- 1Institute for Advanced Marine Research, China University of Geosciences, Guangzhou, China
- 2State Key Laboratory of Biogeology and Environmental Geology, China University of Geosciences, Wuhan, China
- 3Southern Marine Science and Engineering Guangdong Laboratory (Zhuhai), Zhuhai, China
- 4Research Centre for Indian Ocean Ecosystem, Tianjin University of Science and Technology, Tianjin, China
The relationship between Chrysolia dentata and bacteria that play an important role in the carbon cycle is complex yet closely intertwined. However, there is still limited knowledge about how phytoplankton interacts with heterotrophic bacteria under external influences. The transformation and processing of dissolved organic matter (DOM) by epiphytic bacteria for phytoplankton especially, under vitamin B12 (VB12) and abscisic acid (ABA) introducing have rarely been investigated. In this experiment, Marinobacter hydrocarbonoclasticus (CA6) was cultured with Chrysotila dentata to investigate growth and DOM release and transformation under the influence of VB12 and ABA. Three-dimensional (3D)-EEM, combined with fluorescence regional integration and the peak selecting method, was used to obtain fluorescent peaks (B, T, A, and C) and four indexes for DOM analysis. Our findings indicate that the incubation of CA6 significantly enhances the release of protein-like components (peak T) and humic-like components (peak C) during the incubation period. However, 100 μg/L VB12 or abscisic acid does not promote axenic microalgae growth or release of C. dentata-derived DOM. However, they have different effects on C. dentata-CA6 growth and the derived DOM. Abscisic acid can facilitate steady growth of both C. dentata and CA6 cells while enhancing the amounts of humic-like components. Conversely, VB12 inhibits the growth of CA6 and results in a rapid decrease in protein-like signal, but it does not significantly enhance C. dentata growth or the transformation of DOM. This indicates that ABA can support stable co-grown of microalgal and bacteria in a water environment, while VB12 may hinder CA6 bacterial growth, resulting in a less stable co-cultured environment. This study uncovers and verifies the impact of exogenous factors and heterotrophic bacteria in the growth of microalgae, underlining their role in transforming and generating algae-derived dissolved organic matter (DOM) within laboratory settings.
1 Introduction
Dissolved organic matter (DOM), which is composed of a mixture of different molecular weights, stores 700 billion tonnes in the global ocean and represents one of the largest reservoirs of organic carbon in the global carbon cycle (Hansell et al., 2012). DOM is transformed by marine microheterotrophs, which are associated with phytoplankton metabolism, degradation by heterotrophic bacteria, chemoautotrophy by archaea, feeding and excretion by zooplankton, and viral lysis. This leads to the emergence of recalcitrance on the ecosystems level (Seymour et al., 2017; Dittmar et al., 2021). Among these processes, microalgal metabolism constitutes the primary source of unstable DOM that is subsequently converted into other unstable or recalcitrant forms through enzymatic reactions catalyzed by heterotrophic bacteria. The fraction of DOM capable of absorbing and emitting fluorescence corresponds to chromophoric dissolved organic matter (CDOM) (Uusikivi et al., 2010; Zhang et al., 2010). CDOM in the ocean typically consists of protein-like fluorescent substances and humic-like fluorescent substances (Coble, 2007). Marine protein-like compounds are mainly observed as tryptophan-like and tyrosine-like compounds, which are associated with the metabolism of phytoplankton and bacteria (Nieto-Cid et al., 2005). The production of humic-like compounds is associated with microbial oxidation, organic matter degradation, as well as UV and blue light (Stedmon and Markager, 2005).
The metabolic processes of microalgae living in the aquatic environment release various compounds into their surroundings, such as carbohydrates, amino acids, polysaccharides, proteins, nucleic acids, and other metabolites that alter the chemical environment in their vicinity (Seymour et al., 2017). These stimulate heterotrophic bacteria to colonize the inter-algal environment. In turn, bacteria also release trace elements, vitamins, chelators, etc., creating a favorable environment for microalgae (Seymour et al., 2017). Heterotrophic bacteria can utilize and transform up to 50% of the carbon fixed by phytoplankton (Fuhrman and Azam, 1982). Additionally, Heterotrophic bacteria can consume individual molecules from unstable DOM (LDOM), as well as fragments of DOM modified by extracellular enzymes from bacteria. At the same time, bacteria and microalgae jointly control the process of transforming some of the refractory DOM (RDOM) organic molecules, which may be affected by photodegradation. RDOM is then conserved in the ocean as part of the carbon cycle. Additionally, studies have shown that amino acid uptake at the marine surface is dominated by α-Amastigotes phylum and at higher concentrations γ-Amastigotes and Bacteroides phylum also utilize amino acids (Alonso-Sáez et al., 2007). In summary, microbial-algal interactions play a significant role in global biogeochemical cycling of carbon, nitrogen and phosphorus.
Chrysotila dentata, a calcified protozoan belonging to the phylum Staphylinidae, is approximately 13-15 μm in size. It produces a complex exoskeleton of calcium carbonate scales and plays a significant role as a major marine producer and phytoplankton community in the ocean (Balch, 2018; Peled-Zehavi and Gal, 2021). Additionally, it contributes to around 15% of marine primary productivity (Yang et al., 2001). Chrysotila dentata is a calcifying granulite algae that can be found in the Bohai Sea, China (Liu et al., 2019), and its growth involves two mechanisms of carbon fixation: photosynthesis and calcification (OzAki et al., 2001). This has important implications for the biogeochemical cycling of marine carbon (Cros and Fortuño, 2002).
In addition to microalgae and bacteria signaling molecules in the interalgal environment, such as the hormone ABA and vitamin VB12, often influence the growth and metabolism of algal systems. VB12 is an organometallic cofactor produced by bacteria that most microalgal metabolism depends on (Kräutler, 2005). Several studies have shown that heterotrophic bacteria provide vitamin B12 to most of the surrounding microalgae, which can use VB12 as a cofactor in specific enzymatic reactions for synthesizing methionine synthase and methamphetamine. (Croft et al., 2005; Wagner-Döbler et al., 2010; Helliwell et al., 2011). In addition, the phytohormone ABA regulates plant developmental processes under abiotic stress conditions. However, the physiological roles of ABA, which has been confirmed to exist in cells, have been little investigated in algae (Tietz et al., 1989). Green algae and rotifer species have developed protective mechanisms to adapt to light and drought environments. Studies have demonstrated ABA’s involvement in desiccation (Holzinger and Becker, 2015) and salinity (Cowan and Rose, 1991) induced stress responses, initiation of the cell cycle (Kobayashi and Tanaka, 2016; Kobayashi et al., 2016), as well as in increasing lipid accumulation in Chlorella (Wu et al., 2018) altering Chamydomonas reinhardtii’s uptake of HCO3- (Rensing et al., 2008).
Therefore, elucidating the effects of exogenous factors on DOM metabolism in algal symbiosis systems is crucial for understanding the global carbon cycle. In this study, Marinobacter hydrocarbonoclasticus CA6 was co-cultured with chrysotile dentata to investigate to investigate changes in algal-derived CDOM using the EEM fluorescence technique. This research will enhance our comprehension of how exogenous factors (ABA and VB12) impact algal-derived DOM in the marine carbon cycle.
2 Materials and methods
2.1 Chrysotila dentata culture conditions
C. dentata was isolated from Bohai Bay, China and subsequently preserved in the laboratory. Before the experiment began, after undergoing 2-3 generations of domestication in an artificial medium, 500 μg/ml penicillin (penlclllln), kanamycln and chloramphenlcol were added to remove the phycosphere bacteria for 1-2 days. Then, the absence of monoclonal colonies was tested in C. dentata medium using smear plates and DAPI fluorescent staining. Subsequently, the algal cells were gravity filtered or “micro-extracted” once through a 0.65μm polycarbonate membrane. It was gently resuspended in artificial seawater using sterile forceps and filtered again before being evenly divided into individual conical bottles. During the during the experiment, the temperature was maintained at 25 oC and 100 μmol photons m-2s-1 were irradiated with a photoperiod of light: dark = 12h:12h cycles.
2.2 Isolation and identification of CA6
The bacterial genomic DNA was extracted using TIANamp Bacteria Kit (Tiangen Biotech Co., Ltd., Beijing, China) with the universal primers 27F (5’-AGAGTTTGATCCTGGCAG-3’) and 1492R (5’-TACGGTTACCTTGTTACGACTT-3’). The bacteria universal 16S rDNA sequences were amplified by PCR, and the resulting products were submitted to Huada Company (Huada, China) for sequencing. The obtained sequences were compared with bacterial sequences in Genbank, revealing a similarity of 99.46% with Marinobacter hydrocarbonoclasticus strain RMR 21.
The CA6 were then diluted 10-fold and cultured in 2216E medium for 4 days. After that, monoclonal CA6 cells were selected and placed in 20 ml of liquid 2216E medium, followed by incubation at 28 oC on a rotary shaker (180 rpm) for 3 days. Subsequently, the CA6 cells were collected by centrifugation at room temperature in a 50 ml centrifuge tube at 5000 rpm for 4 min. The collected CA6 cells were washed three times with ASW. After incubating C. dentata cells for 1 day, add the solution containing the previously mentioned CA6 cells to it, totaling to a volume of 3 ml.
2.3 Biochemical characterization of bacteria
Bacterial physiological and biochemical tests, including Maltose, Mannitol, Aesculin, Xylose, and Salicylic acid were conducted on samples of bacteria co-cultured with algae for a duration of 2 days.
2.4 Marinobacter hydrocarbonoclasticus and C. dentata abundance
The CA6 cells were counted using a 2216E plate dilution method, and 100 μL of bacterial fluid was counted daily from the algal-bacteria co-culture groups. Additionally, 100 μL of algal solution was mounted daily onto a blood cell counting slide and enumerated with a microscope to monitor the abundance of C. dentata cells (Olympus BX51, Japan).
2.5 Excitation-emission matrix with peaks pick
CDOM was measured using a fluorescence spectrophotometer (Hitachi F-7100, Tokyo, Japan). The photomultiplier was operated at 700 V, with the fluorescence excitation (Ex) ranging from 200 to 450 nm and the fluorescence emission (Em) ranging from 250 to 550 nm. The Ex and Em were spaced at intervals of 5 nm, and the scan rate was set as 12,000 nm min-1. Prior to measurement, the instrument was calibrated according to the provided instructions. To remove most of the Raman scattering, ultrapure water was used for blank correction in order to obtain accurate DOM spectra. Multiple fluorescence peaks A, C, B, and T were then extracted through area integration in this experiment. The first two peaks represented humic-like components, while the last two peaks represented protein-like components. The fluorescence intensity was measured in arbitrary units (a.u.) for all data. Four indicators (FI, HIX, BIX and β/α) were employed to describe the CDOM. An FI > 1.8 indicates that the DOM was predominantly generated by microorganisms. BIX can be utilized as an indicator of DOM traceability in aqueous environments. HIX signifies the extent of organic matter humification, whereas β/α denotes the ratio between newly produced and highly decomposed DOM.
2.6 Bacteria and algae derived DOM collection and experimental setup
This experiment was conducted in an artificial medium (ASW) to investigate the effect of different exogenous factors on the growth of C. dentata and DOM release in algae-CA6 co-culture environment. The experimental conical flasks were subjected to pre-acid and pure ultra-pure water washing to remove the input of exogenous organic carbon prior to the start of the experiment. The cell concentration of C. dentata cells was (1.194 ± 0.288) × 102 cells/L on day 0. ABA and VB12 at a concentration of 100 μg/L were added on day 0, and after 1 day of incubation, 3 ml CA6 was added. The following treatment groups were set up: (1) blank group, axenic C. dentata group; (2) CA6 group, axenic C. dentata + CA6 group; (3) ABA group, axenic C. dentata + CA6 group; (4) VB12 group, axenic C. dentata + VB12 group; (5) ABA treated group, axenic C. dentata + CA6 + ABA group; (6) VB12 treated group, axenic C. dentata + CA6 + VB12 group; C. dentata were incubated in the above treatments for 5 days.
From each group, 10 ml of sample was collected and filtered as the CDOM fraction through a 0.22 μm polycarbonate membrane (Millipore, USA). The filtered samples were then stored in a refrigerator at 4 oC for scanning.
2.7 Statistical analysis
In this study, the general linear model of SPSS (version 26.0) was used to examine changes in sample characteristics, allowing for the investigation of relationships between six groups/samples and variables. The amount of variation was analyzed using Microsoft Excel 2021 (Microsoft, WA, USA). Correlations between CA6 abundance, C. dentata abundance, fluorescence index, and fluorescence intensity were analyzed using Person correlations in the R language. Measurements are expressed as mean ± standard deviation (SD).
3 Results
3.1 C. dentata abundance
Considering that algal circle factors may be one of the reasons affecting the growth metabolism of microalgae cells (Cao et al., 2021), this paper initially measured the changes in cell abundance during the growth of C. dentata after treatment with different exogenous factors ABA and VB12. ABA (p > 0.05) reduced the total growth of C. dentata cells by approximately 1.65% under the same light conditions (100 μmol/m-2s-1) and photoperiod (light: dark = 12:12h). In contrast, the same concentration of VB12 (p > 0.05) increased the total cell growth by approximately 9.37% (Figure 1B). When counting the daily growth of C. dentata, it was found that adding VB12 did not affect normal cell growth, but adding ABA caused a significant (p < 0.05) decrease in cell abundance at day 4 (Figure 1A).
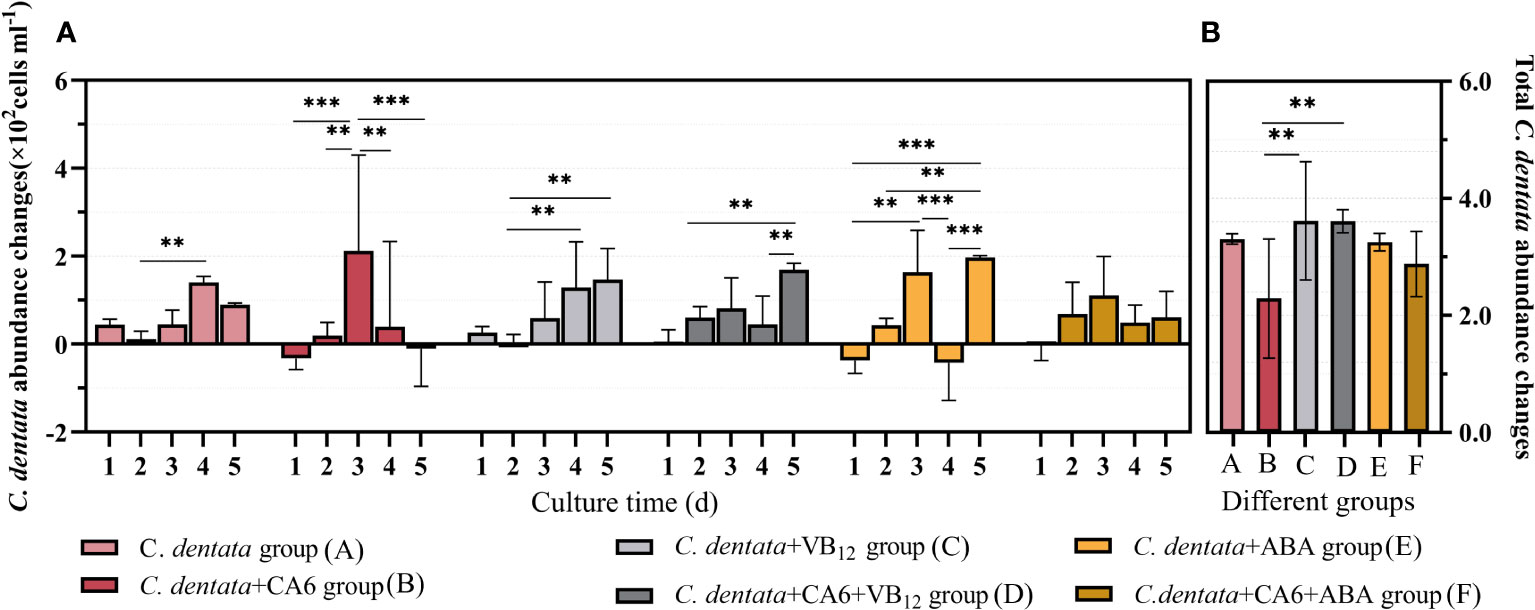
Figure 1 The number of C. dentata cells increased daily during 5-day incubation period under various stimulations (A); The total change in C. dentata (B); ** represents p < 0.05; *** represents p < 0.001.
As the growth and development of C. dentata in the aquatic environment are closely related to bacteria, previous studies have found that CA6 significantly increases cell abundance and photosynthetic capacity of C. dentata, as well as positively affecting the release of C. dentata-derived DOM. To further investigate how exogenous factors affect C. dentata growth in complex water environments, this paper investigates the effect of adding CA6 to axenic C. dentata together with ABA or VB12 exogenous factors on microalgae cells growth. No statistically significant differences (p > 0.05) were found between the C. dentata co-cultured with CA6 group and the axenic C. dentata group when calculating the total change in microalgal cell abundance. The growth trends of microalgal cells varied from day to day when the same concentration (100 μg/L) of exogenous factors and the same volume (3 ml) of CA6 were added. It was observed that both the C. dentata co-cultured CA6 group and the C. dentata group with ABA showed a consistent daily increase in cell abundance, with no statistical difference in their daily growth rates. ABA and CA6 (p > 0.05) reduced total cell growth by approximately 12.88% under identical culture conditions, while VB12 and CA6 (p > 0.05) increased total cell growth by approximately 9.22%, but this difference was no statistically significant (p < 0.05) (Figure 1B).
The abundance of C. dentata cells responded differently to the addition of exogenous factors and bacteria, VB12 restored the overall growth of C. dentata cells after being influenced by CA6 in the C. dentata-bacterial interaction group, while ABA treatment stabilized the daily growth of C. dentata cells in a CA6-dependent manner, suggesting an ABA/CA6 interaction and implying that VB12 and ABA are involved in the growth and development of C. dentata cells.
3.2 Marinobacter hydrocarbonoclasticus CA6 abundance
In order to investigate the potential effects of exogenous factors on heterotrophic bacterium CA6 in the Phytoplankton–bacteria interaction system, we examined the cell abundance of CA6. It was observed that the abundance of CA6 cells fluctuated within a certain range in the C. dentata-CA6 co-culture group without the addition of exogenous factors. However, when ABA was added to this co-culture group, the CA6 cells exhibited greater stability and no statistically significant changes were observed per day (p > 0.05). On the other hand, in the C. dentata group with VB12 supplementation, there was a significantly decrease (p < 0.05) in bacterial cell abundance on days 1 and 4, which continued to decline throughout the culture period (Figure 2A). The average cell abundance of CA6 in the C. dentata-bacteria co-cultured group, as well as in the C. dentata-CA6 co-cultured groups with added VB12 and ABA, were 0.73, 1.02, and 1.22 (×107 cells/ml), respectively. The abundance of CA6 cells was significantly increased (p < 0.05) by approximately 68.88% under the influence of ABA (Figure 2B).
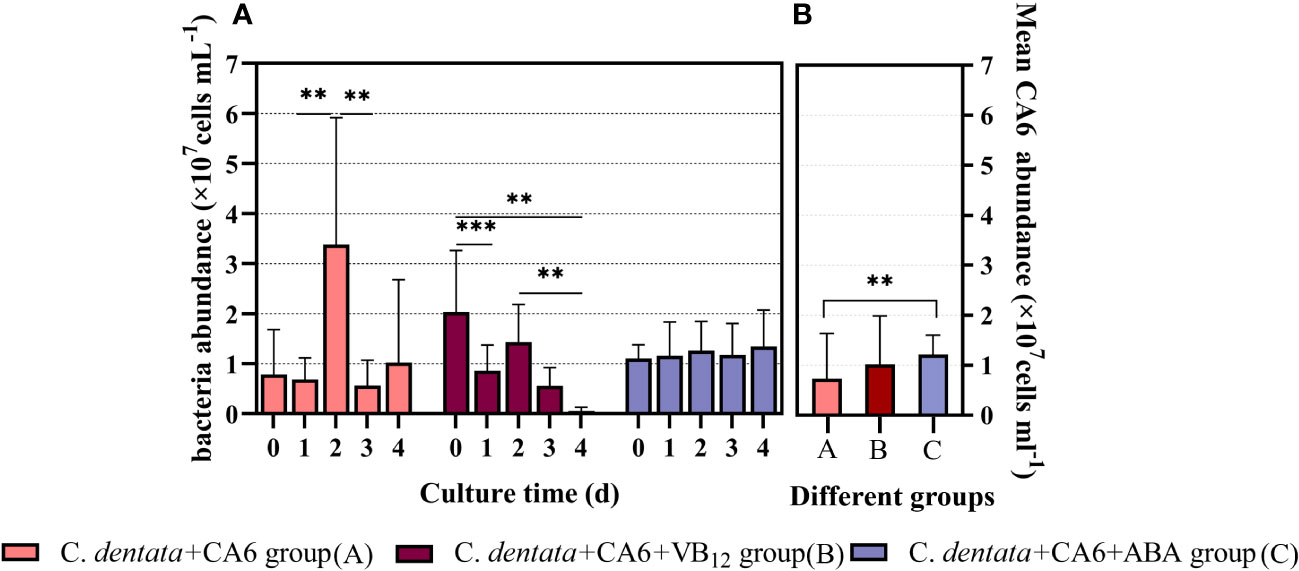
Figure 2 M. hydrocarbonoclasticus CA6 cell numbers were measured after 4 days of incubation under different stimulations (A); The mean abundance of CA6 cells (B); ** represents p < 0.05; *** represents p < 0.001.
3.3 Identification of physiological and biochemical characterization of CA6
Since the abundance of heterotrophic bacterial CA6 cells showed different variations during the addition of exogenous factors VB12 and ABA, it can be inferred that these exogenous factors had a positive impact on the response of heterotrophic bacteria. This suggest that ABA and VB12 might have influenced the growth and metabolism of CA6. To study the characteristics of CA6 growth in the phytoplankton–bacteria co-culture group, physiological and biochemical measurements were taken from CA6 after 2 days of co-culturing with C. dentata. It was observed that under different culture environments, CA6 exhibited varying catabolic capacities for different organic substances (Table 1). In pure culture conditions using 2216E medium, the ability of CA6 to decompose organic matter was limited as it could only decompose maltose and release salicylic acid among five organic substances. When C. dentata was co-cultured with CA6, the decomposition ability of CA6 improved significantly. It enabled the decomposition of maltose, aesculin, mannitol, and the release of salicylic acid. When 100 μg/L of VB12 was added to the C. dentata-CA6 co-cultured group, all five organic compounds (maltose, mannitol, aesculin, xylose, and salicylic acid) were effectively utilized and released. In the C. dentata-CA6 co-cultured group with the same concentration of ABA, the bacteria were able to decompose three organic substances: maltose, mannitol and aesculin. In conclusion, the degradation ability of CA6 improved when co-cultured with C. dentata and reached its maximum when VB12 was added under these conditions. When adding the same concentration of ABA, the ability of CA6 to decomposed organic matter did not differ significantly from that without exogenous factors; However, it exhibited stronger decomposition ability compared to when CA6 was cultured alone.
3.4 The peaks of DOM fluorescence
It has been found that VB12 and ABA factors may be involved in the growth and metabolic processes of phycosphere bacteria. Therefore, during the incubation period, CDOM in the medium was sampled, and different types of DOM fluorescence were measured and calculated. The excitation emission spectra of four organic matter peaks were determined as Peak B, Peak T, Peak A, and Peak C (Figure 3), with their positions shown in Table 2. These Peaks have been identified in the literature as tyrosine-like, tryptophan-like, humic-like and humic-like samples respectively representing chromophoric dissolved organic matter.
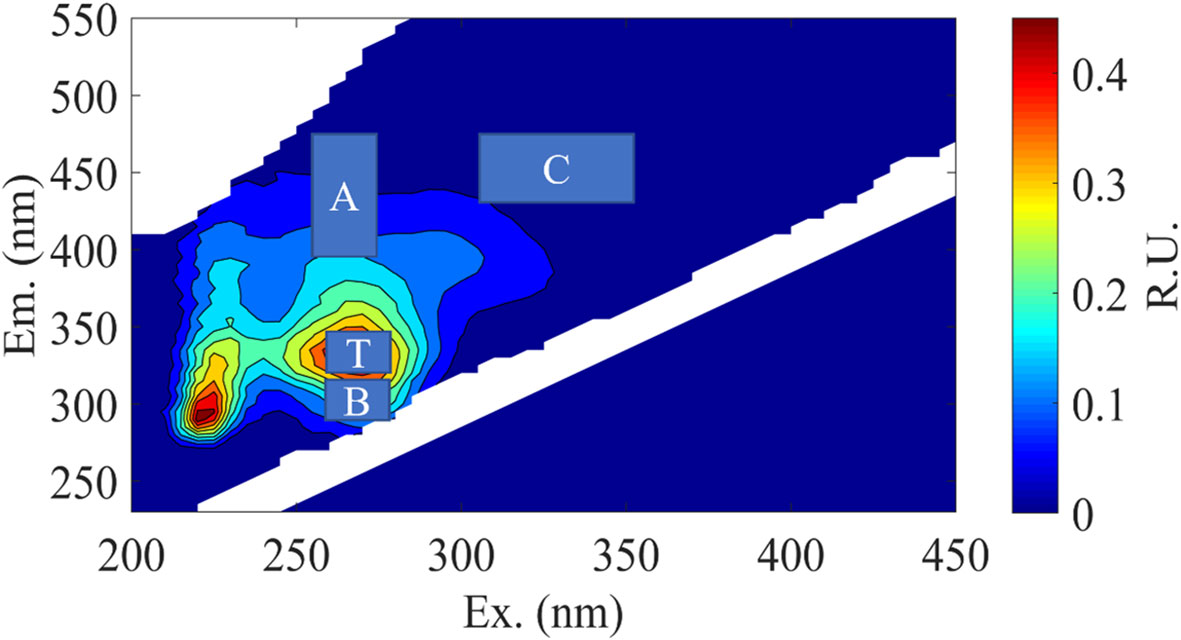
Figure 3 Representative excitation-emission matrices (EEMs) of DOM treatments in C. dentata- M. hydrocarbonoclasticus co-cultures during incubation. The main peak areas of the EEMs were depicted in “A” and “C” represent humic-like components, while “B” and “T” represent protein-like components.

Table 2 The EEM fluorescence center regions are attributed to organic matter sources different from those in previous studies.
3.5 Changes in peaks of DOM fluorescence
Statistics on the daily changes in CDOM amounts were conducted to examine the effects of adding the same concentration of VB12 or ABAto axenic C. dentata groups or C. dentata-CA6 co-cultured groups. The results revealed that the addition of exogenous factors ABA or VB12 to axenic C. dentata on the first day led to a significant decrease (p < 0.05) in tyrosine-like component (peak B), tryptophan-like component (peak T), and humic-like components (peak A, C) in the culture medium (Figure 4). Furthermore, there was a significant increase (p < 0.05) in the release of tyrosine-like component by adding ABA on the third day of C. dentata-CA6 co-cultured group. On day four, VB12 addition resulted in significant decrease (p < 0.05) in humic-like substances (peak A) within the C. dentata co-culture group, while no significant effect was observed in the ABA-added C. dentata-CA6 group.
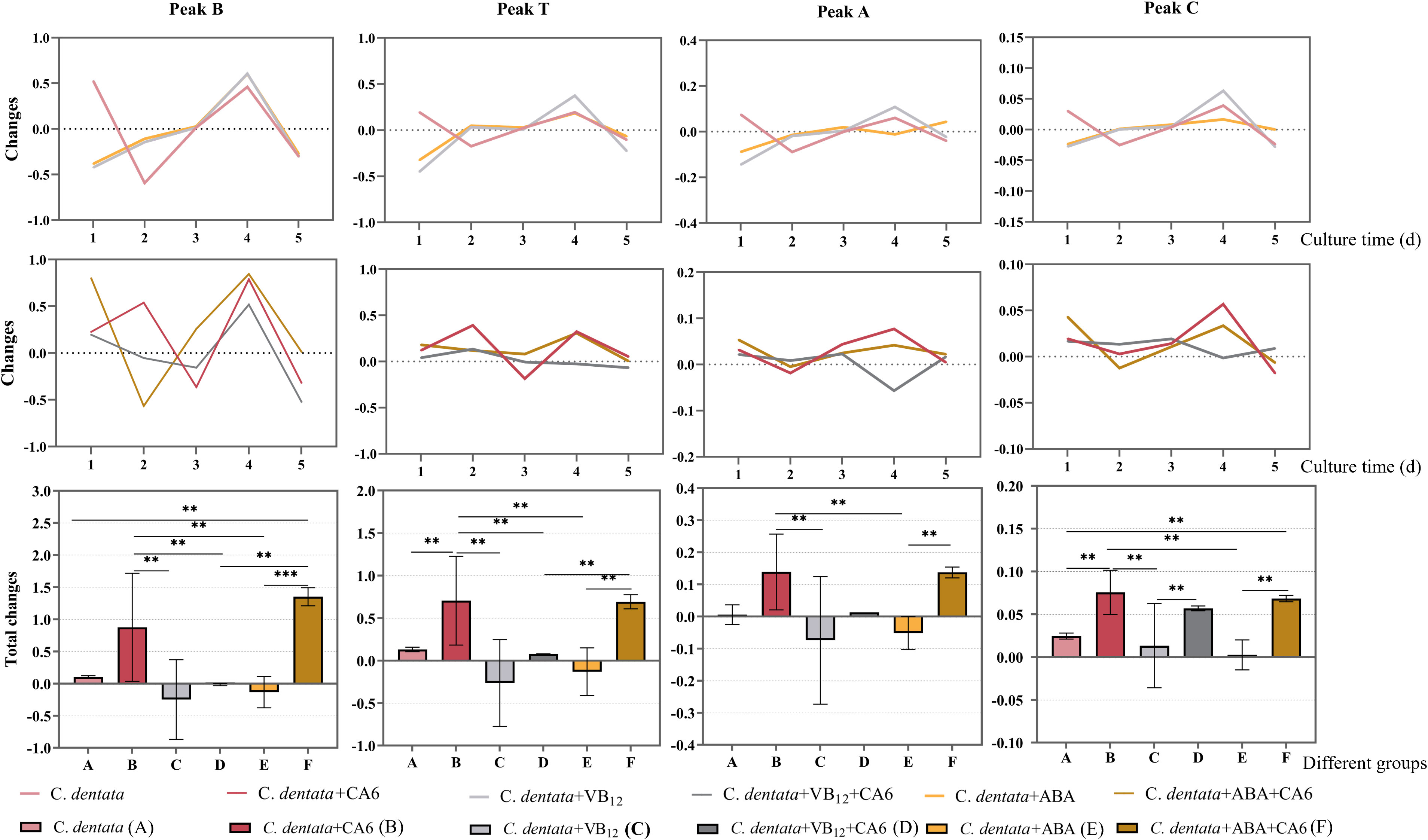
Figure 4 Fluorescence peaks in DOM samples include peak B, peak T, peak A, and peak C as the fluorescence components of each column, respectively. The broken line represents the change in the amount of fluorescence peaks daily, while the bar graph represents the total change in fluorescence peaks. Pink, red, light grey, dark grey, yellow and brown represent respectively the C. dentata group, C. dentata-CA6 co-culture group, C. dentata with VB12 group, C. dentata-CA6 co-cultured with VB12 group, C. dentata with ABA group and C. dentata-CA6 co-cultured with ABA. ** represents p < 0.05; *** represents p < 0.001.
During incubation, it was observed that the addition of CA6 to axenic C. dentata significantly increased (p < 0.05) the total amount of variation of tryptophan-like component and humic-like component (peak C), while there was no statistically significantly difference in the increase of tyrosine-like component and humic-like component (peak A) (Figure 4). Incubating microalgae alone with exogenous factors VB12 or ABA at a concentration of 100 μg/L revealed that ABA and VB12 reduced (p > 0.05) the total release of protein-like fluorophores components and humic-like fluorophores components, respectively. The total release of tyrosine-like components was significantly increased (p < 0.05) by the addition of CA6 and ABA to axenic C. dentata. Meanwhile, when VB12 or ABA was added to the algal-bacterial co-cultured group, it was found that VB12 significantly (p < 0.05) reduced the overall change in tyrosine-like and tryptophan-like substances in the medium, while humus-like substances (peak A) decreased (p > 0.05), but had no effect on humic substances (peak C). In contrast, the release of tyrosine-like and humic substance (peak A) from DOM in C. dentata-CA6 co-cultured group increased (p > 0.05), and the total release of tryptophan-like substance (peak C) decreased (p > 0.05) due to ABA compared to VB12.
On the other hand, it was found that the addition of ABA significantly reduced (p < 0.05) the total change in humic-like component (peak A) and tryptophan-like substances in the medium compared to adding ABA to microalgal-bacterial co-cultured group in axenic C. dentata cultures. The presence or absence of CA6 in the presence of VB12 or ABA added to axenic C. dentata cultures played a significant role in the transforming humic-like components (peak C) (p < 0.05) in the medium for peak C humic-like substance.
3.6 Fluorescence indices
The spectral indices (FI, BIX, HIX, and β/α) of the six different treatment groups (axenic C. dentata group, C. dentata co-cultured with CA6 group, C. dentata added with VB12 group, C. dentata-CA6 co-cultured with VB12 group, C. dentata added with ABA group, and C. dentata-CA6 co-cultured with ABA group) varied during the incubation period as shown in Figure 5. The FI values for the six groups greater than 1.8 (Figure 5B). The mean β/α values ranged from 1.070 to1.269 (Figure 5A). The mean HIX values ranged from 0.140 to 0.233, and the HIX values showed an increasing trend during culture time (Figure 5C). The mean BIX values ranged from 1.308 to 1.593 (Figure 5D). The BIX, FI, and β/α exhibited similar trends, initially increasing from day 0 to day 2 and then gradually decreasing. Previous studies have indicated that an FI > 1.8 signifies that microorganisms are the primary producers of the fluorescent component of CDOM (Lavonen et al., 2015).
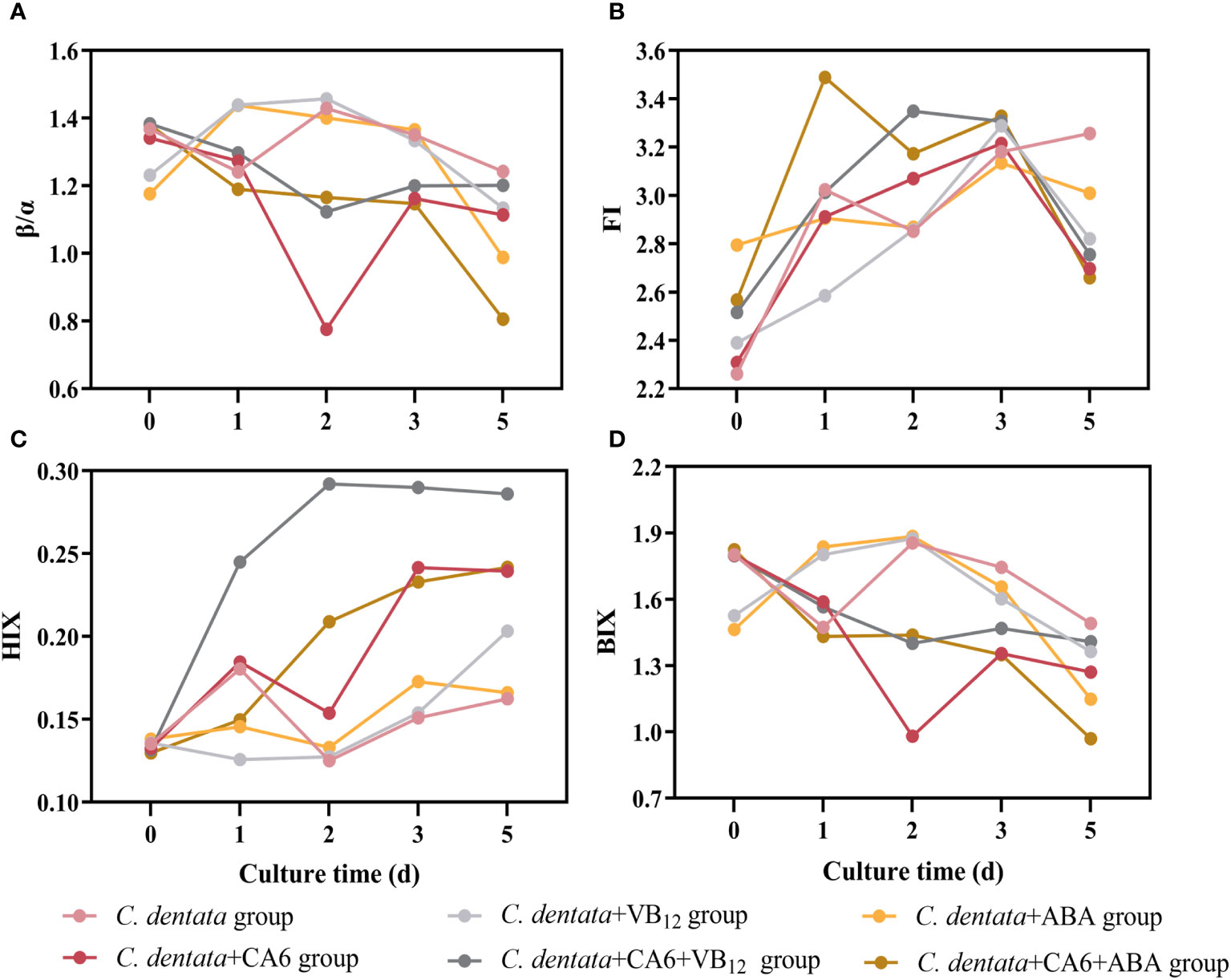
Figure 5 Four spectral indices, β/α (A), FI (B), HIX (C), and BIX (D) were measured during incubation for the six culture groups.
4 Discussion
4.1 Growth of C. dentata and CA6 under different treatments
VB12 is a necessary cofactor in the growth process of microalgae. During the incubation period, when 100 μg/L of VB12 was added to axenic C. dentata, no significant difference was observed in the abundance change of microalgae cells. However, if the same concentration of VB12 was added to co-cultured C. dentata-CA6, it significantly increased cell abundance of C. dentata. These results indicate that VB12 promotes microalgae growth more significantly only when C. dentata-CA6 coexist. Meanwhile, it was observed that a concentration of 100 μg/L of VB12 had an inhibitory effect on CA6, causing its number to decrease almost to zero during the final period of incubation. This is because most microalgae cells do not possess relevant genes to uptake VB12 from the environment for their own growth (Kazamia et al., 2012). VB12 is uniquely synthesized by a few bacteria and archaebacteria, thereby influencing microalgal growth and metabolism (Crofts et al., 2013). However, adding exogenous VB12 can inhibit the expression of growth-related genes in bacteria (Kazamia et al., 2012). Meanwhile, the release of Salicylic acid in C. dentata-CA6 co-cultured with VB12 group may inhibits bacterial biofilm production (Bryers et al., 2006). VB12 regulates bacterial expression of the cobA operon through a riboswitch, thereby affecting the stability of the host milieu (Li et al., 2020).
Meanwhile, in this study, we did not find any statistically significant difference in the amount of change when ABA was added to axenic C. dentata group or when the C. dentata-CA6 co-cultured with ABA group was examined. However, the presence of ABA in the phycosphere favored stabilizing the daily abundance of C. dentata cells and helped maintain microalgae stability in their environment. Additionally, ABA, which is present in the phycosphere, acts as a stress-regulating hormone that contributes to adaptability and resistance to various abiotic stresses by increasing antioxidant enzymes activity within cells (Yoshida et al., 2003; Hauser et al., 2017). In higher plants, environmental stresses such as photo-oxidative stress, water stress, low temperature stress, and heavy metal stress can increase intracellular levels of ROS (Dat et al., 2000). Fortunately, ABA can increase the activities of CAT, APX, SOD, glutathione peroxidase, and glutathione reductase to induce a response for the elimination of ROS (Yoshida et al., 2003). In this study, it was observed that adding ABA to the algal-bacterial CA6 co-culture enhanced the stability of cell abundance for both C. dentata and CA6 during incubation. This confirms earlier findings that ABA promotes the stabilization of cellular abundance. However, previous studies have shown that exogenously added ABA can enhance the growth of green alga Chlamydomonasreinhardtii (Yoshida et al., 2003), which differs from our research may be due to different types of microalgae being used.
4.2 Correlation analysis of DOM influenced by CA6
Correlation analysis of multiple components in the cultural groups using the R language revealed that the variations in DOM were different under different treatment conditions. When the microalgae were cultured alone (Figure 6A), there was a negative correlation between the abundance of C. dentata cells and β/α, as well as a negative correlation between the peaks of DOM fluorescence and BIX and β/α, but a positive correlation with HIX. In the absence of heterotrophic bacteria and exogenous factors, the production of new DOM by C. dentata decreased, while the humification process of protein- and humic-like substances in solution increased. This suggests that without bacterial involvement in the axenic C. dentata culture, the effectively transformation of DOM into humic-like substances did not occur (Zhao et al., 2019); However, the degree of humification in the medium was increased due to the influence of light (Broman et al., 2019), pH (Liu et al., 2022) and so on.
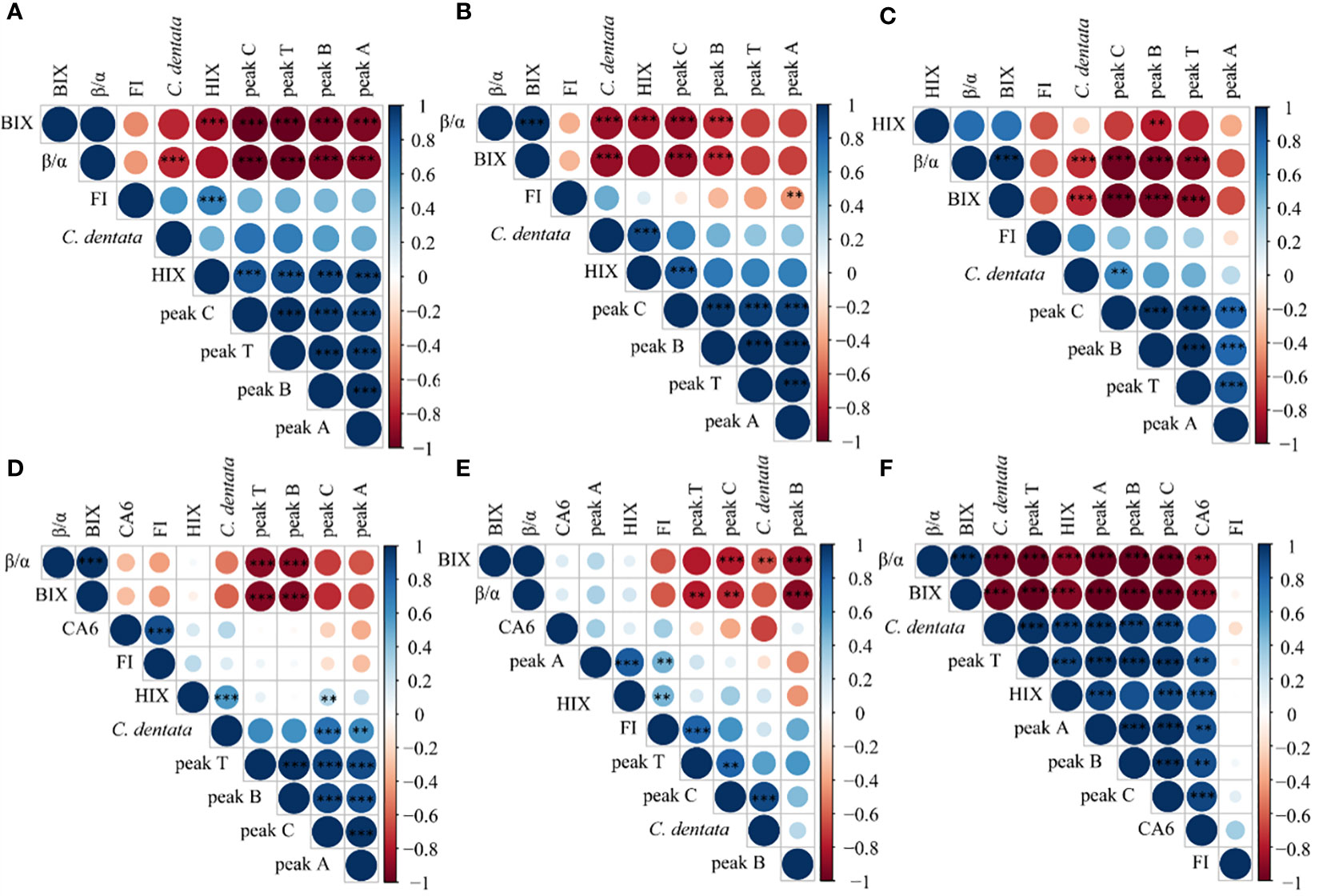
Figure 6 Correlation analysis was conducted on C. dentata, DOM fluorescence peaks and DOM fluorescence indicators in 6 incubation groups over a period of 5 days; axenic C. dentata group, (A); C. dentata incubated with VB12 group, (B); C. dentata incubated with ABA group, (C); C. dentata-CA6 co-cultured group, (D); C. dentata-CA6 incubated with VB12 group, (E); and C. dentata-CA6 incubated with ABA, (F). ** represents p < 0.05; *** represents p < 0.001.
When CA6 was added to the C. dentata solution (Figure 6D), there was a positive correlation between the abundance of CA6 cells and FI, as well as HIX was positively correlated with peak C and microalgae abundance. Additionally, peak B and peak T were negatively correlated with BIX, β/α. Peak A showed a positive correlation with microalgae abundance. The addition of CA6 during microalgal incubation revealed that as the abundance of the microalgal cells increased, they released DOM into the surrounding environment (Vidal-Melgosa et al., 2021), resulting in an increased degree of humification in the solution. This indicates that CA6 can effectively consume protein-like substances (peak B, peak T) in the solution and transform into humus-like substances (peak C, peak A) at the same time (Zhao et al., 2019). On the other hand, the CDOM can limit the bacterial community in natural water (Carlson and Ducklow, 1996). Gammaproteobacteria are known to become more abundance in DOM, and utilize the enrichment of complex DOM substrate and large DOM molecules released from phytoplankton (Sarmento and Gasol, 2012; Zhao et al., 2019). In this study CA6 was identified as a type of γ-proteobacteria that effectively transforms protein-like components into humic-like components.
4.3 Correlation analysis of DOM influenced by VB12
When 100 μg/L of VB12 was added to the axenic C. dentata solution (Figure 6B), it was observed that the cell abundance of C. dentata showed a positive correlation with HIX and a negative correlation with β/α and BIX. Additionally, both peak B and peak C were negatively correlated with β/α and BIX. On the other hand, peak C exhibited a positive correlation with HIX, while peak A showed a negative correlation with FI. The addition of VB12 to the axenic microalgae incubation demonstrated that VB12 could fulfill the essential requirement for amino acids biosynthesis and support normal growth of microalgae in the medium (Li et al., 2020). Furthermore, due to the near absence of bacteria in the solution, microorganisms had low activity and played a minimal role in consuming or transforming DOM. However, it was observed that DOM continuously underwent “deteriorated” as humic-like substances (peak C) underwent deepening humification, which may possible be impacted by other factors in solution (Broman et al., 2019; Liu et al., 2022).
However, when the same concentration of VB12 was added to the C. dentata and CA6 co-culture group (Figure 6E), it was observed that the abundance of C. dentata cells showed a negative correlation with BIX and a positive correlation with peak C. Additionally, peak B and peak C exhibited a negative correlation with BIX and β/α. Peak T displayed a negative correlation with β/α and a positive correlation with FI. Furthermore, peak A demonstrated a positive correlation with HIX and FI. The β/α represents the ratio of CDOM production to decomposition in the water column, while the BIX is used as a retrospective index for DOM characterization. Both the β/α and BIX reached their peak values on day 2 of the incubation, indicating maximum microbial metabolism on this day. In contrast, the HIX values showed a continuous increase from day 0 to day 5. A higher HIX suggests a higher content of high molecular weight aromatic compounds (Huguet et al., 2009), indicating an increase in humic substances content and humification starting from day 0 of incubation. It was observed that adding VB12 during algal-bacterial interactions resulted in achieving the highest degree of humification.In natural environments, microalgae do not carry out life activities alone; They often coexist with bacteria in the water environments. If VB12 is added to the co-cultured environment of C. detnata-CA6, the riboswitch binds of bacteria to VB12 and forms the pseudoknot that prevents ribosome from binding with ribosome-binding site (RBS) (Li et al., 2020). In this study, 100 μg/L of VB12 was inhibited the homeostasis of the CA6 in the phycosphere of C. dentata of the host, and disrupted their symbiotic relationship. The abundance of CA6 to utilize and transform of DOM in the solution is low. As C. dentata grows, DOM cannot be effectively consumed, resulting in an increasing concentration of protein-like substances and humus-like substances in the solution.
4.4 Correlation analysis of DOM influenced by ABA
When 100 μg/L ABA was added to the incubation of C. dentata (Figure 6C), it was observed that the cell abundance of C. dentata had a negative correlation with BIX and β/α, and a positive correlation with peak C. Peak B was negatively correlated with BIX, β/α, and HIX. Peaks T and C were negatively correlated with BIX and β/α. ABA is an anti-adversity hormone (Zeevaart and Creelman, 1988) which can increase the activity of antioxidant enzymes capable of eliminating ROS in higher plants, on the other hand, ABA can enhance the photosynthetic activity of microalgae by protecting their photosynthetic system from reactive oxygen species (ROS) (Yoshida et al., 2003) thus improving the resistance of higher plants and microalgae to adverse conditions. In this study, it was found that after adding ABA at the beginning of the incubation period of C. dentata, there was no deepening of DOM humification in solution as microalgal cell abundance increased. It is hypothesized that the addition of ABA may have maintained cellular activity in C. dentata while simultaneously preserving the freshness of the released DOM during incubation.
To further investigate, when the same concentration of ABA was added to the co-cultured environment of C. dentata-CA6 (Figure 6F), C. dentata exhibited a higher efficiency in secreting protein-like substances compared to CA6’s utilized efficiency in converting DOM. Unconsumed protein-like substances may become inert and less susceptible to decomposition due to other factors (Broman et al., 2019; Liu et al., 2022), while humic-like substances produced by bacterial consumption were preserved in solution, thereby increasing the degree of humification for the DOM.
5 Conclusion
In this study, we analyzed the effects of exogenous factors (ABA and VB12) on the growth of C. dentata and C. dentata-derived DOM transformation in the presence of M. hydrocarbonoclasticus CA6 for the first time. We found that CA6 effectively consumed and transformed DOM derived from C. dentata. 100 μg/L VB12 inhibited the consumption and transformation of DOM from C. dentata by suppressing the proliferation of CA6, thereby breaking the symbiotic relationship between C. dentata and CA6. Additionally, 100 μg/L ABA was observed to reduce the degree of DOM humification and maintain its freshness in axenic cultures of C. dentata. Furthermore, ABA influenced both the efficiency of DOM consumption and transformation in the C. dentata-CA6 co-cultured environments affecting the degree of DOM humification.
Data availability statement
The original contributions presented in the study are included in the article/supplementary material. Further inquiries can be directed to the corresponding author.
Author contributions
XW: Formal analysis, Methodology, Writing – original draft, Writing – review & editing. JS: Conceptualization, Funding acquisition, Methodology, Project administration, Resources, Supervision, Visualization, Writing – review & editing.
Funding
The author(s) declare financial support was received for the research, authorship, and/or publication of this article. This research was financially supported by the National Key Research and Development Project of China (2019YFC1407800), the National Natural Science Foundation of China (41876134), the Changjiang Scholar Program of Chinese Ministry of Education (T2014253) to JS, and supported by State Key Laboratory of Biogeology and Environmental Geology, China University of Geosciences (GKZ22Y656), JS also acknowledges support by Projects of Southern Marine Science and Engineering Guangdong Laboratory (Zhuhai) (SML2021SP204, SML2022005).
Conflict of interest
The authors declare that the research was conducted in the absence of any commercial or financial relationships that could be construed as a potential conflict of interest.
Publisher’s note
All claims expressed in this article are solely those of the authors and do not necessarily represent those of their affiliated organizations, or those of the publisher, the editors and the reviewers. Any product that may be evaluated in this article, or claim that may be made by its manufacturer, is not guaranteed or endorsed by the publisher.
References
Alonso-Sáez L., Arístegui J., Pinhassi J., Gómez-Consarnau L., González J. M., Vaqué D., et al. (2007). Bacterial assemblage structure and carbon metabolism along a productivity gradient in the NE Atlantic Ocean. Aquat. Microbial. Ecol. 46 (1), 43–53. doi: 10.3354/ame046043
Balch W. M. (2018). The ecology, biogeochemistry, and optical properties of coccolithophores. Ann. Rev. Mar. Sci. 10, 71–98. doi: 10.1146/annurev-marine-121916-063319
Broman E., Asmala E., Carstensen J., Pinhassi J., Dopson M. (2019). Distinct coastal microbiome populations associated with autochthonous-and allochthonous-like dissolved organic matter. Front. Microbiol. 10, 2579. doi: 10.3389/fmicb.2019.02579
Bryers J. D., Jarvis R. A., Lebo J., Prudencio A., Kyriakides T. R., Uhrich K. (2006). Biodegradation of poly (anhydride-esters) into non-steroidal anti-inflammatory drugs and their effect on Pseudomonas aeruginosa biofilms in vitro and on the foreign-body response in vivo. Biomaterials 27 (29), 5039–5048. doi: 10.1016/j.biomaterials.2006.05.034
Cao J. Y., Wang Y. Y., Wu M. N., Kong Z. Y., Lin J. H., Ling T., et al. (2021). RNA-seq Insights Into the Impact of Alteromonas macleodii on Isochrysis galbana. Front. Microbiol. 12, 711998. doi: 10.3389/fmicb.2021.711998
Carlson C. A., Ducklow H. W. (1996). Growth of bacterioplankton and consumption of dissolved organic carbon in the Sargasso Sea. Aquat. Microbial. Ecol. 10 (1), 69–85. doi: 10.3354/ame010069
Coble P. G. (1996). Characterization of marine and terrestrial DOM in seawater using excitation-emission matrix spectroscopy. Mar. Chem. 51 (4), 325–346. doi: 10.1016/0304-4203(95)00062-3
Coble P. G. (2007). Marine optical biogeochemistry: the chemistry of ocean color. Chem. Rev. 107 (2), 402–418. doi: 10.1021/cr050350+
Coble P. G., Del Castillo C. E., Avril B. (1998). Distribution and optical properties of CDOM in the Arabian Sea during the 1995 Southwest Monsoon. Deep Sea Res. Part II: Topical Stud. Oceanography 45 (10-11), 2195–2223. doi: 10.1016/S0967-0645(98)00068-X
Cowan A. K., Rose P. D. (1991). Abscisic acid metabolism in salt-stressed cells of Dunaliella salina: Possible interrelationship with β-carotene accumulation. Plant Physiol. 97 (2), 798–803. doi: 10.1104/pp.97.2.798
Croft M. T., Lawrence A. D., Raux-Deery E., Warren M. J., Smith A. G. (2005). Algae acquire vitamin B12 through a symbiotic relationship with bacteria. Nature 438 (7064), 90–93. doi: 10.1038/nature04056
Crofts T. S., Seth E. C., Hazra A. B., Taga M. E. (2013). Cobamide structure depends on both lower ligand availability and CobT substrate specificity. Chem. Biol. 20 (10), 1265–1274. doi: 10.1016/j.chembiol.2013.08.006
Cros L., Fortuño J. M. (2002). Atlas of northwestern Mediterranean coccolithophores. Scientia Marina 66 (S1), 1–182. doi: 10.3989/scimar.2002.66s11
Dat J., Vandenabeele S., Vranova E. V. M. M., Van Montagu M., Inzé* D., Van Breusegem F. (2000). Dual action of the active oxygen species during plant stress responses. Cell. Mol. Life Sci. CMLS 57, 779–795. doi: 10.1007/s000180050041
Dittmar T., Lennartz S. T., Buck-Wiese H., Hansell D. A., Santinelli C., Vanni C., et al. (2021). Enigmatic persistence of dissolved organic matter in the ocean. Nat. Rev. Earth Environ. 2 (8), 570–583. doi: 10.1038/s43017-021-00183-7
Fuhrman J., Azam F. (1982). Thymidine incorporation as a measure of heterotrophic bacterioplankton production in marine surface waters: evaluation and field results. Mar. Biol. 66, 109–120. doi: 10.1007/BF00397184
Hansell D. A., Carlson C. A., Schlitzer R. (2012). Net removal of major marine dissolved organic carbon fractions in the subsurface ocean. Global Biogeochem. Cycles 26 (1). doi: 10.1029/2011GB004069
Hauser F., Li Z., Waadt R., Schroeder J. I. (2017). SnapShot: abscisic acid signaling. Cell 171 (7), 1708–1708. e1700. doi: 10.1016/j.cell.2017.11.045
Helliwell K. E., Wheeler G. L., Leptos K. C., Goldstein R. E., Smith A. G. (2011). Insights into the evolution of vitamin B12 auxotrophy from sequenced algal genomes. Mol. Biol. Evol. 28 (10), 2921–2933. doi: 10.1093/molbev/msr124
Holzinger A., Becker B. (2015). Desiccation tolerance in the streptophyte green alga Klebsormidium: the role of phytohormones. Communicative Integr. Biol. 8 (4), e1059978. doi: 10.1080/19420889.2015.1059978
Huguet A., Vacher L., Relexans S., Saubusse S., Froidefond J.-M., Parlanti E. (2009). Properties of fluorescent dissolved organic matter in the Gironde Estuary. Organic Geochem. 40 (6), 706–719. doi: 10.1016/j.orggeochem.2009.03.002
Kazamia E., Czesnick H., Nguyen T. T. V., Croft M. T., Sherwood E., Sasso S., et al. (2012). Mutualistic interactions between vitamin B12-dependent algae and heterotrophic bacteria exhibit regulation. Environ. Microbiol. 14 (6), 1466–1476. doi: 10.1111/j.1462-2920.2012.02733.x
Kobayashi Y., Ando H., Hanaoka M., Tanaka K. (2016). Abscisic acid participates in the control of cell cycle initiation through heme homeostasis in the unicellular red alga Cyanidioschyzon merolae. Plant Cell Physiol. 57 (5), 953–960. doi: 10.1093/pcp/pcw054
Kobayashi Y., Tanaka K. (2016). Transcriptional regulation of tetrapyrrole biosynthetic genes explains abscisic acid-induced heme accumulation in the unicellular red alga Cyanidioschyzon merolae. Front. Plant Sci. 7, 1300. doi: 10.3389/fpls.2016.01300
Kräutler B. (2005). Vitamin B12: chemistry and biochemistry. Biochem. Soc. Trans. 33 (4), 806–810. doi: 10.1042/BST0330806
Lavonen E., Kothawala D. N., Tranvik L. J., Gonsior M., Schmitt-Kopplin P., Köhler S. (2015). Tracking changes in the optical properties and molecular composition of dissolved organic matter during drinking water production. Water Res. 85, 286–294. doi: 10.1016/j.watres.2015.08.024
Li J., Ge Y., Zadeh M., Curtiss R. III, Mohamadzadeh M. (2020). Regulating vitamin B12 biosynthesis via the cbiM Cbl riboswitch in Propionibacterium strain UF1. Proc. Natl. Acad. Sci. 117 (1), 602–609. doi: 10.1073/pnas.1916576116
Liu H., Zhang X., Sun J., Satheeswaran T., Zhang G., Li H., et al. (2019). Morphology, phylogenetic position, and ecophysiological features of the coccolithophore Chrysotila dentata (Prymnesiophyceae) isolated from the Bohai Sea, China. Phycologia 58 (6), 628–639. doi: 10.1080/00318884.2019.1644477
Liu Y., Wang X., Sun J. (2022). Transformations of diatom-derived dissolved organic matter by bacillus pumilus under warming and acidification conditions. Front. Microbiol. 13, 833670. doi: 10.3389/fmicb.2022.833670
Murphy K. R., Stedmon C. A., Waite T. D., Ruiz G. M. (2008). Distinguishing between terrestrial and autochthonous organic matter sources in marine environments using fluorescence spectroscopy. Mar. Chem. 108 (1-2), 40–58. doi: 10.1016/j.marchem.2007.10.003
Nieto-Cid M., Álvarez-Salgado X. A., Gago J., Pérez F. F. (2005). DOM fluorescence, a tracer for biogeochemical processes in a coastal upwelling system (NW Iberian Peninsula). Mar. Ecol. Prog. Ser. 297, 33–50. doi: 10.3354/meps297033
OzAki N., Sakuda S., Nagasawa H. (2001). Isolation and some characterization of an acidic polysaccharide with anti-calcification activity from coccoliths of a marine alga, Pleurochrysis carterae. Biosci. Biotechnol. Biochem. 65 (10), 2330–2333. doi: 10.1271/bbb.65.2330
Para J., Coble P. G., Charrìère B., Tedetti M., Fontana C., Sempere R. (2010). Fluorescence and absorption properties of chromophoric dissolved organic matter (CDOM) in coastal surface waters of the northwestern Mediterranean Sea, influence of the Rhône River. Biogeosciences 7 (12), 4083–4103. doi: 10.5194/bg-7-4083-2010
Peled-Zehavi H., Gal A. (2021). Exploring intracellular ion pools in coccolithophores using live-cell imaging. Adv. Biol. (Weinh) 5 (6), e2000296. doi: 10.1002/adbi.202000296
Rensing S. A., Lang D., Zimmer A. D., Terry A., Salamov A., Shapiro H., et al. (2008). The Physcomitrella genome reveals evolutionary insights into the conquest of land by plants. Science 319 (5859), 64–69. doi: 10.1126/science.1150646
Sarmento H., Gasol J. M. (2012). Use of phytoplankton-derived dissolved organic carbon by different types of bacterioplankton. Environ. Microbiol. 14 (9), 2348–2360. doi: 10.1111/j.1462-2920.2012.02787.x
Seymour J. R., Amin S. A., Raina J.-B., Stocker R. (2017). Zooming in on the phycosphere: the ecological interface for phytoplankton–bacteria relationships. Nat. Microbiol. 2 (7), 1–12. doi: 10.1038/nmicrobiol.2017.65
Stedmon C. A., Markager S. (2005). Tracing the production and degradation of autochthonous fractions of dissolved organic matter by fluorescence analysis. Limnol. Oceanography 50 (5), 1415–1426. doi: 10.4319/lo.2005.50.5.1415
Stedmon C. A., Markager S., Bro R. (2003). Tracing dissolved organic matter in aquatic environments using a new approach to fluorescence spectroscopy. Mar. Chem. 82 (3-4), 239–254. doi: 10.1016/S0304-4203(03)00072-0
Tietz A., Ruttkowski U., Kohler R., Kasprik W. (1989). Further investigations on the occurrence and the effects of abscisic acid in algae. Biochem. Und Physiol. Der Pflanzen 184 (3-4), 259–266. doi: 10.1016/S0015-3796(89)80011-3
Uusikivi J., Vähätalo A. V., Granskog M. A., Sommaruga R. (2010). Contribution of mycosporine-like amino acids and colored dissolved and particulate matter to sea ice optical properties and ultraviolet attenuation. Limnol. Oceanography 55 (2), 703–713. doi: 10.4319/lo.2009.55.2.0703
Vidal-Melgosa S., Sichert A., Francis T. B., Bartosik D., Niggemann J., Wichels A., et al. (2021). Diatom fucan polysaccharide precipitates carbon during algal blooms. Nat. Commun. 12 (1), 1150. doi: 10.1038/s41467-021-21009-6
Wagner-Döbler I., Ballhausen B., Berger M., Brinkhoff T., Buchholz I., Bunk B., et al. (2010). The complete genome sequence of the algal symbiont Dinoroseobacter shibae: a hitchhiker's guide to life in the sea. ISME J. 4 (1), 61–77. doi: 10.1038/ismej.2009.94
Wu G., Gao Z., Du H., Lin B., Yan Y., Li G., et al. (2018). The effects of abscisic acid, salicylic acid and jasmonic acid on lipid accumulation in two freshwater Chlorella strains. J. Gen. Appl. Microbiol. 64 (1), 42–49. doi: 10.2323/jgam.2017.06.001
Yang T.-N., Wei K.-Y., Gong G.-C. (2001). Distribution of coccolithophorids and coccoliths in surface ocean off northeastern Taiwan. Botanical Bull. Academia Sin. 42. doi: 10.7016/bbas.200110.0287
Yoshida K., Igarashi E., Mukai M., Hirata K., Miyamoto K. (2003). Induction of tolerance to oxidative stress in the green alga, Chlamydomonas reinhardtii, by abscisic acid. Plant Cell Environ. 26 (3), 451–457. doi: 10.1046/j.1365-3040.2003.00976.x
Zeevaart J. A., Creelman R. A. (1988). Metabolism and physiology of abscisic acid. Annu. Rev. Plant Physiol. Plant Mol. Biol. 39 (1), 439–473. doi: 10.1146/annurev.pp.39.060188.002255
Zhang Y., Zhang E., Yin Y., Van Dijk M. A., Feng L., Shi Z., et al. (2010). Characteristics and sources of chromophoric dissolved organic matter in lakes of the Yungui Plateau, China, differing in trophic state and altitude. Limnol. Oceanography 55 (6), 2645–2659. doi: 10.4319/lo.2010.55.6.2645
Keywords: Chrysotila dentata, Marinobacter hydrocarbonoclasticus, chromophoric DOM, fluorescence indices, fluorescence peaks, vitamin-B12, ABA
Citation: Wang X and Sun J (2024) Exogenous factors impact on bacterial-microalgal growth and chromophoric dissolved organic matter: a case study of Chrysotila dentata and Marinobacter hydrocarbonoclasticus. Front. Mar. Sci. 11:1337111. doi: 10.3389/fmars.2024.1337111
Received: 12 November 2023; Accepted: 02 January 2024;
Published: 22 January 2024.
Edited by:
Danny Ionescu, Leibniz-Institute of Freshwater Ecology and Inland Fisheries (IGB), GermanyCopyright © 2024 Wang and Sun. This is an open-access article distributed under the terms of the Creative Commons Attribution License (CC BY). The use, distribution or reproduction in other forums is permitted, provided the original author(s) and the copyright owner(s) are credited and that the original publication in this journal is cited, in accordance with accepted academic practice. No use, distribution or reproduction is permitted which does not comply with these terms.
*Correspondence: Jun Sun, cGh5dG9wbGFua3RvbkAxNjMuY29t