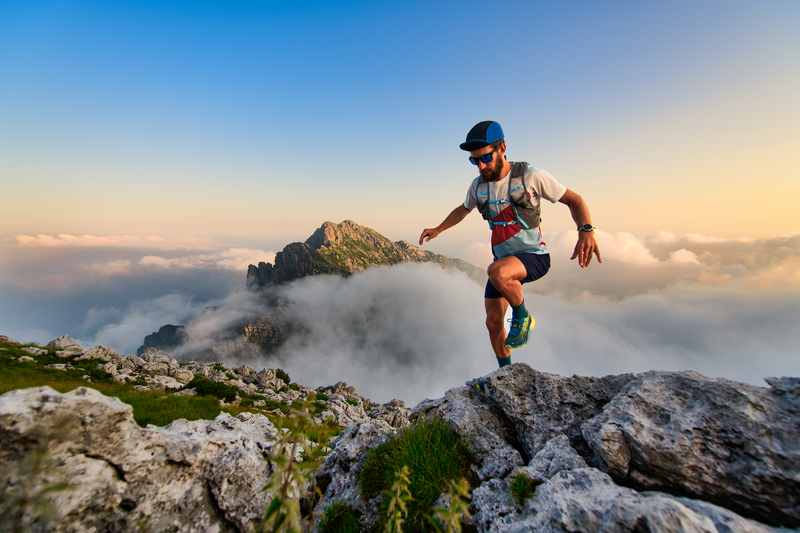
95% of researchers rate our articles as excellent or good
Learn more about the work of our research integrity team to safeguard the quality of each article we publish.
Find out more
ORIGINAL RESEARCH article
Front. Mar. Sci. , 14 February 2024
Sec. Marine Biology
Volume 11 - 2024 | https://doi.org/10.3389/fmars.2024.1327650
This article is part of the Research Topic Current and Future Threats to Marine Zooplankton in Changing Polar Oceans and Their Potential for Adaption and Coping View all 5 articles
Introduction: Currently, Arctic marine ecosystems are witnessing the most rapid physical changes worldwide, leading to shifts in pelagic and benthic communities and food web structure, concomitant with the introduction of boreal species. Gelatinous zooplankton or jellyfish represent one particular group of which several boreal species are prone to undergo significant poleward range expansions and population increases in the Arctic in the course of the ongoing changes. Historically, jellyfish were considered a trophic dead-end, but an increasing number of studies using modern tools have highlighted their role as major prey items in marine food webs. In this study, we aimed to verify the role of jellyfish and other metazoans as food sources in the Arctic polar night food web, when pelagic resources are limited.
Methods: We identified the diet of different bentho-pelagic amphipod species in the Atlantifying Kongsfjorden (West Svalbard) during the polar night. We regularly sampled lysianassoid and gammarid amphipods using baited traps and hand nets over a period of one month during the polar night and identified their diet spectrum by applying DNA metabarcoding (COI) to their stomach contents.
Results: We demonstrate that all investigated species are omnivorous. Fish species including polar cod and snailfish, likely in the shape of carrion, played an important role in the diet of the scavengers Orchomenella minuta and Anonyx sarsi. Predation and potential scavenging on jellyfish contributed to the diet of all four investigated species, particularly for the species Gammarus setosus and G. oceanicus, as evidenced by high read abundances and high frequencies of occurrence. Besides jellyfish, crustaceans and macroalgae were important components of the diet of the two Gammarus species.
Discussion: The diverse jellyfish community present in Kongsfjorden in the polar night is clearly being utilized as a food source, either through pelagic feeding or feeding on jelly-falls, albeit to a different extent in the local amphipod community. These findings provide novel insights into the Arctic food web during the polar night and represent the first evidence of feeding on natural (non-experimental) jelly-falls.
The Arctic is warming at a faster pace than the ocean average, which has led to decreasing sea-ice extent and thickness over the past decades (Polyakov et al., 2012; Carmack et al., 2015; Rantanen et al., 2022). Due to Arctic amplification, the warming rates in Svalbard are locally up to seven times higher than the ocean average, resulting in temperature increases of more than 1.25°C per decade between 1979-2021 (Rantanen et al., 2022). Additionally, the European Arctic, in general, is increasingly impacted by Atlantic water masses, mainly by the West Spitsbergen Current, flowing northward along the west coast of Svalbard. Due to several factors linked to climate change, the inflow of saline, warm Atlantic water has increased in the past years (Polyakov et al., 2017; Wang et al., 2020) and was shown to vary seasonally in the northern Barents Sea, with stronger inflow pulses between autumn and winter (Lundesgaard et al., 2022). This progressing “Atlantification” will lead to further sea-ice retreat in the eastern Eurasian Basin (Polyakov et al., 2017) and accelerated dramatic warming of the European Arctic. Correspondingly, the Svalbard archipelago is under increasing influence of Atlantic waters of the West Spitsbergen Current. However, the influence and extent of Atlantification differs among Svalbard fjords, depending on their hydrographic dynamics, the presence of glaciers and of natural barriers such as sills (Cisek et al., 2017; Promińska et al., 2017). Due to the absence of a sill, Kongsfjorden, located on the west coast of Spitsbergen, has a direct connection to the West Spitsbergen Current and has now transitioned into an Atlantic regime (De Rovere et al., 2022). Therefore, Kongsfjorden is often depicted as a sentinel for the ongoing change and has been used as a study model for the future Arctic (Piskozub, 2017; Bischof et al., 2019). Whereas the inflow of Atlantic waters used to be largely prevented during wintertime in the past due to differences in water density between shelf and fjord, an increased inflow is now more commonly observed due to atmospheric and oceanic warming (Tverberg et al., 2019). As a result, a warming of about 2°C per decade has been recorded during the polar night (November to February) in Kongsfjorden, with water temperature increasing from 0.3°C in 2004 to 4°C in 2017 (Geoffroy et al., 2018; Cottier and Porter, 2020).
These physical changes lead to biotic shifts in community structures and ecosystem functioning (Wassmann et al., 2011). Boreal species are advected into the Arctic with the inflowing Atlantic waters, and warming and sea-ice retreat have allowed several species, for example the copepod Calanus finmarchicus or the amphipod Themisto compressa, to establish populations in newly colonized areas (Schröter et al., 2019; Tarling et al., 2022). Gelatinous zooplankton represent a typical group for which such climate-change driven range shifts have been projected and observed (Mańko et al., 2020; Mańko et al., 2022; Pantiukhin et al., 2023). Recent evidence highlights that boreal jellyfish species increasingly colonize Arctic waters (Geoffroy et al., 2018) or have become more abundant (Mańko et al., 2020; Pantiukhin et al., 2023). Poleward range shifts with an increase in suitable habitat space have been projected for dominant species of scyphozoans, hydrozoans and ctenophores (Pantiukhin et al., under review). Several jellyfish species are known to overwinter in coastal Arctic ecosystems, e.g., Cyanea capillata, Mertensia ovum and Bolinopsis infundibulum (Purcell et al., 2018). During the polar night in Kongsfjorden, we observed a diverse jellyfish community with multiple species, including larval and juvenile ctenophores, siphonophores, hydrozoans (e.g., Aglantha digitale) and scyphozoans (e.g., C. capillata and Periphylla periphylla) (Havermans et al., 2023). These jellyfish could be a food resource for pelagic and benthic communities, particularly during the polar night when food supply from primary production is limited. Jellyfish are known to increase rapidly in biomass and form large blooms under favorable environmental conditions, and hence, they could represent significant prey items for higher trophic levels (Richardson et al., 2009; Schaub et al., 2018). Furthermore, when these blooms die off, the accumulation of jellyfish carcasses on the seafloor can provide a resource for benthic communities (Chelsky et al., 2016; Sweetman et al., 2016). Nonetheless, jellyfish were long considered a “trophic dead-end”, and are still frequently missing as a component of food web models (Verity and Smetacek, 1996; Hamilton, 2016). This lack of consideration is also related to the limits of traditional examination methods, which usually overlook the rapidly digested soft-bodied food items, including gelatinous organisms. Trophic markers have too limited taxonomic resolution and experiments may not necessarily display natural feeding habits. To overcome these limitations, DNA metabarcoding of stomach contents represents one method that allows for a more reliable assessment of the role of jellyfish as a food source (Hays et al., 2018). For example, jellyfish and chaetognaths have been identified as dominant prey for the northern shrimp Pandalus borealis, a keystone species in the Arctic (Urban et al., 2022). A limited number of studies have investigated the role of jelly-falls for (bentho-)pelagic scavengers but food-fall experiments revealed the attraction of a specific scavenging community, including lysianassoid amphipods, decapod crustaceans and Atlantic hagfish (Sweetman et al., 2014; Sweetman and Chapman, 2015; Dunlop et al., 2017a; Dunlop et al., 2017b; Scheer et al., 2022). Besides these experimental approaches, so far, there is no direct evidence for scavenging on naturally sunken jellyfish carcasses.
In the past, biological activity was assumed to be low during the polar night, due to the absence of sunlight, and thus food limitation (Piepenburg, 2005; Smetacek and Nicol, 2005). However, several studies observed very active animal communities in Kongsfjorden during that season, both for zooplankton and benthic organisms (Nygård et al., 2012; Berge et al., 2015; Balazy et al., 2021). Here, we aimed to elucidate the diet of the amphipod community in Kongsfjorden during the polar night. We aimed to determine whether jellyfish play a role in the diet of several species of (bentho-)pelagic amphipods, both in the shape of active predation or scavenging on jelly-falls, particularly during the polar night when the role of jellyfish may be more pronounced due to limitation of other pelagic sources. The taxa sampled included the lysianassoid species Anonyx sarsi Steele and Brunel, 1968 and Orchomenella minuta (Krøyer, 1846), as well as the species Gammarus setosus Dementieva, 1931 and G. oceanicus Segerstråle, 1947. In the shallow waters of Kongsfjorden, A. sarsi and O. minuta represent an important part of the scavenging community (Legeżyńska, 2001; Legezynska, 2002). Feeding on carrion, these scavengers play a major role in nutrient cycling and maintaining ecosystem function in marine habitats (Britton, 1994). The true arctic gammarid species, G. setosus, and its boreal counterpart, G. oceanicus, play an important role in the Kongsfjorden food web as well (Węsławski and Kotwicki, 2018; Węsławski et al., 2018; Grabowski et al., 2019; Węsławski et al., 2021). Despite their importance, little is known about the feeding habits of these amphipod communities during the polar night (Nygård et al., 2012; Berge et al., 2015; Balazy et al., 2021). For A. sarsi, a diet dominated by polychaetes was previously reported (Sainte-Marie, 1986; Legeżyńska, 2008), while O. minuta was found to feed mainly on decomposed carrion, with large fractions of unidentifiable material in its stomachs (Oliver and Slattery, 1985; Legeżyńska, 2008). Additionally, crustaceans were part of the diet of O. minuta, assumed to result from predation (Legeżyńska, 2008). Gammarus setosus and G. oceanicus were previously found to efficiently feed on macroalgae (e.g., Jonne et al., 2006; Andersson et al., 2009; Beermann et al., 2018; Alberts-Hubatsch et al., 2019; Vader and Tandberg, 2019). These diet studies were based on morphological identifications, trophic markers, or inferred from feeding experiments (e.g., Jonne et al., 2006; Legeżyńska, 2008; Legeżyńska et al., 2012). Overall, it is known that scavenging amphipods often combine different feeding strategies, such as scavenging, predation and detritus feeding (Havermans and Smetacek, 2018). Thus, benthic communities are affected by the seasonal changes in the pelagic regime for example via export of phytodetritus (Piepenburg, 2005). However, Legeżyńska et al. (2012) did not observe any difference of benthic amphipod diets between summer and winter.
To overcome the limits related to traditional methods, we applied DNA metabarcoding to investigate different amphipod species’ polar night diets and the potential role of jellyfish and other metazoans as food source. We hypothesized that because of the presumed food limitation during the polar night, the investigated amphipods make use of a broad food spectrum including jellyfish. Furthermore, we hypothesized, that by applying DNA metabarcoding to the stomach contents, we can identify so far overlooked prey items.
Scavenging amphipods were collected using a baited trap in the harbor of Ny-Ålesund (Figure 1) (Svalbard, Norway) over a six-week period from mid-January to mid-February of 2022, allowing us to get a robust representation of the temporal variation in feeding (Figure 2). The bait contained dried squid (Dosidiscus gigas), anchovy (Encrasicholina spp.) and shrimps (unspecified) wrapped in a fine mesh to prevent amphipods from feeding on it. The trap was left in the water for approximately 24 hours. Gammarid amphipods were sampled at the water surface using a hand net. All individuals were then kept in separate beakers filled with unfiltered seawater until fixation to prevent further feeding. All collected amphipods were identified and stored in 96% ethanol. A total of 180 amphipods belonging to four different species were used for stomach content analysis: the lysianassoid scavenging species A. sarsi (n = 85) and O. minuta (n = 49), as well as the two gammarids G. setosus (n = 40) and G. oceanicus (n = 6).
Figure 1 General map of Svalbard, Kongsfjorden and the harbor of Ny-Ålesund. The red dot marks the sampling area. Copyright: Norwegian Polar Institute.
Figure 2 Timeline of the sampling events: each dot represents a sampling event; color coded numbers indicate the species names and sample size (blue: Anonyx sarsi; green: Orchomenella minuta; pink: Gammarus spp.). Numbers in brackets indicate the number of specimens remaining after data curation and used in the analyses.
Prior to dissection, the length of each individual was measured from the head to the telson, to the closest millimeter. Amphipod stomachs were dissected using two sharp pincers, which were flame-cleaned with 70% ethanol between every dissection. Details on the dissected specimens can be found in Supplementary Table 1 (Supplementary Material). DNA was isolated from stomach contents using the DNeasy Blood & Tissue kit (Qiagen), following the manufacturer’s instructions, with an elution volume of 100 µl. An extraction blank was added every 23 samples to check for potential contamination while handling the samples, and to detect cross-contamination between samples.
In order to identify the stomach contents of the investigated amphipods, the library preparation and sequencing were carried out by AllGenetics & Biology SL (www.allgenetics.eu). We targeted a fragment of the cytochrome c oxidase 1 gene (COI) which has been extensively used for the detection of a wide range of taxa in diet studies, including jellyfish (Wangensteen et al., 2018; Siegenthaler et al., 2019; Rodríguez-Barreras et al., 2020; Dischereit et al., 2022; Urban et al., 2022). The modified and highly degenerated Leray-XT primers were used to amplify the 313 bp long target fragment, the forward primer mlCOIintF-XT (5’-GGWACWRGWTGRACWITITAYCCYCC-3’) (Wangensteen et al., 2018) and the reverse primer jgHCO2198 (5’-TAIACYTCIGGRTGICCRAARAAYCA-3’) (Geller et al., 2013). These included a tail with a binding site for the universal Illumina adapters, that was attached to the fragment during the second PCR (F: ACACTCTTTCCCTACACGACGCTCTTCCGATCT; R: GTGACTGGAGTTCAGACGTGTGCTCTTCCGATCT). A two-step PCR protocol was applied for the library preparation. In the first step, the Leray fragment was amplified, which was done in PCR triplicates, to increase the likelihood of detecting rarer taxa. This PCR was carried out in a final volume of 12.5 µl, containing 2.5 µl of DNA template, 0.5 µM of the aforementioned Leray-XT primers, 6.25 µl of Supreme NZYTaq 2x Green Master Mix (NZYTech), and ultrapure water up to 12.5 µl. For some samples (Supplementary Table 1, Supplementary Material), an optimization of the PCR mix, likely because of a lesser DNA concentration, was necessary, which was done by increasing the amount of DNA template with up to 4.5 µl and increasing the amount of Supreme NZYTaq 2x Green Master Mix (NZYTech) up to 7.8 µl in order to successfully amplify the target fragment. The PCR conditions were set as follows: initial denaturation at 95°C for 5 min, followed by 35 cycles at 95°C for 30 s, 54°C for 45 s, 72°C for 45 s, and a final extension step at 72°C for 7 min. The PCR triplicates were then pooled before continuing with the second PCR. In the second PCR, oligonucleotide indices were attached to the fragments, allowing the pooling of different libraries. For this, 2.5 µl of raw PCR product from PCR1 and Illumina universal adapters, including individual indices i5 and i7 for every sample and control (AATGATACGGCGACCACCGAGATCTACAC-i5-ACACTCTTTCCCTACACGA; CAAGCAGAAGACGGCATACGAGAT-i7-GTGACTGGAGTTCAGACGTG) were used. The same PCR conditions as in the first PCR were applied, but with only 5 cycles and an annealing temperature of 60°C. Each PCR included one PCR negative control to detect potential contamination during PCRs. Finally, the sizes of the libraries were verified in a 2% agarose gel stained with GreenSafe (NZYTech) and checked via UV imaging. Before pooling, the libraries were purified using the Mag-Bind RXNPure Plus magnetic beads (Omega Biotek), following the manufacturer’s instructions. DNA quantity was measured using a Qubit with the dsDNA HS Assay (Thermo Fisher Scientific), before pooling the libraries equimolar. The samples were then sequenced on an Illumina NovaSeq sequencing platform producing 250 bp paired-end reads.
We used the MJOLNIR pipeline (v.1.1) (github.com/uit-metabarcoding/MJOLNIR) with the default parameters for the COI Leray-XT marker. Within this pipeline, OBItools (Boyer et al., 2016) was used for paired-end alignment and read-length filtering (using a phred quality score threshold of 30). We used VSEARCH to remove chimeric sequences (Rognes et al., 2016) and we applied the SWARM algorithm (d=13, maximum distance between sequences to be clustered together) for MOTU clustering (Mahé et al., 2015). Thereafter, we used Ecotag (Boyer et al., 2016) together with owi_add_taxonomy (Wangensteen and Turon, 2017), for the taxonomic assignment, using a custom reference database (DUFA_20210723, available from github.com/uit-metabarcoding/MJOLNIR). Finally, we used the LULU algorithm to remove pseudogenes from the dataset, since these are likely a result of errors during sequencing and PCR (Frøslev et al., 2017).
In terms of data curation, we removed Molecular Operational Taxonomic Units (MOTUs) with less than 5 reads on a sample-by-sample basis from the final dataset, to minimize the risk of false positive occurrence of MOTUs as a result of tag-switching in the final dataset (sensu Alberdi et al., 2018; Siegenthaler et al., 2019). In a next step, we performed a blank correction, which involved removing those MOTUs for which the abundance in the extraction controls and PCR negative controls was higher than 10% of the total reads of that MOTU (Wangensteen and Turon, 2017). The taxonomic assignment of the remaining MOTUs was then checked against the BOLD database using BOLDigger (Buchner and Leese, 2020) and was corrected when higher identity scores were reached in this database. As a next step, all MOTUs with less than an 85% match were removed from the dataset, as well as those assigned to insects, bacteria and terrestrial organisms. In order to remove the sequences belonging to the host individuals, we removed all MOTUs assigned to Anonyx spp., Gammarus spp., Orchomenella spp. or Tryphosidae. We did this for all samples to minimize the risk of tag-witching and thus reads being assigned to the wrong sample. For those amphipods for which we could not accurately assign potential host DNA in terms of taxonomic identification, individuals were identified morphologically. Three specimens were identified molecularly as Calliopius laeviusculus (Krøyer, 1838), since these were not our target taxa, they were removed from the dataset, and all reads belonging to this species were removed. We also removed reads that were assigned to potential parasites. After analyzing their proportions of the total remaining reads, phytoplankton and rotifers were also excluded from further analyses for two main reasons. First, we assume that the reads likely resulted from secondary predation by other prey items and second the genetic marker used targets metazoan DNA more efficiently (Wangensteen et al., 2018). After these curation steps, a number of stomach samples did not contain any reads. For this reason, 81 out of 180 individual stomach samples were removed. Further information on the stomach samples, including those removed from the data set, can be found in Supplementary Table 1 (Supplementary Material).
For data visualization, reads were transformed to relative read abundances (RRAs) on a sample per sample basis by calculating the relative proportion of reads obtained for one prey item compared to the overall reads obtained for the sample. Additionally, relative frequency of occurrence (%FOO) was calculated as the relative proportion of the detected occurrences of a prey item compared to the overall detections of prey items for one sample. Additionally, the absolute frequency of occurrence (FOO) was calculated for major prey groups for each amphipod species. We implemented a new metric for evaluating the importance of a food item for each of the amphipod species. This metric was calculated by multiplying average RRA and FOO for the major prey groups and subsequently assigned based on the range of values to three categories (<100: low; 101-499: medium; >500: high).
Explorative and statistical analyses were performed using RStudio and the packages tidyverse and vegan (Wickham et al., 2019; Oksanen et al., 2020; R Core Team, 2021). A non-metric multidimensional scaling model (NDMS) was used to identify differences and overlaps in the diet composition. The 4th root transformed RRAs were used to calculate Bray-Curtis dissimilarity values. Two outliers had to be removed to create the model (AStoZ030 and AStoZ117). The different clusters were identified using 95% confidence ellipses computed onto the model using the stat_ellipse function, assuming a multivariate t distribution. We used a PERMANOVA with 1000 permutations to identify significant differences between the prey. As PERMANOVA requires homogeneity in the data, this was tested using an ANOVA with the betadispers function of the vegan package (Oksanen et al., 2020). Pairwise testing was performed to further identify significant differences in the food composition between the three amphipod groups using the command pairwise.adonis from the package pairwiseADONIS (Martinez Arbizu, 2017). We applied the Bonferroni correction to adjust the p-values after pairwise comparison (Dunn, 1961).
Two samples failed to generate sequence data, and were thus excluded from the study. We removed 81 additional samples which no longer contained any reads after we applied the aforementioned curation steps. This left us with a final dataset including: 34 out of 85 individuals of A. sarsi, 36 out of 49 individuals of O. minuta, 24 out of 40 individuals of Gammarus setosus and 3 out of 6 individuals of G. oceanicus (Figure 2). Because of the small samples size for G. oceanicus, the two Gammarus species were sometimes grouped into Gammarus spp. in the following sections of the manuscript. Overall, A. sarsi had the highest percentage of empty stomachs, with 60% of the stomachs being excluded after data curation, while for O. minuta only 27% of the stomachs appeared to be empty (Table 1). For Gammarus spp., 41% of stomachs were considered empty, due to a lack of resulting reads after data curation (Table 1). Further information on the analyzed stomach samples can be found in Supplementary Table 1 (Supplementary Material).
Table 1 Number of reads recovered at the different analytical steps for each amphipod species, as well as number of MOTUs after the bioinformatic processing and number of MOTUs after data curation.
The raw sequencing output varied between 24 million and 34 million reads for the different amphipod taxa (Table 1). On average, this resulted in about 400,000 reads per sample for A. sarsi, 546,000 reads per sample for O. minuta, and 512,000 reads per sample for Gammarus spp. During the bioinformatics analysis, approximately 45% and 43% of the reads were kept for A. sarsi and Gammarus spp., respectively, while only 27% were kept for O. minuta. However, after the data curation described above, 0.04% and 0.12% of the initial reads remained for A. sarsi and Gammarus spp., respectively, while for O. minuta 4% of the initial reads remained after the data curation (Table 1). A total of 30,562,081 reads belonged to the amphipod species and were thus removed from the dataset, comprising a total of 15,442,760 reads assigned to A. sarsi, a total of 4,737,455 reads assigned to O. minuta or Tryphosidae and 9,736,865 reads assigned to Gammarus spp. Overall reads assigned to phytoplankton and rotifers accounted for 3.55%, 1.36% and 40.23% of the overall reads of dietary items for Gammarus spp., O. minuta and A. sarsi, respectively (Supplementary Figures 1, 2, Supplementary Materials). For A. sarsi, overall reads assigned to phytoplankton were only 0.85%. The reads belonging to rotifers resulted mainly from one Anonyx individual (AStoZ126), for which these made up the majority of the dietary reads.
We implemented a new metric for assessing the importance of a certain diet component for each amphipod taxon, which was calculated by multiplying FOO with average RRAs and subsequently assigning this value to three categories: low, medium and high importance (Table 2). For A. sarsi and O. minuta, fish species were of highest importance as prey, accounting for on average 43% and 38%, respectively. Fish was the only food item which was classified as of high importance for A. sarsi, while crustaceans were also of high importance in the diet of O. minuta. For Gammarus spp., the most important prey compared to other ingested taxa appeared to be jellyfish. Although the two scavenger species’ diets overlapped with fish being an important food item for both species, the overlap was less pronounced for other food items. The diets of the gammarids differed strongly to those of the scavengers. In the NMDS model of the prey spectra of the three taxa, the clusters of A. sarsi and O. minuta largely overlapped, while the centroids indicated some difference (Figure 3). These differences of diet were also confirmed by the PERMANOVA (p < 0.001; betadispers: not significant) and by additional pairwise testing which highlighted the differences in the food sources used by the three taxa. The amphipod taxa only shared two prey items (C. capillata and Semibalanus spp.), to different extents, with both C. capillata and Semibalanus spp. showing higher FOO and RRA in the stomachs of Gammarus spp., while these were similarly low for A. sarsi and O. minuta. (Table 2, Figures 4, 5).
Table 2 Food items detected in the guts of the amphipod taxa, with A = Anonyx sarsi (n=34); O = Orchomenella minuta (n=36); G = Gammarus spp. (n=27).
Figure 3 NMDS plot representing the prey composition of the different amphipod taxa (A. sarsi; O. minuta; Gammarus spp.). Each dot represents the food composition of one individual. The distance between the dots indicates the difference between the food compositions of two individuals. Ellipses represent 95% confidence intervals assuming a multivariate t distribution. Diamonds represent the centroids of each cluster.
Figure 4 Relative read abundances (RRAs) of metazoan and macroalgae items found in the stomach contents of the scavenger species Anonyx sarsi (upper panel, n=34) and Orchomenella minuta (lower panel, n=36). Bars represent different individuals sorted according to sampling dates in January and February 2022. Donut plots in reduced colors represent average RRA over all individuals.
Figure 5 Relative read abundances (RRAs) of metazoan and macroalgae items found in the stomach contents of Gammarus spp. (G. setosus and G. oceanicus). Bars represent different individuals sorted according to sampling dates in January and February 2022. Donut plots in reduced colors represent average RRA over all individuals.
The two scavenger species A. sarsi and O. minuta relied on a broad prey spectrum, displayed by a high intraspecific variability (Figures 3, 4). Overall, 23 different taxa were recovered for A. sarsi and 44 different taxa were recovered for O. minuta. However, one or two taxa dominated in the diet of most of the individuals.
In the diet of A. sarsi, reads belonging to fish species had the highest %FOO and highest average RRAs, highlighting that fish were of high importance. Fish reads were assigned to on average 43% over all samples. The most important fish species were polar cod (Boreogadus saida) and snailfish (Liparis fabricii) (Table 2, Figure 4), which accounted for 16% and 20% of the reads, respectively. Polychaetes, mollusks and crustaceans were of overall medium importance, with averaged RRA between 13 and 16%. Jellyfish (cnidarians and ctenophores), and macroalgae accounted for a small fraction of the reads and occurred in only a few stomachs. Jellyfish were detected in only three individuals and were represented by Scyphozoa (Cyanea capillata) and Hydrozoa (siphonophore Nanomia cara). One individual of A. sarsi yielded reads that were exclusively assigned to the macroalga Laminaria digitata in its stomach.
The diet of O. minuta was dominated by different fish species, of which most reads were assigned to polar cod (B. saida) and snailfish (L. fabricii), representing 20% and 16% of averaged prey reads, respectively (Table 2, Figure 4). Moreover, DNA assigned to B. saida and L. fabricii was found in the guts of almost 50% of the individuals of O. minuta and fish was thus assigned to be of high importance. Additionally, crustaceans were classified of high importance with 32 occurrences and accounting for on average 26% of the RRA. Within the crustaceans, other amphipod species accounted on average for most of the RRA. The guts of many individuals had high RRAs assigned to the black guillemot (Cephus grylle). For A. sarsi, reads belonging to this bird species were only found in very few stomachs. We found jellyfish to be of low importance in the diet of O. minuta, with reads assigned to scyphozoans (C. capillata), hydrozoans (Opercularella lacerata, Tiaropsis multicirrata and Leptothecata) and ctenophores (Bolinopsis infundibulum). Hydrozoans were only found in a small number of stomachs, with low RRAs, with the exception of three individuals. Macroalgae were detected in the guts of several individuals but, in most cases, they did not represent dominant food items. Reads assigned to cephalopods are likely a result from DNA shed by the squid bait but were only found in the guts of few individuals of both scavenger species.
No clear differences could be observed between the diet composition of the two Gammarus species, G. setosus and G. oceanicus (Figure 5). Due to this and to the low number of individuals of G. oceanicus, the diet of the two species were further analyzed together as Gammarus spp. Similar to the scavenging amphipods, the stomach contents of the different individuals were mostly dominated by one or two taxa. The prey spectrum of Gammarus spp. was narrower than that of the two scavenger species, with only 17 different food items detected. In general, the diet of Gammarus spp. was comprised of three major prey groups: jellyfish, macroalgae and crustaceans. Jellyfish (mainly scyphozoans) were most frequently detected and in high RRAs, thus they were classified as a prey item of high importance. On average, Cnidaria accounted for 42% of the RRAs, with 35% of reads assigned to the scyphozoan Cyanea capillata (Table 2, Figure 5). Other jellyfish were detected in lower RRAs and in less FOO; these reads belonged to Hydrozoa (Nanomia cara and Obelia longissima) and Ctenophora (Bolinopsis infundibulum). Macroalgae were also found to be a diet component of high importance, as demonstrated by the high FOO and high average RRAs. Crustaceans were classified as prey of medium importance, and most of the crustacean reads were assigned to the barnacle Semibalanus spp.
A decreased availability of pelagic food sources is expected during the polar night due to the lower ambient light, which limits primary production. Polar zooplankton taxa show different strategies to cope with such food limitation. Some amphipod and copepod species reduce their feeding rate or overwinter in a state of arrested development, referred to as diapause, relying on lipid storage (Hagen, 1999; Hagen and Auel, 2001; Auel et al., 2002). Recently, it has been shown that some crustacean zooplankton taxa such as under-ice amphipods switch to an omnivorous feeding mode, increasing their feeding flexibility (Kunisch et al., 2023).
We excluded several individuals from the further analyses in this study, as the number of reads corresponding to diet items was zero after the data curation and stomachs were thus declared empty. Empty stomachs inferred by DNA reads could indicate irregular feeding or recent starvation undergone by these individuals. Most of these specimens belonged to Anonyx sarsi (60% empty stomachs) and Gammarus spp. (41% empty stomachs), while less specimens of Orchomenella minuta (27%) had to be removed for this reason. In a previous investigation, 36.4% of A. sarsi collected in the Saint Lawrence Estuary during summertime had empty stomachs (Sainte-Marie, 1986). The slightly higher proportion of empty stomachs in A. sarsi, in Kongsfjorden in the polar night may be due to more irregular feeding events or longer periods of starvation during the polar night. A. sarsi was found to survive long periods of starvation of up to 30 days with almost no mortality (Sainte-Marie and Hargrave, 1987). For the same sampling period, only 27% of the stomachs of O. minuta were empty. This supports the findings of an earlier study that suggested that smaller lysianassoid species such as O. minuta increase their opportunistic feeding behavior during periods with limited food resources, whereas larger species such as Anonyx spp. may not extend their prey spectrum and can endure longer periods of starvation (Legeżyńska, 2008). Larger lysanassoid abundances were detected in the polar night during previous baited trap studies (Nygård et al., 2012; Balazy et al., 2021). This may not necessarily reflect local seasonal changes in abundances, but only a higher attraction to the bait for these amphipod species, which may be caused by limited food supply during the polar night. Further studies are needed to provide reliable estimates of amphipod abundances across the different seasons.
We found both metazoans and macroalgae to be part of the diet of all investigated amphipod species. The presence of a combination of metazoan and macroalgal DNA in the stomachs of the scavenging lysianassoids underlines that omnivory might be common among a large variety of taxa during the Arctic polar night (Kunisch et al., 2023). The two scavenger species exhibited a broader food spectrum than the gammarids. For the Gammarus species, major food items were jellyfish, other crustaceans and macroalgae. This difference in prey diversity may be explained by a higher degree of opportunistic feeding in the scavengers, a generally higher availability of different food items as carrion or prey to the scavengers and/or higher mobility characterizing lysianassoid amphipods (Havermans and Smetacek, 2018).
In the diet of A. sarsi, fish species were found to be of high importance, pointed out by both high average RRAs and high FOO. Annelids (mainly polychaetes), crustaceans and mollusks were of medium importance. Fish had also been reported to be part of the diet of Anonyx nugax (Legeżyńska, 2008) whereas high amounts of unidentified food items in the stomachs of A. sarsi were assumed to be soft tissue of fish (Sainte-Marie, 1986; Legeżyńska, 2008). By applying DNA metabarcoding, we could confirm that fish are an important prey item of A. sarsi, mainly the gelatinous snailfish (Liparis fabricii). This arctic fish species spawns during the polar night while the larvae are most abundant in May (Ponton et al., 1993). As many arctic fish species, Liparis spp. have undergone a northward shift with increasing water temperatures (Eriksen et al., 2012). Therefore, the role of this fish species in the diet of scavenger species deserves to be further investigated. Polychaetes have previously been reported to constitute an important fraction of the diet of A. sarsi (Sainte-Marie, 1986; Legeżyńska, 2008); as well as calanoid copepods, to a lesser extent (Werner et al., 2004). These observations, corroborated by our results, reflect the omnivory and the large flexibility of A. sarsi in terms of food choice. As previous studies were done in spring and summer, ours is the first investigation of the polar night diet of A. sarsi using DNA metabarcoding, allowing us to determine the food spectrum with a high taxonomic resolution. The reported broad prey spectrum, along with omnivory and high mobility, may be important traits of this species to cope with the food limitations of the Arctic polar night.
Similar to A. sarsi, different fish species played an important role in the diet of O. minuta, but also other crustaceans were identified as food items of high importance. As O. minuta was previously hypothesized to mainly feed on decomposed carrion, previous morphology-based diet studies could not provide clear identification of the major fraction of the stomach contents (Oliver and Slattery, 1985; Legeżyńska, 2008). So far, crustaceans were known to account for 20 to 30% of the diet of O. minuta during summertime and were hypothesized to be the result from predation rather than scavenging (Legeżyńska, 2008). However, it is generally challenging and speculative to disentangle whether signatures of small-sized food items (e.g., crustaceans, polychaetes) in the diets of lysianassoid species may have been acquired as a result of scavenging or predation. Our DNA-based approach identified fish as the most important food source of O. minuta collected during the polar night, which was not known to be an important food for this scavenger. In a number of individuals, belonging to both scavenger species, DNA of the black guillemot (Cephus grylle) was detected. This seabird was observed frequently in the sampling area throughout our sampling events. We thus assume that the low RRAs, assigned to this species, were a result of ingesting environmental DNA shed by this species, e.g. through feces, or resulted from feeding on a black guillemot carcass. Many scavenger taxa in shallow waters display complex feeding habits and can switch to different feeding strategies besides scavenging (Britton, 1994). This complex feeding behavior, found in previous studies on A. sarsi and O. minuta (e.g., Sainte-Marie and Lamarche, 1985; Sainte-Marie, 1986), was confirmed here over the six-week sampling period. Similar to A. sarsi, this flexibility may facilitate the survival of O. minuta in periods of food limitations such as during the polar night.
Anonyx sarsi and O. minuta are known to co-exist in the same habitats of sublittoral zones in Kongsfjorden, but seem to prefer different microhabitats and use different feeding strategies (Sainte-Marie, 1984; Sainte-Marie, 1986; Legeżyńska, 2001). In Kongsfjorden in summer, the diet of A. sarsi was dominated by polychaetes whereas these were of only minor importance in O. minuta. Stomachs of the latter species, in turn, contained higher amounts of amorphic organic matter and detritus than those of A. sarsi (Legeżyńska, 2008). Although in the current study the polar night diets of both species considerably overlap, with fish as dominant component, they were characterized by marked differences in other dominant diet components. Correspondingly, the mouthpart morphology of A. sarsi seems to be adapted to carrion feeding whereas the mouthparts of Orchomenella allow for a more generalized feeding (Sainte-Marie, 1984; Legeżyńska, 2008). Therefore, the different lysianassoid species probably exploit different size fractions of the same prey items (Legeżyńska, 2008). Different carrion-feeding traits of co-existing lysianassoid amphipods have also been documented in Antarctic fjord systems (Seefeldt et al., 2017). These differences in feeding behavior and prey preference, along with some differences in microhabitat use and/or physiological trade-offs, may contribute to a partitioning of resources between A. sarsi and O. minuta (Schoener, 1974; Connell, 1983; Schoener, 1986). With the method we applied, we could not distinguish whether the two scavengers target different size fractions or parts of carrion. Nonetheless, a limited overlap and the wide range of prey species fed upon points towards a rather limited potential for interspecific competition for food between the scavenger species.
Seventeen different food taxa were detected in the gammarid stomachs, with reads being assigned to jellyfish, macroalgae and crustaceans. Among all food sources, macroalgal reads were characterized by the highest number of different MOTUs. Jellyfish and macroalgae were seemingly of high importance within the diet of Gammarus spp., highlighted by the combination of high FOO and high average RRAs. Marine gammarids, including G. setosus and G. oceanicus, are commonly associated with macroalgae in northern Europe and were shown to be effective consumers of algal material (e.g., Jonne et al., 2006; Andersson et al., 2009; Beermann et al., 2018; Alberts-Hubatsch et al., 2019; Vader and Tandberg, 2019).
Our findings revealed a large metazoan fraction in the stomach contents of arctic Gammarus spp., indicating a largely omnivorous feeding during the polar night. Contrastingly, a morphological stomach content analysis of G. setosus during summer revealed that macroalgae and crustaceans only accounted for small proportions of the diet (Legeżyńska et al., 2012). Thus, the larger metazoan fraction found in the current study may reflect a flexible adaptation to the seasonal limitation in food sources. The elevated proportion of macroalgae observed in the current study may be caused by the degradation of macrophytes during this season, which makes the material more usable for mesograzers, compared to that of alive macroalgae (Duggins and Eckman, 1997; Hop et al., 2002; Quijón et al., 2008). Correspondingly, the high-arctic pack-ice species Gammarus wilkitzkii Birula, 1897, exhibits a carnivorous-omnivorous feeding throughout the year (Poltermann, 2001; Arndt, 2002; Kunisch et al., 2023). However, the feeding habits of G. wilkitzkii vary on spatial and temporal scales. Correspondingly, Werner and Auel (2005) found seasonal changes in the diet of this amphipod with more herbivorous signals during summer and higher levels of carnivory during winter. Nevertheless, trophic ecology of the majority of Gammarus species is still largely unknown and when it has been investigated, it was commonly done in the form of feeding experiments (e.g., Jonne et al., 2006; Andersson et al., 2009), which may not reflect natural feeding preferences.
There were no clear differences in the diets of Gammarus setosus and G. oceanicus investigated here. Both species are similar in size, and have been reported to exhibit similar feeding behaviors, habitat preferences and reproduction time, and thus assumed to be interspecific competitors in the rocky shores around Svalbard (Węsławski and Legeżyńska, 2002; Węsławski et al., 2021). In our investigation, both species fed on crustaceans, hydrozoans and scyphozoans in similar read proportions, but this result has to be regarded with caution as only three individuals of the investigated gammarids could be identified as G. oceanicus, while the remaining individuals were identified as G. setosus. However, as the habitats of both species are affected by the ongoing Atlantification, information on the diet of the two species is crucial, facilitating the prediction of possible consequences of shifts in the species’ geographic distributions (Węsławski et al., 2021).
The frequent presence of jellyfish DNA in the guts of these two gammarid species represents a novel finding. The most detected jellyfish was the scyphozoan Cyanea capillata. We observed several C. capillata alive or floating dead at the water surface during sampling. As a result, the gammarids may possibly either feed on tissue or mucus that is shed by the jellyfish. Alternatively, we hypothesize that the gammarids may use this large scyphozoan as shelter and protection from other predators, as observed for several hyperiid amphipods (Condon and Norman, 1999; Fleming et al., 2014; Havermans et al., 2019), which could have led to the ingestion of DNA shed by the jellyfish. However, we did not observe gammarids attached to these jellyfish. Thus, potential interactions of gammarids and scyphozoans still requires further investigation.
Reads assigned to different jellyfish species were detected in the stomachs of both lysianassoid species, including scyphozoans, as well as different hydrozoans. Additionally, ctenophores were detected in the stomachs of O. minuta. The term “jelly-falls” is traditionally used for gelatinous carcasses that end up visibly on the seafloor, such as carcasses of large scyphozoans (e.g., Periphylla periphylla or C. capillata), or rather intact thaliacean remains (e.g., salps and doliolids) (Lebrato et al., 2012). Smaller hydrozoans, colonial siphonophores and the highly delicate ctenophores have so far not yet been reported as “jelly-falls”, probably because they rapidly disintegrate after dying. Hence, the detected jellyfish DNA could result from a combination of scavenging on large carcasses (C. capillata), predation on pelagic hydromedusae and ctenophores or from the ingestion of environmental DNA in the water (e.g. from shed mucus) for the species reported with low RRAs. The ctenophore Bolinopsis infundibulum was found in relatively high frequencies in the stomachs of O. minuta, which suggests an active predation by the amphipod, since these fragile ctenophores are unlikely to end up as “jelly-falls”. Several scyphozoan individuals of C. capillata and Periphylla periphylla were observed during sampling, either swimming at the surface or as floating carcasses, thus it seems reasonable to assume scavenging on their carcasses that have sunk to the seafloor. So far, feeding on jellyfish carcasses or “jelly-falls” by lysianassoid amphipods and other taxa had only been documented in experimental settings (e.g., with baited camera deployments) (e.g, Sweetman et al., 2014; Dunlop et al., 2017b; Scheer et al., 2022). Therefore, our findings represent first evidence of potential scavenging on naturally sunken jellyfish carcasses. In this study, C. capillata was the only scyphozoan detected in the stomachs of all investigated amphipods. Previous experimental studies highlight generally a higher attraction of scavengers (e.g., hagfish) to Periphylla than to Cyanea bait (Sweetman et al., 2014). Hagfish were less abundant at the carcasses of C. capillata, assumingly because this species sheds toxic mucus. Whereas this could affect fish species, other scavengers might be tolerant to these toxins (Shanks and Graham, 1988; Lassen et al., 2011; Sweetman et al., 2014). The absence of P. periphylla in the stomachs of the amphipods is thus surprising. It might be that the scavengers were not attracted by this jellyfish species or that the coverage in reference databases are not sufficient for this species. Nonetheless, the COI Leray-XT primers were shown to be efficient in detecting gelatinous zooplankton reads, particularly for scypho- and hydrozoan species (Dischereit et al., 2022; Urban et al., 2022). Some ctenophore or tunicate taxa may however be more efficiently picked up with other markers, e.g. 18S rDNA (Günther et al., 2021). This indicates also the advantage of applying multi-marker metabarcoding and of further efforts to render reference databases more comprehensive for further investigations.
Few studies have investigated the nutritional value of different jellyfish species (e.g., Doyle et al., 2007; Wakabayashi et al., 2016; Stenvers et al., 2020). The nutritional value of scyphozoans largely increases during the formation of gonads (Stenvers et al., 2020). For example, in C. capillata, the gonad tissue has a considerably higher energetic value compared to the bell tissue (Doyle et al., 2007) and also the scyphozoan Aurelia aurita was found to have some specific fatty acids only present in the gonad tissue (Stenvers et al., 2020). Hence, jellyfish can provide the predators with macronutrients and essential fatty acids (Wakabayashi et al., 2016; Stenvers et al., 2020). Nevertheless, Cyanea builds up gonads usually during the summer months (in the North Sea) (Holst and Jarms, 2007), so this highly nutritious tissue might not be available for the amphipods during the polar night. In the current study, we found that especially the scyphozoan C. capillata was part of the diet of all investigated amphipod species. It contributed mainly to the overall diet of Gammarus spp., with high reads and occurrences, compared to lower occurrences and reads in the stomachs of the two lysianassoid species. From our results, we cannot derive how a potential seasonal change towards a more jellyfish-dominated diet may impact energy flows in the food web. Hence, we argue for further studies on the nutritional value of gelatinous zooplankton in the Arctic food web.
Overall, we can conclude that the observed diverse and abundant jellyfish community of Kongsfjorden during the polar night could be a food source for arctic gammarid amphipods during this period. Although the read amounts for the two lysianassoid species were low compared to those of other dominant food items such as fish, this study presents the first evidence of jellyfish serving as food item for these species. In the light of ongoing climate change, jellyfish could potentially gain a more important role as prey in future benthic and pelagic communities. Indeed, different jellyfish species, including Cyanea capillata, are expected to benefit from the observed changes in the Arctic (Pantiukhin et al., under review). The scyphozoan C. capillata was part of the polar night diet of all investigated species and represented the dominant prey item in Gammarus spp., but several other jellyfish such as ctenophores could also be detected. Due to the fast degradation of jellyfish tissue and the methodological limitations of traditional stomach content analyses, jellyfish have most likely been overlooked as major prey in the past. This has led to an underestimation of the role of jellyfish as a food source for (bentho-)pelagic amphipods, therefore neglecting their role in the functioning of polar coastal food webs. To conclude, our findings corroborate the paradigm shift from jellyfish as “trophic dead-end” to a potentially major role as prey for various trophic levels (Hays et al., 2018). In the light of the observed and projected increase of abundances of several jellyfish species in Arctic waters as a result of the ongoing Atlantification (Pantiukhin et al., 2023; Pantiukhin et al., under review), the role of jellyfish in fjord ecosystems as well as in the open ocean needs to be further clarified in order to make reliable predictions on the food web of the “new Arctic”.
The datasets presented in this study can be found in online repositories. The names of the repository/repositories and accession number(s) can be found below: https://www.ncbi.nlm.nih.gov/sra/PRJNA1013147, PRJNA1013147.
The manuscript presents research on animals that do not require ethical approval for their study.
AD: Conceptualization, Data curation, Formal Analysis, Writing – original draft, Investigation, Methodology, Visualization. JB: Writing – review & editing, Methodology. BL: Writing – review & editing, Methodology. OW: Writing – review & editing, Formal Analysis, Methodology. SN: Writing – review & editing, Formal Analysis. CH: Conceptualization, Funding acquisition, Writing – original draft, Resources, Supervision.
The author(s) declare financial support was received for the research, authorship, and/or publication of this article. This study has been conducted in the framework of the Helmholtz Young Investigator Group “ARJEL -Arctic Jellies” with the project number VH-NG-1400, funded by the Helmholtz Society and the Alfred Wegener Institute Helmholtz Centre for Polar and Marine Research. This study was further supported by the Helmholtz Research Programme “Changing Earth -Sustaining our Future” Topic 6, Subtopic 6.1. BL was funded by the French Polar Institute Paul Emile Victor (Project IPEV 1190 MADFOOD 2) and was supported by a Fellowship from the Hanse-Wissenschaftskolleg Intitute for Advanced Study, Delmenhorst, Germany. OW is a member of the research group 2021 SGR 01271 funded by the Generalitat de Catalunya (AGAUR) and is funded by project MARGECH (PID2020-118550RB) of the Spanish Ministry of Science and Innovation (MCIN/AEI/10.13039/501100011033). JB was funded by the German Federal Agency for Nature Conservation (BfN; grant numbers 3519532201 and 3519532201, LABEL project).
The sampling was conducted during the AWIPEV KOP-183 winter campaign entitled “Arctic gelatinous zooplankton in the Polar night”. A permit for this project was obtained and registered under Research in Svalbard RIS-11789. We are very grateful to the AWIPEV coordination for organizing our campaign, and to AWIPEV station leader Gregory Tran and logistics engineer Yohann Dulong for their skillful support on site. We are also thankful for the support of Kings Bay Marine Lab, in particular the engineer Marine Ilg and the captain of the MS Teisten, Erlend Havenstrøm, for their assistance during sampling.
The authors declare that the research was conducted in the absence of any commercial or financial relationships that could be construed as a potential conflict of interest.
All claims expressed in this article are solely those of the authors and do not necessarily represent those of their affiliated organizations, or those of the publisher, the editors and the reviewers. Any product that may be evaluated in this article, or claim that may be made by its manufacturer, is not guaranteed or endorsed by the publisher.
The Supplementary Material for this article can be found online at: https://www.frontiersin.org/articles/10.3389/fmars.2024.1327650/full#supplementary-material
Alberdi A., Aizpurua O., Gilbert M. T. P., Bohmann K. (2018). Scrutinizing key steps for reliable metabarcoding of environmental samples. Methods Ecol. Evol. 9 (1), 134–147. doi: 10.1111/2041-210X.12849
Alberts-Hubatsch H., Slater M. J., Beermann J. (2019). Effect of diet on growth, survival and fatty acid profile of marine amphipods: implications for utilisation as a feed ingredient for sustainable aquaculture. Aquaculture Environ. Interact. 11, 481–491. doi: 10.3354/aei00329
Andersson S., Persson M., Moksnes P.-O., Baden S. (2009). The role of the amphipod Gammarus locusta as a grazer on macroalgae in Swedish seagrass meadows. Mar. Biol. 156, 969–981. doi: 10.1007/s00227-009-1141-1
Arndt C. (2002). Feeding ecology of the arctic ice-amphipod Gammarus wilkitzkii: physiological, morphological and ecological studies. Berichte zur Polar-und Meeresforschung (Reports Polar Mar. Research) 405, 1–74.
Auel H., Harjes M., Da Rocha R., Stübing D., Hagen W. (2002). Lipid biomarkers indicate different ecological niches and trophic relationships of the Arctic hyperiid amphipods Themisto abyssorum and T. libellula. Polar Biol. 25 (5), 374–383. doi: 10.1007/s00300-001-0354-7
Balazy P., Anderson M. J., Chelchowski M., Włodarska-Kowalczuk M., Kuklinski P., Berge J. (2021). Shallow-water scavengers of polar night and day – An arctic time-lapse photography study. Front. Mar. Sci. 8. doi: 10.3389/fmars.2021.656772
Beermann J., Boos K., Gutow L., Boersma M., Peralta A. C. (2018). Combined effects of predator cues and competition define habitat choice and food consumption of amphipod mesograzers. Oecologia 186, 645–654. doi: 10.1007/s00442-017-4056-4
Berge J., Daase M., Renaud P. E., Ambrose W. G., Darnis G., Last K. S., et al. (2015). Unexpected levels of biological activity during the polar night offer new perspectives on a warming Arctic. Curr. Biol. 25 (19), 2555–2561. doi: 10.1016/j.cub.2015.08.024
Birula A. A. (1897). Recherches sur la biologie et zoogéographie principalement des mers russes: 2. Hydrozoa, Polychaeta et Crustacea, etc. Annuaire Musée Zoologique l‘Académie Imperiale Des. Sci. St.-Petersbourg 2, 78–116.
Bischof K., Convey P., Duarte P., Gattuso J.-P., Granberg M., Hop H., et al. (2019). “Kongsfjorden as harbinger of the future Arctic: knowns, unknowns and research priorities,” in The ecosystem of Kongsfjorden, Svalbard. Eds. Hop H., Wiencke C. (Springer Nature Switzerland AG), 537–562. doi: 10.1007/978-3-319-46425-1_14
Boyer F., Mercier C., Bonin A., Le Bras Y., Taberlet P., Coissac E. (2016). obitools: A unix-inspired software package for DNA metabarcoding. Mol. Ecol. Resour. 16 (1), 176–182. doi: 10.1111/1755-0998.12428
Britton J. (1994). Marine carrion and scavengers. Oceanography Mar. Biology: Annu. Rev. 32, 369–434.
Buchner D., Leese F. (2020). BOLDigger–a Python package to identify and organise sequences with the Barcode of Life Data systems. Metabarcoding Metagenomics 4, e53535. doi: 10.3897/mbmg.4.53535
Carmack E., Polyakov I., Padman L., Fer I., Hunke E., Hutchings J., et al. (2015). Toward quantifying the increasing role of oceanic heat in sea ice loss in the new Arctic. Bull. Am. Meteorological Soc. 96 (12), 2079–2105. doi: 10.1175/BAMS-D-13-00177.1
Chelsky A., Pitt K. A., Ferguson A. J., Bennett W. W., Teasdale P. R., Welsh D. T. (2016). Decomposition of jellyfish carrion in situ: Short-term impacts on infauna, benthic nutrient fluxes and sediment redox conditions. Sci. Total Environ. 566, 929–937. doi: 10.1016/j.scitotenv.2016.05.011
Cisek M., Makuch P., Petelski T. (2017). Comparison of meteorological conditions in Svalbard fjords: Hornsund and Kongsfjorden. Oceanologia 59 (4), 413–421. doi: 10.1016/j.oceano.2017.06.004
Condon R. H., Norman M. (1999). Commensal associations between the hyperiid amphipod, Themisto australis, and the scyphozoan jellyfish, Cyanea capillata. Mar. Freshw. Behav. Physiol. 32 (4), 261–267. doi: 10.1080/10236249909379054
Connell J. H. (1983). On the prevalence and relative importance of interspecific competition: evidence from field experiments. Am. Nat. 122 (5), 661–696. doi: 10.1086/284165
Cottier F., Porter M. (2020). “The marine physical environment during the polar night,” in POLAR NIGHT marine ecology: life and light in the dead of night. Eds. Berge J., Johnsen G., Cohen &J. (Cham: Springer), 17–36). doi: 10.1007/978-3-030-33208-2_2
Dementieva T. (1931). On the variability of the Amphipoda of the northern seas. Akademiia Nauk SSSR Trudy Okeanolografii Instituta 1, 65–82.
De Rovere F., Langone L., Schroeder K., Miserocchi S., Giglio F., Aliani S., et al. (2022). Water masses variability in inner Kongsfjorden (Svalbard) during 2010–2020. Front. Mar. Sci. 9. doi: 10.3389/fmars.2022.741075
Dischereit A., Wangensteen O. S., Præbel K., Auel H., Havermans C. (2022). Using DNA metabarcoding to characterize the prey spectrum of two co-occurring Themisto amphipods in the rapidly changing atlantic-arctic gateway Fram Strait. Genes 13 (11), 2035. doi: 10.3390/genes13112035
Doyle T. K., Houghton J. D., McDevitt R., Davenport J., Hays G. C. (2007). The energy density of jellyfish: estimates from bomb-calorimetry and proximate-composition. J. Exp. Mar. Biol. Ecol. 343 (2), 239–252. doi: 10.1016/j.jembe.2006.12.010
Duggins D., Eckman J. (1997). Is kelp detritus a good food for suspension feeders? Effects of kelp species, age and secondary metabolites. Mar. Biol. 128, 489–495. doi: 10.1007/s002270050115
Dunlop K. M., Jones D. O., Sweetman A. K. (2017a). Direct evidence of an efficient energy transfer pathway from jellyfish carcasses to a commercially important deep-water species. Sci. Rep. 7 (1), 1–4. doi: 10.1038/s41598-017-17557-x
Dunlop K. M., Jones D. O., Sweetman A. K. (2017b). Scavenging processes on jellyfish carcasses across a fjord depth gradient. Limnology Oceanography 63 (3), 1146–1155. doi: 10.1002/lno.10760
Dunn O. J. (1961). Multiple comparisons among means. J. Am. Stat. Assoc. 56 (293), 52–64. doi: 10.1080/01621459.1961.10482090
Eriksen E., Prokhorova T., Johannesen E. (2012). Long term changes in abundance and spatial distribution of pelagic Agonidae, Ammodytidae, Liparidae, Cottidae, Myctophidae and Stichaeidae in the Barents Sea. Diversity Ecosyst., 109–126. doi: 10.5772/36549
Fleming N. E., Harrod C., Griffin D. C., Newton J., Houghton J. D. (2014). Scyphozoan jellyfish provide short-term reproductive habitat for hyperiid amphipods in a temperate near-shore environment. Mar. Ecol. Prog. Ser. 510, 229–240. doi: 10.3354/meps10896
Frøslev T. G., Kjøller R., Bruun H. H., Ejrnæs R., Brunbjerg A. K., Pietroni C., et al. (2017). Algorithm for post-clustering curation of DNA amplicon data yields reliable biodiversity estimates. Nat. Commun. 8 (1), 1188. doi: 10.1038/s41467-017-01312-x
Geller J., Meyer C., Parker M., Hawk H. (2013). Redesign of PCR primers for mitochondrial cytochrome c oxidase subunit I for marine invertebrates and application in all-taxa biotic surveys. Mol. Ecol. Resour. 13 (5), 851–861. doi: 10.1111/1755-0998.12138
Geoffroy M., Berge J., Majaneva S., Johnsen G., Langbehn T. J., Cottier F., et al. (2018). Increased occurrence of the jellyfish Periphylla periphylla in the European high Arctic. Polar Biol. 41 (12), 2615–2619. doi: 10.1007/s00300-018-2368-4
Grabowski M., Jabłońska A., Weydmann-Zwolicka A., Gantsevich M., Strelkov P., Skazina M., et al. (2019). Contrasting molecular diversity and demography patterns in two intertidal amphipod crustaceans reflect Atlantification of High Arctic. Mar. Biol. 166 (12), 155. doi: 10.1007/s00227-019-3603-4
Günther B., Fromentin J.-M., Metral L., Arnaud-Haond S. (2021). Metabarcoding confirms the opportunistic foraging behaviour of Atlantic bluefin tuna and reveals the importance of gelatinous prey. PeerJ 9, e11757. doi: 10.7717/peerj.11757
Hagen W. (1999). Reproductive strategies and energetic adaptations of polar zooplankton. Invertebrate Reprod. Dev. 36 (1-3), 25–34. doi: 10.1080/07924259.1999.9652674
Hagen W., Auel H. (2001). Seasonal adaptations and the role of lipids in oceanic zooplankton. Zoology 104 (3-4), 313–326. doi: 10.1078/0944-2006-00037
Hamilton G. (2016). The secret lives of jellyfish: Long regarded as minor players in ocean ecology, jellyfish are actually important parts of the marine food web. Nature 531 (7595), 432–435.
Havermans C., Auel H., Hagen W., Held C., Ensor N. S., Tarling G. A. (2019). Predatory zooplankton on the move: Themisto amphipods in high-latitude marine pelagic food webs. Adv. Mar. Biol. 82, 51–92. doi: 10.1016/bs.amb.2019.02.002
Havermans C., Murray A., Dischereit A. (2023). Presence data of gelatinous zooplankton in the Polar Night in Kongsfjorden, Svalbard, in 2022 PANGAEA. doi: 10.1594/PANGAEA.955899
Havermans C., Smetacek V. (2018). Bottom-up and top-down triggers of diversification: A new look at the evolutionary ecology of scavenging amphipods in the deep sea. Prog. Oceanography 164, 37–51. doi: 10.1016/j.pocean.2018.04.008
Hays G. C., Doyle T. K., Houghton J. D. (2018). A paradigm shift in the trophic importance of jellyfish? Trends Ecol. Evol. 33 (11), 874–884. doi: 10.1016/j.tree.2018.09.001
Holst S., Jarms G. (2007). Substrate choice and settlement preferences of planula larvae of five Scyphozoa (Cnidaria) from German Bight, North Sea. Mar. Biol. 151, 863–871. doi: 10.1007/s00227-006-0530-y
Hop H., Pearson T., Hegseth E. N., Kovacs K. M., Wiencke C., Kwasniewski S., et al. (2002). The marine ecosystem of Kongsfjorden, Svalbard. Polar Res. 21 (1), 167–208. doi: 10.3402/polar.v21i1.6480
Jonne K., Helen O.-K., Tiina P., Ilmar K., Henn K. (2006). Seasonal changes in situ grazing of the mesoherbivores Idotea baltica and Gammarus oceanicus on the brown algae Fucus vesiculosus and Pylaiella littoralis in the central Gulf of Finland, Baltic Sea. Hydrobiologia 554, 117–125. doi: 10.1007/s10750-005-1011-x
Krøyer H. (1838). Grönlands amfipoder det konigelige danske videnskabernes selskabs naturvidenskabelige og mathematiske afhandlinger. 4.
Kunisch E. H., Graeve M., Gradinger R., Flores H., Varpe Ø., Bluhm B. A. (2023). What we do in the dark: Prevalence of omnivorous feeding activity in Arctic zooplankton during polar night. Limnology Oceanography 68, 1835–1851. doi: 10.1002/lno.12389
Lassen S., Helmholz H., Ruhnau C., Prange A. (2011). A novel proteinaceous cytotoxin from the northern Scyphozoa Cyanea capillata (L.) with structural homology to cubozoan haemolysins. Toxicon 57 (5), 721–729. doi: 10.1016/j.toxicon.2011.02.004
Lebrato M., Pitt K. A., Sweetman A. K., Jones D. O., Cartes J. E., Oschlies A., et al. (2012). Jelly-falls historic and recent observations: a review to drive future research directions. Hydrobiologia 690, 227–245. doi: 10.1007/s10750-012-1046-8
Legeżyńska J. (2001). Distribution patterns and feeding strategies of lysianassoid amphipods in shallow waters of an Arctic fjord. Polish Polar Res. 22 (3-4), 173–186.
Legeżyńska J. (2008). Food resource partitioning among Arctic sublittoral lysianassoid amphipods in summer. Polar Biol. 31 (6), 663–670. doi: 10.1007/s00300-008-0404-5
Legeżyńska J., Kędra M., Walkusz W. (2012). When season does not matter: Summer and winter trophic ecology of Arctic amphipods. Hydrobiologia 684, 189–214. doi: 10.1007/s10750-011-0982-z
Legezynska J. (2002). Ekologia padlinozernych bezkregowcow w Arktycznym fiordzie.(Ecology of scavenging invertebrates in an Arctic fjord.) (Poland: University of Gdansk).
Lundesgaard Ø., Sundfjord A., Lind S., Nilsen F., Renner A. H. (2022). Import of Atlantic Water and sea ice controls the ocean environment in the northern Barents Sea. Ocean Sci. 18 (5), 1389–1418. doi: 10.5194/os-18-1389-2022
Mahé F., Rognes T., Quince C., De Vargas C., Dunthorn M. (2015). Swarm v2: highly-scalable and high-resolution amplicon clustering. PeerJ 3, e1420. doi: 10.7717/peerj.1420
Mańko M. K., Gluchowska M., Weydmann-Zwolicka A. (2020). Footprints of Atlantification in the vertical distribution and diversity of gelatinous zooplankton in the Fram Strait (Arctic Ocean). Prog. Oceanography 189, 102414. doi: 10.1016/j.pocean.2020.102414
Mańko M. K., Merchel M., Kwaśniewski S., Weydmann-Zwolicka A. (2022). Atlantification alters the reproduction of jellyfish Aglantha digitale in the European Arctic. Limnology Oceanography 67 (8), 1836–1849. doi: 10.1002/lno.12170
Nygård H., Berge J., Søreide J. E., Vihtakari M., Falk-Petersen S. (2012). The amphipod scavenging guild in two Arctic fjords: seasonal variations, abundance and trophic interactions. Aquat. Biol. 14 (3), 247–264. doi: 10.3354/ab00394
Oksanen J., Blanchet F. G., Friendly M., Kindt R., Legendre P., McGlinn D., et al. (2020) vegan: community ecology package. Available at: https://CRAN.R-project.org/package=vegan.
Oliver J. S., Slattery P. N. (1985). Destruction and opportunity on the sea floor: Effects of gray whale feeding. Ecology 66 (6), 1965–1975. doi: 10.2307/2937392
Pantiukhin D., Verhaegen G., Kraan C., Jerosch K., Neitzel P., Hoving H.-J. T., et al. (2023). Optical observations and spatio-temporal projections of gelatinous zooplankton in the Fram Strait, a gateway to a changing Arctic Ocean. Front. Mar. Sci. 10. doi: 10.3389/fmars.2023.987700
Piepenburg D. (2005). Recent research on Arctic benthos: Common notions need to be revised. Polar Biol. 28 (10), 733–755. doi: 10.1007/s00300-005-0013-5
Piskozub J. (2017). Svalbard as a study model of future High Arctic coastal environments in a warming world. Oceanologia 59 (4), 612–619. doi: 10.1016/j.oceano.2017.06.005
Poltermann M. (2001). Arctic sea ice as feeding ground for amphipods–food sources and strategies. Polar Biol. 24, 89–96. doi: 10.1007/s003000000177
Polyakov I. V., Pnyushkov A. V., Alkire M. B., Ashik I. M., Baumann T. M., Carmack E. C., et al. (2017). Greater role for Atlantic inflows on sea-ice loss in the Eurasian Basin of the Arctic Ocean. Science 356 (6335), 285–291. doi: 10.1126/science.aai8204
Polyakov I. V., Walsh J. E., Kwok R. (2012). Recent changes of Arctic multiyear sea ice coverage and the likely causes. Bull. Am. Meteorological Soc. 93 (2), 145–151. doi: 10.1175/BAMS-D-11-00070.1
Ponton D., Gagné J., Fortier L. (1993). Production and dispersion of freshwater, anadromous, and marine fish larvae in and around a river plume in subarctic Hudson Bay, Canada. Polar Biol. 13, 321–331. doi: 10.1007/BF00238359
Promińska A., Cisek M., Walczowski W. (2017). Kongsfjorden and Hornsund hydrography–comparative study based on a multiyear survey in fjords of west Spitsbergen. Oceanologia 59 (4), 397–412. doi: 10.1016/j.oceano.2017.07.003
Purcell J. E., Juhl A. R., Mańko M. K., Aumack C. F. (2018). Overwintering of gelatinous zooplankton in the coastal Arctic Ocean. Mar. Ecol. Prog. Ser. 591, 281–286. doi: 10.3354/meps12289
Quijón P. A., Kelly M. C., Snelgrove P. V. (2008). The role of sinking phytodetritus in structuring shallow-water benthic communities. J. Exp. Mar. Biol. Ecol. 366 (1-2), 134–145. doi: 10.1016/j.jembe.2008.07.017
Rantanen M., Karpechko A. Y., Lipponen A., Nordling K., Hyvärinen O., Ruosteenoja K., et al. (2022). The Arctic has warmed nearly four times faster than the globe since 1979. Commun. Earth Environ. 3 (1), 168. doi: 10.1038/s43247-022-00498-3
R Core Team. (2021) R: A language and environment for statistical computing. Available at: https://www.R-project.org/.
Richardson A. J., Bakun A., Hays G. C., Gibbons M. J. (2009). The jellyfish joyride: causes, consequences and management responses to a more gelatinous future. Trends Ecol. Evol. 24 (6), 312–322. doi: 10.1016/j.tree.2009.01.010
Rodríguez-Barreras R., Godoy-Vitorino F., Præbel K., Wangensteen O. S. (2020). DNA metabarcoding unveils niche overlapping and competition among Caribbean sea urchins. Regional Stud. Mar. Sci. 40, 101537. doi: 10.1016/j.rsma.2020.101537
Rognes T., Flouri T., Nichols B., Quince C., Mahé F. (2016). VSEARCH: A versatile open source tool for metagenomics. PeerJ 4, e2584. doi: 10.7717/peerj.2584
Sainte-Marie B. (1984). Morphological adaptations for carrion feeding in four species of littoral or circalittoral lysianassid amphipods. Can. J. Zoology 62 (9), 1668–1674. doi: 10.1139/z84-244
Sainte-Marie B. (1986). Feeding and swimming of lysianassid amphipods in a shallow cold-water bay. Mar. Biol. 91, 219–229. doi: 10.1007/BF00569437
Sainte-Marie B., Hargrave B. (1987). Estimation of scavenger abundance and distance of attraction to bait. Mar. Biol. 94, 431–443. doi: 10.1007/BF00428250
Sainte-Marie B., Lamarche G. (1985). The diets of six species of the carrion-feeding lysianassid amphipod genus Anonyx and their relation with morphology and swimming behaviour. Sarsia 70 (2-3), 119–126. doi: 10.1080/00364827.1985.10420624
Schaub J., Hunt B. P., Pakhomov E. A., Holmes K., Lu Y., Quayle L. (2018). Using unmanned aerial vehicles (UAVs) to measure jellyfish aggregations. Mar. Ecol. Prog. Ser. 591, 29–36. doi: 10.3354/meps12414
Scheer S. L., Sweetman A., Piatkowski U., Rohlfer E. K., Hoving H.-J. T. (2022). Food fall-specific scavenging response to experimental medium-sized carcasses in the deep sea. Mar. Ecol. Prog. Ser. 685, 31–48. doi: 10.3354/meps13973
Schoener T. W. (1974). Resource partitioning in ecological communities: Research on how similar species divide resources helps reveal the natural regulation of species diversity. Science 185 (4145), 27–39. doi: 10.1126/science.185.4145.27
Schoener T. W. (1986). “Resource partitioning,” in Community ecology: pattern and process. Eds. Kikkawa J., Andersen D. (Blackwell Scientific Publications).
Schröter F., Havermans C., Kraft A., Knüppel N., Beszczynska-Möller A., Bauerfeind E., et al. (2019). Pelagic amphipods in the Eastern Fram Strait with continuing presence of Themisto compressa based on sediment trap time series. Front. Mar. Sci. 6. doi: 10.3389/fmars.2019.00311
Seefeldt M. A., Campana G. L., Deregibus D., Quartino M. L., Abele D., Tollrian R., et al. (2017). Different feeding strategies in Antarctic scavenging amphipods and their implications for colonisation success in times of retreating glaciers. Front. Zoology 14 (1), 1–15. doi: 10.1186/s12983-017-0248-3
Segerstråle S. G. (1947). New observations on the distribution and morphology of the amphipod, Gammarus zaddachi Sexton, with notes on related species. J. Mar. Biol. Assoc. United kingdom 27 (1), 219–244. doi: 10.1017/S002531540001417X
Shanks A. L., Graham W. M. (1988). Chemical defense in a scyphomedusa. Mar. Ecology-Progress Ser. 45, 81–86. doi: 10.3354/meps045081
Siegenthaler A., Wangensteen O. S., Benvenuto C., Campos J., Mariani S. (2019). DNA metabarcoding unveils multiscale trophic variation in a widespread coastal opportunist. Mol. Ecol. 28 (2), 232–249. doi: 10.1111/mec.14886
Smetacek V., Nicol S. (2005). Polar ocean ecosystems in a changing world. Nature 437 (7057), 362–368. doi: 10.1038/nature04161
Steele D. H., Brunel P. (1968). Amphipoda of the atlantic and arctic coasts of North America: anonyx (Lysianassidae). J. Fisheries Board Canada 25 (5), 943–1060. doi: 10.1139/f68-087
Stenvers V., Chi X., Javidpour J. (2020). Seasonal variability of the fatty acid composition in Aurelia aurita (Cnidaria: Scyphozoa): implications for gelativore food web studies. J. Plankton Res. 42 (4), 440–452. doi: 10.1093/plankt/fbaa026
Sweetman A. K., Chapman A. (2015). First assessment of flux rates of jellyfish carcasses (jelly-falls) to the benthos reveals the importance of gelatinous material for biological C-cycling in jellyfish-dominated ecosystems. Front. Mar. Sci. 2. doi: 10.3389/fmars.2015.00047
Sweetman A. K., Chelsky A., Pitt K. A., Andrade H., van Oevelen D., Renaud P. E. (2016). Jellyfish decomposition at the seafloor rapidly alters biogeochemical cycling and carbon flow through benthic food-webs. Limnology Oceanography 61 (4), 1449–1461. doi: 10.1002/lno.10310
Sweetman A. K., Smith C. R., Dale T., Jones D. O. (2014). Rapid scavenging of jellyfish carcasses reveals the importance of gelatinous material to deep-sea food webs. Proc. R. Soc. B: Biol. Sci. 281 (1796), 20142210. doi: 10.1098/rspb.2014.2210
Tarling G. A., Freer J. J., Banas N. S., Belcher A., Blackwell M., Castellani C., et al. (2022). Can a key boreal Calanus copepod species now complete its life-cycle in the Arctic? Evidence and implications for Arctic food-webs. Ambio 51, 333–344. doi: 10.1007/s13280-021-01667-y
Tverberg V., Skogseth R., Cottier F., Sundfjord A., Walczowski W., Inall M. E., et al. (2019). “The Kongsfjorden transect: seasonal and inter-annual variability in hydrography,” in The ecosystem of Kongsfjorden, Svalbard. Eds. Hop H., Wiencke C. (Cham: Springer), 49–104. doi: 10.1007/978-3-319-46425-1_3
Urban P., Præbel K., Bhat S., Dierking J., Wangensteen O. S. (2022). DNA metabarcoding reveals the importance of gelatinous zooplankton in the diet of Pandalus borealis, a keystone species in the Arctic. Mol. Ecol. 31 (5), 1562–1576. doi: 10.1111/mec.16332
Vader W., Tandberg A. H. S. (2019). Gammarid amphipods (Crustacea) in Norway, with a key to the species. Fauna norvegica 39, 12–25. doi: 10.5324/fn.v39i0.2873
Verity P. G., Smetacek V. (1996). Organism life cycles, predation, and the structure of marine pelagic ecosystems. Mar. Ecol. Prog. Ser. 130, 277–293. doi: 10.3354/meps130277
Węsławski J. M., Dragańska-Deja K., Legeżyńska J., Walczowski W. (2018). Range extension of a boreal amphipod Gammarus oceanicus in the warming Arctic. Ecol. Evol. 8 (15), 7624–7632. doi: 10.1002/ece3.4281
Węsławski J. M., Kotwicki L. (2018). Macro-plastic litter, a new vector for boreal species dispersal on Svalbard. Polish Polar Res. 39 (1), 165–174. doi: 10.24425/118743
Węsławski J. M., Legeżyńska J. (2002). Life cycles of some Arctic amphipods. Polish Polar Res. 23, 253–264.
Węsławski J. M., Legeżyńska J., Kotwicki L., Mazurkiewicz M., Olenin S. (2021). Gammarus (Amphipoda) species competitive exclusion or coexistence as a result of climate change in the Arctic? Polish Polar Res. 42, 287–302. doi: 10.24425/ppr.2021.138586
Wakabayashi K., Sato H., Yoshie-Stark Y., Ogushi M., Tanaka Y. (2016). Differences in the biochemical compositions of two dietary jellyfish species and their effects on the growth and survival of Ibacus novemdentatus phyllosomas. Aquaculture Nutr. 22 (1), 25–33. doi: 10.1111/anu.12228
Wang Q., Wekerle C., Wang X., Danilov S., Koldunov N., Sein D., et al. (2020). Intensification of the Atlantic Water supply to the Arctic Ocean through Fram Strait induced by Arctic sea ice decline. Geophysical Res. Lett. 47 (3), e2019GL086682. doi: 10.1029/2019GL086682
Wangensteen O. S., Palacín C., Guardiola M., Turon X. (2018). DNA metabarcoding of littoral hard-bottom communities: high diversity and database gaps revealed by two molecular markers. PeerJ 6, e4705. doi: 10.7717/peerj.4705
Wangensteen O., Turon X. (2017). “Metabarcoding techniques for assessing biodiversity of marine animal forests,” in Marine animal forests. The ecology of benthic biodiversity hotspots. Eds. Rossi S., Bramanti L., Gori A., Orejas C. (Springer), 445–473. doi: 10.1007/978-3-319-17001-5_53-1
Wassmann P., Duarte C. M., Agusti S., Sejr M. K. (2011). Footprints of climate change in the Arctic marine ecosystem. Global Change Biol. 17 (2), 1235–1249. doi: 10.1111/j.1365-2486.2010.02311.x
Werner I., Auel H. (2005). Seasonal variability in abundance, respiration and lipid composition of Arctic under-ice amphipods. Mar. Ecol. Prog. Ser. 292, 251–262. doi: 10.3354/meps292251
Werner I., Auel H., Kiko R. (2004). Occurrence of Anonyx sarsi (Amphipoda: Lysianassoidea) below Arctic pack ice: an example for cryo-benthic coupling? Polar Biol. 27, 474–481. doi: 10.1007/s00300-004-0616-2
Keywords: Gammarus, Anonyx, Orchomenella, Kongsfjorden, Svalbard, Arctic ocean, gelatinous zooplankton, jelly-falls
Citation: Dischereit A, Beermann J, Lebreton B, Wangensteen OS, Neuhaus S and Havermans C (2024) DNA metabarcoding reveals a diverse, omnivorous diet of Arctic amphipods during the polar night, with jellyfish and fish as major prey. Front. Mar. Sci. 11:1327650. doi: 10.3389/fmars.2024.1327650
Received: 26 October 2023; Accepted: 09 January 2024;
Published: 14 February 2024.
Edited by:
Jean-Pierre Desforges, University of Winnipeg, CanadaReviewed by:
Erin Kunisch, Norwegian University of Science and Technology, NorwayCopyright © 2024 Dischereit, Beermann, Lebreton, Wangensteen, Neuhaus and Havermans. This is an open-access article distributed under the terms of the Creative Commons Attribution License (CC BY). The use, distribution or reproduction in other forums is permitted, provided the original author(s) and the copyright owner(s) are credited and that the original publication in this journal is cited, in accordance with accepted academic practice. No use, distribution or reproduction is permitted which does not comply with these terms.
*Correspondence: Annkathrin Dischereit, YW5ua2F0aHJpbi5kaXNjaGVyZWl0QGF3aS5kZQ==
Disclaimer: All claims expressed in this article are solely those of the authors and do not necessarily represent those of their affiliated organizations, or those of the publisher, the editors and the reviewers. Any product that may be evaluated in this article or claim that may be made by its manufacturer is not guaranteed or endorsed by the publisher.
Research integrity at Frontiers
Learn more about the work of our research integrity team to safeguard the quality of each article we publish.