- Marine Biodiversity Research Group, Department of Biology, Faculty of Science, Ramkhamhaeng University, Bangkok, Thailand
Coastal and marine ecosystems play a major role in the global carbon cycle. Connected marine and coastal ecosystems are commonly observed in the Western Gulf of Thailand. Little is known about the blue carbon potential of these interconnected ecosystems and seascapes. This study aims to quantify blue carbon stocks in the interconnected seagrass-coral reef-sandy coastal ecosystems at Samui Island, the Western Gulf of Thailand. At each study site, the samples of seagrasses, algae, and sediments, were collected from the different zones along a transect of interconnected sandy beach-seagrass bed-coral reef habitats, and the organic carbon contents were quantified using elemental analysis and loss on ignition (LOI). Our findings indicate that the habitats may provide a potential blue carbon opportunity. With a total area of 178.04 hectares (ha), consisting of sand (47.70 ha), seagrass beds (122.44 ha), macroalgal beds (2.40 ha), and live corals (5.50 ha), the estimated carbon storage was as much as 9,222.75 MgC; 74.03% of which was stored in sediment, while the rest was as biomass (25.97%). About 96 percent of the total carbon storage was found in seagrass beds (122.44 ha) with a total amount of carbon storage of 8,876.99 MgC, consisting of 8,781.01 MgC and 95.98 MgC of shallow- and deep-seagrass beds, respectively. The carbon stocks in seagrass, algal biomass, and sediment ranged from 1.58 - 19.10 MgC.ha-1, 2.51 -10.45 MgC.ha-1, and 0.93 - 58.46 MgC.ha-1, respectively. Comparing the carbon storage at each study site, Ko Tan showed the highest value of carbon storage, accounting for 4,232.21 MgC, followed by Ao Phangka (2,901.83 MgC), Ao Thong Tanod (1,459.57 MgC) and Ko Mudsum (629.14 MgC). The quantities of carbon stocks varied considerably among microhabitats and the connectivity of these coastal and marine ecosystems may support the carbon stocks potential of the interconnected ecosystems. Ultimately, the findings from this study provide baseline data that supports Thailand’s nationally determined contribution and highlight the importance of interconnected coastal ecosystems in carbon sequestration and storage that should not be overlooked.
Introduction
Global climate change affects both natural and human systems, making them more vulnerable to climate-related hazards and risks which are expected to be more frequent and intensive. The latest IPCC Assessment Report (AR6) highlights its confidence in the widespread and rapid changes in the climate condition of this planet, leading to the occurrence of climate extremes and adverse impacts. Moreover, long-term impacts are projected revealing the greater magnitudes than currently observed (IPCC and Core Writing Team, 2023). Both terrestrial and marine ecosystems are affected by climate variability leading to ecosystem deterioration, changes in species distribution and abundance, and the quality of ecosystem services (Cheung et al., 2010; Grimm et al., 2013; Oliveira et al., 2022; Venegas et al., 2023). Climate change also generates drastic impacts on humans, for instance, human health impacts due to degraded quality of ecosystem services (Teasdale and Panegyres, 2023), impacts on human activities and livelihoods due to climate extremes such as storms, sea level rise (Cinner et al., 2012; El-Masry et al., 2022), etc.
As climate change impacts have become critical challenges that require immediate and effective action, a global society pays much attention to making several efforts of climate mitigation and adaptation, creating momentum for carbon neutral and net-zero greenhouse gas (GHG) commitments and policies worldwide to haul an increase of the global temperature to 1.5 °C (Elliott et al., 2023; Xu et al., 2023). While the United Nations Framework Convention on Climate Change (UNFCCC) strengthens global collaboration and provides all nations with a global framework for addressing climate change, many countries translated and created their own policies, and strategic and implementation plans for climate mitigation and adaptation (Chan et al., 2022). Various instruments and mechanisms have been recommended e.g., climate technology, decarbonization, carbon capture technology, nature-based solutions, market-based mechanisms, etc. to be applied for relevant sectors along with enhancing cross-sectoral collaboration to achieve climate targets and the Sustainable Development Goals (Kuramochi et al., 2020; Zhou et al., 2020; Kim et al., 2023). For Thailand’s 2nd Updated Nationally Determined Contribution (NDC)1, the government commits to increasing the greenhouse gas (GHG) reduction target from 30% to 40% by 2030 compared to the business-as-usual scenario and will continue putting great efforts to meet the long-term goal of carbon neutrality by 2050 and net-zero GHG emission by 2065. Besides decarbonization, nature-based solutions become one of the emerging tools for climate mitigation, particularly for developing countries because they are cost-effective and provide various co-benefits (Giordano et al., 2020; Seddon et al., 2020; Johnson et al., 2022).
Nature-based solutions were mostly focused on terrestrial ecosystems; currently, the roles of coastal and marine ecosystems have been increasingly discussed as a massive carbon sink, known as ‘blue carbon’, estimated that at least 30% of the anthropogenic atmospheric carbon dioxide can be sequestrated (Macreadie et al., 2019; Duarte de Paula Costa and Macreadie, 2022). Coastal vegetated ecosystems such as mangroves, salt marshes, and seagrass meadows are known as important carbon sinks because they can sequestrate atmospheric carbon dioxide and store carbon in their biomass and sediments (Duarte et al., 2013). Inclusion of macroalgae as a potential blue carbon is still controversial as there is still a large knowledge gap, particularly the understanding of its carbon flux (Krause-Jensen et al., 2018). Some current studies identify macroalgae, particularly kelp forest, as emerging blue carbon which may create a high mitigation impact and research attention on the roles of macroalgae as a blue carbon is growing amongst the scientific community (Kuwae et al., 2022; Howard et al., 2023; Pessarrodona et al., 2023). It is estimated that mangroves store an average of 937 tC.ha-1 with a carbon burial of about 174 gC m-2 year-1 (Alongi, 2012). Seagrass beds could store 19.9 Pg of organic carbon (Fourqurean et al., 2012). The estimation of carbon stocks in the seagrass ecosystems in Southeast Asia revealed that the total carbon storage within seagrass meadows is approximately 121.9 Mg.ha−1 with its capacity to accumulate 5.8 – 6.8 TgC year−1 (Stankovic et al., 2021). Saltmarsh ecosystem can store between 0.4 and 6.5 Pg of organic carbon in the sediments and about 4.8–87.3 TgC is stored in their biomass. (Mcleod et al., 2011; Duarte et al., 2013). However, understanding of their roles and capacities in climate mitigation as well as the uncertainty and reliability of using these blue carbon ecosystems are questionable (Williamson and Gattuso, 2022; Howard et al., 2023). In addition, conservation and restoration of blue carbon ecosystems also provide co-benefits for enhanced biodiversity, increased fisheries resources, improved water quality, and other ecosystem services (Hagger et al., 2022).
While focusing on the aforementioned coastal vegetated ecosystems, coral reefs and other habitats dominated by calcifying organisms seem to have less attention in the context of blue carbon. This might be because of the concern over the instability of the carbon budget of coral reef systems (Ware et al., 1992; Suzuki and Kawahata, 2003). Beside, these habitats contribute to climate change adaptation, through energy dissipation and sediment generation, rather than to climate mitigation. And they can release carbon dioxide through a calcification process, making them a net CO2 source (Lovelock and Duarte, 2019). Actually, the tropical coral reef ecosystem consists of other microhabitats i.e., macroalgal beds, seagrass beds, sediments, etc. that could have some potential opportunities for long-term carbon sequestration and storage. In addition, the study on blue carbon potential should concern the adjacent or associated habitats rather than a standalone single habitat because in some areas these habitats are closely interconnected (Du et al., 2020; Carlson et al., 2021). Ecological connectivity of coastal and marine ecosystems plays a vital role in promoting the viability and sustainability of marine biodiversity and ecosystems as well as the quality of ecosystem services (Barbier, 2017; Balbar and Metaxas, 2019). The dispersal and movement of eggs, spores, larvae and adult individuals of various marine and coastal organisms across populations, communities and ecosystems promote abundance and biodiversity (Fontoura et al., 2022). Moreover, some characteristics of an ecosystem may support the others. For example, coral reefs can support seagrass beds to store carbon by protecting them from strong waves and winds (James et al., 2023) and by contributing to blue carbon storage capacity of adjacent seagrass beds (Guerra-Vargas et al., 2020). In turn, seagrass beds contribute to natural filtration of sediment and nutrients and buffering ocean and coastal acidification for adjacent calcifying algae and corals (Bergstrom et al., 2019; Du et al., 2020).
In Thai waters, there are approximately 238.7 km2 of coral reefs, lying close to the shore of 17 coastal provinces and islands, harboring at least 389 coral species (DMCR, 2023). Some coral reefs in Thailand are associated with other coastal ecosystems, particularly seagrass beds and mangroves. In addition, different types of microhabitats (e.g., sandy substrate, seagrass beds, and macroalgal beds) can be found in/near coral reefs. In Thailand, perhaps similar to other countries, the study of the blue carbon potential of these microhabitats is overlooked and poorly understood. In this regard, we aim to quantify carbon stock within sandy beach-seagrass-coral reef-connected ecosystems in the Western Gulf of Thailand to understand the roles of microhabitats and associated ecosystems in climate mitigation. This is the first assessment of a potential carbon stock in sandy beach-seagrass-coral reef-connected ecosystems in Thailand, providing baseline information for supporting the national climate policies and promoting the restoration and conservation of these ecosystems for climate mitigation.
Materials and methods
Study sites and sampling
Mu Ko Samui is an archipelago located 20 km off the coast of Surat Thani Province, in the western Gulf of Thailand (9°30′N 100°00′E), consisting of 20 islands. Ko Samui and nearby islands are surrounded by coral reefs with a total area of 30.9 km2. The coral reef status is quite poor with a low portion of live coral coverage. Seagrass beds are mostly found along the western and southern parts with a total area of 8.02 km2. Four seagrass species are found at the study sites including Enhalus acoroides, Halodule uninervis, Halophila minor, and Halophila ovalis (DMCR, 2023). Six genera of macroalgae genus Caulerpa, Halimeda, Padina, Turbinaria, Gracilaria and Laurencia were reported (Mayakun and Prathep, 2005). The coral reef ecosystems that have diverse microhabitats such as seagrass beds and macroalgae growing on coral rubbles and dead corals were selected for this study, including four study sites: Ao Phangka, Ao Thong Tanod, Ko Tan, and Ko Mudsum (Figure 1).
For each study site, sampling of biomass and sediments were conducted in the following zones (Figure 2): 1) sandy zone A, which is located in the intertidal zone between the shoreline and shallow seagrass beds with a depth of less than 0.5 m; 2) shallow seagrass zone, which is an area of seagrass beds, located in the intertidal zone with a depth range of 0.5 – 1 m; 3) sandy zone B, which is located between the shallow seagrass beds and macroalgae zone with a depth range of 1 – 1.5 m; 4) macroalgal zone, which is an area of macroalgae growing on coral rubbles and dead corals with a depth range of 1.5 – 2 m; 5) live coral zone, which mostly consists of live corals, located next to the macroalgae zone with a depth range of 2 – 2.5 m; 6) deep seagrass zone, which is an area of seagrass beds, located next to the reef zone with a depth range of 2.5 – 3 m; and 7) sandy zone C, which is sandy substrate located at the outermost zone, next to the deep macroalgae zone, with a depth range of 4 – 5 m. The size of each zone was estimated based on the GIS maps of marine and natural resources of the Department of Marine and Coastal Resources incorporating with the findings obtained from the underwater surveys at the study sites.
A stratified randomized sampling method was applied for each zone and study site. For seagrass and macroalgal studies, at least 5 quadrats (0.5× 0.5 m) were randomly placed on three plots of seagrass beds and macroalgal beds in order to estimate seagrass and macroalgal cover (%) and species composition. For biomass sampling, the quadrats with more than 75% coverage were used for collecting seagrass and algal samples. The total area was 0.25 m2 for each quadrat. Seagrass samples were taken to 15–20 cm depth or more depending on whether the species has a vertical rhizome or root system. The samples were rinsed in freshwater, and each part of the seagrass was separated into leaves (above-ground part) and rhizome and root (below-ground part). Macroalgal samples were collected from coral rubbles and dead corals. All samples were stored in labeled plastic bags and kept in an ice box to maintain the temperature below 4°C until further processing in the laboratory.
For each zone, sediment samples were collected using cores pushed down to 20 cm depth from each zone. Core sampling was carried out using acrylic pipes (100 cm long, 60 mm inner diameter). The sediment cores were placed in Zip-lock® plastic bags to prevent contamination. The samples were placed in an icebox to maintain the temperature below 4°C and transported to the laboratory, for further analysis.
Laboratory procedures
Samples from each species of seagrass were separated into above (leaves) and below ground (roots and rhizomes) parts, calcareous epiphytes present in the seagrass and macroalgal biomass were removed by submerging them in 10% HCl, followed by washing with distilled water in the laboratory. The samples were oven-dried at 60 °C for 48–72 hrs until they reached a constant weight. The dry weight of the above and below ground parts was recorded and total biomass for each species was calculated as well as for each vegetative part. Seagrass and macroalgal samples were crushed into powder and 20 mg of subsamples were sent for percentage of organic carbon analysis with a CHN analyzer. Loss on Ignition (LOI) was used to determine the organic carbon of each sediment sample. Prior to ignition, sediment samples were oven-dried at 60 °C for 48–72 hrs and then combusted in a muffle furnace at a temperature of 550 °C for 6 hrs (Heiri et al., 2001).
Determination of organic carbon content and carbon storage
Carbon content of biomass can be directly interpreted from the percentage of organic carbon in the samples obtained from the elemental analysis and it was corrected by subtracting inorganic carbon content of ash. For sediment, the results from the loss on ignition method mean the loss of organic matter (e.g., carbon, hydrogen, nitrogen, oxygen, sulfur, etc.). Thus, the percentage of organic carbon of seagrass sediment can be determined using a relationship between the percentages of loss on ignition and organic carbon as the following equations: if % LOI < 0.2, %Corg = 0.40×%LOI – 0.21 (r2 = 0.87); if % LOI > 0.2, %Corg = 0.43×%LOI – 0.33 (r2 = 0.96) (Fourqurean et al., 2012). Carbon storage of each species of seagrasses and macroalgae was determined by multiplying the biomass and carbon content. Ultimately, the total carbon storage of all microhabitats for each study site was estimated using the multiplication of calculated carbon storage of seagrasses, macroalgae, sediments collected from each zone and the total area of each seagrass beds, macroalgal beds and sandy substrates (Table 1).
Data analyses
All data were checked for normality and homogeneity of variances. When assumptions were not met the data were log10 or log10 (x+1) transformed. One-way Analysis of Variance was used to test differences in the mean percentage of organic carbon content and mean biomass for each seagrass species and each study site. The differences in percentage of organic carbon content and biomass for each algal species and for each study site were tested using t-test and one-way ANOVA, respectively. Similarly, one-way ANOVA was performed to test the variation of bulk density, organic carbon content and carbon storage for each zone and each station. Tukey’s Honest Significant Difference (HSD) tests were used to determine significant differences between pairs. All statistical analyses were performed using R version 4.3.1.
Results
Microhabitats and associated ecosystems
Coral reefs at our study sites were found next to shallow seagrass beds. For Ao Thong Tanod and Ko Tan, seagrass beds can be found in the intertidal zone and in the deep zone, located seaward next to the coral reefs, while Ao Phangka and Ko Mudsum had no deep seagrass beds. At all study sites, macroalgal beds were found on coral rubbles and dead corals. Also, organic carbon could be possibly stored in sediments in all zones (Figures 1, 3).
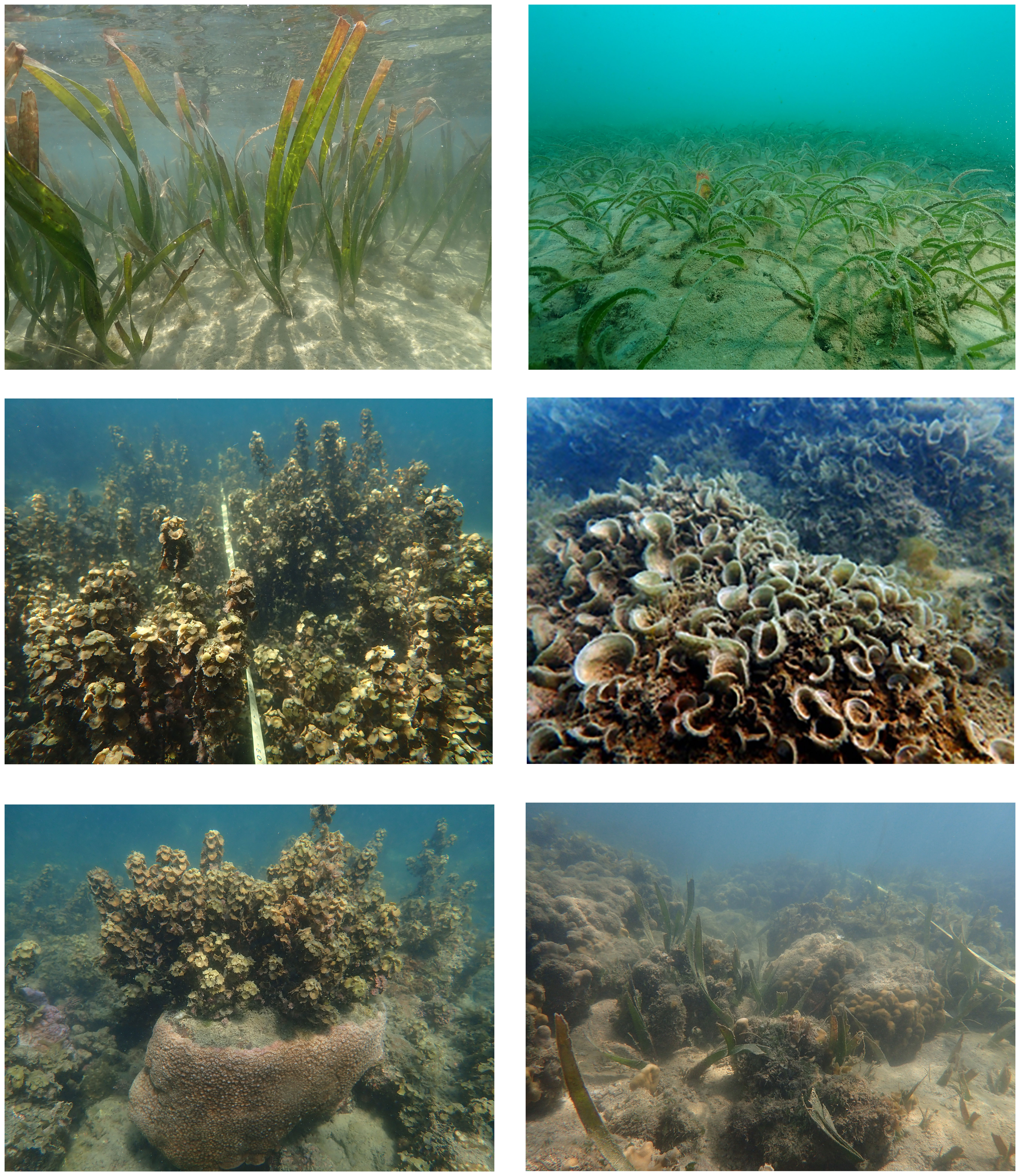
Figure 3 Underwater photographs showing some seagrasses and macroalgal microhabitats at the study sites.
The estimated area of seagrass beds ranged from 8.16 - 57.6 ha and 0.40 - 2.30 ha for shallow and deep seagrass beds, respectively. Enhalus acoroides and Halodule uninervis were found at most study sites except Ko Mudsum. The seagrass beds at Ko Mudsum were dominated by Halophila ovalis with a little proportion of Halophila minor. H. ovalis was found with a small proportion at Ao Thong Tanod, while disappearing at Ao Phangka and Ko Tan. The means of seagrass coverage ranged from 45.34 - 65.14%. The high means of seagrass coverage were found at Ko Tan and Ao Thong Tanod and the coverage varied spatially across study sites (F= 6.786, p=0.0024). The mean seagrass coverage at Ko Tan was significantly different from those at Ao Phangka (p<0.05) and Ko Mudsum (p<0.05), but similar to those at Ao Thong Tanod (p>0.05) (Figure 4).
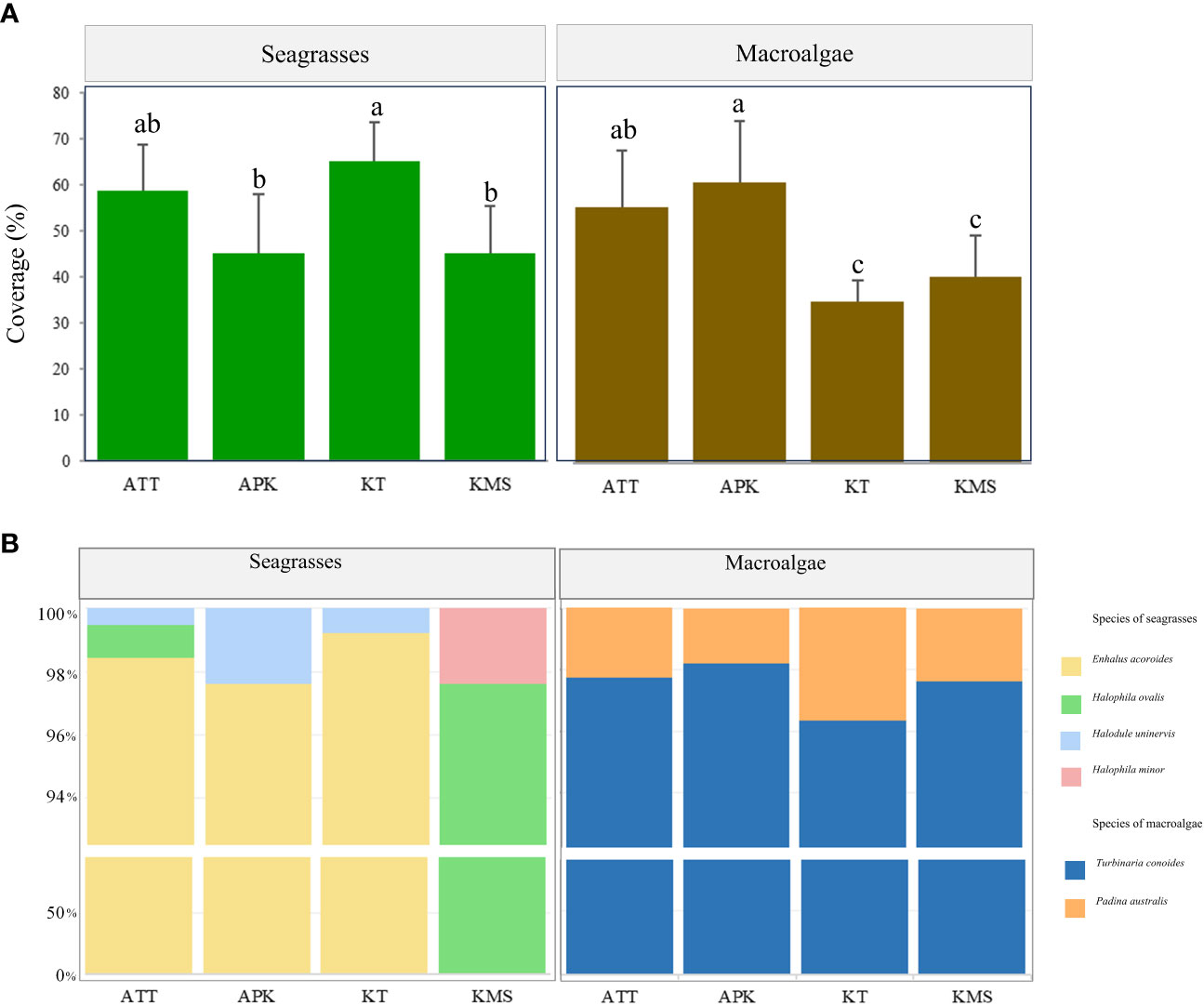
Figure 4 Coverage (A) and species composition (B) of seagrasses and macroalgae at each study site (Remarks: ATT, Ao Thong Tanod; APK, Ao Phangka; KT, Ko Tan; KMS, Ko Mudsum; Different letters on the (A) indicate statistically significant differences, p<0.05).
Macroalgal beds were found on coral rubbles and dead corals at all study sites with the range of estimated area of 0.40 - 1.00 ha, consisting of two algae: Turbinaria conoides and Padina australis. T. conoides was a dominant algal species at all study sites. The mean coverage of macroalgal beds ranged from 35.07 – 60.88%. The highest coverage was found at Ao Phangka, followed by Ao Thong Tanod, Ko Mudsum, and Ko Tan. The coverages of macroalgal beds varied spatially across study sites (F= 10.464, p=0.00023). The means of macroalgal coverage at Ao Phangka and Ao Thong Tanod were significantly different from those at Ko Tan (p<0.05) and Ko Mudsum (p<0.05) (Figure 4).
Carbon storage in seagrass beds
In this study, we found that the average biomass of seagrasses ranged from 76.65 - 572.31 g-DWm-2 with an average biomass of 283.10 ± 36.02 g-DWm-2. The highest average biomass was found at Ao Thong Tanod (558.97 ± 69.08 g-DWm-2), followed by Ao Phangka (572.314 ± 107.82 g-DWm-2), Ko Tan (529.55 ± 71.31 g-DWm-2), and Ko Mudsum (76.65 ± 33.84g-DWm-2). No spatial variation in the biomass of seagrasses was found among study sites (F= 1.334, p=0.285). However, the biomass of seagrasses at all study sites varied considerably among seagrasses (p<0.05). Overall, Enhalus acoroides showed the highest average biomass (553.61 ± 2.03 g-DWm-2), while Halophila ovalis had the lowest one (90.17 ± 2.19 g-DWm-2) (Figure 5). All seagrasses had a higher below-ground biomass than above-ground biomass (Figure 6). Overall, the average carbon content of seagrasses ranged from 6.66 – 33.41% of biomass with an average carbon content of 21.49 ± 2.75%. The highest average carbon content was found in E. acoroides (31.93 ± 0.27%), followed by Halophila minor (24.34 ± 3.00%), Halodule uninervis (20.96 ± 0.24%), H. ovalis (10.63 ± 0.57%). No spatial variation in the carbon content of seagrass was found among study sites (F= 0.352, p=0.788). The average carbon content of E. acoroides was significantly different from those of H. uninervis (p<0.01), H. ovalis (p<0.01), and H. minor (p<0.01). Carbon storage in seagrass biomass can be calculated based on the biomass and carbon content in seagrasses, revealing the carbon storage in seagrass biomass ranged from 1.58 - 19.10 MgC.ha-1 with an average total carbon storage of 7.5± 0.95 MgC.ha-1. E. acoroides had the highest average carbon storage (18.68 ± 0.19 MgC.ha-1), followed by H. minor (6.86 ± 0.85 MgC.ha-1), H. ovalis (2.04 ± 0.04 MgC.ha-1), and H. uninervis (1.99 ± 0.04 MgC.ha-1). (Figure 5).
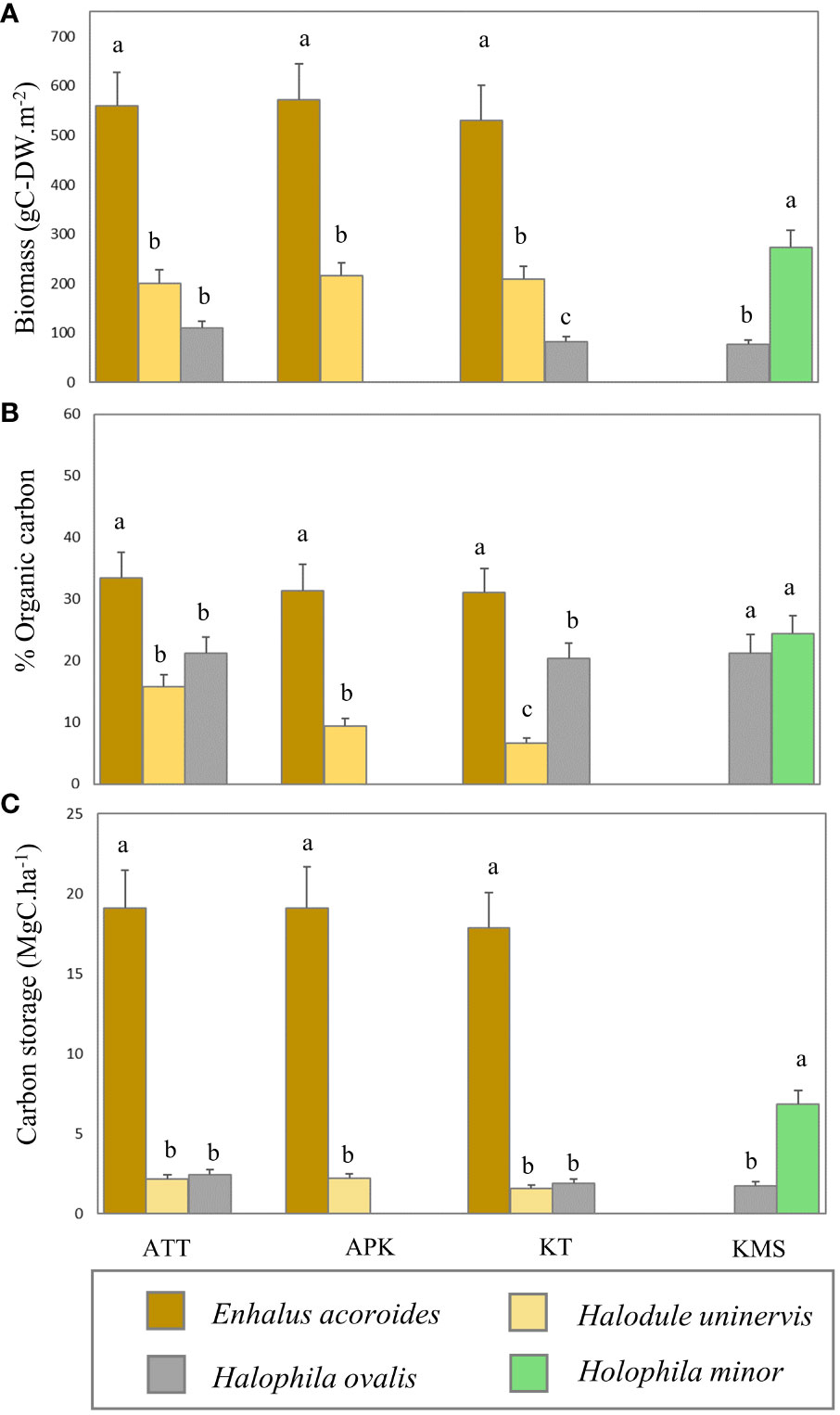
Figure 5 Variation of biomass (A), organic carbon content (B), and carbon storage (C) in different seagrass species (ATT, Ao Thong Tanod; APK, Ao Phangka; KT, Ko Tan; KMS, Ko Mudsum). (Different letters indicate statistically significant differences, p<0.05).
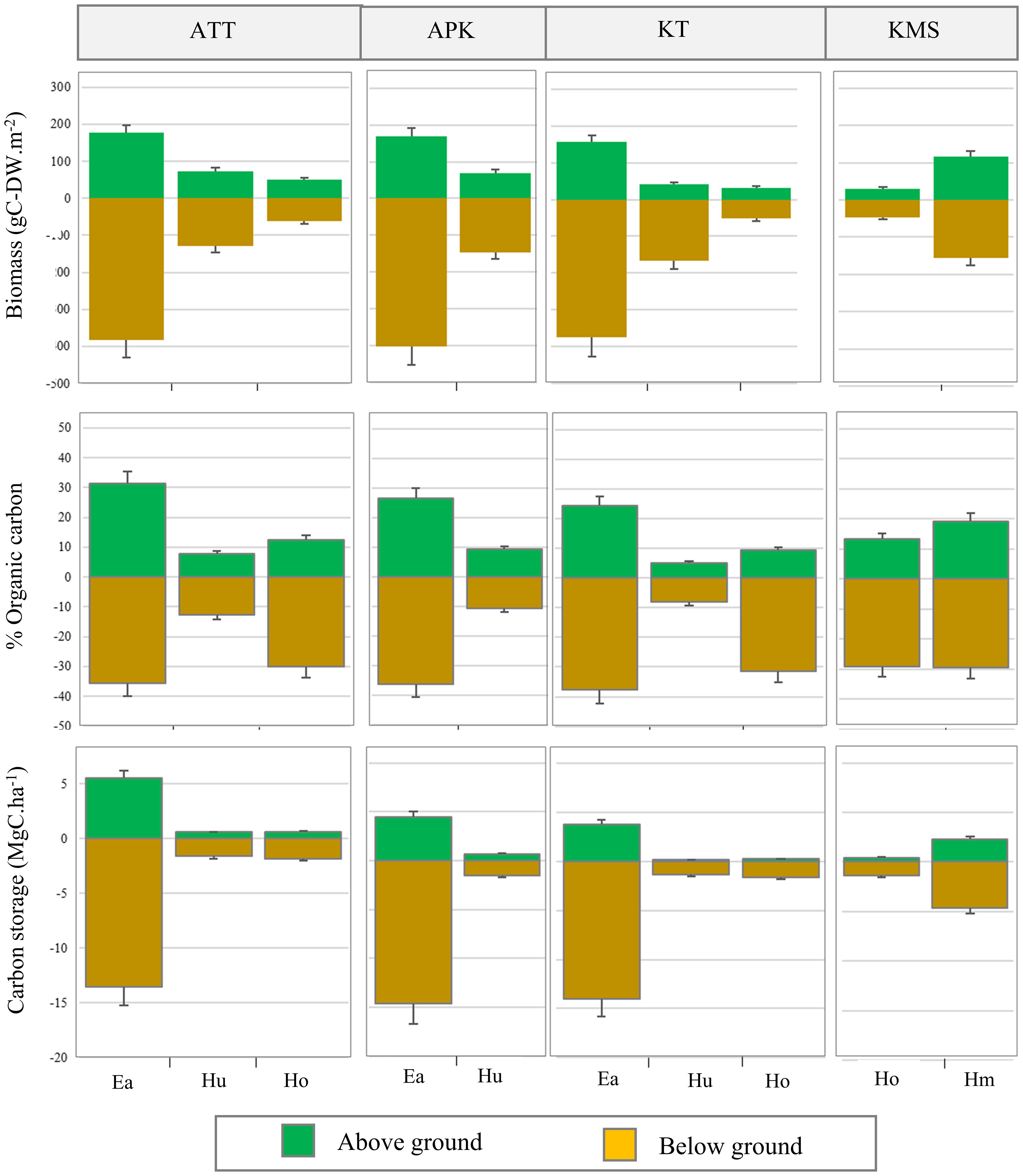
Figure 6 Above-ground and below-ground biomass, organic carbon content, and carbon storage in different seagrass species (ATT, Ao Thong Tanod; APK, Ao Phangka; KT, Ko Tan; KMS, Ko Mudsum).
Carbon storage in macroalgal beds
Macroalgal beds had their biomass range of 209.32 - 414.84 g-DWm-2 with an average biomass of 302.23 ± 38.57 g-DWm-2. The highest average biomass was found at Ko Mudsum (414.83 ± 51.27 g-DWm-2), followed by Ao Thong Tanod (377.69 ± 46.68 g-DWm-2), Ko Tan (353.34 ± 43.56 g-DWm-2), and Ao Phangka (339.14 ± 47.46 g-DWm-2). The mean macroalgal biomass at Ko Tan was different from those at the other study sites (F=7.608, p=0.001). Overall, Turbinaria conoides had the higher biomass (371.25 ± 47.25 g-DWm-2), compared with Padina australis having the lower one (233.21 ± 29.89 g-DWm-2). Overall, the carbon content of macroalgae ranged from 10.34 - 27.68% of biomass with an average carbon content of 17.88 ± 2.26%. The highest average carbon content was found in T. conoides (22.94 ± 0.67%) and P. australis had an average carbon content of 12.82 ± 0.36%. No spatial variation in carbon content was found among study sites (F= 2.239, p=0.115). Nevertheless, the carbon content between T. conoides and P. australis was significantly different (t= 6.229, p <0.001). In terms of carbon storage, macroalgal biomass can store the carbon in a range of 2.51 -10.45 MgC.ha-1 with an average carbon storage of 5.71 ± 0.72 MgC.ha-1. T. conoides showed the highest average carbon storage (8.46 ± 0.18 MgC.ha-1) and P. australis can store the carbon of 2.95 ± 0.05 MgC.ha-1 (Figure 7).
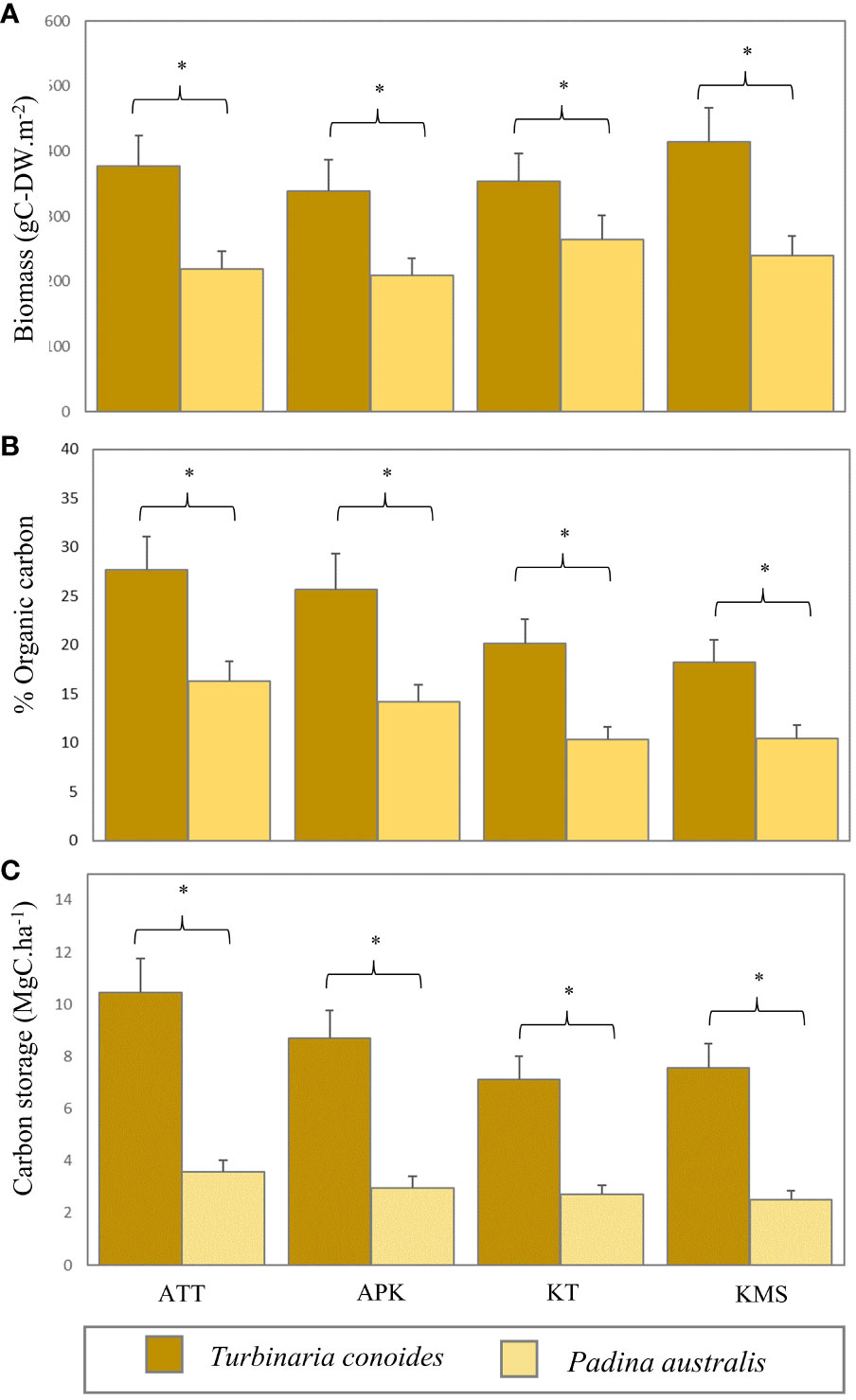
Figure 7 Biomass (A), organic carbon content (B), and carbon storage (C) of Turbinaria conoides and Padina australis in each study site (ATT, Ao Thong Tanod; APK, Ao Phangka; KT, Ko Tan; KMS, Ko Mudsum) (* denotes significant difference, p<0.05).
Carbon storage in sediments
The dry bulk densities of sediment obtained from all zones and study sites ranged from 0.16 – 0.54 g.cm-3 with an average dry bulk of 0.364 ± 0.05 g.cm-3. The highest dry bulk was found at Ko Tan (0.43 ± 0.05 g.cm-3), followed by Ao Thong Tanod (0.35 ± 0.77 g.cm-3), Ao Phangka (0.34 ± 0.03 g.cm-3), and Ko Mudsum (0.33 ± 0.05 g.cm-3). The dry bulk density of sediment at all study sites varied among study sites and zones (F= 2.970, p=0.037). Sediment samples collected from the sandy zone A and sandy zone B, and deep seagrass zones tend to have a higher bulk density. Overall, the carbon content of sediment ranged from 0.59 – 9.11% of dry sediments with an average carbon content of 2.36 ± 0.31%. The highest carbon content was found in the sediments collected from shallow seagrass zone (6.60 ± 0.65%), followed by deep seagrass zone (3.46 ± 0.32%), macroalgal zone (3.01 ± 0.50%), live coral zone (1.75 ± 0.28%), sandy zone C (0.81 ± 0.07%), sandy zone B (0.76 ± 0.7%), and sandy zone A (0.71 ± 0.06%).
The quantity of carbon stored in sediment samples ranged from 0.93 – 58.46 MgC.ha-1 with an average total carbon storage of 20.36 MgC.ha-1. The highest average carbon storage was found at Ao Thong Tanod (23.89 MgC.ha-1), followed by Ko Tan (20.69 MgC.ha-1), Ko Mudsum (18.39 MgC.ha-1), and Ao Phangka (17.81 MgC.ha-1). Seagrass and macroalgae provided the highest contribution to the amount of carbon in sediment. The sediments with the highest carbon storage were found in shallow seagrass zone (55.04 MgC.ha-1), followed by deep seagrass zone (40.23 MgC.ha-1), macroalgal zone (31.26 MgC.ha-1), live coral zone (16.46 MgC.ha-1), sandy zone C (3.61 MgC.ha-1), sandy zone B (3.47 MgC.ha-1), Sandy zone A (2.38 MgC.ha-1) (Figure 8).
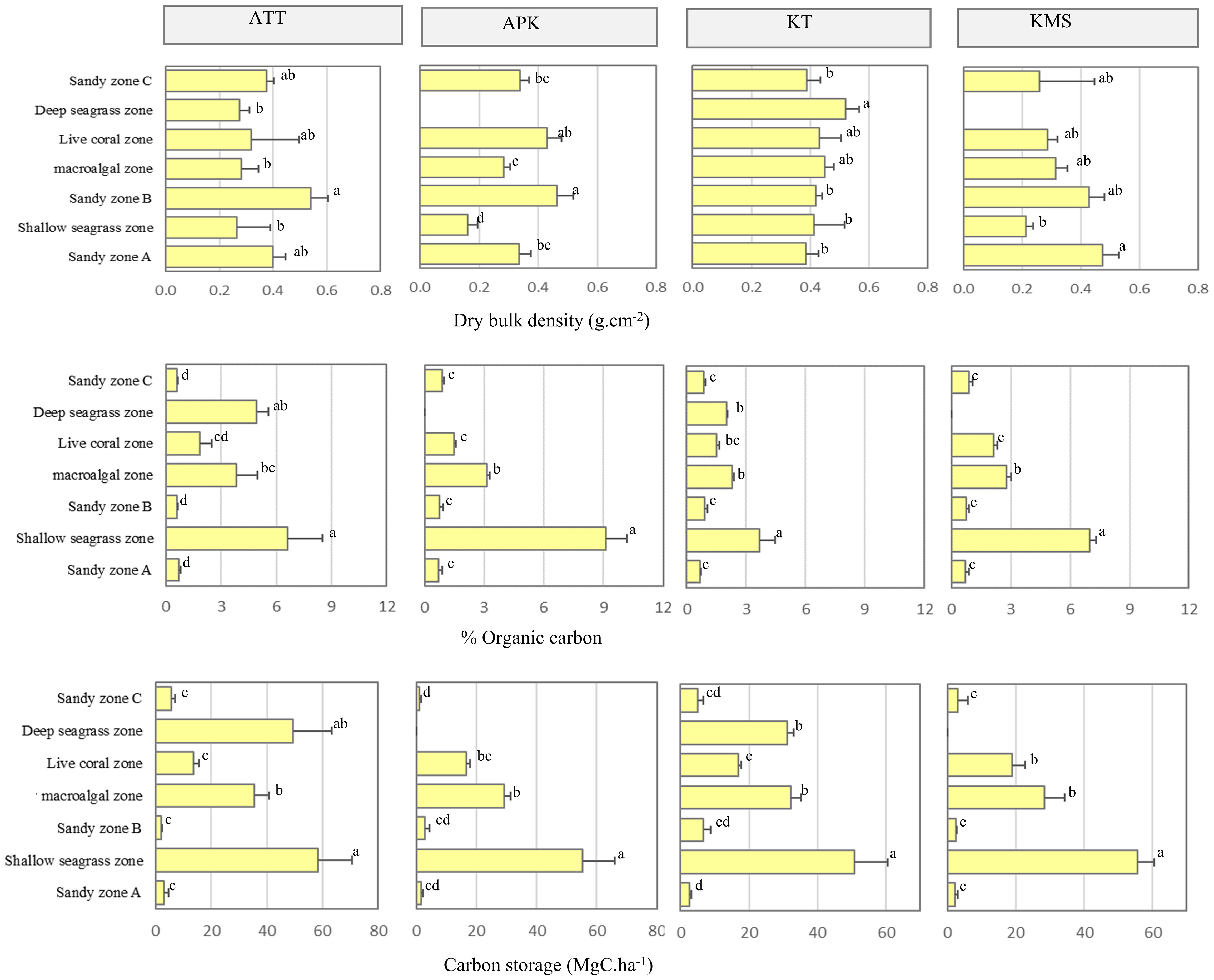
Figure 8 Variation of bulk density, organic carbon content, and carbon storage in sediments obtained from different zones and study sites (ATT, Ao Thong Tanod; APK, Ao Phangka; KT, Ko Tan; KMS, Ko Mudsum) (Different letters indicate statistically significant differences, p<0.05).
Total carbon storage
In this section, the total carbon storage of each zone and study site was calculated using the carbon storage in biomass (both above- and below-ground) and the estimated total areas of each zone/microhabitat. Overall, the four study sites (total area = 178.04 hectares) could store as much as 9,222.75 MgC, 74.03% (6,827.49 MgC) of which was stored in sediment, while the rest was as biomass (2,395.26 MgC, 25.97%). About 96 percent of the total carbon storage was found in seagrass beds (122.44 hectares) with a total amount of carbon storage of 8,876.99 MgC, consisting of 8,781.01 MgC and 95.98 MgC of shallow- and deep-seagrass beds, respectively. The portion of carbon stored in seagrass biomass and in sediment between shallow seagrass beds (Biomass: Sediment = 1:2.7) and deep-seagrass beds (Biomass : Sediment = 1:18.9) was quite different. The carbon store in seagrass biomass tended to be higher in shallow seagrass beds. About 2.40 hectares of macroalgal beds were found in the coral reefs at four study sites, having a carbon storage of 102.54 MgC; of which, 73.91% was stored in sediment and the rest was in algal biomass. Sediments in coral reefs had a higher carbon storage (98.33 MgC) than those in the sediment samples collected from sandy zones A, B, or C, which had a range of carbon storage of 34.8 – 56.88 MgC. Comparing the carbon storage of each study site, Ko Tan showed the highest value of carbon storage, accounting for 4,232.22 MgC, followed by Ao Phangka which was the second-richness of carbon storage with a total storage of 2,105.60 MgC, Ao Thong Tanod (1,459.57 MgC) and Ko Mudsum (629.15 MgC). These were mainly contributed by the availability of shallow seagrass beds (Table 2 and Figure 9).
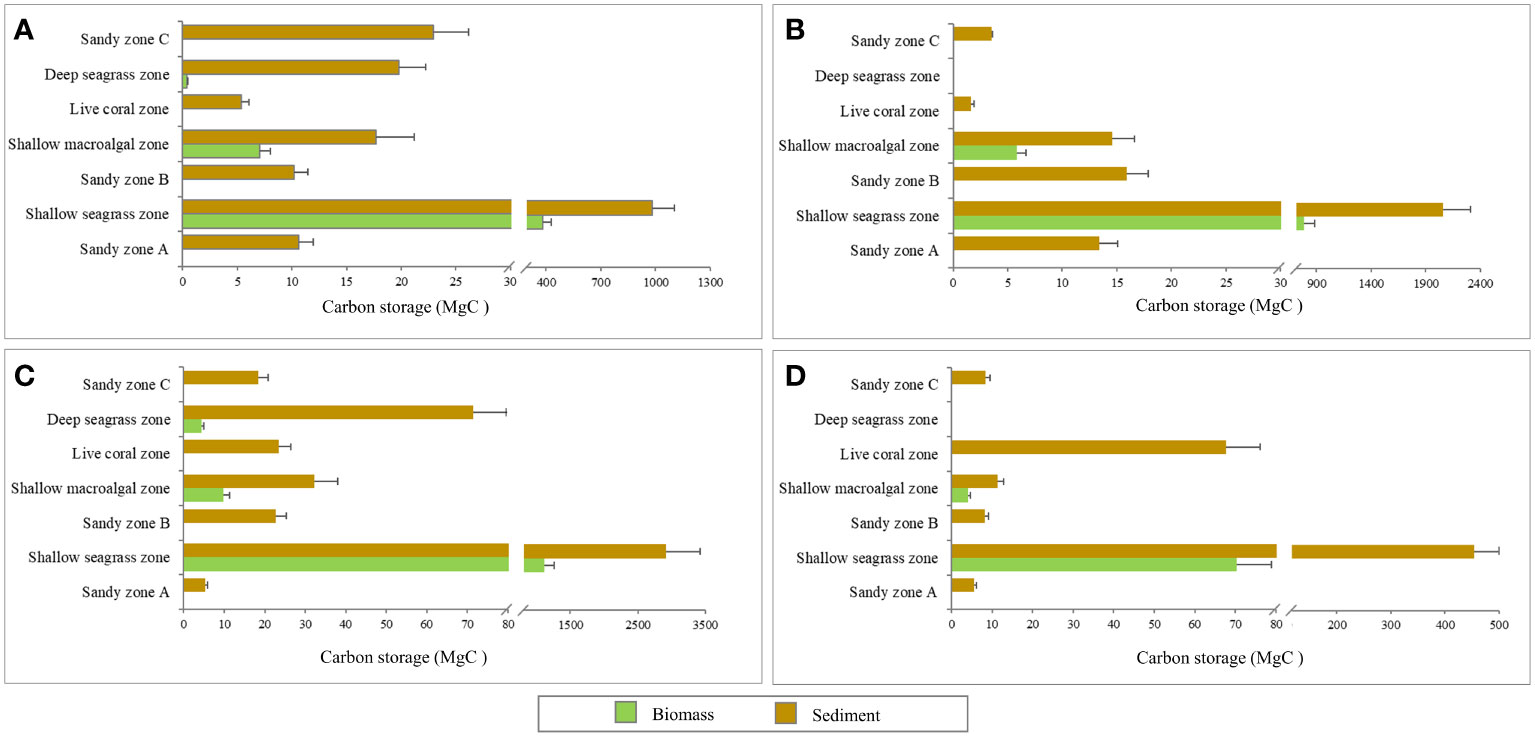
Figure 9 Estimated total carbon storage in different zones and study sites, Ao Thong Tanod (A), Ao Phangka (B), Ko Tan (C), and Ko Mudsum (D).
Discussion
This study quantifies the carbon storage in microhabitats and associated ecosystems in/near coral reefs in the Western Gulf of Thailand. To respond to the objective, four study sites with diverse microhabitats, specifically seagrass and algal beds, were selected to reflect their blue carbon potential. Seagrass beds are the major habitat contributing microhabitat functions to the integrity of the coral reef ecosystem, (Du et al., 2020; Nanami, 2022) and at the same time, the coral reefs also enhance seagrass beds in terms of their blue carbon function (Guerra-Vargas et al., 2020). Our surveys indicated that seagrass coverage ranged from 45.34 to 65.14% with four seagrass species found at the study sites, which corresponds to what was reported by DMCR (2023). For macroalgae, Mayakun and Prathep (2005) reported that four genera of macroalgae genus Caulerpa, Halimeda, Padina, Turbinaria, Gracilaria and Laurencia were found at Ko Samui and nearby islands but only two of which (i.e., Padina, Turbinaria) were found at our study sites. Klomjit et al. (2021) reported that 15 species were found on some degraded reefs at Ko Samui with a coverage of 37 – 59%. These macroalgae generally grow on coral rubbles and dead corals. They interact with corals, affecting reef stability (Eggertsen et al., 2021). Some macroalgae, for instance, Lobophora has interaction with Porites corals, but little is known about its interaction (Klomjit et al., 2022). Macroalgae are also important sources of organic carbon in coral reef ecosystems (Naumann et al., 2012) and may be the opportunities for carbon storage (Krause-Jensen and Duarte, 2016).
In this study, we found that, with constant coverage, the variation of seagrass biomass is influenced by seagrass species, but it does not vary spatially. Several studies indicate that different species of seagrass yield different amounts of biomass (Duarte et al., 1998; Huang et al., 2006). Additionally, seagrass biomass can be varied through the different substrates. The biomass of seagrasses growing on muddy substrate tended to be around three times biomass greater than that on sandy substrate (Prathep et al., 2010). In this study, we found that three seagrasses i.e., Enhalus acoroides, Halophila ovalis, and Holophila minor, had a higher below-ground biomass than above-ground biomass, except Halodule uninervis that had a higher above-ground biomass. Higher below-ground biomass is observed in most seagrass species because they can store reserve energy for their metabolism and growth during the unsuitable condition for growth e.g., limited light and below-ground biomass can resist mortality from physical disturbance (McMahon et al., 2013; Collier et al., 2021). Anyway, the greatest factor affecting the loss of seagrass biomass is that the change in coverage due to natural and anthropogenic disturbances as well as water pollution. These can cause impacts on epifaunal and infaunal communities and carbon concentration in the sediment (Stallings et al., 2014; Githaiga et al., 2019; Herrera et al., 2022).
Based on our study, the seagrass ecosystem can store carbon as much as 19.10 MgC.ha-1 of biomass, the amount of carbon in sediment is about 74% and the rest is stored in seagrass biomass. The proportion of sedimentary organic carbon and biomass is relatively low compared with other studies. This may be because of the characteristics of the substrate which is sandy and located far from sediment sources. Muddy sand has higher organic carbon storage than sand and mud (Wang et al., 2021). Seagrass beds located close to mangroves or river mouths tend to have higher organic carbon than those located offshore. Generally, the carbon content in seagrass sediments is influenced by the structural complexity of seagrasses, water quality particularly turbidity, water depth, and wave height (Adams et al., 2016; Samper-Villarreal et al., 2016). In this study, we also found that the sedimentary carbon in shallow seagrass beds is about two times higher than that in deeper seagrass beds. Also, the deeper seagrass beds are relatively small and patchy compared with the shallow ones and they are located next to coral reefs, more exposed to wave action, resulting in lower sedimentary carbon in deep seagrass beds. This variation could be influenced by several factors e.g., the distance from the shoreline, landscape configuration, and sediment characteristics (Diesing et al., 2017; Ricart et al., 2017).
Macroalgal beds are one of the main primary producers and also play important roles in the biogeochemical cycling of carbon in coral reef ecosystems and become one of the nature-based climate solutions (Krause-Jensen et al., 2018). Based on our findings, as much as 5.71 and 31.26 MgC.ha-1, were stored as biomass and in sediment, respectively, reflecting the great potential for blue carbon. Macroalgae are still the contributors to the carbon sequestration in sediments in reef systems and open waters (Pessarrodona et al., 2023). However, overgrown macroalgae in the reef system may reflect reef degradation as it is usually found on dead corals and coral rubbles, which could be resulted from mass coral bleaching events and prolonged exposure to sediment stress (Sutthacheep et al., 2013; Yeemin et al., 2013). In addition, some corals can be affected by macroalgal competition, affecting coral growth, recruitment, photosynthesis, etc. (Clements et al., 2020; Rölfer et al., 2021), leading to the overall stability of the reef system (Yeemin et al., 2022). The amount of macroalgal biomass can be changed as they are generally controlled by herbivores (Adam et al., 2015).
Regarding our estimation, the four study sites could store as much as 9,222.76 MgC, with a major contribution from seagrass beds, showing a great blue carbon potential. It is important to emphasize that the microhabitats in coral reef ecosystems, particularly seagrass beds and algal beds, and other associated ecosystems e.g. sandy substrates provide a co-creation for climate change mitigation and resilience. Ecosystem functions of each connected ecosystems provide benefits to each other. Seascape connectivity facilitates exchange of carbon and other elements and materials across coastal ecosystems through the flow of particulate organic matter (Saavedra-Hortua et al., 2023). Seagrass beds in/near coral reefs receive benefits from coral reefs by being protected from wind and waves and they gain organic sediments from corals and other organisms living in the coral reefs (Guerra-Vargas et al., 2020). Sandy bottoms and beaches, even with no vegetation, are also important areas for carbon stock, particularly undisturbed ones (Phang et al., 2015). Macroalgae are also the sources of carbon for coastal and ocean systems, macroalgal biomass tend to be exported into near-shore sediments. The spatial distribution of the biomass and total organic carbon (TOC), are influenced by several physical environmental factors and the extent of the infralittoral zone around depositional areas (Erlania et al., 2023). These illustrate that associated coastal and marine ecosystems are highly connected. Thus, the assessment of the blue carbon potential may need to be considered as a whole connected systems rather than a standalone ecosystem.
The conservation and protection of these connected ecosystems should be made to maintain their regulating and other services (York et al., 2018; Nanami, 2022). Area-based conservation such as marine protected areas helps maintain both the ecosystem health and organic carbon stored in seagrass ecosystem (Reyes et al., 2022). In addition, seagrass restoration can be one of the nature-based solutions for climate actions and carbon offsetting (Kuwae et al., 2022). Several studies show that the restoration of blue carbon ecosystems provides great benefits and monetizes carbon storage value (Greiner et al., 2013; Qu et al., 2023). As the study sites are well-known marine tourism destinations, it is a good opportunity to engage the tourism sector by embedding restoration activities into tourism packages, supporting carbon-neutral tourism. Besides, blue carbon ecosystems can be a source of carbon offsetting for those tour operators to compensate for tourism carbon footprint (Gössling et al., 2023). Additionally, it is vital to have coral reef restoration measures and plans to mitigate threats and local stressors that cause the deterioration of coral reefs and other associated ecosystems (Suraswadi and Yeemin, 2013) to support coral reef recovery and resilience (Sutthacheep et al., 2022). Ultimately, the findings from this study provide baseline data that supports Thailand’s nationally determined contribution and highlights the importance of coral reefs and associated ecosystems in carbon sequestration and storage that should not be overlooked.
Data availability statement
The raw data supporting the conclusions of this article will be made available by the authors, without undue reservation.
Author contributions
TY: Conceptualization, Formal analysis, Methodology, Validation, Writing – original draft, Writing – review & editing, Funding acquisition, Project administration, Supervision. MS: Conceptualization, Formal analysis, Methodology, Project administration, Supervision, Writing – original draft, Writing – review & editing. SP: Data curation, Formal analysis, Investigation, Methodology, Visualization, Writing – original draft. WK: Data curation, Formal analysis, Investigation, Methodology, Visualization, Writing – original draft. CC: Data curation, Formal analysis, Investigation, Methodology, Visualization, Writing – original draft. WS: Conceptualization, Data curation, Formal analysis, Investigation, Methodology, Software, Validation, Writing – original draft, Writing – review & editing.
Funding
The author(s) declare financial support was received for the research, authorship, and/or publication of this article. This research was supported by Thailand Science Research and Innovation (TSRI), the National Science, Research and Innovation Fund (NSRF) by the Program Management Unit Competitiveness (PMUC), and Ramkhamhaeng University (RU) (Grant number: C10F650250).
Acknowledgments
We are most grateful to the staff of Marine Biodiversity Research Group, Department of Biology, Faculty of Science, Ramkhamhaeng University, for their support and assistance in the field. A great attitude must be expressed to local communities and tour operators, who provided valuable local information for research planning.
Conflict of interest
The authors declare that the research was conducted in the absence of any commercial or financial relationships that could be construed as a potential conflict of interest.
Publisher’s note
All claims expressed in this article are solely those of the authors and do not necessarily represent those of their affiliated organizations, or those of the publisher, the editors and the reviewers. Any product that may be evaluated in this article, or claim that may be made by its manufacturer, is not guaranteed or endorsed by the publisher.
Footnotes
References
Adam T. C., Burkepile D. E., Ruttenberg B. I., Paddack M. J. (2015). Herbivory and the resilience of Caribbean coral reefs: knowledge gaps and implications for management. Mar. Ecol. Prog. Ser. 520, 1–20. doi: 10.3354/meps11170
Adams M. P., Hovey R. K., Hipsey M. R., Bruce L. C., Ghisalberti M., Lowe R. J., et al. (2016). Feedback between sediment and light for seagrass: Where is it important? Limnology Oceanography 61 (6), 1937–1955. doi: 10.1002/lno.10319
Alongi D. M. (2012). Carbon sequestration in mangrove forests. Carbon Manage. 3 (3), 313–322. doi: 10.4155/cmt.12.20
Balbar A. C., Metaxas A. (2019). The current application of ecological connectivity in the design of marine protected areas. Global Ecol. Conserv. 17, e00569. doi: 10.1016/j.gecco.2019.e00569
Barbier E. B. (2017). Marine ecosystem services. Curr. Biol. 27 (11), R507–R510. doi: 10.1016/j.cub.2017.03.020
Bergstrom E., Silva J., Martins C., Horta P. (2019). Seagrass can mitigate negative ocean acidification effects on calcifying algae. Sci. Rep. 9 (1), 1932. doi: 10.1038/s41598-018-35670-3
Carlson R. R., Evans L. J., Foo S. A., Grady B. W., Li J., Seeley M., et al. (2021). Synergistic benefits of conserving land-sea ecosystems. Global Ecol. Conserv. 28, e01684. doi: 10.1016/j.gecco.2021.e01684
Chan S., Hale T., Deneault A., Shrivastava M., Mbeva K., Chengo V., et al. (2022). Assessing the effectiveness of orchestrated climate action from five years of summits. Nat. Climate Change 12 (7), 628–633. doi: 10.1038/s41558-022-01405-6
Cheung W. W. L., Lam V. W. Y., Sarmiento J. L., Kearney K., Watson R., Zeller D., et al. (2010). Large-scale redistribution of maximum fisheries catch potential in the global ocean under climate change. Global Change Biol. 16 (1), 24–35. doi: 10.1111/j.1365-2486.2009.01995.x
Cinner J. E., McClanahan T. R., Graham N. A. J., Daw T. M., Maina J., Stead S. M., et al. (2012). Vulnerability of coastal communities to key impacts of climate change on coral reef fisheries. Global Environ. Change-Human Policy Dimensions 22 (1), 12–20. doi: 10.1016/j.gloenvcha.2011.09.018
Clements C. S., Burns A. S., Stewart F. J., Hay M. E. (2020). Seaweed-coral competition in the field: effects on coral growth, photosynthesis and microbiomes require direct contact. Proc. R. Soc. B: Biol. Sci. 287 (1927), 20200366. doi: 10.1098/rspb.2020.0366
Collier C. J., Langlois L. M., McMahon K. M., Udy J., Rasheed M., Lawrence E., et al. (2021). What lies beneath: Predicting seagrass below-ground biomass from above-ground biomass, environmental conditions and seagrass community composition. Ecol. Indic. 121, 107156. doi: 10.1016/j.ecolind.2020.107156
Diesing M., Kröger S., Parker R., Jenkins C., Mason C., Weston K. (2017). Predicting the standing stock of organic carbon in surface sediments of the North–West European continental shelf. Biogeochemistry 135 (1), 183–200. doi: 10.1007/s10533-017-0310-4
DMCR (2023). State of Marine and Coastal Resources and Coastal Erosion, Thailand National Report 2022. Department of Marine and Coastal Resources, Ministry of Natural Resources and Environment. Bangkok. 407 pages.
Du J., Hu W., Nagelkerken I., Sangsawang L., Loh K. H., Ooi J. L.-S., et al. (2020). Seagrass meadows provide multiple benefits to adjacent coral reefs through various microhabitat functions. Ecosystem Health Sustainability 6 (1), 1812433. doi: 10.1080/20964129.2020.1812433
Duarte C. M., Losada I. J., Hendriks I. E., Mazarrasa I., Marbà N. (2013). The role of coastal plant communities for climate change mitigation and adaptation. Nat. Climate Change 3 (11), 961–968. doi: 10.1038/nclimate1970
Duarte C. M., Merino M., Agawin N. S. R., Uri J., Fortes M. D., Gallegos M. E., et al. (1998). Root production and belowground seagrass biomass. Mar. Ecol. Prog. Ser. 171, 97–108. doi: 10.3354/meps171097
Duarte de Paula Costa M., Macreadie P. I. (2022). The evolution of blue carbon science. Wetlands 42 (8), 109. doi: 10.1007/s13157-022-01628-5
Eggertsen M., Larsson J., Porseryd T., Åkerlund C., Chacin D. H., Berkström C., et al. (2021). Coral-macroalgal interactions: Herbivory and substrate type influence growth of the macroalgae Eucheuma denticulatum (N.L. Burman) Collins & Hervey 1917 on a tropical coral reef. J. Exp. Mar. Biol. Ecol. 542-543, 151606. doi: 10.1016/j.jembe.2021.151606
Elliott C., Schumer C., Ross K., Gasper R., Singh N. (2023). A logical framework for net-zero climate action. Front. Climate 5. doi: 10.3389/fclim.2023.1128498
El-Masry E. A., El-Sayed M. K., Awad M. A., El-Sammak A. A., Sabarouti M. A. E. (2022). Vulnerability of tourism to climate change on the Mediterranean coastal area of El Hammam–EL Alamein, Egypt. Environment. Dev. Sustainability 24 (1), 1145–1165. doi: 10.1007/s10668-021-01488-9
Erlania B., Bellgrove A., Macreadie P. I., Young M. A., Holland O. J., Clark Z., et al. (2023). Patterns and drivers of macroalgal ‘blue carbon’ transport and deposition in near-shore coastal environments. Science of The Total Environment 890, 164430. doi: 10.1016/j.scitotenv.2023.164430
Fontoura L., D’Agata S., Gamoyo M., Barneche D. R., Luiz O. J., Madin E. M. P., et al. (2022). Protecting connectivity promotes successful biodiversity and fisheries conservation. Science 375 (6578), 336–340. doi: 10.1126/science.abg4351
Fourqurean J. W., Duarte C. M., Kennedy H., Marbà N., Holmer M., Mateo M. A., et al. (2012). Seagrass ecosystems as a globally significant carbon stock. Nat. Geosci. 5 (7), 505–509. doi: 10.1038/ngeo1477
Giordano R., Pluchinotta I., Pagano A., Scrieciu A., Nanu F. (2020). Enhancing nature-based solutions acceptance through stakeholders’ engagement in co-benefits identification and trade-off analysis. Sci. Total Environ. 713, 136552. doi: 10.1016/j.scitotenv.2020.136552
Githaiga M. N., Frouws A. M., Kairo J. G., Huxham M. (2019). Seagrass removal leads to rapid changes in fauna and loss of carbon. Front. Ecol. Evol. 7. doi: 10.3389/fevo.2019.00062
Gössling S., Balas M., Mayer M., Sun Y.-Y. (2023). A review of tourism and climate change mitigation: The scales, scopes, stakeholders and strategies of carbon management. Tourism Manage. 95, 104681. doi: 10.1016/j.tourman.2022.104681
Greiner J. T., McGlathery K. J., Gunnell J., McKee B. A. (2013). Seagrass restoration enhances “Blue Carbon” sequestration in coastal waters. PloS One 8 (8), e72469. doi: 10.1371/journal.pone.0072469
Grimm N. B., Staudinger M. D., Staudt A., Carter S. L., Chapin F. S. III, Kareiva P., et al. (2013). Climate-change impacts on ecological systems: introduction to a US assessment. Front. Ecol. Environ. 11 (9), 456–464. doi: 10.1890/120310
Guerra-Vargas L. A., Gillis L. G., Mancera-Pineda J. E. (2020). Stronger together: do coral reefs enhance seagrass meadows “Blue carbon” Potential? Frontiers in. Mar. Sci. 7, 1–14. doi: 10.3389/fmars.2020.00628
Hagger V., Waltham N. J., Lovelock C. E. (2022). Opportunities for coastal wetland restoration for blue carbon with co-benefits for biodiversity, coastal fisheries, and water quality. Ecosystem Serv. 55, 101423. doi: 10.1016/j.ecoser.2022.101423
Heiri O., Lotter A. F., Lemcke G. (2001). Loss on ignition as a method for estimating organic and carbonate content in sediments: reproducibility and comparability of results. J. Paleolimnology 25 (1), 101–110. doi: 10.1023/A:1008119611481
Herrera M., Tubío A., Pita P., Vázquez E., Olabarria C., Duarte C. M., et al. (2022). Trade-offs and synergies between seagrass ecosystems and fishing activities: A global literature review. Front. Mar. Sci. 9. doi: 10.3389/fmars.2022.781713
Howard J., Sutton-Grier A. E., Smart L. S., Lopes C. C., Hamilton J., Kleypas J., et al. (2023). Blue carbon pathways for climate mitigation: Known, emerging and unlikely. Mar. Policy 156, 105788. doi: 10.1016/j.marpol.2023.105788
Huang X., Huang L., Li Y., Xu Z., Fong C. W., Huang D., et al. (2006). Main seagrass beds and threats to their habitats in the coastal sea of South China. Chin. Sci. Bull. 51 (2), 136–142. doi: 10.1007/s11434-006-9136-5
IPCC, Core Writing Team (2023). Summary for Policymakers. In: Climate Change 2023: Synthesis Report. Contribution of Working Groups I, II and III to the Sixth Assessment Report of the Intergovernmental Panel on Climate Change. Eds. Lee H., Romero J. (Geneva, Switzerland: IPCC), 1–34. doi: 10.59327/IPCC/AR6-9789291691647.001
James R. K., Keyzer L. M., van de Velde S. J., Herman P. M. J., van Katwijk M. M., Bouma T. J. (2023). Climate change mitigation by coral reefs and seagrass beds at risk: How global change compromises coastal ecosystem services. Science of The Total Environment. 857, 159576. doi: 10.1016/j.scitotenv.2022.159576
Johnson B. A., Kumar P., Okano N., Dasgupta R., Shivakoti B. R. (2022). Nature-based solutions for climate change adaptation: A systematic review of systematic reviews. Nature-Based Solutions 2, 100042. doi: 10.1016/j.nbsj.2022.100042
Kim W., Song C., Lee S. K., Choi G., Yang R., Bak I., et al. (2023). A way forward for climate technology transfer and sustainable development goals. Environ. Sci. Policy 142, 29–41. doi: 10.1016/j.envsci.2023.01.009
Klomjit A., Vieira C., Mattos F. M. G., Sutthacheep M., Sutti S., Kim M.-S., et al. (2022). Diversity and ecology of Lobophora species associated with coral reef systems in the western Gulf of Thailand, including the description of two new species. Plants 11, 3349. doi: 10.3390/plants11233349
Klomjit A., Yeemin T., Rangseethampanya P., Ruengthong C., Samsuvan W., Sutthacheep M. (2021). The abundance of macroalgae and herbivory in some degraded reefs and coral recovery at Samui Islands. Ramkhamhaeng Int. J. Sci. Technol. 4 (1), 35–42.
Krause-Jensen D., Duarte C. M. (2016). Substantial role of macroalgae in marine carbon sequestration. Nat. Geosci. 9 (10), 737–742. doi: 10.1038/ngeo2790
Krause-Jensen D., Lavery P., Serrano O., Marbà N., Masque P., Duarte C. M. (2018). Sequestration of macroalgal carbon: the elephant in the Blue Carbon room. Biol. Lett. 14 (6), 20180236. doi: 10.1098/rsbl.2018.0236
Kuramochi T., Roelfsema M., Hsu A., Lui S., Weinfurter A., Chan S., et al. (2020). Beyond national climate action: the impact of region, city, and business commitments on global greenhouse gas emissions. Climate Policy 20 (3), 275–291. doi: 10.1080/14693062.2020.1740150
Kuwae T., Watanabe A., Yoshihara S., Suehiro F., Sugimura Y. (2022). Implementation of blue carbon offset crediting for seagrass meadows, macroalgal beds, and macroalgae farming in Japan. Mar. Policy 138, 104996. doi: 10.1016/j.marpol.2022.104996
Lovelock C. E., Duarte C. M. (2019). Dimensions of Blue Carbon and emerging perspectives. Biol. Lett. 15 (3), 20180781. doi: 10.1098/rsbl.2018.0781
Macreadie P. I., Anton A., Raven J. A., Beaumont N., Connolly R. M., Friess D. A., et al. (2019). The future of Blue Carbon science. Nat. Commun. 10 (1), 1–13. doi: 10.1038/s41467-019-11693-w
Mayakun J., Prathep A. (2005). Seasonal variations in diversity and abundance of macroalgae at Samui Island, Surat Thani Province, Thailand. Songklanakarin J. Sci. Technol. 27 (11), 653.
Mcleod E., Chmura G. L., Bouillon S., Salm R., Björk M., Duarte C. M., et al. (2011). A blueprint for blue carbon: toward an improved understanding of the role of vegetated coastal habitats in sequestering CO2. Front. Ecol. Environ. 9 (10), 552–560. doi: 10.1890/110004
McMahon K., Collier C., Lavery P. S. (2013). Identifying robust bioindicators of light stress in seagrasses: A meta-analysis. Ecol. Indic. 30, 7–15. doi: 10.1016/j.ecolind.2013.01.030
Nanami A. (2022). Co-occurrence of seagrass vegetation and coral colonies supports unique fish assemblages: a microhabitat-scale perspective. PeerJ 10, e14466. doi: 10.7717/peerj.14466
Naumann M. S., Richter C., Mott C., el-Zibdah M., Manasrah R., Wild C. (2012). Budget of coral-derived organic carbon in a fringing coral reef of the Gulf of Aqaba, Red Sea. J. Mar. Syst. 105-108, 20–29. doi: 10.1016/j.jmarsys.2012.05.007
Oliveira B. M., Boumans R., Fath B. D., Harari J. (2022). Coastal ecosystem services and climate change: Case study for integrated modeling and valuation. Global Ecol. Conserv. 38, e02240. doi: 10.1016/j.gecco.2022.e02240
Pessarrodona A., Franco-Santos R. M., Wright L. S., Vanderklift M. A., Howard J., Pidgeon E., et al. (2023). Carbon sequestration and climate change mitigation using macroalgae: a state of knowledge review. Biological Reviews 98 (6), 1945–1971. doi: 10.1111/brv.12990
Phang V. X. H., Chou L. M., Friess D. A. (2015). Ecosystem carbon stocks across a tropical intertidal habitat mosaic of mangrove forest, seagrass meadow, mudflat and sandbar. Earth Surface Processes Landforms 40 (10), 1387–1400. doi: 10.1002/esp.3745
Prathep A., Rattanachot E., Tuntiprapas P. (2010). Seasonal variations in seagrass percentage cover and biomass at Koh Tha Rai, Nakhon Si Thammarat Province, Gulf of Thailand. Songklanakarin J. Sci. Technol. 32 (5), 497–504.
Qu Z., Thrush S., Blain C., Lewis N. (2023). Assessing the carbon storage value of kelp forest restoration in the Hauraki Gulf Marine Park, New Zealand: Lessons from no-take Marine Protected Areas. Mar. Policy 154, 105682. doi: 10.1016/j.marpol.2023.105682
Reyes A. G. B., Vergara M. C. S., Blanco A. C., Salmo Iii S. G. (2022). Seagrass biomass and sediment carbon in conserved and disturbed seascape. Ecol. Res. 37 (1), 67–79. doi: 10.1111/1440-1703.12272
Ricart A. M., Pérez M., Romero J. (2017). Landscape configuration modulates carbon storage in seagrass sediments. Estuarine. Coast. Shelf Sci. 185, 69–76. doi: 10.1016/j.ecss.2016.12.011
Rölfer L., Reuter H., Ferse S. C. A., Kubicek A., Dove S., Hoegh-Guldberg O., et al. (2021). Coral-macroalgal competition under ocean warming and acidification. J. Exp. Mar. Biol. Ecol. 534, 151477. doi: 10.1016/j.jembe.2020.151477
Saavedra-Hortua D., Nagelkerken I., Estupinan-Suarez L. M., Gillis L. G. (2023). Effects of connectivity on carbon and nitrogen stocks in mangrove and seagrass ecosystems. Sci. Total Environ. 896, 164829. doi: 10.1016/j.scitotenv.2023.164829
Samper-Villarreal J., Lovelock C. E., Saunders M. I., Roelfsema C., Mumby P. J. (2016). Organic carbon in seagrass sediments is influenced by seagrass canopy complexity, turbidity, wave height, and water depth. Limnology Oceanography 61 (3), 938–952. doi: 10.1002/lno.10262
Seddon N., Chausson A., Berry P., Girardin C. A. J., Smith A., Turner B. (2020). Understanding the value and limits of nature-based solutions to climate change and other global challenges. Philos. Trans. R. Soc. B: Biol. Sci. 375 (1794), 20190120. doi: 10.1098/rstb.2019.0120
Stallings C. D., Brower J. P., Heinlein Loch J. M., Mickle A. (2014). Commercial trawling in seagrass beds: Bycatch and long-term trends in effort of a major shrimp fishery. Mar. Ecol. Prog. Ser. 513, 143–153. doi: 10.3354/meps10960
Stankovic M., Ambo-Rappe R., Carly F., Dangan-Galon F., Fortes M. D., Hossain M. S., et al. (2021). Quantification of blue carbon in seagrass ecosystems of Southeast Asia and their potential for climate change mitigation. Sci. Total Environ. 783, 146858. doi: 10.1016/j.scitotenv.2021.146858
Suraswadi P., Yeemin T. (2013). Coral reef restoration plan of Thailand. Galaxea J. Coral Reef Stud. 15 (S), 428–433. doi: 10.3755/galaxea.15.428
Sutthacheep M., Pengsakun S., Yucharoen M., Klinthong W., Sangmanee K., Yeemin T. (2013). Impacts of the mass coral bleaching events in 1998 and 2010 on the western Gulf of Thailand. Deep-Sea Res. II 96, 25–31. doi: 10.1016/j.dsr2.2013.04.018
Sutthacheep M., Yeemin T., Aliño P. M. (2022). “Coral Reefs of the Western Pacific Ocean in a Changing Anthropocene,” in Coral Reefs of the World, vol. 14 . Ed. Zhang, et al (Cham: Springer International Publishing), 55–98. 199 p.
Suzuki A., Kawahata H. (2003). Carbon budget of coral reef systems: an overview of observations in fringing reefs, barrier reefs and atolls in the Indo-Pacific regions. Tellus B: Chem. Phys. Meteorology 55 (2), 428–444. doi: 10.3402/tellusb.v55i2.16761
Teasdale N., Panegyres P. K. (2023). Climate change in Western Australia and its impact on human health. J. Climate Change Health 12, 100243. doi: 10.1016/j.joclim.2023.100243
Venegas R. M., Acevedo J., Treml E. A. (2023). Three decades of ocean warming impacts on marine ecosystems: A review and perspective. Deep Sea Res. Part II: Topical Stud. Oceanography, 105318. doi: 10.1016/j.dsr2.2023.105318
Wang Q., Wen Y., Zhao B., Hong H., Liao R., Li J., et al. (2021). Coastal soil texture controls soil organic carbon distribution and storage of mangroves in China. CATENA 207, 105709. doi: 10.1016/j.catena.2021.105709
Ware J. R., Smith S. V., Reaka-Kudla M. L. (1992). Coral reefs: sources or sinks of atmospheric CO2? Coral Reefs 11 (3), 127–130. doi: 10.1007/BF00255465
Williamson P., Gattuso J.-P. (2022). Carbon removal using coastal blue carbon ecosystems is uncertain and unreliable, with questionable climatic cost-effectiveness. Front. Climate 4. doi: 10.3389/fclim.2022.853666
Xu D., Abbas S., Rafique K., Ali N. (2023). The race to net-zero emissions: Can green technological innovation and environmental regulation be the potential pathway to net-zero emissions? Technol. Soc., 102364. doi: 10.1016/j.techsoc.2023.102364
Yeemin T., Pengsakun S., Yucharoen M., Klinthong W., Sangmanee K., Sutthacheep M. (2013). Long-term changes of coral communities under stress from sediment. Deep-Sea Res. II 96, 32–40. doi: 10.1016/j.dsr2.2013.04.019
Yeemin T., Tuan V. S., Suharsono (2022). “Coral Reefs of the Western Pacific Ocean in a Changing Anthropocene,” in Coral Reefs of the World, vol. 14 . Ed. Zhang, et al (Cham: Springer International Publishing), 25–53. 199 p.
York P. H., Macreadie P. I., Rasheed M. A. (2018). Blue Carbon stocks of Great Barrier Reef deep-water seagrasses. Biol. Lett. 14 (12), 20180529. doi: 10.1098/rsbl.2018.0529
Keywords: blue carbon, biomass, carbon storage, coral reef, seagrass, macroalgae
Citation: Yeemin T, Sutthacheep M, Pengsakun S, Klinthong W, Chamchoy C and Suebpala W (2024) Quantifying blue carbon stocks in interconnected seagrass, coral reef, and sandy coastline ecosystems in the Western Gulf of Thailand. Front. Mar. Sci. 11:1297286. doi: 10.3389/fmars.2024.1297286
Received: 19 September 2023; Accepted: 16 January 2024;
Published: 12 February 2024.
Edited by:
Nadia S. Santini, INIFAP, MexicoReviewed by:
Joanna Acosta-Velazquez, Universidad Autónoma del Carmen, MexicoCarlos Troche Souza, National Commission for the Knowledge and Use of Biodiversity (CONABIO), Mexico
Copyright © 2024 Yeemin, Sutthacheep, Pengsakun, Klinthong, Chamchoy and Suebpala. This is an open-access article distributed under the terms of the Creative Commons Attribution License (CC BY). The use, distribution or reproduction in other forums is permitted, provided the original author(s) and the copyright owner(s) are credited and that the original publication in this journal is cited, in accordance with accepted academic practice. No use, distribution or reproduction is permitted which does not comply with these terms.
*Correspondence: Wichin Suebpala, d2ljaGluLnNAcnUuYWMudGg=