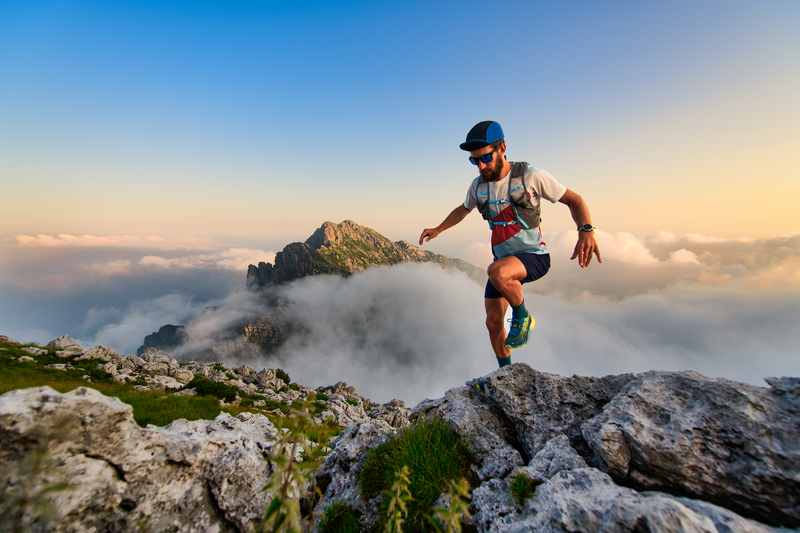
95% of researchers rate our articles as excellent or good
Learn more about the work of our research integrity team to safeguard the quality of each article we publish.
Find out more
ORIGINAL RESEARCH article
Front. Mar. Sci. , 07 February 2024
Sec. Marine Biogeochemistry
Volume 11 - 2024 | https://doi.org/10.3389/fmars.2024.1331940
The long-term burial of organic carbon in marginal seas plays a critical role in Earth’s carbon cycle and climate change. However, the mechanism of organic carbon (OC) burial in the Okinawa Trough (OT) during glacial-interglacial timescales remains unclear. In this study, we analyzed the foraminiferal carbon isotopes, total organic carbon (TOC), and δ13C-TOC over the past 200 ka in core Z1 collected in the central OT. We aimed to reveal the history of OC burial in the middle Okinawa Trough during the past 200 ka, and we combined our findings with relevant paleoenvironmental indices to reveal underlying mechanisms. We found reduced surface primary productivity during MIS 6, which may indicate changes in the pathways of the Kuroshio Current (KC). Furthermore, we observed decoupling between high TOC flux and low OC burial during glacial periods. We proposed that the dilution effect caused by the high sedimentation rate and poor OC preservation during the glacial period resulted in the low TOC content. Ventilation of the North Pacific Intermediate Water (NPIW) regulated the redox conditions of the intermediate water in the Okinawa Trough. Additionally, the intensified Kuroshio Current during interglacial phases led to water column stratification, creating reducing conditions in the bottom water and facilitating improved OC preservation. Subsequently, the enhanced water column oxygenation resulting from the oxygen carried by the intensified glacial NPIW weakened the burial of OC. This study sheds new light on our understanding of the carbon cycle in marginal seas on a glacial-interglacial timescale.
● Different burial regimes of organic carbon were identified on glacial-interglacial time scales in the middle Okinawa Trough
● Sea level fluctuation and terrigenous input influenced productivity during the glacial-interglacial cycle in the middle Okinawa Trough
● Organic carbon burial in the middle Okinawa Trough is primarily regulated by mineralization processes within the water column
Long-term buried organic carbon in marine sediments has a significant impact on the Earth’s carbon cycle at the orbital and millennial scales by regulating atmospheric CO2 levels and thus affecting the global climate (Sigman & Boyle, 2000; Clark et al., 2006; Burdige, 2007; Cartapanis et al., 2016; Keil, 2017). Cartapanis et al. (2016) collected organic carbon (OC) data from hundreds of sediment cores around the world and found that during the last interglacial-glacial cycle (~150 ka), the glacial accumulation rates of OC were consistently higher than the interglacial accumulation rates (Cartapanis et al., 2016). The enhanced export productivity, improved transfer of organic matter and better preservation of organic matter contributed to an increase in organic carbon burial during glacial periods (Cartapanis et al., 2016). Elevated burial of organic carbon due to high productivity and better preservation conditions was also observed in glacial sediments collected from the low-latitude Atlantic, the eastern equatorial Pacific and the Southern Ocean (Bradtmiller et al., 2010; Martínez-García et al., 2014; Cartapanis et al., 2016).
However, high export productivity does not necessarily lead to high OC burial, decoupling between these factors might exist in some regions, and long-term OC burial may be related to other environmental factors. As reported by Li et al. (2018), the main factors controlling the increase in OC burial in the glacial period over the past 91 ka were the Kuroshio Current (KC) intrusion and sea level change in the central OT, which counteracted the low export productivity. To better understand the mechanism of OC burial in global marine sediments and its relation with the carbon cycle, more OC preservation records from different regions and over longer time scales need to be collected.
Approximately 50% of the marginal seas are located in the Western Pacific (Li et al., 2018). As the main depositional areas of terrigenous matter, marginal seas have enormous potential for marine carbon sequestration and as carbon sinks (Bauer et al., 2013; Shao et al., 2016; Dai et al., 2022). The Okinawa Trough is adjacent to the continental shelf of the East China Sea (ECS), and its unique location and topography make it a deposition center in the ECS. Many large rivers flow into the ECS, which causes the trough to receive tremendous detrital matter from East Asia. Compared with the continental shelf, the deeper water and slower sedimentation rate of the OT are conducive to preserving long-scale paleoenvironmental information, providing high-resolution sedimentary records for the reconstruction of tectonic evolution, hydrological environmental changes, and global climate change during the Quaternary (Shinjo et al., 1999; Jian et al., 2000; Kao et al., 2005; Kao et al., 2006; Chang et al., 2009; Ujiié et al., 2016; Li et al., 2017; Zhao et al., 2021; Zhang et al., 2022).
The North Pacific has consistently been a hotspot for millennium- and orbital-scale carbon cycle research (Zhao et al., 2021). Numerous studies have reported the factors influencing organic carbon burial in the OT, such as productivity (Chang et al., 2009; Shao et al., 2016; Li et al., 2017), hydrological conditions (Kao et al., 2005; Ujiié et al., 2016; Li et al., 2018) and redox change (Dou et al., 2015; Zou et al., 2020; Zhao et al., 2021). However, the time scales of these studies are mostly concentrated in the period from the last glacial period to the Holocene, and the burial mechanism of OC in the OT over longer time scales remains an unknown. In this study, we analyzed the carbon isotopes of planktonic foraminifera, TOC and δ13C-TOC generated from site Z1 located in the middle OT (Figure 1) and combined these data with previously published δ13C values of benthic foraminifera and CaCO3 content to explore the impact of mineralization processes and ventilation on OC burial over the past 200 ka.
Figure 1 Major circulation system of the Okinawa Trough. (A) The location of site Z1 (red diamond) and referenced cores (green circle), including IODP U1429 (Zhao et al., 2021), MD01-2404 (Li et al., 2018) and ODP 1202B (Dou et al., 2015). KC: Kuroshio Current; NPIW: North Pacific Intermediate Water; TWC: Tsushima Warm Current; KCE: Kuroshio Current Extension. The solid blue line with an arrow indicates the path of the KC. The solid yellow line with an arrow indicates the spreading path of NPIW from the northeast Pacific. The dashed line with an arrow represents the two passages through which the NPIW enters the Okinawa Trough. (B) The selected section for mean salinity. The red diamond represents site Z1. (C) The salinity in the upper 2000 m along the selected section in (B). Salinity data were collected from ODV: World Ocean Atlas 2018 (awi.de). Figures were generated by Ocean Data View software (Schlitzer, 2021) and with reference to Figure 1 in Zhao et al. (2021).
The sediment core Z1 (28°49´N, 127°20´E) used in this study was collected from the middle continental slope of the Okinawa Trough at a water depth of 940 m in 2015 (Figure 1). The lithology of this 60-meter-long core is relatively uniform, and it is basically composed of clay silt (Dou et al., 2021). The age model of Z1 over the past 200 ka (Figure 2A) was established by Dou et al. (2021) based on planktonic foraminiferal AMS14C dating and by comparing the δ18O record obtained from benthic foraminifera with the global benthic stack LR04. The core was subsampled at intervals of 20 cm, and 280 sediment samples were obtained. The CaCO3 content, carbon, and oxygen isotopes of benthic foraminifera were determined in Dou et al. (2021).
For TOC% analysis, excess 1 N HCl was added to sediment samples to remove inorganic carbon. After mixing, the samples were placed in a 60°C water bath with shaking and left undisturbed for 3 h after the reaction. The acid in the sample was then decanted after centrifugation, and the sample was washed three times with deionized water. The samples were then dried in a 40°C oven, weighed and ground into powder. TOC%measure was analyzed using a Vario EL III elemental analyzer (Elementar, Germany), and the measurement errors were all within the standard deviation of<0.05%. TOC% was further calculated by the TOC%measure and CaCO3% published in Dou et al. (2021), as shown in Equation 1.
In the δ13C-TOC test, the above acidified samples were used, and analysis was performed with a Thermo Deltaplus XL stable isotope mass spectrometer with a test accuracy of ±0.15‰ (VPDB).
For foraminiferal carbon isotope analysis, approximately 10 shells of Globigerinoides ruber (G. ruber) were selected from each sample and tested using a MAT 253 stable isotope mass spectrometer (Finnigan, Germany). The test accuracy was determined based on the National Calcium Carbonate Standard (GBW04405) and International Standard (NBS19), and sample pretreatment and testing were completed at the Third Institute of Oceanography, Ministry of Natural Resources.
The TOC% of core Z1 ranges between 0.93-3.76% (Figure 2C) since Marine Isotope Stage 6 (MIS 6), with an average value of 2.01%. The average TOC% during glacial (MIS 2-4 and MIS 6) and interglacial periods (MIS 1 and MIS 5) is 2.53% and 1.85%, respectively. The variation in TOC% is similar to that of CaCO3% (Dou et al., 2021); both present a higher value during interglacial periods and a lower value during glacial periods (Figure 3D).
Figure 2 (A) Age model of core Z1. Downcore profiles for (B) the content of CaCO3, (C) total organic carbon content, and (D) δ13C value of TOC. (A, B) were analyzed in Dou et al. (2021).
Figure 3 Main geochemical parameters of core Z1. (A) The δ13C of planktonic foraminifera (G) ruber. (B) The δ13C of benthic foraminifera (C) wullerstorfi (Dou et al., 2021). (C) The carbon isotope difference between benthic and planktonic foraminifera Δδ13CP-B. (D) Total organic carbon content. (E) δ13C value of TOC. (F) Sea level fluctuation (Lambeck & Chappell, 2001).
The δ13C-TOC of core Z1 ranges from -29.6 ‰ to -18.0 ‰ (Figure 2D), with an average ratio of -21.8 ‰. The lowest δ13C-TOC value occurred at the MIS 5/4 boundary, which is in agreement with regional methane emissions (Dou et al., 2021), and the δ13C value at other times was relatively stable.
The δ13C of planktonic foraminifera G. ruber (δ13CG. ruber) in Z1 ranges from -6.03 ‰ to 2.80 ‰, with an average value of 0.24 ‰ (Figure 3A). The mean δ13CG. ruberratio during glacial and interglacial periods are 0.64 ‰ and -0.14 ‰, respectively. During the glacial periods, δ13CG. ruber was depleted and greatly fluctuated (Figure 3D).
The carbon isotope data of benthic foraminifera C. wullerstorfi were published in Dou et al. (2021). The δ13CC. The Wullerstorfi value ranges from -2.99 ‰ to 0.88 ‰, with an average value of -0.07‰ (Figure 3B). The general trend was similar to δ13CG. ruber, and the δ13C signal was negative during the glacial period (Dou et al., 2021). The δ13C difference between planktonic foraminifera (G. ruber) and benthic foraminifera (C. wullerstorfi) reflects changes in surface productivity (Jian et al., 2001; Tian et al., 2017). The isotope difference between surface and bottom water was calculated as Equation 2:
The Δδ13CP-B ranges from -6.16 ‰ to 2.07 ‰, with an average value of 0.31 ‰ (Figure 3C). The lowest Δδ13CP-B occurs during MIS 6. The average Δδ13CP-B is -0.18% during MIS 6, compared with the average ratio of 0.77% during MIS 1-5. The dramatic depletion in δ13CG. ruber during MIS 6 are also recorded in adjacent IODP site U1429 (Vats et al., 2021). Furthermore, the Δδ13CP-B ratio remains stable during MIS 1-5, and no glacial-interglacial cycle is identified.
TOC% and foraminiferal carbon isotopes are important proxies for paleo-productivity. An enhancement in sea surface primary productivity results in the consumption of more 12C through photosynthesis, which leads to an enrichment in 13C in surface seawater. At the same time, as organic matter sinks, it degrades and releases 12C, which leads to a depletion of 12C in surface bottom water (Spezzaferri, 1995; Li et al., 2017). As a result, higher sea surface primary productivity corresponds to positive planktonic δ13C and a higher Δδ13CP-B ratio (Jian et al., 2001; Tian et al., 2017). In core Z1, the trends for δ13CG. ruber and Δδ13CP-B ratio are very similar (Figures 3A, B). Positive values of δ13CG. ruber and Δδ13CP-B occurred during MIS 1-5, whereas negative δ13CG. ruber and Δδ13CP-B ratios occurred during MIS 6, indicating much lower surface productivity conditions during MIS 6 (Figure 2C). TOC% was lowest during MIS 6, indicating the lowest level of surface primary productivity, which is consistent with the Δδ13CP-B data (Figure 3C). However, the TOC% also dropped during MIS 2-4, which is not recorded in the Δδ13CP-B ratio.
The variation in surface primary productivity in the mid-OT observed in this study during the glacial-interglacial cycle corresponds well with some previous studies. Li et al. (2017) reconstructed export productivity over the past 91 ka using reactive P from sediment core MD012404 in the mid-OT and found that the productivity was modulated by the penetration depth of NPIW, which hinders the supply of deep high-nutrient water to surface seawater. Shao et al. (2016) proposed that a dry and cold climate resulted in low nutrient levels, while the rising sea level and warm climate during the deglacial period contributed to elevated primary productivity. These reports suggested that low productivity in the OT during glacial periods may be due to water mass intrusion, climate, KC and other factors.
Atmospheric dust input was also high, with dry and cold climates during glacial periods, and Fe and Si carried by aeolian dust further increased the nutrient levels (Li et al., 2017). Furthermore, although the OT received terrestrial sediment input during glacial periods, in the deep water, sufficient time was available for organic matter to be degraded and utilized by marine organisms and eventually be buried in the sediments. δ13C-TOC is often used to distinguish sources of organic matter. The δ13C value of terrestrial organic matter, including C3 plants (δ13C ranging from -23 to -30‰) and freshwater aquatic plants and plankton (δ13C ranging from -25 to -30‰), is more negative. The δ13C of marine organic matter is more positive (usually taken as -19 ~ -22‰), which is composed of marine organisms (δ13C ranging from -10‰ to -22‰) and algae (δ13C ranging from -20‰ to -25‰) (Fontugne & Jouanneau, 1987; Meyers, 1997; Shao et al., 2016). The average δ13C-TOC of core Z1 is -21.8 ‰, and the overall value is stable, indicating that since MIS6, the sedimentary organic matter has basically been of marine authigenic origins.
Based on diverse geochemical parameters (Figure 3), the surface primary productivity may have been significantly affected by sea level variation and terrigenous inputs in the glacial-interglacial cycle. In accordance with previous studies, the content of CaCO3 in the mid-OT was lower during glacial periods and higher during the interglacial periods (Figure 2B) (Chang et al., 2009; Dou et al., 2015; Dou et al., 2021). Additionally, the CaCO3 content was highly synchronized with sea level changes, indicating that the production and burial of CaCO3 in the mid-OT was closely related to sea level fluctuations and greatly influenced by terrigenous input (Jian et al., 2000; Chang et al., 2009). The OT is adjacent to the continental shelf, and the sea level could have dropped by approximately 120 m during the glacial period (Saito et al., 1998). The lower sea level allowed a large amount of terrestrial matter supplied by the paleo-Yangtze River to be directly transported into the OT (Dou et al., 2010; Shao et al., 2016). The sedimentation rate in the OT during the glacial period was much higher than that in the interglacial period. As a result, TOC% and CaCO3% in the glacial period decreased due to the dilution of the terrestrial sediment input during MIS 2-4 and MIS 6. Different from TOC% and CaCO3%, the fluxes of TOC and CaCO3 present higher values during the glacial period and lower values during the interglacial period (Figure 4) due to the higher sedimentation rate during the glacial period. Thus, there is no significant difference in surface primary productivity in MIS 1-5.
Figure 4 Comparison of TOC and CaCO3 accumulation rates in OT. (A) The sedimentation rate of core Z1. (B) The TOC flux. (C) TOC%. (D) CaCO3 flux. (E) CaCO3%. (F) The intensity of the Kuroshio intrusion (Matsuzaki et al., 2019). (G) Sea level fluctuation (Lambeck & Chappell, 2001).
The obviously depleted Δδ13CP-B ratio during MIS 6 indicates low surface primary productivity at that time, and we speculate the difference in subsurface KC upwelling is the major reason for the prominent low surface productivity in OT during MIS 6. During MIS 2 and MIS 6, the sea level is similar (Figure 4G). But the subsurface KC upwelling is strong during MIS 2, and weak during MIS 6 (Vats et al., 2021). During MIS 6, Oligotrophic KC and weak subsurface upwelling led to poor-nutrient surface water. Consequently, the primary productivity is much lower during MIS 6, compared to MIS 2. In addition, enhanced marine hydrodynamics and the intrusion of the oligotrophic KC (Figure 5D) formed a barrier to sediment entry at the edge of the continental shelf (Zhao et al., 2021), further restricting the transport of shelf sediments to the OT, and the reduction in nutrient supply led to the decline in productivity (Lim et al., 2017; Zhao, 2017).
Figure 5 Comparison of TOC burial with major currents in the OT. (A) The OC burial conditions of Z1. (B) The intensity of NPIW ventilation (Worne et al., 2019). (C) Redox conditions of bottom water in the OT (Zhao et al., 2021). (D) The intensity of the Kuroshio intrusion (Matsuzaki et al., 2019). (E) Sea level fluctuation (Lambeck & Chappell, 2001).
Based on the carbon isotopes of planktonic and benthic foraminifera, we found that the high TOC flux during the glacial periods corresponded to a low TOC content (Figures 4B, C). The TOC% in sediments was greatly affected by the dilution effect and the preservation of OC. Previous studies have reported that that preservation of OC is increased during glacial periods, which slightly contradicts our results (Figure 5A) (Martínez-García et al., 2014; Cartapanis et al., 2016; Li et al., 2018).
The variation in OC burial in the mid-OT was closely related to the redox conditions and the ventilation of the bottom water (Li et al., 2018). Based on modern oceanographic observations (Figures 1B, C), strong stratification could be found in the OT, and the KC had little effect on the deep water, which was mainly composed of low-salinity NPIW. Lateral deep water ventilation is closely related to the redox state of deep water (Cartapanis et al., 2011), and the redox environment has a large impact on OC burial. Therefore, deep water ventilation and the mineralization process under the oxidative environment over the past 200 ka may be the main factors controlling OC sequestration in the OT (Zou et al., 2020).
The intermediate water of the OT is mainly composed of the NPIW and the South China Sea Intermediate Water (SCSIW), which enters the OT through the channel east of Taiwan and the Kerama Gap (Figure 1) (Talley, 1993; Nakamura et al., 2013). NPIW forms at high latitudes in the North Pacific, is widely distributed at water depths of 300-800 m and is characterized by low salinity (~34.0-34.3 ‰) and oxidation (50-150 μmol/L) (Talley, 1993; Knudson & Ravelo, 2015; Zhao et al., 2021). Core Z1 was obtained from the mid OT, where it has been demonstrated to be significantly affected by the NPIW (Dou et al., 2015; Zou et al., 2020; Zhao et al., 2021). Therefore, the intrusion of glacial-enhanced NPIW in the OT may directly regulate the redox state of the bottom water (Okazaki et al., 2010; Knudson & Ravelo, 2015; Ujiié et al., 2016; Gong et al., 2019; Worne et al., 2019; Zhao et al., 2021).
With enhanced glacial NPIW ventilation, the oxygen in the water column was replenished, and the intermediate water remained in an oxidized state. The oxic environment was conducive to microbial mineralization. Particulate organic carbon (POC) settling from the upper layers could be fully degraded and utilized through mineralization, resulting in the lower TOC content during the last glacial period and MIS 6. A recent reconstruction of NPIW evolution and redox conditions using authigenic uranium (aU) over the past 400 ka (Figures 5B,C) indicated that the oxic deep water was correlated with intensified NPIW flow (Zhao et al., 2021). Other reconstruction work based on geochemical indices, such as the Mo/Mn ratio and numerical simulation, also illustrated the close relation between glacial NPIW ventilation and oxygen concentration in deep water (Okazaki et al., 2010; Gong et al., 2019; Zou et al., 2020).
With the warming climate and rising sea level, the TOC content showed a large increase in MIS5. Sea ice melt and increased precipitation weakened the formation of the NPIW (Worne et al., 2019), reducing the ventilation of deep water (Zhao et al., 2021). In addition, the stratification of the water column caused by the strengthened KC led to a decline in the oxygen concentration in the deep layer, promoting the burial of TOC under weakened mineralization conditions (Figure 5) (Dou et al., 2015; Zhao et al., 2021). The solubility of oxygen in seawater during the interglacial period likely decreased due to the rise in temperature. Temperature-influenced solubility changes could lead to the generation of hypoxia on geological timescales (Praetorius et al., 2015), so mineralization and OC burial may also be affected by seawater temperature to some extent. Zou et al. (2020) found that the increased Mo/Mn ratio and Uexcess during the warm Bölling-Alleröd period both indicated the expansion of hypoxic intermediate water in the subtropical northwest Pacific.
The TOC content showed a stable trend during the last glacial period (MIS2-4), with a slight increase in MIS3 (Figure 5), which was also speculated to be mainly due to NPIW ventilation. The formation of the NPIW was reduced due to the warm climate in the Holocene, while the KC increasingly influenced the OT (Figure 5) (Shi et al., 2014; Zou et al., 2020). Otherwise, terrestrial organic matter, which is indicated by lower δ13C-TOC values (Figure 3E), would become refractory after long-term transport and degradation, weakening mineralization. In addition, the enhanced stratification in the water column and the reduction in exchange between surface and deep water led to hypoxia in the bottom water, which promoted OC burial (Dou et al., 2015).
The TOC% in the OT is affected by the sedimentation rate and the preservation of OC. During the glacial periods, the significantly higher sedimentation rate resulted in a low TOC% but corresponded to higher TOC flux because of the lower sea level and the greater amounts of sediment originating from the Yangtze River (Figure 6). In terms of OC preservation, we suggested that the limited contribution of the Kuroshio Current to vertical water exchange may only have a large impact on the upper 300-500 m of water under the strengthened Kuroshio Current. The deeper trough is mainly affected by NPIW, SCSIW or North Pacific Deep Water (NPDW) (Dou et al., 2015; Zhao et al., 2021). In a recent reconstruction of OC burial in the Northwest Pacific (water depth: 2670 m), it was shown that vertical water exchange was hindered due to the barrier formed by the enhanced glacial NPIW, so a hypoxic reducing environment was formed in the bottom water, which promoted the burial of OC (Zhang et al., 2022). We assumed that the water depth and topography of the site were crucial considering that core Z1 was collected from the west slope of the OT and the water depth was only 940 m. This location has been greatly affected by terrigenous materials and NPIW, so ventilation and related mineralization play important roles in regulating organic OC burial.
Figure 6 Schematic diagram of OC burial associated with NPIW ventilation and mineralization in mid-OT during glacial (A) and interglacial (B) periods (modified from (Zhang et al., 2022)).
The burial of OC was not correspondingly elevated due to enhanced mineralization because massive amounts of OC were decomposed into dissolved organic carbon (DOC) through microbial degradation and entered the large DOC pool in the ocean (Jiao et al., 2010). Previous studies have also shown that the enhancement in Pacific meridional overturning (PMOC) during the LGM promoted the ventilation of intermediate water in the North Pacific and reduced the nutrient content in the NPIW (Rae et al., 2020; Zhao et al., 2021).
Based on geochemical evidence, we reconstructed the evolution of OC burial and revealed the burial regime in the mid-OT over the past 200 ka. The lowest sea surface productivity mainly occurred in MIS 6, which may be attributed to changes in the paths of the oligotrophic KC. The sea surface productivity was higher and remained stable in MIS 1-5. The flux of TOC was higher in the glacial periods because of the lower sea level and consequently enhanced terrestrial input. The reduced TOC% observed during glacial periods may be due to the dilution effect and poor OC preservation. Glacial-enhanced NPIW ventilation led to bottom water oxidation and promoted mineralization, which regulated the preservation of OC. The water depth and topography of the study site also mattered for the reconstruction of the evolution of OC burial. The stratification caused by the strengthened KC during interglacial periods could have resulted in reducing conditions in bottom water, facilitating better preservation of OC. This study demonstrates that the environmental conditions of diverse time scales and regions affect the burial of organic carbon.
The original contributions presented in the study are included in the article/supplementary material. Further inquiries can be directed to the corresponding authors.
YJ: Data curation, Visualization, Writing – review & editing, Writing – original draft. BZ: Writing – original draft, Data curation, Visualization, Writing – review & editing. TZ: Writing – review & editing, Writing – original draft. JZ: Writing – review & editing. XZ: Writing – review & editing. YD: Writing – review & editing. QL: Writing – review & editing. FC: Writing – review & editing. BH: Writing – review & editing. LD: Writing – review & editing, Writing – original draft.
The author(s) declare financial support was received for the research, authorship, and/or publication of this article. This work was supported by the Laoshan Laboratory (LSKJ202204201), National Key Research and Development Program (2020YFA06083002), the National Science Foundation of China (Nos. 42072332, Nos. 42006186, Nos. 41976192), Shandong Province Natural Science Foundation (No. ZR2021MD074), the Project of China Geological Survey (DD20230647) and the Marine S&T Fund of Shandong Province for Pilot National Laboratory for Marine Science and Technology (QNLM) (2022QNLM050203; 2021QNLM020002).
The authors declare that the research was conducted in the absence of any commercial or financial relationships that could be construed as a potential conflict of interest.
All claims expressed in this article are solely those of the authors and do not necessarily represent those of their affiliated organizations, or those of the publisher, the editors and the reviewers. Any product that may be evaluated in this article, or claim that may be made by its manufacturer, is not guaranteed or endorsed by the publisher.
Bauer J. E., Cai W.-J., Raymond P. A., Bianchi T. S., Hopkinson C. S., Regnier P. A. G. (2013). The changing carbon cycle of the coastal ocean. Nature 504 (7478), 61–70. doi: 10.1038/nature12857
Bradtmiller L. I., Anderson R. F., Sachs J. P., Fleisher M. Q. (2010). A deeper respired carbon pool in the glacial equatorial Pacific Ocean. Earth Planetary Sci. Lett. 299 (3), 417–425. doi: 10.1016/j.epsl.2010.09.022
Burdige D. J. (2007). Preservation of organic matter in marine sediments: controls, mechanisms, and an imbalance in sediment organic carbon budgets? Chem. Rev. 107 (2), 467–485. doi: 10.1002/chin.200720266
Cartapanis O., Bianchi D., Jaccard S. L., Galbraith E. D. (2016). Global pulses of organic carbon burial in deep-sea sediments during glacial maxima. Nat. Commun. 7 (1), 10796. doi: 10.1038/ncomms10796
Cartapanis O., Tachikawa K., Bard E. (2011). Northeastern Pacific oxygen minimum zone variability over the past 70 kyr: Impact of biological production and oceanic ventilation. Paleoceanography 26 (4). doi: 10.1029/2011PA002126
Chang Y. -P., Chen M. -T., Yokoyama Y., Matsuzaki H., Thompson W. G., Kao S. -J., et al. (2009). Monsoon hydrography and productivity changes in the East China Sea during the past 100,000 years: Okinawa Trough evidence (MD012404). Paleoceanography 24 (3). doi: 10.1029/2007PA001577
Clark P. U., Archer D., Pollard D., Blum J. D., Rial J. A., Brovkin V., et al. (2006). The middle Pleistocene transition: characteristics, mechanisms, and implications for long-term changes in atmospheric pCO2. Quaternary Sci. Rev. 25 (23), 3150–3184. doi: 10.1016/j.quascirev.2006.07.008
Dai M., Su J., Zhao Y., Hofmann E. E., Cao Z., Cai W.-J., et al. (2022). Carbon fluxes in the coastal ocean: synthesis, boundary processes, and future trends. Annu. Rev. Earth Planetary Sci. 50 (1), 593–626. doi: 10.1146/annurev-earth-032320-090746
Dou Y., Li Q., Wu Y., Zhao J., Sun C., Cai F., et al. (2021). Carbon and oxygen isotopic characteristics of benthic foraminifera and their paleocean indication significance in the Okinawa Trough since MIS6. Earth Sci. Front. 29 (4), 84. doi: 10.13745/j.esf.sf.2022.1.14
Dou Y., Yang S., Li C., Shi X., Liu J., Bi L. (2015). Deepwater redox changes in the southern Okinawa Trough since the last glacial maximum. Prog. Oceanography 135, 77–90. doi: 10.1016/j.pocean.2015.04.007
Dou Y., Yang S., Liu Z., Clift P. D., Yu H., Berne S., et al. (2010). Clay mineral evolution in the central Okinawa Trough since 28ka: Implications for sediment provenance and paleoenvironmental change. Palaeogeography Palaeoclimatology Palaeoecol. 288 (1), 108–117. doi: 10.1016/j.palaeo.2010.01.040
Fontugne M. R., Jouanneau J.-M. (1987). Modulation of the particulate organic carbon flux to the ocean by a macrotidal estuary: Evidence from measurements of carbon isotopes in organic matter from the Gironde system. Estuarine Coast. Shelf Sci. 24 (3), 377–387. doi: 10.1016/0272-7714(87)90057-6
Gong X., Lembke-Jene L., Lohmann G., Knorr G., Tiedemann R., Zou J. J., et al. (2019). Enhanced North Pacific deep-ocean stratification by stronger intermediate water formation during Heinrich Stadial 1. Nat. Commun. 10 (1), 656. doi: 10.1038/s41467-019-08606-2
Jian Z., Huang B., Kuhnt W., Lin H.-L. (2001). Late quaternary upwelling intensity and east asian monsoon forcing in the south China sea. Quaternary Res. 55 (3), 363–370. doi: 10.1006/qres.2001.2231
Jian Z., Wang P., Saito Y., Wang J., Pflaumann U., Oba T., et al. (2000). Holocene variability of the Kuroshio Current in the Okinawa Trough, northwestern Pacific Ocean. Earth Planetary Sci. Lett. 184 (1), 305–319. doi: 10.1016/S0012-821X(00)00321-6
Jiao N., Herndl G. J., Hansell D. A., Benner R., Kattner G., Wilhelm S. W., et al. (2010). Microbial production of recalcitrant dissolved organic matter: long-term carbon storage in the global ocean. Nat. Rev. Microbiol. 8 (8), 593–599. doi: 10.1038/nrmicro2386
Kao S. J., Horng C. S., Hsu S. C., Wei K. Y., Chen J., Lin Y. S. (2005). Enhanced deepwater circulation and shift of sedimentary organic matter oxidation pathway in the Okinawa Trough since the Holocene. Geophys. Res. Lett. 32 (15). doi: 10.1029/2005GL023139
Kao S. J., Roberts A. P., Hsu S. C., Chang Y. P., Lyons W. B., Chen M. T. (2006). Monsoon forcing, hydrodynamics of the Kuroshio Current, and tectonic effects on sedimentary carbon and sulfur cycling in the Okinawa Trough since 90 ka. Geophys. Res. Lett. 33 (5). doi: 10.1029/2005GL025154
Keil R. (2017). Anthropogenic forcing of carbonate and organic carbon preservation in marine sediments. Annu. Rev. Mar. Sci. 9 (1), 151–172. doi: 10.1146/annurev-marine-010816-060724
Knudson K. P., Ravelo A. C. (2015). North Pacific Intermediate Water circulation enhanced by the closure of the Bering Strait. Paleoceanography 30 (10), 1287–1304. doi: 10.1002/2015PA002840
Lambeck K., Chappell J. (2001). Sea level change through the last glacial cycle. Science 292 (5517), 679–686. doi: 10.1126/science.1059549
Li D., Chang Y.-P., Li Q., Zheng L., Ding X., Kao S.-J. (2018). Effect of sea-level on organic carbon preservation in the Okinawa Trough over the last 91 kyr. Mar. Geology 399, 148–157. doi: 10.1016/j.margeo.2018.02.013
Li D., Zheng L.-W., Jaccard S. L., Fang T.-H., Paytan A., Zheng X., et al. (2017). Millennial-scale ocean dynamics controlled export productivity in the subtropical North Pacific. Geology 45 (7), 651–654. doi: 10.1130/g38981.1
Lim D., Kim J., Xu Z., Jeong K., Jung H. (2017). New evidence for Kuroshio inflow and deepwater circulation in the Okinawa Trough, East China Sea: Sedimentary mercury variations over the last 20 kyr. Paleoceanography 32 (6), 571–579. doi: 10.1002/2017PA003116
Martínez-García A., Sigman D. M., Ren H., Anderson R. F., Straub M., Hodell D. A., et al. (2014). Iron fertilization of the subantarctic ocean during the last ice age. Science 343 (6177), 1347–1350. doi: 10.1126/science.1246848
Matsuzaki K. M., Itaki T., Tada R. (2019). Paleoceanographic changes in the Northern East China Sea during the last 400 kyr as inferred from radiolarian assemblages (IODP Site U1429). Prog. Earth Planetary Sci. 6 (1), 22. doi: 10.1186/s40645-019-0256-3
Meyers P. A. (1997). Organic geochemical proxies of paleoceanographic, paleolimnologic, and paleoclimatic processes. Organic Geochemistry 27 (5), 213–250. doi: 10.1016/S0146-6380(97)00049-1
Nakamura H., Nishina A., Liu Z., Tanaka F., Wimbush M., Park J.-H. (2013). Intermediate and deep water formation in the Okinawa Trough. J. Geophysical Research: Oceans 118 (12), 6881–6893. doi: 10.1002/2013JC009326
Okazaki Y., Timmermann A., Menviel L., Harada N., Abe-Ouchi A., Chikamoto M. O., et al. (2010). Deepwater formation in the north pacific during the last glacial termination. Science 329 (5988), 200–204. doi: 10.1126/science.1190612
Praetorius S. K., Mix A. C., Walczak M. H., Wolhowe M. D., Addison J. A., Prahl F. G. (2015). North Pacific deglacial hypoxic events linked to abrupt ocean warming. Nature 527 (7578), 362–366. doi: 10.1038/nature15753
Rae J. W. B., Gray W. R., Wills R. C. J., Eisenman I., Fitzhugh B., Fotheringham M., et al. (2020). Overturning circulation, nutrient limitation, and warming in the Glacial North Pacific. Sci. Adv. 6 (50), eabd1654. doi: 10.1126/sciadv.abd1654
Saito Y., Katayama H., Ikehara K., Kato Y., Matsumoto E., Oguri K., et al. (1998). Transgressive and highstand systems tracts and post-glacial transgression, the East China Sea. Sedimentary Geology 122 (1), 217–232. doi: 10.1016/S0037-0738(98)00107-9
Schlitzer R. (2021) Ocean data view. Available at: https://odv.awi.de/.
Shao H., Yang S., Cai F., Li C., Liang J., Li Q., et al. (2016). Sources and burial of organic carbon in the middle Okinawa Trough during late Quaternary paleoenvironmental change. Deep Sea Res. Part I: Oceanographic Res. Papers 118, 46–56. doi: 10.1016/j.dsr.2016.10.005
Shi X., Wu Y., Zou J., Liu Y., Ge S., Zhao M., et al. (2014). Multiproxy reconstruction for Kuroshio responses to northern hemispheric oceanic climate and the Asian Monsoon since Marine Isotope Stage 5.1 (∼88 ka). Clim. Past 10 (5), 1735–1750. doi: 10.5194/cp-10-1735-2014
Shinjo R., Chung S.-L., Kato Y., Kimura M. (1999). Geochemical and Sr-Nd isotopic characteristics of volcanic rocks from the Okinawa Trough and Ryukyu Arc: Implications for the evolution of a young, intracontinental back arc basin. J. Geophysical Research: Solid Earth 104 (B5), 10591–10608. doi: 10.1029/1999JB900040
Sigman D. M., Boyle E. A. (2000). Glacial/interglacial variations in atmospheric carbon dioxide. Nature 407 (6806), 859–869. doi: 10.1038/35038000
Spezzaferri S. (1995). Planktonic foraminiferal paleoclimatic implications across the Oligocene-Miocene transition in the oceanic record (Atlantic, Indian and South Pacific). Palaeogeogr. Palaeoclimatol. Palaeoecol. doi: 10.1016/0031-0182(95)00076-X
Talley L. D. (1993). Distribution and formation of north pacific intermediate water. J. Phys. Oceanography 23 (3), 517–537. doi: 10.1175/1520-0485(1993)023<0517:Dafonp>2.0.Co;2
Tian J., Ma X., Zhou J., Wang W. (2017). Subsidence of the northern South China Sea and formation of the Bashi Strait in the latest Miocene: Paleoceanographic evidences from 9-Myr high resolution benthic foraminiferal δ18O and δ13C records. Palaeogeogr. Palaeoclimatol. Palaeoecol. 466, 382–391. doi: 10.1016/j.palaeo.2016.11.041
Ujiié Y., Asahi H., Sagawa T., Bassinot F. (2016). Evolution of the North Pacific Subtropical Gyre during the past 190 kyr through the interaction of the Kuroshio Current with the surface and intermediate waters. Paleoceanography 31 (11), 1498–1513. doi: 10.1002/2015PA002914
Vats N., Singh R. K., Das M., Holbourn A., Gupta A. K., Gallagher S. J., et al. (2021). Linkages between east China sea deep-sea oxygenation and variability in the east asian summer monsoon and kuroshio current over the last 400,000 years. Paleoceanogr. Paleoclimatol. 36 (12), e2021PA004261. doi: 10.1029/2021PA004261
Worne S., Kender S., Swann G. E. A., Leng M. J., Ravelo A. C. (2019). Coupled climate and subarctic Pacific nutrient upwelling over the last 850,000 years. Earth Planetary Sci. Lett. 522, 87–97. doi: 10.1016/j.epsl.2019.06.028
Zhang Y., Hu L., Wu Y., Dong Z., Yao Z., Gong X., et al. (2022). Glacial-Interglacial Variations in Organic Carbon Burial in the Northwest Pacific Ocean Over the Last 380 kyr and its Environmental Implications [Original Research]. Front. Earth Sci. 10. doi: 10.3389/feart.2022.886120
Zhao D. (2017). Sedimentary evolution in northern Okinawa Trough and their environmental response since the last 400 ka (Doctoral, Institute of Oceanology, Chinese Academy of Sciences (in Chinese with English abstract)).
Zhao D., Wan S., Lu Z., Zhai L., Feng X., Shi X., et al. (2021). Delayed collapse of the north pacific intermediate water after the glacial termination. Geophysical Res. Lett. 48 (13), e2021GL092911. doi: 10.1029/2021GL092911
Keywords: organic carbon burial, carbon cycle, foraminiferal carbon isotope, primary productivity, North Pacific Intermediate Water, Kuroshio Current
Citation: Jing Y, Zhang T, Zhu B, Zhao J, Zhao X, Dou Y, Li Q, Cai F, Hu B and Dong L (2024) Organic carbon burial and their implication on sea surface primary productivity in the middle Okinawa Trough over the past 200 ka. Front. Mar. Sci. 11:1331940. doi: 10.3389/fmars.2024.1331940
Received: 02 November 2023; Accepted: 08 January 2024;
Published: 07 February 2024.
Edited by:
Selvaraj Kandasamy, Central University of Tamil Nadu, IndiaReviewed by:
Yuan-Pin Chang, National Sun Yat-sen University, TaiwanCopyright © 2024 Jing, Zhang, Zhu, Zhao, Zhao, Dou, Li, Cai, Hu and Dong. This is an open-access article distributed under the terms of the Creative Commons Attribution License (CC BY). The use, distribution or reproduction in other forums is permitted, provided the original author(s) and the copyright owner(s) are credited and that the original publication in this journal is cited, in accordance with accepted academic practice. No use, distribution or reproduction is permitted which does not comply with these terms.
*Correspondence: Liang Dong, ZG9uZ2xpYW5nQHNqdHUuZWR1LmNu; Jingtao Zhao, emhhb2ppbmd0YW8xMTNAMTYzLmNvbQ==
†These authors have contributed equally to this work
Disclaimer: All claims expressed in this article are solely those of the authors and do not necessarily represent those of their affiliated organizations, or those of the publisher, the editors and the reviewers. Any product that may be evaluated in this article or claim that may be made by its manufacturer is not guaranteed or endorsed by the publisher.
Research integrity at Frontiers
Learn more about the work of our research integrity team to safeguard the quality of each article we publish.