- 1National Marine Science Centre, School of Environment, Science and Engineering, Southern Cross University, Coffs Harbour, NSW, Australia
- 2Centre for Coastal Biogeochemistry, School of Environment, Science and Engineering, Southern Cross University, Lismore, NSW, Australia
- 3School of Geosciences, University of Sydney, Sydney, NSW, Australia
Light is critical to coral growth through endosymbiont photosynthesis but can also act with elevated temperatures to cause coral bleaching. When more light is absorbed than can be used for photosynthesis, elevated irradiance can damage symbiont photosynthetic machinery. Hence, solar-radiation management through shading has been suggested to alleviate coral bleaching during marine heatwaves. Acropora divaricata and Acropora kenti were tested at two temperatures with 30% shading and an unshaded control to determine the relative impacts of light and temperature on coral bleaching severity. The coral bleaching response was assessed by photochemical (pulse amplitude modulated fluorometry), physiological (symbiont density, chlorophyll a concentration, catalase activity, and lipid peroxidation), and physical (mean intensity of grey or ‘percentage whiteness’) markers. Shading significantly reduced the bleaching response in A. divaricata, whilst for some parameters A. kenti responded negatively to shade. In A. divaricata, shading prevented photochemical collapse up to the experiment’s maximum 4.4 degree-heating weeks (DHW). Biomarkers of coral bleaching stress responded to shade and water temperature at 4.4 DHW; catalase activity was greatest in the shaded and ambient temperature treatment. Shading did not reduce the effects of bleaching in A. kenti; the mean intensity of grey and light saturation coefficient was greatest in the shaded treatment. Shading did, however, reduce lipid peroxidation at 3 DHW. Our results suggest shading during thermal stress may only protect some coral species, highlighting the need to consider species-specific responses when evaluating the potential efficacy of coral bleaching interventions.
1 Introduction
The bleaching of corals is a physiological stress response caused by various environmental stressors (Brown, 1997). Coral bleaching is characterised by a loss of pigmentation from the endosymbiotic algae (family: Symbiodiniaceae), a release of Symbiodiniaceae via exocytosis from the coral host, or the shedding of in hospite Symbiodiniaceae-containing host cells to the water column (Gates et al., 1992). On the Great Barrier Reef (GBR), acute warming events known as marine heatwaves (periods of atypical seawater temperature driven by anthropogenic climate change) trigger episodic events of mass coral bleaching, impacting the entire reef system. In 2024, the GBR experienced its fifth mass bleaching event, since 2016, and the seventh mass bleaching event since 1998 (Cantin et al., 2023). Global sea surface temperature is predicted to continue to rise under all assessed emissions scenarios throughout the 21st century, and marine heatwaves are projected to become more frequent and last longer (Méndez et al., 2013). With the upper thermal threshold of marine organisms being exceeded more regularly, an increase in the frequency and magnitude of coral bleaching and mortality is projected over future decades (Pörtner et al., 2022). There is an escalating demand for studies examining possible mitigation solutions that could alleviate the effect of environmental stressors that cause coral bleaching.
The role of high sea surface temperatures in the bleaching process is unequivocal and widely documented (Donner et al., 2005; Ainsworth et al., 2008; Lough et al., 2018). Many previous coral studies have focused on temperature effects only (Hoegh-Guldberg, 1999; Berkelmans, 2002; Ainsworth et al., 2008; Zhao et al., 2021). Second to thermal stress, solar radiation, including ultraviolet radiation (UVB 280 – 320 nm and UVA 320 – 400 nm; Blanckaert et al., 2021) and photosynthetically active radiation (PAR 400 – 700 nm; Lesser and Farrell, 2004) have the greatest influence on bleaching likelihood (Hoegh-Guldberg et al., 2007; Ban et al., 2014; Roth, 2014; Gonzalez-Espinosa and Donner, 2021). Light alone (in the form of UV or PAR) can induce a bleaching response (solar bleaching) (Jones and Hoegh-Guldberg, 2001; Brown et al., 2002), and elevated temperatures are known to elicit a bleaching response (Hoegh-Guldberg and Smith, 1989; Lesser et al., 1990; Lesser and Farrell, 2004; Hill et al., 2005; Brown and Dunne, 2008; Ban et al., 2014). Light is critical to coral growth through endosymbiont photosynthesis (Muscatine et al., 1984; DiPerna et al., 2018). Increases in PAR can lead to photosystem inhibition in Symbiodiniaceae (Brown et al., 1999). Combined elevated light and temperature have caused bleaching beyond that of elevated temperatures alone (Coles and Jokiel, 1978; Downs et al., 2000; Anthony et al., 2007).
Shading reduces light availability on coral reefs (Coles and Jokiel, 1978; Skirving et al., 2017) and reduces coral bleaching under high temperatures (Baker et al., 2008). Circumstances in which reduced light environments have a positive effect, namely through the refugia provided by turbid environments (Wagner et al., 2010), cloud presence (Leahy et al., 2013), and mangrove-shading (Stewart et al., 2021), suggest that natural shading may have localised benefits for protecting corals subjected to ocean warming. For instance, shaded microhabitats provide a refuge from high irradiance; corals growing in crevices and overhang environments have shown less severe and later bleaching during a marine heat wave than corals in open, elevated, and sand microhabitats (Hoogenboom et al., 2017). Solar-radiation management techniques are proposed for reducing incoming solar radiation by increasing cloud albedo (Harrison et al., 2019; Anthony et al., 2020; Harrison, 2024), and if operated continuously over a large enough area, may also decrease sea surface temperature and consequently reduce degree-heating week accumulation. The primary shading interventions proposed to protect corals from thermal stress include shade cloths (Coelho et al., 2017; Berg et al., 2020), fogging using seawater (Bay et al., 2019; Harrison, 2024), reflective surface films (Baird et al., 2019; Bay et al., 2019) and marine cloud brightening (Latham et al., 2013; Harrison, 2018). Marine cloud brightening and fogging techniques are in the early stages of development. Further research into perceived risks of cloud enhancement could include the effects of solar irradiance peaks. Solar irradiance has been shown to peak in partially cloudy conditions (Yordanov et al., 2013). Further investigation into the effects of shade on bleaching stress in various coral species is imperative for determining the efficacy of deploying regional-scale interventions.
Environmental stress can lead to a breakdown in the coral-symbiont relationship through many physiological mechanisms (Lesser and Farrell, 2004). The temperature-mediated (Lesser, 1996; Lesser and Farrell, 2004; Tchernov et al., 2004), and light-driven (Levy et al., 2006; Roberty et al., 2015) build-up of reactive oxygen species (ROS) in Symbiodiniaceae is the physiological process considered predominantly responsible for bleaching during thermal stress events (Suggett et al., 2008; Lesser, 2019). The enzymatic antioxidant system removes accumulations of potentially toxic ROS before substantial oxidative damage (Baird et al., 2009). Increased antioxidant enzyme activity indirectly indicates ROS production in response to environmental stress (Lesser et al., 1990). Increased enzyme activities of superoxide dismutase and catalase are reported with temperature increases, photosynthetically active radiation, and ultraviolet radiation (Lesser et al., 1990). Biomarkers of unattenuated oxidative stress, for instance, lipid peroxidation (Sandeman, 2008), have been used to quantify the damage from unattenuated oxidative stress. Bleaching stress has also been evaluated non-destructively via photographic analysis (Johnson and Goulet, 2007; Winters et al., 2009) and fluorometry (Lesser and Farrell, 2004; Suggett et al., 2012).
Numerous studies have examined the effects of light and temperature on coral physiology (Tagliafico et al., 2022). Still, there is limited information on the shading level and duration required to mitigate coral bleaching, particularly the effects of lower levels of shade. The application of a lower level of shade has been shown to delay bleaching effects (Butcherine et al., 2023), and is indicated to be more feasible for solar radiation management techniques such as marine fogging technology (Tagliafico et al., 2022). To determine the relative impacts of light and temperature on coral bleaching severity, we tested the hypothesis that 30% shade would reduce bleaching impacts in thermally stressed corals. This study investigated shading as an intervention for alleviating bleaching stress during marine heatwaves. Photochemical, physiological, and physical markers were used to assess the response of two common coral species, Acropora kenti, and Acropora divaricata, to temperature and light.
2 Materials and methods
2.1 Coral collection, fragmentation and acclimatisation
Colonies of the branching corals, Acropora tenuis [under taxonomic revision and hereafter referred to as Acropora kenti (Bridge et al., 2023)] and Acropora divaricata (Supplementary Figure 1A) were collected from Jenny Louise Shoal Reef, Great Barrier Reef (16° 44.76S, 146° 20.1E), from a depth of < 5 m, in November 2021. Acropora species were selected for investigation as they are abundant in reef ecosystems and are typically thermally sensitive (van Woesik et al., 2011; Singh et al., 2019). Branching corals that are fast-growing and mature early (i.e., Acropora) contribute to the structural complexity of coral reef ecosystems, yet are more likely than slower-growing coral taxa to bleach and suffer whole-colony mortality in mass bleaching events (Baird and Marshall, 2002). The collected colonies of A. kenti and A. divaricata were transported to the National Marine Science Centre, Coffs Harbour, Australia (30° 16.062S, 153° 8.244E). Over five months, the colonies were acclimatised to ambient light conditions at a water depth of 0.5 m (average daily light integral (DLI) in the month preceding experiment: 8.2 ± 4.88 mol photon m-2 d-1). The colonies were slowly accustomed to ambient light by reducing the level of shade cloth over the tank, to compensate for the varying depths at which they may have been collected (< 5 m). The colonies were kept at the collection site temperature of 26.4°C and fed one-day-old Artemia salina once a week before the experiment. The healthiest six colonies for each species were then fragmented into ~ 5 cm tall samples and mounted onto etched glass pedestals. Each colony yielded eight fragments. For colonies 1 – 3, the fragments from each colony were equally and randomly distributed between the shade treatments of the ambient temperature treatment. For colonies 4 – 6, the same procedure was conducted for the shade treatments of the increased temperature treatment. This was conducted for both species (n = 48, plus four baselines). Fragments were weighed on their pedestals (13.52 ± 0.73 g; mean ± standard deviation) with an Ohaus® PA213 precision balance fitted with a weighing hook following the buoyant weighting technique (Jokiel et al., 1978; Spencer Davies, 1989). The water volume and temperature of aquaria were maintained at 10 L and 25.3°C, respectively. Post-fragmentation, colonies were acclimatised for seven days before being randomly assigned to their experimental tanks.
2.2 Experimental system and treatments
A 23-day manipulative experiment tested the responses of A. kenti and A. divaricata to an orthogonal combination of shade (two levels: an unshaded control and 30% shade, maintained continuously) and temperature (two levels: ambient, 26.4°C and heat stress, 32.6°C). The experiment was conducted outdoors under natural lighting. Sand-filtered seawater (10 µm) was supplied from Charlesworth Bay, Australia (30° 16.028S, 153° 8.356E). Four replicates per treatment equated to 48 × 600 mL experimental tanks per species. The tank volume was chosen to maintain a consistent temperature. The experimental tanks were placed in two 1200 L water baths, one for each species (Supplementary Figure 1B). We randomised the position of experimental tanks within the water baths after 6, 13, and 18 days to limit location effects. The experimental tanks were independently supplied with seawater at a rate of 100 mL min-1. A heat-hold temperature profile (Grottoli et al., 2021) was used, with a ramp-up period (~0.5°C per day), followed by nine days of 32.6°C for the heat stress treatment, while the control temperature was set at 26.4°C. Water temperatures were selected according to ambient temperature at Jenny Louise Shoal Reef (26.4°C; November 2021) and the maximum monthly mean (MMM; 28.6°C), plus a temperature anomaly of 4°C.
Photosynthetically active radiation (PAR; µmol photon m-2 s-1) was measured with Odyssey Submersible PAR loggers (5 min interval; cross-calibrated against a LI-COR® LI-250A light meter with an attached LI-192 underwater quantum sensor). One PAR logger for each light treatment (unshaded and 30% shade) was placed in a water bath at coral depth. Temperature (°C) was measured with HOBO pendant loggers (HOBO MX-22021, Onset, USA; 15 min interval), respectively. Temperature loggers were placed in a separate experimental tank.
Dissolved inorganic nitrate and dissolved inorganic phosphate were determined with 20 mL samples of sand-filtered water taken every two days. Samples were filtered with polyethersulfone hydrophilic, non-sterile 0.45 µm filters (Minisart®, Part 16537—Q) into 30 mL polypropylene vials. Samples were frozen (– 18°C) and stored for analysis. Dissolved inorganic nitrate (3.35 ± 1.14 µmol/L) and phosphate (0.58 ± 0.20 µmol/L) were determined by use of standard methods 4500-No-3 G., 4500-NH3 H., and 4500-P (Eaton, 2005), with a flow injection LaChat 8500 autoanalyzer (Jeffries et al., 2015). Salinity (35 ± 0.42 ppt), dissolved oxygen (7.36 ± 0.3 mg/L), and pH (8.11 ± 0.03) were measured daily (Hach HQ40d).
2.3 Mean intensity of grey and photochemistry
Percentage mean intensity of grey (%MIG) is a colour analysis technique using digital photographs of coral fragments to quantify ‘percentage whiteness’ (McLachlan and Grottoli, 2021). %MIG was used as a physical indication of coral health. Repeated measures of %MIG were conducted at the beginning (day zero) and every three days after that. Measurements were conducted on all coral fragments that remained after each destructive sampling. Algal growth was removed before %MIG measurements. Fragment tips were avoided as these are generally the palest part of a healthy coral colony (Page et al., 2023). Images of the coral side profile were conducted at midday with consistent settings (Canon EOS R6 mirrorless, Canon RF 24-105 f/4 – 7.1 IS STM lens, SS: 1/100, focal length: 70 mm, manual white balance: 6000K, ISO-100, F = 8) in a consistent light environment from a ring light (Neewer® RF550D). Images were converted to greyscale and analysed in ImageJ (ver. 1.53t) following McLachlan and Grottoli (2021). Twenty randomly placed points per fragment were selected and normalised against four white standard points. The analysis avoided areas of the image distorted by surface stress, glare, shadow, or suspended particles. From %MIG images, coral fragments with tissue loss were quantified.
Symbiont photochemistry was measured by pulse amplitude modulated (PAM) fluorometry. Repeated measures were collected at the beginning (day zero) and then every two days thereafter. Measurements were conducted on all coral fragments that remained after each destructive sampling. The fiber-optic probe of the PAM fluorometer (©Heinz Walz diving PAM I) was positioned ~3 mm away from the coral tissue. The dark-adapted photochemical efficiency of open reaction centres (Fv / Fm; dimensionless) was conducted predawn to allow for maximum recovery of the photosystem and relaxation of photoprotective mechanisms. Rapid light curves (RLCs) evaluated photosynthetic performance and provided saturation characteristics of electron transport. RLCs were conducted on dark-adapted (1 h after sunset) corals; actinic light pulses of ~0 – 3121 µmol photon m-2 s-1 were delivered every 20 s. PAM settings were actinic light factor = 1, actinic light intensity = 5, saturation width = 0.8, saturation intensity = 12, signal damping = 2, and gain = 2. The maximum non-photochemical quenching coefficient (dimensionless; NPQ; Stern-Volmer quenching) was obtained from RLCs by selecting the NPQ values determined at the last actinic light step (3121 µmol photon m-2 s-1). The absorption factor was not defined for A. kenti or A. divaricata. Instead, the RLC photosynthetic capacity (relative electron transport rate, rETR) was calculated as PSII quantum yield x PAR. The rETR values were fitted to the Platt et al. (1980) model using the ‘phytotools’ 1.0 package in R (Silsbe and Malkin, 2015). The rise of the light-limited region (alpha (dimensionless); α), the maximum photosynthetic capacity (µmol electron m-2 s-1; rETRMAX), and the light saturation coefficient (µmol photon m-2 s-1; Ek) were determined for each fragment.
2.4 Laboratory analyses
2.4.1 Coral tissue extraction
For laboratory analysis, four fragments of each species per treatment were destructively sampled at baseline [0 degree-heating week (DHW)] and for each treatment at ~1, 3, and 4.4 DHW. Compressed air delivered at 40 L min-1 at 7 bar, from an electric air compressor (Black Ridge, Australia) was used to extract coral tissue. Tissue removal was facilitated using 1.6 mL of ice-cold 0.01 M phosphate-buffered saline (PBS; Sigma-Aldrich P3813). The removed tissue was collected into two pre-weighed tubes (Rangel et al., 2019), snap-frozen in liquid nitrogen, and stored at – 80°C until analysis.
2.4.2 Symbiodiniaceae density and chlorophyll a concentration
To determine symbiont density and chlorophyll a concentration, one of the two collected tissue samples from each coral fragment was centrifuged at 3500 × g for 5 min at 4°C. The supernatant was discarded, and the resultant tissue was weighed to determine tissue wet weight. The pellet was resuspended in 0.75 mL of PBS and homogenised with a TissueLyser LT (Qiagen) at 40 Hz for 2 min. The lysate was centrifuged with the abovementioned settings, the supernatant removed, and the tissue resuspended in 0.75 mL of PBS. Samples were kept on ice throughout. Following further centrifugation, the supernatant was discarded, and the sample was resuspended in 1.6 mL of PBS and divided equally between two microcentrifuge tubes for analysis. From one tube, symbiont density was determined by cell counting with a Neubauer haemocytometer (Marienfield, Germany) and compound microscope (Leica Zoom S6D). The second sample tube was used to determine chlorophyll a concentration. It was centrifuged at 3500 × g for 5 min at 4°C and the supernatant was discarded. Chlorophyll a was extracted with 1.5 mL of 100% undenatured ethanol and incubated at 4°C in the dark overnight. After incubation, samples were centrifuged at 7000 × g for 10 min at 4°C. Chlorophyll a concentration was determined by measuring absorbance at 629 and 665 nm following Warren (2008) and quantified using coefficients from Ritchie (2006). Symbiont density and chlorophyll a concentration were normalised against surface area, obtained using the wax dipping method (Stimson and Kinzie, 1991).
2.4.3 Biochemical assays
The second tissue sample for each fragment was used to assess catalase-specific activity and lipid peroxidation.
A 50 µL aliquot of tissue homogenate was used to estimate catalase (CAT) activity at 240 nm following Beers and Sizer (1952), with modifications for microplate (Li and Schellhorn, 2007). The change in absorbance of 0.01 M hydrogen peroxide was used to estimate catalase activity using a molar extinction coefficient of 43.6 M-1 cm-1. The CAT-specific activity was expressed as units mg-1 protein (Cuéllar Cruz et al., 2009), in which 1 unit of CAT is required to decompose 1.0 µM of H2O2 per minute at 25°C (Weydert and Cullen, 2010).
Lipid peroxidation was determined using a lipid peroxidation assay kit (Sigma-Aldrich; MAK085-1KT). The reaction of malondialdehyde (MDA) and thiobarbituric acid formed a colourimetric product that was measured at 532 nm. The concentration of MDA was estimated from a standard curve and expressed as nmole MDA mg-1 protein.
Protein in cell lysates was determined at 595 nm following Bradford (1976), with bovine serum albumen as the standard. Both coral species were tested for high protein: for A. kenti samples, 20 μL of lysate, and for A. divaricata, 15 μL of lysate was used. The concentration of protein was estimated from a standard curve and expressed as mg mL-1.
All spectrophotometric analyses used a FLUOstar® Omega microplate reader (BMG Labtech, Australia) and were corrected for path length. Results were normalised to tissue weight or protein concentration.
2.5 Statistical analyses
Independent replicates were used to test for significant differences in the CAT, LPO, symbiont density, and chlorophyll a concentration data at the destructive sampling times of 1, 3, and 4.4 DHW. Permutational Multivariate Analysis of Variances (PERMANOVA; Factor 1: temperature, two levels and fixed, Factor 2: shade, two levels and fixed; Anderson, 2017) was conducted in Primer v6 & PERMANOVA+ (Clarke and Gorley, 2006). PERMANOVA analyses used a normalised Euclidean distance dissimilarity matrix and a Type I (CAT and LPO) or Type III (symbiont density and chlorophyll a concentration) sum of squares, with 9999 raw data permutations. Pair-wise comparisons were used when significant main effects were detected (α ≤ 0.05). For the comprehensive set of statistical results, see Supplementary Table 1.
Repeated measures analysis of variance (ANOVA) models were fitted to the data to analyse the variation in %MIG and the PAM parameters of Fv / Fm, NPQ, Ek, rETRMAX, and alpha with SPSS (version 29.0, SPSS Inc., IBM, USA). For each parameter, a full factorial model was initially fitted with time as a within-subject factor and shade and temperature as between-subject factors. There was a maximum of seven sampling events for %MIG and a maximum of eight sampling events for Fv / Fm, NPQ, Ek, rETRMAX, and alpha. Assumptions of sphericity were tested with Mauchly’s test. When this assumption was violated, the degrees of freedom were adjusted with the Greenhouse-Geisser correction factor. Non-significant higher-order interactions were removed first. When significant differences were detected (α ≤ 0.05), pairwise comparisons were adjusted by Bonferroni correction.
Multiple regression analysis tested for a statistically significant relationship (α ≤ 0.05) between chlorophyll a (µg cm-2; Y1) and symbiont density (cells cm-2; (x1)1) and %MIG [(x2)1]. Independent replicates and destructive and non-destructive data collected within a one-day timeframe were used for analysis. Regression analyses were conducted in MATLAB version 7 (MATLAB, 2022).
3 Results
3.1 Environmental variables
The mean ambient temperature remained at or below the MMM (Figure 1A). When the ambient temperature treatments exceeded the MMM for brief periods, the resulting DHW accumulation was small (0.27 DHW). The heat stress treatments accumulated a total of 4.4 DHW. Most of the temperature stress was accumulated after day 11 (Figure 1B). The daily PAR irradiance peaked at 818 ± 406 µmol photon m-2 s-1 (mean ± standard deviation; 10:15 am – 12:35 pm; Figure 1C). This measured irradiance was slightly higher than PAR irradiance for a summer season (1st Dec 2016 – 28th Feb 2017) at Jenny Louise Shoal Reef, GBR; midday average of 680 ± 236 µmol photon m-2 s-1 at a depth of ~4 m (data obtained from eReefs via the Relocatable Coastal Model (https://research.csiro.au/ereefs/models/models-about/recom/), with a 5000 x 5000 m domain (250 m resolution), GBR1 for initialisation and boundary data, and BGC2p0). At midday, cloud cover > 50% dominated the experiment (16 out of 23 days). From days 10 – 12, the peak light (unshaded treatment) was 811 – 1217 µmol photon m-2 s-1 (DLI for days 10 – 12 of 6.24 – 10.66 mol photon m-2 d-1), which decreased on days 13 – 14 to 180 – 252 µmol photon m-2 s-1 (DLI for days 13 – 14 of 2.64 – 3.41 mol photon m-2 d-1). The application of shade (~30%) was most effective at reducing photosynthetically active radiation during times of peak light (10:15 am – 12:35 pm). The experimental DLI in the unshaded and 30% shade treatment was 7.21 ± 2.99 mol photon m-2 d-1 and 4.48 ± 1.80 mol photon m-2 d-1, respectively.
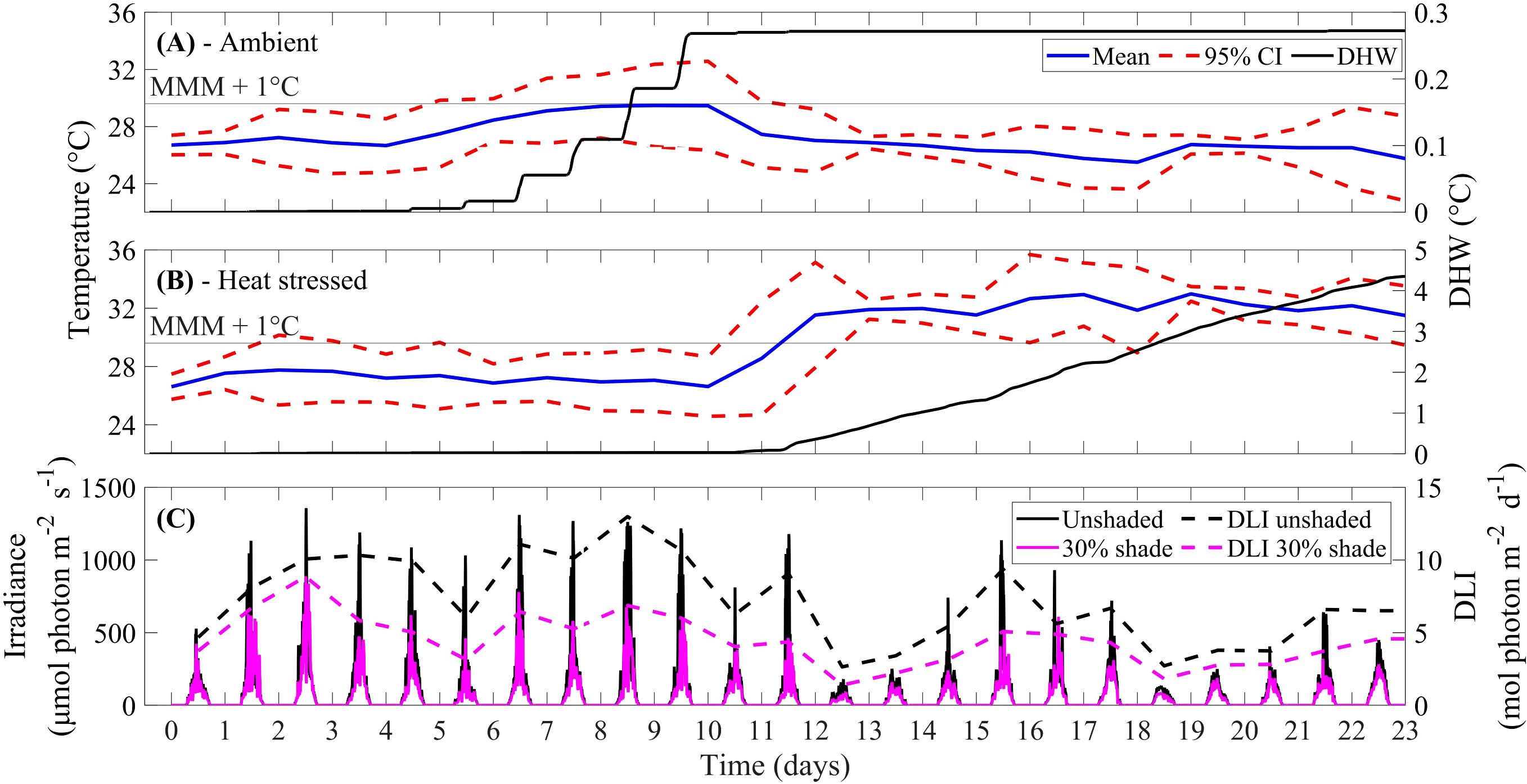
Figure 1. (A) Recorded water temperature (mean temperature per day with 95% confidence interval (CI)) and degree heating week (DHW) accumulation for ambient (nominal 26.4°C) and (B) heat-stressed (nominal 32.6°C) temperature treatments, and maximum monthly mean (MMM; 28.6°C) + 1°C. (C) Logged PAR irradiance (μmol photon m-2 s-1) and daily light integral (DLI; mol photon m-2 d-1) at depth of coral fragments, in the unshaded and 30% shade treatment.
3.2 Mean intensity of grey
For A. kenti, the %MIG was greater in the 30% shade (33.61 ± 15.89) than in the unshaded treatment (31.67 ± 15.89) (P < 0.05; Table 1). For both A. kenti and A. divaricata, %MIG varied through time (Figures 2A, B). %MIG was greater in the heat stress treatment at later sampling times of 14 – 20 days (P < 0.01). No significant difference in %MIG was observed amongst treatments for A. divaricata and A. kenti (P > 0.05) at the start of the experiment. The number of coral fragments with tissue loss was greatest in the heat stress treatment and the unshaded and heat stress treatments for A. kenti and A. divaricata, respectively (Supplementary Table 2).
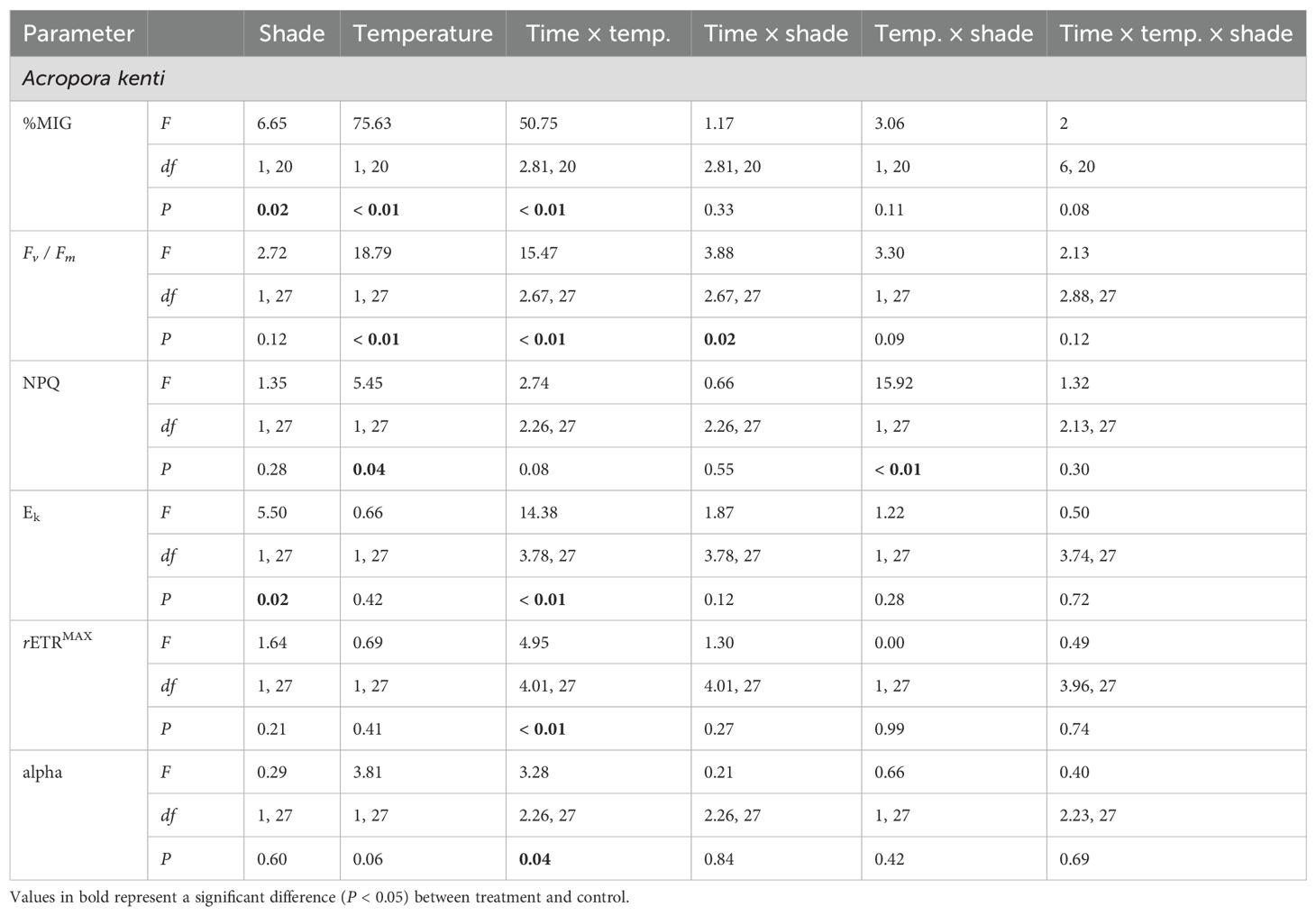
Table 1. Repeated measures ANOVA results for Fv/ Fm, %MIG, NPQ, Ek, rETRMAX, and alpha for Acropora kenti.
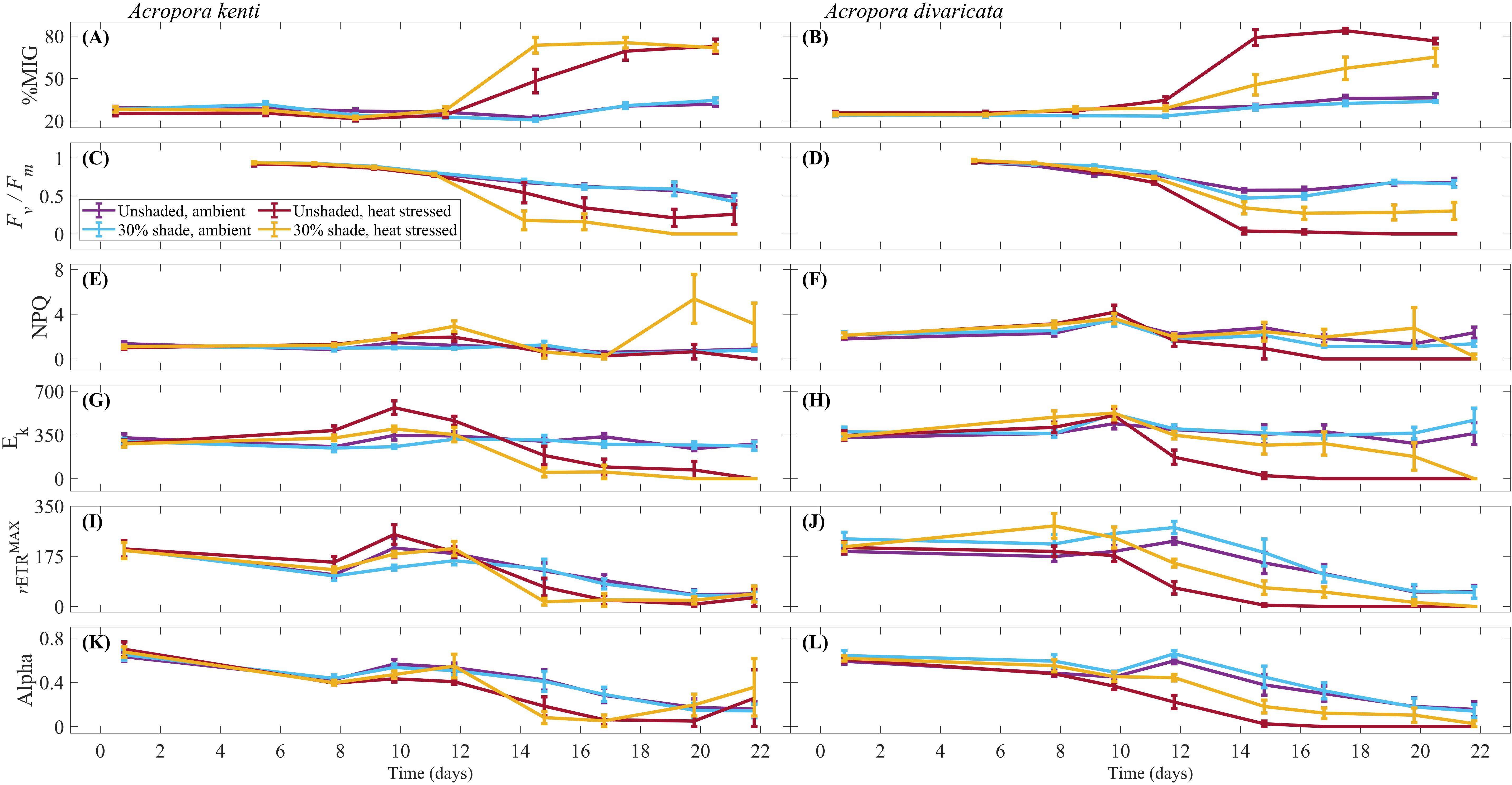
Figure 2. Average percentage mean intensity of grey [%MIG; (A, B)] at 0, 5, 8, 11, 14, 17 and 20 days, maximum quantum yield [dimensionless; Fv / Fm; (C, D)] at 5, 7, 9, 11, 14, 16, 19 and 21 days, non-photochemical quenching [dimensionless; NPQ; (E, F)], light saturation coefficient [μmol photon m-2 s-1; Ek; (G, H)], maximum photosynthetic capacity [μmol electron m-2 s-1; rETRMAX; (I, J)] and alpha [dimensionless; (K, L)] at 0, 7, 9, 12, 14, 16, 19 and 21 days, for Acropora kenti and Acropora divaricata for the treatments of ambient temperature and heat stressed, unshaded and 30% shade, ± standard error.
3.3 Photochemistry
For A. kenti, the Fv / Fm between the temperature treatments varied over time (Table 1; Figure 2C). Fv / Fm in the ambient temperature treatment decreased from 5 to 11, and 16 – 21 days (Bonferroni, P < 0.01). Fv / Fm in the heat stress treatment decreased from 5 to 11 – 21 days (Bonferroni, P < 0.01). Fv / Fm was significantly greater in the ambient temperature treatment at 14 – 19 days (P < 0.01). For A. divaricata, shading prevented photochemical collapse up to the maximum of 4.4 DHW (Figure 2D). Fv / Fm was greater in the 30% shade [0.73 ± 0.26 (dimensionless)] than in the unshaded treatment [0.67 ± 0.32 (dimensionless)] (P < 0.05; Table 2) and varied in the temperature treatments over time (P < 0.01). Fv / Fm was greater in the ambient temperature treatment at 9 – 19 days (P < 0.01). Fv / Fm in the heat stress treatment decreased from 5 to 9 – 21 days (Bonferroni, P < 0.01). Fv / Fm in the ambient temperature treatment decreased from 5 to 11 – 21 days (Bonferroni, P < 0.01). Absolute Fv / Fm values are presented in Figure 2; considering these high values at the start of the experiment, only the relative differences in these values were used to make inferences about the data.
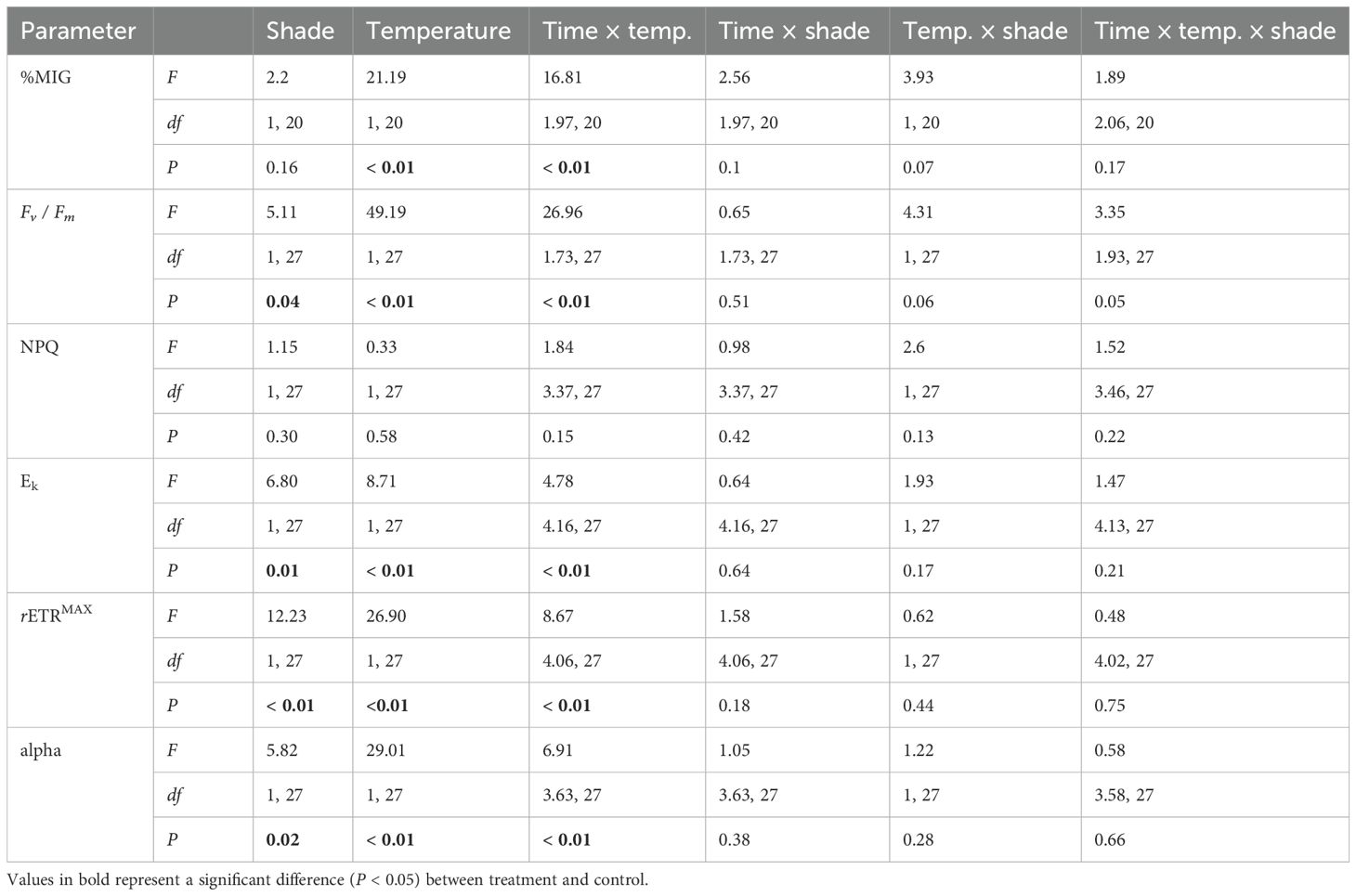
Table 2. Repeated measures ANOVA results for Fv / Fm (dimensionless), %MIG, NPQ (dimensionless), Ek (µmol photon m-2 s-1), rETRMAX (µmol electron m-2 s-1), and alpha (dimensionless) for Acropora divaricata.
For A. kenti, the NPQ varied between temperature treatments in the shaded treatment and between the shade treatments in the heat stress treatment (P < 0.05; Table 1). NPQ was greatest in the 30% shade, and heat stress treatment (Figure 2E). At 21 days, A. kenti was visibly paler, and NPQ was undetectable, whereas for A. divaricata, NPQ was undetectable for the last three sampling times (16 – 21 days; Figure 2F).
For A. kenti, the Ek was greater in the unshaded treatment (233.69 ± 203.90 µmol photon m-2 s-1) than in the 30% shade treatment (194.37 ± 168.2 µmol photon m-2 s-1) and varied between the temperature treatments through time (P < 0.05; Table 1). Ek in the heat stress treatment increased from 0 to 9 – 12 days and decreased from 0 to 14 – 21 days (Bonferroni, P < 0.01; Figure 2G). For A. divaricata, Ek was greater in the 30% shade (283.52 ± 223.89 µmol photon m-2 s-1) than in the unshaded treatment (231.15 ± 218.62 μmol photon m-2 s-1) (P < 0.05; Table 2; Figure 2H) and varied between the temperature treatments through time (P < 0.01). Ek in the heat stress treatment decreased from 0 to 14 – 21 days (Bonferroni, P < 0.01). Ek increased in the ambient temperature treatment between 0 and 19 – 21 days (Bonferroni, P < 0.01). Ek was greater in the heat stress treatment at 7 – 12 days (P < 0.01). Ek was greater in the ambient temperature treatment at 14 – 21 days (P < 0.01).
For A. kenti, the rETRMAX varied between the temperature treatments through time (P < 0.01; Table 1). rETRMAX in the ambient temperature treatment decreased from 0 to 7, 16 – 21 days (Bonferroni, P < 0.01; Figure 2I). rETRMAX in the heat stress treatment decreased from 0 to 14 – 21 days (Bonferroni, P < 0.01). At day 7, rETRMAX was greater in the heat stress treatment; at 14 – 16 days, rETRMAX was greater in the ambient temperature treatment (P < 0.01). For A. divaricata, rETRMAX was greater in the 30% shade (149.67 ± 130.02 µmol electron m-2 s-1) than in the unshaded treatment (112.39 ± 104.88 µmol electron m-2 s-1) and varied between the temperature treatments through time (P < 0.01; Table 2). rETRMAX in the heat stress treatment decreased from 0 to 12 – 21 days (Bonferroni, P < 0.01; Figure 2J). rETRMAX in the ambient temperature treatment decreased from 0 to 16 – 21 days (Bonferroni, P < 0.01). rETRMAX was reduced in the heat stress treatment at 12 – 21 days (P < 0.01).
For A. kenti, the alpha varied between the temperature treatments through time (P < 0.05; Table 1). Alpha in the ambient temperature treatment decreased from 0 to 16 – 21 days (Bonferroni, P < 0.05; Figure 2K). Alpha in the heat stress treatment decreased from 0 to 7 – 21 days (Bonferroni, P < 0.05). Alpha was greater in the ambient temperature treatment at 9, 14, and 16 days (P < 0.05). For A. divaricata, alpha was greater in the 30% shade [0.37 ± 0.28 (dimensionless)] than in the unshaded treatment [0.30 ± 0.26 (dimensionless)] (P < 0.05; Table 2) and varied between the temperature treatments through time (P < 0.01). Alpha in the heat stress treatment decreased from 0 to 14 – 21 days (Bonferroni, P < 0.01; Figure 2L). Alpha in the ambient temperature treatment decreased from 0 to 16 – 21 days (Bonferroni, P < 0.01). Alpha was reduced in the heat stress treatment at 9 – 21 days (P < 0.01).
No differences were observed amongst treatments for A. divaricata and A. kenti at the start of the experiment for each of the abovementioned photochemical parameters (P > 0.05).
3.4 Laboratory analyses
For A. kenti, no significant differences in catalase-specific activity were observed between the shade or temperature treatments (Figures 3A, B). For A. divaricata, the treatments of 30% shade and ambient temperature significantly increased catalase-specific activity at 4.4 DHW (F(1, 15) = 5.68, P < 0.05, and F(1, 15) = 8.13, P < 0.05, respectively). The catalase-specific activity was greater in the shaded than the unshaded treatment (3.91 ± 1.74 units mg-1 protein and 1.80 ± 1.64 units mg-1 protein, respectively (mean ± standard deviation); Figure 3C) and in the ambient temperature than heat stress treatment (3.73 ± 1.66 units mg-1 protein and 1.97 ± 1.92 units mg-1 protein, respectively; Figure 3D).
For A. kenti, the lipid peroxidation was significantly greater in the unshaded (170.07 ± 81.78 nmole MDA mg-1 protein) than in the 30% shade treatment (72.23 ± 56.61 nmole MDA mg-1 protein) at 3 DHW (F(1, 12) = 5.94, P < 0.05; Figure 3E). No significant differences in lipid peroxidation were observed between the temperature treatments (Figure 3F). For A. divaricata, no significant differences in lipid peroxidation were observed between the shade or temperature treatments (Figures 3G, H).
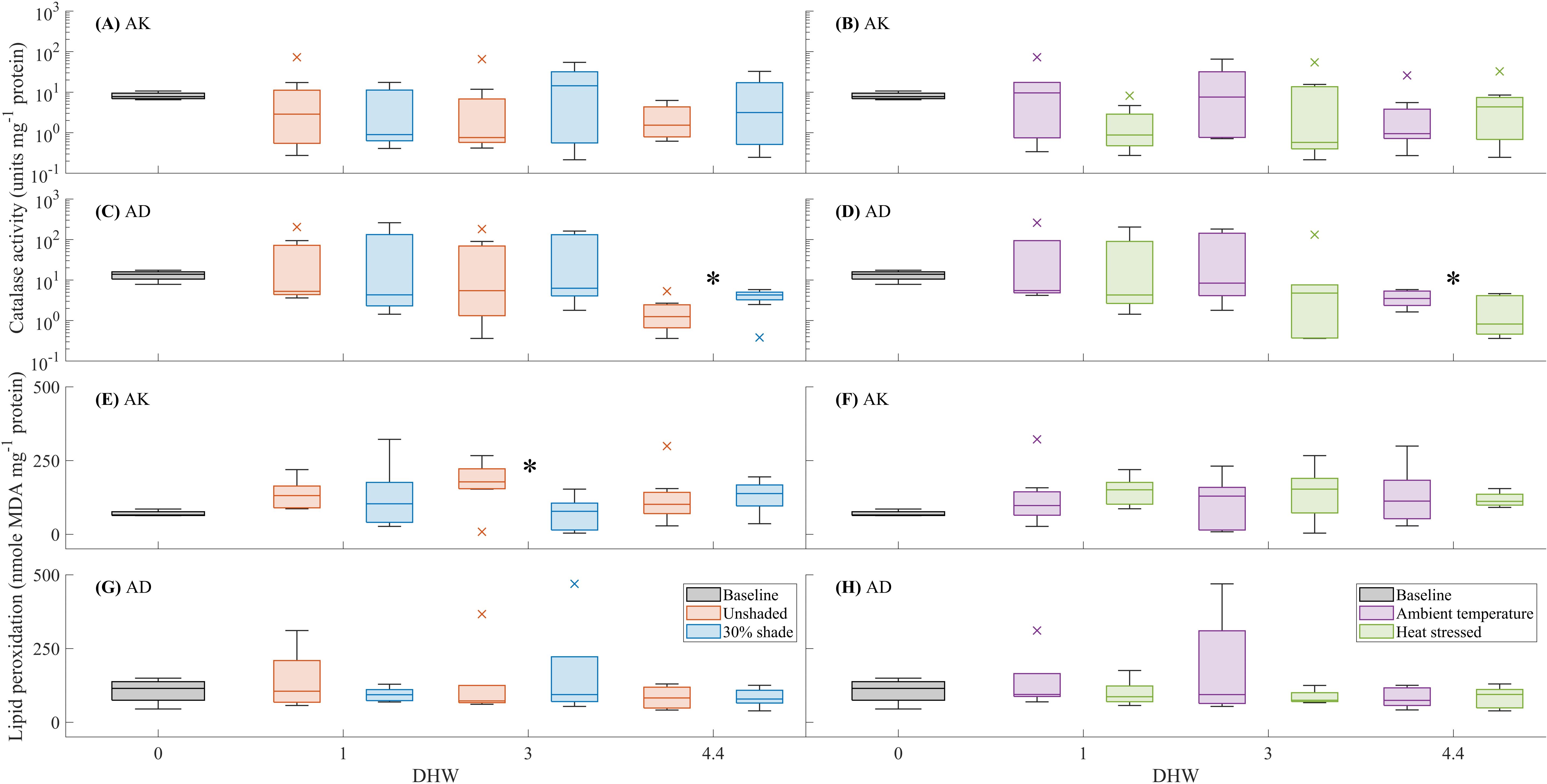
Figure 3. The effects of shade (unshaded or 30% shade) and temperature (ambient or heat stressed) on catalase activity (units mg-1 protein) and lipid peroxidation (nmole MDA mg-1 protein) for Acropora kenti (AK) [(A, B) and (E, F), respectively] and Acropora divaricata (AD) [(C, D) and (G, H), respectively], at 0 (baseline), 1, 3, and 4.4 DHW. The box charts show the median, the lower and upper quartiles, the minimum and maximum values, and the outliers of the data. Outliers (computed using interquartile range) are represented by the cross symbol. Significant differences (statistical analysis of independent replicates) in the shade or temperature treatments are signified by an asterisk (*) symbol.
For A. divaricata, the 30% shade treatment significantly increased symbiont density at 1 and 3 DHW (F(1, 15) = 12.22, P < 0.01, and F(1, 15) = 5.35, P < 0.01, respectively; Figure 4A). Symbiont density was greater in the shaded than in the unshaded treatment at 1 DHW (3.20 × 105 ± 2.70 x 105 cells cm-2 and 1.07 × 105 ± 5.32 × 104 cells cm-2, respectively) and 3 DHW (3.28 × 105 ± 3.65 × 105 cells cm-2 and 1.04 × 105 ± 1.81 × 105 cells cm-2, respectively). At ambient temperature, symbiont density was greater in the shaded than in the unshaded treatment (5.26 × 105 ± 2.39 × 105 cells cm-2 and 1.48 × 105 ± 8.65 × 103 cells cm-2, respectively), at 1 DHW (F(1, 15) = 7.28, P < 0.01). For both A. divaricata and A. kenti at all destructive sampling time points, symbiont density was greater in the ambient temperature than in the heat stress treatment (Figures 4B, D; see Supplementary Table 1). For A. kenti, no significant difference in symbiont density was observed between the shade treatments (Figure 4C).
For A. divaricata, the 30% shade treatment significantly increased chlorophyll a concentration at 1 and 3 DHW (F(1, 15) = 26.35, P < 0.01, and F(1, 15) = 7.32, P < 0.01, respectively; Figure 4E). Chlorophyll a concentration was greater in the shaded than in the unshaded treatment at 1 DHW (3.38 ± 1.38 µg cm-2 and 1.60 ± 0.94 µg cm-2, respectively) and 3 DHW (2.62 ± 2.49 µg cm-2 and 1.31 ± 1.74 µg cm-2, respectively). For A. divaricata, chlorophyll a concentration was greater in the ambient temperature than in the heat stress treatment at 1, 3 and 4.4 DHW (Figure 4F; see Supplementary Table 1). For A. divaricata, no significant differences in chlorophyll a concentration were observed between the shade treatments (Figure 4G). For A. kenti, chlorophyll a concentration was greater in the ambient temperature than in the heat stress treatment at 3 DHW (F(1, 15) = 25.78, P < 0.01; 5.32 ± 2.40 µg cm-2 and 0.57 ± 0.94 µg cm-2, respectively) and 4.4 DHW (F(1, 15) = 19.97, P < 0.01; 4.31 ± 2.40 µg cm-2 and 0.21 < 0.20 µg cm-2, respectively) (Figure 4H).
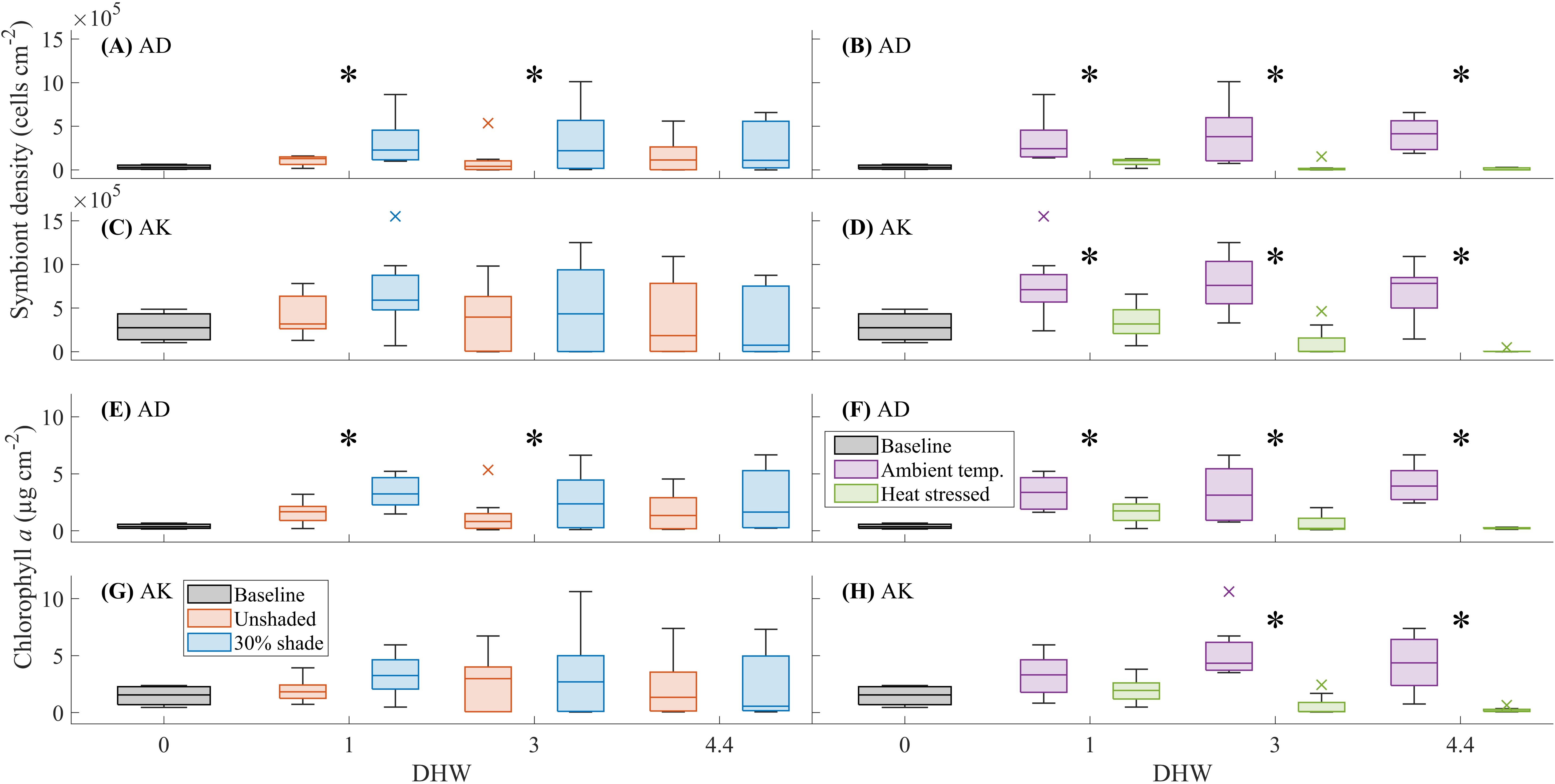
Figure 4. The effects of shade (unshaded or 30% shade) and temperature (ambient or heat stressed) on symbiont density (cells cm-2) and chlorophyll a concentration (µg cm-2) for Acropora divaricata (AD) [(A, B) and (E, F), respectively] and Acropora kenti (AK) [(C, D) and (G, H), respectively], at 0 (baseline), 1, 3, and 4.4 DHW. The box charts show the median, the lower and upper quartiles, the minimum and maximum values, and the outliers of the data. Outliers (computed using interquartile range) are represented by the cross symbol. Significant differences (statistical analysis of independent replicates) in the shade or temperature treatments are signified by an asterisk (*) symbol.
The overall regression between chlorophyll a and symbiont density and %MIG was statistically significant for each species (A. kenti: F(2, 45) = 35.74, P < 0.05, r2 = 0.61; A. divaricata: F(2, 45) = 194.86, P < 0.05, r2 = 0.89). The individual predictors were examined further and indicated that variations in chlorophyll a were best described by symbiont density and %MIG for both species; A. kenti (t = – 5.03, P < 0.05 and t = – 0.69, P < 0.05; Supplementary Figures 2A, B) and A. divaricata (t = 13.59, P < 0.05 and t = – 3.98, P < 0.05, respectively; Supplementary Figures 2D, E). With the combined variables of %MIG and symbiont density, the relationship varied from reasonably to highly linear for A. kenti (0.78) and A. divaricata (0.95), respectively. Over half of the variation in chlorophyll a could be explained for A. kenti (r2 = 0.60), whilst the variation in chlorophyll a was largely explained for A. divaricata (r2 = 0.90; Supplementary Figures 2C, F).
4 Discussion
4.1 The environmental stressors of light and temperature
Elevated temperature was the primary stressor in this experiment and negatively impacted the health of both coral species. Under ambient light (unshaded treatment) conditions, heat stress temperatures exacerbated coral bleaching stress. For each species at 1 DHW (in the heat stress treatment), Fv / Fm, Ek, rETRMAX, and alpha were reduced, and %MIG was greater, compared to the ambient temperature treatment. This decline in coral health occurred with a peak in maximum irradiance on day 11 and after an accumulation of temperature stress. With an accumulation of DHW, Fv / Fm, Ek, rETRMAX, and alpha for A. divaricata were reduced in the heat stress treatment at later sampling times. Similarly, parameters measured for A. kenti displayed a decline in coral health in the heat stress treatment at later sampling times; %MIG was greater, and Fv / Fm, rETRMAX, and alpha were reduced. The catalase activity for A. kenti was reduced at the last destructive sampling time in the heat stress treatment. For both species, chlorophyll a concentration and symbiont density were reduced under heat stress temperatures. Increased temperature was a consistent stressor in this experiment and negatively impacted the health of both coral species.
Shade did not consistently reduce bleaching impacts under heat stress conditions for both coral species. Shading prevented a significant photochemical decline under heat stress but for A. divaricata only. The photochemical parameters of Fv / Fm, Ek, rETRMAX and alpha were greater in the shaded than in the unshaded treatment, and thus displayed a reduced photochemical decline. For A. divaricata, symbiont density and chlorophyll a concentration were reduced in the shaded treatment, whilst no shade effects were shown for A. kenti. For A. kenti, a negative response to shade was shown; %MIG was greatest in the shaded treatment, and Ek was greatest in the unshaded treatment. Species-specific responses were also shown in Butcherine et al. (2023), with Turbinaria reniformis more responsive to shading than Duncanopsammia axifuga. Thirty percent shade for 4 h per day delayed bleaching in T. reniformis whereas D. axifuga was unresponsive to 24 hours of shade beyond 3 DHW. Shading as an intervention may not reduce bleaching in all coral species equally, as evidenced by the species-specific responses to shading in this experiment. The species-specific effects suggest that shade intensity could be increased beyond 30% to observe positive shade benefits for a range of coral species. Conversely, shading may negatively impact coral health, as demonstrated by the responses of A. kenti in this experiment.
Frequent fluctuations in experimental light highlighted the importance of recurrent measurements for tracking short-term changes in coral photochemical health. Light fluctuated daily in the experiment and even hourly with cloud presence. With constant fluctuations in light, acclimatisation is not instantaneous, thus photochemical parameters, such as Ek, may go through changes (Sakshaug et al., 1997; Ralph and Gademann, 2005). Recurrent measurements are important for tracking short-term changes in coral health. A reduced light saturation coefficient (Ek) indicates reduced photochemical quenching and elevated non-photochemical quenching, thus decreasing the light required to saturate photosynthesis. A reduced Ek from increased light exposure was shown in Acropora valenciennesi, Porites cylindrica (Jurriaans and Hoogenboom, 2020), and Siderastrea stellata (Tunala et al., 2019). Changes to Ek were observed over a short timeframe; in comparison to Ek at day zero, Ek was greater before DHW accumulation and then was reduced beyond 1 DHW. Changes in Ek were observed for Turbinaria mesenterina in as few as 5 – 10 days (Anthony and Hoegh-Guldberg, 2003). Roth et al. (2010) also demonstrated photochemical changes over short periods for Acropora yongei. Photochemical declines in coral health, specifically a decreased rETRMAX, Ek, and alpha, have been shown with pathogenic coral disease syndromes (Ralph et al., 2015). Photochemical decline around lesion borders arose from reduced light attenuation from disintegrating tissues and increased light reflectance from an exposed coral skeleton (Enríquez et al., 2005; Ralph et al., 2015). The use of ambient light in this experiment, which varied with prevailing conditions, is most representative of the environmental variations experienced by corals in their natural environment.
4.2 The effect of shade on Acropora divaricata
Shade delayed bleaching effects in A. divaricata. A. divaricata in the ambient temperature and shaded treatment displayed less photochemical and physical bleaching effects. Under heat stress, unshaded A. divaricata fragments had elevated percentage whiteness and undetectable Fv / Fm values from early tissue loss and extreme bleaching from ~ 1 DHW (13 – 14 days). NPQ values exceeded moderate photoinhibition.
Catalase activity was elevated in the ambient temperature treatment, and in the 30% shade treatment. In this experiment, an increased catalase activity in these treatments of reduced heat and light stress perhaps indicates a functioning antioxidant system. In contrast, fragments in the heat stress and unshaded treatment that suffered from tissue loss had significantly reduced catalase activity and a reduced capacity to respond to stress. Elevated antioxidant enzyme activity is indicative of increased levels of reactive oxygen species (Lesser et al., 1990), and catalase activity can be elevated by increased temperature (Higuchi et al., 2008; Butcherine et al., 2023). Consistent with evidence of ROS accumulation triggering coral bleaching, Levin et al. (2016) detected an increased ROS leakage from Symbiodinium cells in a heat stress treatment. In instances where significant tissue necrosis isn’t documented, shading is shown to suppress catalase activity (Butcherine et al., 2023). In our shaded treatment, catalase activity was greater, and an improved coral health was indicated by increased Fv / Fm, Ek, rETRMAX and alpha. We expect that severe bleaching in the unshaded and heat-stressed temperature treatment, demonstrated by tissue necrosis, impacted the activity of catalase. Thus, 30% shade alleviated the physiological bleaching response for A. divaricata.
A. divaricata displayed moderate photoinhibition in the unshaded, heat stress treatment. NPQ as a photoprotective mechanism did not efficiently alleviate light stress in the unshaded treatment. NPQ dissipates excess absorbed excitation energy as heat by the photosystems’ antennae (Ruban, 2016). Before 1 DHW, Fv / Fm steadily declined, and NPQ values suggested a moderate degree of photoinhibition (NPQ 1.5 – 2; Ralph and Gademann, 2005). NPQ may have been functioning at low DHW accumulation to combat the increase in light irradiance experienced by corals transitioning from holding tank to experiment. The peak experimental light was highest before day 13. In Banc-Prandi et al. (2022), higher values of NPQ at lower PAR were shown for corals sampled from colder ambient conditions and thus sensitive to higher irradiance at lower temperatures. In the unshaded, heat stress treatment, NPQ values exceeded moderate photoinhibition but decreased with further DHW accumulation, eventually reaching zero at 4.4 DHW. According to the optical feedback-loop hypothesis of coral bleaching; as Symbiodiniaceae are expelled, reduced Symbiodiniaceae abundance and pigmentation further expose the skeleton (Enríquez et al., 2005). An exposed skeleton disproportionately increases the light available to remaining Symbiodiniaceae, further exacerbating their light stress and accelerating subsequent Symbiodiniaceae expulsion (Enríquez et al., 2005; Marcelino et al., 2013). An increased irradiance under heat stress conditions may have overwhelmed NPQ as a photoprotective mechanism for the thermal dissipation of excess light energy (Enríquez et al., 2005).
Although shading alleviated bleaching for A. divaricata, substantial declines in coral health were still observed in the 30% shade, heat-stressed treatment. The photochemical parameters of Fv / Fm, Ek, rETRMAX and alpha measured for A. divaricata decreased significantly with DHW accumulation. All fragments in the heat stress treatment were recorded with tissue loss at day 20. Similarly, all fragments were recorded with tissue loss in the unshaded, ambient temperature treatment. Regardless of the temperature treatment, unshaded light induced a bleaching response. Acclimatisation to a lower baseline temperature and a lower consistent light level post-summer season could explain the rapid decline in coral health observed in the experiment. Photoacclimation in Symbiodiniaceae occurs continuously and is an adaptation accounted for in the Light Stress Damage algorithm (Skirving et al., 2017). While studies have proposed the rearrangement of coral symbiont community compositions to favour thermally tolerant species when in warmer temperatures (Berkelmans and van Oppen, 2006; Cunning et al., 2018; Claar et al., 2020) following temperature-induced coral bleaching, the reverse may also be applicable. Shuffling the symbiont community composition to a species favourable to the pre-experimental conditions could make fragments vulnerable to increased water temperature and unshaded light conditions. Therefore, both light and temperature are considered stressors in the bleaching response of A. divaricata. The combination of heat-stress temperature and unshaded light conditions may have exacerbated the vulnerability of A. divaricata.
An increased shade intensity under thermal stress may be beneficial for A. divaricata to further reduce bleaching impacts and prevent the photochemical efficiency from decreasing significantly. The optimal level of shade that prevents significant photochemical decline should be determined for a range of coral species, morphologies, and varying environmental conditions. Most studies investigating the effects of reduced light have used shade levels higher than 50% (Tagliafico et al., 2022). However, different coral species are expected to respond differently to variable levels of light (Tilstra et al., 2017; Wangpraseurt et al., 2019; Dobson et al., 2021). The primary shading interventions proposed to protect corals under thermal stress (shade cloths, fogging using seawater, reflective surface films, and marine cloud brightening) revolve around reducing the light environment at the coral surface. Further research is necessary to clarify the appropriate shade level for protecting various corals against environmental stress conditions to ensure the deployment of shade-based interventions is beneficial.
4.3 The effect of shade on Acropora kenti
Photochemical and physical indicators of coral health measured for A. kenti responded negatively to shade. Bleaching responses were more pronounced in the shaded, heat-stressed treatment. Under 30% shade, A. kenti had a reduced Fv / Fm, an increased %MIG, but a reduced lipid peroxidation compared to the unshaded treatment. Lipid peroxidation was greatest at 3 DHW in the unshaded A. kenti. These indicators of coral health were contradictory for A. kenti. In tissues of Agaricia agaricites, Sandeman (2008) also reports increased lipid peroxidation after exposure to light and temperature stress. The conflicting results for A. kenti may be attributed to biomarker sensitivity; different markers capture different aspects of coral health as physical, physiological, or photochemical parameters.
4.4 Considerations
Symbiont density and mean intensity of grey were suitable predictors of chlorophyll a. %MIG and symbiont density were negatively and positively correlated with chlorophyll a, respectively. An increase in percentage whiteness proportional to a loss in chlorophyll a and symbiont density was similarly reported for Goniopora lobata (Chow et al., 2016). A combination of stressors including elevated water temperature resulted in a colour loss, proportional to a decrease in chlorophyll a and Symbiodiniaceae for Acropora formosa in Amid et al. (2018). Our results confer that coral colour is related to pigment concentration, regulated by symbiont density. Chlorophyll a does not, however, directly reflect symbiont abundance as its content varies widely by symbiont cell (Chang et al., 1983). Variability in pigment content may be informative of symbiont photosynthetic capacity; in Scheufen et al. (2017), the amount of pigmentation and/or symbionts required to achieve maximum light absorption varied among species. The unaccounted variation in chlorophyll a may be explained by the time lag between non-destructive sampling of %MIG and destructive sampling of chlorophyll a and symbiont, as the time between sampling was up to ~ 1 DHW. It is important to note that chlorophyll a fluorescence signal may be influenced by tissue thickness. For instance, tissues of Porites spp. have been reported to be shaded from solar irradiance as they are shielded deeper within the skeleton, compared with tissues of Acropora spp (Hoegh-Guldberg, 1999). Within coral tissue, different cell layers have different light environments and light histories (Ralph and Gademann, 2005), so chlorophyll a fluorescence results may depend upon the cell layer sampled. The presence of endolithic algae within thin tissue layers may confound chlorophyll fluorescence. Fine et al. (2004) found dark-adapted Fv / Fm to be significantly higher on a bleached section of the coral colony than from the colony’s isolated symbionts, thus suggesting the fluorescence signal to be dominated by the endolithic algae from Ostreobium sp. Consequently, a deviation in tissue thickness may influence species-specific photochemical responses to environmental stress. We recognise that the initial Fv / Fm values for A. divaricata and A. kenti presented in Figure 2 are higher than those reported in the literature for the symbiont photochemistry of unstressed corals [values of 0.6 to 0.7; Franklin et al. (2004); Bhagooli (2009)]. Thus, conclusions made in this paper regarding Fv / Fm measurements are based on the relative differences between absolute values.
The benefit of reduced light levels during heat-stress conditions may not be universal in a coral reef environment. Low light conditions have been associated with a negative effect on corals. For instance, low light levels can impact coral growth (Comeau et al., 2014), survival, and recruitment (Baird and Hughes, 2000). Akin to the results of this experiment, solar radiation management may alleviate or exacerbate coral bleaching stress depending on the species. Coral reefs are ecologically diverse environments that host multiple coral species. The structural complexity of coral reefs supports this species richness. Given the species-specific responses in this experiment, further investigation is required to assess the effects of shade on coral bleaching in a range of coral morphologies. We also recommend using multiple shading levels to determine the optimal level of shade for reducing bleaching effects caused by environmental stress. These results will inform the feasibility of shade-based interventions for alleviating coral bleaching at reef-scale. Manipulative experiments are used to isolate the effects of specific variables on coral bleaching. They remain a critical tool for understanding the mechanisms underlying the coral bleaching response (McLachlan et al., 2020). Semi-controlled experiments cannot account for all potential drivers of coral bleaching, namely flow dynamics, heterotrophic nutrition, microbial communities, and physical disturbance. We acknowledge that the coral responses observed in the experiment may not be fully representative of those occurring in the natural environment.
5 Conclusions
This study confirmed that moderate levels of shade could alleviate bleaching stress in one of the two coral species selected. While the effects of temperature were consistent across the shade treatments for each species, the impact of shade on bleaching differed between species and the response variable measured. Shade applied continuously reduced the photochemical effects of bleaching under elevated temperature in A. divaricata only. An increased level of shade for this species may be advisable for further protection against coral bleaching impacts. Shade did not provide the same benefits for A. kenti, which, by some indicators, declined more rapidly in the shaded treatment. These results suggest that reduced light intensity during thermal stress accumulation may not benefit all coral species equally, and in some species, a reduced light intensity may negatively impact coral health. This study highlights the importance of investigating species-specific bleaching responses to assess the duration and level of shade most efficient for ameliorating bleaching impacts. Species-specific responses to a solar radiation management technique may implicate reef-scale management strategies, notably in reef locations that host a variety of coral morphologies.
Data availability statement
The raw data supporting the conclusions of this article will be made available by the authors, without undue reservation.
Ethics statement
Ethical approval was not required for the study involving animals in accordance with the local legislation and institutional requirements because Corals are not vertebrates or high-invertebrate organisms.
Author contributions
SE: Writing – original draft, Conceptualization, Data curation, Formal analysis, Investigation, Methodology. PB: Methodology, Writing – review & editing, Data curation. AT: Methodology, Writing – review & editing. CH: Investigation, Methodology, Writing – review & editing. BK: Methodology, Writing – review & editing. KS: Conceptualization, Methodology, Supervision, Writing – review & editing. DH: Conceptualization, Funding acquisition, Methodology, Supervision, Writing – review & editing.
Funding
The author(s) declare financial support was received for the research, authorship, and/or publication of this article. This work was undertaken as part of the Reef Restoration and Adaptation Program (Cooling and Shading sub-program), funded by the partnership between the Australian Government’s Reef Trust and the Great Barrier Reef Foundation.
Acknowledgments
The authors would like to acknowledge the Traditional Owners of the Gumbaynggirr country where this study was conducted and to all Traditional Owners of the Great Barrier Reef and its Catchments as First Nations Peoples holding the hopes, dreams, traditions, and cultures of the Reef. We also acknowledge assistance in fluorometric data collection provided by Lauren Hasson and Luke Austin and assistance in symbiont counts by Lauren Hasson from the cooling and shading sub-program of RRAP. Thank you to Caitlin Younis for advice on fluorometric data collection and settings. We thank Stephan Soule from the National Marine Science for advice on tank farm operation and experimental set-up.
Conflict of interest
The authors declare that the research was conducted in the absence of any commercial or financial relationships that could be construed as a potential conflict of interest.
The author(s) declared that they were an editorial board member of Frontiers, at the time of submission. This had no impact on the peer review process and the final decision.
Publisher’s note
All claims expressed in this article are solely those of the authors and do not necessarily represent those of their affiliated organizations, or those of the publisher, the editors and the reviewers. Any product that may be evaluated in this article, or claim that may be made by its manufacturer, is not guaranteed or endorsed by the publisher.
Supplementary material
The Supplementary Material for this article can be found online at: https://www.frontiersin.org/articles/10.3389/fmars.2024.1333806/full#supplementary-material
Supplementary Figure 1 | Species selection and experimental design. (A) Experimental fragments of Acropora divaricata (1, 2) and Acropora kenti (3, 4). Major tick marks in (A2) and (A4) denote centimeters. (B) Experimental treatments of shade (unshaded and 30% shade) and temperature (ambient, 26.4°C and heat stressed, 32.6°C) with four replicates per treatment, placed in water baths.
Supplementary Figure 2 | Measured versus predicted chlorophyll a (µg cm-2) for symbiont density [cells cm-2; Acropora kenti (A), Acropora divaricata (D)] and percentage mean intensity of grey [%MIG; Acropora kenti (B), Acropora divaricata (E)]. Predictive performance of the model for the combined predictor variables of %MIG and symbiont density [cells cm-2; Acropora kenti (C), Acropora divaricata (F)]; regression scatterplots show predicted versus measured chlorophyll a (µg cm-2). Each point on the scatterplot represents an observation.
SUPPLEMENTARY TABLE 1 | Summary of PERMANOVA analyses, displaying all results for catalase, lipid peroxidation, symbiont density, and chlorophyll a concentration, for Acropora kenti (AK) and Acropora divaricata (AD). Values in bold represent a significant difference (α < 0.05) between treatment and control.
SUPPLEMENTARY TABLE 2 | Number of coral fragments per treatment with tissue loss, at %MIG sampling days. Fragments of repeated measures were used (four fragments per treatment).
References
Ainsworth T., Hoegh-Guldberg O., Heron S., Skirving W., Leggat W. (2008). Early cellular changes are indicators of pre-bleaching thermal stress in the coral host. J. Exp. Mar. Biol. Ecol. 364, 63–71. doi: 10.1016/j.jembe.2008.06.032
Amid C., Olstedt M., Gunnarsson J. S., Le Lan H., Tran Thi Minh H., Van den Brink P. J., et al. (2018). Additive effects of the herbicide glyphosate and elevated temperature on the branched coral Acropora formosa in Nha Trang, Vietnam. Environ. Sci. pollut. Res. 25, 13360–13372. doi: 10.1007/s11356-016-8320-7
Anderson M. J. (2017). “Permutational multivariate analysis of variance (PERMANOVA),” in Wiley StatsRef: Statistics Reference Online. Eds. Balakrishnan N., Colton T., Everitt B., Piegorsch W., Ruggeri F., Teugels J. L. Massey University, Auckland, New Zealand: John Wiley & Sons, Ltd, 1–15.
Anthony K., Connolly S., Hoegh-Guldberg O. (2007). Bleaching, energetics, and coral mortality risk: Effects of temperature, light, and sediment regime. Limnol. Oceanogr. 52, 716–726. doi: 10.4319/lo.2007.52.2.0716
Anthony K. R. N., Helmstedt K. J., Bay L. K., Fidelman P., Hussey K. E., Lundgren P., et al. (2020). Interventions to help coral reefs under global change—A complex decision challenge. Public Library Sci. 15, 1–14. doi: 10.1371/journal.pone.0236399
Anthony K. R. N., Hoegh-Guldberg O. (2003). Variation in coral photosynthesis, respiration and growth characteristics in contrasting light microhabitats: an analogue to plants in forest gaps and understoreys? Funct. Ecol. 17, 246–259. doi: 10.1046/j.1365-2435.2003.00731.x
Baird A., Marshall P. (2002). Mortality, growth and reproduction in scleractinian coral following bleaching on the Great Barrier Reef. Mar. Ecol. Prog. Ser. 237, 133–141. doi: 10.3354/meps237133
Baird A. H., Bhagooli R., Ralph P. J., Takahashi S. (2009). Coral bleaching: the role of the host. Trends Ecol. Evol. 24, 16–20. doi: 10.1016/j.tree.2008.09.005
Baird A. H., Hughes T. P. (2000). Competitive dominance by tabular corals: an experimental analysis of recruitment and survival of understorey assemblages. J. Exp. Mar. Biol. Ecol. 251, 117–132. doi: 10.1016/S0022-0981(00)00209-4
Baird M., Mongin M., Bouget E. (2019). T13-Ultra-Thin Surface Films. A report provided to the Australian Government by the Reef Restoration and Adaptation Program. Available online at: https://gbrrestoration.org/resources/reports/. (Accessed July 11, 2023).
Baker A. C., Glynn P. W., Riegl B. (2008). Climate change and coral reef bleaching: An ecological assessment of long-term impacts, recovery trends and future outlook. Estuar. Coast. Shelf Sci. 80, 435–471. doi: 10.1016/j.ecss.2008.09.003
Ban S. S., Graham N. A. J., Connolly S. R. (2014). Evidence for multiple stressor interactions and effects on coral reefs. Global Change Biol. 20, 681–697. doi: 10.1111/gcb.12453
Banc-Prandi G., Evensen N. R., Barshis D. J., Perna G., Moussa Omar Y., Fine M. (2022). Assessment of temperature optimum signatures of corals at both latitudinal extremes of the Red Sea. Conserv. Physiol. 10, 2051–1434. doi: 10.1093/conphys/coac002
Bay L., Quigley K., Randall C., Negri A., van Oppen M., Webster N., et al. (2019). T3: Intervention Technical Summary. A report provided to the Australian Government by the Reef Restoration and Adaptation Program. Available online at: https://gbrrestoration.org/resources/reports/. (Accessed July 11, 2023).
Beers R. F., Sizer I. W. (1952). A spectrophotometric method for measuring the breakdown of hydrogen peroxide by catalase. J. Biol. Chem. 195, 133–140. doi: 10.1016/S0021-9258(19)50881-X
Berg J. T., David C. M., Gabriel M. M., Bentlage B. (2020). Fluorescence signatures of persistent photosystem damage in the staghorn coral Acropora cf. pulchra (Anthozoa: Scleractinia) during bleaching and recovery. Mar. Biol. Res. 16, 643–655. doi: 10.1080/17451000.2021.1875245
Berkelmans R. (2002). Time-integrated thermal bleaching thresholds of reefs and their variation on the Great Barrier Reef. Mar. Ecol. Prog. Ser. 229, 73–82. doi: 10.3354/meps229073
Berkelmans R., van Oppen M. J. H. (2006). The role of zooxanthellae in the thermal tolerance of corals: a ‘nugget of hope’ for coral reefs in an era of climate change. Proc. R. Soc. B: Biol. Sci. 273, 2305–2312. doi: 10.1098/rspb.2006.3567
Bhagooli R. (2009). Symbiont dependent thermal bleaching susceptiblity in two reef-building corals, stylophora pistillata and platygyra ryukyuensis. University of Mauritius Research Journal 15, 1–20.
Blanckaert A. C. A., de Barros Marangoni L. F., Rottier C., Grover R., Ferrier-Pagès C. (2021). Low levels of ultra-violet radiation mitigate the deleterious effects of nitrate and thermal stress on coral photosynthesis. Mar. pollut. Bull. 167, 112257. doi: 10.1016/j.marpolbul.2021.112257
Bradford M. M. (1976). A rapid and sensitive method for the quantitation of microgram quantities of protein utilizing the principle of protein-dye binding. Anal. Biochem. 72, 248–254. doi: 10.1006/abio.1976.9999
Bridge T. C. L., Cowman P. F., Quattrini A. M., Bonito V. E., Sinniger F., Harii S., et al. (2023). A tenuis relationship: traditional taxonomy obscures systematics and biogeography of the ‘Acropora tenuis’ (Scleractinia: Acroporidae) species complex. Zool. J. Linn. Soc. 1–24. doi: 10.1093/zoolinnean/zlad062
Brown B. (1997). Coral bleaching: Causes and consequence. Coral Reefs 1, 65–74. doi: 10.1007/s003380050249
Brown B. E., Ambarsari I., Warner M. E., Fitt W. K., Dunne R. P., Gibb S. W., et al. (1999). Diurnal changes in photochemical efficiency and xanthophyll concentrations in shallow water reef corals: evidence for photoinhibition and photoprotection. Coral Reefs 18, 99–105. doi: 10.1007/s003380050163
Brown B., Dunne R. (2008). Solar radiation modulates bleaching and damage protection in a shallow water coral. Mar. Ecol. Prog. Ser. 362, 99–107. doi: 10.3354/meps07439
Brown B., Dunne R., Goodson M., Douglas A. (2002). Experience shapes the susceptibility of a reef coral to bleaching. Coral Reefs 21, 119–126. doi: 10.1007/s00338-002-0215-z
Butcherine P., Tagliafico A., Ellis S. L., Kelaher B. P., Hendrickson C., Harrison D. (2023). Intermittent shading can moderate coral bleaching on shallow reefs. Front. Mar. Sci. 10. doi: 10.3389/fmars.2023.1162896
Cantin N. E., James N., Stella J. (2023). Aerial surveys of the 2024 mass coral bleaching event on the Great Barrier Reef. Available online at: https://www.aims.gov.au/research-topics/environmental-issues/coral-bleaching/coral-bleaching-events (Accessed May 21, 2024).
Chang S. S., Prézelin B. B., Trench R. K. (1983). Mechanisms of photoadaptation in three strains of the symbiotic dinoflagellate Symbiodinium microadriaticum. Mar. Biol. 76, 219–229. doi: 10.1007/BF00393021
Chow M. H., Tsang R. H. L., Lam E. K. Y., Ang P. (2016). Quantifying the degree of coral bleaching using digital photographic technique. J. Exp. Mar. Biol. Ecol. 479, 60–68. doi: 10.1016/j.jembe.2016.03.003
Claar D. C., Starko S., Tietjen K. L., Epstein H. E., Cunning R., Cobb K. M., et al. (2020). Dynamic symbioses reveal pathways to coral survival through prolonged heatwaves. Nat. Commun. 11, 6097. doi: 10.1038/s41467-020-19169-y
Clarke K. R., Gorley R. N. (2006). PRIMER v6: User Manual/tutorial (Plymouth Routines in Multivariate Ecological Research) (Plymouth, UK: PRIMER-E Ltd).
Coelho V., Fenner D., Caruso C., Bayles B., Huang Y., Birkeland C. (2017). Shading as a mitigation tool for coral bleaching in three common Indo-Pacific species. J. Exp. Mar. Biol. Ecol. 497, 152–163. doi: 10.1016/j.jembe.2017.09.016
Coles S. L., Jokiel P. L. (1978). Synergistic effects of temperature, salinity and light on the hermatypic coral Montipora verrucosa. Mar. Biol. 49, 187–195. doi: 10.1007/BF00391130
Comeau S., Carpenter R. C., Edmunds P. J. (2014). Effects of irradiance on the response of the coral Acropora pulchra and the calcifying alga Hydrolithon reinboldii to temperature elevation and ocean acidification. J. Exp. Mar. Biol. Ecol. 453, 28–35. doi: 10.1016/j.jembe.2013.12.013
Cuéllar Cruz M., Castaño I., Arroyo O., Peñas A. (2009). Oxidative stress response to menadione and cumene hydroperoxide in the opportunistic fungal pathogen Candida glabrata. Memórias do Instituto Oswaldo Cruz 104, 649–654. doi: 10.1590/S0074-02762009000400020
Cunning R., Silverstein R. N., Baker A. C. (2018). Symbiont shuffling linked to differential photochemical dynamics of Symbiodinium in three Caribbean reef corals. Coral Reefs 37, 145–152. doi: 10.1007/s00338-017-1640-3
DiPerna S., Hoogenboom M., Noonan S., Fabricius K. (2018). Effects of variability in daily light integrals on the photophysiology of the corals Pachyseris speciosa and Acropora millepora. Public Library Sci. 13, 1–20. doi: 10.1371/journal.pone.0203882
Dobson K. L., Ferrier-Pagès C., Saup C. M., Grottoli A. G. (2021). The Effects of Temperature, Light, and Feeding on the Physiology of Pocillopora damicornis, Stylophora pistillata, and Turbinaria reniformis Corals. Water 13, 2048. Available at: https://www.mdpi.com/2073-4441/13/15/2048.
Donner S. D., Skirving W. J., Little C. M., Oppenheimer M., Hoegh-Guldberg O. (2005). Global assessment of coral bleaching and required rates of adaptation under climate change. Global Change Biol. 11, 2251–2265. doi: 10.1111/j.1365-2486.2005.01073.x
Downs C. A., Mueller E., Phillips S., Fauth J. E., Woodley C. M. (2000). A molecular biomarker system for assessing the health of coral (Montastraea faveolata) during heat stress. Mar. Biotechnol. 2, 533–544. doi: 10.1007/s101260000038
Eaton A. D. (2005). Standard methods for the examination of water and wastewater (21st ed.). APHA-AWWA-WEF. American Water Works Association & Water Environment Federation. American Public Health Association.
Enríquez S., Méndez E. R., Prieto R. I. (2005). Multiple scattering on coral skeletons enhances light absorption by symbiotic algae. Limnol. Oceanogr. 50, 1025–1032. doi: 10.4319/lo.2005.50.4.1025
Fine M., Steindler L., Loya Y. (2004). Endolithic algae photoacclimate to increased irradiance during coral bleaching. Mar. Freshw. Res. 55, 115–121. doi: 10.1071/MF03120
Franklin D., Hoegh-Guldberg O., Jones R., Berges J. (2004). Cell death and degeneration in the symbiotic dinoflagellates of the coral Stylophora pistillata during bleaching. Mar. Ecol. Prog. Ser. 272, 117–130. doi: 10.3354/meps272117
Gates R. D., Baghdasarian G., Muscatine L. (1992). Temperature stress causes host cell detachment in symbiotic cnidarians: implications for coral bleaching. Biol. Bull. 182, 324–332. doi: 10.2307/1542252
Gonzalez-Espinosa P. C., Donner S. D. (2021). Cloudiness reduces the bleaching response of coral reefs exposed to heat stress. Global Change Biol. 27, 3474–3486. doi: 10.1111/gcb.15676
Grottoli A. G., Toonen R. J., van Woesik R., Vega Thurber R., Warner M. E., McLachlan R. H., et al. (2021). Increasing comparability among coral bleaching experiments. Ecol. Appl. 31, e02262. doi: 10.1002/eap.2262
Harrison D. P. (2018). “Could localized marine cloud brightening buy coral reefs time?,” in Paper presented at the Ocean Sciences Meeting 2018, Portland, Oregon, USA.
Harrison D. P. (2024). “An overview of environmental engineering methods for reducing coral bleaching stress,” in Oceanographic Processes of Coral Reefs: Physical and Biological Links in The Great Barrier Reef. Eds. Wolanski E., Kingsford M. (CRC Press, Florida, USA).
Harrison D. P., Harrison L., Baird M., Utembe S., Schofield R., Escobar Correa R., et al. (2019). T14: environmental modelling of large scale solar radiation management. A report provided to the Australian government from the reef restoration and adaptation program. Available online at: https://gbrrestoration.org/resources/reports/. (Accessed July 11, 2023).
Higuchi T., Fujimura H., Arakaki T., Oomori T. (2008). “Activities of antioxidant enzymes (SOD and CAT) in the coral Galaxea fascicularis against increased hydrogen peroxide concentrations in seawater,” in Paper presented at the Proceedings of the 11th International Coral Reef Symposium, Ft. Lauderdale, Florida.
Hill R., Frankart C., Ralph P. J. (2005). Impact of bleaching conditions on the components of non-photochemical quenching in the zooxanthellae of a coral. J. Exp. Mar. Biol. Ecol. 322, 83–92. doi: 10.1016/j.jembe.2005.02.011
Hoegh-Guldberg O. (1999). Climate change, coral bleaching and the future of the world’s coral reefs. Mar. Freshw. Res. 50, 839–866. doi: 10.1071/MF99078
Hoegh-Guldberg O., Mumby P. J., Hooten A. J., Steneck R. S., Greenfield P., Gomez E., et al. (2007). Coral reefs under rapid climate change and ocean acidification. Science 318, 1737–1742. doi: 10.1126/science.1152509
Hoegh-Guldberg O., Smith G. J. (1989). The effect of sudden changes in temperature, light and salinity on the population density and export of zooxanthellae from the reef corals Stylophora pistillata Esper and Seriatopora hystrix Dana. J. Exp. Mar. Biol. Ecol. 129, 279–303. doi: 10.1016/0022-0981(89)90109-3
Hoogenboom M. O., Frank G. E., Chase T. J., Jurriaans S., Álvarez-Noriega M., Peterson K., et al. (2017). Environmental drivers of variation in bleaching severity of acropora species during an extreme thermal anomaly. Front. Mar. Sci. 4. doi: 10.3389/fmars.2017.00376
Jeffries T. C., Schmitz Fontes M. L., Harrison D. P., Van-Dongen-Vogels V., Eyre B. D., Ralph P. J., et al. (2015). Bacterioplankton dynamics within a large anthropogenically impacted urban estuary. Front. Microbiol. 6. doi: 10.3389/fmicb.2015.01438
Johnson C. E., Goulet T. L. (2007). A comparison of photographic analyses used to quantify zooxanthella density and pigment concentrations in Cnidarians. J. Exp. Mar. Biol. Ecol. 353, 287–295. doi: 10.1016/j.jembe.2007.10.003
Jokiel P. L., Maragos J. E., Franzisket L. (1978). “Coral growth: buoyant weight technique,” in Coral Reefs: Research Methods. Eds. Stoddart D. R., Johannes R. E.(Paris, France: UNESCO), 581.
Jones R. J., Hoegh-Guldberg O. (2001). Diurnal changes in the photochemical efficiency of the symbiotic dinoflagellates (Dinophyceae) of corals: photoprotection, photoinactivation and the relationship to coral bleaching. Plant Cell Environ. 24, 89–99. doi: 10.1046/j.1365-3040.2001.00648.x
Jurriaans S., Hoogenboom M. O. (2020). Seasonal acclimation of thermal performance in two species of reef-building corals. Mar. Ecol. Prog. Ser. 635, 55–70. Available at: https://www.int-res.com/abstracts/meps/v635/p55-70/.
Latham J., Kleypas J., Hauser R., Parkes B., Gadian A. (2013). Can marine cloud brightening reduce coral bleaching? Atmos. Sci. Lett. 14, 214–219. doi: 10.1002/asl2.442
Leahy S. M., Kingsford M. J., Steinberg C. R. (2013). Do clouds save the great barrier reef? satellite imagery elucidates the cloud-SST relationship at the local scale. Public Library Sci. 8, e70400. doi: 10.1371/journal.pone.0070400
Lesser M. P. (1996). Elevated temperatures and ultraviolet radiation cause oxidative stress and inhibit photosynthesis in symbiotic dinoflagellates. Limnol. Oceanogr. 41, 271–283. doi: 10.4319/lo.1996.41.2.0271
Lesser M. P. (2019). Phylogenetic signature of light and thermal stress for the endosymbiotic dinoflagellates of corals (Family Symbiodiniaceae). Limnol. Oceanogr. 64, 1852–1863. doi: 10.1002/lno.11155
Lesser M. P., Farrell J. H. (2004). Exposure to solar radiation increases damage to both host tissues and algal symbionts of corals during thermal stress. Coral Reefs 23, 367–377. doi: 10.1007/s00338-004-0392-z
Lesser M. P., Stochaj W. R., Tapley D. W., Shick J. M. (1990). Bleaching in coral reef anthozoans: effects of irradiance, ultraviolet radiation, and temperature on the activities of protective enzymes against active oxygen. Coral Reefs 8, 225–232. doi: 10.1007/bf00265015
Levin R. A., Beltran V. H., Hill R., Kjelleberg S., McDougald D., Steinberg P. D., et al. (2016). Sex, scavengers, and chaperones: transcriptome secrets of divergent symbiodinium thermal tolerances. Mol. Biol. Evol. 33, 2201–2215. doi: 10.1093/molbev/msw119
Levy O., Yacobi Y., Dubinsky Z., Stambler N. (2006). Diel ‘tuning’ of coral metabolism: Physiological responses to light cues. J. Exp. Biol. 209, 273–283. doi: 10.1242/jeb.01983
Li Y., Schellhorn H. E. (2007). Rapid kinetic microassay for catalase activity. J. Biomol. Techniques 18, 185–187.
Lough J. M., Anderson K. D., Hughes T. P. (2018). Increasing thermal stress for tropical coral reefs: 1871–2017. Sci. Rep. 8, 6079. doi: 10.1038/s41598-018-24530-9
Marcelino L. A., Westneat M. W., Stoyneva V., Henss J., Rogers J. D., Radosevich A., et al. (2013). Modulation of light-enhancement to symbiotic algae by light-scattering in corals and evolutionary trends in bleaching. Public Library Sci. 8, 1–9. doi: 10.1371/journal.pone.0061492
Méndez C., Simpson N. P., Johnson F. X. (2023). Contribution of Working Groups I, II and III to the Sixth Assessment Report of the Intergovernmental Panel on Climate Change. Retrieved from Geneva, Switzerland. Available at: https://www.ipcc.ch/report/ar6/syr/.
McLachlan R. H., Grottoli A. G. (2021). Image analysis to quantify coral bleaching using greyscale model. Protocolsio. 1–14. doi: 10.17504/protocols.io.bx8wprxe
McLachlan R. H., Price J. T., Solomon S. L., Grottoli A. G. (2020). Thirty years of coral heat-stress experiments: a review of methods. Coral Reefs 39, 885–902. doi: 10.1007/s00338-020-01931-9
Muscatine L., Falkowski P. G., Porter J. W., Dubinsky Z. (1984). Fate of Photosynthetic Fixed Carbon in Light- and Shade-Adapted Colonies of the Symbiotic Coral Stylophora pistillata. Proc. R. Soc. London Ser. B Biol. Sci. 222, 181–202. Available at: http://www.jstor.org/stable/36012.
Page C. A., Giuliano C., Bay L. K., Randall C. J. (2023). High survival following bleaching underscores the resilience of a frequently disturbed region of the Great Barrier Reef. Ecosphere 14, 1–22. doi: 10.1002/ecs2.4280
Platt T., Gallegos C. L., Harrison W. G. (1980). Photoinhibition of photosynthesis in natural assemblages of marine phytoplankton. J. Mar. Res. 38, 687–701. Available at: https://elischolar.library.yale.edu/journal_of_marine_research/1525.
Pörtner H.-O., Roberts D. C., Tignor M., Poloczanska E. S., Mintenbeck K., Alegría A., et al. (2022). Climate change 2022: impacts, adaptation and vulnerability. Working group II contribution to the IPCC sixth assessment report. Available online at: https://www.ipcc.ch/report/sixth-assessment-report-working-group-ii/. (Accessed July 11, 2023).
Ralph P. J., Gademann R. (2005). Rapid light curves: A powerful tool to assess photosynthetic activity. Aquat. Bot. 82, 222–237. doi: 10.1016/j.aquabot.2005.02.006
Ralph P. J., Hill R., Doblin M. A., Davy S. K. (2015). “Theory and application of pulse amplitude modulated chlorophyll fluorometry in coral health assessment,” in Woodley C. M., Downs C. A., Bruckner A., Porter J. W., Galloway S. B. eds. Diseases of Coral. New Jersey: John Wiley & Sons, Inc., 506–523.
Rangel M. S., Erler D., Tagliafico A., Cowden K., Scheffers S., Christidis L. (2019). Quantifying the transfer of prey δ15N signatures into coral holobiont nitrogen pools. Mar. Ecol. Prog. Ser. 610, 33–49. doi: 10.3354/meps12847
Ritchie R. J. (2006). Consistent sets of spectrophotometric chlorophyll equations for acetone, methanol and ethanol solvents. Photosynth. Res. 89, 27–41. doi: 10.1007/s11120-006-9065-9
Roberty S., Fransolet D., Cardol P., Plumier J. C., Franck F. (2015). Imbalance between oxygen photoreduction and antioxidant capacities in Symbiodinium cells exposed to combined heat and high light stress. Coral Reefs 34, 1063–1073. doi: 10.1007/s00338-015-1328-5
Roth M. S. (2014). The engine of the reef: photobiology of the coral–algal symbiosis. Front. Microbiol. 5. doi: 10.3389/fmicb.2014.00422
Roth M. S., Latz M. I., Goericke R., Deheyn D. D. (2010). Green fluorescent protein regulation in the coral Acropora yongei during photoacclimation. J. Exp. Biol. 213, 3644–3655. doi: 10.1242/jeb.040881
Ruban A. V. (2016). Nonphotochemical chlorophyll fluorescence quenching: mechanism and effectiveness in protecting plants from photodamage. Plant Physiol. 170, 1903–1916. doi: 10.1104/pp.15.01935
Sakshaug E., Bricaud A., Dandonneau Y., Falkowski P., Kiefer D., Legendre L., et al. (1997). Parameters of photosynthesis: Definitions, theory and interpretation of results. J. Plankton Res. 20, 1637–1670. doi: 10.1093/plankt/20.3.603
Sandeman I. (2008). Light driven lipid peroxidation of coral membranes and a suggested role in calcification. Rev. Biol. Trop. 56, 1–9. doi: 10.15517/rbt.v56i0.5574
Scheufen T., Iglesias-Prieto R., Enríquez S. (2017). Changes in the number of symbionts and symbiodinium cell pigmentation modulate differentially coral light absorption and photosynthetic performance. Front. Mar. Sci. 4. doi: 10.3389/fmars.2017.00309
Silsbe G., Malkin S. (2015). Phytotools: Phytoplankton Production Tools, an R package. Available online at: https://cran.r-project.org/web/packages/phytotools/index.html. (accessed July 11, 2023).
Singh T., Iijima M., Yasumoto K., Sakai K. (2019). Effects of moderate thermal anomalies on Acropora corals around Sesoko Island, Okinawa. PloS One 14, e0210795. doi: 10.1371/journal.pone.0210795
Skirving W., Enríquez S., Hedley J., Dove S., Eakin C., Mason R., et al. (2017). Remote sensing of coral bleaching using temperature and light: progress towards an operational algorithm. Remote Sens. 10, 1–19. doi: 10.3390/rs10010018
Spencer Davies P. (1989). Short-term growth measurements of corals using an accurate buoyant weighing technique. Mar. Biol. 101, 389–395. doi: 10.1007/BF00428135
Stewart H., Kline D., Chapman L., Altieri A. (2021). Caribbean mangrove forests act as coral refugia by reducing light stress and increasing coral richness. Ecosphere 12, 1–17. doi: 10.1002/ecs2.3413
Stimson J., Kinzie R. A. (1991). The temporal pattern and rate of release of zooxanthellae from the reef coral Pocillopora damicornis (Linnaeus) under nitrogen-enrichment and control conditions. J. Exp. Mar. Biol. Ecol. 153, 63–74. doi: 10.1016/S0022-0981(05)80006-1
Suggett D., Dong L., Lawson T., Lawrenz E., Torres L., Smith D. (2012). Light availability determines susceptibility of reef building corals to ocean acidification. Coral Reefs 32, 1–13. doi: 10.1007/s00338-012-0996-7
Suggett D. J., Warner M. E., Smith D. J., Davey P., Hennige S., Baker N. R. (2008). Photosynthesis and production of hydrogen peroxide by symbiodinium (Pyrrhophyta) phylotypes with different thermal tolerances. J. Phycol. 44, 948–956. doi: 10.1111/j.1529-8817.2008.00537.x
Tagliafico A., Baker P., Kelaher B., Ellis S., Harrison D. (2022). The effects of shade and light on corals in the context of coral bleaching and shading technologies. Front. Mar. Sci. 9. doi: 10.3389/fmars.2022.919382
Tchernov D., Gorbunov M. Y., de Vargas C., Narayan Yadav S., Milligan A. J., Häggblom M., et al. (2004). Membrane lipids of symbiotic algae are diagnostic of sensitivity to thermal bleaching in corals. Proc. Natl. Acad. Sci. U. States America 101, 13531–13535. doi: 10.1073/pnas.0402907101
Tilstra A., Wijgerde T., Dini-Andreote F., Eriksson B. K., Salles J. F., Pen I., et al. (2017). Light induced intraspecific variability in response to thermal stress in the hard coral Stylophora pistillata. PeerJ 5, e3802. doi: 10.7717/peerj.3802
Tunala L. P., Tâmega F. T. S., Duarte H. M., Coutinho R. (2019). Stress factors in the photobiology of the reef coral Siderastrea stellata. J. Exp. Mar. Biol. Ecol. 519, 151188. doi: 10.1016/j.jembe.2019.151188
van Woesik R., Sakai K., Ganase A., Loya Y. (2011). Revisiting the winners and the losers a decade after coral bleaching. Mar. Ecol. Prog. Ser. 434, 67–76. Available at: https://www.int-res.com/abstracts/meps/v434/p67-76/.
Wagner D. E., Kramer P., van Woesik R. (2010). Species composition, habitat, and water quality influence coral bleaching in southern Florida. Mar. Ecol. Prog. Ser. 408, 65–78. Available at: https://www.int-res.com/abstracts/meps/v408/p65-78.
Wangpraseurt D., Jacques S., Lyndby N., Holm J. B., Pages C. F., Kühl M. (2019). Microscale light management and inherent optical properties of intact corals studied with optical coherence tomography. J. R. Soc. Interface 16, 1–10. doi: 10.1098/rsif.2018.0567
Warren C. R. (2008). Rapid measurement of chlorophylls with a microplate reader. J. Plant Nutr. 31, 1321–1332. doi: 10.1080/01904160802135092
Weydert C. J., Cullen J. J. (2010). Measurement of superoxide dismutase, catalase and glutathione peroxidase in cultured cells and tissue. Nat. Protoc. 5, 51–66. doi: 10.1038/nprot.2009.197
Winters G., Holzman R., Blekhman A., Beer S., Loya Y. (2009). Photographic assessment of coral chlorophyll contents: Implications for ecophysiological studies and coral monitoring. J. Exp. Mar. Biol. Ecol. 380, 25–35. doi: 10.1016/j.jembe.2009.09.004
Yordanov G., Midtgård O.-M., Saetre T., Nielsen H., Norum L. (2013). Overirradiance (Cloud enhancement) events at high latitudes. Photovoltaics IEEE J. 3, 271–277. doi: 10.1109/JPHOTOV.2012.2213581
Keywords: Scleractinia, irradiance, solar-radiation management, environmental stressor, climate change, oxidative stress, photochemistry, physiological
Citation: Ellis SL, Butcherine P, Tagliafico A, Hendrickson C, Kelaher BP, Schulz KG and Harrison DP (2024) Shading responses are species-specific in thermally stressed corals. Front. Mar. Sci. 11:1333806. doi: 10.3389/fmars.2024.1333806
Received: 06 November 2023; Accepted: 31 July 2024;
Published: 13 September 2024.
Edited by:
Hajime Kayanne, The University of Tokyo, JapanReviewed by:
Christine Ferrier-Pagès, Centre Scientifique de Monaco, MonacoJoshua Patterson, University of Florida, United States
Copyright © 2024 Ellis, Butcherine, Tagliafico, Hendrickson, Kelaher, Schulz and Harrison. This is an open-access article distributed under the terms of the Creative Commons Attribution License (CC BY). The use, distribution or reproduction in other forums is permitted, provided the original author(s) and the copyright owner(s) are credited and that the original publication in this journal is cited, in accordance with accepted academic practice. No use, distribution or reproduction is permitted which does not comply with these terms.
*Correspondence: Sophia L. Ellis, c29waGlhLmVsbGlzQHNjdS5lZHUuYXU=
†ORCID: Sophia L. Ellis, orcid.org/0000-0002-0133-4307
Peter Butcherine, orcid.org/0000-0002-6104-2437
Alejandro Tagliafico, orcid.org/0000-0002-7305-7943
Conor Hendrickson, orcid.org/0000-0001-7747-304X
Brendan P. Kelaher, orcid.org/0000-0002-7505-4412
Kai G. Schulz, orcid.org/0000-0002-8481-4639
Daniel. P Harrison, orcid.org/0000-0001-8353-6107