- 1Red Sea Research Center (RSRC), King Abdullah University of Science and Technology (KAUST), Thuwal, Saudi Arabia
- 2Department of Allied Medical Sciences, Zarqa University College, Al-Balqa Applied University (BAU), Al-Salt, Jordan
Sunlight, including ultraviolet (UVA and UVB) and photosynthetically active radiation (PAR), is vital for the physiology of invertebrates with symbiotic Symbiodiniaceae. While the effects of UVB and PAR are well-studied, the impact of UVA remains underexplored. This study investigates the effects of varying UVA and PAR intensities on the metabolic, oxidative, and photosynthetic responses of Cassiopea andromeda jellyfish. Over 18 days, 24 medusae were exposed to four light treatments: low PAR (± low UVA) and high PAR (± high UVA). Results showed significant increases in jellyfish mass and umbrella diameter, with no differences between treatment groups. PAR intensity primarily drove aerobic respiration and photosynthesis, with reduced PAR enhancing ETS activity and chlorophyll-a concentration, while UVA had less effect. ETS activity was positively correlated with chlorophyll-a concentration but negatively with jellyfish size. Both high PAR and high UVA exposure increased lipid peroxidation (LPO), with the highest levels under combined high PAR and UVA. These findings show that UVA does not directly affect photosynthesis but might enhances oxidative stress when combined with high PAR, increasing LPO. Despite oxidative stress, jellyfish showed consistent growth and normal morphology, highlighting their resilience to varying light. Color changes linked to PAR exposure indicated shifts in algal symbiont density. This study highlights the adaptive capacity of C. andromeda jellyfish to fluctuating light, emphasizing PAR’s role in regulating metabolism and oxidative stress. It also offers new insights into UVA’s underexplored impact on jellyfish physiology, paving the way for future research on UVA’s broader effects on marine invertebrates.
1 Introduction
Light is essential for the growth, survival, and physiological functioning of cnidarians that host symbiotic Symbiodiniaceae (Muscatine and Cernichiari, 1969; Osinga et al., 2011; Stambler and Dubinsky, 2004; Main and Goodbody-Gringley, 2010). Solar radiation includes ultraviolet radiation (UVR) and photosynthetically active radiation (PAR). Notably, UVR accounts for approximately 6% of the total solar radiation reaching the sea surface; however, this percentage varies depending on factors such as time and location (Moan, 2001). Physiologically active incident solar UVR is classified into two categories based on wavelength: ultraviolet B (UVB, 280–315 nm) and ultraviolet A (UVA, 315–400 nm). UVA and UVB differ significantly in terms of biological activity and physical properties (e.g., energy content, abundance in solar UVR, and water penetration capacity). UVA accounts for approximately 95% of the total incident UVR, whereas UVB accounts for approximately 5% of it (Moan, 2001). However, UVA has a significantly higher ability to penetrate seawater than UVB. Biologically, UVB can induce the formation of reactive oxygen species (ROS) within cells, leading to cytotoxic DNA lesions that compromise genomic stability and disrupt cellular homeostasis (Lesser, 1996; Lesser and Farrell, 2004; Lu and Wu, 2005; Rastogi et al., 2010).UVA, in contrast, is well known for activating the photoreactivating enzymes (PRE) to repair UVR-induced DNA damage (Hearst, 1995; Sancar, 1996; Rastogi et al., 2010), while also having the potential to adversely affect cellular functions through ROS formation (Lu and Wu, 2005; Rijstenbil, 2001). For instance, sea urchins exposed to UVA radiation for brief period (30 min) showed increased generation of ROS, which led to sperm damage and impaired fertilization (Lu and Wu, 2005). UVA also increased lipid peroxidation (LPO) and superoxide dismutase activity in Ditylum brightwellii (Rijstenbil, 2001). Furthermore, it triggered photoaging via the DAF-16 pathway in Caenorhabditis elegans (Prasanth et al., 2016). While several studies have focused on UVB (± UVA), only a few have investigated the biological effects of UVA. For example, UVB at intensities similar to those encountered at depths shallower than 5 m in the Red Sea caused DNA damage and photoinhibition in Stylophora pistillata (Winters et al., 2003; Baruch et al., 2005). Furthermore, it reduced symbiotic dinoflagellate photosynthesis (thus reducing holobiont fecundity) and caused oxidative stress-mediated cell death in shallow water corals (Lesser, 1996; Lesser and Farrell, 2004; Torres-Pérez and Armstrong, 2012). In general, UVR exerts dose-dependent biological effects on exposed species, such as Atlantic cod ‘Gadus morhua’ and red seabream ‘Pagrus major’ (Alves and Agustí, 2020).
In nature, several species have evolved a plethora of defense mechanisms to counteract the harmful effects of UVR. For example, some species evade the harmful effects of UVR by producing or acquiring UVR-absorbing pigments (e.g., carotenoid and mycosporine-like amino acids [MAAs]), which confer photoprotection against the damaging effects of UVR (Roy, 2000; Rossbach et al., 2020). Furthermore, many species have evolved DNA repair machinery (e.g., base excision repair and photolyases [photoenzymes]; Sinha and Häder, 2002; Rastogi et al., 2010). Photoenzymes use chromophores and a redox-active coenzyme (flavin adenine dinucleotide). They bind to damaged DNA and repair it with energy from near UVA and blue light (350–450 nm). Photoenzymes utilize this energy to split cyclobutane pyrimidine dimers, thereby restoring the DNA to its native state (Hearst, 1995; Sancar, 1996).
The zooxanthellate jellyfish (genus Cassiopea) is a mixotrophic epibenthic cnidarian similar to corals. Unlike most scyphozoans, Cassiopea forms a strong endo-symbiotic interaction with dinoflagellates belonging to the family Symbiodiniaceae (LaJeunesse et al., 2018). In nature, few jellyfish species [e.g., Linuche unguiculata and Mastigias sp.; Kremer et al., 1990; McCloskey et al., 1994] form such an association. Endosymbiont photosynthesis might provide the all of the energy required by jellyfish under ideal light conditions (Cates, 1975; Verde and McCloskey, 1998). To maximize photosynthesis, jellyfish need sufficient amounts of PAR. Dinoflagellate density (i.e., inside oral arm and bell tissue) might be high (i.e., up to 2.68 × 103 algae per mg protein; Verde and McCloskey, 1998). Consequently, jellyfish maximize their exposure to light by attaching themselves to surfaces in a unique upside-down orientation (i.e., with the help of mucus and continual umbrella contraction; Gohar and Eisawy, 1960). Zooxanthellate jellyfish are frequently found in a range of marine environments, including coral reefs and seagrass habitats (Fleck and Fitt, 1999; Arai, 2001; Todd et al., 2006; Niggl and Wild, 2009; Jantzen et al., 2010). However, they exhibit a preference for specific environments, primarily favoring calm, shallow lagoons and mangrove coastal areas. In addition to photosynthesis, endosymbiotic dinoflagellates produce UVR-absorbing MAAs, which provide jellyfish with photoprotection against UVR-induced damage (Banaszak and Trench, 1995). Ecologically, Cassiopea is crucial to the coral reef’s food web and nutrient cycling (Jantzen et al., 2010; Niggl et al., 2010; Zarnoch et al., 2020). Additionally, in recent years, there has been a growing trend of cultivating these jellyfish as a food source for medusivores (Pierce, 2005).
The Red Sea region is well known for its high levels of solar radiation and UVR (Khogali and Albar, 1992). This sea is characterized by its oligotrophic nature and low concentration of chromophoric dissolved organic matter (i.e., organic molecules in water that absorb light, derived from decaying organic materials, influencing optical properties in aquatic environments). Consequently, PAR and UVR can deeply penetrate the water (Kheireddine et al., 2017; Overmans and Agustí, 2019, 2020). Therefore, the shallow water inhabitants of this unique ecosystem are frequently overexposed to excessive PAR and UVR dosages (Overmans and Agustí, 2019).
Cassiopea demonstrates significant resilience to various environmental factors like elevated temperatures, pollution and UVB exposure (Aljbour et al., 2017, 2018, 2019, 2023). However, few studies have examined the cellular and physiological effects of UVA on this jellyfish, with only one detailed investigation to date (Aljbour et al., 2023). Where the authors studied UVA in combination with UVB and not UVA alone. They concluded that UVA had no effect on jellyfish physiology or development following their exposure to UVB. They also discovered that UVB (± UVA) caused greater cellular respiration in jellyfish. Klein et al. (2016) found that UVB has a deleterious impact on the health and survival of Cassiopea polyps. According to Banaszak and Trench (1995), UVR exposure increased MAA synthesis in symbiotic Cassiopea. However, previous research did not provide clear information on the biological significance of UVA (Banaszak and Trench, 1995; Klein et al., 2016; Aljbour et al., 2023). Therefore, in the present study, we analyzed the impact of varying PAR and UVA levels on the metabolism, oxidative stress responses, and physiological performance of jellyfish. We investigated the influence of UVA on jellyfish responses, including survival, growth, aerobic respiration, chlorophyll-a content, and LPO, while considering the varying effects of high and low PAR intensities. In addition, we explored the role of PAR intensity as an independent factor affecting jellyfish performance. We conducted experiments by exposing jellyfish to four distinct light conditions: low PAR (LP), low PAR + UVA (LPLA), high PAR (HP), and high PAR + UVA (HPHA). This is the first comprehensive study to investigate the differential roles of UVA in mediating cellular responses of Cassiopea sp. under varying light conditions. The findings of this study are expected to provide valuable insights into the biology and ecology of this jellyfish, shedding light on its responses to environmental variations and potential implications for marine ecosystems.
2 Materials and methods
2.1 Jellyfish sampling and maintenance
By carefully scooping, we collected Cassiopea andromeda jellyfish medusae (Figure 1) from shallow waters of King Abdullah University of Science and Technology (KAUST) in the central Red Sea. The jellyfish were quickly transferred to the Coastal and Marine Resources Core Lab (CMR) at KAUST using large iceboxes, a process that took less than half an hour. In CMR, the collected medusae were maintained for 2 months in a temperature-controlled (27.5 ± 0.7°C) aquarium tank (300 L volume capacity) supplied with flow-through filtered seawater (FSW, 20 μm, salinity 40.5 ± 0.5‰) until the experiment started. The aquarium was illuminated with PAR using Radion LEDs (EcoTech Marine, Allentown, PA, USA) on a 12 h/12 h light/dark cycle. The PAR intensity varied from 500 μmol·m−2·s−1 at the water surface to 171 μmol·m−2·s−1 at the bottom of the 0.40 m deep aquarium (Figure 2).
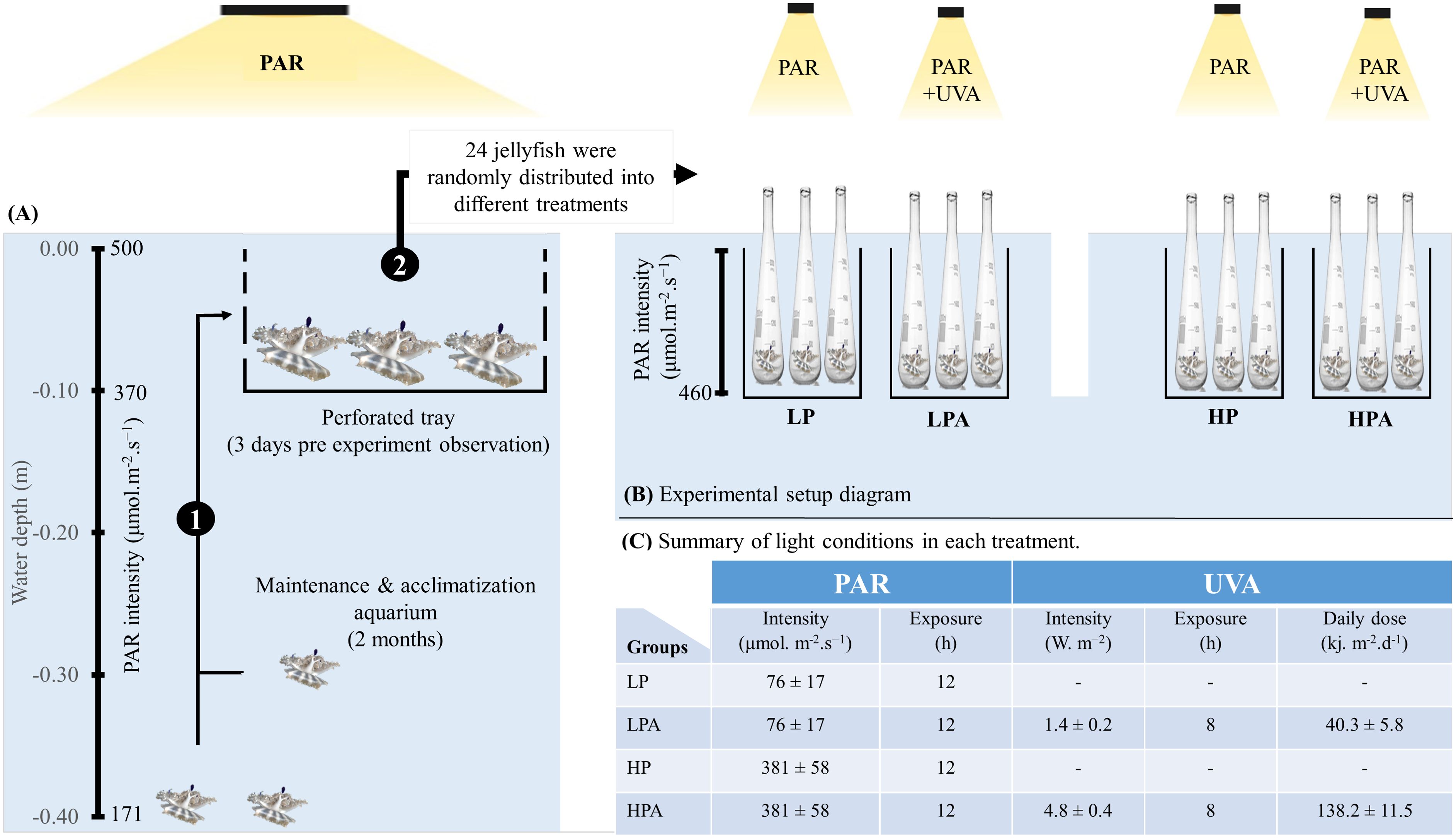
Figure 2. Schematic Representation of jellyfish rearing and experimental setup (A, B). (1) Jellyfish were placed in a perforated tray (at a depth of 0.10 m for 3 days) within the rearing tank to allow for closer observation prior to the start of the experiment. (2) Jellyfish were randomly distributed into four treatments: LP: low PAR (−UVA), LPLA: low PAR (+UVA), HP: high PAR (−UVA), and HPHA: high PAR (+UVA). Each organism served as a biological replicate and was placed in an individual incubation quartz flask. (C) Summarizes the values of photosynthetically active radiation (PAR) and ultraviolet radiation type A (UVA) light exposure conditions for each treatment. For more detailed explanations, please refer to the materials and methods section. This figure was adopted from Aljbour et al., 2023.
2.2 Experimental design, PAR and UVA exposure
Before the experiment started, all medusae were kept for 3 days in a perforated tray submerged at a depth of 0.10 m in the same maintenance aquarium (i.e., exposed to PAR at roughly 370 μmol·m-2·s-1; Figure 2). The primary objective of these three days was to separate the selected medusae from the rest of the aquarium, enabling closer monitoring of them. We carefully selected these jellyfish using the criteria developed by Aljbour et al. (2017, 2019). These criteria verified that the jellyfish were morphologically healthy, with no missing oral arms, broken umbrellas, or perforated bells.
To investigate the effects of varying UVA and PAR intensities on the metabolic, oxidative, and photosynthetic responses of Cassiopea andromeda jellyfish, 24 medusae (avg. wet body mass = 2.0 g, range 1.3–3.1 g; avg. bell diameter = 3.5 cm, range 3.1–4.1 cm) were randomly assigned to four treatment groups (N = 6/group). Namely, LP (PAR = 76 ± 17 μmol·m−2·s−1), LPLA (PAR = 76 ± 17 μmol·m−2·s−1 and UVA = 1.4 ± 0.2 W·m−2), HP (PAR = 381 ± 58 μmol·m−2·s−1), and HPHA (PAR = 381 ± 58 μmol·m−2·s−1 and UVA = 4.8 ± 0.4 W·m−2). Radion LEDs were used for PAR light, whereas UVA was supplied using F71T12 UVA 100W lamp (peak emission at 350nm). In all treatment groups, PAR lights were turned on at 7:00 AM and off at 7:00 PM, whereas UVA lamps were turned on at 9:00 AM and off at 5:00 PM. The experiment lasted 17 days, not including the sampling day (day 18).
Jellyfish medusae were individually incubated in separate 2.0-L wide-mouth (7 cm in diameter) quartz flasks. During the course of the experiment, all physical parameters were held tightly controlled (i.e., temperature = 27.7°C ± 0.5°C) and salinity = 40.5‰ ± 0.5‰). Jellyfish were fed (to repletion) with freshly hatched Artemia nauplii every second day, with feeding occurring between 7.00–8.00 AM. We performed 90% water exchange (i.e., we removed 90% of the incubation water and replaced it with temperature-controlled FSW) roughly 24 hours post feeding. The initial values of mass and bell diameter of the jellyfish were measured 1 day before the experiment (day 0) began and at day 16 (i.e., 1 day before sampling) using the procedure of Aljbour et al. (2017, 2019). Briefly, Jellyfish bell diameters were measured with scaled glass beakers at full relaxation. After careful hand removal, excess water was shaken off and absorbed with tissue without direct contact. The jellyfish were weighed within 30 seconds in a beaker of seawater (from the incubation aquarium) to the nearest 0.1 g. Notably, jellyfish were not fed for at least 24 h before their size was measured.
2.3 Tissue sampling and homogenization
On day 18 (UVA turned off), we collected two oral arms per tube from the medusae according to the methodology described by Aljbour et al. (2017). These samples were immediately frozen in liquid nitrogen and stored at −80°C until analysis. Oral samples designated for electron transport system (ETS) analysis and spectroscopy were homogenized using specialized homogenization buffers and a motorized glass homogenizer. Further processing steps were then tailored to the specific requirements of each analysis type. By contrast, for LPO analysis, the oral arms were freeze-dried before analysis.
2.4 ETS activity
We measured ETS activity in oral arm homogenates using the typical iodonitrotetrazolium (INT) reduction assay (Packard, 1971; Owens and King, 1975). We followed the detailed technique outlined by Aljbour et al. (2019). In this assay, ETS enzymes in the reaction mixture (which contains electron donors) facilitate the reduction of INT to INT formazan. We applied the hypothesized stoichiometric relationship, where 2.0 µmol of INT formazan corresponds to 1.0 µmol of molecular oxygen consumption. The changes in absorbance caused by the formation of INT formazan were continuously monitored at 490 nm using a temperature-controlled microplate spectrometer (SpectraMax, San Jose, CA, USA) set at 28°C. We used the corrected slopes in our calculations, and the results were expressed as micrograms of oxygen per hour per gram of wet mass (µg O2·h−1·g−1 WM) and micrograms of oxygen per hour per milligram of protein (µg O2·h−1·mg−1 protein). Protein concentrations were determined using the Bradford (1976) method.
2.5 LPO assay
We quantified malondialdehyde (MDA) content using a calorimetric LPO assay kit (CAT NO: MAK085, Sigma-Aldrich, St. Louis, MO, USA). This kit primarily relies on the thiobarbituric acid-reactive substances protocol developed by Uchiyama and Mihara (1978). Using an Eppendorf Mixmate Microplate Shaker, we subjected the freeze-dried oral arm tissues to two 10-min runs at 2000 rpm, thoroughly homogenizing them in ice-cold MDA lysis buffer. Then, we followed the precise protocols outlined in the test kit. The absorbance was measured at 532 nm using a microplate spectrometer (SpectraMax). The results were provided in ng MDA per mg dry mass (ng MDA·mg−1 DM).
2.6 Chlorophyll-a, MAA extraction, and absorbance spectra
In brief, using a glass homogenizer, the oral arms were homogenized in three volumes of 0.2 FSW. The homogenate was then centrifuged (3000 × g for 5 min at 4°C), and the pellets were resuspended in 1.0 mL of methanol (100% HPLC grade), sonicated for 15 min, and kept in darkness at 4°C for 24 h. The supernatant was centrifuged again and then transferred to a quartz cuvette. The absorbance spectra wavelengths between 280 and 700 nm were immediately read using a UV/Vis/NIR spectrophotometer (Lambda 1050, PerkinElmer, Waltham, MA, USA). As a reference, we used a quartz cuvette with ethanol. [Chlorophyll-a] was determined using the following equation: μg Chl-a· ml−1 = 16.29A666.2 − 8.54A652.0 (Porra et al., 1989). The results were reported in μg Chl-a· g−1 WM. In the UV band, we explored the absorbance spectra to identify the absorption peaks of photoprotective MAAs (Banaszak et al., 2006).
2.7 Confirmation of the Cassiopea jellyfish species identity
2.7.1 DNA extraction, COI and 16S amplification and sequencing
DNA was extracted from six specimens’ oral arms using the DNeasy® Blood and Tissue Kit (Qiagen), following the manufacturer’s protocol. The 16S ribosomal RNA (16S) and mitochondrial cytochrome c oxidase subunit I (COI) were amplified using the primers described in Muffett and Miglietta (2023): COI (FishF1_fwd 5’-TCAACCAACCACAAAGACATTGGCAC-3′; med-cox1-rev 5′-TGGTGNGCYCANACNATRAANCC-3’); 16S (med-rn1-fwd 5’-GACTGTTTACCAAAGACATAGC-3’; med-rn1-rev 5’-AAGATAGAAACCTTCCTGTC-3’). PCR products were obtained using the Platinum™ SuperFi II PCR 2 × Master Mix with the following thermal cycling conditions: initial denaturation at 98°C for 30 s, followed by 35 amplification cycles (98°C for 19 s, 58°C for 10 s, 72°C for 20 s) and a final extension at 72°C for 5 min. PCR products were visualized by gel electrophoresis and further purified with 1.5x AMPure XP Beads (Beckman Coulter, Germany). Purified products were sequenced bidirectionally using the ABI BigDye® Terminator v3.1 Cycle Sequencing Kit on a 3730xl Genetic Analyzer at KAUST Bioscience Core Lab. Ab1 trace files were manually checked, trimmed and consensus sequences from each amplicon were generated with Geneious prime 2024.0.2 for further phylogenetic analyses.
2.7.2 Phylogenetic analysis
Alignments were performed using the E–INS–i option in MAFFT 7.130b (Katoh and Standley, 2013) and manually checked using BioEdit 7.2.5 (Hall, 1999). Sequence datasets from each locus were analyzed individually. The final 16S and COI datasets were composed by 25 and 93 sequences, respectively. Maximum likelihood (ML) phylogenetic reconstructions were obtained using RAxML v.8 (Stamatakis, 2014) on the CIPRES server (Miller, Pfeiffer, & Schwartz). The GTR+GAMMA substitution model was applied and the branch support was assessed by 500 rapid bootstrap replicates. The obtained phylogenetic trees were visualized using the ‘iTol Interactive Tree of Life’ online platform. Notably, all sequences (excluding our own) and the species names used in this analysis were extracted and assigned based on Muffett and Miglietta (2023). All sequences are under submission to NCBI.
2.8 Statistical analysis
We examined data for adherence with statistical test assumptions (such as normality and homoscedasticity) prior to performing each statistical test. Shapiro–Wilk test was used to determine data normality, and Bartlett test was used to determine variance homogeneity among groups. Within each experimental group, we applied paired t-test to analyze differences between the initial and final average measurements of mass and diameter. This allowed us to track the growth of the jellyfish in the groups over the course of the experiment. The effect of varying PAR and UVA intensity on different response parameters were examined using one-way analysis of variance (ANOVA). In cases where ANOVA findings were significant, post-hoc testing (multiple comparison tests) was performed. These post-hoc tests helped in accurately identifying the specific groups that exhibited significant differences. Notably, we excluded a single outlier from the LPLA group in the ETS activity dataset for all tests related to ETS activity. To analyze the correlation between variables, we applied Pearson’s moment correlation analysis. R software (R Foundation for Statistical Computing, Vienna, Austria) was used for statistical analysis. A significance threshold of p <.05 was used to determine statistical significance and reject the null hypothesis in each test.
3 Results
3.1 Jellyfish growth and total protein content
Wet body mass and bell diameter of jellyfish increased significantly over time in all treatment groups (Welch two-sample t-test: all p-values <.05; Figures 3A, B), with no signs of abnormal behavior or morphological issues. The magnitude of growth (gain) in mass and bell diameter, however, was similar across all treatment groups (One-Way ANOVA: mass F(3, 20) = 1.81, p = .177; bell F(3, 20) = 1.53, p = .237; Figures 4A, B). Similarly, total protein content did not differ significantly among treatments groups (One-Way ANOVA: F(3, 20) = .857, p = .48; Figure 4C). Additionally, mass and bell diameter were strongly positively correlated (Pearson’s r(22) = .93138, p <.0001; Figure 5A).
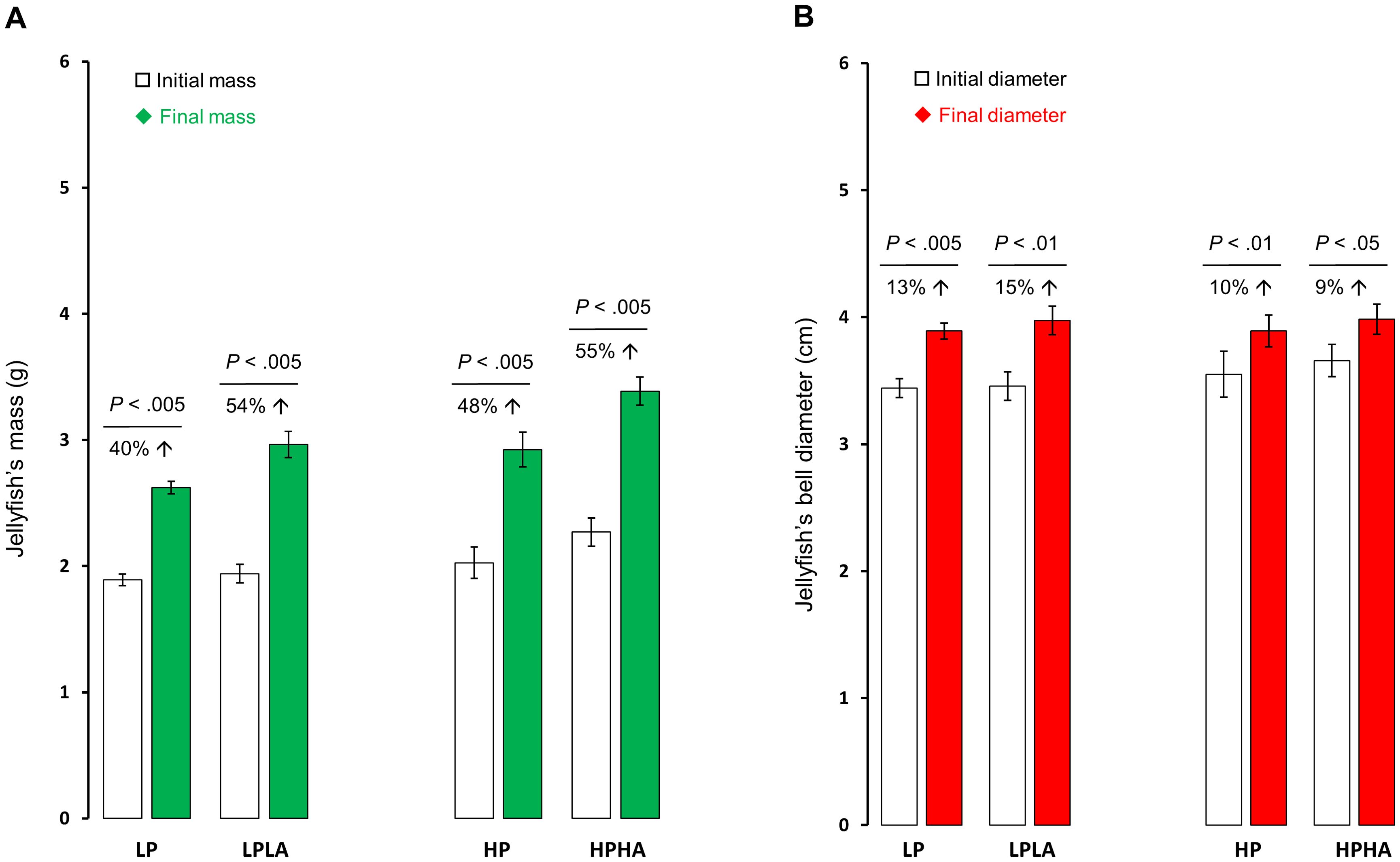
Figure 3. Medusae mass and bell diameter changes pre and post exposure to PAR and UVA. Panel (A) displays alterations in the average mass in grams, whereas (B) illustrates changes in the average bell diameter in centimeters. We conducted paired t-tests to analyze the data. The percent increase and the corresponding p-value from the t-test are indicated above the bars. The bars in the graph depict the mean, and the error bars represent the standard error of the mean. Each group had a sample size of 6. LP: low PAR (−UVA), LPLA: low PAR (+UVA), HP: high PAR (−UVA), and HPHA: high PAR (+UVA).
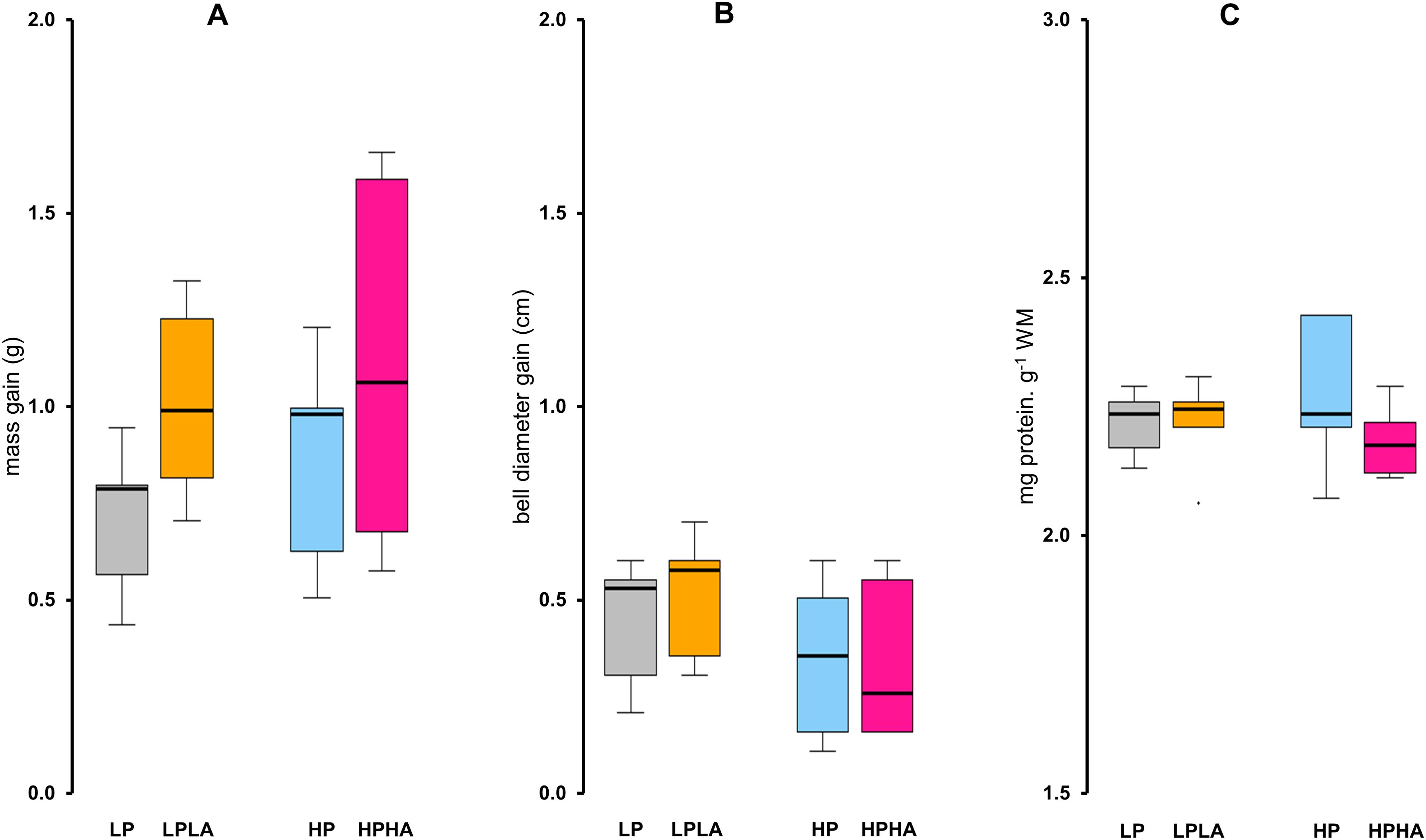
Figure 4. Effects of varying PAR and UVA intensities on the mass, bell diameter, and total protein content of Cassiopea medusae. (A) displays the increase in mass in grams, and (B) illustrates the increase in bell diameter in centimeters. The increase in these parameters was determined by subtracting the initial values from the final values. (C) displays the total protein content. Each group had a sample size of 6. One-Way ANOVA and Tukey’s multiple comparison tests revealed no significant differences among any of the groups. The box represents the interquartile range (IQR), with a line at the median. Whiskers extend to 1.5 times the IQR, and points outside this range are considered outliers. LP: low PAR (−UVA), LPLA: low PAR (+UVA), HP: high PAR (−UVA), and HPHA: high PAR (+UVA).
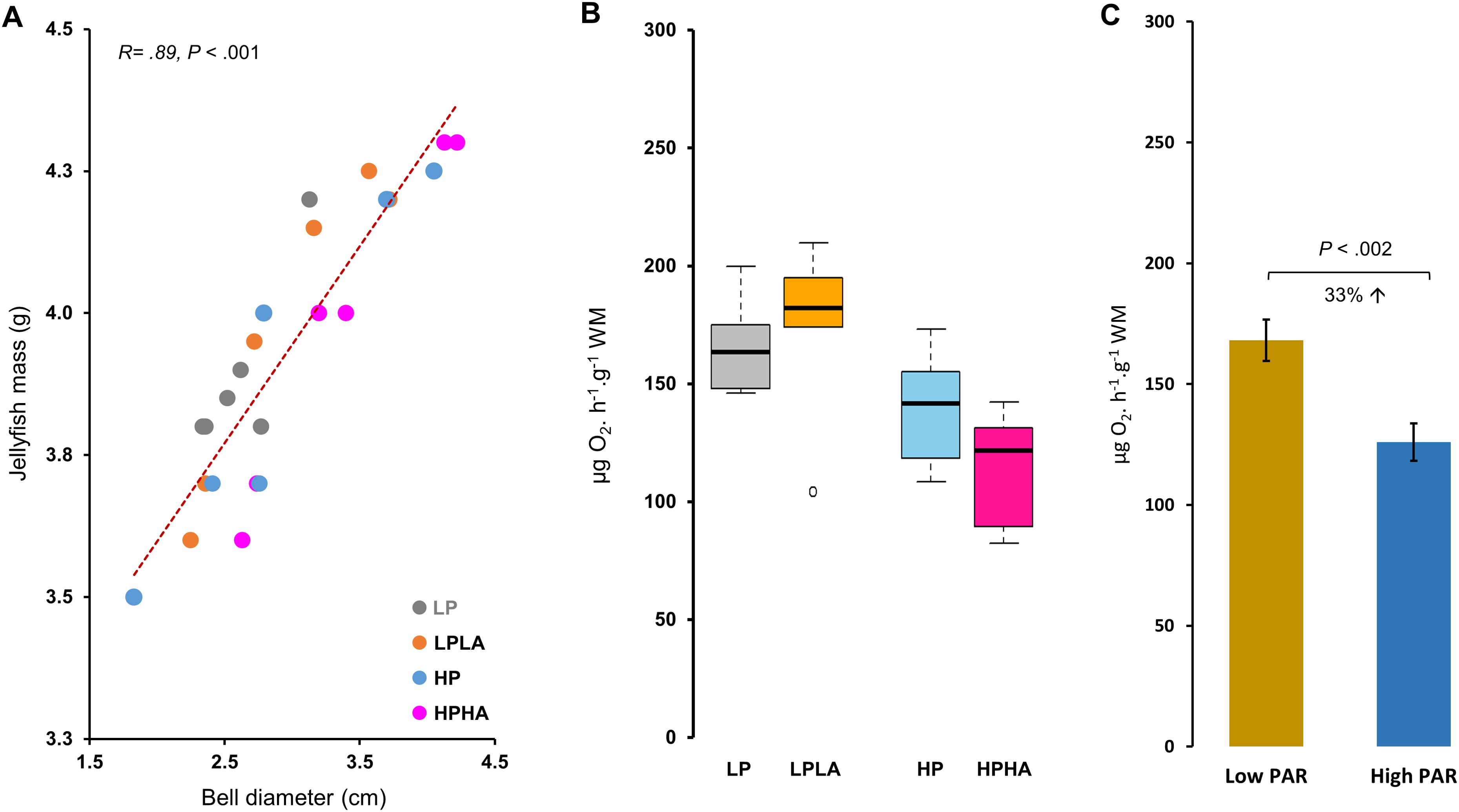
Figure 5. Correlation between the mass and bell diameter of jellyfish and cellular respiration in Cassiopea medusae under PAR and UVA. In (A), the correlation between the final wet mass and bell diameter was analyzed using Pearson’s correlation test (N = 24). (B) presents ETS activity, a proxy for cellular respiration, with different letters above the boxes indicating statistical significance as determined by One-Way ANOVA and Tukey’s multiple comparison test. Each group had 6 jellyfish, except LPLA with 5 after removing an outlier. (C) depicts ETS activity in the low and high PAR groups. LP: low PAR (−UVA), LPLA: low PAR (+UVA), HP: high PAR (−UVA), and HPHA: high PAR (+UVA).
3.2 ETS activity (aerobic cellular respiration)
ETS activity differed significantly among the groups (One-Way ANOVA: F(3, 19) = 5.28, p = .008; Figure 5B). Tukey’s HSD post hoc tests showed that ETS activity was significantly higher in LP (by 46%, p = .022) in LPLA (52%, p = .012) compared to HPHA. No other comparisons were significant (p >.05). Overall, ETS activity was 33% higher in the low PAR treatment (LP and LPLA combined) than in the high PAR treatment (HP and HPHA combined) (Welch two-sample t-test: t(20.23) = 3.57, p = .002; Figure 5C). Pearson’s correlation analysis revealed a significant negative correlation between ETS activity and jellyfish mass (r(21) = −0.470, p <.05; Figure 6).
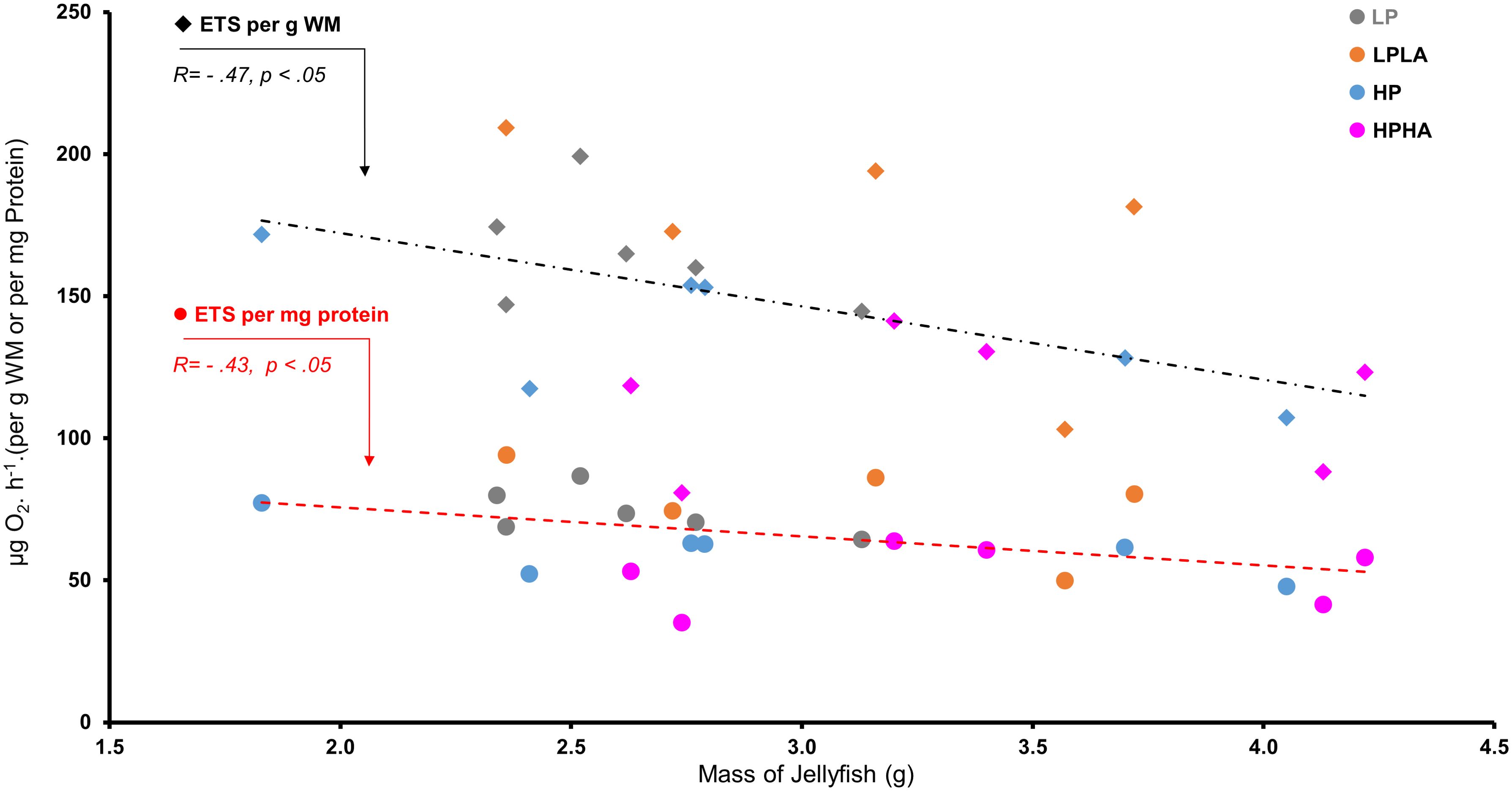
Figure 6. Correlation between mass and ETS activity in Cassiopea medusae under PAR and UVA. The correlation was analyzed using Pearson’s correlation test (N = 23). To ensure unbiased findings, an outlier was removed from the ETS activity dataset, and its corresponding value was eliminated from the jellyfish mass dataset. LP: low PAR (−UVA), LPLA: low PAR (+UVA), HP: high PAR (−UVA), and HPHA: high PAR (+UVA).
3.3 [Chlorophyll-a]
One-Way ANOVA and Tukey’s multiple comparison test found no significant differences in chlorophyll-a concentrations between the groups. Nevertheless, chlorophyll-a concentration was 52% higher in jellyfish exposed to low PAR (LP and LPLA combined) compared to those exposed to high PAR (HP and HPHA; Welch two-sample t-test, t(22) = 2.98, p<.01). Additionally, Pearson’s correlation analysis revealed a significant positive correlation between chlorophyll-a concentration and ETS activity (r(21) = 0.59, p <.01; Figure 7B).
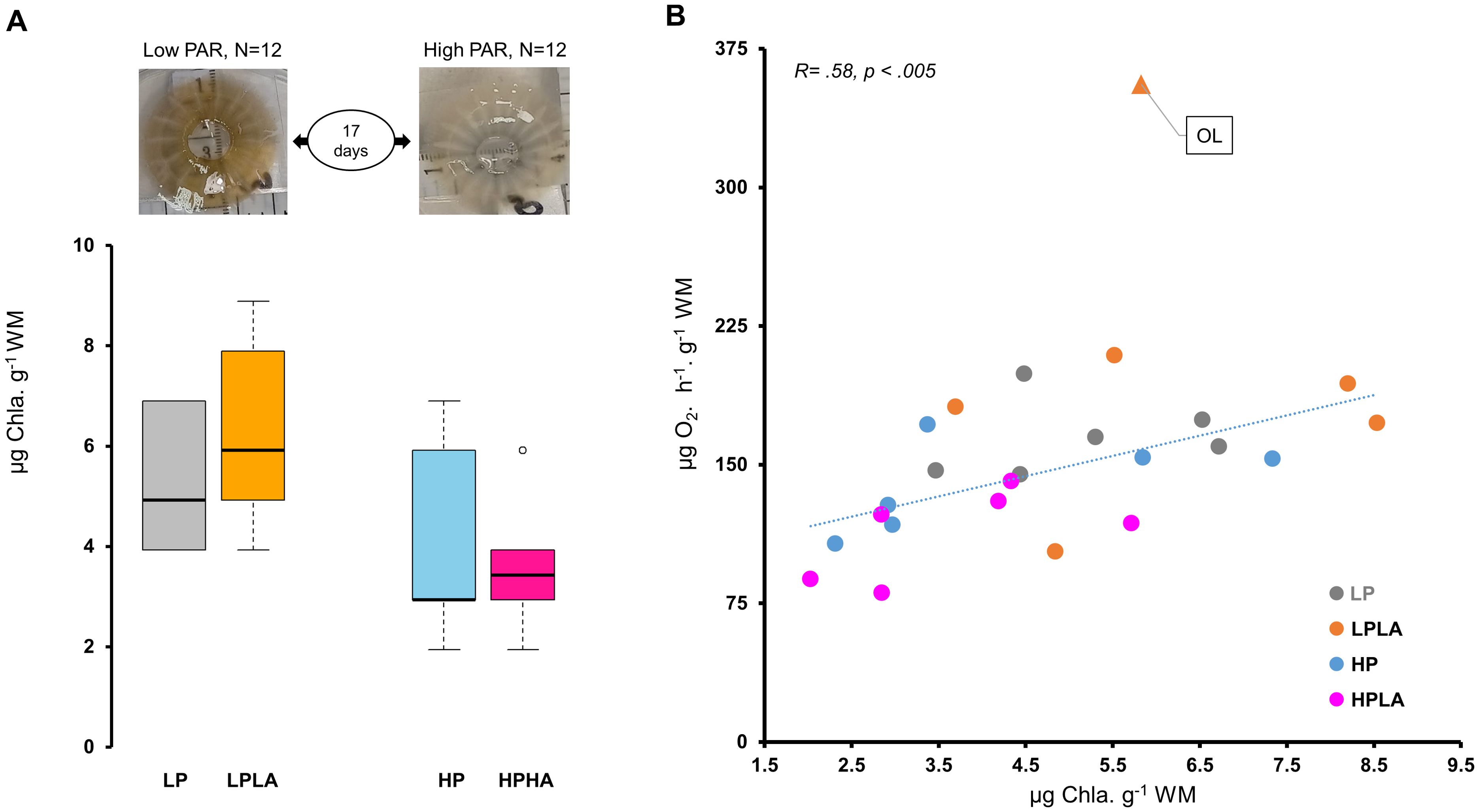
Figure 7. Chlorophyll-a concentration and its correlation with ETS activity in Cassiopea medusae under varying PAR and UVA conditions. (A) shows the effect of varying PAR and UVA intensities on the color and chlorophyll-a concentration in Cassiopea bells across different treatment groups. One-Way ANOVA and Tukey’s multiple comparison test found no significant differences in chlorophyll-a concentrations between the groups. (B) presents correlation between chlorophyll-a concentration and ETS activity as analyzed using Pearson’s correlation test (N = 23). To ensure unbiased findings, an outlier was removed from the ETS activity data, and its corresponding value was eliminated from the chlorophyll-a dataset during the test. However, the data point is depicted on the plot and indicated by “OL” and a triangle symbol (▲). LP: low PAR (−UVA), LPLA: low PAR (+UVA), HP: high PAR (−UVA), and HPHA: high PAR (+UVA).
3.4 MDA concentration (lipid peroxidation)
MDA content differed significantly among the groups (One-Way ANOVA (F(3, 20) = 41.77, p <.001; Figure 8A). Tukey’s HSD post hoc tests revealed significant differences: MDA was 20% higher in HPHA than HP (p <.05), 53% higher in HP than LP (p < 0.001), 55% higher in HP than LPLA (p < 0.001), 86% higher in HPHA than LPLA (p < 0.001), and 19% higher in HPHA than HP (p <.001). The comparison between LPLA and LP was not significant (p = .998). Overall, MDA was 69% higher in jellyfish exposed to high PAR (HP and HPHA) compared to those exposed to low PAR (LP and LPLA; Welch two-sample t-test: t(18.251) = -9.0216, p <.001; Figure 8B).
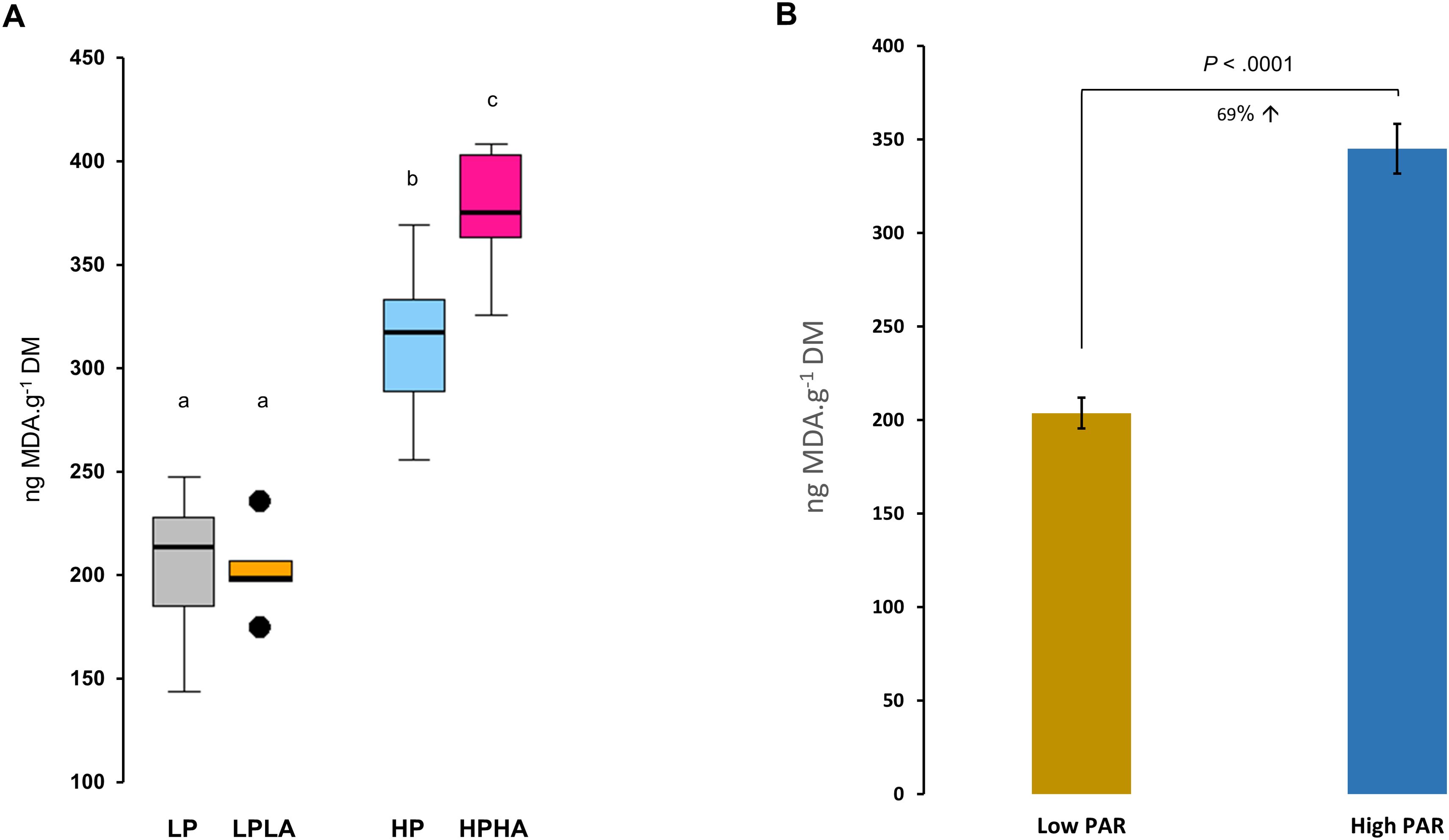
Figure 8. Effects of varying PAR and UVA intensities on lipid peroxidation (LPO) in Cassiopea medusae. (A) presents the MDA concentration, a proxy of LPO, with different letters above the boxes indicating statistical significance as determined by One-Way ANOVA and Tukey’s multiple comparison test. (B) depicts the MDA concentrations in the low PAR (LP and LPLA combined) vs high PAR (HP and HPHA combined) groups. Error bars extend over one standard error (± SE). p-value above bars indicates the statistical significance of Welch two-sample t-test. LP: low PAR (−UVA), LPLA: low PAR (+UVA), HP: high PAR (−UVA), and HPHA: high PAR (+UVA).
3.5 Optical properties of jellyfish (UV–Vis absorbance spectrum)
Jellyfish exposed to low PAR (i.e., LP and LPLA) appeared darker in color compared to those exposed to high PAR (i.e., HP and HPHA), which appeared brighter (Figure 7A). All groups showed similar absorbance spectra in the 250–400 nm wavelength range (Figure 9). However, the magnitudes of absorption were not identical across the experimental groups. Jellyfish samples exhibited two absorption peaks in the UVR region: one in the 330–341 nm range, corresponding to MAA absorption peaks, and another in the 267–272 nm range, within the ultraviolet C region.
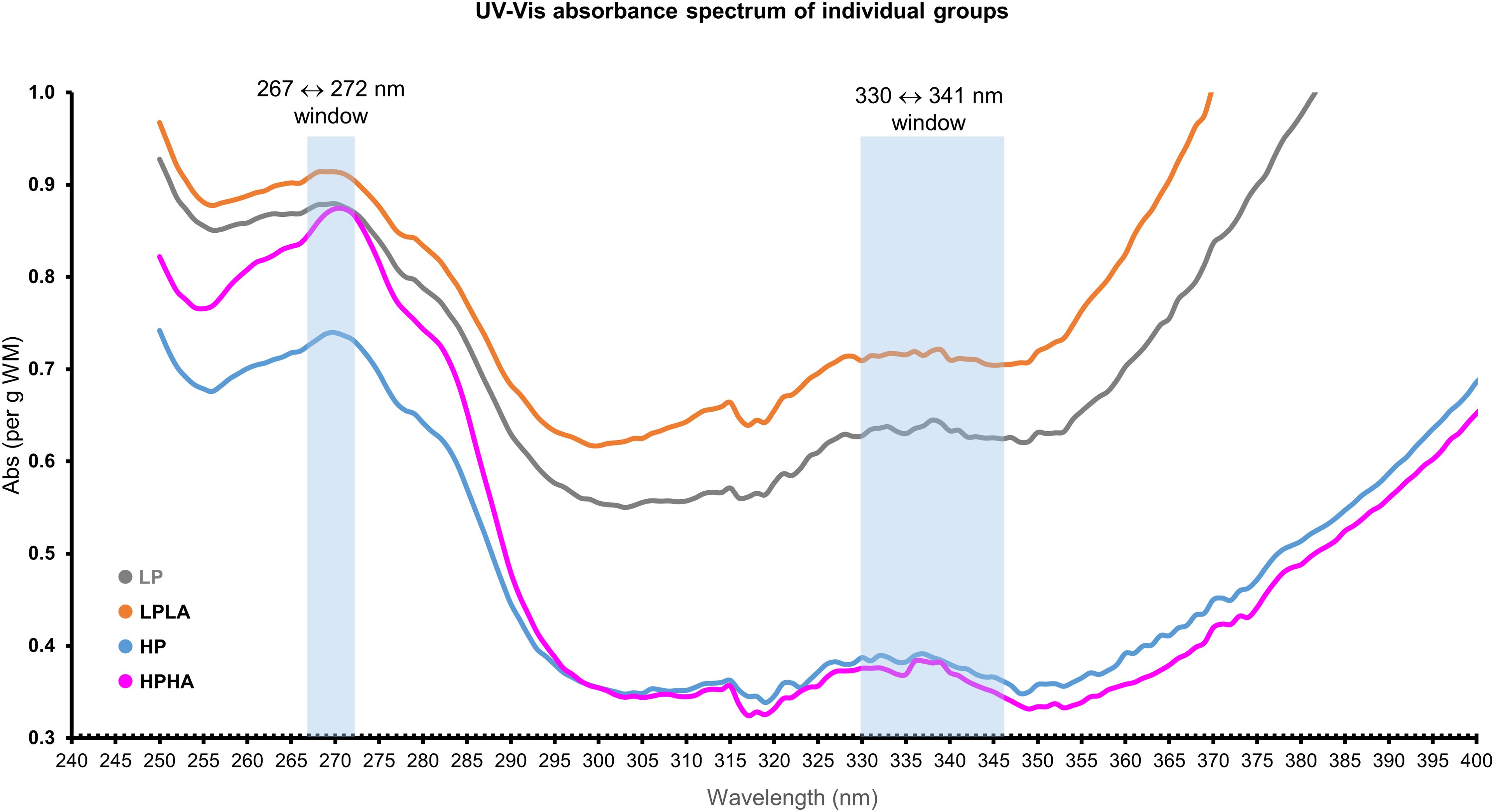
Figure 9. The absorbance spectra of Cassiopea oral arms in methanol represent the average values for each treatment group, standardized to the mass of the homogenized tissue before plotting.
3.6 Confirmation of the Cassiopea jellyfish species identity
The results of the COI and 16S phylogenetic tree analysis confirmed that the species used in this experiment is Cassiopea andromeda (Figures 10, 11).
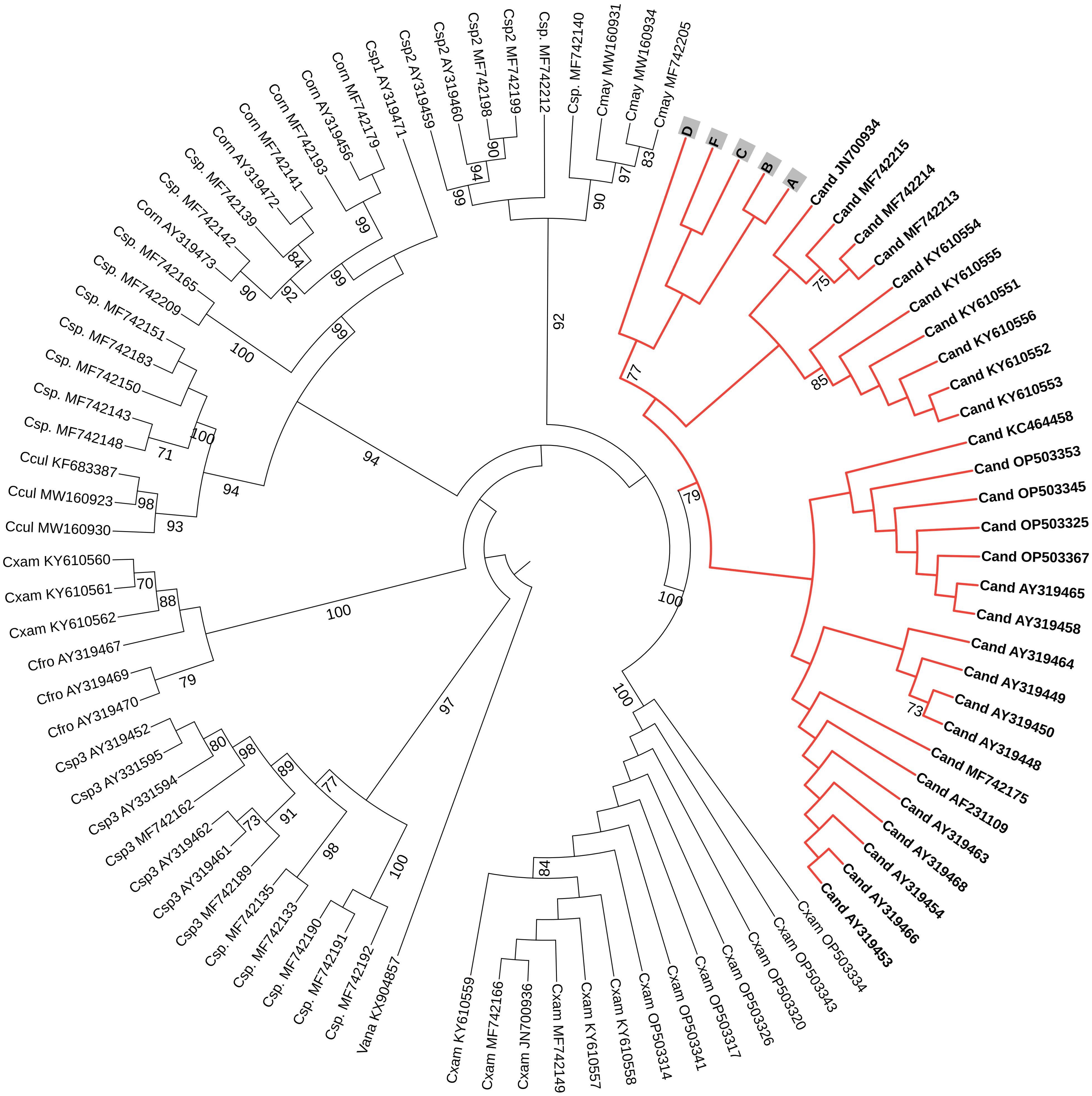
Figure 10. A circular phylogenetic tree, based on COI sequences, depicts the relationships among major Cassiopea jellyfish species. Bootstrap values < 70 were not shown to avoid bulkiness of the tree. At the tip of each branch, the names (abbreviated with the first letter of the genus and the first three letters of the species) and accession numbers are displayed. Cand: Cassiopea andromeda, Cxam: Cassiopea xamachana, Cmay: Cassiopea mayeri, Ccul: Cassiopea culionensis, Cfro: Cassiopea frondosa, Corn: Cassiopea ornata, Csp.: Cassiopea sp., Csp1: Cassiopea sp. 1, Csp2: Cassiopea sp. 2, Csp3: Cassiopea sp. 3, and Vana stands for Versurga anadyomene (an outgroup). The letters A, B, C, D, and F represent the jellyfish from this current experiment. Species names were assigned according to Muffett and Miglietta (2023).
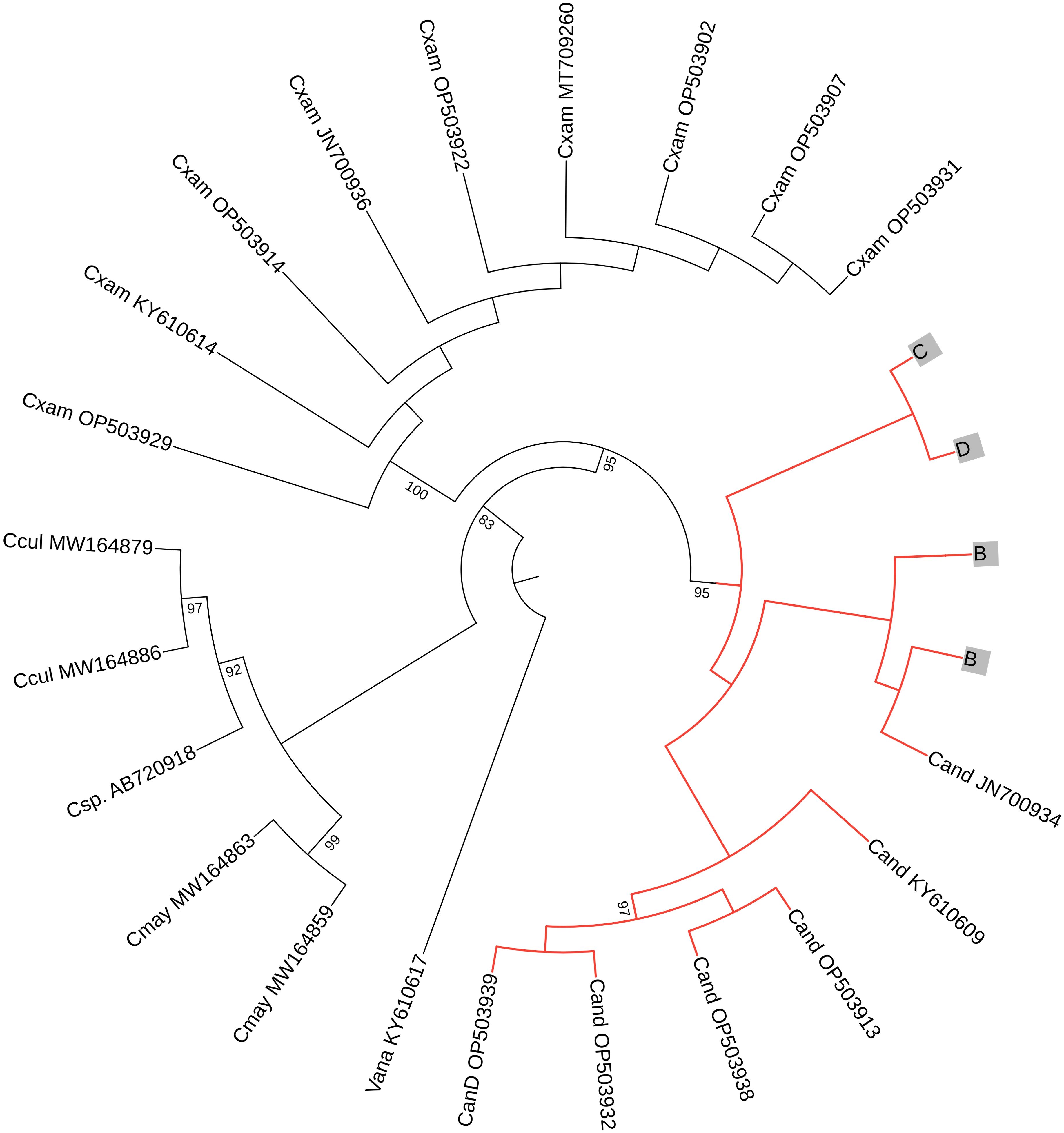
Figure 11. A circular phylogenetic tree, based on 16S sequences, depicts the relationships among major Cassiopea jellyfish species. Bootstrap values < 70 were not shown to avoid bulkiness of the tree. At the tip of each branch, the names (abbreviated with the first letter of the genus and the first three letters of the species) and accession numbers are displayed. Cand: C. andromeda, Cxam: C. xamachana, Cmay: C.mayeri, Ccul: C. culionensis, and the Vana stands for Versurga anadyomene (an out group). The letters (B–E) represent the jellyfish from this current experiment. Species names were assigned according to Muffett and Miglietta (2023).
4 Discussion
Our study highlights the significant role of light intensity, particularly PAR, in shaping the metabolic and photosynthetic responses of C. andromeda jellyfish, with high PAR exposure leading to increased oxidative stress, as indicated by higher MDA concentrations. While both PAR and UVA influenced oxidative stress, PAR was the primary factor affecting respiratory metabolism (ETS activity) and chlorophyll-a content. Interestingly, light intensity did not significantly alter growth metrics or protein content, suggesting that jellyfish can maintain growth under varying light conditions. These findings emphasize the broader ecological importance of light as an environmental factor, influencing not only jellyfish physiology but potentially other marine invertebrates, underscoring the need for further research on the impacts of PAR and UVR on marine organisms.
Light intensity, its variations, and UVR are critical factors influencing the survival, photosynthetic efficiency, and overall physiology of zooxanthellate symbiotic cnidarians, such as corals (Muscatine and Cernichiari, 1969; Kühl et al., 1995; Osinga et al., 2011; Aljbour et al., 2023). These factors play a similar role in shaping the metabolic and oxidative stress responses of jellyfish, as seen in our study, further highlighting the importance of light as a key environmental driver for marine invertebrates. It is well established that organisms respond differently to variations in light intensity and UVR. For instance, we previously showed that the mass of Cassiopea jellyfish exposed to PAR (460 μmol·m−2·s−1) increased by up to 40% (Aljbour et al., 2023), while light intensities (≥49 μmol·m−2·s−1) significantly reduced the growth rate of Acropora cervicornis (Main and Goodbody-Gringley, 2010). In contrast, in the present study, despite the varying PAR intensities (76–381 μmol·m−2·s−1) and UVA exposure, all jellyfish exhibited consistent growth (40%–55%), with no significant impact from light intensity or UVA on their growth. Our findings suggest that light intensity, whether in isolation or combined with UVA, did not affect jellyfish growth or protein content. Additionally, no signs of abnormal morphology, such as perforated bells or missing oral arms, were observed, further emphasizing the resilience of jellyfish to different light conditions. These results align with the broader understanding of light’s critical role in shaping the physiology and metabolic responses of marine invertebrates, such as corals and jellyfish.
UVR, particularly UVB, is widely known to negatively affect marine species, primarily by inducing oxidative stress and DNA damage (Lesser, 1996; Lesser and Farrell, 2004; Lu and Wu, 2005; Rastogi et al., 2010; Aljbour et al., 2023). In contrast, the effects of UVA, which has been less studied, can vary greatly among different organisms. For instance, UVA exposure enhanced the production of bioactive metabolites, such as xanthophylls (fucoxanthin and diadinoxanthin) and MAAs, in marine microalgae like Nitzschia closterium and Isochrysis zhangjiangensis after three days of exposure (Huang et al., 2018). Additionally, seasonal UVA variations induced hormonal and behavioral changes in marine annelids via a ciliary opsin (Veedin Rajan et al., 2021). In Antarctic mat-forming cyanobacteria, UVA mitigated the negative effects of UVB on bacterial growth rates (Quesada et al., 1995), with growth rates increasing linearly as the UVA-to-UVB ratio rose. For aerobic organisms, respiratory ETS activity (a proxy of aerobic respiration) is the most efficient pathway for energy production (ATP). In this study, ETS activity in Cassiopea jellyfish was significantly higher under low PAR treatments (LP and LPLA) compared to the combined high PAR and UVA treatment (HPHA), suggesting that low PAR favored higher metabolic enzyme activity. Our findings suggests that PAR intensity, rather than UVA exposure, was the primary driver of mitochondrial ETS activity. These results align with our earlier findings (Aljbour et al., 2023), where UVA exposure did not significantly alter aerobic respiration rates in Cassiopea or amplify UVB effects. Interestingly, this response contrasts with other organisms, such as Amynthas gracilis and Selenastrum capricornutum, where UVA exposure reduced respiration rates (Chuang et al., 2006; Beardall et al., 1997). This suggests that the metabolic response of Cassiopea jellyfish to UVA and PAR exposure is highly species-specific and might reflect adaptations to its natural light environment. Furthermore, the observed negative correlation between ETS activity and jellyfish mass suggests that smaller individuals maintain higher respiration rates, potentially as an adaptive mechanism to optimize energy production under specific environmental conditions. Overall, our results highlight the nuanced role of PAR intensity in regulating the energy metabolism of Cassiopea and underscore the resilience of this species to UVA exposure.
Zhou et al. (2016) found that UVA protected Pocillopora damicornis corals by mitigating the harmful effects of UVB on photosynthesis. The study also showed that the combined exposure to UV radiation and elevated seawater temperatures resulted in a reduction in chlorophyll-a concentration in the corals. Similarly, Mengelt and Prézelin (2005) showed that UVA enhanced carbon fixation and improved resistance to UVR-induced inhibition in the diatom genus Pseudo-nitzschia, providing these diatoms a competitive advantage in waters with high UVR incidence. In this study, chlorophyll-a concentration and coloration in Cassiopea jellyfish were mainly influenced by PAR intensity, with higher concentrations and darker coloration in low PAR (LP and LPLA) and brighter coloration in high PAR, while UVA exposure appeared to have minimal effect on these factors. Therefore, it seems that UVA, under the experimental conditions, does not directly influence photosynthesis. These changes in chlorophyll-a concentration and coloration suggest an associated changes in algal endosymbiont density under varying PAR conditions. Our findings are consistent with those published by Mortillaro et al. (2009) and Mammone et al. (2021), who found that PAR intensity has a major effect on Cassiopea photobiology. They reported higher chlorophyll-a concentrations in low light conditions but did not include UVA in their experimental setup. Mortillaro et al. (2009) also found that PAR intensity had a substantial impact on fatty acid synthesis and translocation by zooxanthellae in Cassiopea. We found a positive correlation between chlorophyll-a concentration and ETS activity in jellyfish, suggesting that elevated aerobic respiration rates are linked to chlorophyll-a concentration (a proxy for algal density) in low PAR-exposed jellyfish. Overall, these findings indicate that UVA does not directly affect photosynthesis in jellyfish, while PAR, chlorophyll-a concentration, ETS activity, and jellyfish coloration are closely related factors.
In symbiotic cnidarians, ROS formation is the primary mechanism through which UVR causes cellular damage (Lesser, 1996; Torres-Pérez and Armstrong, 2012; Donner et al., 2017; Nordborg et al., 2021). Oxidative stress can have harmful effects on marine organisms, including cellular damage, disruption of cellular processes, and coral bleaching. For instance, UVA exposure significantly increased lipid peroxidation (LPO) and superoxide dismutase activity in the diatom D. brightwellii (Rijstenbil, 2001). In this study, MDA concentrations, an indicator of LPO and oxidative stress, were significantly elevated in jellyfish exposed to high PAR, especially in the HPHA group. Overall, MDA levels were 69% higher in high PAR conditions compared to low PAR conditions, with UVA exposure further exacerbating oxidative stress. These findings suggest that high PAR intensity is the primary driver of lipid peroxidation in jellyfish. However, the involvement of aerobic respiration as a primary source of ROS can be ruled out due to the contrasting results between LPO (high) and ETS activity (low) in jellyfish exposed to high PAR.
Jellyfish are protein-rich, particularly in the mesoglea, where proteins make up about 50% of the dry weight, with collagen accounting for roughly 50% of the total protein (Ding et al., 2011; Khong et al., 2015). Environmental factors, such as temperature changes and heavy metal pollution, can significantly impact protein content in Cassiopea, with heat stress leading to decreased protein levels (Aljbour et al., 2017) and pollution causing protein reduction due to increased energy demands for maintenance (Aljbour et al., 2018). In contrast, protein concentrations in the current study were similar across all treatment groups, suggesting that protein was not being used for energy production under the experimental conditions.
5 Conclusion
Our study highlights the complex interactions between light intensity, UVA exposure, and the physiological responses of Cassiopea andromeda jellyfish. While high PAR exposure led to increased oxidative stress, as evidenced by elevated lipid peroxidation (LPO), the jellyfish demonstrated remarkable resilience, maintaining consistent growth, protein concentrations, and a 100% survival rate across all treatments. Notably, despite the elevated LPO in high PAR conditions, the jellyfish’s overall performance, including their metabolic activity (ETS) and coloration, was not significantly impaired. These findings suggest that C. andromeda jellyfish can tolerate variations in light intensity and UVA exposure, which highlights their adaptability to fluctuating environmental conditions. Our results underscore the broader ecological importance of light as a key environmental factor, shaping the physiology of marine invertebrates, and stress the need for further research on the impacts of light and UVR on marine organisms in diverse habitats.
Data availability statement
The raw data supporting the conclusions of this article will be made available by the authors, without undue reservation.
Ethics statement
The manuscript presents research on animals that do not require ethical approval for their study.
Author contributions
SMA: Conceptualization, Data curation, Formal analysis, Investigation, Methodology, Visualization, Writing – original draft, Writing – review & editing. SA: Conceptualization, Formal analysis, Funding acquisition, Resources, Writing – review & editing.
Funding
The author(s) declare financial support was received for the research, authorship, and/or publication of this article. King Abdullah University of Science and Technology provided funding support for this research through baseline funding allocated to SA.
Acknowledgments
We would like to acknowledge the CMR team at KAUST, specially Freddie B. Lampos, Dondon, Dr. Nabeel Alikunhi, and Dr. Zenon Batang. In addition, I would like to extend my sincere thanks to Mohammad Hilmi from the National University of Singapore for his assistance in setting up the experiment as well as Aussama Ebd Alzeem for his excellent assistance in the field. We also deeply thank Dr. Ricardo Alves from the BCL labs at KAUST for his help in the molecular work. We would like to acknowledge the valuable feedback provided by the editor and reviewers.
Conflict of interest
The authors declare that the research was conducted in the absence of any commercial or financial relationships that could be construed as a potential conflict of interest.
Publisher’s note
All claims expressed in this article are solely those of the authors and do not necessarily represent those of their affiliated organizations, or those of the publisher, the editors and the reviewers. Any product that may be evaluated in this article, or claim that may be made by its manufacturer, is not guaranteed or endorsed by the publisher.
References
Aljbour S. M., Al-Horani F., Kunzmann A. (2018). Metabolic and oxidative stress responses of the jellyfish Cassiopea to pollution in the Gulf of Aqaba, Jordan. Mar. pollut. Bull. 130, 271–278. doi: 10.1016/j.marpolbul.2018.03.044
Aljbour S. M., Alves R. N., Agustí S. (2023). Aerobic respiration, biochemical composition, and glycolytic responses to ultraviolet radiation in Jellyfish Cassiopea SP. Front. Mar. Sci. 9. doi: 10.3389/fmars.2022.1031977
Aljbour S. M., Zimmer M., Al-Horani F., Kunzmann A. (2019). Metabolic and oxidative stress responses of the jellyfish Cassiopea sp. to changes in seawater temperature. J. Sea Res. 145, 1–7. doi: 10.1016/j.seares.2018.12.002
Aljbour S. M., Zimmer M., Kunzmann A. (2017). Cellular respiration, oxygen consumption, and trade-offs of the jellyfish Cassiopea sp. in response to temperature change. J. Sea Res. 128, 92–97. doi: 10.1016/j.seares.2017.08.006
Alves R., Agustí S. (2020). Effect of ultraviolet radiation (UVR) on the life stages of fish. Rev. Fish Biol. Fisheries 30, 335–372. doi: 10.1007/s11160-020-09603-1
Arai M. N. (2001). Pelagic coelenterates and eutrophication: A review. Hydrobiologia 451, 69–87. doi: 10.1023/A:1011840123140
Banaszak A. T., Santos M. G. B., LaJeunesse T. C., Lesser M. P. (2006). The distribution of mycosporine-like amino acids (Maas) and the phylogenetic identity of symbiotic dinoflagellates in cnidarian hosts from the Mexican Caribbean. J. Exp. Mar. Biol. Ecol. 337, 131–146. doi: 10.1016/j.jembe.2006.06.014
Banaszak A. T., Trench R. K. (1995). Effects of ultraviolet (UV) radiation on marine microalgal-invertebrate symbioses. II. @ the synthesis of mycosporine-like amino acids in response to exposure to UV in anthopleura elegantissima and Cassiopea xamachana. J. Exp. Mar. Biol. Ecol. 194, 233–250. doi: 10.1016/0022-0981(95)00073-9
Baruch R., Avishai N., Rabinowitz C. (2005). UV incites diverse levels of DNA breaks in different cellular compartments of a branching coral species. J. Exp. Biol. 208, 843–848. doi: 10.1242/jeb.01496
Beardall J., Berman T., Markager S., Martinez R., Montecino V. (1997). The effects of ultraviolet radiation on respiration and photosynthesis in two species of microalgae. Can. J. Fisheries Aquat. Sci. 54, 687–696. doi: 10.1139/f96-320
Bradford M. M. (1976). A rapid and sensitive method for the quantitation of microgram quantities of protein utilizing the principle of protein-dye binding. Analytical Biochem. 72, 248–254. doi: 10.1016/0003-2697(76)90527-3
Cates N. (1975). Productivity and organic consumption in Cassiopea and Conductylus. J. Exp. Mar. Biol. Ecol. 18, 55–59. doi: 10.1016/0022-0981(75)90016-7
Chuang S. C., Lai W. S., Chen J. H. (2006). Influence of ultraviolet radiation on selected physiological responses of earthworms. J. Exp. Biol. 209, 4304–4312. doi: 10.1242/jeb.02521
Ding J.-F., Li Y., Xu J., Su X., Gao X., Yue F. (2011). Study on effect of jellyfish collagen hydrolysate on anti-fatigue and anti-oxidation. Food Hydrocolloids 25, 1350–1353. doi: 10.1016/j.foodhyd.2010.12.013
Donner S., Rickbeil G., Heron S. (2017). A new, high-resolution global mass coral bleaching database. PloS One 12, e0175490. doi: 10.1371/journal.pone.0175490
Fleck J., Fitt W. K. (1999). Degrading mangrove leaves of rhizophora mangle linne provide a natural cue for settlement and metamorphosis of the upside-down jellyfish Cassiopea xamachana Bigelow. J. Exp. Mar. Biol. Ecol. 234, 83–94. doi: 10.1016/S0022-0981(98)00140-3
Gohar H. A. F., Eisawy A. M. (1960). The development of Cassiopea andromeda. (Cairo: Fouad I University Press) 11, 148–190.
Hall T. A. (1999). BioEdit: A user-friendly biological sequence alignment editor and analysis program for windows 95/98/NT. Nucleic Acids Symposium Ser. 41, 95–98.
Hearst J. (1995). The structure of photolyase: Using photon energy for DNA repair. Science 268, 1858–1859. doi: 10.1126/science.7604259
Huang J. J., Lin S., Xu W., Cheung P. C. (2018). Enhancement of the production of bioactive microalgal metabolites by ultraviolet radiation (UVA 365 nm). J. Agric. Food Chem. 66, 10215–10224. doi: 10.1021/acs.jafc.8b03789
Jantzen C., Wild C., Rasheed M., El-Zibdah M., Richter C. (2010). Enhanced pore-water nutrient fluxes by the upside-down jellyfish Cassiopea sp. in a Red Sea coral reef. Mar. Ecol. Prog. Ser. 411, 117–125. doi: 10.3354/meps08623
Katoh K., Standley D. M. (2013). MAFFT multiple sequence alignment software version 7: Improvements in performance and usability. Mol. Biol. Evol. 30, 772–780. doi: 10.1093/molbev/mst010
Kheireddine M., Ouhssain M., Claustre H., Uitz J., Gentili B., Jones B. (2017). Assessing pigment-based phytoplankton community distributions in the red sea. Front. Mar. Sci. 4. doi: 10.3389/fmars.2017.00132
Khogali A., Albar O. F. (1992). A study of solar ultraviolet-radiation at makkah solar station. Solar Energy 48, 79–87. doi: 10.1016/0038-092x(92)90036-a
Khong N. M., Yusoff F. M., Jamilah B., Basri M., Maznah I., Chan K. W., et al. (2015). Nutritional composition and total collagen content of three commercially important edible jellyfish. Food Chem. 196, 953–960. doi: 10.1016/j.foodchem.2015.09.094
Klein S. G., Pitt K. A., Carroll A. R. (2016). Surviving but not thriving: inconsistent responses of zooxanthellate jellyfish polyps to ocean warming and future UV-B scenarios. Sci. Rep. 6. doi: 10.1038/srep28859
Kremer P., Costello J., Kremer J., Canino M. (1990). Significance of photosynthetic endosymbionts to the carbon budget of the scyphomedus linuche unguiculata. Limnology Oceanography 35, 609–624. doi: 10.4319/lo.1990.35.3.0609
Kühl M., Cohen Y., Dalsgaard T., Jørgensen B., Revsbech N. (1995). Microenvironment and photosynthesis of zooxanthellae in Scleractinian corals studied with microsensors for O2, ph and light. Mar. Ecol. Prog. Ser. 117, 159–172. doi: 10.3354/meps117159
LaJeunesse T. C., Parkinson J. E., Gabrielson P. W., Jeong H. J., Reimer J. D., Voolstra C. R., et al. (2018). Systematic revision of Symbiodiniaceae highlights the antiquity and diversity of coral endosymbionts. Curr. Biol. 28 (16), 2570–2580. doi: 10.1016/j.cub.2018.07.008
Lesser M. P. (1996). Elevated temperatures and ultraviolet radiation cause oxidative stress and inhibit photosynthesis in symbiotic dinoflagellates. Limnology Oceanography 41, 271–283. doi: 10.4319/lo.1996.41.2.0271
Lesser M. P., Farrell J. H. (2004). Exposure to solar radiation increases damage to both host tissues and algal symbionts of corals during thermal stress. Coral Reefs 23, 367–377. doi: 10.1007/s00338-004-0392-z
Lu X. Y., Wu R. S. S. (2005). UV induces reactive oxygen species, damages sperm, and impairs fertilization in the sea urchin Anthocidaris crassispina. Mar. Biol. 148, 51–57. doi: 10.1007/s00227-005-0049-7
Main K. L., Goodbody-Gringley G. (2010). “Effect of light on the growth of four coral species in land-based nursery systems,” in Book of abstract, linking science to management: A conference and workshop on the florida keys marine ecosystem, 19–22 october 2010(Duck Key, Florida), 19–22.
Mammone M., Ferrier-Pagés C., Lavorano S., Rizzo L., Piraino S., Rossi S. (2021). High photosynthetic plasticity may reinforce invasiveness of upside-down zooxanthellate jellyfish in Mediterranean Coastal Waters. PloS One 16 (3), e0248814. doi: 10.1371/journal.pone.0248814
McCloskey L. R., Muscatine L., Wilkerson F. P. (1994). Daily photosynthesis, respiration, and carbon budgets in a tropical marine jellyfish (mastigias sp). Mar. Biol. 119, 3–22. doi: 10.1007/bf00350101
Mengelt C., Prézelin B. (2005). UVA enhancement of carbon fixation and resilience to UV inhibition in the genus pseudo-nitzschia may provide a competitive advantage in high UV surface waters. Mar. Ecol. Prog. Ser. 301, 81–93. doi: 10.3354/meps301081
Moan J. (2001). “Visible light and UV radiation,” in Radiation at home outdoors and in the workplace. Eds. Brune A., Hellborg R., Persson B. R. R., Pääkkönen R. (Scandinavian Science Publisher, Oslo), 69–85.
Mortillaro J. M., Pitt K. A., Lee S. Y., Meziane T. (2009). Light intensity influences the production and translocation of fatty acids by zooxanthellae in the jellyfish Cassiopea sp. J. Exp. Mar. Biol. Ecol. 378, 22–30. doi: 10.1016/j.jembe.2009.07.003
Muffett K., Miglietta M. P. (2023). Demystifying cassiopea species identity in the Florida keys: Cassiopea Xamachana and Cassiopea andromeda coexist in shallow waters. PloS One 18 (3), e0283441. doi: 10.1371/journal.pone.0283441
Muscatine L., Cernichiari E. (1969). Assimilation of photosynthetic products of zooxanthellae by a reef coral. Biol. Bull. 137, 506–523. doi: 10.2307/1540172
Niggl W., Naumann M. S., Struck U., Manasrah R., Wild C. (2010). Organic matter release by the benthic upside-down jellyfish Cassiopea sp. fuels pelagic food webs in coral reefs. J. Exp. Mar. Biol. Ecol. 384, 99–106. doi: 10.1016/j.jembe.2019.104845
Niggl W., Wild C. (2009). Spatial distribution of the upside-down jellyfish Cassiopea sp. within fringing coral reef environments of the northern Red Sea: implications for its life cycle. Helgoland Mar. Res. 64, 281–287. doi: 10.1007/s10152-009-0181-8
Nordborg F., Brinkman D., Ricardo G., Agustí S., Negri A. (2021). Comparative sensitivity of the early life stages of a coral to heavy fuel oil and UV radiation. Sci. Total Environ. 781, 146676. doi: 10.1016/j.scitotenv.2021.146676
Osinga R., Schutter M., Griffioen B., Wijffels R. H., Verreth J. A., Shafir S., et al. (2011). The biology and economics of coral growth. Mar. Biotechnol. 13, 658–671. doi: 10.1007/s10126-011-9382-7
Overmans S., Agustí S. (2019). Latitudinal gradient of UV attenuation along the highly transparent red sea basin. Photochem. Photobiol. 95, 1267–1279. doi: 10.1111/php.13112
Overmans S., Agustí S. (2020). Unraveling the seasonality of UV exposure in reef waters of a rapidly warming (Sub-)tropical sea. Front. Mar. Sci. 7. doi: 10.3389/fmars.2020.00111
Owens T. G., King F. D. (1975). The measurement of respiratory electron-transport-system activity in marine zooplankton. Mar. Biol. 30, 27–36. doi: 10.1007/bf00393750
Packard T. T. (1971). The measurement of respiratory electron transport activity in marine plankton. J. Mar. Res. 29, 235–244.
Pierce J. (2005). A system for mass culture of Upside-down jellyfish Cassiopea spp as a potential food item for medusivores in captivity. Int. Zoo Yearbook 39, 62–69. doi: 10.1111/j.1748-1090.2005.tb00005
Porra R. J., Thompson W. A., Kriedemann P. E. (1989). Determination of accurate extinction coefficients and simultaneous equations for assaying chlorophylls a and B extracted with four different solvents: Verification of the concentration of chlorophyll standards by atomic absorption spectroscopy. Biochim. Biophys. Acta (BBA) - Bioenergetics 975, 384–394. doi: 10.1016/s0005-2728(89)80347-0
Prasanth M. I., Santoshram G. S., Bhaskar J. P., Balamurugan K. (2016). Ultraviolet-A triggers photoaging in model nematode Caenorhabditis elegans in a DAF-16 dependent pathway. AGE 38 (1). doi: 10.1007/s11357-016-9889-y
Quesada A., Mouget J.-L., Vincent W. F. (1995). Growth of Antarctic cyanobacteria under ultraviolet radiation: UVA counteracts UVB inhibition. J. Phycology 31, 242–248. doi: 10.1111/j.0022-3646.1995.00242.x
Rastogi R., Richa, Kumar A., Tyagi M., Sinha R. (2010). Molecular mechanisms of ultraviolet radiation-induced DNA damage and repair. J. Nucleic Acids 2010, 1–32. doi: 10.4061/2010/592980
Rijstenbil J. (2001). Effects of periodic, low UVA radiation on cell characteristics and oxidative stress in the marine planktonic diatom Ditylum brightwellii. Eur. J. Phycology 36, 1–8. doi: 10.1080/09670260110001735138
Rossbach S., Overmans S., Kaidarova A., Kosel J., Agustí S., Duarte C. M. (2020). Giant clams in shallow reefs: UV-resistance mechanisms of Tridacninae in the Red Sea. Coral Reefs 39, 1345–1360. doi: 10.1007/s00338-020-01968-w
Roy S. (2000). “Strategies for the minimization of UV-induced damage,” in The effects of UV radiation in the marine environment. Cambridge environ. Chem. Ser., 10. Eds. De Mora S., Demers S., Vernet M. (Cambridge University Press, Cambridge), 177–205.
Sancar A. (1996). No “End of history” for photolyases. Science 272, 48–49. doi: 10.1126/science.272.5258.48
Sinha R., Häder D. (2002). UV-induced DNA damage and repair: a review. Photochemical Photobiological Sci. 1, 225–236. doi: 10.1039/b201230h
Stamatakis A. (2014). RAxML version 8: A tool for phylogenetic analysis and post-analysis of large phylogenies. Bioinformatics 30, 1312–1313. doi: 10.1093/bioinformatics/btu033
Stambler N., Dubinsky Z. (2004). Corals as light collectors: An integrating sphere approach. Coral Reefs 24, 1–9. doi: 10.1007/s00338-004-0452-4
Todd B. D., Thornhill D. J., Fitt W. K. (2006). Patterns of inorganic phosphate uptake in Cassiopea xamachana: a bioindicator species. Mar. pollut. Bull. 52, 515–552. doi: 10.1016/j.marpolbul.2005.09.044
Torres-Pérez J., Armstrong R. (2012). Effects of UV radiation on the growth, photosynthetic and photoprotective components, and reproduction of the Caribbean shallow-water coral Porites furcata. Coral Reefs 31, 1077–1091. doi: 10.1007/s00338-012-0927-7
Uchiyama M., Mihara M. (1978). Determination of malonaldehyde precursor in tissues by Thiobarbituric Acid Test. Analytical Biochem. 86, 271–278. doi: 10.1016/0003-2697(78)90342-1
Veedin Rajan V. B., Häfker N. S., Arboleda E., Poehn B., Gossenreiter T., Gerrard E., et al. (2021). Seasonal variation in UVA light drives hormonal and behavioural changes in a marine annelid via a ciliary opsin. Nat. Ecol. Evol. 5, 204–218. doi: 10.1038/s41559-020-01356-1
Verde E. A., McCloskey L. R. (1998). Production respiration and photophysiology of the mangrove jellyfish Cassiopea xamachana symbiotic with zooxanthellae: effect of jellyfish size and season. Mar. Ecol. Prog. Ser. 168, 147–162. doi: 10.3354/meps168147
Winters G., Loya Y., Röttgers R., Beer S. (2003). Photoinhibition in shallow-water colonies of the coral Stylophora pistillata as measured in situ. Limnology Oceanography 48, 1388–1393. doi: 10.4319/lo.2003.48.4.1388
Zarnoch C. B., Hossain N., Fusco E., Alldred M., Hoellein T. J., Perdikaris S. (2020). Size and density of upside-down jellyfish, Cassiopea sp., and their impact on benthic fluxes in a Caribbean lagoon. Mar. Environ. Res. 154, 104845. doi: 10.1016/j.marenvres.2019.104845
Keywords: ultraviolet A (UVA), aerobic respiration, lipid peroxidation, oxidative stress, Red Sea, Cassiopea andromeda, upside-down jellyfish, endosymbionts
Citation: Aljbour SM and Agustí S (2024) Illuminating Cassiopea jellyfish: biochemical revelations from metabolism to coloration under ultraviolet A and photosynthetically active radiation. Front. Mar. Sci. 11:1348864. doi: 10.3389/fmars.2024.1348864
Received: 03 December 2023; Accepted: 02 December 2024;
Published: 19 December 2024.
Edited by:
Patrick J. Neale, Smithsonian Environmental Research Center (SI), United StatesReviewed by:
Christine Ferrier-Pagès, Centre Scientifique de Monaco, MonacoMarta Mammone, Texas A&M University at Galveston, United States
Copyright © 2024 Aljbour and Agustí. This is an open-access article distributed under the terms of the Creative Commons Attribution License (CC BY). The use, distribution or reproduction in other forums is permitted, provided the original author(s) and the copyright owner(s) are credited and that the original publication in this journal is cited, in accordance with accepted academic practice. No use, distribution or reproduction is permitted which does not comply with these terms.
*Correspondence: Samir M. Aljbour, U2FtaXIuYWxqYm91ckBiYXUuZWR1Lmpv