy for water column turbidity. Previous work has also indicated a positive relationship between the number of turbid images recorded and the number of predators observed, which negatively interact with mussel survival (Alder et al., 2022). All images with < 0.5 m of visibility (i.e., where adjacent mussel groups were not clearly visible) were categorised as turbidity-obscured images. All images and videos with ≥ 0.5 m of visibility (i.e., where mussel groups were visible) were categorised as clear (Supplementary Figure 1).
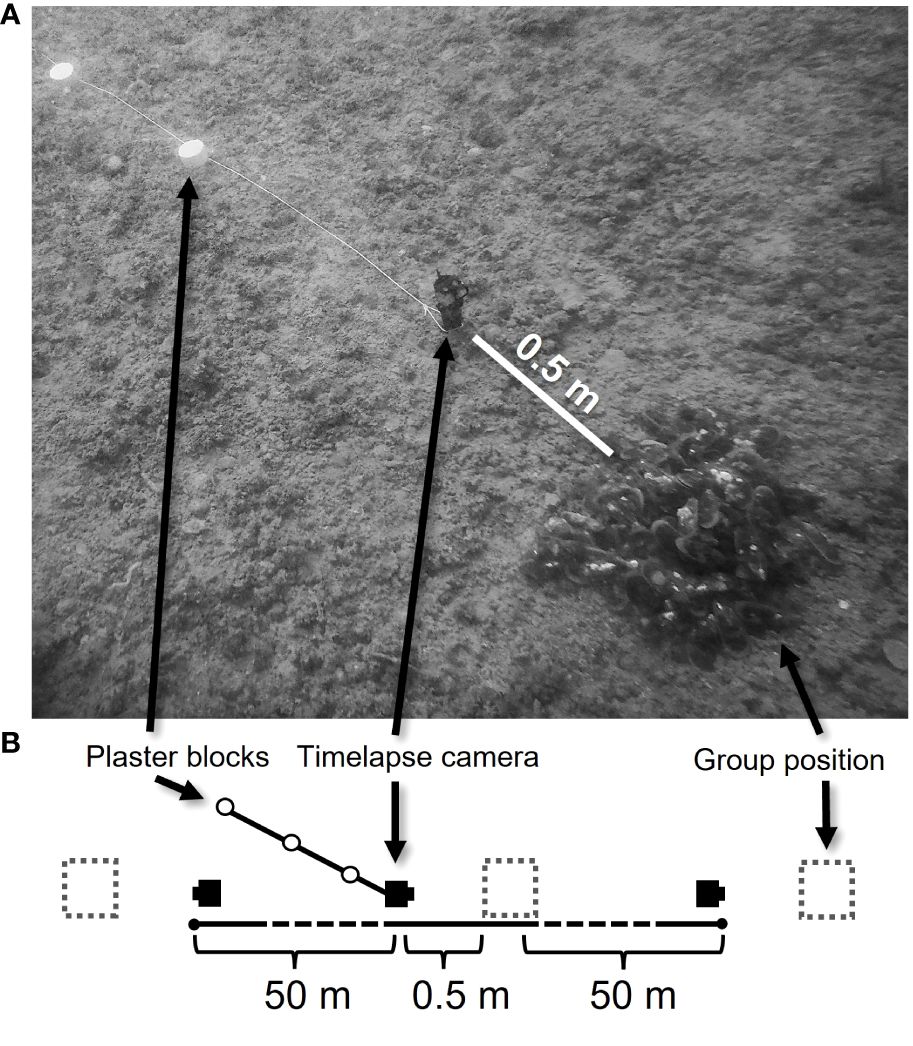
Figure 2 (A) Experimental set-up at each site. (B) Top-down view of experimental set-up. Dashed squares represent the position of each mussel group (100 green-lipped mussels each) deployed to the seafloor.
To account for differences in environmental variables that may influence mussel loss and predator abundance at sites during the study period (Perry et al., 2018; Rueda et al., 2019), four environmental parameters (based on daily totals and means) were compared among deployments:
● Total rainfall (mm), wind speed (m/s), and wind direction (degrees): Used as a proxy for storm events and wave action that are known to influence fish assemblage structure (Friedlander and Parrish, 1998) or hydrodynamic dislodgement of mussel clumps (De Paoli et al., 2015). These environmental data for the period of each deployment were obtained from the NIWA CliFlo database (https://cliflo.niwa.co.nz/).
● Moon phase (Days ± full moon): The number of days before/after full moon were also included as larger tides can contribute to the dislodgement of shellfish placed on the seafloor and lunar phases are known to influence animal activity (e.g., in temperate fish, Hartill et al., 2003; Hanson et al., 2008).
Experimental set-up
Prior to each experimental translocation, adult mussels (shell length (SL) 91.5 ± 9.7 mm SD) sourced from long-line aquaculture farms were completely detached from growth substrate (similar to how they would be harvested for restoration) and separated into groups of 100 mussels each. All groups were taken from seawater, held in tanks circulating unfiltered seawater, and deployed to the seafloor within 48 hours of harvest. At each of the eleven experimental sites, three groups of mussels were placed at individual patches with 50 m spacings in between (300 mussels per site). This distance was considered sufficient to maintain similarities in site characteristics (e.g., depth, substrate type, predominant current directions) while minimising the potential for autocorrelation in fish communities among experimental groups within sites (Mellin et al., 2010; Figure 2).
A programmable timelapse camera was attached to a metal stake and placed 0.5 m away from each experimental mussel group (three per site) and 0.25 m above the seafloor (Kaiser Baas X450 4K Action Camera, 14 MP; based on the design specifications of CoralCam, Greene et al., 2020). This field of view allowed cameras to capture images of predator and non-predator species in the vicinity of mussel groups. This arrangement also helped to standardise depth-of-field and minimise any potential hydrodynamic disturbance to the mussels. All cameras were programmed to take one image every 10 minutes during peak daylight hours (06:30 – 18:30) for the first four days following placement on the seafloor. Due to the logistical constraints of overnight timelapse photography, images were only gathered during daylight hours and did not account for nocturnal predator activity.
Mussel loss
Following each translocation period, all remaining mussels were recovered. Mussel survival was obtained for each group by dividing the number of live mussels at recovery by the total number of mussels at deployment (n = 100). Due to inclement weather limiting site access, deployment lengths ranged between one – two weeks. To account for differences in deployment lengths, mussel survival was divided by the number of days to obtain mussel loss per day (similar to Alder et al., 2020).
Image analysis of community composition
Image analysis followed a similar protocol to Alder et al., 2022. All captured digital images were assessed chronologically and categorised based on depth-of-field by the same reviewer. For both turbidity-obscured and clear images, all visible mobile species were counted and identified to the lowest taxonomic level. Counts followed a MaxN approach (similar to Priede and Merrett, 1996) where all identifiable species present within 1 m of the camera (i.e., just beyond the extent of experimental mussel groups) were recorded. For digital timelapse images, daily species counts were calculated for each site by summing the total counts of each species captured across the 228 images taken each day (i.e., one picture per camera every 10 minutes between 06:30 and 18:30, three cameras per site). Mobile species were classified as potential predators or non-predators of mussels based on previous knowledge of feeding preferences. Previous work (Alder et al., 2020, 2022) considered the following as potential predators: Australasian snapper (Pagrus auratus), spotted estuary smooth-hound (a.k.a rig shark, Mustelus lenticulatus), and the Sydney Octopus (Octopus tetricus). Non-predators included: White trevally (Pseduocaranx dentex), Parore (Girella tricuspidata), Porcupine fish (Tragulichthys jaculiferus), reef squid (Sepioteuthis lessoniana), velvet leatherjacket (Meuschenia scaber), yellow-eyed mullet (Aldrichetta forsteri), and spotties (Notolabrus celidotus) (Russell, 1983; Anderson, 1987; Michael, 1993; Anderson, 1997; Sazima, 1998; Raubenheimer et al., 2005; Grober-Dunsmore et al., 2007; Kendrick and Francis, 2010; King and Clark, 2010; Hauraki Gulf Forum, 2023).
Data analyses
Comparing mussel loss among sites
Due to the non-normal nature of mussel loss data, an independent-samples Kruskal-Wallis test was used to assess variations in daily mussel loss among sites. Dunn’s pairwise comparisons were used to assess patterns of daily mussel loss among sites.
Comparing community composition among sites
Variations in community compositions based on averaged species counts were assessed among sites using a multivariate permutational analysis of variance (PERMANOVA, 9999 permutations). A similarity of percent contribution (SIMPER, multivariate) routine was run to determine which species contributed most to variations observed among deployments. Since differences in the number of turbidity-obscured images directly contributes to the number of possible counts for each deployment/site, a general linear model (GLM, univariate) was used to assess the total proportion of turbidity-obscured images among sites (fixed 11 levels), days (fixed 5 levels: Day 0-4), and the interaction between the two. To assess whether the daily number of turbidity-obscured images influenced the number of predators observed among each deployment, a Pearson’s correlation coefficient was used to determine the degree of correlation between the total number of daily turbidity-obscured images to summed daily predator counts at each site.
Relating community composition to environmental parameters
To account for varying levels of data resolution across both species counts and environmental parameters (turbid images, moon phase, tidal range, total rainfall, water velocity, wind speed, and wind direction; normalised), daily species counts (square-root transformed) were generated by summing total daily counts across all digital timelapse cameras for each species at every site (1-11) and day (0-4). To investigate whether patterns in species compositions were related to differences in environmental parameters during the first four days of each deployment (i.e., the timelapse operating period), a series of multivariate routines were utilized. The first routine applied was RELATE, which tested for similarities among resemblance matrices for daily community compositions and environmental parameters. This routine calculated Spearman rank coefficients to determine the degree of correlation between the biological and environmental resemblance matrices, with Rho values approaching 1 indicating high similarity between the two matrices. To further investigate the correlation between species compositions and specific environmental parameters, a biota and environmental (BEST) routine utilizing a BIOENV method was employed, with 9999 permutations used to search through all possible combinations of environmental factors. This method determined which set of environmental factors best explained the patterns in the species compositions among the different sites. Finally, a distance-based linear model (DistLM) using the BEST function was used to determine the degree of variation that each environmental variable contributed to patterns in the species compositions, with models selected based on Akaike’s information criterion corrected for small sample sizes (AICc). Marginal tests were performed to investigate the contribution of specific environmental parameters to patterns in species community composition among sites.
Predicting causes of mussel loss among sites
Linear mixed effect (LME, Gaussian distribution) models were used to compare daily mussel loss (response variable) to the percentage of turbid images, water velocity, total predator and nonpredator abundances (explanatory variables, fixed factors) across all sites (random factor) for the first four days (random factor) following deployment. Daily mussel loss was calculated by taking the mussel loss per day and subtracting it from the initial number mussels for each group (all starting at 100 on day 0). In the LME, groups were nested within site and days were included to account for repeat measures.
Predicting causes of mussel loss among deployments
LME models (Gaussian distribution) were then used to compare mean daily mussel loss (averaged among the three groups at each site, response variable) to daily tidal range, mean wind direction, mean wind speed, total rainfall, moon phase (days ± full moon), total predator and nonpredator abundance (explanatory variables, fixed factors) across all sites (random factor) for the first four days following deployment.
The data were transformed as required to meet the assumptions of normality for all cases and tested for heterogeneity of variance using Levene’s tests. When p-values were significant (p < 0.05), Bonferroni post hoc tests were used. All GLMs were conducted using IBM SPSS statistics 29. All multivariate analyses (PERMANOVA, SIMPER, PCA, RELATE, BEST, DistLM) were conducted using Primer-E v7+PERMANOVA. All LMEs were conducted using R v4.3.2. Standardized parameters were obtained by fitting models to a standardized version of the dataset. 95% Confidence Intervals (CIs) and p-values were computed using a Wald t-distribution approximation.
Results
Mussel loss among sites
At the end of the translocation period, corrected mussel loss was significantly different among sites (Test statistic (10) = 25.921, p = 0.004). Pairwise comparisons revealed a two-fold higher daily loss among most eastern sites compared with western sites (Figure 3).
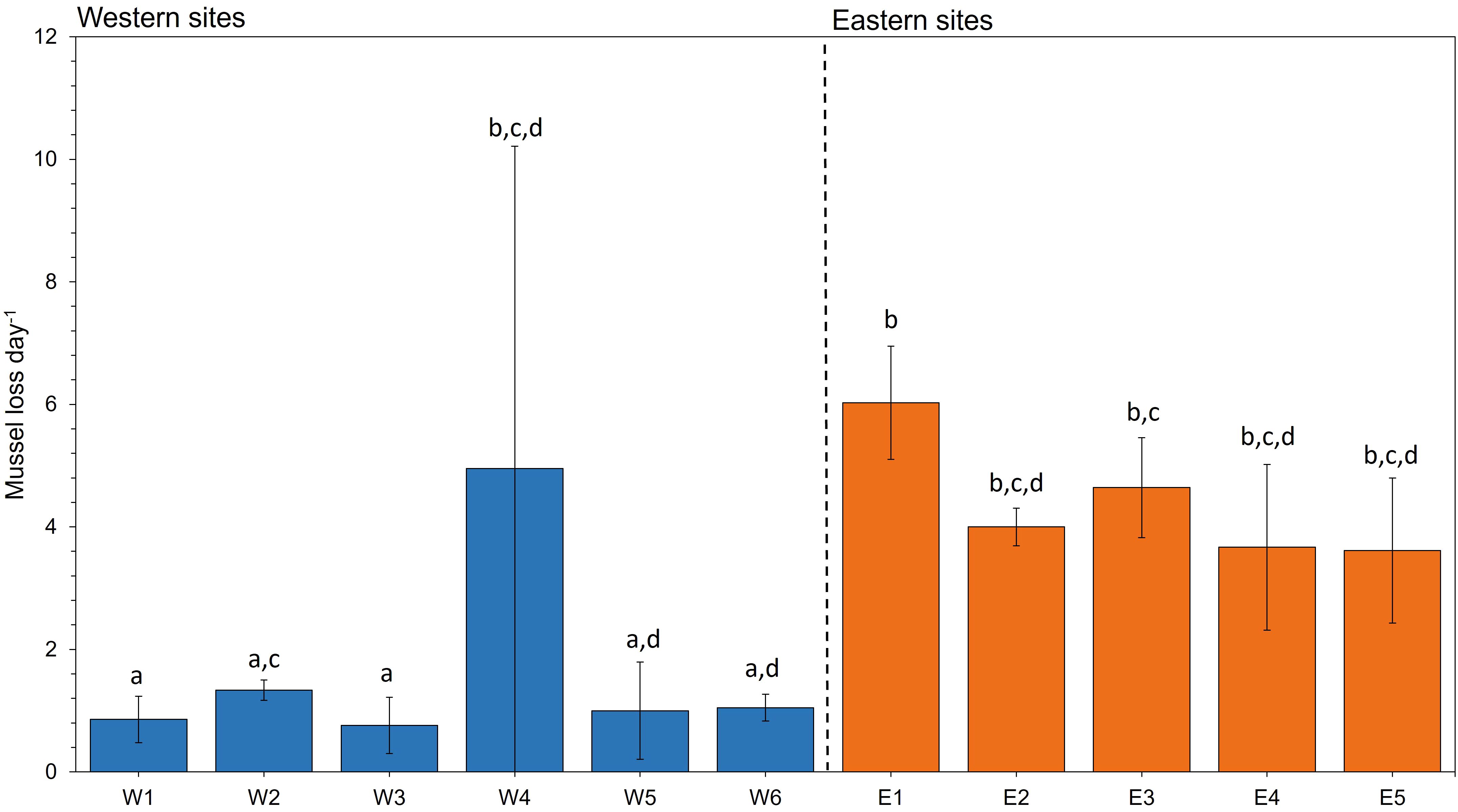
Figure 3 Mean ± SD mussel loss day-1 across all groups at each site. Mussel loss refers to the mean number of mussels lost from the initial 100 mussels to the final number recovered at the end of each deployment. Lower-case letters represent significant differences based on post hoc analysis of mussel loss among mussel groups at each site (n = 3) from an independent-samples Kruskal-Wallis test.
Comparing community composition among sites
A total of 9665 digital timelapse images were captured across three separate deployments and 11 sites between October and November 2022. Of the images gathered, 4993 were classified as clear and 4672 were classified as turbidity-obscured images. From all of the clear images with identifiable mobile species, a total of 1212 individuals from 10 different species were counted (Supplementary Table 2). For all the camera observations of mobile species, 1142 were of potential predator species and 70 were of non-predator species, with Australasian snapper accounting for 93% of the individuals observed (Supplementary Figure 2). Comparisons of community compositions based on hourly counts detected significant differences among sites (square root transformation, pseudo-F10,1065 = 15.134, p = 0.0001). Pairwise tests detected similar patterns in species assemblages across most sites, with generally higher predator abundances detected at eastern sites compared to western sites (Figure 4A).
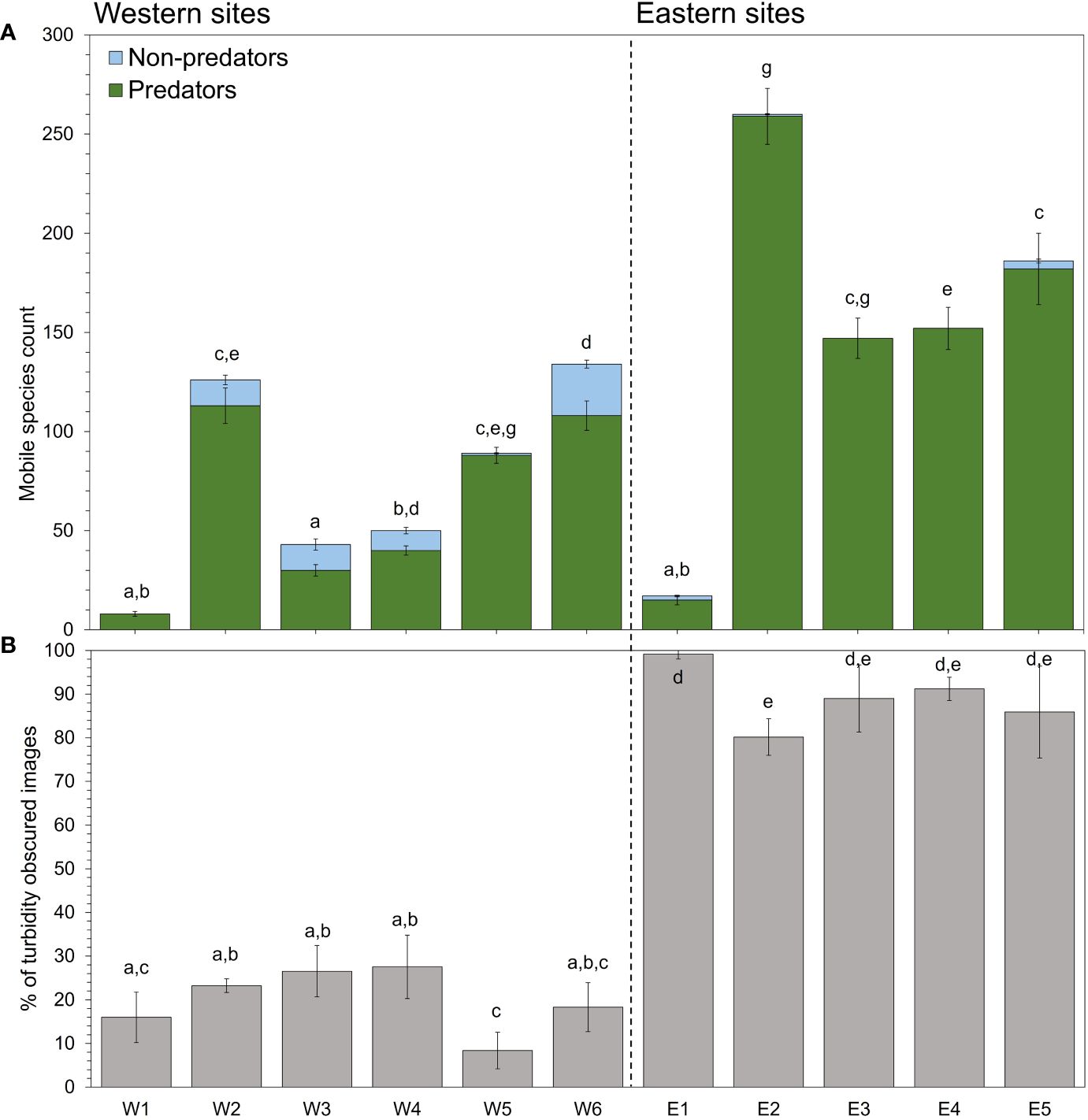
Figure 4 (A) Total number of predators vs. non-predators counted at each site. Count refers to total mobile species counts summed for each camera (n = 3) at each site. Lower-case letters represent significant differences based on post hoc analyses of group means from a PERMANOVA of species counts among sites. Error bars represent the SD of counts across cameras for each site. (B) Mean percent of turbidity-obscured images across all useable images from each camera at each site.
There were no clear daily patterns that emerged in the total daily counts of all predator species among sites. The proportion of turbidity-obscured images were significantly different among sites (square-root transformation, F10,148 = 62.6, p = 0.001), days (F4,148 = 34.5, p = 0.001), and the interaction between the two (F40,148 = 2.0, p = 0.004; Figure 4B). Despite a high variation in the number of turbidity-obscured images among sites and areas, a positive correlation was detected when the number of turbid images were compared against summed daily species counts, suggesting a positive relationship between turbid sites and predator presence (Pearson’s r = 0.412, p = 0.001; Figure 4B). Post-hoc analyses revealed a significantly higher proportion of turbidity-obscured images were recorded for eastern sites compared with western sites.
Relating community composition to environmental parameters among sites
Comparisons of environmental parameters among sites demonstrated that differences in the number of turbid images, depth, and effective fetch contributed to most of the variation across both areas (Figure 5A). Comparisons among normalised daily environmental parameters and square-root transformed species counts revealed little correlation (RELATE, rho = 0.067). Of the environmental parameters examined, total rainfall, moon phase, the proportion of turbid images, and water velocity were most closely related to patterns in species abundance (BEST correlation = 0.239). Overall, environmental parameters explained 41.3% of the total variation observed in patterns of species counts across all sites (DistLM). A distance-based redundancy analysis (dbRDA) indicated that the turbid images (Pseudo-F = 5.86, p = 0.005), moon phase (Pseudo-F = 8.94, p < 0.001), and water velocity (Pseudo-F = 7. 76, p < 0.001) helped drive 32.5% (dbRDA1) and 8.8% (dbRDA2) and of the total variation in daily community compositions (Figure 5B).
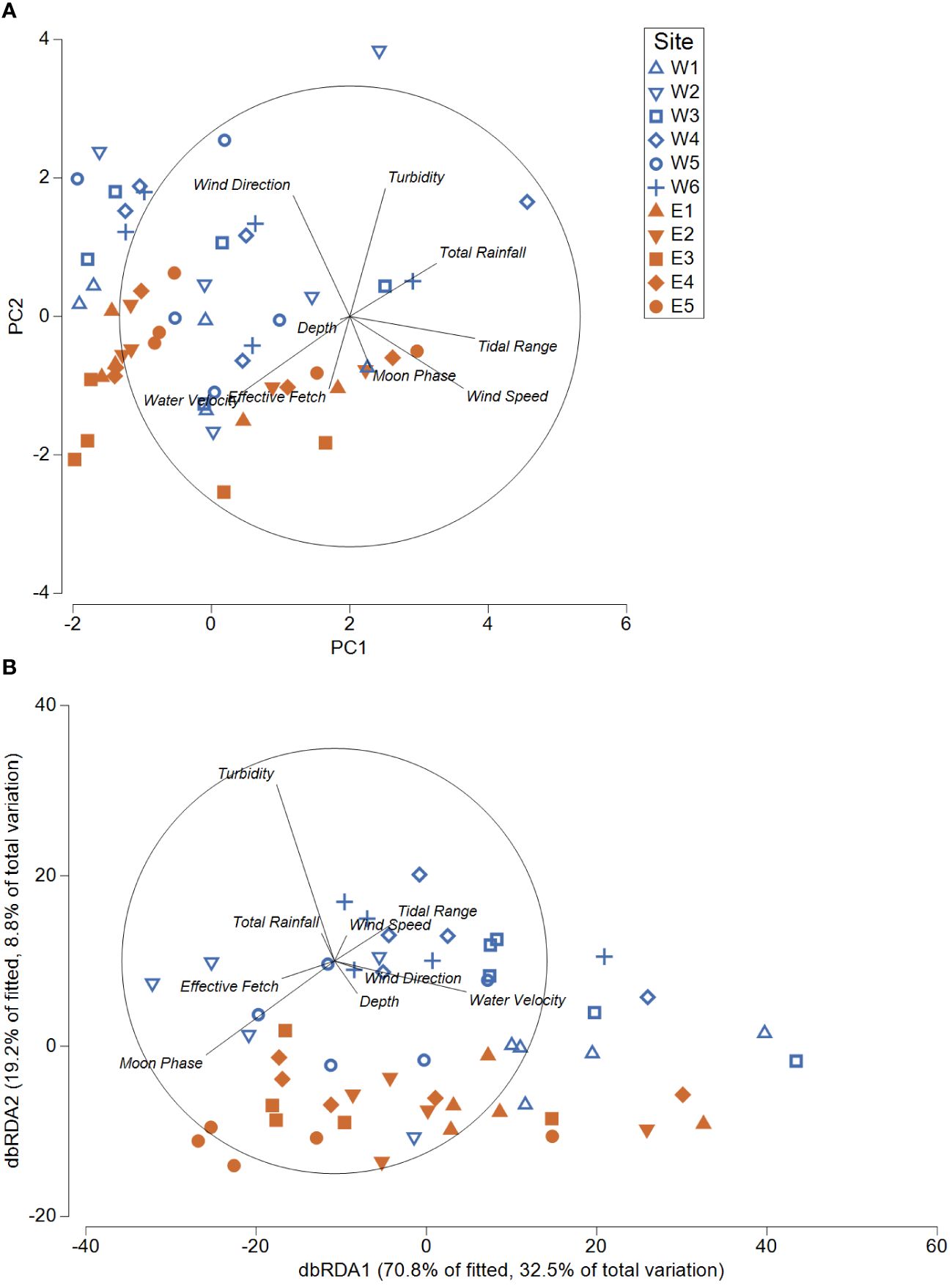
Figure 5 (A) Principal component analysis (PCA) comparing day 0 – 4 environmental parameters (site and deployment characteristics) for all sites in the Western and Eastern areas. (B) Distance based redundancy analysis (dbRDA) indicating environmental predictors that describe 41.3% of the variation in community composition (square-root transformed) for all sites in Western and Eastern areas. Blue indicates western sites (outside of historic range) and orange indicates eastern sites (within the historic range).
Predicting causes of mussel loss among sites
An assessment of the site-specific factors used to predict mussel survival (i.e., daily mussel loss per group) following transfer to the seafloor revealed that mussel survival was positively correlated with water velocity (β = 0.19, 95% CI [0.15, 0.24], t(156) = 8.15, p < 0.001), and negatively correlated with the total number of predators (β = -0.09, 95% CI [-0.16, -0.01], t(156) = -2.28, p = 0.024), and the proportion of turbidity-obscured images (-0.07, 95% CI [-0.10, -0.03], t(156) = -3.50, p < 0.001).
Predicting causes of mussel loss among deployments
An assessment of the deployment-specific factors used to predict mussel survival (i.e., mean daily mussel loss per site) following transfer to the seafloor revealed that mussel survival was negatively correlated with total rainfall (β = -0.17, 95% CI [-0.19, -0.05], t(43) = -3.96, p << 0.001), moon phase (β = -0.95, 95% CI [-1.08, 0.27], t(43) = -4.01, p << 0.001), and tidal range (β = -10.83, 95% CI [-7.40, 2.29], t(43) = -3.23, p = 0.001).
Discussion
Site selection is a critical component of species reintroductions that has a profound impact on the success of reintroduction efforts (IUCN/SSC, 2013; Ravit et al., 2014; Stadtmann and Seddon, 2020). For biogenic species like shellfish, the quality of site selection assessments can be improved through experimental translocations that consider variables associated with the recipient habitat (e.g., water quality, substrate type, predator abundance) and translocations (e.g., transport time, handling stress, time of year; Cochran-Biederman et al., 2015; Stadtmann and Seddon, 2018; Alder et al., 2022). To date, mussel reintroductions within the Hauraki Gulf of the North Island of New Zealand have predominantly relied on the translocation of mussels from aquaculture to sites on the seafloor (Wilcox et al., 2018). While all of the past sites selected for translocations underwent rigorous vetting processes, restored reefs have shown variable rates of success with only a small number of reefs persisting for more than 2 years (e.g., Motuora mussel reef, Sea et al., 2022; Roberts et al., 2023) and others being lost due to a mixture of site-related factors (e.g., possible sediment loading, predation, and/or hydrodynamic dislodgement, Wilcox et al., 2018; Wilcox and Jeffs, 2019; Alder et al., 2020). The resulting variability in mussel survival among previous translocations has signalled a need to refine suitable site criteria for future, large-scale efforts. There is mounting interest to use small-scale experimental plots as a starting point at a site, following protocols outlined by Benjamin et al. (2023). However, given the commitment of resources towards these trials and the scale and range of possible candidate sites across the Hauraki Gulf, there is a need to develop additional tools to prioritise where these will be installed. The results of this study suggest that multi-day observations of water characteristics, weather events, and an estimation of potential predator impact around small groups of mussels can quickly improve expectations of translocated mussel survival across a large range of candidate sites. This method provides additional scaffolding to the site selection process and helps identify site-specific criteria that might influence mussel survival at larger experimental plots or subsequent deployments.
Rapid, small-scale experimental translocations can provide particularly valuable insight to optimise site selection, especially when ecological knowledge is limited (Stadtmann and Seddon, 2018; Benjamin et al., 2023). This may be especially prevalent when the recipient environment has been drastically altered from its original historic state, which is the case of the historic range (i.e., the eastern sites in this study) of dense subtidal mussel reefs in the Hauraki Gulf, North Island (Paul, 2012) and the Marlborough Sounds, South Island of New Zealand (Handley et al., 2017). This study provides evidence that the suitability of sites within the historic range of mussel reefs are not as conducive to mussel survival when compared to areas outside, which is a now widely accepted viewpoint (i.e., that “past species distributions do not indicate current suitability and that current species’ distribution does not guarantee future suitability”, Seddon et al., 2014). The higher percentage of turbid images observed at historic mussel reef sites, coupled with the presence of soft-sediment deposits, represent a significant difference in the environmental conditions between both areas. This is likely a result of a number of large-scale interacting stressors, such as deforestation along the system’s catchment, the infilling of wetlands for agriculture, urban development, and the removal of sediment-stabilising seafloor habitat (i.e., dredging of historic mussel reefs; Kelly and Sim-Smith (2023)). While previous work has indicated that, when protected, translocated adult mussels can survive in these conditions (68% survival after 500 days, McLeod et al., 2012), these results suggest that when left unprotected (which is the case for most translocations) there is greater potential for mussel reintroduction success outside of the historic range of dense mussel reefs. Furthermore, by incorporating observations of mussel loss, water clarity, weather phenomena, and predator abundance from short-term translocations, it is possible to reduce the uncertainty of site-specific risks and shift towards a more realistic understanding of potential post-deployment scenarios. These methods can be particularly useful in the decision-making process, especially when co-developing restoration projects across a wide variety of stakeholders. Future work should consider how these data can be used to rank sites, possibly as a site-selection tool, to prioritise where additional resources should be committed for future experimental translocations and/or larger-scale deployments.
A central aim of mussel restoration is to increase the biodiversity of reef areas (Sea et al., 2022). In some areas this will also mean attracting predators to a novel food source (e.g., Wilcox and Jeffs, 2019; Alder et al., 2020, 2022). In this study, timelapse camera imagery provided a snapshot of the potential magnitude of impact predators could have at different sites and provided some indication for locations where this external stressor could be minimised. While the spatial distribution of species assemblages can be inherently variable due to biotic (e.g., prey availability) and/or abiotic (e.g., habitat structure and availability) factors, differences in predator counts between both areas helped provide additional evidence for what large-scale efforts will have to account for as projects progress and grow. Risk assessments that include mobile species surveys can also shed light on additional considerations for the long-term persistence of restored reefs. For example, observations by mussel farmers indicate that fish species like Parore or Spottys, which in this study were considered non-predators due to their inability to consume adult mussels, might contribute towards a large proportion of spat losses (i.e., young mussel life stages that are seeded onto growth lines; personal observations; also, Australasian snapper, Underwood et al., 2023). This provides further impetus to consider how species communities might limit mussel reefs at the beginning (e.g., with respect to founder survival) and later stages (e.g., with respect to larval recruit survival) of reef establishment. Future work should seek to address the actual impact of large mobile species on mussel survival in larger-scale deployments and within natural mussel reef systems to help support consideration of additional approaches or measures for reintroducing mussels to areas with a higher abundance of predators.
While the eastern section of the Hauraki Gulf was once capable of supporting vast areas of filter-feeders (Paul, 2012), it is possible that the interactions among a lack of water clarity, depth, and water velocity at these sites contributed to mussel mortality following transfer to the seafloor and the subsequent attraction of predators (similar to observations in Alder et al., 2022). The importance of water clarity as a predictor of mussel loss in both Alder et al. (2022) and here, suggests that areas dominated by very fine soft sediment, like the eastern sites in this study, also have larger amounts of suspended sediment, which could have impacted translocated mussels’ ability to feed – possibly contributing to higher natural losses and the subsequent attraction of nearby predators through an odour plume (Shin et al., 2002). Since restoration in the Hauraki Gulf mainly relies on the use of mussels from suspension aquaculture, changes in food quality and quantity could have outsized impacts on mussel ability to acclimate to novel conditions (Wilcox et al., 2018; Alder et al., 2020, 2021).
The importance of depth as a predictor of mussel loss is consistent with recent work that has examined the relationships of exposure, depth (Toone et al., 2023) and water velocity (Benjamin et al., 2023) in supporting restored green-lipped mussel survival and increased larval settlement at restored sites. For example, interactions between wind direction and depth within turbid environments can have different implications for primary production, which likely impacts the abundance or quality of food available for translocated mussels. While the resuspension of sediment in shallow coastal areas can limit the depth range of certain biogenic species, such as seagrass (Carr et al., 2010), it can also avail nutrients that stimulate primary productivity and increased biomass of filter feeders (as seen with the freshwater clam Corbicula fluminea; Zhang et al., 2023). Conversely, while sediment resuspension might benefit primary production, secondary production can be inhibited, thereby reducing the capacity to support higher trophic levels (e.g., as suggested by Jin et al., 2022). Future work should consider ways to compare primary and secondary production at prospective sites and whether differences correspond with variations in translocated mussel survival.
The importance of water flow as a predictor of mussel loss is not surprising as it has limited the establishment of intertidal mussel reefs through the physical dislodgement of translocated mussels (e.g., for blue mussels Mytilus edulis in De Paoli et al., 2015). For subtidal restoration, differences in water flow also likely influences the magnitude of interaction with additional external stressors like predation. For example, higher water velocities may also increase the range of odour plumes released by individuals that perish naturally (i.e., not as a result of predation), thus attracting predators from a wider area (i.e., as seen with sea stars and Perna viridis in Shin et al., 2002 and green-lipped mussels in Wilcox and Jeffs, 2019). Conversely, high water flow could also benefit the establishment of mussel reefs on the seafloor by ensuring the delivery of sufficient quantities of high-quality planktonic food, limiting sediment settlement, and/or reducing predator access (including humans; as seen with Perna viridis in Rajagopal et al., 1998). High water flow also appears to be a feature of locations where both remnant and restored mussel reefs persist throughout the Hauraki Gulf. While more work is needed to clarify the importance of water flow and depth (especially across subtidal soft-sediment sites), these observations provide evidence for further consideration of this interaction. Future work should consider how to incorporate additional monitoring or modelling of water flow to identify suitable habitat.
The dominance of Australasian snapper across all sites is consistent with the known ubiquity of this species across a range of habitats throughout the Hauraki Gulf (Kendrick and Francis, 2002; Burrows et al., 2008; Alder et al., 2022). Australasian snapper is a generalist species that consume a wide variety of prey that includes benthic infauna, epifauna, bivalves, crustaceans and small fish (Godfriaux, 1969; Parsons et al., 2014). At rocky reef sites, snapper predation is known to modify the environment by regulating grazer numbers, such as kina (Evechinus chloroticus), which indirectly promotes the growth of macroalgae (Babcock et al., 1999; Shears and Babcock, 2002). Their relationship with modifying natural and restored mussel reefs remains unclear, however this study provides evidence that, regardless of the location, snapper will likely use reef sites for foraging opportunities (e.g., possibly as a result of enhanced infaunal diversity, Sea et al., 2022). Still, differences in snapper numbers and their relationship with mussel survival has proven useful to understand optimal times of year for mussel reintroductions (Alder et al., 2022) and now appear to serve as an indicator species to help understand spatial variations of mussel survival.
While Australasian snapper dominated the areas where this study’s sites were selected, it is also possible that the timelapse camera methodology used by this study has a bias towards documenting the often gregarious and sometimes residential nature of this species (Parsons et al., 2014). However, the use of multiple cameras over multiple days continues to address causes for mussel loss and provides critical information on the predicted impacts reintroduction activities have on large mobile species. While the span of coverage this method provides is somewhat comprehensive, the use of videos captured at similar intervals to the cameras used in this study (e.g., every hour) could capture some of the potential activity and species behaviour missed by still photos. Furthermore, this methodology did not capture nocturnal activity, which may have resulted in the unexplained loss of mussels from sites like E1. Future work should consider comparing different remote camera techniques (e.g., videography vs. photography) and capturing nocturnal activity to further clarify whether Australasian snapper are the predominant mobile species visiting newly translocated mussel groups within a week of deployment to the seafloor. Using these approaches at both natural and restored reefs could provide additional understanding of the interactions among mussel reef structure and the potential pressure of predator species.
Rapid, small-scale experimental translocations such as those in this study can easily be integrated as an additional step into the methods and risk analyses currently used to develop mussel reef restoration projects. An updated process that considers the use of these surveys could look like the following (new steps in bold):
1) Discussion with vested community groups to gain an idea of the historical presence of the target species and where there is interest to pursue restoration across a number of candidate sites.
2) Generate a list of sites to consider further and plan small-scale experimental translocations to further assess water clarity, predator abundance, water velocity, and mussel loss from small, discrete groups.
3) From these screening translocations, use variations in mussel losses and observations from timelapse imagery to help rank and prioritise a short-list of sites for more detailed assessments of site suitability.
4) Consider whether additional rapid, experimental translocations are warranted or consider increasing the scale of translocation efforts (e.g., experimental mussel patches following Benjamin et al., 2023).
This study supports the use of small, experimental translocations to quickly screen a large list of candidate sites to inform and refine site selection. These simulations can help tease apart site (e.g., m2) and area (e.g., km2) specific factors that will contribute to mussel loss, while providing additional data towards understanding patterns within the suite of factors that make a recipient environment suitable for mussel survival. Variations in mussel loss per day among sites in this study suggest that the selection of appropriate restoration sites should offer additional consideration to the degree of exposure (e.g., wind direction, effective fetch, depth, water velocity), water quality (e.g., water clarity), and existing biotic communities (e.g., predators) prior to the deployment of additional experimental mussel plots or larger-scale deployments. The assessment of these factors with low-cost programmable timelapse cameras continue to support their utility for providing a window into the interactions between reintroduced mussels and their recipient environment. The use of small, discrete groups of mussels quickly reduced uncertainty, cost, and effort, alleviating some of the pressures encountered with large-scale deployments. These data have already been used to provide stakeholder groups with additional information, which has benefitted the decision-making process for upcoming restoration projects.
Data availability statement
The datasets presented in this study can be found in online repositories. The names of the repository/repositories and accession number(s) can be found below: 10.17608/k6.auckland.24791340.
Ethics statement
The manuscript presents research on animals that do not require ethical approval for their study.
Author contributions
AA: Conceptualization, Formal analysis, Investigation, Methodology, Project administration, Visualization, Writing – original draft. JH: Conceptualization, Funding acquisition, Supervision, Writing – review & editing.
Funding
The author(s) declare that financial support was received for the research, authorship, and/or publication of this article. We thank The Nature Conservancy New Zealand, the Mussel Reef Restoration Trust, and the Auckland Foundation for funding this research.
Acknowledgments
We wish to also extend our gratitude to Revive our Gulf, L. Beamish (NTkT), I. Penrose (NTkT), C. Shaw (NMST), M. Williams (NMST), A. Jeffs, C. Bennet, S. Kelly, D. Immenga, M. Azhar, E. Lis, B. Masters, E. Ferretti, S. Roberts, P. Caiger, J. Rapson, S. Schenone, C. Byron, K. Burnham, F. Mackechnie, J. Good, E. Benjamin, and others who consistently set aside time to help with experimental design, collections, translocations, and recoveries.
Conflict of interest
The authors declare that the research was conducted in the absence of any commercial or financial relationships that could be construed as a potential conflict of interest.
Publisher’s note
All claims expressed in this article are solely those of the authors and do not necessarily represent those of their affiliated organizations, or those of the publisher, the editors and the reviewers. Any product that may be evaluated in this article, or claim that may be made by its manufacturer, is not guaranteed or endorsed by the publisher.
Supplementary material
The Supplementary Material for this article can be found online at: https://www.frontiersin.org/articles/10.3389/fmars.2024.1354257/full#supplementary-material
References
Alder A., Jeffs A., Hillman J. R. (2020). Considering the use of subadult and juvenile mussels for mussel reef restoration. Restor. Ecol. 29, 1–10. doi: 10.1111/rec.13322
Alder A., Jeffs A. G., Hillman J. R. (2021). The importance of stock selection for improving transplantation efficiency. Restor. Ecol. 29 (3), e13561. doi: 10.1111/rec.13561
Alder A., Jeffs A., Hillman J. (2022). Timing mussel deployments to improve reintroduction success and restoration efficiency. Mar. Ecol. Prog. Ser. 698, 69–83. doi: 10.3354/meps14157
Anderson T. A. (1987). Quantitative and qualitative aspects of the digestive system of the luderick, Girella tricuspidata (Pisces, Kyphosidae) (Sydney, Australia: University of Sydney).
Anderson T. J. (1997). Habitat selection and shelter use by Octopus tetricus. Mar. Ecol. Prog. Ser. 150, 137–148. doi: 10.3354/meps150137
Babcock R. C., Kelly S., Shears N. T., Walker J. W., Willis T. J. (1999). Changes in community structure in temperate marine reserves. Mar. Ecol. Prog. Ser. 189, 125–134. doi: 10.3354/meps189125
Barton J. A., Willis B. L., Hutson K. S. (2017). Coral propagation: a review of techniques for ornamental trade and reef restoration. Rev. Aquacult. 9, 238–256. doi: 10.1111/raq.12135
Bayraktarov E., Brisbane S., Hagger V., Smith C. S., Wilson K. A., Lovelock C. E., et al. (2020). Priorities and motivations of marine coastal restoration research. Front. Mar. Sci. 7, 1–14. doi: 10.3389/fmars.2020.00484
Benjamin E. D., Handley S. J., Jeffs A., Olsen L., Toone T. A., Hillman J. R. (2023). Testing habitat suitability for shellfish restoration with small-scale pilot experiments. Conserv. Sci. Pract. 5 (2), e12878. doi: 10.1111/csp2.12878
Bennett C., Matunga H., Steyl S., Borell P., Dionisio R., Hāpuku A. (2021). Mana whenua engagement in Crown and Local Authority-initiated environmental planning processes: A critique based on the perspectives of Ngāi Tahu environmental kaitiaki. New Z. Geogr. 77, 63–75. doi: 10.1111/nzg.12304
Bertolini C., Montgomery W. I., O'connor N. E. (2018). Habitat with small inter-structural spaces promotes mussel survival and reef generation. Mar. Biol. 165, 1–11. doi: 10.1007/s00227-018-3426-8
Buhle E. R., Ruesink J. L. (2009). Impacts of invasive oyster drills on olympia oyster (Ostrea luridaCarpenter 1864) recovery in Willapa Bay, Washington, United States. J. Shellf. Res. 28, 87–96. doi: 10.2983/035.028.0115
Burrows M. T., Harvey R., Robb L. (2008). Wave exposure indices from digital coastlines and the prediction of rocky shore community structure. Mar. Ecol. Prog. Ser. 353, 1–12. doi: 10.3354/meps07284
Capelle J. J., Leuchter L., Wit M., Hartog E., Bouma T. J. (2019). Creating a window of opportunity for establishing ecosystem engineers by adding substratum: a case study on mussels. Ecosphere 10, 1–16. doi: 10.1002/ecs2.2688
Carr J., D'odorico P., Mcglathery K., Wiberg P. (2010). Stability and bistability of seagrass ecosystems in shallow coastal lagoons: Role of feedbacks with sediment resuspension and light attenuation. J. Geophys. Res. 115, 1–14. doi: 10.1029/2009JG001103
Cochran-Biederman J. L., Wyman K. E., French W. E., Loppnow G. L. (2015). Identifying correlates of success and failure of native freshwater fish reintroductions. Conserv. Biol. 29, 175 –186. doi: 10.1111/cobi.12374
Cochrane K. L. (1999). Complexity in fisheries and limitations in the increasing complexity of fisheries management. ICES J. Mar. Sci. 56, 917–926. doi: 10.1006/jmsc.1999.0539
Commito J. A., Thrush S. F., Pridmore R. D., Hewitt J. E., Cummings V. J. (1995). Dispersal dynamics in a wind-driven benthic system. Limnol. Oceanogr. 40, 1513–1518. doi: 10.4319/lo.1995.40.8.1513
Delorme N. J., Venter L., Rolton A., Ericson J. A. (2021). Integrating animal health and stress assessment tools using the green-lipped mussel Perna canaliculus as a case study. J. Shellf. Res. 40, 1–25. doi: 10.2983/035.040.0109
De Paoli H., Van De Koppel J., Van Der Zee E., Kangeri A., Van Belzen J., Holthuijsen S., et al. (2015). Processes limiting mussel bed restoration in the Wadden-Sea. J. Sea Res. 103, 42–49. doi: 10.1016/j.seares.2015.05.008
De Paoli H., Van Der Heide T., Van Den Berg A., Silliman B. R., Herman P. M. J., Van De Koppel J. (2017). Behavioral self-organization underlies the resilience of a coastal ecosystem. Proc. Natl. Acad. Sci. 114, 8035–8040. doi: 10.1073/pnas.1619203114
Elsäßer B., FARIÑAS-FRANCO J. M., WILSON C. D., KREGTING L., ROBERTS D. (2013). Identifying optimal sites for natural recovery and restoration of impacted biogenic habitats in a special area of conservation using hydrodynamic and habitat suitability modelling. J. Sea Res. 77, 11–21. doi: 10.1016/j.seares.2012.12.006
Fischer J., Lindenmayer D. B. (2000). An assessment of the published results of animal relocations. Biol. Conserv. 96, 1–11. doi: 10.1016/S0006-3207(00)00048-3
Fitzsimons J. A., Branigan S., Gillies C. L., Brumbaugh R. D., Cheng J., Deangelis B. M., et al. (2020). Restoring shellfish reefs: Global guidelines for practitioners and scientists. Conserv. Sci. Pract. 2, 1–11. doi: 10.1111/csp2.198
Floyd T., Williams J. (2004). Impact of green crab (Carcinus maenas L.) predation on a population of soft-shelled clams (Mya arenaria L.) in the southern Gulf of St. Lawrence. J. Shellf. Res. 23, 457–462.
Friedlander A. M., Parrish J. D. (1998). Temporal dynamics of fish communities on an exposed shoreline in Hawaii. Environ. Biol. Fishes 53, 1–18.
George A. L., Kuhajda B. R., Williams J. D., Cantrell M. A., Rakes P. L., Shute J. R. (2009). Guidelines for propagation and translocation for freshwater fish conservation. Fisheries 34, 529–545. doi: 10.1577/1548-8446-34.11.529
Godfriaux B. L. (1969). Food of predatory demersal fish in Hauraki Gulf. New Z. J. Mar. Freshw. Res. 3, 518–544. doi: 10.1080/00288330.1969.9515315
Greene A., Forsman Z., Toonen R. J., Donahue M. J. (2020). CoralCam: a flexible, low-cost ecological monitoring platform. HardwareX 7, 1–19. doi: 10.1016/j.ohx.2019.e00089
Grober-Dunsmore R., Frazer T. K., Beets J. P., Lindberg W. J., Zwick P., Funicelli N. A. (2007). Influence of landscape structure on reef fish assemblages. Landscape Ecol. 23, 37–53. doi: 10.1007/s10980-007-9147-x
Handley S. J., Gibbs M., Swales A., Olsen G., Ovenden R., Bradley A. (2017). A 1,000 years history of seabed change in Pelorus Sound/Te Hoire, Marlborough (Nelson, New Zealad: NIWA).
Hanson K. C., Arrosa S., Hasler C. T., Suski C. D., Philipp D. P., Niezgoda G., et al. (2008). Effects of lunar cycles on the activity patterns and depth use of a temperate sport fish, the largemouth bass, Micropterus salmoides. Fish. Manage. Ecol. 15, 357–364. doi: 10.1111/j.1365-2400.2008.00634.x
Hartill B. W., Morrison M. A., Smith M. D., Boubée J., Parsons D. M. (2003). Diurnal and tidal movements of snapper (Pagrus auratus, Sparidae) in an estuarine environment. Mar. Freshw. Res. 54, 1–11. doi: 10.1071/MF02095
Hauraki Gulf Forum. (2023). State of our Gulf 2023 (State of the Environment Report). Auckland, New Zealand: Hauraki Gulf Forum, 1–99.
IUCN/SSC (2013). Guidelines for reintroductions and other conservation translocations. Ed. Commission I. S. S. (Gland, Switzerland: International Union for Conservation of Nature).
Jahn P., Cagua F., Molles L., Ross J., Germano J. (2022). Kiwi translocation review: are we releasing enough birds and to the right places? New Z. J. Ecol. 46, 1–19. doi: 10.20417/nzjecol.46.1
Jin H., Van Leeuwen C. H. A., Van De Waal D. B., Bakker E. S. (2022). Impacts of sediment resuspension on phytoplankton biomass production and trophic transfer: Implications for shallow lake restoration. Sci. Total Environ. 808, 12. doi: 10.1016/j.scitotenv.2021.152156
Johnson K. D., Smee D. L. (2014). Predators influence the tidal distribution of oysters (Crassostrea virginica). Mar. Biol. 161, 1557–1564. doi: 10.1007/s00227-014-2440-8
Kendrick T. H., Francis M. P. (2002). Fish assemblages in the Hauraki gulf, New Zealand. New Zealand J. Mar. Freshwater Res. 36 (4), 699–717.
Kendrick T. H., Francis M. P. (2010). Fish assemblages in the Hauraki Gulf, New Zealand. New Z. J. Mar. Freshw. Res. 36, 699–717. doi: 10.1080/00288330.2002.9517124
King K. J., Clark M. R. (2010). The food of rig (Mustelus lenticulatus) and the relationship of feeding to reproduction and condition in Golden Bay. New Z. J. Mar. Freshw. Res. 18, 29–42. doi: 10.1080/00288330.1984.9516026
Kelly S., Sim-Smith C. (2023). State of our Gulf 2023 (State of the environment report 2023). (Auckland, New Zealand). 99 pp.
Martinez-Baena F., Raoult V., Taylor M. D., Gaston T. F., Mcleod I., Bishop M. J. (2023). Trophic structure of temperate Australian oyster reefs within the estuarine seascape: a stable isotope analysis. Estuaries Coasts 46, 844–859. doi: 10.1007/s12237-022-01157-8
McLeod I. M., Parsons D. M., Morrison M. A., Le Port A., Taylor R. B. (2012). Factors affecting the recovery of soft-sediment mussel reefs in the Firth of Thames, New Zealand. Mar. Freshw. Res. 63, 1–7. doi: 10.1071/MF11083
Mellin C., Bradshaw C. J. A., Meekan M. G., Caley M. J. (2010). Environmental and spatial predictors of species richness and abundance in coral reef fishes. Global Ecol. Biogeogr. 19, 212–222. doi: 10.1111/j.1466-8238.2009.00513.x
Michael S. W. (1993). Reef sharks and rays of the world. A guide to their identification, behaviour and ecology (Monterey, CA: Annapolis). Sea Challengers.
Moseby K., Carthey A., Schroeder T. (2015). “The influence of predators and prey naivety on reintroduction success: Current and future directions,” in Advances in reintroduction biology of Australian and New Zealand fauna. Eds. Armstrong D. P., Hayward M. W., Moro D., Seddon P. J. (CSIRO Publishing, Melbourne, Australia).
Nguyen T. V., Ragg N. L. C., Alfaro A. C., Zamora L. N. (2020). Physiological stress associated with mechanical harvesting and transport of cultured mussels (Perna canaliculus): A metabolomics approach. Aquaculture 529, 1–8. doi: 10.1016/j.aquaculture.2020.735657
Parsons D. M., Sim-Smith C. J., Cryer M., Francis M. P., Hartill B., Jones E. G., et al. (2014). Snapper (Chrysophrys auratus): a review of life history and key vulnerabilities in New Zealand. New Z. J. Mar. Freshw. Res. 48, 256–283. doi: 10.1080/00288330.2014.892013
Paul L. J. (2012). A history of the Firth of Thames dredge fishery for mussels: use and abuse of a coastal resource. New Zealand Aquatic Environment and Biodiversity Report (Wellington, New Zealand: Ministry of Agriculture and Forestry).
Paul-Burke K., Ngarimu-Cameron R., Paul W., Burke J., Cameron K., O’brien T., et al. (2022). Ngā tohu o te taiao: Observing signs of the natural world to identify seastar over-abundance as a detriment to shellfish survival in Ōhiwa Harbour, Aotearoa/New Zealand. New Z. Sociol. 37, 1–25.
Perry D., Staveley T. A. B., Hammar L., Meyers A., Lindborg R., Gullström M. (2018). Temperate fish community variation over seasons in relation to large-scale geographic seascape variables. Can. J. Fish. Aquat. Sci. 75, 1723–1732. doi: 10.1139/cjfas-2017-0032
Priede I. G., Merrett N. R. (1996). Estimation of abundance of abyssal demersal fishes: a comparison of data from trawls and baited cameras. J. Fish Biol. 49, 207–216. doi: 10.1111/j.1095-8649.1996.tb06077.x
Pusack T. J., Kimbro D. L., White J. W., Stallings C. D. (2019). Predation on oysters is inhibited by intense or chronically mild, low salinity events. Limnol. Oceanogr. 64, 81–92. doi: 10.1002/lno.11020
Rajagopal S., Venugopalan V. P., Nair K. V. K., Van Der Velde G., Jenner H. A. (1998). Settlement and growth of the green mussel Perna viridis (L.) in coastal waters: influence of water velocity. Aquat. Ecol. 32, 313–322. doi: 10.1023/A:1009941714978
Raubenheimer D., Zemke-White W. L., Phillips R. J., Clements K. D. (2005). Algal macronutrients and food selection by the omnivorous marine fish Girella tricuspidata. Ecology 86, 2601–2610. doi: 10.1890/04-1472
Ravit B., Cooper K., Buckley B., Comi M., Mccandlish E. (2014). Improving management support tools for reintroducing bivalve species (eastern oyster [Crassostrea virginica Gmelin]) in urban estuaries. Integr. Environ. Assess. Manage. 10, 555–565. doi: 10.1002/ieam.1553
Roberts S. M., Reeves S. E., Bossie A., Cottingham A., Jeffs A., Hillman J. R. (2023). Determining mussel restoration success: An Australasian case study. Biol. Conserv. 285, 110235. doi: 10.1016/j.biocon.2023.110235
Robinson N. M., Dexter N., Brewster R., Maple D., Macgregor C., Rose K., et al. (2020). Be nimble with threat mitigation: lessons learned from the reintroduction of an endangered species. Restor. Ecol. 28, 29–38. doi: 10.1111/rec.13028
Rueda A., Cagigal L., Antolínez J. A. A., Albuquerque J. C., Castanedo S., Coco G., et al. (2019). Marine climate variability based on weather patterns for a complicated island setting: the New Zealand case. Int. J. Climatol. 39, 1777–1786. doi: 10.1002/joc.5912
Russell B. C. (1983). The food and feeding habits of rocky reef fish of north-eastern New Zealand. New Z. J. Mar. Freshw. Res. 17, 121–145. doi: 10.1080/00288330.1983.9515991
Sazima I. (1998). Field evidence for suspension feeding in Pseudo dentex, with comments on ram filtering in other jacks (Carangidae). Environ. Biol. Fish. 53, 225–229. doi: 10.1023/A:1007492803796
Schotanus J., Capelle J. J., Paree E., Fivash G. S., Van De Koppel J., Bouma T. J. (2020). Restoring mussel beds in highly dynamic environments by lowering environmental stressors. Restor. Ecol. 28, 1124–1134. doi: 10.1111/rec.13168
Sea M. A., Hillman J. R., Thrush S. F. (2022). Enhancing multiple scales of seafloor biodiversity with mussel restoration. Sci. Rep. 12, 13. doi: /10.1038/s41598-022-09132-w
Seddon P. J., Armstrong D. P., Maloney R. F. (2007). Developing the science of reintroduction biology. Conserv. Biol. 21, 303–312. doi: 10.1111/j.1523-1739.2006.00627.x
Seddon P. J., Griffiths C. J., Soorae P. S., Armstrong D. P. (2014). Reversing defaunation: restoring species in a changing world. Science 345, 406–412. doi: 10.1126/science.1251818
Shears N. T., Babcock R. C. (2002). Marine reserves demonstrate top-down control of community structure on temperate reefs. Oecologia 132, 131–142. doi: 10.1007/s00442-002-0920-x
Shin P. K. S., Yau F. N., Chow S. H., Tai K. K., Cheung S. G. (2002). Responses of the green-lipped mussel Perna viridis (L.) to suspended solids. Mar. pollut. Bull. 45, 157–162. doi: 10.1016/S0025-326X(01)00298-3
Sievers M., Brown C. J., Buelow C. A., Hale R., Ostrowski A., Saunders M. I., et al. (2022). Greater consideration of animals will enhance coastal restoration outcomes. BioScience 72, 1088–1098. doi: 10.1093/biosci/biac088
Stadtmann S., Seddon P. J. (2018). Release site selection: reintroductions and the habitat concept. Oryx 54, 687–695. doi: 10.1017/S0030605318001199
Stadtmann S., Seddon P. J. (2020). Release site selection: reintroductions and the habitat concept. Oryx. 54 (5), 687–695.
Swan K. D., Mcpherson J. M., Seddon P. J., Moehrenschlager A. (2016). Managing marine biodiversity: the rising diversity and prevalence of marine conservation translocations. Conserv. Lett. 9, 239–251. doi: 10.1111/conl.2016.9.issue-4
Tedford K. N., Castorani M. C. N. (2022). Meta-analysis reveals controls on oyster predation. Front. Mar. Sci. 9, 1–14. doi: 10.3389/fmars.2022.1055240
Toone T. A., Hillman J. R., Benjamin E. D., Handley S., Jeffs A. (2023). Out of their depth: The successful use of cultured subtidal mussels for intertidal restoration. Conserv. Sci. Pract. 5 (4), 1–11. doi: 10.1111/csp2.12914
Towns D. R., Ballantine W. J. (1993). Conservation and restoration of New Zealand island ecosystems. Trends Ecol. Evol. 8, 452–457. doi: 10.1016/0169-5347(93)90009-E
Underwood L. H., Van Der Reis A., Jeffs A. G. (2023). Diet of snapper (Chrysophrys auratus) in green-lipped mussel farms and adjacent soft-sediment habitats. Aquacult. Fish Fish. 3, 268–286. doi: 10.1002/aff2.113
Van Heezik Y., Seddon P. J. (2018). Animal reintroductions in peopled landscapes: moving towards urban-based species restorations in New Zealand. Pacific Conserv. Biol. 24, 1–11. doi: 10.1071/PC18026
Whitlow W. L., Rice N. A., Sweeney C. (2003). Native species vulnerability to introduced predators: testing an inducible defense and refuge from predation. Biol. Invasions 5, 23–31. doi: 10.1023/A:1024059025890
Wilcox M., Jeffs A. (2019). Impacts of sea star predation on mussel bed restoration. Restor. Ecol. 27, 189–197. doi: 10.1111/rec.12831
Wilcox M., Kelly S., Jeffs A. (2018). Ecological restoration of mussel beds onto soft-sediment using transplanted adults. Restor. Ecol. 26, 581–590. doi: 10.1111/rec.12607
Yund P., Gaines S. D., Bertness M. D. (1991). Cylindrical tube traps for larval sampling. Limnol. Oceanogr. 36, 1167–1177. doi: 10.4319/lo.1991.36.6.1167
Keywords: site selection, shellfish restoration, translocation, species reintroduction, green-lipped mussel, timelapse cameras
Citation: Alder A and Hillman JR (2024) Where the wild things aren’t: exploring the utility of rapid, small-scale translocations to improve site selection for shellfish restoration. Front. Mar. Sci. 11:1354257. doi: 10.3389/fmars.2024.1354257
Received: 12 December 2023; Accepted: 20 March 2024;
Published: 23 April 2024.
Edited by:
Dorte Krause-Jensen, Aarhus University, DenmarkReviewed by:
David M. Hudson, Remote Ecologist, United StatesBenjamin Belgrad, Dauphin Island Sea Lab, United States
Copyright © 2024 Alder and Hillman. This is an open-access article distributed under the terms of the Creative Commons Attribution License (CC BY). The use, distribution or reproduction in other forums is permitted, provided the original author(s) and the copyright owner(s) are credited and that the original publication in this journal is cited, in accordance with accepted academic practice. No use, distribution or reproduction is permitted which does not comply with these terms.
*Correspondence: Al Alder, YWwuYWxkZXJAYXVja2xhbmQuYWMubno=