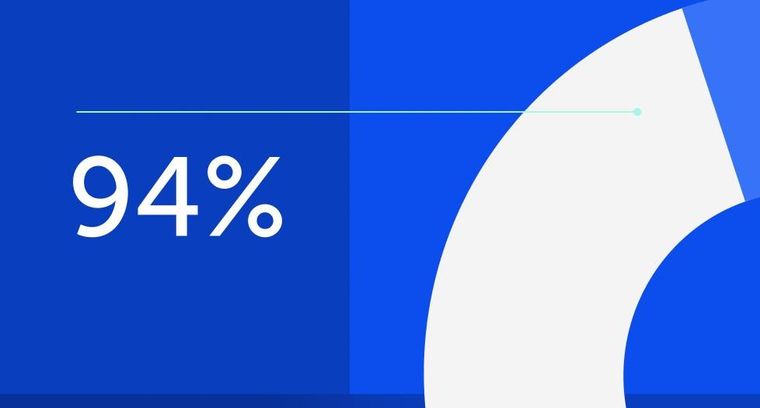
94% of researchers rate our articles as excellent or good
Learn more about the work of our research integrity team to safeguard the quality of each article we publish.
Find out more
ORIGINAL RESEARCH article
Front. Mar. Sci., 05 April 2024
Sec. Ocean Observation
Volume 11 - 2024 | https://doi.org/10.3389/fmars.2024.1337929
This article is part of the Research TopicTime-Series Observations of Ocean Acidification: a Key Tool for Documenting Impacts on a Changing PlanetView all 18 articles
Ocean acidification, caused by the absorption of carbon dioxide (CO2) from the atmosphere into the ocean, ranks among the most critical consequences of climate change for marine ecosystems. Most studies have examined pH and CO2 trends in the open ocean through oceanic time-series research. The analysis in coastal waters, particularly in island environments, remains relatively underexplored. This gap in our understanding is particularly important given the profound implications of these changes for coastal ecosystems and the blue economy. The present study focuses on the ongoing monitoring effort that started in March 2020 along the east coast of Gran Canaria, within the Gando Bay, by the CanOA-1 buoy. This monitoring initiative focuses on the systematic collection of multiple variables within the CO2 system, such as CO2 fugacity (fCO2), pH (in total scale, pHT), total inorganic carbon (CT), and other hydrographic variables including sea surface salinity (SSS), sea surface temperature (SST) and wind intensity and direction. Accordingly, the study allows the computation of the CO2 flux (FCO2) between the surface waters and the atmosphere. During the study period, stational (warm and cold periods) behavior was found for all the variables. The lowest SST values were recorded in March, with a range of 18.8-19.3°C, while the highest SST were observed in September and October, ranging from 24.5-24.8°C. SST exhibited an annual increase with a rate of 0.007°C yr-1. Warmer months increased SSS, while colder periods, influenced by extreme events like tropical storms, led to lower salinity (SSS=34.02). The predominant Trade Winds facilitated the arrival of deeper water, replenishing seawater. The study provided insights into atmospheric CO2. Atmospheric fCO2 averaged 415 ± 4 µatm (2020-2023). Surface water fCO2sw presented variability, with the highest values recorded in September and October, peaking at 437 µatm in September 2021. The lowest values for fCO2sw were found in February 2021 (368 µatm). From 2020 to 2023, surface water fCO2sw values displayed an increasing rate of 1.9 µatm yr-1 in the study area. The assessment of fCO2sw decomposition into thermal and non-thermal processes revealed the importance of SST on the fCO2sw. Nevertheless, in the present study, it is crucial to remark the impact of non-thermal factors on near-shallow coastal regions. Our findings highlight the influence of physical factors such as tides, and wind effect to horizontal mixing in these areas. The CT showed a mean concentration of 2113 ± 8 μmol kg-1 and pH at in-situ temperature (pHT,IS) has a mean value of 8.05 ± 0.02. The mean FCO2 from 2020 to 2023 was 0.34 ± 0.04 mmol m-2 d-1 (126 ± 13 mmol m-2 yr-1) acting as a slight CO2 source. In general, between May and December were the months when the area was a source of CO2. Extrapolating to the entire 6 km2 of Gando Bay, the region sourced 33 ± 4 Tons of CO2 yr-1.
Over the past two centuries, there has been an exponential increase in atmospheric CO2 concentrations as a result of anthropogenic activities (Denman et al., 2007; Takahashi et al., 2009; Lynas et al., 2021; Friedlingstein et al., 2022), also indicated in the 6th IPCC Report (IPCC, 2022; IPCC is the Intergovernmental Panel on Climate Change). A substantial portion of this anthropogenic CO2 is directly transferred to the ocean, accounting for about 26% of the total anthropogenic CO2 emissions (Friedlingstein et al., 2022). The ocean’s capacity to absorb CO2, has exhibited an increase from 1.0 ± 0.3 gigatons of carbon per year (Gt C yr-1) in 1960 to 2.5 ± 0.6 Gt C yr-1 in the 2010 to 2019 period (Friedlingstein et al., 2020). This transfer of CO2 from the atmosphere to the ocean has profound repercussions on the marine chemistry and ecosystems (Wollast and Mackenzie, 1989; Walsh, 1991; Falkowski and Wilson, 1992), for example influencing the potential acidification of coastal marine waters (Borges and Gypensb, 2010; Wallace et al., 2014; Carstensen and Duarte, 2019). The oceanic pH has decreased by 0.1 units since the onset of the Industrial Revolution, representing a 26% increase in ocean acidification over the past two centuries (Doney et al., 2009). Projections suggest that the global CO2 concentration will increase by more than 500 parts per million (ppm) by the end of this century, leading to a pH decrease of 0.4 units from the preindustrial values (Orr et al., 2005; Jiang et al., 2023).
To comprehend the evolution of any variable, such as temperature, atmospheric and oceanic CO2, pH, sea level, etc., and their relationship to climate change, the establishment of long-term time series is essential. It is widely acknowledged that observing stations, particularly fixed stations, constitute the most reliable data source for investigating and estimating CO2 fluxes between the atmosphere and the ocean (Takahashi et al., 2014; Bates and Johnson, 2020; Skjelvan et al., 2022). An important development in this regard is the Global Ocean Acidification Network (GOA-ON; http://www.goa-on.org/), which aims to coordinate, promote, and sustain long-term observations of the carbonate system at both local and national scales. Measurements of the CO2 system have predominantly focused on open waters, while coastal regions are underrepresented in the Global Carbon Budget (Friedlingstein et al., 2022) due to limited observational data, insufficient high-frequency monitoring, and the complexity of modelling these diverse environments (Takahashi et al., 2002; González Dávila et al., 2005; González-Dávila et al., 2007; Santana-Casiano et al., 2007; Bates, 2012; Bates et al., 2014; González-Dávila and Santana-Casiano, 2023).
The European Time Series in the Ocean at the Canary Islands (ESTOC), situated in the Northeast Atlantic at 29°10’N - 15°30’ W, where the ocean reaches 3600 meter depth, has been instrumental in collecting hydrographic and CO2 system measurements for more than 25 years (Santana-Casiano et al., 2007; González-Dávila et al., 2010; Bates et al., 2014; Takahashi et al., 2014; González-Dávila and Santana-Casiano, 2023). Since 1995, ESTOC has observed a consistent increase in seawater salinity-normalized inorganic carbon (NCT), fugacity of CO2 (fCO2), and anthropogenic CO2 at rates of 1.17 ± 0.07 μmol kg-1, 2.1 ± 0.1 μatm yr-1, and 1.06 ± 0.11 mmol kg-1 yr-1, respectively (González-Dávila and Santana-Casiano, 2023). For the same period, pHT normalized to 21°C has declined at a rate of 0.002 ± 0.0001 pH units yr-1 within the top 100 meters of the water column. ESTOC has provided valuable insights into the impact of Trade Winds on the atmosphere-ocean CO2 transfer, resulting in seasonal variability in the CO2 system (González-Dávila et al., 2003).
While these CO2 trends have been studied in the open ocean, there is a lack of extensive information on coastal zones, which, despite covering only 7-10% of the total ocean surface area and less than 0.5% of the ocean volume (Laruelle et al., 2013), serve as a critical interface between land, atmosphere, and ocean (Bauer et al., 2013). Coastal zones concentrate up to 30% of the primary production and organic matter remineralization in coastal shelf areas (Walsh et al., 1988; de Haas et al., 2002; Bauer et al., 2013). Consequently, they exhibit high uptake and release of dissolved inorganic carbon and partial pressure of CO2 (pCO2 or fCO2) (Thomas et al., 2005).
The behavior of coastal zones with respect to CO2 exchange is complex and depends on several factors (Walsh and Dieterle, 1994; Chen, 2004; Borges, 2005; Borges et al., 2005, 2006; Cai et al., 2006; McNeil, 2010; Shaw and McNeil, 2014; Terlouw et al., 2019; Gac et al., 2020). Existing studies highlight the need for long-term coastal time series data, as many estimates have extrapolated values from specific coastal regions to a global scale (Borges, 2005; Borges et al., 2005; Cai et al., 2006; Chen et al., 2013). There are latitudinal variations in coastal regions, with mid- and high-latitude shelf systems generally functioning as net CO2 sinks (-0.33 Pg C yr-1), while low-latitude shelf systems tend to act as net CO2 sources (0.11 Pg C yr-1) (Borges et al., 2005; Cai et al., 2006; Chen et al., 2013). In broad terms, the global continental shelves exhibit a net CO2 uptake, with estimates ranging from approximately -0.25 (Cai, 2011) to -0.4 Pg C yr-1 (Chen et al., 2013). Shallow near-shore coastal areas including estuaries, salt marshes, coral reefs, coastal upwelling systems, and mangroves, act as sources of CO2 to the atmosphere (Bouillon et al., 2008; Chen and Borges, 2009), with estuaries being the major contributors to this ocean-atmosphere CO2 flux. Coastal ecosystems are particularly characterized by substantial and variable inputs of nutrients discharged by rivers. These inputs trigger strong seasonal and interannual variability in the carbonate system (Gypens et al., 2009, 2011).
Further research is needed to acquire additional CO2 data for scaling air-water CO2 fluxes in outer estuaries, which may exert a substantial influence on the overall flux of estuarine systems (Borges and Frankignoulle, 2002; Borges, 2005). The same is true for near-shallow coastal areas on islands, where data is lacking and where the CO2 system is intricately linked to biological activities, physical processes, wind regimes, precipitation patterns and the significant input of nutrients and carbon from the land via rivers and runoff. Moreover, the coastal ocean, which extends from the open ocean to the continental margins, is one of the most biogeochemically active domains within the biosphere (Gattuso et al., 1998).
Despite all the previously reported studies, information on CO2 monitoring in islands are scarce. This study represents the first scientific effort dedicated to monitoring the CO2 system within coastal areas of the Canary Islands, employing a time series approach. While global studies on coastal areas exist, islands such as the Canary Islands offer natural laboratories conducive to monitoring the transfer of CO2 between the atmosphere and the ocean. Moreover, according to the 6th IPCC report, islands are one of the most vulnerable regions to the impact of climate change. The main objective of this study was to quantify variations in CO2 fugacity (fCO2), pH (at total scale), Total Inorganic Carbon (CT), and atmosphere-ocean CO2 flux (FCO2) in Gando Bay, a coastal region located to the east of the island of Gran Canaria. This study covers the first three years of observations, with a particular focus on elucidating the diverse processes governing the atmosphere-ocean CO2 transfer and studying the first seasonal variability of the CO2 system in the coastal waters of the Gando Bay to have a preliminary trend of each variable.
The CanOA-1 buoy is located within the Canary Islands, on the eastern side of the island of Gran Canaria (Figure 1), off the northwest coast of Africa and in shallow coastal waters (27.930°N; 15.365°W; at 12 m depth), within a military area that prevents vandalism with controlled access. This geographical location places the islands between two important oceanic features: the African upwelling to the east and the oligotrophic waters of the North Atlantic Subtropical Gyre. The Canary Islands are also influenced by the Canary Current, which delineates the eastern boundary of the subtropical gyre (Knoll et al., 2002). The prevailing winds are the Trade Winds.
Figure 1 Study area map. The Gando Bay belongs to Gran Canaria Island. Red flag represents the location of buoy and green point, the ESTOC station.
The CanOA-1 buoy structure is equipped with an array of seven sensors, including sea surface temperature and salinity (SST and SSS respectively; SBE 37-SI/SIP Thermosalinometer MicroCAT sensor manufactured by Sea-Bird Scientific –accuracy for SST is ±0.002°C and for SSS is ±0.01 units), fluorescence (Cyclops-7F from Turner Designs with a detection limit of 0.03 µg L-1), dissolved oxygen concentration (Optode 4835 Oxymeter, manufactured by Aanderaa with an accuracy<0.1 µmol L-1), photometric pH (SAMI-pH meter from Sunburst, precision<0.003 ppm and accuracy ±0.01 units), pCO2 (partial pressure of CO2, here expressed as fugacity of CO2 – fCO2; measured with a CO2-pro CV sensor from PrOceanus - precision ±0.01 ppm and accuracy ±0.5%, using a Non-Dispersive Infrared Detector - NDIR) operating on a three-hour schedule, measuring the molar fraction of CO2 (xCO2) and converted internally to pCO2 in seawater using a CO2-permeable membrane. An external Sea-Bird Scientific pump (SBE-5) supplies seawater from outside the buoy body (60 cm), including copper-intake tubing to reduce biofouling effects. An internal zero determination is made every 24 hours to eliminate any signal drift. The pCO2 was also measured using a Battelle system (model 635108H1010), which assesses the xCO2 in both seawater and the atmosphere every three hours using equilibration-CO2 Infrared detection. Atmospheric xCO2 data from the Battelle system at 2.5 m above sea level, were calculated to 10 m (Hsu et al., 1994) and compared with those obtained at the ICOS Izaña Atmospheric Research Station (Tenerife, Canary Islands) and provided by the Agencia Estatal de Meteorología (AEMET). The agreement was better than ± 3 ppm. After the first year of work, the Batelle system had to be repaired so the Izaña data was used. Various meteorological variables (wind speed, wind direction, air temperature, humidity, atmospheric pressure, precipitation, solar radiation, and GPS coordinates – Gill MaxiMet GMX 501 GPS) were measured. All the sensors were installed at a depth of 1.5 m depth except for the meteorological station that was located 2.5 m above sea level. All data are free and the last 2000 data are available in real-time on the free Telegram app under Boya Morgan (@QUIMAbot).
Despite all the sensors installed on the buoy, the pH, chlorophyll and oxygen data have not been used due to their low stability and biofouling problems. In this sense, the pH sensor showed high variability and the pH data used in the manuscript were computed from total alkalinity to salinity relationship.
The buoy was visited every 2-3 months for inspection and maintenance. In addition, to determine the sensitivity and accuracy of the sensors, 23 surface water samples (with duplicates) were collected throughout the observation period. These samples were analyzed in the laboratory for total alkalinity (AT), total dissolved inorganic carbon (CT), and oxygen concentration. AT and CT were determined using the VINDTA 3C system (Mintrop et al., 2000) with Certified Reference Material (CRM) from batches 108, 122, 163, 177 and 196, provided by A. Dickson (Scripps Institute of Oceanography, University of South California, San Diego, United States) with allowed accuracy of ±1.5 μmol kg-1 for both CT and AT. Oxygen was measured using the Winkler method (Granéli and Granéli, 1991). These laboratory measurements were subsequently used to verify the response of the various sensors (see below) and to establish relationships with continuous salinity data. Additionally, each measurement of CO2 obtained by the Battelle sensor was calibrated every 3-hours prior to analysis using a zero and an external CO2 gas cylinder with a known concentration of 553.35 ± 0.02 ppm traceable to the World Meteorological Organization.
The Battelle sensor provides xCO2 values while the PrOceanus sensors provide pCO2. The xCO2 data were converted to pCO2 (pCO2,equ; Dickson et al., 2007) (Equation 1). The pCO2,equ is the partial pressure of CO2 in the equilibrator, Patm (atm) is the atmospheric pressure and the expression for water vapor (pH2O) is given in Equation 2. SST (K) is the sea surface temperature and SSS is the measured salinity. Once pCO2 was obtained, the fCO2 was calculated (Equation 3). The coefficients (cm3 mol-1) and are given by Equations 4 and 5, respectively.
To determine the CO2 flux (FCO2) between the atmosphere and the sea surface water, Equations 6 and 7 were used:
where 0.24 is a conversion factor to have the flux in mmol m-2 d-1, S is the CO2 solubility in mol dm-3 atm-1 (Weiss, 1970), and ΔfCO2 is the difference between fCO2 in seawater and atmosphere (fCO2 SW-fCO2 atm). k is the gas transfer velocity given by Wanninkhof (2014), where w is the wind velocity in m s-1 (at 10 m height), Sc is the Schmidt number which considers the kinematic viscosity of seawater divided by the gas diffusion coefficient (Wanninkhof, 2014). The FCO2 flux depends on the difference between fCO2 in the seawater and the atmosphere, the temperature, and the wind speed. If the flux is negative, the ocean acts as a sink, and if it is positive, it acts as a source. Wind speeds were averaged from two hours before and two hours after each study point.
The fCO2Tmean was calculated at the approximate annual mean temperature (21°C) (Takahashi, 1993) to obtain the temperature-independent fCO2 (Equation 8). Hence, the annual non-thermal effect is obtained by the difference of the minimum and the maximum values obtained in Equation 9. The same process was followed to know the thermal effect (Equation 10) on the average observed fCO2. The annual thermal effect was determined by the difference of the minimum and maximum values (Equation 11).
The thermal to non-thermal ratio (T/NT) shows the importance of both (physical and biological) effects. It was calculated by dividing the terms in Equations 9 and 11 ( indicating that when the ratio is greater than 1, the temperature effect dominates over the other effects.
Total dissolved inorganic carbon (CT), pH at in situ temperature (pHT,IS) and normalized to a mean temperature of 21°C (pHT, T=21°C) were calculated with the Excel program CO2sys (Pierrot et al., 2021) using total alkalinity (computed from salinity) and the measured pCO2 in seawater. The carbonic acid dissociation constants of Lueker et al. (2000), the HSO4- dissociation constant of Dickson (1990) and the value of [B]I determined by Lee et al. (2010) were used.
Alkalinity concentrations used in the calculations (data not shown) were obtained from in situ samples (n=23, collected every 2-3 months) and normalized to a salinity (SSS) of 35 (NAT = AT/SSS·35). A constant value for NAT = 2292.3 ± 2.8 μmol kg-1 was obtained (similar to that obtained at the ESTOC site, located 60 miles north of the buoy site, González-Dávila et al., 2010), confirming that alkalinity is controlled by salinity variability and is not affected by atmospheric CO2 increase or spring-summer primary productivity. In addition, since the SAMI sensor failed due to bubbles in the tubing, the pH was calculated in the total scale (pHT) with alkalinity determined from salinity and pCO2 variables.
The AT-CT pair of discrete data were used to test pCO2 sensor values (23 pairs) and other carbonate system variables. The computed pCO2 values and those provided by the sensor for the same day and time of the day were within ± 6 μatm. Calculated pHT values from the AT-CT discrete values pair and those from AT from salinity and sensor pCO2 data were within ± 0.01 pH units. Moreover, discrete CT concentrations (n = 23) and those determined from AT from salinity and sensor pCO2 data were within ± 3 μmol kg-1.
The surface water displacement was calculated using the Ekman Equations 12 and 13, which include variables such as DE (Ekman depth), z (depth of interest, in this case, 8 meters), f (Coriolis parameter), and Az (turbulent viscosity coefficient). The solution to this equation yields a displacement angle of 45° when z is 0, meaning that the surface current flows at an angle of 45° to the right of the wind direction (Pond and Pickard, 1983). This calculated value was then added to the measured wind direction for further analysis.
The trend analysis performed on the observed data includes inherent seasonal variability, which is influenced by sampling irregularities throughout the study period. To mitigate this seasonality, a seasonal detrending approach was implemented, in line with methodologies used in other time series analyses (e.g., Bates, 2012; González-Dávila and Santana-Casiano, 2023). The data were organized into corresponding monthly bins, spanning the time series from 2020 to 2023. Within each month, the mean and standard deviation were computed. Anomalies were then determined by subtracting the monthly mean from each data point within the dataset. This procedure effectively mitigated the temporal non-uniformity present in the data. Furthermore, a harmonic fitting technique was applied, similar to methods previously used in studies such as those conducted at the ESTOC site (González-Dávila et al., 2010). This fitting allows the determination, in a single step, of both seasonal effects (terms c, d, e, f) and interannual trends (b, Equation 14) as a function of time for the variable considered (y), expressed as an annual fraction (x). The results of this harmonic fitting analysis gave trends that closely aligned with those obtained within the estimated error margins for each considered parameter.
The study area is characterized by a seasonal amplitude of SST as depicted in Figure 2A, with a range of approximately 5-6°C, fluctuating between 19°C and 24.8°C. The lowest SST were recorded in March, ranging from 18.8 - 19.3°C, while the highest SST were observed in September-October, ranging from 24.5 - 24.8°C. It is noteworthy that the minimum SST was registered in the year 2022, with SST reaching 18.8°C. During the period from 2020 to 2023, the average SST was 21.2 ± 1.6°C. Even if three years of data are not enough to obtain a significant trend analysis, it is observed that the surface waters in the study area exhibit a warming trend at a rate of 0.007°C yr-1 (Table 1; SupplementaryFigure SI-1), a value that is consistent with solid trends shown by 25 years of ESTOC data (i.e. González-Dávila and Santana-Casiano, 2023). It is worth noting that there is a seasonal shift in SST, with the warmest temperatures typically occurring in July and August, comparable to the usually warmer months of September and October (Curbelo-Hernández et al., 2021; González-Dávila and Santana-Casiano, 2023), indicating that factors other than warming are acting on these coastal waters.
Figure 2 Sea Surface Temperature (SST, °C) (A), Sea Surface Salinity, rain (mm) and wind speed (m s-1) for the stronger winds > 7 m s-1 (B), and all the wind data [(C), m s-1] recorded at the CanOA-1 site. The lines in (A) and (B) correspond to the harmonic fit of the observed data.
Table 1 Variables for the equations of interannual trend of the different variables monitored in the Gando Bay, according with Equation 14 where x is the year fraction of each observation y.
The SSS exhibited distinct characteristics during the 2020-2023 period (Figure 2B). The SSS reached its maximum salinities, ranging from 36.92 to 37.04, during the months of September and October. At certain time points, the SSS dropped to as low as 34 (e.g., SSS = 34.02 in September 2022), coinciding with periods of heavy rainfall (Figure 2B). The mean SSS observed throughout the study period was 36.71 ± 0.14. It was also observed that coinciding with the dominance of the Trade Winds from May to September (Figure 2B, green data, and Figure 2C), when the wind speed was above 7 m s-1, low anomalous salinities were registered with respect to those described by the harmonic fit. The salinity has increased by 0.02 ± 0.001 during the observed period (Table 1; Figure 2B; Supplementary Figure SI-1).
Figure 3A shows that both CO2 sensors provided highly consistent data (linear relationship with r2 = 0.962, where the root mean square deviation (RMSD) was 2.9 µatm, n = 4600). The temporal overview of the fCO2 in both the atmosphere and surface water within the study area is shown in Figure 3A. The average atmospheric fCO2,atm was 415 ± 4 µatm. Surface water fCO2sw showed variability, with the highest values occurring in September and October, reaching a maximum of 444 µatm (in September 2021). Conversely, the lowest fCO2sw values were observed during the coldest months, particularly in February and March, when they hovered around 368 µatm. Consequently, a more pronounced seasonal fCO2sw amplitude of about 55 - 60 µatm, was observed in the surface water compared to the atmosphere (about 12 µatm). Remarkably, in March 2023, the fCO2sw values did not reach the lowest values observed in previous years, only decreasing to 384 µatm. This trend suggests that the surface water is undersaturated with CO2 relative to the atmosphere in cold months, whereas it becomes oversaturated in warm months. As mentioned above, three years of data provide only a first estimate of any trend, including the fact that more local events are acting on coastal areas than in open ocean waters making more complex the calculation of a definitive trend. Nevertheless, the fCO2sw values for the period 2020-2023 increase with an annual rate of 1.9 ± 0.1 µatm yr-1 (Table 1) in the study area, considering both the harmonic fitting (Equation 14; Figure 3A; Table 1) and the detrended calculation (Supplementary Figure SI-1). This value is similar to that observed at the ESTOC site (González-Dávila and Santana-Casiano, 2023), which supports the values observed during these three years in Gando Bay.
Figure 3 Evolution of fCO2 in the atmosphere and ocean (A), the components of the fCO2sw, considering the thermal (fCO2,therm) and non-thermal processes (fCO2,non−therm) (B), the total inorganic carbon (CT) (estimated in red and measured in black) and seawater salinity-normalized inorganic carbon (NCT) at SSS= 36.8 (C), and the pH at total scale, both at in situ temperature (pHT,IS) and at constant temperature of 21°C (pHT,21) (D), in the CanOA-1 site. The lines correspond to the harmonic fit of the data.
Figure 3B shows the decomposition of fCO2sw together with the observed values (black dots) to assess the influence of thermal and non-thermal processes. In this study area, SST appears to control fCO2sw, although the contributions of other physical mixing processes and biological factors should not be overlooked.
Figure 3C shows CT measured with discrete samples and the CT estimated from fCO2 and AT derived from SSS, in the study area. Concentrations decreased from colder to warmer months, with an average concentration of 2113 ± 8 μmol kg-1 (Figure 3C). Maximum CT typically occurred from mid-March to April, averaging 2123 ± 7 μmol kg-1. Minimum concentrations were observed at the end of October, with an average of 2101 ± 3 μmol kg-1. These observations suggest a seasonal amplitude of about 20 μmol kg-1. During the observation period, CT showed an increase of 2.2 ± 0.2 μmol kg-1 yr-1 (Table 1). However, when the CT data were normalized to a constant salinity of 36.8 (NCT = CT/SSS·36.8) (Figure 3C), which removes precipitation and evaporation effects, the rate of increase was reduced to 1.0 ± 0.1 μmol kg-1 yr-1, a value similar to the oceanic ESTOC site (González-Dávila and Santana-Casiano, 2023).
The computed pH measurements, both in situ (pHT,IS) and calculated at a constant temperature of 21°C (pHT,21), are shown in Figure 3D. The pHT,IS displayed a decreasing trend from winter to summer, with a mean value of 8.05 ± 0.02, for the studied period. The maximum pHT,IS was typically recorded between February and March, with a mean of 8.07 ± 0.01, while minimum pHT,IS occurred between September and October, averaging 8.03 ± 0.01. This variation represents a decrease of about 0.04 units from winter to summer. Characterizing a rate of change in a variable such as pH will need an extended time series of data. However, when detrended pH data at ESTOC site (period 1995-2023, Gonzalez-Dávila and Santana-Casiano, 2023) and those at the CanOA-1 site are plotted together (Figure 4), it is shown the coastal pH values are following the same pH trend than that at oceanic waters, this last one decreasing at 0.002 ± 0.0002 units yr-1 (Figure 4; Supplementary Figure SI-1). It is also consistent with other oceanic carbon time series (Bates et al., 2014).
Figure 4 Detrended pH data in total scale measured at ESTOC (in black, González-Dávila and Santana-Casiano, 2023) and CanOA sites (in red).
Examination of the variability of fCO2sw and the corresponding CO2 flux (Figure 5) revealed periods of oversaturation (higher levels of fCO2sw than those in fCO2atm) from May and June to November and December, approximately. During these months, the coastal zone acted as a source of CO2, releasing it from the ocean into the atmosphere. Conversely, during the rest of the year, the coastal zone acted as a carbon sink. It is noteworthy that on average ΔfCO2 is lower during the period June to November than during the period December to June (Figure 3A). However, the highest FCO2 coincided with the June to November period, indicating the effect of the increased wind intensity. In this region, the prevailing Trade Winds exhibit their maximum strength in July and August, with wind speeds reaching 16 m s-1 (Figure 2C). During this period, it is common for wind speeds to consistently exceed 7 m s-1 each year (Santana-Casiano et al., 2007).
Figure 5 CO2 flux (FCO2) in the CanOA-1 site measured with two sensors as described in the experimental section. PrOc referred to the ProOceanus sensor and Batt is to the Battle sensor.
The mean annual flux of FCO2 showed different patterns in the studied years when considered from March to March, with values of 0.70 ± 0.03 mmol m-2 d-1 (2020), -0.22 ± 0.04 mmol m-2 d-1 (in 2021), and 0.55 ± 0.04 mmol m-2 d-1 (in 2022). The average flux from 2020 to 2023 was 0.34 ± 0.04 mmol m-2 d-1 (126 ± 13 mmol m-2 yr-1), acting as a slight CO2 source. The years 2020 and 2022 were registered as CO2 sources with 255 mmol m-2 yr-1 and 202 mmol m-2 yr-1, respectively. Year 2021 acted as a slight sink of 79 mmol m-2 yr-1, related to lower SST in the area during winter months (Figure 2A). When extrapolated to the entire 6 km2 area of Gando Bay (Figure 1), the region acted as a slight CO2 source for the entire 2020-2022 period, quantified at 33 ± 3 tons of CO2 yr-1.
Long-term time series data of CO2 variables in coastal ecosystems are a rare but essential resource for assessing their response to climate change. In particular, time-series data that include at least two carbonate system variables are essential for understanding the underlying processes governing observed trends. In regions close to the coastline, such as the studied area, which has a depth of approximately 10 meters, variables such as wind intensity and direction, SST, and primary production exert certain influence.
In the context of this specific study, it is evident that forcing factors such as strong winds and precipitation events, such as tropical storms Theta (November 2020), Filomena (January 2021), and Hermine (September 2022), have a discernible impact on sea surface salinity (SSS, Figure 2B). Wu et al. (2021) previously demonstrated the influence of extreme events, such as typhoons, on the net atmosphere-ocean CO2 exchange in the East China Sea, due to the water mixing and biological drawdown. In addition, the increase in SST, and also the atmospheric temperature due to extreme events such as heat waves occurring in the Canary Islands (Suárez-Molina and Sanz, 2022) also affects the CO2 system through the dependence of fCO2 on SST. In this sense, the Canary Islands have experienced several heat waves (AEMET, 2023): three in 2021 (11 days, 5 in August and 3 + 3 in September), two in 2022 (3 + 3 in July) and 26 days in 2023 (5 + 5 days in August and 16 days in October).
The study area, a coastal region with a natural barrier in the form of Mountain Gando (Figure 1), approximately 100 meters in height (IDE Canarias - https://visor.grafcan.es/), experiences fluctuations in wind intensity and direction, particularly within a few meters (approximately 50 meters) above the surface. These wind-related variations influence the water circulation patterns within the Gando Bay, resulting in lower SSS (Figure 2B) during periods of strong winds or winds blowing from certain directions. In this regard, a comprehensive analysis of the prevailing winds in the region and the surface water direction (Supplementary Figure SI-2) shows maximum wind speeds of 15 m s-1 and an average of 8.2 ± 2.1 m s-1 throughout the study period. The Trade Winds, which blow from the northeast direction (Van Camp et al., 1991), shift to the north-northwest in the study area due to the sheltering effect of the prominent Gando Mountain, forming a small peninsula that changes the wind direction (Supplementary Figure SI-2). These prevailing winds lead to a surface water column displacement of up to 8 meters in the W-SW direction at times. The calculation of this surface water displacement was achieved using the Ekman Equations (see experimental section).
Consistent findings, as established by Pond and Pickard (1983), confirm that surface currents in the Northern Hemisphere flow at an angle of 45° to the right of the prevailing wind direction. This displacement of surface water instigates the upward movement of deep water to compensate for the lost volume, a phenomenon elucidated by Kämpf and Chapman (2016). The replenishing seawater from deeper areas outside of the bay (about 150 m water depth), exhibits specific characteristics, with an average temperature of 22°C, a mean salinity of 36.4, a substantial CT concentration of 2117 μmol kg-1, and a mean pHT,IS of 8.03 (Curbelo-Hernández et al., 2023). Notably, these values differ from the typical surface conditions in the bay during June-August, which include SST of 23.5°C, SSS reaching up to 36.7, CT concentrations of 2105 μmol kg-1, and a pHT,IS of 8.04. In this sense, it was observed every year that there was no increase in SST from June to September, but the SST was relatively constant or even decreased by July-August consisting with the strongest predominant Trade winds blowing in the area that favored the entrance of deeper water from outside of the bay. Depending on the wind strength and the moment when that force is exerted, it is observed that the increase in SST does not consistently rise but rather slows down due to the arrival of colder water and increased surface mixing. During these periods of decreasing SST, an increase in SSS variability was observed, also related to the arrival of deeper water with lower salinity and pH and higher CT (Figure 2B).
Efforts were made to discern any potential correlation with tidal intensity throughout the lunar period (SupplementaryFigure SI-3). Although some instances of alignment between full and new moon phases and variations in SSS were identified, these occurrences lacked temporal consistency. Consequently, while tidal effects may exert some influence, they do not appear to constitute a primary or determinative factor within this particular environment. Nonetheless, it is worth noting that tidal height plays a crucial role in carbon exchange within estuaries and river mouth regions, as highlighted in previous studies (Ortega et al., 2005; Bauer et al., 2013).
The hydrographic features of Gando Bay are manifested in the seasonal and interannual variability of the CO2 variables. The interannual increase of fCO2sw, quantified at 1.9 µatm yr-1 (Table 1; Figures 3A, B), considering only three years of data is close to the observed value at the oceanic station ESTOC of 2.1 ± 0.1 µatm yr-1 (González-Dávila and Santana-Casiano, 2023), indicating the important control of the increased atmospheric CO2 concentrations in the seawater concentration. Further years of observation are required to confirm this trend in Gando Bay. During the year, thermodynamic effects control the observed variability (Figure 3B) with a T/NT ratio of 2.0 ± 0.1, but other physical and biological effects should not be ignored. From February to November (Figure 3B, black dots), the increase in SST led to an increase in measured fCO2sw (a slope of 10 ± 0.2 μatm °C-1 was calculated). According to Takahashi (1993), the theoretical change should be 17 μatm °C-1 (indicated by the red dots in Figure 3B). The observed lower slope in Gando Bay includes both the effects of the arrival of deeper, less saline water with highly variable fCO2sw content and changes in the productivity of the area (blue dots in Figure 3B). Oxygen data from the sensor (data not shown) were strongly affected by biofouling during the early years of study until a flow system with a copper intake located far from the buoy body was included. However, there is not enough oxygen data to decompose the nonthermal component between biological and water mixing processes. Moreover, there is a seagrass bed (locally known as sebadales) in the vicinity of the buoy which is known for its robust biological activity characteristics (Duarte and Krause-Jensen, 2017; Serrano et al., 2021). As mentioned above, the study area is impacted by the influence of the Trade Winds (wind direction and intensity), and the presence of the nearby mountain, all of which contribute to its unique characteristics, especially during the summer months period. The physical factors should also consider the influence of tides, wind, and horizontal mixing (Xue et al., 2016) on the impact of mixing processes between coastal and open ocean waters, mainly driven by horizontal advection, leading to changes in SSS and productivity, a phenomenon that should also affect the present study.
This outcome differs from the prevailing pattern in coastal regions, where changes in CT due to non-thermal forcings are expected to dominate, especially in mid-latitudes (Cao et al., 2020; Torres et al., 2021). This unexpected result can be attributed to the shallow nature and low productivity of the coastal area studied (Arístegui et al., 2001), which differs from the conditions reported by these authors. Moreover, in the northern region of Gran Canaria (station 16.5°W), research by Curbelo-Hernández et al. (2021) revealed a T/NT ratio of 2.1, comparable to the results obtained in Gando Bay. Therefore, it can be inferred that in Gando Bay, despite the impact of non-thermal processes including encompassing biological activity and advective mixing, the thermal component controls the observed seasonality in the fCO2sw. These climatological results parallel findings from other coastal studies, such as those conducted in Hawaii and Australian regions, where temperature dominantly controls fCO2sw (Shaw and McNeil, 2014; Terlouw et al., 2019). In the northern coastal regions of the Atlantic Ocean, non-thermal processes control the fCO2sw (Gac et al., 2020).
According to the observations, the decrease in pHT,IS at the CanOA-1 site followed the same behavior as that at the oceanic ESTOC site, (Figure 4), and was comparable to other coastal areas such as SOMLIT-Brest, where pH decreased by -0.0026 ± 0.0004 yr-1. The observed diurnal variation is linked with the diel biological cycle, such as in the Bay of Brest and the tidal cycles in Roscoff (Gac et al., 2020, 2021), and was of a similar magnitude to the seasonal variability. These results are consistent with previous studies of coastal seas in NW Europe that estimated ocean acidification based on seasonal cruises or voluntary observing ship surveys (Clargo et al., 2015; Ostle et al., 2016; Omar et al., 2019). Ocean acidification rates in North Sea surface waters ranged from -0.0022 yr-1 (period 2001–2011; Clargo et al., 2015) to -0.0035 yr-1 (period 1984–2014; Ostle et al., 2016), with a recent estimate of -0.0024 yr-1 in the northern North Sea (Omar et al., 2019). When the temperature effect is eliminated, pHT,21 shows an average of 8.05 ± 0.02 and follows an inverse pattern compared to pHT,IS. pHT,21 increases from February to September (mean of 8.07 ± 0.01) related to the increase of biological activity and decreases from September to February (mean of 8.03 ± 0.01) due to vertical mixing with deeper seawater from out of the bay and possible with higher nutrient concentrations. Unfortunately, we did not measure the biological activity, the nutrient concentration in the buoy location and oxygen data are not enough accurate to estimate this component. Despite its status as a coastal zone, the biodiversity in the area appears to be insufficient to absorb excess atmospheric CO2 due to the results of T/NT ratio. Consequently, this leads to acidification levels in the region that are similar to those observed at the ESTOC oceanic station in the Northeast Atlantic, characterized by an interannual variability of -0.002 pH units yr-1 (Santana-Casiano et al., 2007; González-Dávila et al., 2010; González-Dávila and Santana-Casiano, 2023).
The recorded CT with an average of 2113 ± 8 μmol kg-1 (for the study period) were close to the observed concentrations at the ESTOC station for the years 1995-2020 with an average of 2109.5 ± 9.6 μmol kg-1. These concentrations converge if the observed annual CT increase at ESTOC of 1.09 ± 0.10 μmol kg-1 yr-1 is applied (Bates et al., 2014). However, the observed trend of increase in CT over the three-year period studied (2.2 ± 0.4 μmol kg-1 yr-1) is twice that observed in open oceanic waters at ESTOC. When the NCT data are considered (Figure 3C, blue open circles), the trend is reduced to 1 ± 0.1, as that observed in ESTOC. Therefore, we assume that most of the anomalies with respect to the harmonic function are related to the arrival of deeper waters into the area, which could bring more remineralized (positive anomalies) or more productive (negative anomalies) waters. As a result, the NCT does not decrease after the end of March, but keeps relatively constant concentrations until July, when the productivity of the area compensates the physical processes. The detectable seasonal amplitude of 15 μmol kg-1 in NCT after July (Figure 3C) is associated with CO2 consumption by organisms for the production of organic matter and the exchange of CO2 between the atmosphere and the surface layer. In this coastal area, the influence of the Trade Winds seems to be more important due to horizontal advection and seawater renewal in the bay.
The variability of fCO2 in both the atmosphere and seawater describes the studied system as an overall CO2 source. The surface water was undersaturated from late October to mid-June. For the rest of the year, the surface water was supersaturated and the system acted as a source. The calculated mean FCO2 over the study period is 0.35 ± 0.04 mmol m-2 d-1 (126 ± 13 mmol m-2 yr-1), with peak outgassing occurring between August and November, and maximum ingassing observed between February and March. This shift from a sink to a source role coincides temporally with the onset of Trade Winds, which typically blow at high and constant velocity in the Canary Islands from mid-June. This climatology is consistent with previous coastal studies, such as those conducted in the Hawaiian region, where the influence of Trade Winds is prominent and affects the CO2 system in coastal waters (Terlouw et al., 2019). The occurrence and strength of the dominant northeast and east Trade Winds between 1973 and 2009 have been previously studied (Garza et al., 2012) and reported velocities ranging from 0.8 to 8.2 m s-1, with summer being the period of higher intensity. Furthermore, the significance of winds and mixing processes becomes clear when comparing these results with coastal studies along the East Australian coast, where mixing processes are relatively low (McNeil, 2010; Shaw and McNeil, 2014), or along the Northwest coast of the North Atlantic Ocean, where coastal systems act as CO2 sinks primarily due to SST effects at higher latitudes (Boehme et al., 1998).
The FCO2 results here are comparable to other coastal stations such as in Brest (France, Gac et al., 2020) and Hawaii (Terlouw et al., 2019). In Brest, at higher latitudes than the Canary Islands, the authors estimated fluxes of 0.18 ± 0.10, 0.11 ± 0.12, and 0.39 ± 0.08 mol m–2 yr–1 in three coastal stations. In Hawaii, it is also important to highlight how the coastal areas could be a strong source of CO2 from the ocean with 1.24 ± 0.33 mol m−2 yr−1, and close to the equilibrium with 0.05 ± 0.02 and 0.00 ± 0.03 mol m−2 yr−1 at the other two coastal stations. In the coastal waters of the Mediterranean Sea, the coastal waters of the Gulf of Trieste act as a CO2 sink in winter, especially in the presence of strong wind events (FCO2 up to −11.9 mmol m−2 d−1; Cantoni et al., 2012; Ingrosso et al., 2016). In the Gando Bay region, with a net annual outgassing flux of 0.26 mol m-2 yr-1 (period 2020-2022), with values of 0.20 mol m-2 yr-1 for the years 2020 and 2022, but ingassing CO2 at -0.08 mol m-2 yr-1 for the year 2021 due to lower winter SST values, the influence of Trade Winds is responsible for a temporary increase in fluxes, causing the mean values to remain positive (0.13 ± 0.01 mol m-2 yr-1), a phenomenon also observed at the ESTOC station (González-Dávila et al., 2003; Santana-Casiano et al., 2007; González-Dávila and Santana-Casiano, 2023). Additionally, Curbelo-Hernández et al. (2021) reported an average FCO2 of -0.25 ± 0.04 mol m-2 yr-1 for the oceanic waters near Gran Canaria, which is consistent with the behavior observed in the Northeast Atlantic Ocean (-0.6 mol m-2 yr-1) (Takahashi et al., 2009).
Considering the mean flux determined at the coastal buoy site within Gando Bay (6 km2), a net annual outgassing flux of 33 ± 4 Tons of CO2 per year through the bay is calculated, which is close to equilibrium. If we consider the values determined in the open ocean waters of the Canary Islands (Curbelo-Hernández et al., 2021), where a CO2 sink of -0.24 ± 0.04 mol m-2 yr-1 was calculated, that is, -170 Tons of CO2 yr-1 for the entire coastal area of the Canary Islands. Coastal areas located in the easternmost part of the archipelago are affected by the arrival of Northwest African coastal waters (Curbelo-Hernández et al., 2021). The Gando Bay, with an area of only 6 km2, causes the amount of CO2 absorbed by the Canary region to decrease by 9%.
Coastal regions at lower latitudes typically act as sources of CO2 to the atmosphere, while high latitudes tend to act as sinks (Chen, 2004; Cao et al., 2020). Notably, the boundary for this behavior typically occurs around 30°N (Cai et al., 2006), placing the study area in the transition zone between low and high latitudes. Consequently, it is consistent with the result that this coastal area of the Canary Islands acts as a source while it is almost in equilibrium. The flux depends on several factors such as SST, ΔfCO2, and wind speed. This makes different mixing processes and biological activity in both the coastal and open ocean environments crucial influencers of the carbonate system parameters and controllers of the CO2 air-sea exchange. In the case of this study area, it remains relatively unaffected by rivers, agriculture, or other anthropogenic activities that could disrupt the biological activity and the physical processes that control the carbonate system in the region.
The findings of this research clearly emphasize the need to explore additional coastal areas, since the hydrodynamic and CO2 system-altering phenomena exhibit pronounced locality. Their effects vary from one area to another. In addition, studies should also extend the observations for at least 10 years to allow more accurate estimates of rates of change.
The coastal zones of islands require vigilant monitoring to accurately quantify the carbon balance and its consequences as an essential tool for effective governance. The economic well-being of these islands is significantly intertwined with the health of their coastal zones.
Within the Canary Islands, the CanOA-1 station, an integral part of the international GOA-ON network, is located in the eastern region of Gran Canaria, specifically in the Bay of Gando. Data from this station show a discernible seasonal pattern in the variables defining the CO2 system. This study highlights the importance of physical processes, especially horizontal mixing, biological influences (hypothesis because no biological data are collected), and sea surface temperature, in facilitating the transfer of CO2 from the atmosphere to the ocean.
The three-year data allow us to know that the sea surface temperature (SST) shows seasonal fluctuations, with the highest temperatures occurring in September-October and the lowest in March. The contributions of both thermal and non-thermal processes to the seasonal fCO2 were investigated with vertical mixing, wind stress, and biological forcing as principal components. The T/NT ratio of 2.0 ± 0.1 implies that SST plays a controlling role in modulating fCO2sw, although the influence of other factors should not be neglected. fCO2sw increases at a rate of 1.91 µatm yr-1, which is consistent with the observed increase in NCT in these coastal waters. The CO2 transfer from the atmosphere to the surface waters causes a decrease of pHT,IS in the region. Furthermore, the concentration of total inorganic carbon (NCT) showed an annual increase of 1.0 μmol kg-1 yr-1 in the surface waters of the bay. The occurrence of extreme events, such as tropical storms, and the most persistent Trade Winds direction, which are modified by the geological structure of the bay, affect the physico-chemical properties of the region, renewing the bay waters with deeper seawater, affecting SSS, pH, fCO2, and CT.
According to the data collected at the CanOA-1 site, the Gando Bay is almost in equilibrium, with a total CO2 release from the ocean to the atmosphere between 2020 and 2023, of 33 ± 4 Tons of CO2 yr-1, with the year 2021 acting as a slight sink. Small changes in SST between years, together with variability related to the prevailing strength of the Trade Winds, which affect both water exchange and CO2 fluxes, control the Gando Bay site. This underscores the critical importance of continuous monitoring and quantification of CO2 concentrations in seawater, especially in coastal regions and on islands worldwide, in order to estimate the CO2 contribution of coastal regions to the global ocean.
The raw data supporting the conclusions of this article will be made available by the authors, without undue reservation.
AG: Data curation, Formal analysis, Funding acquisition, Investigation, Writing – original draft. AA-R: Formal analysis, Writing – review & editing. DG-S: Formal analysis, Investigation, Writing – review & editing. MG-D: Conceptualization, Formal analysis, Funding acquisition, Investigation, Writing – review & editing. JC: Formal analysis, Funding acquisition, Investigation, Writing – review & editing.
The author(s) declare that financial support was received for the research, authorship, and/or publication of this article. This study was supported by the Government of the Canary Islands the Loro Parque Foundation through the CanBIO project, CanOA subproject (2019-2023) and the CARBOCAN agreement (Consejería de Transición Ecológica, Lucha contra el Cambio Climático y Planificación Territorial, Gobierno de Canarias). Discrete sampling and maintenance were also supported by the FeRIA project (PID2021-123997NB-100), the Spanish Ministerio de Ciencia e Innovación.
Special thanks go to the Mando Aéreo de Canarias (MACAN), its officers and all the members of the Base Aérea de Gando, and to all the marine supporting members for providing support (personnel and boats), assistance and surveillance.
The authors declare that the research was conducted in the absence of any commercial or financial relationships that could be construed as a potential conflict of interest.
All claims expressed in this article are solely those of the authors and do not necessarily represent those of their affiliated organizations, or those of the publisher, the editors and the reviewers. Any product that may be evaluated in this article, or claim that may be made by its manufacturer, is not guaranteed or endorsed by the publisher.
The Supplementary Material for this article can be found online at: https://www.frontiersin.org/articles/10.3389/fmars.2024.1337929/full#supplementary-material
AEMET (2023). “Olas de calor en España desde 1975,” in Área de Climatología y Aplicaciones Operativas. Spain: AEMET. Available at: https://www.aemet.es/es/conocermas/recursos_en_linea/publicaciones_y_estudios/estudios/detalles/olascalor.
Arístegui J., Hernández-León S., Montero M. F., Gómez M. (2001). The seasonal planktonic cycle in coastal waters of the Canary Islands. Scientia Marina 65, 51–58. doi: 10.3989/scimar.2001.65s151
Bates N. R. (2012). Multi-decadal uptake of carbon dioxide into subtropical mode water of the North Atlantic Ocean. Biogeosciences 9, 2649–2659. doi: 10.5194/bg-9-2649-2012
Bates N. R., Astor Y. M., Church M. J., Currie K., Dore J. E., González-Dávila M., et al. (2014). A time-series view of changing surface ocean chemistry due to ocean uptake of anthropogenic CO2 and ocean acidification. Oceanography 27, 126–141. doi: 10.5670/oceanog.2014.16
Bates N. R., Johnson R. J. (2020). Acceleration of ocean warming, salinification, deoxygenation and acidification in the surface subtropical North Atlantic Ocean. Commun. Earth Environ. 1, 33. doi: 10.1038/s43247-020-00030-5
Bauer J. E., Cai W. J., Raymond P. A., Bianchi T. S., Hopkinson C. S., Regnier P. A. G. (2013). The changing carbon cycle of the coastal ocean. Nature 504, 61–70. doi: 10.1038/nature12857
Boehme S. E., Sabine C. L., Reimers C. E. (1998). CO2 fluxes from a coastal transect: A time-series approach. Mar. Chem. 63, 49–67. doi: 10.1016/S0304-4203(98)00050-4
Borges A. V. (2005). Do we have enough pieces of the jigsaw to integrate CO2 fluxes in the coastal ocean? Estuaries 28, 3–27. doi: 10.1007/BF02732750
Borges A. V., Delille B., Frankignoulle M. (2005). Budgeting sinks and sources of CO2 in the coastal ocean: Diversity of ecosystem counts. Geophys. Res. Lett. 32, 1–4. doi: 10.1029/2005GL023053
Borges A. V., Frankignoulle M. (2002). Distribution of surface carbon dioxide and air-sea exchange in the upwelling system off the Galician coast. Global Biogeochem. Cycles 16, 13–11. doi: 10.1029/2000GB001385
Borges A. V., Gypensb N. (2010). Carbonate chemistry in the coastal zone responds more strongly to eutrophication than ocean acidification. Limnol. Oceanogr. 55, 346–353. doi: 10.4319/lo.2010.55.1.0346
Borges A. V., Schiettecatte L. S., Abril G., Delille B., Gazeau F. (2006). Carbon dioxide in European coastal waters. Estuarine Coast. Shelf Sci. 70, 375–387. doi: 10.1016/j.ecss.2006.05.046
Bouillon S., Borges A. V., Castañeda-Moya E., Diele K., Dittmar T., Duke N. C., et al. (2008). Mangrove production and carbon sinks: a revision of global budget estimates. Global biogeochem. cycles 22, 1–12. doi: 10.1029/2007GB003052
Cai W. J. (2011). Estuarine and coastal ocean carbon paradox: CO2 sinks or sites of terrestrial carbon incineration? Annu. Rev. Mar. Sci. 3, 123–145. doi: 10.1146/annurev-marine-120709-142723
Cai W. J., Dai M., Wang Y. (2006). Air-sea exchange of carbon dioxide in ocean margins: A province-based synthesis. Geophys. Res. Lett. 33, 1–4. doi: 10.1029/2006GL026219
Cantoni C., Luchetta A., Celio M., Cozzi S., Raicich F., Catalano G. (2012). Carbonate system variability in the gulf of Trieste (north Adriatic sea). Estuarine Coast. Shelf Sci. 115, 51–62. doi: 10.1016/j.ecss.2012.07.006
Cao Z., Yang W., Zhao Y., Guo X., Yin Z., Du C., et al. (2020). Diagnosis of CO2 dynamics and fluxes in global coastal oceans. Natl. Sci. Rev. 7, 786–797. doi: 10.1093/nsr/nwz105
Carstensen J., Duarte C. M. (2019). Drivers of pH variability in coastal ecosystems. Environ. Sci. Technol. 53, 4020–4029. doi: 10.1021/acs.est.8b03655
Chen C. A. (2004). Exchanges of carbon in the coastal seas. Eds. Field C. B., Raupach M. R. SCOPE. Washington DC: Island Press, 62.
Chen C. T. A., Borges A. V. (2009). Reconciling opposing views on carbon cycling in the coastal ocean: Continental shelves as sinks and near-shore ecosystems as sources of atmospheric CO2. Deep Sea Res. Part II: Topical Stud. Oceanogr. 56, 578–590. doi: 10.1016/j.dsr2.2009.01.001
Chen C. A., Huang T. H., Chen Y. C., Bai Y., He X., Kang Y. (2013). Air-sea exchanges of the coin the world’s coastal seas. Biogeosciences 10, 6509–6544. doi: 10.5194/bg-10-6509-2013
Clargo N. M., Salt L. A., Thomas H., De Baar H. J. (2015). Rapid increase of observed DIC and pCO2 in the surface waters of the North Sea in the 2001-2011 decade ascribed to climate change superimposed by biological processes. Mar. Chem. 177, 566–581. doi: 10.1016/j.marchem.2015.08.010
Curbelo-Hernández D., González-Dávila M., González A. G., González-Santana D., Santana-Casiano J. M. (2021). CO2 fluxes in the Northeast Atlantic Ocean based on measurements from a surface ocean observation platform. Sci. Total Environ. 775, 145804. doi: 10.1016/j.scitotenv.2021.145804
Curbelo-Hernández D., González-Dávila M., Santana-Casiano J. M. (2023). The carbonate system and air-sea CO2 fluxes in coastal and open-ocean waters of the Macaronesia. Front. Mar. Sci. 10, 1094250. doi: 10.3389/fmars.2023.1094250
de Haas H., van Weering T. C., de Stigter H. (2002). Organic carbon in shelf seas: sinks or sources, processes and products. Continent. Shelf Res. 22, 691–717. doi: 10.1016/S0278-4343(01)00093-0
Denman K. L., Brasseur G. P., Ciais P., Cox P. M. (2007) Couplings between changes in the climate system and biogeochemistry. Available online at: https://www.researchgate.net/publication/224942928.
Dickson G. (1990). The standard potential of the reaction: AgCl(s) + 1 2H2(g) = Ag(s) + HCl(aq), and the standard acidity constant of the ion HSO4- in synthetic seawater from 273.15 to 318.15 K. J. Chem. Thermodynam. 22, 113–127. doi: 10.1016/0021-9614(90)90074-Z
Dickson A. G., Sabine C. L., Christian J. R., (eds) (2007). Guide to best practices for ocean CO2 measurements. (Sidney, British Columbia: North Pacific Marine Science Organization), 191. (PICES Special Publication 3; IOCCP Report 8). doi: 10.25607/OBP-1342
Doney S. C., Fabry V. J., Feely R. A., Kleypas J. A. (2009). Ocean acidification: The other CO2 problem. Annu. Rev. Mar. Sci. 1, 169–192. doi: 10.1146/annurev.marine.010908.163834
Duarte C. M., Krause-Jensen D. (2017). Export from seagrass meadows contributes to marine carbon sequestration. Front. Mar. Sci. 4. doi: 10.3389/fmars.2017.00013
Falkowski P. G., Wilson C. (1992). Phytoplankton productivity in the North Pacific ocean since 1900 and implications for absorption of anthropogenic CO2. Nature 358, 741–743. doi: 10.1038/358741a0
Friedlingstein P., O’Sullivan M., Jones M. W., Andrew R. M., Gregor L., Hauck J., et al. (2022). Global carbon budget 2022. Earth Syst. Sci. Data 14, 4811–4900. doi: 10.5194/essd-14-4811-2022
Friedlingstein P., O’Sullivan M., Jones M. W., Andrew R. M., Hauck J., Olsen A., et al. (2020). Global carbon budget 2020. Earth Sys. Sci. Data 12, 3269–3340. doi: 10.5194/essd-12-3269-2020
Gac J. P., Marrec P., Cariou T., Grosstefan E., Macé É., Rimmelin-Maury P., et al. (2021). Decadal dynamics of the CO2 system and associated ocean acidification in coastal ecosystems of the north east Atlantic Ocean. Front. Mar. Sci. 8. doi: 10.3389/fmars.2021.688008
Gac J. P., Marrec P., Cariou T., Guillerm C., Macé É., Vernet M., et al. (2020). Cardinal buoys: an opportunity for the study of air-sea CO2 fluxes in coastal ecosystems. Front. Mar. Sci. 7. doi: 10.3389/fmars.2020.00712
Garza J. A., Chu P. S., Norton C. W., Schroeder T. A. (2012). Changes of the prevailing trade winds over the islands of Hawaii and the North Pacific. J. Geophys. Res.: Atmos. 117, 1–18. doi: 10.1029/2011JD016888
Gattuso J. P., Frankignoulle M., Wollast R. (1998). Carbon and carbonate metabolism in coastal aquatic ecosystems. Annu. Rev. Ecol. System. 29, 405–434. doi: 10.1146/annurev.ecolsys.29.1.405
González-Dávila M., Santana-Casiano J. M. (2023). Long-term trends of pH and inorganic carbon in the Eastern North Atlantic: the ESTOC site. Front. Mar. Sci. 10. doi: 10.3389/fmars.2023.1236214
González-Dávila M., Santana-Casiano J. M., González-Dávila E. F. (2007). Interannual variability of the upper ocean carbon cycle in the northeast Atlantic Ocean. Geophys. Res. Lett. 34, 1–7. doi: 10.1029/2006GL028145
González Dávila M., Santana-Casiano J. M., Merlivat L., Barbero-Muñoz L., Dafner E. V. (2005). Fluxes of CO2 between the atmosphere and the ocean during the POMME project in the northeast Atlantic Ocean during 2001. J. Geophys. Res. C: Oceans 110, 1–14. doi: 10.1029/2004JC002763
González-Dávila M., Santana-Casiano J. M., Rueda M. J., Llinás O. (2010). The water column distribution of carbonate system variables at the ESTOC site from 1995 to 2004. Biogeosciences 7, 3067–3081. doi: 10.5194/bg-7-3067-2010
González-Dávila M., Santana-Casiano J. M., Rueda M. J., Llinás O., González‐Dávila E. F. (2003). Seasonal and interannual variability of sea‐surface carbon dioxide species at the European Station for Time Series in the Ocean at the Canary Islands (ESTOC) between 1996 and 2000. Global Biogeochem. Cycles 17 (3).
Granéli W., Granéli E. (1991). Automatic potentiometric determination of dissolved oxygen. Mar. Biol. 108, 341–348. doi: 10.1007/BF01344349
Gypens N., Borges A. V., Lancelot C. (2009). Effect of eutrophication on air–sea CO2 fluxes in the coastal Southern North Sea: a model study of the past 50 years. Global Change Biol. 15, 1040–1056. doi: 10.1111/j.1365-2486.2008.01773.x
Gypens N., Lacroix G., Lancelot C., Borges A. V. (2011). Seasonal and inter-annual variability of air–sea CO2 fluxes and seawater carbonate chemistry in the Southern North Sea. Prog. oceanogr. 88, 59–77. doi: 10.1016/j.pocean.2010.11.004
Hsu S. A., Meindl E. A., Gilhousen D. B. (1994). Determining the power-law wind-profile exponent under near-neutral stability conditions at sea. J. Appl. Meteorol. Climatol. 33, 757–765. doi: 10.1175/1520-0450(1994)033<0757:DTPLWP>2.0.CO;2
Ingrosso G., Giani M., Comici C., Kralj M., Piacentino S., De Vittor C., et al. (2016). Drivers of the carbonate system seasonal variations in a Mediterranean gulf. Estuarine Coast. Shelf Sci. 168, 58–70. doi: 10.1016/j.ecss.2015.11.001
IPCC (2022). “Climate Change 2022: Impacts, Adaptation, and Vulnerability,” in Contribution of Working Group II to the Sixth Assessment Report of the Intergovernmental Panel on Climate Change. Eds. Pörtner H.-O., Roberts D. C., Tignor M., Poloczanska E. S., Mintenbeck K., Alegría A., Craig M., Langsdorf S., Löschke S., Möller V., Okem A., Rama B. (Cambridge University Press, Cambridge, UK and New York, NY, USA). 3056 pp. doi: 10.1017/9781009325844
Jiang L. Q., Dunne J., Carter B. R., Tjiputra J. F., Terhaar J., Sharp J. D., et al. (2023). Global surface ocean acidification indicators from 1750 to 2100. J. Adv. Model. Earth Syst. 15, e2022MS003563. doi: 10.1029/2022MS003563
Kämpf J., Chapman P. (2016). “Upwelling Systems of the World,” in Upwelling Systems of the World. Switzerland: Springer Cham. doi: 10.1007/978-3-319-42524-5
Knoll M., Hernández-Guerra A., Lenz B., López Laatzen F., Machín F., Müller T. J., et al. (2002). The eastern boundary current system between the Canary islands and the African coast. Deep-Sea Res. Part II: Topical Stud. Oceanogr. 49, 3427–3440. doi: 10.1016/S0967-0645(02)00105-4
Laruelle G. G., Dürr H. H., Lauerwald R., Hartmann J., Slomp C. P., Goossens N., et al. (2013). Global multi-scale segmentation of continental and coastal waters from the watersheds to the continental margins. Hydrol. Earth Sys. Sci. 17, 2029–2051. doi: 10.5194/hess-17-2029-2013
Lee K., Kim T. W., Byrne R. H., Millero F. J., Feely R. A., Liu Y. M., et al (2010). The universal ratio of boron to chlorinity for the North Pacific and North Atlantic oceans. Geochimica et Cosmochimica Acta 74 (6), 1801–1811.
Lueker T. J., Dickson A. G., Keeling C. D. (2000). Ocean pCO2 calculated from dissolved inorganic carbon, alkalinity, and equations for K1 and K2: Validation based on laboratory measurements of CO2 in gas and seawater at equilibrium. Mar. Chem. 70, 105–119. doi: 10.1016/S0304-4203(00)00022-0
Lynas M., Houlton B. Z., Perry S. (2021). Greater than 99% consensus on human caused climate change in the peer-reviewed scientific literature. Environ. Res. Lett. 16, 114005. doi: 10.1088/1748-9326/ac2966
McNeil B. I. (2010). Diagnosing coastal ocean CO2 interannual variability from a 40-year hydrographic time-series station off the east coast of Australia. Global Biogeochem. Cycles 24, 1–17. doi: 10.1029/2010GB003870
Mintrop L., Pérez F. F., González-Dávila M., Santana-Casiano J. M., Körtzinger A. (2000). Alkalinity determination by potentiometry: Intercalibration using three different methods. Cienc. Marinas 26, 23–37. doi: 10.7773/cm.v26i1.573
Omar A. M., Thomas H., Olsen A., Becker M., Skjelvan I., Reverdin G. (2019). Trends of ocean acidification and pCO2 in the northern North Sea 2003–2015. J. Geophys. Res.: Biogeosci. 124, 3088–3103. doi: 10.1029/2018JG004992
Orr J. C., Fabry V. J., Aumont O., Bopp L., Doney S. C., Feely R. A., et al. (2005). Anthropogenic ocean acidification over the twenty-first century and its impact on calcifying organisms. Nature 437, 681–686. doi: 10.1038/nature04095
Ortega T., Ponce R., Forja J., Gómez-Parra A. (2005). Fluxes of dissolved inorganic carbon in three estuarine systems of the Cantabrian Sea (north of Spain). J. Mar. Syst. 53, 125–142. doi: 10.1016/j.jmarsys.2004.06.006
Ostle C., Willliamson P., Artioli Y., Bakker D. C., Birchenough S. N. R., Davis C. E., et al. (2016). Carbon dioxide and ocean acidification observations in UK waters. Synthesis report with a focus on 2010–2015. doi: 10.13140/RG.2.1.4819.4164
Pierrot D., Epitalon J.-M., Orr J. C., Lewis E., Wallace D. W. R. (2021). Excel program developed for CO2 system calculations – version 3.0. GitHub repository.
Pond S., Pickard G. (1983). Introductory dynamical oceanography. 2nd ed (Oxford: Pergramon Press), 9–159.
Santana-Casiano J. M., González-Dávila M., Rueda M. J., Llinás O., González-Dávila E. F. (2007). The interannual variability of oceanic CO2 parameters in the northeast Atlantic subtropical gyre at the ESTOC site. Global Biogeochem. Cycles 21, 1–16. doi: 10.1029/2006GB002788
Serrano O., Gómez-López D. I., Sánchez-Valencia L., Acosta-Chaparro A., Navas-Camacho R., González-Corredor J., et al. (2021). Seagrass blue carbon stocks and sequestration rates in the Colombian Caribbean. Sci. Rep. 11, 11067. doi: 10.1038/s41598-021-90544-5
Shaw E. C., McNeil B. I. (2014). Seasonal variability in carbonate chemistry and air-sea CO2 fluxes in the southern Great Barrier Reef. Mar. Chem. 158, 49–58. doi: 10.1016/j.marchem.2013.11.007
Skjelvan I., Lauvset S. K., Johannessen T., Gundersen K., Skagseth Ø. (2022). Decadal trends in ocean acidification from the Ocean Weather Station M in the Norwegian Sea. J. Mar. Syst. 234, 103775. doi: 10.1016/j.jmarsys.2022.103775
Suárez-Molina D., Sanz R. (2022). Episodios cálidos y olas de calor en Canarias durante los meses de junio a septiembre (JJAS) de 2021. Rev. Tiempo y Clima 5, 13–15.
Takahashi T., Olafsson J., Goddard J. G., Chipman D. W., Sutherland S. C. (1993). Seasonal variation of CO2 and nutrients in the high‐latitude surface oceans: A comparative study. Global Biogeochem. Cycles 7 (4), 843–878. doi: 10.1029/93GB02263
Takahashi T., Sutherland S. C., Chipman D. W., Goddard J. G., Ho C., Newberger T., et al. (2014). Climatological distributions of pH, pCO2, total CO2, alkalinity, and CaCO3 saturation in the global surface ocean, and temporal changes at selected locations. Mar. Chem. 164, 95–125. doi: 10.1016/j.marchem.2014.06.004
Takahashi T., Sutherland S. C., Sweeney C., Poisson A., Metzl N., Tilbrook B., et al. (2002). Global sea-air CO2 flux based on climatological surface ocean pCO2, and seasonal biological and temperature effects. Deep-Sea Res. Part II: Topical Stud. Oceanogr. 49, 1601–1622. doi: 10.1016/S0967-0645(02)00003-6
Takahashi T., Sutherland S. C., Wanninkhof R., Sweeney C., Feely R. A., Chipman D. W., et al. (2009). Climatological mean and decadal change in surface ocean pCO2, and net sea-air CO2 flux over the global oceans. Deep-Sea Res. Part II: Topical Stud. Oceanogr. 56, 554–577. doi: 10.1016/j.dsr2.2008.12.009
Terlouw G. J., Knor L. A. C. M., De Carlo E. H., Drupp P. S., Mackenzie F. T., Li Y. H., et al. (2019). Hawaii coastal seawater CO2 Network: A statistical evaluation of a decade of observations on tropical Coral Reefs. Front. Mar. Sci. 6. doi: 10.3389/fmars.2019.00226
Thomas H., Bozec Y., Elkalay K., De Baar H. J. W., Borges A. V., Schiettecatte L. S. (2005). Controls of the surface water partial pressure of CO2 in the North Sea. Biogeosciences 2, 323–334. doi: 10.5194/bg-2-323-2005
Torres O., Kwiatkowski L., Sutton A. J., Dorey N., Orr J. C. (2021). Characterizing mean and extreme diurnal variability of ocean CO2 system variables across marine environments. Geophys. Res. Lett. 48, 1–12. doi: 10.1029/2020GL090228
Van Camp L., Nykjaer L., Mittelstaedt E., Schlittenhardt P. (1991). Upwelling and boundary circulation off Northwest Africa as depicted by infrared and visible satellite observations. Prog. Oceanogr. 26, 357–402. doi: 10.1016/0079-6611(91)90012-B
Wallace R. B., Baumann H., Grear J. S., Aller R. C., Gobler C. J. (2014). Coastal ocean acidification: The other eutrophication problem. Estuarine Coast. Shelf Sci. 148, 1–13. doi: 10.1016/j.ecss.2014.05.027
Walsh J. J. (1991). Importance of continental margins in the marine biogeochemical cycling of carbon and nitrogen. Nature 350, 53–55. doi: 10.1038/350053a0
Walsh J. J., Biscaye P. E., Csanady G. T. (1988). The 1983–1984 shelf edge exchange processes (SEEP)—I experiment: hypotheses and highlights. Continent. Shelf Res. 8, 435–456. doi: 10.1016/0278-4343(88)90063-5
Walsh J. J., Dieterle D. A. (1994). CO2 cycling in the coastal ocean. I–A numerical analysis of the southeastern Bering Sea with applications to the Chukchi Sea and the northern Gulf of Mexico. Prog. Oceanogr. 34, 335–392. doi: 10.1016/0079-6611(94)90019-1
Wanninkhof R. (2014). Relationship between wind speed and gas exchange over the ocean revisited. Limnol. Oceanogr. 12, 351–362. doi: 10.4319/lom.2014.12.351
Weiss R. F. (1970). The solubility of nitrogen, oxygen and argon in water and seawater. Deep-Sea Res. Oceanogr. Abst. 17, 721–735. doi: 10.1016/0011-7471(70)90037-9
Wollast R., Mackenzie F. T. (1989). “Global biogeochemical cycles and climate,” in Climate and Geo-Sciences: A Challenge for Science and Society in the 21st Century (Springer Netherlands, Dordrecht), 453–473.
Wu Y., Dai M., Guo X., Chen J., Xu Y., Dong X., et al. (2021). High-frequency time-series autonomous observations of sea surface pCO2 and pH. Limnol. Oceanogr. 66, 588–606. doi: 10.1002/lno.11625
Keywords: CO2 observations, coastal waters, times-series, Canary Islands, acidification
Citation: González AG, Aldrich-Rodríguez A, González-Santana D, González-Dávila M and Santana-Casiano JM (2024) Seasonal variability of coastal pH and CO2 using an oceanographic buoy in the Canary Islands. Front. Mar. Sci. 11:1337929. doi: 10.3389/fmars.2024.1337929
Received: 13 November 2023; Accepted: 11 March 2024;
Published: 05 April 2024.
Edited by:
Abed El Rahman Hassoun, Helmholtz Association of German Research Centres (HZ), GermanyReviewed by:
Constantin Frangoulis, Hellenic Centre for Marine Research (HCMR), GreeceCopyright © 2024 González, Aldrich-Rodríguez, González-Santana, González-Dávila and Santana-Casiano. This is an open-access article distributed under the terms of the Creative Commons Attribution License (CC BY). The use, distribution or reproduction in other forums is permitted, provided the original author(s) and the copyright owner(s) are credited and that the original publication in this journal is cited, in accordance with accepted academic practice. No use, distribution or reproduction is permitted which does not comply with these terms.
*Correspondence: Aridane G. González, YXJpZGFuZS5nb256YWxlekB1bHBnYy5lcw==; J. Magdalena Santana-Casiano, bWFnZGFsZW5hLnNhbnRhbmFAdWxwZ2MuZXM=
Disclaimer: All claims expressed in this article are solely those of the authors and do not necessarily represent those of their affiliated organizations, or those of the publisher, the editors and the reviewers. Any product that may be evaluated in this article or claim that may be made by its manufacturer is not guaranteed or endorsed by the publisher.
Research integrity at Frontiers
Learn more about the work of our research integrity team to safeguard the quality of each article we publish.