- 1Coral Reef Research Center of China, Guangxi Laboratory on the Study of Coral Reefs in the South China Sea, School of Marine Sciences, Guangxi University, Nanning, China
- 2Southern Marine Science and Engineering Guangdong Laboratory (Guangzhou), Guangzhou, China
Relatively high-latitude coral reefs could be potential “refuges” for corals under climate change. One of the most important aspects limiting their availability as refuges is low-temperature stress. However, the mechanisms underlying the response of coral holobionts to low-temperature stress is unclear. In this study, we aimed to explore the underlying mechanisms by recording the maximum quantum yields of photosystem II (Fv/Fm) and transcriptome responses of Porites lutea under acute (1–2 weeks) and chronic (6–12 weeks) low-temperature stress at 20°C and 14°C. The P. lutea samples were collected from a relatively high-latitude coral reef in the South China Sea (109°00′–109°15′E and 21°00′–21°10′ N). The study suggested that: (1) Under acute low-temperature stress, the Fv/Fm of Symbiodiniaceae dropped by 64%, which was significantly higher than the 49% observed under chronic stress. Low-temperature stress inhibited photosystem II(PSII) functioning, with greater inhibition under acute stress. (2) Downregulation of sugar metabolism-related genes under low-temperature stress implied that the decrease in energy was due to obstruction of PSII. (3) Under low-temperature stress, calcification-related genes were downregulated in coral hosts, possibly because of energy deprivation caused by inhibited photosynthesis, Symbiodiniaceae expulsion, and oxidative phosphorylation uncoupling in mitochondria. (4) Acute low-temperature stress induced the upregulation of genes related to the TNF signaling pathway and endoplasmic reticulum stress, promoting apoptosis and coral bleaching. However, these phenomena were not observed during chronic stress, suggesting acclimation to chronic low-temperature stress and a greater survival pressure of acute low-temperature stress on coral holobionts. In conclusion, low-temperature stress inhibits Symbiodiniaceae PSII functioning, reducing energy production and affecting calcification in coral holobionts. Acute low-temperature stress is more threatening to coral holobionts than chronic stress.
1 Introduction
Coral reef ecosystems rank among the most important global ecosystems, with high levels of biodiversity and primary productivity (Moberg and Folke, 1999). In recent years, climate change and anthropogenic stressors have precipitated degradation of coral reefs. Many coral species are on the verge of extinction (Carpenter et al., 2008; Heron et al., 2016; Hughes et al., 2017). Anomalies in sea surface temperature (SST) have led to extensive coral bleaching events, and elevated SST is the main cause of coral bleaching (McGowan and Theobald, 2017; Karnauskas, 2020). High-latitude reefs are postulated to serve as thermal refuges for corals (Greenstein and Pandolfi, 2008; Beger et al., 2014; Camp et al., 2018). However, modern ecological surveys and geological evidence suggest that low-temperature stress is an important factor limiting the survival of corals in high-latitude coral reefs (Yu et al., 2004; Sommer et al., 2018; Tuckett and Wernberg, 2018).
In high-latitude reefs, coral bleaching may be induced by low winter temperatures and acute SST declines due to upwelling, tidal activities, and tropical cyclones (Carrigan and Puotinen, 2014; Rayson et al., 2015; Schlegel et al., 2017; Rayson et al., 2018; Green et al., 2019; Riegl et al., 2019). For example, a severe cold spell in January 1981 caused coral bleaching in Florida (Schlegel et al., 2021). Under low-temperature stress, the photosystem II (PSII) of Symbiodiniaceae is damaged, resulting in the accumulation of reactive oxygen species (ROS) in tissues (Tracey et al., 2003; Marangoni et al., 2021). Excess ROS causes tissue damage and the expulsion of Symbiodiniaceae from corals, ultimately leading to the bleaching and death of coral holobionts (Jones et al., 1998; Tracey et al., 2003; Thornhill et al., 2008; Marangoni et al., 2021). RNA-seq analyses revealed that coral hosts modulate immunity, apoptosis, and autophagy while suppressing metabolic activity under low-temperature stress (Wuitchik et al., 2021; Huang et al., 2022). However, the responses of coral holobionts to temp perturbations seem to vary with changes in the stress intensity, rate, and duration (Evensen et al., 2021). The density and photosynthetic rate of Symbiodiniaceae show a lesser decrease under acute low-temperature stress (5 h) than under chronic stress (2 weeks) (Marangoni et al., 2021). Furthermore, previous research demonstrated inhibition of photosynthesis at the onset of low-temperature stress, and coral holobionts gradually adapted to the conditions, with chlorophyll a content and Symbiodiniaceae density gradually restored within 2–3 weeks (Roth et al., 2012).
It’s imperative to study the response of coral holobionts to low temperatures, though the underlying mechanisms are still unclear. We aimed to explore these mechanisms by investigating the changes in maximum quantum yields in photosystem II (Fv/Fm) and transcriptional responses under acute and chronic low-temperature stress in Porites lutea. Weizhou Island (WI) located at 109°00′–109°15′E and 21°00′–21°10′ N, is considered a potential refuge for corals in the South China Sea during global warming. The annual mean SST on WI is 24.6°C; the lowest monthly mean SST in winter is 17.3°C, varying from 14.7°C to 20°C, and the highest monthly mean SST in summer is 30.4°C, varying from 29.5°C to 31.1°C (Yu and Jiang, 2004; Chen et al., 2013). WI was subjected to extreme low-temperature events in 1984, 1985, 1989 and 2008,with lowest monthly mean SST as low as 14.7°C, 16°C, 15.5°C and 14.4°C, respectively (Chen et al., 2009; Chen et al., 2013). The corals of WI might be threatened by low temperatures (Southward et al., 1995; Yu et al., 2019). P. lutea is a dominant coral species around WI, and its principal symbiotic Symbiodiniaceae species is Cladocopium C15 (Chen et al., 2019; Yu et al., 2019). Exploring the response mechanisms of P. lutea to acute and chronic low temperatures will further our understanding of the cold tolerance mechanisms of coral holobionts around WI.
2 Materials and methods
2.1 Coral sampling and experimental design
Three colonies of P. lutea were collected from the WI reef in September 2021 at a 3–5 m water depth. Three colonies were randomly assigned to each treatment. The samples were cultured in an indoor aquarium for 30 days prior to acute and chronic temperature stress experiments. Experiments utilized temperature gradients of 26°C, 20°C, and 14°C, with an initial temperature of 26°C. The lowest temperatures are in reference to the extreme low temperatures in WI(Chen et al., 2009; Chen et al., 2013). The sample were taken in the beginning of temperature decrease as the control treatment. In acute stress treatment, the temperature decreased by one gradient weekly, while in the chronic stress treatment, the temperature reduced by 1°C weekly (Figure 1). Three biological replicates were performed for each temperature gradient.
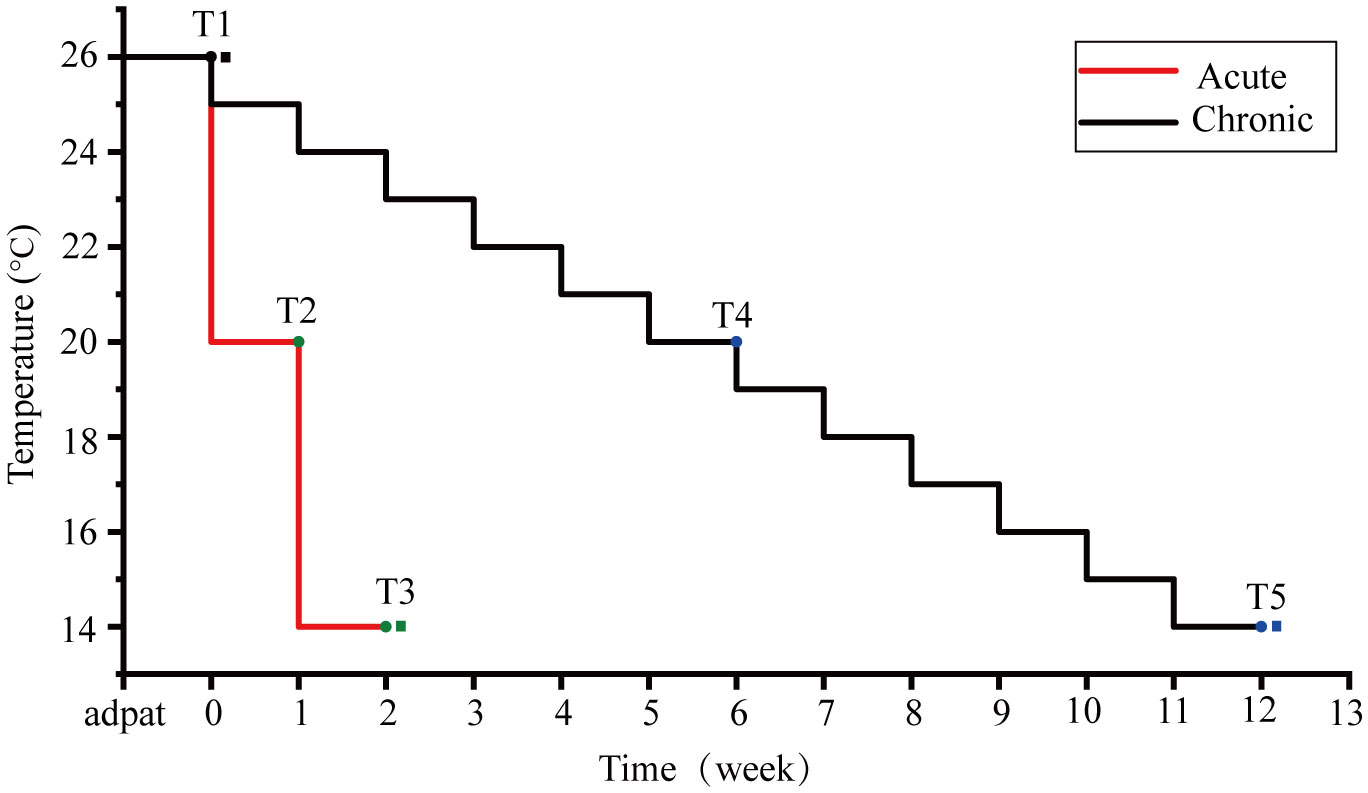
Figure 1 Temperature settings for acute and chronic stress groups. The red line represents the temperature change in the acute stress group, and the black line represents the temperature change in the chronic stress group. T1 is the control group, T2 and T3 are timepoints of the acute stress group, and T4 and T5 are timepoints the chronic stress group. Circles are the maximum quantum yields of photosystem II (Fv/Fm) measurement points and squares are transcriptome sampling points.
2.2 Symbiodiniaceae Fv/Fm determination
The Fv/Fm of Symbiodiniaceae was determined in the acute and chronic stress groups with different temperature gradients. The Fv/Fm of the Symbiodiniaceae was measured using a portable pulse amplitude modulation fluorometer (Diving-PAM) (Ralph et al., 1999). The coral holobionts were dark-adapted for 30 min before the Fv/Fm measurements. The fibreoptic cable was maintained approximately 1 mm from the coral surface. Kruskal–Wallis analysis was performed on the Fv/Fm data based on different temperature gradients for different stress groups.
We computed the hypothermic critical threshold Fv/Fm measurements (Evensen et al., 2021), which were fitted to log-logistic dose–response curves using the “drc” package in R (Ritz et al., 2016), with model selection based on Akaike’s Information Criterion and individual curves fit for each experiment. From these, an ED50 parameter (effective dose 50) was obtained for each experiment, representing the x-value at the inflection point of the curve (in this case the temperature) where Fv/Fm values in the model fit were 50% lower than the starting values of the model. This provided a quantitative thermal threshold, designated as the Fv/FmED50, for P. lutea in each experiment (Evensen et al., 2021). All code used in the statistical analyses is available in the Supplementary Material.
2.3 RNA-seq analyses
Transcriptomic analyses were performed on control samples (maintained at 26°C) and those exposed to extreme low-temperature stress (14°C). Coral samples were washed with sterile water, and approximately 50 mg of each was excised using forceps, placed into lyophilization tubes, and promptly snap-frozen in liquid nitrogen. Library construction was performed using the Illumina TruSeq RNA Sample Prep Kit.Total RNA was extracted from the coral samples using TRIzol® Reagent. Then RNA quality was determined by 5300 Bioanalyser (Agilent) and quantified using the ND-2000 (NanoDrop Technologies). mRNA was isolated from the total RNA using magnetic bead-labeled oligonucleotides (dT) and was used as a template to synthesize cDNA strands via reverse transcription using reverse transcriptase. cDNA was blunt-ended by adding End Repair Mix, followed by the addition of an “A” base at the 3′ end to prepare the RNA sequencing library. All mRNAs transcribed from the samples were sequenced using an Illumina NovaSeq 6000 sequencing platform. The raw sequences were deposited in the NCBI Sequence Read Archive (SRA) database (accession number: PRJNA1026091).
The base distribution and quality fluctuations of sequenced sequences were counted using fastp to meet the criteria of Q30 > 80% for base quality values and a sequencing error rate of < 0.1% (Chen et al., 2018). After quality control, The remaining high-quality clean reads were mapped to the reference transcriptomes using HISAT2(Kim et al., 2015). Coral hosts and Symbiodiniaceae’s reference transcriptomes derived from http://plut.reefgenomics.org/. The Symbiodiniaceae were referred to as Cladocopium C15, and the coral hosts were referred to as P. lutea. The mapped reads of each sample were assembled by StringTi in a reference-based approach(Pertea et al., 2015).
To identify DEGs (differential expression genes) between two different treatment, the expression level of each transcript was calculated according to the transcripts per million reads (TPM) method. RSEM was used to quantify gene abundances(Li and Dewey, 2011). Essentially, differential expression analysis was performed using the DESeq2(Love et al., 2014). DEGs with |log2FC|≧1 and FDR≤ 0.05 were considered to be significantly different expressed genes. Two pairwise comparisons were designated in the DEG analyses:CK26 versus A14, CK26versus C14.
KEGG functional-enrichment analysis were performed to identify which DEGs were significantly enriched in pathways at Bonferroni-corrected P-value ≤0.05 compared with the whole-transcriptome background. KEGG pathway analysis were carried out by KOBAS (Xie et al., 2011). In addition, the expression of genes in the samples was subjected to principal component analysis (PCA) to explore the relationship between the samples and the magnitude of variation.
3 Results
3.1 The Fv/Fm variation of P. lutea-symbiotic Symbiodiniaceae under acute and chronic low-temperature stress
Acute and chronic low-temperature stress altered the appearance of corals. At 20°C, the color became lighter without reaching bleaching. At 14°C, the color was lighter than at 20°C, and in the acute stress group, there was localized pre-bleaching (Figure 2A). The Fv/Fm values decreased under acute and chronic low-temperature stress. At 26°C (CK), the Fv/Fm was 0.61 ± 0.03 (mean ± SD) and 0.60 ± 0.05 (mean ± SD) in the acute and chronic stress groups, respectively (Figure 2B; Supplementary Table S1). At 20°C, a decline in Fv/Fm was observed, but it was not significant (Kruskal–Wallis test, chronic: P = 0.077 acute: P = 0.085). At 14°C, both the acute and chronic stress groups exhibited significant Fv/Fm reduction compared to control (Kruskal–Wallis test, chronic: P < 0.001, acute: P< 0.001), registering values of 0.22 ± 0.03 (mean ± SD) and 0.31 ± 0.05 (mean ± SD), equating to 36% and 51% of the control values, respectively (Figure 2B; Supplementary Table S1). At 14°C, the Fv/Fm of the acute stress group was significantly lower (Kruskal–Wallis test, P = 0.001) than that of the chronic stress group (Figure 2B).
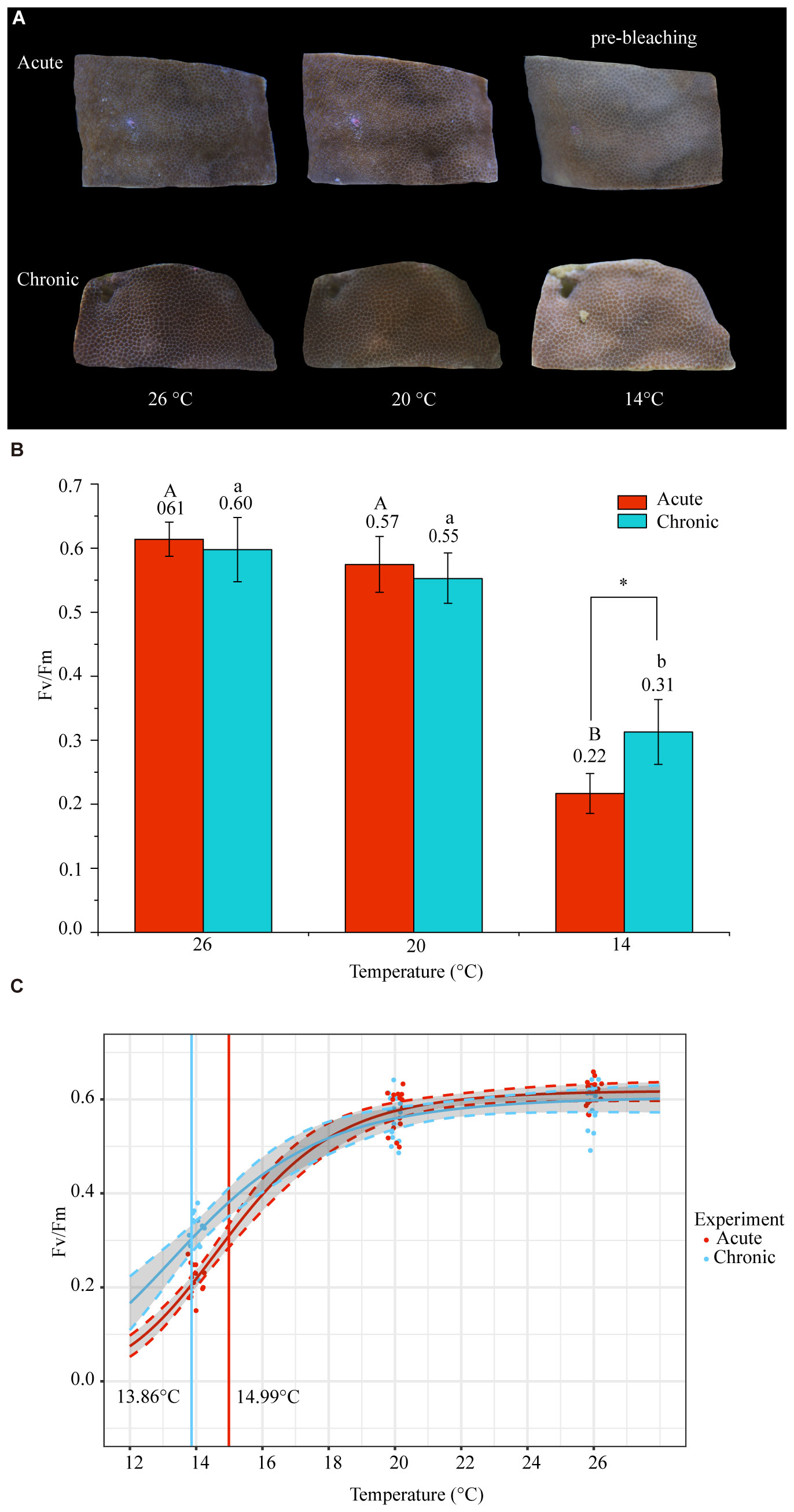
Figure 2 Changes in physiological indicators under acute and chronic low-temperature stress. (A) Appearance of corals during low-temperature stress. At 26°C, normal color was observed with extended tentacles; at 20°C, the color became lighter without reaching bleaching; at 14°C, the color became lighter than at 20°C, and in the acute stress group, there was localized pre-bleaching. (B) Maximum quantum yields of photosystem II (Fv/Fm) of P. lutea under different temperature conditions and during acute and chronic stress. ‘A’ and ‘a’ represent the differences between temperatures in the acute and chronic stress groups, respectively (Kruskal–Wallis test, P < 0.05). * represents significant differences (Kruskal–Wallis test, P < 0.05) between acute and chronic stresses at the same temperature. (C) Fv/Fm fit to log-logistic, dose-response curves (dashed lines indicate 95% confidence intervals). Curve fitting was used to determine Fv/FmED50 (effective dose 50).
The Fv/FmED50 represents the temperature at which 50% of the photosynthetic efficiency is lost relative to the baseline temperature. From Fv/FmED50, we found that the cold threshold was 14.99°C for acute stress and 13.86°C for chronic stress (Figure 2C). This indicates that PSII functioning in Symbiodiniaceae was inhibited by low-temperature stress at 14°C, whereby the acute stress group exhibited greater inhibition than the chronic stress group.
3.2 Transcriptional changes in P. lutea-symbiotic Symbiodiniaceae under acute and chronic low-temperature stress
Transcriptome analysis was performed on samples from the 26°C (CK), acute 14°C (A14), and chronic 14°C (C14) groups. The percentage of Q30 bases in clean data is above 93.2%. (Supplementary Table S2). The expression of Symbiodiniaceae genes was quantitatively analyzed using RSEM to obtain a quantitative index in terms of transcripts per million (TPM) (Supplementary Table S3). PCA showed that PC1 and PC2 accounted for 31.00% and 19.29% of the total variance, respectively (Figure 3A). Differential expression analysis between groups was performed using DESeq2. Compared with expression levels of the control group, the acute 14°C group had 487 DEGs, among which 200 genes were upregulated and 287 were downregulated; meanwhile, the chronic 14°C group had 2,566 DEGs, among which 1,041 were upregulated 1,525 were downregulated (Figures 3C, D; Supplementary Table S4). Of these, 299 DEGs were common to both groups, 188 were specific to the acute 14°C group, and 2,267 were specific to the chronic 14°C group (Figure 3B; Supplementary Table S4).
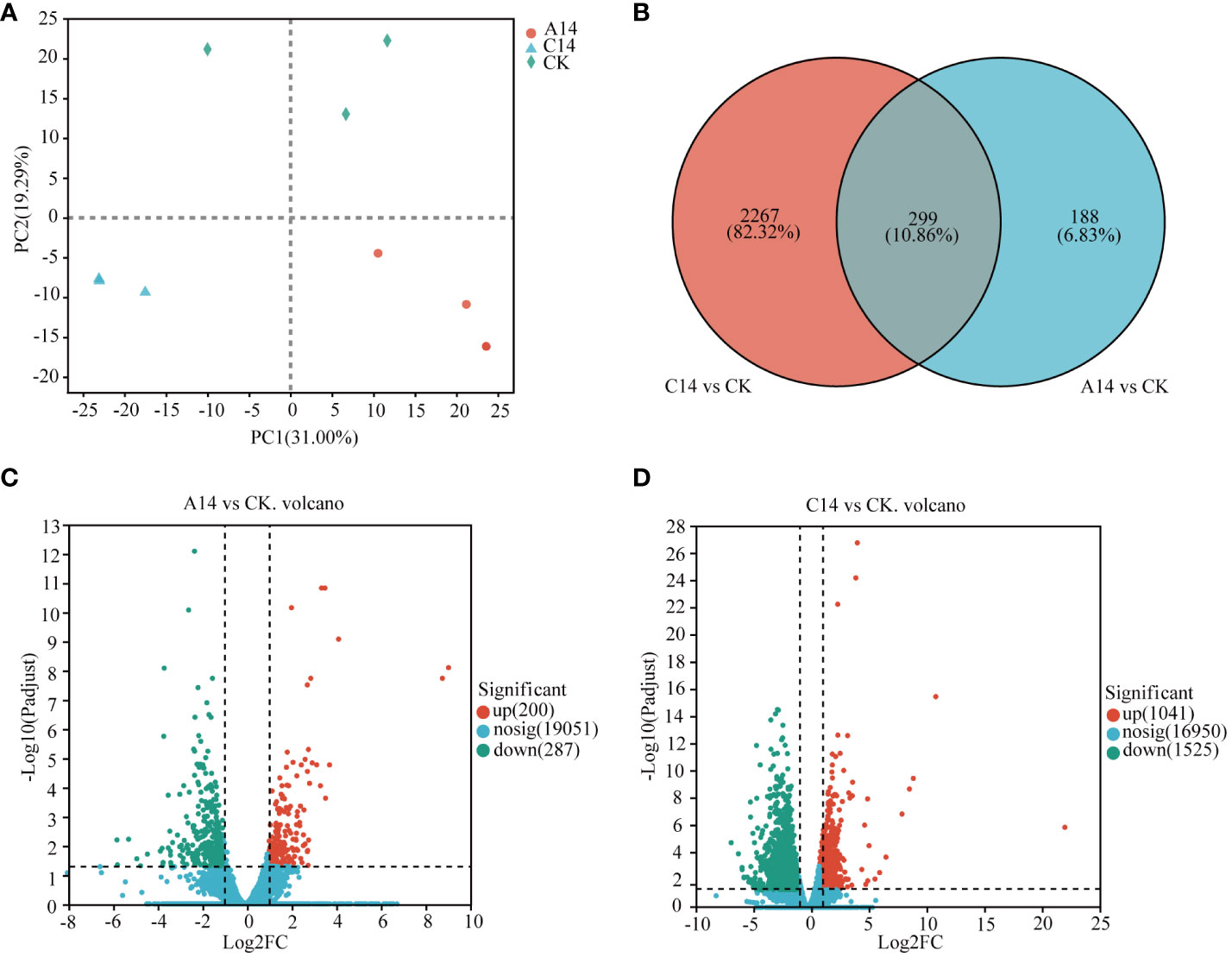
Figure 3 Differential expression of genes(DEGs) in Symbiodiniaceae under acute and chronic stress. (A) PCA between samples. (B) Venn analysis of Symbiodiniaceae DEGs, where red circles represent the number of DEGs in the chronic 14 °C group, and blue circles represent the number of differentially expressed genes in the acute 14 °C group. (C) Volcano plot of Symbiodiniaceae DEGs in the acute 14°C group. (D) Volcano plot of DEGs in the chronic 14°C group, where red dots indicate significantly upregulated genes, green dots indicate significantly downregulated genes, and blue dots represent non-significant differentially expressed genes. A, acute stress; C, chronic stress; CK, control; 14, 14°C.
In the 14°C chronic stress group, ribosomes, pyrimidine metabolism, alanine, aspartate and glutamate metabolism, starch and sucrose metabolism, pyruvate metabolism, and carbon fixation in photosynthetic organisms comprised pathways that were enriched among downregulated genes (Figures 4A, B; Supplementary Table S5). The main DEGs were the ribosomal constitutive protein-related genes, glutamate dehydrogenase (GDH2), phosphoglycerate kinase (PGK), glucoamylase (SGA1), and beta-glucosidase (bglX), among others. Although the glutathione metabolic pathway was not enriched among the upregulated genes, glutathione S-transferase (GST) expression was significantly upregulated (Figure 4C; Supplementary Table S5).
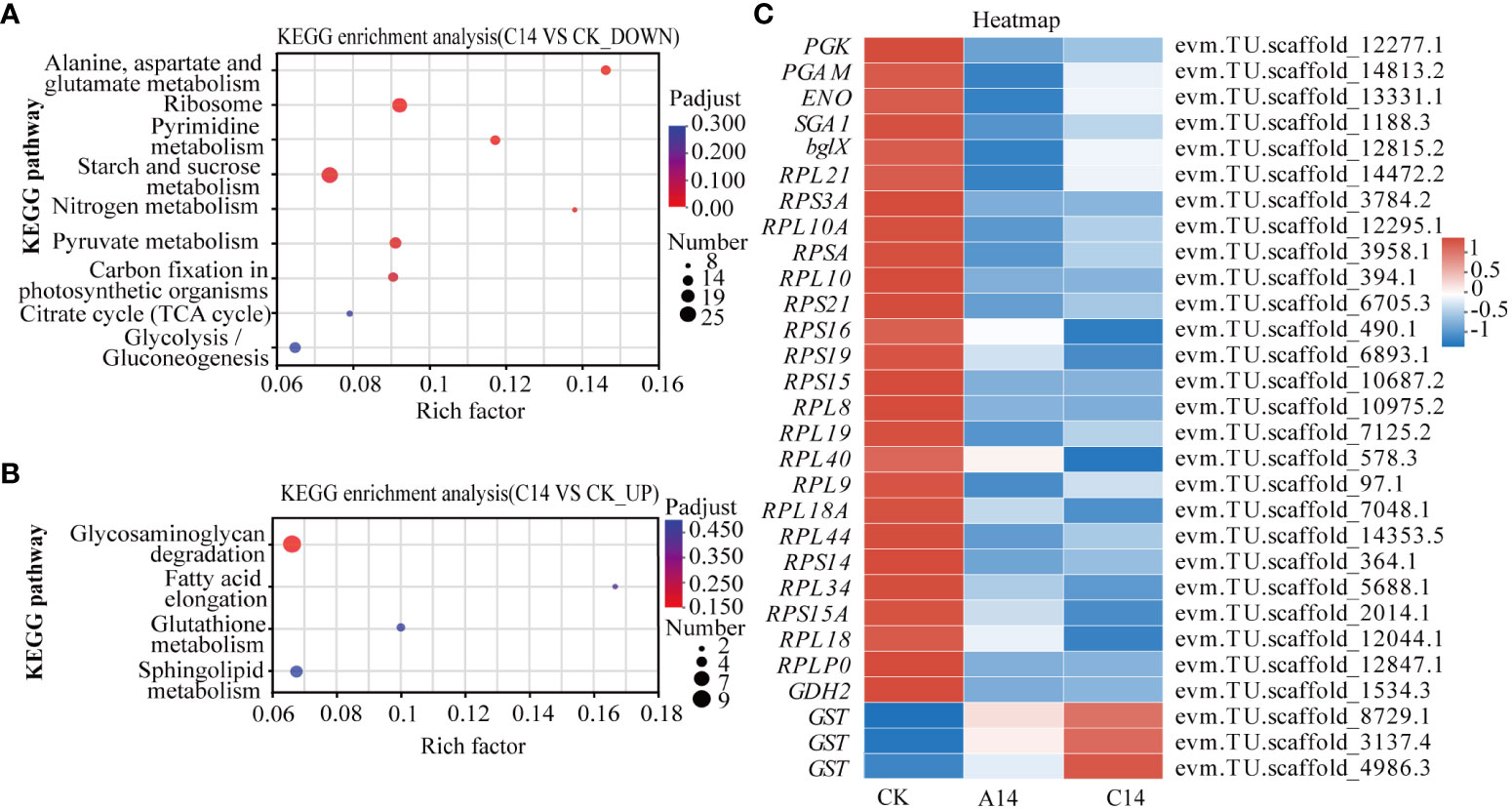
Figure 4 KEGG enrichment and heatmap of DEGs in Symbiodiniaceae under acute and chronic low-temperature stress. (A) KEGG enrichment results of significantly downregulated genes under chronic 14°C stress. (B) KEGG enrichment results of significantly upregulated genes under chronic 14°C stress, where the size of the dots indicates how many genes are in the pathway, and the color of the dots corresponds to different Padjust ranges. (C) Heatmap of key DEGs in Symbiodiniaceae under acute and chronic low-temperature stress. The color in the graph indicates the expression value of the gene after normalization treatment. A, acute stress; C, chronic stress; CK, control; 14, 14°C.
Based on Padjust < 0.05, there were no enriched pathways in the acute 14°C stress group. However, the PGK, SGA1, and bglX were significantly downregulated. Meanwhile, the ribosomal constitutive protein-related genes, GDH2 and GST were upregulated (Figure 4C; Supplementary Table S5).
3.3 Transcriptional changes in coral hosts under acute and chronic low-temperature stress
The expression of coral host genes was quantitatively analyzed using RSEM to obtain a quantitative index in terms of TPM (Supplementary Table S6). The results of PCA showed that PC1 and PC2 accounted for 43.5% and 23.78% of the total variance, respectively (Figure 5A). Compared with the control group (CK), the acute 14°C group (A14) had 5,703 DEGs, among which 1,943 were upregulated and 3,760 were downregulated (Figure 5C). Meanwhile, the chronic 14°C group (C14) had 4,637 DEGs, of which 1,565 were upregulated and 3,072 were downregulated (Figure 5D; Supplementary Table S7). Among these DEGs 1,885 were common to both groups, 3,818 were specific to the acute 14°C group and 2, 752 were common to the chronic 14°C group (Figure 5B; Supplementary Table S7).
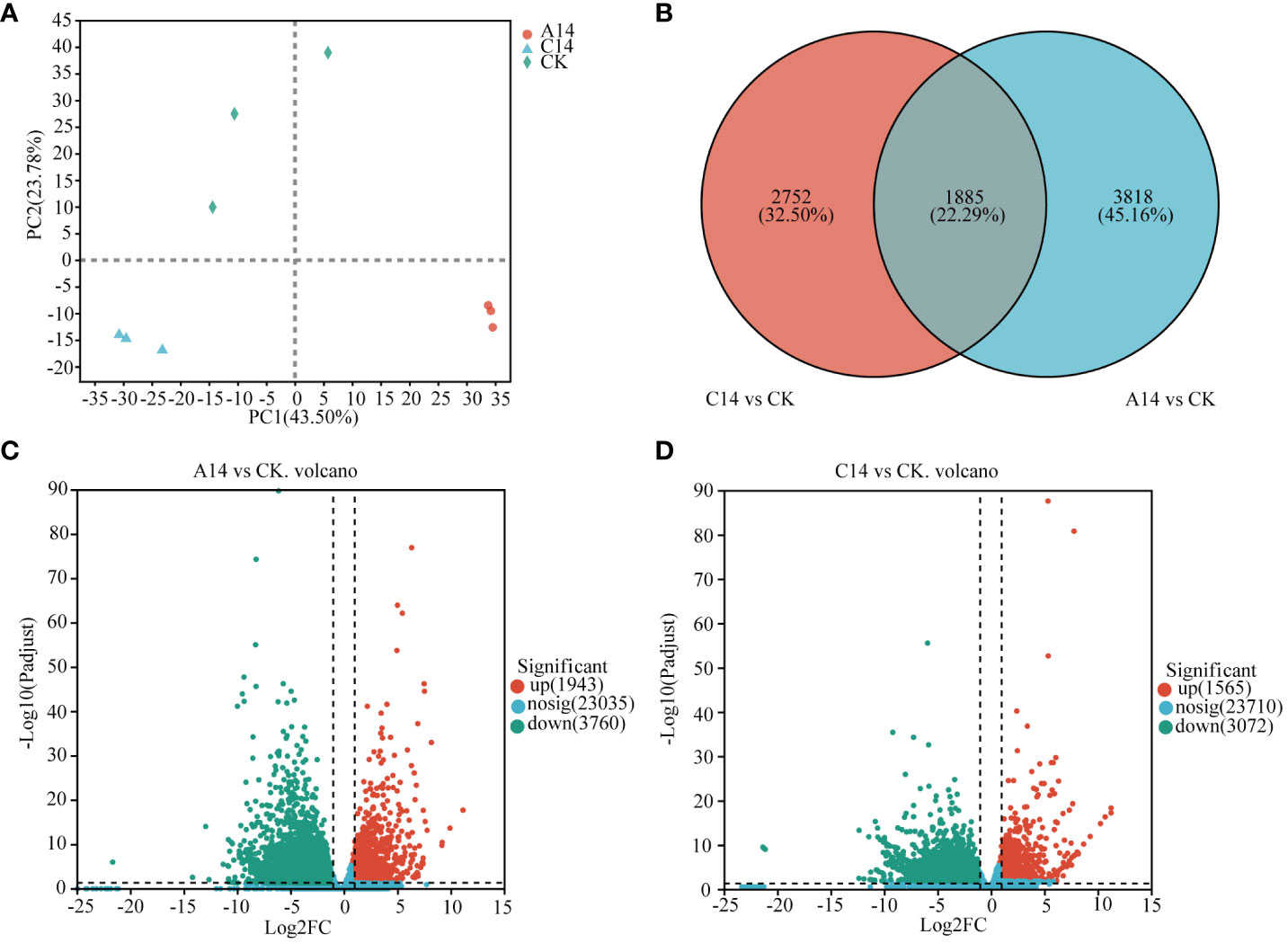
Figure 5 DEGs of coral host under acute and chronic low-temperature stress. (A) PCA between samples. (B) Venn analysis of host DEGs, where red circles represent the number of DEGs in the chronic 14°C group, and blue circles represent the number of differentially expressed genes in the acute 14°C group. (C) Volcano plot of host DEGs in the acute 14°C group. (D) Volcano plot of host DEGs in the chronic 14°C group, where red dots indicate upregulated genes, green dots indicate downregulated genes, and blue dots represent non-significant differentially expressed genes. A, acute stress; C, chronic stress; CK, control; 14, 14°C.
Based on a threshold of Padjust < 0.05, apoptosis-multiple species, protein processing in endoplasmic reticulum (ER), TNF signaling pathway, and oxidative phosphorylation pathway were significantly enriched among the upregulated genes in the acute 14°C group (Figure 6A; Supplementary Table S7). The major DEGs were caspase 3 (Casp3), caspase 8 (Casp8), caspase 9 (Casp9), apoptosis regulator Bcl-X (bcl-xL), heat shock 70kDa protein 1 (HSPA1), ER chaperone BiP (BIP), molecular chaperone HtpG (HSP90A), ubiquitin-conjugating enzyme E2G1 (UBE2G1), TNF receptor-associated factor 2 (TNFR2), the gene related to NADH dehydrogenase, succinate dehydrogenase, cytochrome c reductase, and cytochrome c oxidase. In addition, the mitochondrial uncoupling protein (UCP2_3) was significantly upregulated (Figure 6E; Supplementary Table S7).
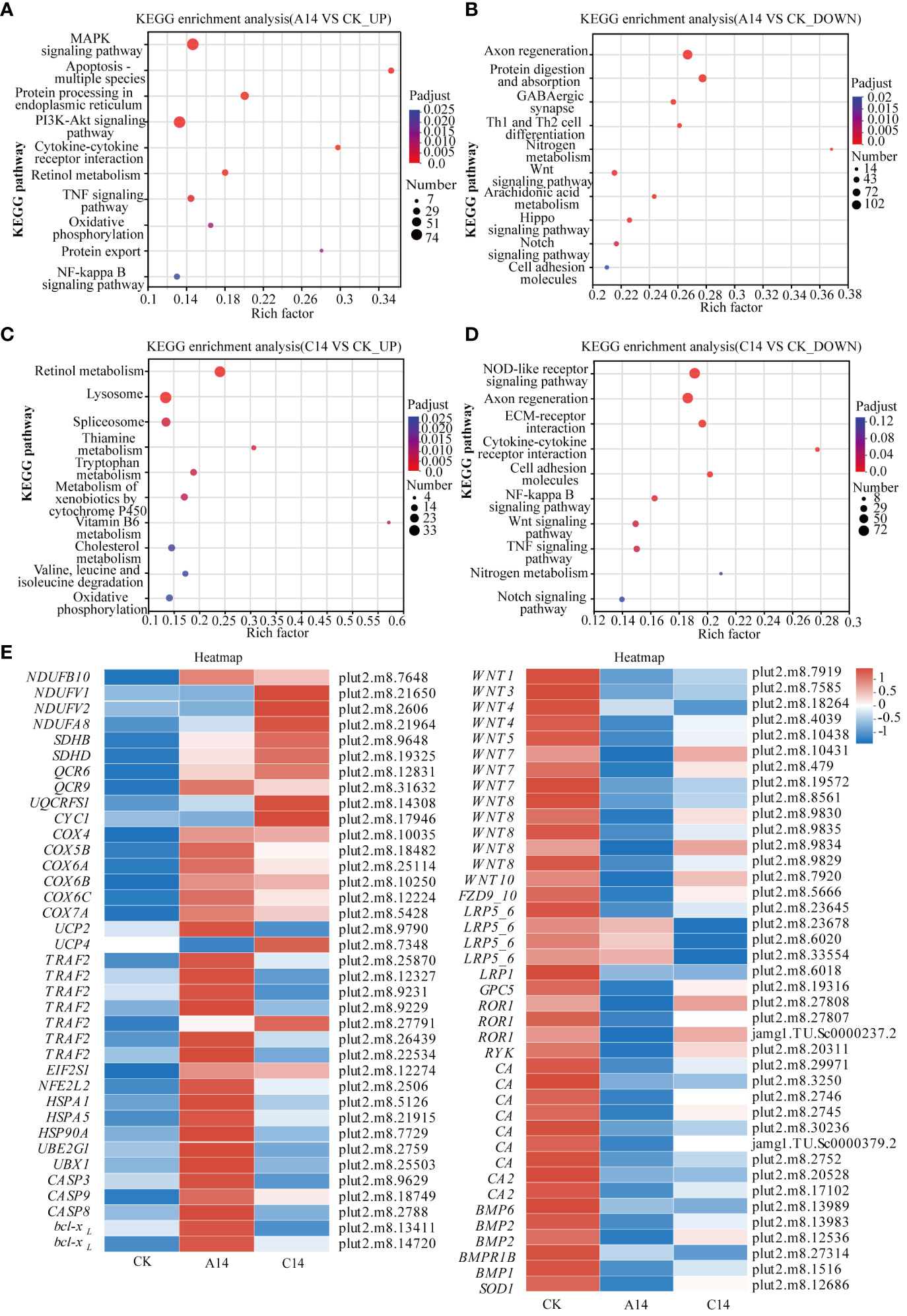
Figure 6 KEGG enrichment and heatmap of DEGs in P. lutea under acute and chronic low-temperature stress. (A) KEGG enrichment results for significantly upregulated genes in the acute 14 °C group. (B) KEGG enrichment results for significantly downregulated genes in the acute 14 °C group. (C) KEGG Enrichment results for significantly upregulated genes in the chronic 14 °C group. (D) KEGG enrichment results for significantly downregulated genes in the chronic 14 °C group, where the size of the dots indicates the number of genes in the pathway, and the color of the dots corresponds to different P adjust ranges. (E) Heatmap of key DEGs in P. lutea under acute and chronic low-temperature stress. The color indicates the expression value of the gene after normalization treatment. A, acute stress; C, chronic stress; CK, control; 14 = 14 °C.
The Wnt signaling pathway, Notch signaling pathway, nitrogen metabolism, and other pathways were enriched among the downregulated genes (Figure 6B; Supplementary Table S7). The main DEGs were the wingless-type MMTV integration site family (WNTs), frizzled 9/10 (FZD9_10), and low density lipoprotein receptor-related protein 5/6 (LRP5_6). In addition, carbonic anhydrase (CA) and bone morphogenetic protein (BMP), associated with calcification and oxidative stress-associated superoxide dismutase (SOD1), respectively, were significantly downregulated (Figure 6E; Supplementary Table S7).
In the chronic 14°C group, retinol metabolism, lysosomes, oxidative phosphorylation, and other pathways were enriched among upregulated genes (Figure 6C; Supplementary Table S7). The major DEGs were those related to NADH dehydrogenase, succinate dehydrogenase, cytochrome c reductase, and cytochrome c oxidase. Additionally, mitochondrial uncoupling protein (UCP4) was significantly upregulated (Figure 6E; Supplementary Table S7).
NF-kappa B, Wnt, and TNF signaling pathways, among others, were enriched among the downregulated genes (Figure 6D). The major DEGs were WNTs, LRP5_6, and TNFR2. In addition, the apoptosis-related genes Casp8, calcification-related CAs, and BMPs were significantly downregulated (Figure 6E; Table S7).
4 Discussion
4.1 Acute stress inhibits PSII functioning in P. lutea-symbiotic Symbiodiniaceae more than chronic low-temperature stress
The Fv/Fm of Symbiodiniaceae was significantly reduced under acute and chronic stress at 14°C, measuring 0.22 ± 0.03 and 0.31 ± 0.05, respectively. The cold threshold was 14.99°C for acute stress and 13.86°C for chronic stress based on the Fv/FmED50 parameter. When photosynthesis is working at peak efficiency, Fv/Fm values for Symbiodiniaceae typically range between 0.50 and 0.70 (Tunala et al., 2019). Under 14°C, the Fv/Fm values of P. lutea-symbiotic Symbiodiniaceae were always < 0.5, indicating that photosystem II functioning was inhibited. In a previous study, Fv/FmED50 was used to determine the temperature threshold for bleaching (Evensen et al., 2021). At 14°C, the acute stress group reached the bleaching threshold and coral holobionts were in a pre-bleaching state, but the chronic stress group did not reach the bleaching threshold. This indicates that acute low-temperature stress has a higher propensity to induce coral bleaching compared to chronic stress. Following an 8-day temperature decrease from 22°C to 12°C, the Fv/Fm values of coral holobionts remained stable (Büchel and Wilhelm, 1993; Keshavmurthy et al., 2021). However, coral holobionts exposed acutely to 10°C showed a significant decrease in Fv/Fm and died within 48 h of stress (Keshavmurthy et al., 2021). The Fv/Fm of Montipora digitata decreased after 3, 6, and 12 h of 20°C stress, but not significantly after 18 h, and recovered within 72 h of stress (Tracey et al., 2003). These phenomena could be attributed to the effects of stress rate or duration on the photophysiological response of Symbiodiniaceae.
Our results show that low-temperature stress has an inhibitory effect on photosynthesis in P. lutea-symbiotic Symbiodiniaceae. Notably, acute low-temperature stress inhibits photosystem II functioning of Symbiodiniaceae to a much greater extent than chronic stress.
4.2 Low-temperature stress leads to energy deprivation in P. lutea-symbiotic Symbiodiniaceae
Under acute and chronic low-temperature stress, PGK, SGA1, bglX, GDH2, and ribosomal protein-related genes were significantly downregulated, while GST was significantly upregulated. The glycolysis and hydrolysis of cellulose and starch to glucose are interconnected with the energy productivity of organisms. In glycolysis, PGK catalyzes the acyl phosphate transfer from 1,3-bisphosphoglycerate to the high-energy phosphate group of ADP, resulting in the production of ATP (Joshi et al., 2016). Low expression of PGK could lead to reduced production of pyruvate and ATP, causing energy deprivation in Symbiodiniaceae (Rosa-Téllez et al., 2017). Additionally, a correlation was observed between the reduction in Fv/Fm and the diminished activity of PGK. Low PGK activity impedes the regeneration of essential electron acceptors for the photosynthetic electron transport chain, thereby affecting photosynthesis (Rosa-Téllez et al., 2017). The SGA1 gene related to β-glucosidase and the bglX gene related to glucoamylase are involved in the hydrolysis of cellulose and starch to glucose, respectively. The downregulation of SGA1 and bglX during acute and chronic low-temperature stress further implies energy deprivation in Symbiodiniaceae.
Energy is important for maintaining physiological functions. Low-temperature stress inhibited PSII functioning in P. lutea-symbiotic Symbiodiniaceae, culminating in a decline in glucose production and, consequently, an energy shortage. Energy deprivation triggers a series of reactions that threaten the survival of organisms (Mia et al., 2006). Symbiodiniaceae coordinates the relationship between resistance to low-temperature stress and basal metabolism to maintain long-term survival. For example, in P. lutea-symbiotic Symbiodiniaceae, GST, related to glutathione metabolism.Glutathione S-transferases (GSTs) have glutathione peroxidase activity, through which they perform an oxygen-detoxifying function(Mannervik, 1985). GSTs play a significant role in decreasing ROS to protecting against oxidative stress (Liu et al., 2016). GST is upregulated in response to ROS overproduction stimulated by acute and chronic low-temperature stress (Ravelo et al., 2022). The downregulation of ribosomal protein-related genes and GDH2 involved in ammonia assimilation in Symbiodiniaceae. Photosynthates are important for the tricarboxylic acid (TCA) cycle, a process that provides energy and substrates for nitrogen metabolism (Zhang and Fernie, 2018). Protein synthesis and ammonia assimilation are important processes in nitrogen metabolism (Zhang and Fernie, 2018). Ribosomes are the main site of protein synthesis (Cassaignau et al., 2020). Glutamate dehydrogenase 2 (GDH2) operating at the interface of carbon and nitrogen metabolism has the potential capacity to assimilate inorganic N by combining ammonium with 2-Oxoglutarate to form glutamate (Dubois et al., 2003).It has been observed that reduction in photosynthetic rate was accompanied by the decrease in the activities of GDH (Singh et al., 2016).The downregulation of ribosomal protein-related genes and GDH2 could potentially be ascribed to substrate scarcity and energy deprivation. In summary, P. lutea-symbiotic Symbiodiniaceae PSII functioning is inhibited under low-temperature stress, which affects energy production by Symbiodiniaceae and leads to an energy shortage.
4.3 Low-temperature stress limits the calcification of P. lutea
In this study, the genes related to CA, BMP, and the Wnt signaling pathway were downregulated in coral hosts under acute and chronic low-temperature stress. The genes related to uncoupling proteins (UCPs), oxidative phosphorylation-related NADH dehydrogenase, succinate dehydrogenase, and cytochrome c oxidase were upregulated. Moreover, the gene related to superoxide dismutase (SOD) was significantly downregulated under acute stress and downregulated under chronic stress, but the differences were not significant (P > 0.05).
CA is associated with calcification in coral holobionts and facilitates the reversible hydration of CO2 to bicarbonate, thereby sustaining the CO2/HCO3− equilibrium (Furla et al., 2000; Zoccola et al., 2009; Bertucci et al., 2013; Hemond et al., 2014; Hopkinson et al., 2015). Extracellular CA plays a role in the uptake of CO2. Intracellular CA can convert ingested and respired CO2 into HCO3− (Bertucci et al., 2013; Hopkinson et al., 2015). CA is pivotal in both supplying carbon for calcification and facilitating carbon-concentrating mechanisms during Symbiodiniaceae photosynthesis (Hopkinson et al., 2015).Where PSII functioning is impaired, and the ATP and NADPH required for carbon assimilation may be reduced(Burlacot and Peltier, 2023). Thus, the reduction of inorganic carbon that can be fixed(Burlacot and Peltier, 2023). This reduction could lead to a downregulation of CA.The downregulation of CAs could potentially hinder the assimilation of inorganic carbon from seawater by coral holobionts, thereby constraining calcification (Tambutté et al., 2007). Additionally, BMP participate in the formation of cartilage and bone in animals and constitute an essential component of the skeletal organic matrix in corals (Mass et al., 2016). The downregulation of BMPs also indicates that coral calcification was affected. The Wnt signaling pathway has been demonstrated to promote osteogenesis in animals and potentially affect coral calcification (Broun et al., 2005; Guder et al., 2006; Plickert et al., 2006; Duffy et al., 2010; Hemond et al., 2014). The downregulation of the genes associated with Wnt signaling pathway, CAs, and BMPs revealed potential constraints of coral calcification under low-temperature stress.
Calcification is related to the energy supply. The photosynthesis of Symbiodiniaceae provides the energy for calcification, thereby serving as a crucial role for coral calcification (Hatcher, 1988). Under energy loss, coral hosts regulate various metabolic activities to maintain the stability of coral holobionts (Rinkevich, 1996; Sokolova, 2013; Tang et al., 2020; Leinbach et al., 2021). In this study, photosystem II functioning was inhibited in Symbiodiniaceae under acute and chronic low-temperature stress, culminating in a reduction in energy acquisition by the coral host. Furthermore, previous studies have indicated that low-temperature stress impairs Symbiodiniaceae’s PSII, elevating ROS levels in tissues (Jones et al., 1998; Tracey et al., 2003; Thornhill et al., 2008; Marangoni et al., 2021). Utilizing antioxidant enzymes to scavenge excess ROS is vital for coral redox homeostasis (Zhang et al., 2022). However, in this study, the genes related to the antioxidant enzyme SOD in P. lutea were not upregulated under low-temperature stress. It is possible that P. lutea scavenges excess ROS by expelling Symbiodiniaceae with impaired photosynthesis. Therefore, the host would have a reduced demand for the antioxidant enzyme. Previous studies have demonstrated that damaged Symbiodiniaceae expulsion diminishes coral host ROS levels under low-temperature stress (Higuchi et al., 2015; Marangoni et al., 2021). This also resulted in a reduction in the energy supply derived from Symbiodiniaceae to the host.
Upregulation of genes related to UCPs may induce energy loss in the coral host. UCP-mediated oxidative phosphorylation decoupling in mitochondria potentially reduces mitochondrial ROS and contributes to antioxidative effects (Mailloux and Harper, 2011; Slocińska et al., 2016). However, UCP decoupling decreases oxidative phosphorylation efficiency, limiting the energy harnessed from the electron transport chain for ATP synthesis (Slocinska et al., 2016). Under acute and chronic low-temperature stress, the genes related to NADH dehydrogenase, succinate dehydrogenase, cytochrome c reductase, and cytochrome c oxidase related to oxidative phosphorylation were upregulated in P. lutea, but the expression of genes related to ATP synthesis did not change significantly. This also implies oxidative phosphorylation uncoupling in P. lutea. Under acute and chronic low-temperature stress, P. lutea may facilitate phosphorylation uncoupling through UCP-related genes upregulation, partially leading to energy loss.
Low-temperature stress inhibited Symbiodiniaceae PSII functioning, prompted expulsion from the coral host, and uncoupled oxidative phosphorylation, leading to energy deprivation. Under an energy shortage, P. lutea limits calcification by downregulating CAs and BMPs.
4.4 Under low temperature, acute stress induces P. lutea apoptosis, whereas chronic stress facilitates acclimation
Our results showed upregulation of genes associated with apoptotic processes in coral hosts under acute low-temperature stress, including Casp3, Casp8, Casp9, bcl-xL, and TNF signaling pathway-related gene TRAF2, and ER stress-related genes EIF2S1, NRF2, HSPA1, HSPA5, HSP90, UBE2G1, and UBX1, among others. However, under chronic low-temperature stress, key genes associated with apoptotic processes, such as TNFR2, HSP90, and Casp3, among others, either remained unchanged or were downregulated.
Apoptosis is pivotal in development, morphogenesis, and immunity (Shao et al., 2016). However, excessive apoptosis leads to coral bleaching (Desalvo et al., 2008; DeSalvo et al., 2012; Yu et al., 2017). Apoptosis is a form of programmed cell death that occurs in multicellular organisms and is triggered by the proteolytic caspase cascade (Shao et al., 2016). Caspase 8 and caspase 9 are promoters that regulate apoptosis (Tummers and Green, 2021). Caspase 3 is central to apoptosis regulation (Yu et al., 2017). Its activation usually indicates that the cell has undergone apoptosis (Yu et al., 2017). The apoptotic genes Casp3, Casp8, and Casp9 was upregulated, thereby substantiating the induction of apoptosis in P. lutea. Anti-apoptotic bcl-xL was upregulated in our study. This indicates a counterbalance stage between apoptosis and anti-apoptosis in coral. Previous studies have shown that apoptotic and anti-apoptotic processes coexist within cells (Kvitt et al., 2016; Majerova et al., 2021). The balance between anti-apoptotic and pro-apoptotic genes within the cell dictates the ultimate apoptotic rate(Kvitt et al., 2016; Majerova et al., 2021; Jiang et al., 2023). This also suggests that P. lutea under acute stress at 14°C was in a pre-bleaching stage. If low-temperature stress continues, then P. lutea might become bleached and die owing to apoptosis. However, if conditions are restored to a suitable temperature, P. lutea might recover from the pre-bleaching state.
TRAF2, related to the TNF signaling pathway, was upregulated in response to acute low-temperature stress. The TNF signaling pathway is pivotal in modulating immunity, inflammation, apoptosis, and cell proliferation and differentiation (Cho et al., 2003). Previous studies have shown that activation of the TNF signaling pathway mediated by TNF-α can trigger apoptosis and bleaching in corals (Cho et al., 2003). The TNF receptor ligand family (TNFRSF/TNFSF) is a central mediator of apoptosis (Quistad et al., 2014). Upon external stimulation, TNF binds to TNF receptors, initiating downstream signaling cascades that culminate in apoptosis (Quistad et al., 2014). This study indicates that under acute low-temperature stress, P. lutea induces apoptosis through the TNF signaling pathway. This could be an important mechanism of cold bleaching.
Excessive ER stress can precipitate apoptosis (Haas, 1994; Springer et al., 1999). Peptides undergo assembly, folding, and posttranslational modifications in the ER (Haas, 1994; Springer et al., 1999). Only properly folded proteins enter the Golgi apparatus from the ER via transport vesicles. ER stress, induced by the accumulation of misfolded proteins, triggering the unfolded protein response (Haas, 1994; Springer et al., 1999). Under acute low-temperature stress, increased EIF2S1 and NRF2 expression potentially signals unfolded protein response transmission to the nucleus. The final misfolded proteins are retro-translocated to the cytoplasm and undergo ubiquitin-mediated proteasomal degradation via the ER-associated degradation process (Maor-Landaw et al., 2014). Heat shock proteins and ubiquitin-coupling enzyme E2 play important roles in this process (Maor-Landaw et al., 2014). The genes involved in ER-associated degradation and ubiquitin-mediated hydrolysis are upregulated. This suggests that P. lutea experiences ER stress and uses these mechanisms to process the aggregated misfolded proteins in response to acute low-temperature stress. However, if these stressors are not relieved, then apoptosis is triggered.
Under chronic low-temperature stress, TNFR2, HSP90, Casp3, and other genes remained unchanged or downregulated, indicating that the cells did not enter the apoptosis stage. Therefore, acute low-temperature stress presents a heightened threat to coral holobionts compared to chronic low-temperature stress.
5 Conclusion
Low-temperature stress impedes the potential of relatively high-latitude coral reefs to function as refuges for coral. In this study, the response mechanisms of coral holobionts to low-temperature stress was explored. Our study indicates that P. lutea from high-latitude coral reefs in the South China Sea manifests photophysiological and gene expression responses to both acute and chronic low-temperature stress. Low-temperature stress resulted in a significant decrease in the Fv/Fm of Symbiodiniaceae, indicating the inhibition of PSII functioning, which might have contributed to energy deprivation in both Symbiodiniaceae and coral hosts. During low-temperature stress, calcifications-related genes were downregulated, possibly because of the reduced investment of energy-scarce coral hosts in calcification. Additionally, the acute stress group displayed a markedly diminished Fv/Fm relative to the chronic stress group, potentially catalyzing coral bleaching and mortality through induced cell apoptosis, thereby constituting a more severe threat to coral holobionts.
Data availability statement
The original contributions presented in the study are publicly available. This data can be found here: https://www.ncbi.nlm.nih.gov, BioProject: PRJNA1026091.
Ethics statement
Ethical approval was not required for the study involving animals in accordance with the local legislation and institutional requirements because no subjects requiring ethical clearance were involved.
Author contributions
XW: Data curation, Formal analysis, Validation, Visualization, Writing – original draft. KY: Conceptualization, Funding acquisition, Methodology, Writing – review & editing. ZQ: Conceptualization, Investigation, Methodology, Supervision, Writing – review & editing. SC: Software, Validation, Visualization, Writing – review & editing. NP: Software, Validation, Visualization, Writing – review & editing. ML: Software, Validation, Visualization, Writing – review & editing.
Funding
The author(s) declare financial support was received for the research, authorship, and/or publication of this article. This research was supported by the National Natural Science Foundation of China (42090041, 42030502, 42206157), the Natural Science Foundation of Guangxi Province (2022GXNSFBA035449), and the Guangxi Scientific Projects (nos. AD17129063 and AA17204074).
Conflict of interest
The authors declare that the research was conducted in the absence of any commercial or financial relationships that could be construed as a potential conflict of interest.
Publisher’s note
All claims expressed in this article are solely those of the authors and do not necessarily represent those of their affiliated organizations, or those of the publisher, the editors and the reviewers. Any product that may be evaluated in this article, or claim that may be made by its manufacturer, is not guaranteed or endorsed by the publisher.
Supplementary material
The Supplementary Material for this article can be found online at: https://www.frontiersin.org/articles/10.3389/fmars.2024.1321865/full#supplementary-material
References
Beger M., Sommer B., Harrison P. L., Smith S. A. D., Pandolfi J. M. (2014). Conserving potential coral reef refuges at high latitudes. Diversity Distributions 20, 245–257. doi: 10.1111/ddi.12140
Bertucci A., Moya A., Tambutté S., Allemand D., Supuran C. T., Zoccola D. (2013). Carbonic anhydrases in anthozoan corals—A review. Bioorganic Medicinal Chem. 21, 1437–1450. doi: 10.1016/j.bmc.2012.10.024
Broun M., Gee L., Reinhardt B., Bode H. R. (2005). Formation of the head organizer in hydra involves the canonical Wnt pathway. Development 132, 2907–2916. doi: 10.1242/dev.01848
Burlacot A., Peltier G. (2023). Energy crosstalk between photosynthesis and the algal CO2-concentrating mechanisms. Trends Plant Sci. 28 (7), 795–807. doi: 10.1016/j.tplants.2023.03.018
Büchel C., Wilhelm C. (1993). In vivo analysis of slow chlorophyll fluorescence induction kinetics in algae: progress, problems and perspextives. Photochem. Photobiol. 58, 137–148. doi: 10.1111/j.1751-1097.1993.tb04915.x
Camp E. F., Schoepf V., Mumby P. J., Hardtke L. A., Rodolfo-Metalpa R., Smith D. J., et al. (2018). The future of coral reefs subject to rapid climate change: lessons from natural extreme environments. Front. Mar. Sci. 5. doi: 10.3389/fmars.2018.00004
Carpenter K. E., Abrar M., Aeby G., Aronson R. B., Banks S., Bruckner A., et al. (2008). One-third of reef-building corals face elevated extinction risk from climate change and local impacts. Science 321, 560–563. doi: 10.1126/science.1159196
Carrigan A. D., Puotinen M. (2014). Tropical cyclone cooling combats region-wide coral bleaching. Global Change Biol. 20, 1604–1613. doi: 10.1111/gcb.12541
Cassaignau A. M. E., Cabrita L. D., Christodoulou J. (2020). How does the ribosome fold the proteome? Annu. Rev. Biochem. 89 (1), 389–415. doi: 10.1146/annurev-biochem-062917-012226
Chen B., Yu K., Liang J., Huang W., Wang G., Su H., et al. (2019). Latitudinal variation in the molecular diversity and community composition of Symbiodiniaceae in coral from the South China Sea. Front. Microbiol. 10. doi: 10.3389/fmicb.2019.01278
Chen T., Li S., Yu K., Zheng Z., Wang L., Chen T. (2013). Increasing temperature anomalies reduce coral growth in the Weizhou Island, northern South China Sea. Estuarine Coast. Shelf Sci. 130, 121–126. doi: 10.1016/j.ecss.2013.05.009
Chen T., Yu K., Shi Q., Li S., Price G. J., Wang R., et al. (2009). Twenty-five years of change in scleractinian coral communities of Daya Bay (northern South China Sea) and its response to the 2008 AD extreme cold climate event. Chin. Sci. Bulletin. 54, 2107–2117. doi: 10.1007/s11434-009-0007-8
Chen S., Zhou Y., Chen Y., Gu J. (2018). fastp : an ultra-fast all-in-one FASTQ preprocessor. Bioinformatics 34 (17), i884–i890. doi: 10.1093/bioinformatics/bty560
Cho K.-H., Shin S.-Y., Lee H.-W., Wolkenhauer O. (2003). Investigations into the analysis and modeling of the TNFα-mediated NF-κB-signaling pathway. Genome Res. 13, 2413–2422. doi: 10.1101/gr.1195703
DeSalvo M. K., Estrada A., Sunagawa S., Medina S. M. (2012). Transcriptomic responses to darkness stress point to common coral bleaching mechanisms. Coral Reefs 31, 215–228. doi: 10.1007/s00338-011-0833-4
Desalvo M. K., Voolstra C. R., Sunagawa S., Schwarz J. A., Stillman J. H., Coffroth M. A., et al. (2008). Differential gene expression during thermal stress and bleaching in the Caribbean coral Montastraea faveolata. Mol. Ecol. 17, 3952–3971. doi: 10.1111/j.1365-294X.2008.03879.x
Dubois F., Tercé-Laforgue T., Gonzalez-Moro M.-B., Estavillo J.-M., Sangwan R., Gallais A., et al. (2003). Glutamate dehydrogenase in plants: is there a new story for an old enzyme? Plant Physiol. Biochem. 41 (6), 565–576. doi: 10.1016/S0981-9428(03)00075-5
Duffy D. J., Plickert G., Kuenzel T., Tilmann W., Frank U. (2010). Wnt signaling promotes oral but suppresses aboral structures in Hydractinia metamorphosis and regeneration. Development 137, 3057–3066. doi: 10.1242/dev.046631
Evensen N. R., Fine M., Perna G., Voolstra C. R., Barshis D. J. (2021). Remarkably high and consistent tolerance of a Red Sea coral to acute and chronic thermal stress exposures. Limnol. Oceanogr. 66, 1718–1729. doi: 10.1002/lno.11715
Furla P., Allemand D., Orsenigo M.-N. (2000). Involvement of H+-ATPase and carbonic anhydrase in inorganic carbon uptake for endosymbiont photosynthesis. Am. J. Physiology-Regulatory Integr. Comp. Physiol. 278, R870–R881. doi: 10.1152/ajpregu.2000.278.4.R870
Green R. H., Lowe R. J., Buckley M. L., Foster T., Gilmour J. P. (2019). Physical mechanisms influencing localized patterns of temperature variability and coral bleaching within a system of reef atolls. Coral Reefs 38, 759–771. doi: 10.1007/s00338-019-01771-2
Greenstein B. J., Pandolfi J. M. (2008). Escaping the heat: range shifts of reef coral taxa in coastal Western Australia. Global Change Biol. 14, 513–528. doi: 10.1111/j.1365-2486.2007.01506.x
Guder C., Pinho S., Nacak T. G., Schmidt H. A., Hobmayer B., Niehrs C., et al. (2006). An ancient Wnt-Dickkopf antagonism in Hydra. Development 133, 901–911. doi: 10.1242/dev.02265
Haas I. G. (1994). BiP (GRP78), an essential hsp70 resident protein in the endoplasmic reticulum. Experientia 50, 1012–1020. doi: 10.1007/BF01923455
Hatcher B. G. (1988). Coral reef primary productivity: A beggar's banquet. Trends Ecol. Evolution 3, 106–111. doi: 10.1016/0169-5347(88)90117-6
Hemond E. M., Kaluziak S. T., Vollmer S. V. (2014). The genetics of colony form and function in Caribbean Acropora corals. BMC Genomics 15, 1133. doi: 10.1186/1471-2164-15-1133
Heron S. F., Maynard J. A., van Hooidonk R., Eakin C. M. (2016). Warming trends and bleaching stress of the world’s coral reefs 1985–2012. Sci. Rep. 6, 38402. doi: 10.1038/srep38402
Higuchi T., Agostini S., Casareto B. E., Suzuki Y., Yuyama I. (2015). The northern limit of corals of the genus Acropora in temperate zones is determined by their resilience to cold bleaching. Sci. Rep. 5, 18467. doi: 10.1038/srep18467
Hopkinson B. M., Tansik A. L., Fitt W. K. (2015). Internal carbonic anhydrase activity in the tissue of scleractinian corals is sufficient to support proposed roles in photosynthesis and calcification. J. Exp. Biol. 218, 2039–2048. doi: 10.1242/jeb.118182
Huang W., Yang E., Yu K., Meng L., Wang Y., Liang J., et al. (2022). Lower cold tolerance of tropical Porites lutea is possibly detrimental to its migration to relatively high latitude refuges in the South China Sea. Mol. Ecol. 31, 5339–5355. doi: 10.1111/mec.16662
Hughes T. P., Kerry J. T., Álvarez-Noriega M., Álvarez-Romero J. G., Anderson K. D., Baird A. H., et al. (2017). Global warming and recurrent mass bleaching of corals. Nature 543, 373–377. doi: 10.1038/nature21707
Jiang L., Liu C.-Y., Cui G., Huang L.-T., Yu X.-L., Sun Y.-F., et al. (2023). Rapid shifts in thermal reaction norms and tolerance of brooded coral larvae following parental heat acclimation. Mol. Ecol. 32 (5), 1098–1116. doi: 10.1111/mec.16826
Jones R. J., Hoegh-Guldberg O., Larkum A. W. D., Schreiber U. (1998). Temperature-induced bleaching of corals begins with impairment of the CO2 fixation mechanism in zooxanthellae. Plant Cell Environment 21, 1219–1230. doi: 10.1046/j.1365-3040.1998.00345.x
Joshi R., Karan R., Singla-Pareek S. L., Pareek A. (2016). Ectopic expression of Pokkali phosphoglycerate kinase-2 (OsPGK2-P) improves yield in tobacco plants under salinity stress. Plant Cell Rep. 35, 27–41. doi: 10.1007/s00299-015-1864-z
Karnauskas K. B. (2020). Physical diagnosis of the 2016 great barrier reef bleaching event. Geophysical Res. Letters 47, e2019GL086177. doi: 10.1029/2019GL086177
Keshavmurthy S., Beals M., Hsieh H. J., Choi K. S., Chen C. A. (2021). Physiological plasticity of corals to temperature stress in marginal coral communities. Sci. Total Environment 758, 143628. doi: 10.1016/j.scitotenv.2020.143628
Kim D., Langmead B., Salzberg S. L. (2015). HISAT: a fast spliced aligner with low memory requirements. Nat. Methods 12 (4), 357–360. doi: 10.1038/nmeth.3317
Kvitt H., Rosenfeld H., Tchernov D. (2016). The regulation of thermal stress induced apoptosis in corals reveals high similarities in gene expression and function to higher animals. Sci. Rep. 6, 30359. doi: 10.1038/srep30359
Leinbach S. E., Speare K. E., Rossin A. M., Holstein D. M., Strader M. E. (2021). Energetic and reproductive costs of coral recovery in divergent bleaching responses. Sci. Rep. 11, 23546. doi: 10.1038/s41598-021-02807-w
Li B., Dewey C. N. (2011). RSEM: accurate transcript quantification from RNA-Seq data with or without a reference genome. BMC Bioinf. 12 (1), 323. doi: 10.1186/1471-2105-12-323
Liu S., Liu F., Jia H., Yan Y., Wang H., Guo X., et al. (2016). A glutathione S-transferase gene associated with antioxidant properties isolated from Apis cerana cerana. Sci. Nature 103 (5), 43. doi: 10.1007/s00114-016-1362-3
Love M. I., Huber W., Anders S. (2014). Moderated estimation of fold change and dispersion for RNA-seq data with DESeq2. Genome Biol. 15 (12), 550. doi: 10.1186/s13059-014-0550-8
Mailloux R. J., Harper M.-E. (2011). Uncoupling proteins and the control of mitochondrial reactive oxygen species production. Free Radical Biol. Med. 51, 1106–1115. doi: 10.1016/j.freeradbiomed.2011.06.022
Majerova E., Carey F. C., Drury C., Gates R. D. (2021). Preconditioning improves bleaching tolerance in the reef-building coral Pocillopora acuta through modulations in the programmed cell death pathways. Mol. Ecol. 30, 3560–3574. doi: 10.1111/mec.15988
Mannervik B. (1985). Glutathione peroxidase. Methods Enzymol. Acad. Press 113, 490–495. doi: 10.1016/S0076-6879(85)13063-6
Maor-Landaw K., Karako-Lampert S., Ben-Asher H. W., Goffredo S., Falini G., Dubinsky Z., et al. (2014). Gene expression profiles during short-term heat stress in the red sea coral Stylophora pistillata. Global Change Biol. 20, 3026–3035. doi: 10.1111/gcb.12592
Marangoni L., Rottier C., Ferrier-Pagès C. (2021). Symbiont regulation in Stylophora pistillata during cold stress: an acclimation mechanism against oxidative stress and severe bleaching. J. Exp. Biol. 224, jeb235275. doi: 10.1242/jeb.235275
Mass T., Putnam H. M., Drake J. L., Drake J. L., Zelzion E., Gates R. D., et al. (2016). Temporal and spatial expression patterns of biomineralization proteins during early development in the stony coral Pocillopora damicornis. Proc. R. Soc. B: Biol. Sci. 283, 20160322. doi: 10.1098/rspb.2016.0322
McGowan H., Theobald A. (2017). ENSO weather and coral bleaching on the great barrier reef, Australia. Geophysical Res. Lett. 44, 10,601–610,607. doi: 10.1002/2017GL074877
Mia O. H., Kenneth R. N. A., Sean R. C. (2006). Energetic cost of photoinhibition in corals. Mar. Ecol. Prog. Series 313, 1–12. doi: 10.3354/meps313001
Moberg F., Folke C. (1999). Ecological goods and services of coral reef ecosystems. Ecol. Economics 29, 215–233. doi: 10.1016/S0921-8009(99)00009-9
Pertea M., Pertea G. M., Antonescu C. M., Chang T.-C., Mendell J. T., Salzberg S. L. (2015). StringTie enables improved reconstruction of a transcriptome from RNA-seq reads. Nat. Biotechnol. 33 (3), 290–295. doi: 10.1038/nbt.3122
Plickert G., Jacoby V., Frank U., Müller W. A., Mokady O. (2006). Wnt signaling in hydroid development: Formation of the primary body axis in embryogenesis and its subsequent patterning. Dev. Biol. 298, 368–378. doi: 10.1016/j.ydbio.2006.06.043
Quistad S. D., Stotland A., Barott K. L., Smurthwaite C. A., Hilton B. J., Grasis J. A., et al. (2014). Evolution of TNF-induced apoptosis reveals 550 My of functional conservation. Proc. Natl. Acad. Sci. 111, 9567–9572. doi: 10.1073/pnas.1405912111
Ralph P. J., Gademann R., Larkum A. W. D., Schreiber U. (1999). In situ underwater measurements of photosynthetic activity of coral zooxanthellae and other reef-dwelling dinoflagellate endosymbionts. Mar. Ecol. Prog. Series 180, 139–147. doi: 10.3354/meps180139
Ravelo S. F., Posadas N., Conaco C. (2022). Transcriptome analysis reveals the expressed gene complement and acute thermal stress response of acropora digitifera endosymbionts. Front. Mar. Sci. 9. doi: 10.3389/fmars.2022.758579
Rayson M. D., Ivey G. N., Jones N. L., Fringer O. B. (2018). Resolving high-frequency internal waves generated at an isolated coral atoll using an unstructured grid ocean model. Ocean Modelling 122, 67–84. doi: 10.1016/j.ocemod.2017.12.007
Rayson M. D., Ivey G. N., Jones N. L., Lowe R. J., Wake G. W., McConochie J. D. (2015). Near-inertial ocean response to tropical cyclone forcing on the Australian North-West Shelf. J. Geophysical Res.: Oceans 120, 7722–7751. doi: 10.1002/2015JC010868
Riegl B., Glynn P. W., Banks S., Keith I., Rivera F., Vera-Zambrano M. (2019). Heat attenuation and nutrient delivery by localized upwelling avoided coral bleaching mortality in northern Galapagos during 2015/2016 ENSO. Coral Reefs 38, 773–785. doi: 10.1007/s00338-019-01787-8
Rinkevich B. (1996). Do reproduction and regeneration in damaged corals compete for energy allocation? Mar. Ecol. Prog. Series 143, 297–302. doi: 10.3354/meps143297
Ritz C., Baty F., Streibig J. C., Gerhard D. (2016). Dose-response analysis using R. PloS One 10, e0146021. doi: 10.1371/journal.pone.0146021
Rosa-Téllez S., Anoman A. D., Flores-Tornero M., Toujani W., Alseek S., Fernie A. R., et al. (2017). Phosphoglycerate kinases are co-regulated to adjust metabolism and to optimize growth. Plant Physiol. 176, 1182–1198. doi: 10.1104/pp.17.01227
Roth M. S., Goericke R., Deheyn D. D. (2012). Cold induces acute stress but heat is ultimately more deleterious for the reef-building coral Acropora yongei. Sci. Rep. 2, 240. doi: 10.1038/srep00240
Schlegel R. W., Darmaraki S., Benthuysen J. A., Filbee-Dexter K., Oliver E. C. J. (2021). Marine cold-spells. Prog. Oceanogr. 198, 102684. doi: 10.1016/j.pocean.2021.102684
Schlegel R. W., Oliver E. C. J., Wernberg T., Smit A. J. (2017). Nearshore and offshore co-occurrence of marine heatwaves and cold-spells. Prog. Oceanogr. 151, 189–205. doi: 10.1016/j.pocean.2017.01.004
Shao Y., Li C., Zhang W., Duan X., Li Y., Jin C., et al. (2016). Molecular cloning and characterization of four caspases members in Apostichopus japonicus. Fish Shellfish Immunol. 55, 203–211. doi: 10.1016/j.fsi.2016.05.039
Singh M., Singh V. P., Prasad S. M. (2016). Responses of photosynthesis, nitrogen and proline metabolism to salinity stress in Solanum lycopersicum under different levels of nitrogen supplementation. Plant Physiol. Biochem. 109, 72–83. doi: 10.1016/j.plaphy.2016.08.021
Slocinska M., Barylski J., Jarmuszkiewicz W. (2016). Uncoupling proteins of invertebrates: A review. IUBMB Life. 68, 691–699. doi: 10.1016/j.fsi.2016.05.039
Slocińska M., Rosinski G., Jarmuszkiewicz W. (2016). Activation of mitochondrial uncoupling protein 4 and ATP-sensitive potassium channel cumulatively decreases superoxide production in insect mitochondria. Protein Pept. Letters 23, 63–68. doi: 10.1002/iub.1535
Sokolova I. M. (2013). Energy-limited tolerance to stress as a conceptual framework to integrate the effects of multiple stressors. Integr. Comp. Biol. 53, 597–608. doi: 10.1093/icb/ict028
Sommer B., Beger M., Harrison P. L., Babcock R. C., Pandolfi J. M. (2018). Differential response to abiotic stress controls species distributions at biogeographic transition zones. Ecography 41, 478–490. doi: 10.1111/ecog.02986
Southward A. J., Hawkins S. J., Burrows M. T. (1995). Seventy years' observations of changes in distribution and abundance of zooplankton and intertidal organisms in the western English Channel in relation to rising sea temperature. J. Thermal Biol. 20, 127–155. doi: 10.1016/0306-4565(94)00043-I
Springer S., Spang A., Schekman R. (1999). A primer on vesicle budding. Cell 97, 145–148. doi: 10.1016/S0092-8674(00)80722-9
Tambutté S., Tambutté E., Zoccola D., Caminiti N., Lotto S., Moya A., et al. (2007). Characterization and role of carbonic anhydrase in the calcification process of the azooxanthellate coral Tubastrea aurea. Mar. Biol. 151, 71–83. doi: 10.1007/s00227-006-0452-8
Tang J., Ni X., Wen J., Wang L., Luo J., Zhou Z. (2020). Increased Ammonium Assimilation Activity in the Scleractinian Coral Pocillopora damicornis but Not Its Symbiont After Acute Heat Stress. Front. Mar. Sci. 7. doi: 10.3389/fmars.2020.565068
Thornhill D. J., Kemp D. W., Bruns B. U., Fitt W. K., Schmidt G. W. (2008). Correspondence between cold tolerance and temperate biogeography in a western atlantic symbiodinium(dinophyta) lineagei. J. Phycol. 44, 1126–1135. doi: 10.1111/j.1529-8817.2008.00567.x
Tracey S., William C. D., Ove H.-G. (2003). Photosynthetic responses of the coral Montipora digitata to cold temperature stress. Mar. Ecol. Prog. Series 248, 85–97. doi: 10.3354/meps248085
Tuckett C. A., Wernberg T. (2018). High latitude corals tolerate severe cold spell. Front. Mar. Sci. 5. doi: 10.3389/fmars.2018.00014
Tummers B., Green D. R. (2021). The evolution of regulated cell death pathways in animals and their evasion by pathogens. Physiol. Rev. 102, 411–454. doi: 10.1152/physrev.00002.2021
Tunala L. P., Tâmega F. T. S., Duarte H. M., Coutinho R. (2019). Stress factors in the photobiology of the reef coral Siderastrea stellata. J. Exp. Mar. Biol. Ecol. 519, 151188. doi: 10.1016/j.jembe.2019.151188
Wuitchik D. M., Almanzar A., Benson B. E., Brennan S., Chavez J. D., Liesegang M. B., et al. (2021). Characterizing environmental stress responses of aposymbiotic Astrangia poculata to divergent thermal challenges. Mol. Ecol. 30 (20), 5064–5079. doi: 10.1111/mec.16108
Xie C., Mao X., Huang J., Ding Y., Wu J., Dong S., et al. (2011). KOBAS 2.0: a web server for annotation and identification of enriched pathways and diseases. Nucleic Acids Res. 39 (suppl_2), W316–W322. doi: 10.1093/nar/gkr483
Yu X., Huang B., Zhou Z., Tang J., Yu Y. (2017). Involvement of caspase3 in the acute stress response to high temperature and elevated ammonium in stony coral Pocillopora damicornis. Gene 637, 108–114. doi: 10.1016/j.gene.2017.09.040
Yu K., Jiang M. (2004). Latest forty two years’ sea surface temperature change of Weizhou Island and its influence on coral reef ecosystem. Chin. J. Appl. Ecol. 2004 (03), 506–510. doi: 10.13287/j.1001−9332.2004.0110
Yu W., Wang W., Yu K., Wang Y., Huang X., Huang R., et al. (2019). Rapid decline of a relatively high latitude coral assemblage at Weizhou Island, northern South China Sea. Biodiversity Conserv. 28, 3925–3949. doi: 10.1007/s10531-019-01858-w
Yu K.-F., Zhao J.-X., Liu T.-S., Wei G.-J., Wang P.-X., Collerson K. D. (2004). High-frequency winter cooling and reef coral mortality during the Holocene climatic optimum. Earth Planetary Sci. Letters 224, 143–155. doi: 10.1016/j.epsl.2004.04.036
Zhang Y., Chen R.-W., Liu X., Zhu M., Li Z., Wang A., et al. (2022). Oxidative stress, apoptosis, and transcriptional responses in Acropora microphthalma under simulated diving activities. Mar. pollut. Bulletin. 183, 114084. doi: 10.1016/j.marpolbul.2022.114084
Zhang Y., Fernie A. R. (2018). On the role of the tricarboxylic acid cycle in plant productivity. J. Integr. Plant Biol. 60 (12), 1199–1216. doi: 10.1111/jipb.12690
Keywords: relatively high-latitude coral reef, low-temperature stress, coral holobionts, Fv/Fm, apoptosis
Citation: Wei X, Yu K, Qin Z, Chen S, Pan N and Lan M (2024) The acute and chronic low-temperature stress responses in Porites lutea from a relatively high-latitude coral reef of the South China Sea. Front. Mar. Sci. 11:1321865. doi: 10.3389/fmars.2024.1321865
Received: 15 October 2023; Accepted: 23 January 2024;
Published: 15 February 2024.
Edited by:
Aldo Cróquer, The Nature Conservancy, Dominican RepublicReviewed by:
Guoxin Cui, King Abdullah University of Science and Technology, Saudi ArabiaLei Jiang, Chinese Academy of Sciences (CAS), China
Copyright © 2024 Wei, Yu, Qin, Chen, Pan and Lan. This is an open-access article distributed under the terms of the Creative Commons Attribution License (CC BY). The use, distribution or reproduction in other forums is permitted, provided the original author(s) and the copyright owner(s) are credited and that the original publication in this journal is cited, in accordance with accepted academic practice. No use, distribution or reproduction is permitted which does not comply with these terms.
*Correspondence: Kefu Yu, a2VmdXl1QHNjc2lvLmFjLmNu