- 1Soil, Water and Ecosystem Sciences Department, University of Florida, Gainesville, FL, United States
- 2Department of Forest, Fisheries and Geomatic Sciences, University of Florida, Gainesville, FL, United States
- 3St. Johns River Water Management District, Palatka, FL, United States
Introduction: Successful management and mitigation of harmful algal blooms (HABs) requires an in-depth understanding of the physiology and nutrient utilization of the organisms responsible. We explored the preference of various nitrogen (N) and phosphorus (P) substrates by two novel groups of HAB-forming phytoplankton originating from the Indian River Lagoon (IRL), Florida: 1) a consortium of picocyanobacteria (Crocosphaera sp. and ‘Synechococcus’ sp.) and 2) ananochlorophyte (Picochlorum sp.).
Methods: Short-term kinetic uptake experiments tested algal use and affinity for inorganic and organic N substrates (ammonium (NH4+), nitrate (NO3-), urea, and an amino acid (AA) mixture) through 15N and 13C isotope tracing into biomass.
Results: Picocyanobacteria exhibited Michaelis-Menten type uptake for the AA mixture only, while nanochlorophytes reached saturation for NH4+, the AA mixture, and urea at or below 25 µM-N. Both picocyanobacteria and nanochlorophyte cultures had highest affinity (Vmax/Ks) for NH4+ followed by the AA mixture and urea. Neither culture showed significant uptake of isotopically-labeled nitrate. Disappearance of glucose-6-phosphate (G6P) added to culture medium suggesting use of organic P by both cultures was confirmed by detection of alkaline phosphatase activity and the tracing of 13C-G6P into biomass.
Discussion: Together, our results suggest that these HAB-forming phytoplankton groups are able to use a variety of N and P sources including organic forms, and prefer reduced forms of N. These traits are likely favorable under conditions found in the IRL during periods of significant competition for low concentrations of inorganic nutrients. Bloom-forming phytoplankton are therefore able to subsist on organic or recycled forms of N and P that typically dominate the IRL nutrient pools.
1 Introduction
Harmful algal blooms (HABs) have become an increasing problem in ecosystems subject to cultural eutrophication around the world (Anderson et al., 2002; Glibert et al., 2005; Howarth and Marino, 2006; Heiser et al., 2008). Well-documented and significant impacts of HABs on aquatic ecosystems and local economies have led to efforts to reduce both anthropogenic nitrogen (N) and phosphorus (P) loads (Conley et al., 2009; Lewis et al., 2011; Paerl et al., 2016). However, disentangling the complex interactions between the conventional “bottom-up” (e.g., nutrients) and “top-down” (e.g., grazing) controls on bloom formation, as well as other biogeochemical factors, such as climatic and hydrologic conditions, have complicated efforts to model HAB dynamics (Burkholder et al., 2006; Glibert et al., 2010; Gowen et al., 2012; Davidson et al., 2014; Rothenberger and Calomeni, 2016; Glibert et al., 2023). It is also recognized that modeling and managing HABs requires information on the character (e.g., toxin, motility, size, growth rates) of the dominant species involved in the blooms and the specific factors that select for its success (e.g., nutrient preferences, temperature and light optima) (Burford et al., 2020; Glibert, 2020; Glibert et al., 2023; Lima et al., 2023). In this study we examined the nutrient preferences of the phytoplankton species that dominated a major bloom event in the Indian River Lagoon and discuss how these preferences may have contributed to in their success.
The Indian River Lagoon (IRL) is a subtropical estuary on the Atlantic coast of Florida and designated by the USEPA as an “Estuary of National Significance” in 1990, joining the list of 28 sites in the National Estuary Program (www.onelagoon.org/irlnep/). The IRL is also an example of a system that has experienced a recent regime shift in the magnitude and species composition of blooms (Phlips et al., 2021). In 2011, the northern IRL experienced a bloom colloquially called the “Superbloom” due to its historic spatial extent, intensity, and duration (from Spring through Winter of 2021) (LaPointe et al., 2015; Phlips et al., 2015; Phlips et al., 2021). The bloom spanned across three major sub-basins of the IRL (Mosquito Lagoon, Banana River lagoon and the northern IRL), with phytoplankton biomass levels consistently exceeding the established bloom threshold for the IRL of 2 µg carbon ml-1 (Phlips et al., 2021) for most of 2021, with peak chlorophyll a values exceeding 100 µg carbon ml-1. Pico-planktonic cyanobacteria and nano-planktonic eukaryotes dominated the 2011 bloom and continued to dominate most blooms in the following ten years (Lopez et al., 2021; Phlips et al., 2021). Although the species involved in these blooms were not associated with the production of toxins, recurring intense blooms of these taxa have been implicated as a major causal factor in the widespread and persistent losses (58%) of critical seagrass habitat in the IRL, due to severe benthic light limitation (i.e., extended periods of time with Secchi disk depths between 0.2 and 0.5 m) (Phlips et al., 2015; Kang et al., 2015; Phlips et al., 2015; Morris et al., 2022).
It has been suggested that the post-regime shift blooms in the IRL have in part been driven by shifts in the distribution of internal nutrient pools from the benthos to water column phytoplankton (Phlips et al., 2015; Phlips et al., 2021), and N-enrichment from sewage and septic tank pollution (LaPointe et al., 2015; Barile, 2018). Both of these sources heighten the potential role of ammonium and organic forms of nitrogen and phosphorus as nutrient sources for phytoplankton production. Earlier research on nutrient limitation of phytoplankton production in the IRL observed frequent N-limitation, with periods of phosphorus or NP co-limitation in the northern basins (Phlips et al., 2002), indicating that both N and P dynamics are important factors in bloom formation.
It has been hypothesized that one of the reasons for the dominance of pico-cyanobacteria and nano-eukaryotes (e.g., the chlorophyte Picochlorum and the pelagophyte Aureoumbra lagunensis) in many of the major blooms in the IRL from 2011-2021 is their ability to effectively compete for inorganic and organic forms of nitrogen and phosphorus, provided by external loads and internal recycling of nutrients (Phlips et al., 2015; Phlips et al., 2021). Several studies in other ecosystems have yielded information supporting such a hypothesis. In a study of Laguna Madre in Texas, the nano-eukaryote Aureoumbra lagunensis was shown to have a high N/P ratio and was able to grow well under low inorganic phosphorus levels, likely due to the use of organic sources (Liu et al., 2001). A recent review of the response of freshwater phytoplankton to organic nutrient forms highlights observations that increasing prominence of organic forms of nitrogen (e.g., urea) and phosphorus appears to be favoring cyanobacteria and chlorophytes (Reinl et al., 2022). In three studies of organic nitrogen uptake by coastal marine phytoplankton, regenerated forms of nitrogen, such as NH4 and urea, were important nutrient sources for production, particularly for pico- and nanoplanktonic taxa (Wafar et al., 2004; Moschonas et al., 2017; Dames et al., 2023). A number of HAB-forming species have also been shown to have a preference for ammonium (NH4+) over nitrate (NO3-) (Glibert et al., 2014). In more general terms, the high surface area to volume ratios associated with small-celled phytoplankton can be advantageous under nutrient-limiting conditions (Smith and Kalff, 1982; Raven, 1998; Reynolds, 2006; Behrenfeld et al., 2008).
Collectively, the above traits may allow bloom-forming species to compete during conditions of low inorganic nutrient inputs and persist on recycled forms of nutrients such as NH4+ and organic nitrogen and phosphorus. To date, there is no documentation on nitrogen or phosphorus preferences for the groups responsible for the ‘Superbloom’. The objective of this study was to utilize kinetic uptake experiments to investigate the use of specific inorganic and organic forms of N and P by two dominant ‘Superbloom’ groups. We hypothesized that these two taxa would be able to use nitrogen and/or phosphorus at low concentrations and possibly show a preference for organic substrates, as seen in some other HAB-forming species.
2 Methods
2.1 Isolation and culturing of bloom species
Strains of the pico and nanoplanktonic algae were isolated from natural water samples collected from the northern IRL near Titusville, FL. Isolation procedures included shake-dilution, size fractionation through differential filtration, antibiotic treatment, and streak plating. Three phytoplankton groups were isolated and maintained in culture, including a 2-5 µm non-flagellated chlorophyte (nanochlorophyte), and a mixed culture of spherical picocyanobacteria in the 0.7-1.5 µm size range. Subsequent analysis of cultures collected from the IRL have identified the cyanobacteria as Crocosphaera sp. and Synechococcus sp., and the non-flagellated eukaryote as Pichochlorum sp. (Chlorophyta) (Galimany et al., 2020). The two cultures will be referred to as ‘picocyanobacteria’ and ‘nanochlorophyte’, respectively in this paper.
After isolation, uni-algal cultures were maintained in the laboratory using an artificial seawater medium at salinity of 30 as described in Phlips et al. (1989). All cultures were continuously stirred and grown in a temperature-controlled culture room at 26-28˚C under incident irradiance of 100 µE on a 12 hr light:12 hr dark cycle. Prior to uptake experiments, biomass from the cultures were transferred to either “low N” (DIN : DIP ratio = 6.5) or “low P” (DIN : DIP ratio = 100) medium and cultured for another month in order to reach N-depleted or P-depleted conditions, respectively. P-depleted media was amended with a lower concentration of metals as well as a bicarbonate buffer (pH 8.5) to minimize interferences encountered with subsequent P digestion and colorimetric procedures.
2.2 Uptake of 15N-labelled substrates
Three experimental replicates were created by combining batches of N-depleted picocyanobacteria culture or nanochlorophyte culture. From these replicates, 40 mL aliquots were spiked with 15N-enriched substrates in triplicate to trace N uptake. The N substrates used were 98 atom % 15NH4+, 15NO3-, 15N-urea, and a dually labeled 13C/15N-algal amino acid (AA) mixture (Cambridge Isotope Laboratory, Inc., Andover, MA, USA) added to produce four different concentrations. For the picocyanobacteria experiments, final concentrations of 0.1, 1, 2, and 10 µM-N were used. Final concentrations were increased to 0.5, 1, 10, and 25 µM-N for the nanochlorophyte experiment in order to achieve substrate saturating conditions for calculation of kinetic parameters.
Following enrichment, incubations were carried out for one hour at approximately 21-22°C and under 100-200 μE of fluorescent light. Incubations were terminated by gentle filtration of the full 40 mL volume onto glass fiber filters (Whatman GF/F, 0.7 µm particle retention). Filters of unenriched algal biomass and the corresponding filtrate were also collected from each replicate of unenriched culture for characterization of biomass nutrient content, isotope (15N and 13C) natural abundance, and dissolved nutrient conditions of the culture. All filters and filtrate were stored frozen until analysis.
2.3 Phosphorus uptake incubations
As with the N-uptake experiments, three experimental replicates were created from by combining P-depleted cultures. Replicates were additionally diluted using a 1:1 ratio of culture to low-nutrient artificial seawater (Brightwell Aquatics NeoMarine; approximately 36 ppt) in order to further reduce any media interferences with TP digestions. Diluted culture was then spiked with either DIP (as KH2PO4) or DOP (as glucose-6-phosphate) solutions to reach initial concentrations of 5, 10, 20, and 50 µM-P. Unlike N-uptake experiments, aliquots were filtered through 0.2 µm membrane syringe-filters using hand pressure every five (5) minutes for nanochlorophyte experiments and every 7.5 minutes for picocyanobacteria for a total incubation time of either 20 minutes or 30 minutes, respectively. This difference in incubation time was due to difficulties encountered when filtering picocyanobacteria cultures, potentially due to thick extracellular polysaccharides in the culture (De Philippis and Vincenzini, 1998).
Aliquots of unenriched culture (i.e. controls) were also taken at the end of the incubations to quantify background DIP and DOP levels and to ensure there were no significant changes over the time course of both experiments. Unenriched cultures from each replicate were also filtered through glass fiber filters (Whatman GF/F, 0.7 µm particle retention) for isotope (15N and 13C) natural abundance and biomass nutrients. The corresponding filtrate was collected for dissolved nutrients, and all filters and filtrate were frozen until analysis.
2.4 Confirmation of organic P uptake
Confirmation of DOP uptake was assessed with tracing of 13C-DOP into algal biomass. Cultures from August 2016 were spiked with a 99 atom % 13C-labeled glucose-6-phosphate (G6P, Cambridge Isotope Laboratory, Inc., Andover, MA, USA) to trace incorporation of the 13C into biomass. Only one experimental replicate was used per concentration, and final concentrations of 13C-G6P were the same as those used in DOP-uptake experiments. Additionally, incubations were carried out for the same total time used for each species (i.e. 30 minutes for picocyanobacteria; 20 minutes for nanochlorophyte) and were terminated by filtration of biomass onto 0.7 µm GF/F filters. Filters were stored frozen until analysis.
Phosphatase enzymes can potentially hydrolyze added organic P compounds with subsequent uptake of glucose (Sharma et al., 2005). Therefore, to help validate the assumption of 13C incorporation representing DOP uptake, extracellular alkaline phosphatase activity (APA) assays were also used to determine the potential for external hydrolysis of added G6P. APA experiments were carried out on two separate occasions, once in June 2015 and again in August 2016. The later experiment used the same cultures as those used in the P-uptake experiments. APA assays were conducted using the substrate 4-Methylumbelliferyl phosphate (MUF-P) which fluoresces with cleavage by the alkaline phosphatase enzyme (Marx et al., 2001; Liao et al., 2014). Similar to P-uptake experiments, culture replicates were diluted to 50% with buffer (pH 8.5) in order to minimize background interference from the culture medium. The diluted culture was then incubated with MUF-P at saturating concentrations (500 µM) in black 96-well microplates for approximately four hours at room temperature under dark conditions. Fluorescence of MUF was detected on a Biotek Synergy HT microplate reader with an excitation wavelength of 360 nm and emission wavelength of 460 nm. Corrections were made for background culture and MUF-P fluorescence, as well as potential “quenching” of fluorescence by the culture itself (German et al., 2011).
2.5 Nutrient analyses and cell counts
For isotope-based experiments, both enriched and natural abundance 15N and 13C and total particulate organic nitrogen (PON) and carbon (POC) content was determined after drying of the filters (70°C for approximately 24 hours). For enriched biomass, subsamples of the filters were run on a ThermoFinnigan MAT Delta Plus XL isotopic ratio mass spectrometer (Thermo Fisher Scientific Inc., Waltham, MA) equipped with a Costech ECS 4010 elemental analyzer (Costech Analytical Technologies Inc., Valencia, CA) (Inglett et al., 2004). Natural abundance isotopic ratios were determined on full filters. Natural abundance 15N and 13C values are expressed using delta (δ) notation as per mil (‰) differences from standard N2 or PDB.
Particulate phosphorus (POP) content of the biomass on filters was measured after persulfate digestion to orthophosphate (Suzumura, 2008) using a Shimadzu UV-1800 (Shimadzu Scientific Instruments Inc., Columbia, MD) as described by Murphy and Riley (1962). Total dissolved phosphorus (TDP) of culture filtrate was analyzed by the same digestion and colorimetric methods, while DIP was analyzed on un-digested samples as orthophosphate (i.e. SRP). Dissolved inorganic N (DIN) as ammonium (NH4-N) and nitrate (NOx-N) from filtrate was analyzed by the Analytical Research Laboratory at the University of Florida (Gainesville, FL) following EPA methods 350.1 and 353.2, respectively (EPA 1993a; EPA 1993b). For N-uptake experiments, dissolved free amino acids (DFAA) were measured on by reverse-phase HPLC following modification of the pre-column o-phthaldialdehyde derivatization technique (Lindroth and Mopper, 1979; Duan and Bianchi, 2007).
For all uptake experiments, unenriched culture biomass from each experimental replicate was preserved with glutaraldehyde (final concentration approximately 2%) for enumeration by light and fluorescence microscopy (Phlips et al., 1999; Phlips et al., 2015).
2.6 Kinetic calculations and statistical analyses
Given the low background N concentrations in the cultures and short incubation time, all N-uptake rates were calculated assuming 98 atom percent (at.%) enrichment over the course of the incubations (Dugdale and Goering, 1967; Fan et al., 2003). Specific uptake velocities (V) were calculated from Equation 2-1:
By multiplying V by the PN or PC content to yield ρvolume (µg atom-element hr-1) and then dividing by cell density to obtain ρcell (fg atom-element cell-1 hr-1), specific uptake velocities were converted to yield the cell-absolute uptake rates reported.
For substrates reaching saturation, kinetic parameters can be calculated using the Michaelis-Menten model from Equation 2-2:
where Vmax is the maximum uptake rate (hr-1), S is the substrate concentration (µM-N), and Ks is the half-saturation constant (µM-N). Specific velocities (V) were used rather than cell-absolute rates (pcell) to compute kinetic parameters in order to compare with values typically reported in the literature. Kinetic parameters (i.e. Vmax and Ks) are only reported when the Michaelis-Menten model fit was significant. Otherwise, first order uptake was fit to a linear equation.
For P-uptake, DIP uptake rates were calculated as the disappearance of SRP from solution over time; DOP concentrations were calculated as the difference between TDP and SRP concentration for each time point, and uptake rates were calculated similarly. The uptake rate for each initial P concentration was therefore assumed to be the slope of mean DIP or DOP concentrations plotted against incubation time.
All tests were carried out using Rstudio (R version 3.3.2) with significance set to α=0.05. Michealis-Menten curves were fitted using the nls function, and any differences in means were compared using Tukey-Kramer pos-hoc tests following an ANOVA.
3 Results
3.1 Culture conditions
For both N-uptake experiments, dissolved NH4-N and NO3-N concentrations were low but not completely depleted (Table 1). Molar DIN : DIP ratios suggest that cultures were N-limited at the time of the experiments. Conversely, cultures used in P-uptake experiments had molar DIN : DIP ratios greater than 16, indicating that P-limiting conditions were maintained (Table 1). Particulate nutrient ratios were all moderately higher than the Redfield ratio (molar N:P = 16:1). For example, in N-limitation experiments picocyanobacteria and nanochlorophyte molar N:P ratios were 21:1 and 28:1, respectively (Table 1), while for P-limitation experiments, picocyanobacteria and nanochlorophyte N:P ratios were 19:1 and 26:1, respectively (Table 2). The difference in the degree of 15N discrimination between N-limited and P-limited cultures (approximately 7.95‰ and 11.91‰ for picocyanobacteria and nanochlorophyte cultures, respectively) gives additionally support for the limitation status of the N uptake experiment cultures as N-limited cells would incorporate greater amounts of the heavier N isotope (Inglett and Reddy, 2006; Inglett et al., 2007).
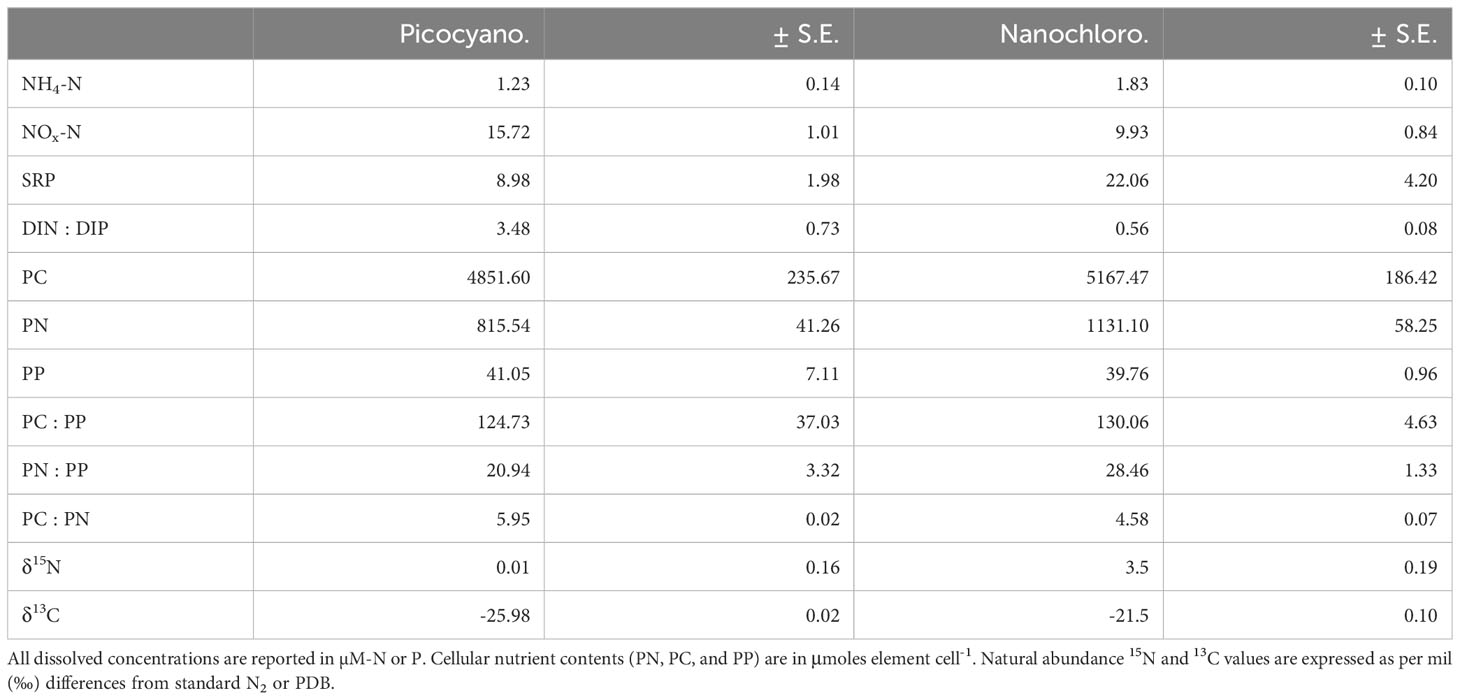
Table 1 Concentrations of select nutrients and ratios from cultures used in N uptake experiments (n=3).
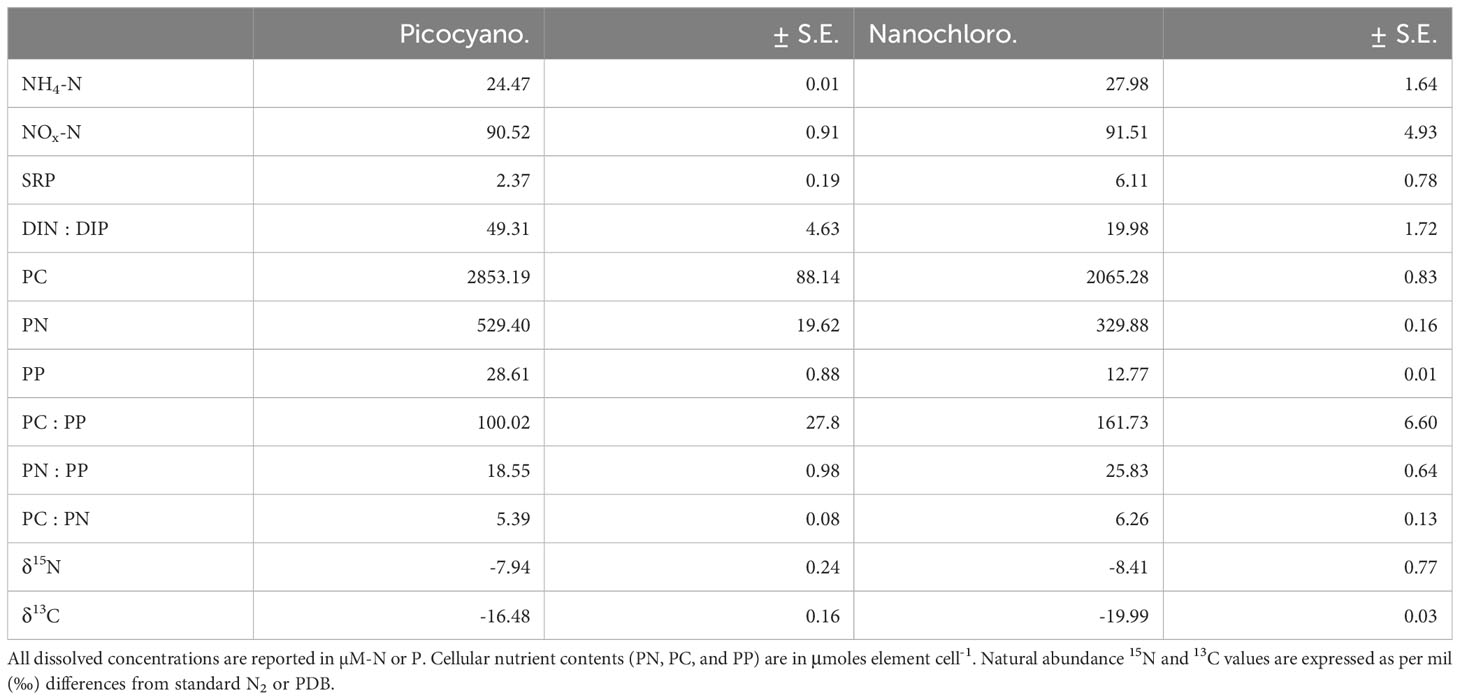
Table 2 Concentrations of select nutrients and ratios from cultures used in P uptake experiments (n=3).
3.2 Nitrogen uptake kinetics
Cell-specific uptake rates (pcell) in both picocyanobacteria and nanochlorophyte cultures were highest for NH4+ followed by the AA mixture, urea and then NO3- (Figures 1, 2). For the latter substrate, uptake by picocyanobacteria was virtually negligible. Excess NO3- in nanochlorophyte cultures may have complicated the detection and calculation of NO3- uptake and therefore no uptake equation is reported. The only substrate that appeared to reach saturation at the concentration range tested for picocyanobacteria (0.1-10 µM-N) was the AA mixture, and uptake for the other three substrates was linear (Table 3). When the range of N added for nanochlorophyte cultures was extended to 25 µM-N, N uptake showed evidence of saturation for NH4+, AA, and Urea (Figure 2).
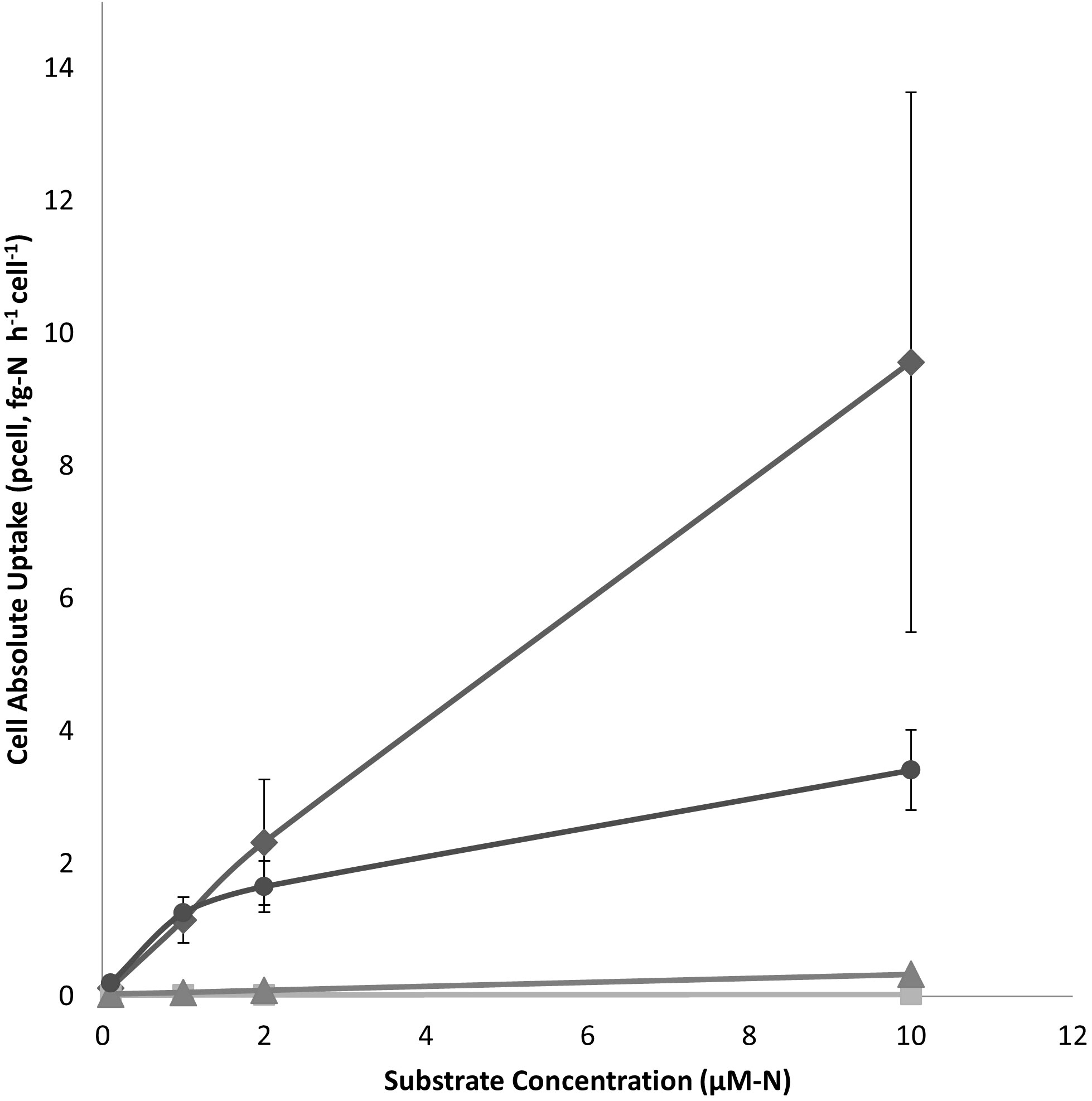
Figure 1 Cell-absolute uptake rates (pcell) of ammonia (♦), AA mixture (●), urea (▲), and nitrate (■) substrates by picocyanobacterial cultures. Each point represents the mean of three experimental replicates ± one S.E.
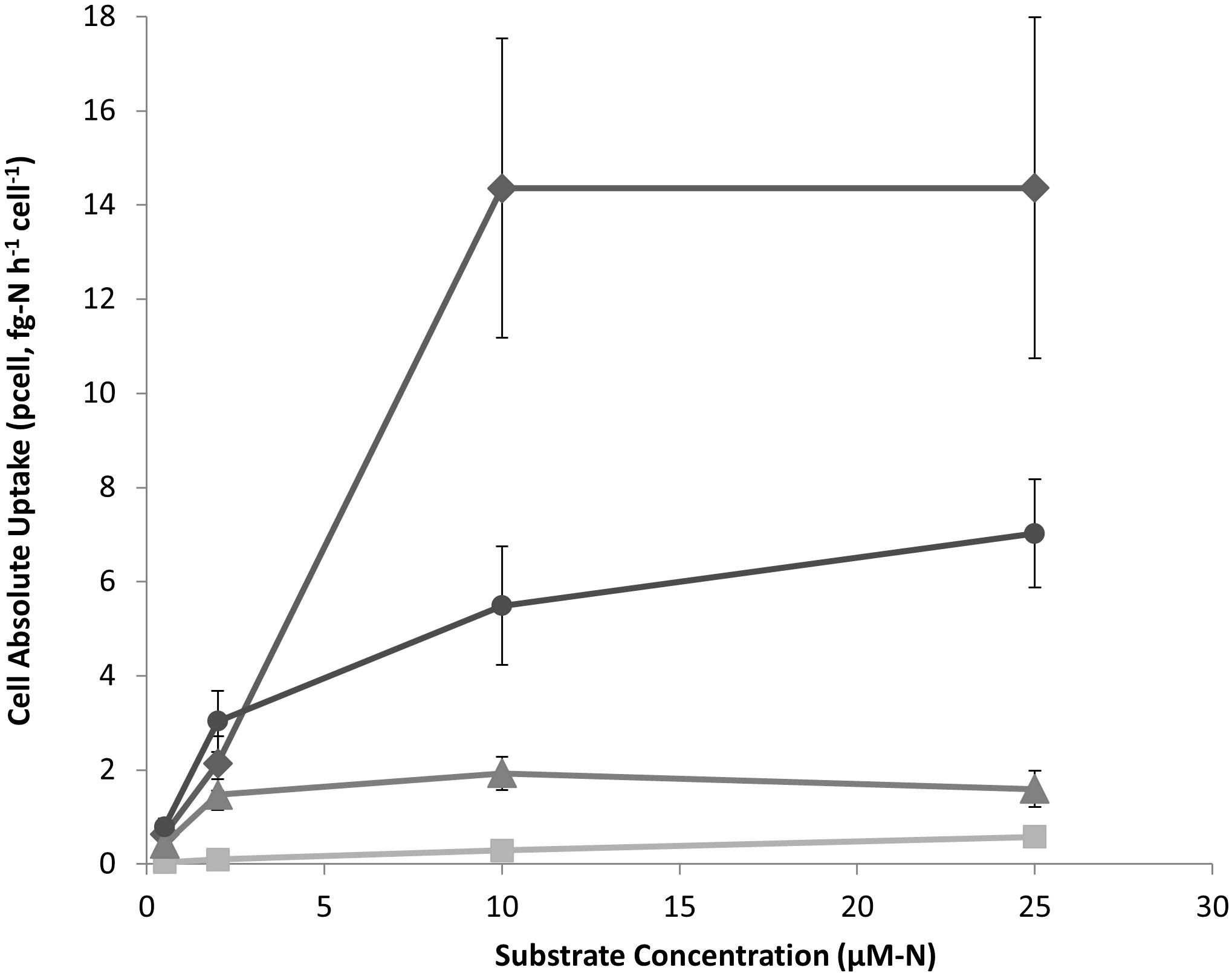
Figure 2 Cell-absolute uptake rates (pcell) of ammonia (♦), AA mixture (●), urea (▲), and nitrate (■) substrates by nanochlorophyte cultures. Each point represents the mean of three experimental replicates ± one S.E.
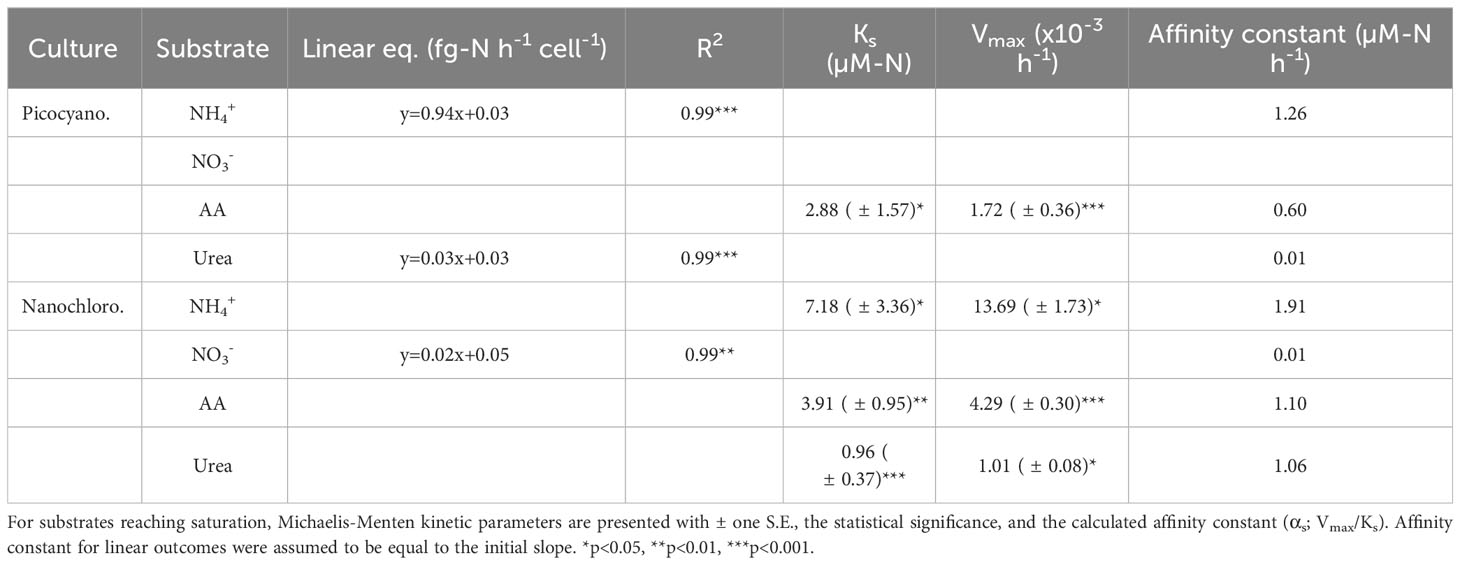
Table 3 Linear equations and model R2 with statistical significance for cell-absolute uptake rates (pcell, fg-N cell-1 hr-1) of N-uptake experiments.
Half-saturation constants (Ks) for nanochlorophyteswere of similar magnitude for NH4+ (7.18 ± 3.36 µM-N) and AA (3.91 ± 0.95 µM-N) but was lowest for urea (0.96 ± 0.37 µM-N) (Table 3). Affinity constants (αs) values calculated from Michaelis-Menten fits were comparable across the two cultures and different substrates, although was highest for NH4+ (1.91) followed by AA (1.10) and urea (1.06) for nanochlorophytes (Table 3). The calculated maximum uptake rate (Vmax) of NH4+ was an order of magnitude higher for nanochlorophytes (13.69 ± 1.73)x10-3 hr-1) compared to picocyanobacteria ((1.72 ± 0.36)x10-3 hr-1) (Table 3).
Another metric commonly used to assess preference for different substrates in the Relative Preference Index (RPI), calculated as the ratio of uptake relative to substrate availability (McCarthy et al., 1977):
where RPIx is the preference of substrate x, Ux is the uptake rate of substrate x, and X is the concentration of substrate x. As urea concentrations were not measured directly, only RPI for NH4+, NO3- and AA are reported. RPI values for NH4+ was significantly higher than NO3- (p<0.0005) for both cultures and higher than AA for picocyanobacteria, suggesting strongest preference for ammonium (Figure 3).
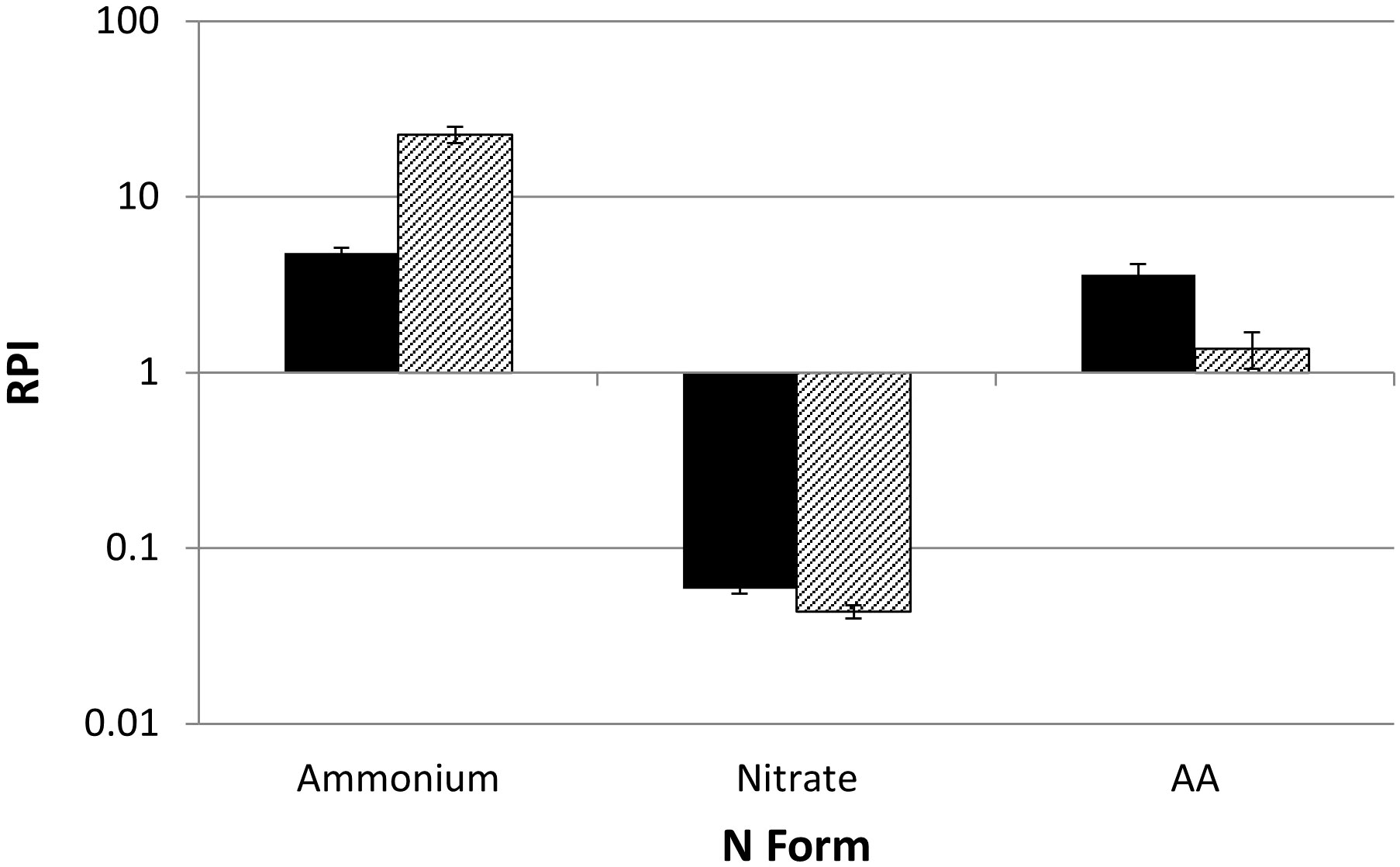
Figure 3 The relative preference index (RPI) for three of the four N substrates used in N-uptake experiments for both nanochlorophyte (black) and picocyanobacteria (shaded) cultures (n=3 ± one S.E.). Values greater than one indicate preference for substrate relative to ambient supply.
Amino acid carbon (isotopically labeled) uptake was also measured for confirmation of organic N use. Plotting absolute uptake (i.e., mass-element hr-1) of N relative to C reveals that both cultures appear to take up C and N at a constant rate (Figure 4). The C:N ratio of specific uptake (i.e. the slope of line) for both cultures deviates below the C:N ratio of the AA mixture (approximately 3.8 by mass), suggesting greater N uptake relative to C. This divergence was more apparent for the picocyanobacteria cultures.
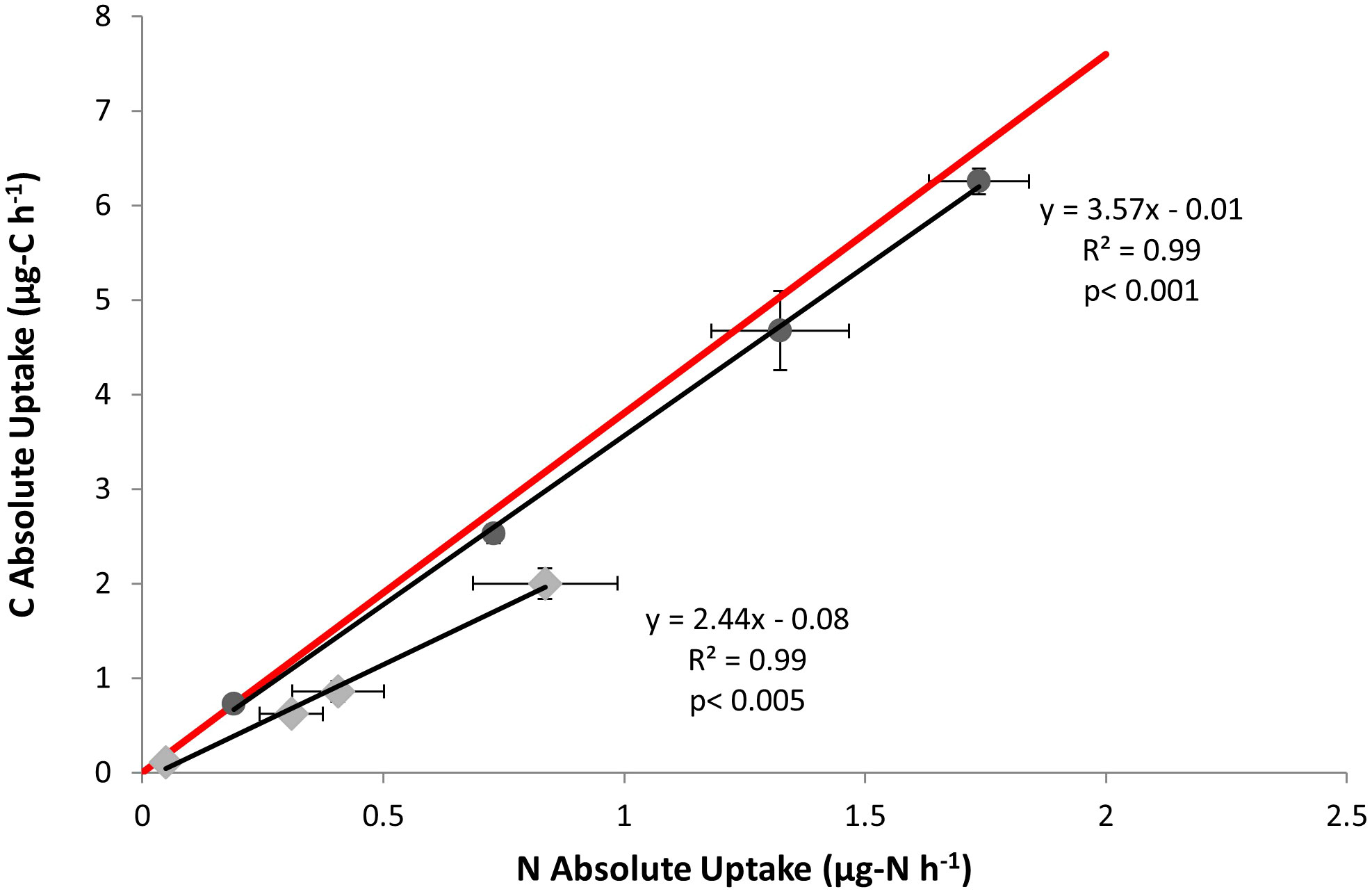
Figure 4 Specific uptake rates (pvolume) of N and C for the each four concentrations of AA mixture used in pico-cyanobacteria (♦) and nanochlorophyte (●) experiments. Each point represents the mean of three experimental replicates ± one S.E. The red line indicates C:N ratio (by mass) of the AA mixture used.
3.3 DIP and DOP uptake
Uptakes velocities (pg-P h-1 cell-1) are presented in Figure 5 for both cultures and P forms. It did not appear that any of the experiments reached saturating conditions as all cell-specific rates were linear up to the 50 µM-P tested. However, DOP uptake by the nanochlorophyte culture followed a logarithmic pattern. Additionally, for picocyanobacteria, uptake rates were actually higher for DOP compared to DIP, while for the nanochlorophyte cultures, DIP uptake rates at each concentration were typically double those for DOP (Figure 5).
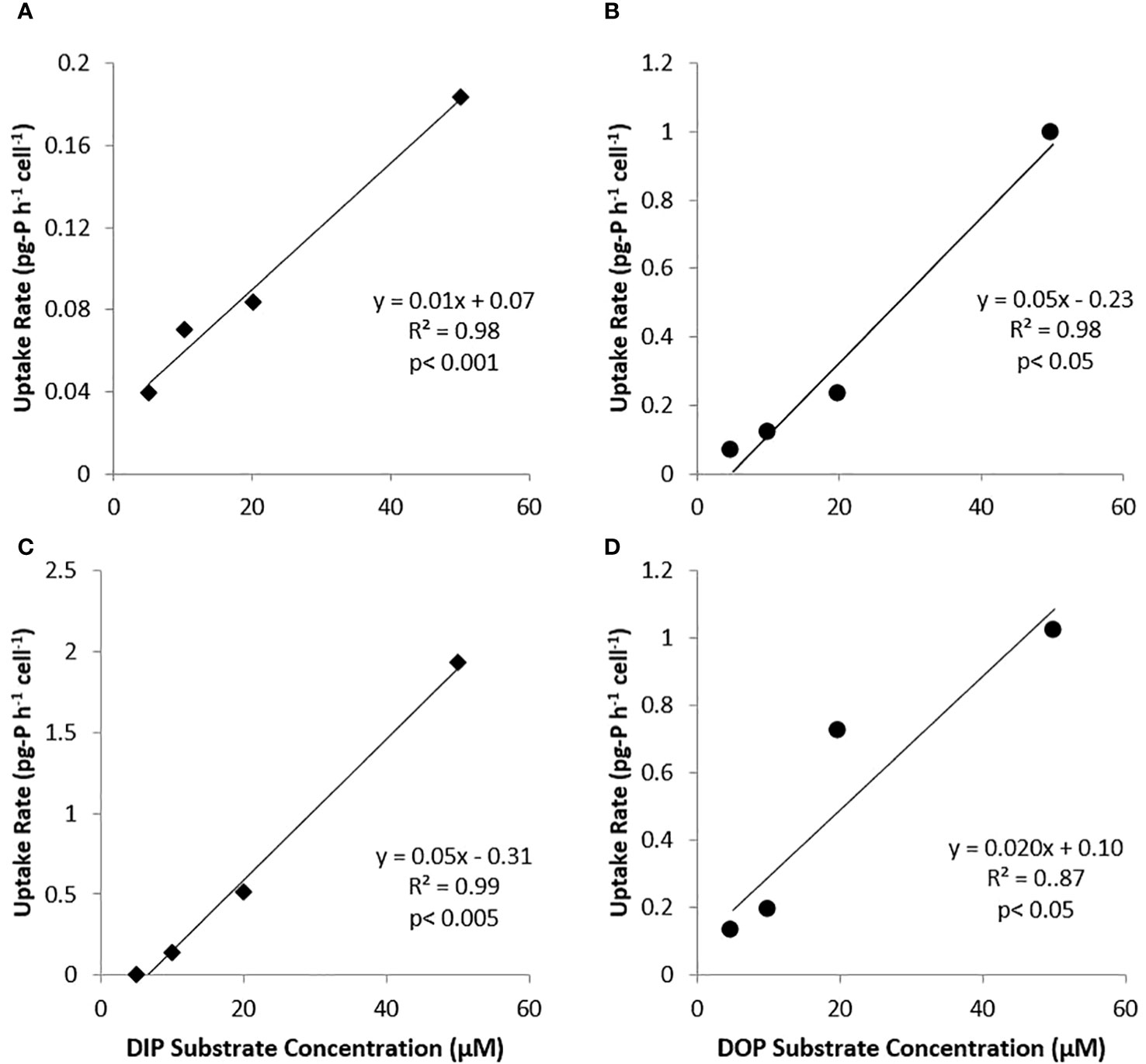
Figure 5 Plots of DIP (♦) and DOP (●) uptake velocities (pg-P h-1 cell-1) by substrate concentration for picocyanobacteria (A, B) and nanochlorophyte (C, D) cultures.
APA assays and 13C-glucose-6-phosphate spikes showed additional signs of organic P use in cultures. APA was detected in both cultures and was an order of magnitude higher for picocyanobacteria when corrected for differences in cell density (Table 4). Even though both cultures showed uptake of DOP, APA was not detected in picocyanobacteria cultures from the 2016 experiment, and APA was an order of magnitude lower for the nanochlorophyte culture when compared to the 2015 experiment but similar when corrected for differences in cell densities. Additionally, background APA was not detected for culture filtrate, suggesting that alkaline phosphatase was largely associated with the particulate material greater than 0.7 µm. Enrichment from 13C-G6P uptake was detected in biomass from both cultures. Carbon uptake relative to organic P uptake increased logarithmically with DOP concentration for the nanochlorophyte cultures but linearly over the range tested for picocyanobacteria (Figure 6).
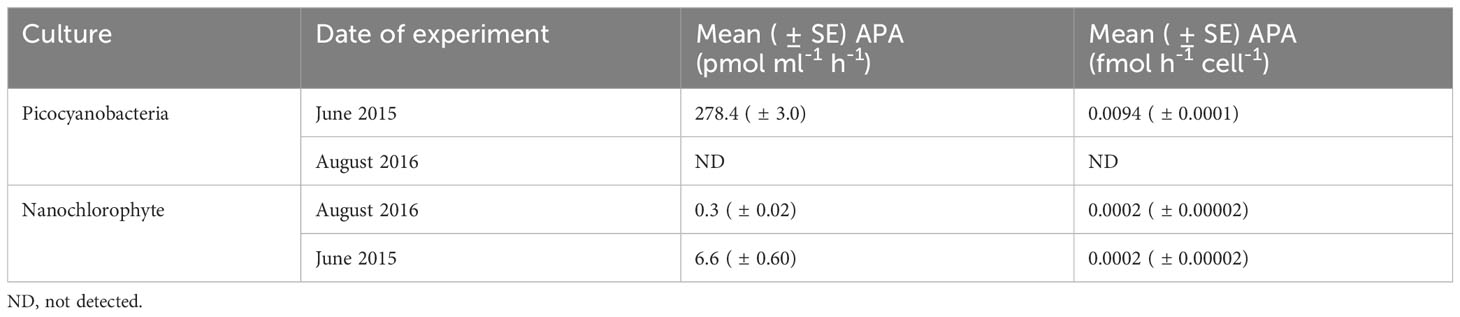
Table 4 Total and cell-corrected alkaline phosphatase activity (APA) for picocyanobacterial and nanochlorophyte cultures used in P-uptake experiments in 2015 and 2016.
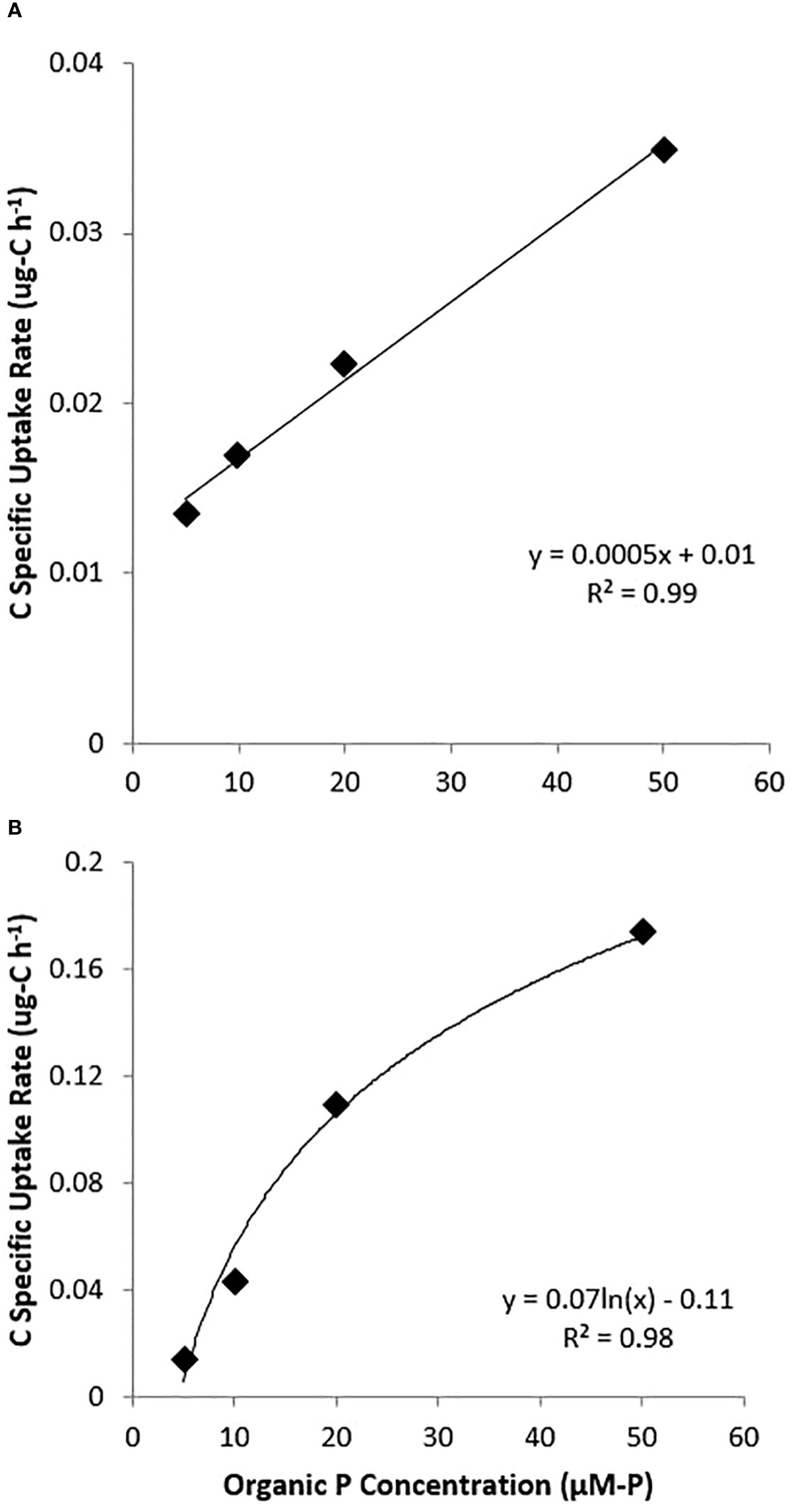
Figure 6 Uptake rate of carbon calculated for picocyanobacteria (A) and nanochlorophyte (B) cultures spiked with 13C-labeled DOP. Each point represents one experimental replicate.
4 Discussion
4.1 Nitrogen form preference
Previous research, both in field and culture uptake experiments, has shown a variety of forms of inorganic and organic nitrogen can initiate and sustain phytoplankton blooms (Table 5). The results of our study add useful information about the nutritional preference of novel bloom-forming taxa in the IRL and support the hypothesis that the picocyanobacteria and nanoeukaryote that dominated the 2011 ‘Superbloom’ can effectively utilize a variety of nitrogen compounds, including organic forms, and helps explain their success during a period of time in the ecosystem when recycled forms of nitrogen (i.e., NH4+ and organic forms) were enhanced.
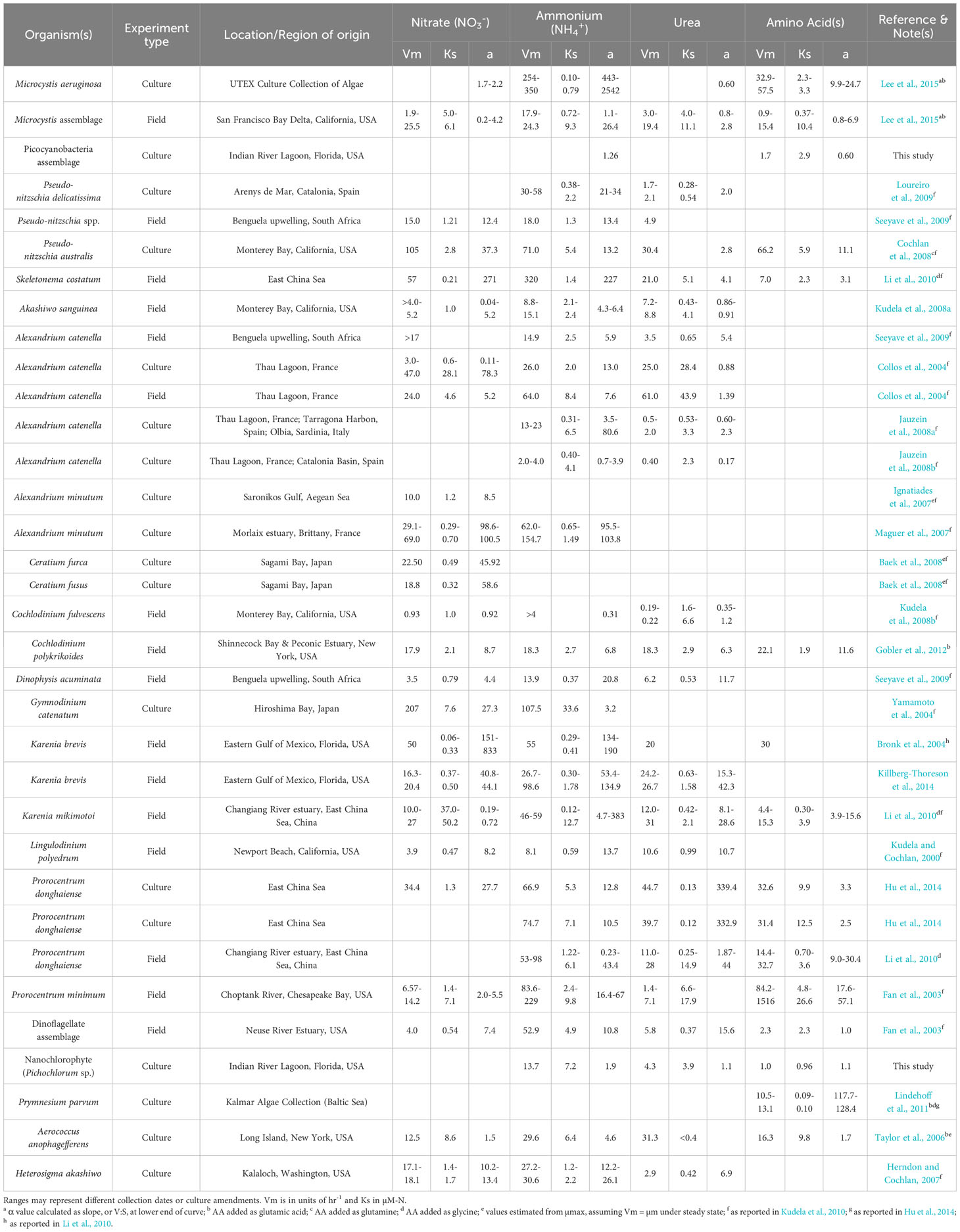
Table 5 Comparison of nitrogen uptake kinetic parameters for various coastal HAB species from published field and culture-based experiments.
Kinetic parameters are a key component to trait-based phytoplankton community models, although the variation in these parameters is typically observed in short-term experiments which has made scaling to models difficult when considering longer time frames, wider ranges in substrate concentrations, and taxonomic variation (Smith et al., 2009; Edwards et al., 2012; Smith et al., 2014). Phytoplankton with low Ks values are theorized to be competitive at low ambient nutrient concentrations, such as those typical in the IRL, and Ks values observed for picocyanobacteria and nanochlorophyte compare with those for other coastal bloom-forming species (Table 5). The proposed physiological trade-off between maximum uptake (i.e., Vmax) and the half-saturation constant (i.e., Ks) at the cellular level would suggest that phytoplankton can either grow quickly at high nutrient concentrations or are more competitive at low nutrient concentrations, respectively.
One potential limitation of these kinetic terms, however, is that they may not reflect a dynamic balance between acquisition and assimilation machinery within the cells, both of which may not respond immediately to substrate deficiency or saturation (Aksnes and Cao, 2011). Additionally, there is still debate as to whether this trade-off exists (Friksen et al., 2013). Rather, the affinity term has been more clearly defined as the ratio of Vmax and Ks, or the initial slope of the Michaelis-Menten curve (Healey, 1980). Here, both picocyanobacteria and nanochlorophyte cultures had highest affinity for NH4+ followed by the AA mixture and urea (Table 3).
For picocyanobacteria, uptake of NH4+ by was rapid and linear (Figure 1-1, Tables 1–3), suggesting cells were not saturated even at the highest concentration tested (10 µM-N) which exceeded the range of DIN concentrations observed during the IRL 2011 ‘Superbloom’ (mean values of 2.54 ± 0.34 µM NH4+ and 1.70 ± 0.21 µM NO3-) (LaPointe et al., 2015). For NO3-, uptake was also linear, but rates were very low or undetectable (Figure 1-2). Linear uptake may instead be a result of diffusion into the cell (Lomas and Glibert, 1999b), although it has also been suggested that non-saturable kinetics at higher concentrations may be a potential adaptation for uptake at high concentrations (Fan et al., 2003). Notably, our range of 15N-DIN concentrations exceeded the range of ambient concentrations specifically observed during the 2011 ‘Superbloom’, and it is unlikely phytoplankton in the IRL would need to adapt to high NH4+ concentrations that can inhibit uptake or growth of competing phytoplankton (Glibert et al., 2016; Kang et al., 2020). Indeed, picocyanobacteria showed relatively high but linear uptake rates for NH4+, which may be indicative of the latter case, while the uptake response for NO3- may represent the baseline diffusion rates into cells.
As expected, our results showed significant preference for NH4+ compared to NO3- for both picocyanobacteria and nanochlorophyte groups (Figure 3). It is well established that for many phytoplankton, NH4+ is more easily transported across cell membranes relative to NO3- and its reduction pathway results in a lower net energetic cost for the cell. Various species have exhibited higher growth rates when grown on NH4+ versus NO3- (e.g., Herndon and Cochlan, 2007), although it has been shown in certain cases that diatoms in particular can be NO3- opportunists (Lomas and Glibert, 1999a). There is significant evidence that these preferences are controlled at the cellular level, with diatoms exhibiting different transporters and transporter affinities compared to cyanobacteria, chlorophytes, and dinoflagellates (Song and Ward, 2007; Chan et al., 2011; Kang and Chang, 2014).
The preference of N forms is not only a function of favorable uptake, but also repression as well, where for example, even at low (µM) concentrations, NH4+ has been shown to inhibit uptake of NO3- in cultures and in field experiments (Cochlan and Harrison, 1991; Lomas and Glibert, 1999a; Lomas and Glibert, 1999b). This may explain why NO3- concentrations in cultures were approximately five and 13-fold higher than NH4+ at initiation of the experiments (Table 1), despite equal additions at the onset of culturing, and why picocyanobacteria did not appear to utilize much of the added 15NO3-.
4.2 The Role of DON in blooms
Despite cultures displaying highest affinity for NH4+, the 2011 ‘Superbloom’ and subsequent blooms in the northern IRL are not typically associated with high dissolved inorganic nutrients (i.e. DIN, SRP), but rather overall high total dissolved nutrients concentrations (i.e. TDN, TDP) and/or high TDN : TDP ratios due to high scavenging of inorganic nutrients (LaPointe et al., 2015). It seems likely then that organic N substrates play some sort of role in triggering and/or sustaining the recent blooms in the IRL (Gobler et al., 2013; LaPointe et al., 2015). Recent genomic studies demonstrate that uptake of organic substrates appears widespread in marine and coastal picoplankton (Yelton et al., 2016; Muñoz-Marín et al., 2020; Hagström et al., 2021), and our results provide the first direct and confirmed evidence for uptake of organic substrates by the picocyanobacteria and nanochlorophyte groups. These results are also consistent with findings in other coastal systems that DON additions can trigger picocyanobacteria and small-celled eukaryotes including Aureoumbra lagunensis (D.A. Stockwell, DeYoe, Hargraves and P.W. Johnson) blooms observed in the IRL following the 2011 ‘Superbloom’ (Berg et al., 1997; Glibert et al., 2001; Glibert et al., 2004; Gobler et al., 2013; Kang et al., 2015; Ivey et al., 2020). Urea in particular has been implicated in several coastal HABs as an available DON source (Anderson et al., 2002; Glibert et al., 2005; Glibert et al., 2006), but while it is considered to be a more favorable N-source than NO3-, the necessity of urease enzymes can make acquisition more energy intensive when compared to NH4+ (Berman and Bronk, 2003). We did not specifically measure urease activity in the cultures, and background urea concentrations were not measured in order to calculate an RPI value for urea. However, both cultures showed direct uptake of urea and the AA mixture, which suggests the picocyanobacteria and nanochlorophyte can use DON sources at low concentrations if needed.
There is evidence that a wide variety of marine phytoplankton taxa have the ability to hydrolyze peptides extracellularly and utilize smaller fragments as a source of N (Mulholland and Lee, 2009). As previously noted, cultures appeared to uptake both C and N from the AA mixture they were supplied with (Figure 4). In the nanochlorophyte culture, the ratio of C:N was almost equal to that of the supplied AA indicating a more direct AA uptake and possible organic C use. Mixotrophy, is another trait with potential advantages and tradeoffs over strict heterotrophy or photoautotrophy for potential HAB-forming dinoflagellates observed in the IRL (Phlips et al., 2015). Despite the incorporation of 13C from the AA mixture into picocyanobacteria and nanochlorophyte cultures during the short-term incubation used in these experiments, these alone are not indicative of mixotrophy. Importantly, under definitions of mixotrophy requiring cell-ingestion (Flynn et al., 2013), any observed incorporation of organic N and P by the picocyanobacteria and nanoeukaryote taxa was likely osmotrophic.
In contrast to the results of the nanochlorophyte culture, in the picocyanobacterial culture there was faster incorporation of AA N over C (Figure 4). The specific mechanism explaining this pattern of uptake is unknown; however, one explanation may be the preferential use of more N-rich amino acids. Previous research using 14C-labeled substrates has also suggested that some phytoplankton possess cell-surface enzymes that specifically target N from amino acids and leave the remaining C behind (Berman and Bronk, 2003). Additionally, extraneous C from the dissolved OM pool can be excreted while the N is still retained (Berman and Bronk, 2003).
4.3 Inorganic versus organic phosphorus utilization
Although N enrichment has been the focus in more N-limited estuaries, there is also evidence for periods of P-limitation on phytoplankton in the north-central region of the IRL (Phlips et al., 2002). Indeed, there is a well-documented gradient in P availability with lower TP and TDP concentrations in the northern basins and decreasing N:P ratios moving from north to south in the lagoon (Sigua et al., 2000; Phlips et al., 2002; LaPointe et al., 2015). This gradient has historically been attributed to higher P-loading in the watersheds feeding the central and southern IRL (Sigua and Tweedale, 2003), but P limitation can also arise in certain coastal waters if the majority of the TDP is in the form of DOP rather than DIP (Yamamoto et al., 2004).
During the 2011 ‘Superbloom’, ambient DIP and TDP concentrations in affected basins were less than 1 and 2 µM, respectively (LaPointe et al., 2015). Picocyanobacteria have been shown in other lagoon systems to be adept at scavenging DIP at low concentrations, outcompeting other taxa such as diatoms (Collos et al., 2009). Although some marine phytoplankton may have half-saturation values in the nanomolar concentrations, indicating adaptation to low-P environments (Lomas et al., 2014), we could not calculate kinetic parameters for DIP or DOP uptake due to experimental limitations. Given methodological constraints of measuring P colorimetrically at low concentrations in the culture medium, we were only able to measure uptake kinetics of DIP and DOP to a final concentration of 5 µM-P; however, uptake was typically linear throughout the concentration range, signifying that cultures were not P-saturated at concentrations beyond those naturally seen in the IRL.
Incorporation of 13C-G6P also indicates that the picocyanobacteria and nanochlorophyte species are capable of using organic P sources whether directly or indirectly. Jauzein et al. (2010) measured 33PO4-P uptake kinetics in the toxic dinoflagellate Alexandrium catenella under P-limiting conditions and found that cells were best at obtaining inorganic P through expression of APA at sub-micromolar levels of DIP. In this study, APA was detected in most of the picocyanobacteria and nanochlorophyte cultures with similar background DIP concentrations, but was only significant in picocyanobacteria cultures in June 2015 (Table 4). DOP uptake was observed even in the cultures without measurable APA indicating that direct use of DOP compounds likely occurred.
Phosphatases are widely occurring among marine and coastal microbes, and picoplankton species. HAB species able to utilize the organic P pool through extracellular enzyme activity likely have a significant advantage under otherwise P-limiting conditions (Dyhrman and Ruttenberg, 2006; Kathuria and Martiny, 2011; Ivancic et al., 2012; Burthold and Shumann, 2020). It has been suggested that differential APA responses to P loading may be able to influence species abundance within the phytoplankton community (Nicholson et al., 2006).
Additionally, picocyanobacteria and nanochlorophyte biomass grown under both P- and N-limited conditions maintained cellular N:P ratios moderately above Redfield ratio (i.e., 16:1). This suggests that the minimum cell quotas for phosphorus in both groups are lower than the ‘typical’ value represented by the Redfield ratio for oceanic phytoplankton populations. As pointed out by Reynolds (2006), the “Redfield ratio is not diagnostic but an approximation to a normal condition”. Normal Redfield ratios for individual phytoplankton species vary widely (Klausmeier et al., 2004; Weber and Deutsch, 2010; Quigg et al., 2011; Martiny et al., 2014). For example, studies of the bloom-forming nanoeukaryote Aureoumbra lagunensis in Laguna Madre, Texas, revealed well-above average Redfield ratios attributed to the species low cell quotas for phosphorus (Liu et al., 2001; DeYoe et al., 2007). Similarly, several studies of the picocyanobacteria Synechococcus have shown ratios above the Redfield ratio in response to variations in cell quotas for phosphorus and other elements (Twining et al., 2010; Cunningham and John, 2017; Liu et al., 2019).
There is also evidence that phytoplankton are able to lower cellular P-demands in order to survive these periods, potentially by altering the composition of membrane lipids or otherwise reallocating internal P supplies (Geider and La Roche, 2002; Arrigo, 2005; Van Mooy et al., 2009). For example, some cyanobacteria are particularly adept at accumulating and storing P as polyphosphate following P uptake which may be adaptive to low or fluctuating inorganic P supplies (Hagemann et al., 2019), a trait which has also been observed in certain microalgae (Sanz-Luque et al., 2020 and references therein). Similarly, N can be stored by cyanobacteria internally in accessory pigments such as phycocyanins and has been observed to be promoted in environments with more reduced N which may allow for blooms to sustain beyond bioavailable N supply (Gladfelter et al., 2022). Although the entire phytoplankton community in the northern IRL may experience episodic P-limitation, there appears to be evidence suggesting the picocyanobacteria and nanoeukaryote species are adept at exploiting this stressor.
4.4 Ecological considerations
In the Indian River Lagoon, there has been regime shift towards a greater predominance of blooms involving smaller-celled phytoplankton, such as those observed in the 2011 ‘Superbloom’ (Phlips et al., 2021). Our culture experiments show these groups prefer reduced N species and use organic N under N-limiting conditions. Dugdale and Goering (1967) proposed a conceptual model of marine pelagic food webs where allochthonous inputs (i.e., NO3-) lead to dominance by either diatoms, while regenerated sources (NH4+ and other reduced N forms) to a dominance by mixotrophic dinoflagellates, picocyanobacteria, and heterotrophic bacteria. Further experimentation has shown that systems with reduced N species (i.e., NH4+ and urea) favoring mixotrophic dinoflagellates and small-celled phytoplankton and bacteria (Berg et al., 1997; Glibert et al., 2001; Berg et al., 2003).
The interplay between nutrient delivery and water residence time can also be an important factor in lagoonal systems, and periods of high residence time can correspond with HABs despite decreased external nutrient inputs (Phlips et al., 2015; Phlips et al., 2020; Cira et al., 2021). Historically in the IRL, diatoms and eurythermal dinoflagellates typically dominated blooms during colder months of the year (Phlips et al., 2015), paralleling similar spring-to-summer bloom succession exhibited in the pelagic ocean and certain temperate estuaries (Townsend et al., 1994; Smayda and Trainer, 2010). During late spring and summer, large rainfall events, pulses of bioavailable nutrients and warm temperatures were linked with the occurrence of blooms of Pyrodinium bahamense (Phlips et al., 2004; Phlips et al., 2015). Small-celled, fast-growing diatoms and picocyanobacteria have also been shown to respond quickly to pulses of nutrients (Varona-Cordero et al., 2014; Burthold and Shumann, 2020), and notably these groups bloomed prior to the 2011 ‘Superbloom’ (Phlips et al., 2021). Conversely, the 2011’Superbloom’ also followed an extended drought and historically cold winter triggering a significant loss of benthic vegetation and elevated water-column nutrients (Phlips et al., 2015; Phlips et al., 2021). Not only is seagrass biomass a competitor for inorganic nutrients and a significant sink for N and P, but a loss in seagrass can alter the sediment environment to favor nutrient fluxes to the water-column itself (McGlathery et al., 2007 and references therein, Delgard et al., 2013). In the Baltic Sea for example, there is significant evidence that cyanobacterial blooms are sustained by a cycle of hypoxia, nutrient flux from the sediments, and blooms that eventually crash (Vahtera et al., 2007; Funkey et al., 2014; Zilius et al., 2014). The loss of seagrass uptake along with the input of organic matter from dying seagrass biomass may have triggered similar conditions in the IRL by releasing available N and P from sediment mineralization. A lack of competition following seagrass die-off was linked to picocycanobacteria blooms in Florida Bay, although high organic N loading and subsequent high concentrations of NH4+ among other stressors were concluded to contribute to the state change (Phlips et al., 1999; Glibert et al., 2023).
In all, the small-celled phytoplankton in the 2011 ‘Superbloom’ were likely strong competitors under inorganic nutrient limitation, and the turnover of productivity from reduced grazing, bacterial degradation, and a loss of benthic SAV likely favored phytoplankton groups able to utilize recycled and organic nutrients (i.e., NH4+, DON, DOP). Subsequent blooms of the small-celled pelagophyte A. lagunensis with other nanoplankton in 2013, 2015,5-16, and 2018-2019 (Gobler et al., 2013; Phlips et al., 2015; Phlips et al., 2021) and a novel nanoplanktonic cyanobacteria bloom in 2020 (Lopez et al., 2021) further support the theory of a shift in regimes in the IRL that may in part be due to alterations to nutrient forms and availability (Phlips et al., 2015; Phlips et al., 2021).
5 Conclusions
There is significant evidence that certain groups of HAB-forming phytoplankton are particularly adept at using ammonium and organic N and P forms (Glibert et al., 2014; Glibert et al., 2016 and references therein). The results from this study demonstrate for the first time that the bloom-forming groups responsible for the 2011 ‘Superbloom’ in the IRL are also directly capable of using organic N and P forms with a strong preference for NH4+ over NO3-. These traits are likely favorable during periods of low inorganic nutrients or to sustain blooms when N and P are recycled as reduced or organic compounds. These conditions may be seen in the IRL prior to bloom-initiation when external supply is low (e.g., drought) or when there is a significant turnover of the productivity during the bloom either from grazing or bacterial turnover. In the IRL, the shift in blooms of larger-sized phytoplankton such as diatoms and dinoflagellates to blooms of picocyanobacteria and smaller-celled eukaroytes may be driven by changes in nutrient supply but is likely linked to larger forces such as climate as well (Phlips et al., 2015).
The results from this study are useful for parameterizing ecosystem models that incorporate the physiology of HABs; however, such models must also incorporate ecological stoichiometry and predator-prey relationships (Flynn, 2010; Glibert, 2012). As both carbon and nutrients can potentially be exchanged between different phytoplankton or accumulated internally to be recycled for later blooms, it important to better understand the trophic interactions between pico- and nanoplankton, zooplankton and other mixotrophic plankton feeders, and the broader bacterioplankton community (i.e. the “microbial loop”) in the IRL (Flynn et al., 2019). This study also indicates that future considerations of water quality in northern IRL basins should include both N and P. It is increasingly evident that the species responsible for recent blooms have diverse, but potentially predictable, nutritional preferences.
Data availability statement
The raw data supporting the conclusions of this article will be made available by the authors, without undue reservation.
Author contributions
JP: Data curation, Formal analysis, Investigation, Methodology, Writing – original draft. PI: Conceptualization, Funding acquisition, Investigation, Methodology, Resources, Supervision, Writing – review & editing. EP: Conceptualization, Funding acquisition, Methodology, Project administration, Writing – review & editing. ML: Conceptualization, Funding acquisition, Project administration, Writing – review & editing.
Funding
The author(s) declare financial support was received for the research, authorship, and/or publication of this article. The research was in part supported by the St. Johns River Water Management District (Palatka, Florida, USA), Contract #27887. Edward Phlips is in part supported by the USDA National Institute of Food and Agriculture, Hatch Project 1017098.
Conflict of interest
The authors declare that the research was conducted in the absence of any commercial or financial relationships that could be construed as a potential conflict of interest.
Publisher’s note
All claims expressed in this article are solely those of the authors and do not necessarily represent those of their affiliated organizations, or those of the publisher, the editors and the reviewers. Any product that may be evaluated in this article, or claim that may be made by its manufacturer, is not guaranteed or endorsed by the publisher.
References
Aksnes D. L., Cao F. J. (2011). Inherent and apparent traits in microbial nutrient uptake. Mar. Ecol. Prog. Ser. 440, 41–51. doi: 10.3354/meps09355
Anderson D. M., Glibert P. M., Burkholder J. M. (2002). Harmful algal blooms and eutrophication: nutrient sources, composition, and consequences. Estuaries 25, 704–726. doi: 10.1007/BF02804901
Arrigo K. R. (2005). Marine microorganisms and global nutrient cycles. Nature 437, 349–355. doi: 10.1038/nature04159
Baek S. H., Shimode S., Han M. S., Kikuchi T. (2008). Growth of dinoflagellates, Ceratium furca and Ceratium fusus in Sagami Bay, Japan: The role of nutrients. Harmful Algae 7 (6), 729–739. doi: 10.1016/j.hal.2008.02.007
Barile P. J. (2018). Widespread sewage pollution of the Indian River Lagoon system, Florida (USA) resolved by spatial analyses of macroalgal biogeochemistry. Mar. pollut. Bull. 128, 557–574. doi: 10.1016/j.marpolbul.2018.01.046
Behrenfeld M. J., Halsey K. H., Milligan A. J. (2008). Evolved physiological responses of phytoplankton to their integrated growth environment. Philos. T. R. Soc B 363, 2687–2703. doi: 10.1098/rstb.2008.0019
Berg G. M., Balode M., Purina I., Bekere S., Bechemin C., Maestrini S. Y. (2003). Plankton community composition in relation to availability and uptake of oxidized and reduced nitrogen. Aquat. Microb. Ecol. 30, 263–274. doi: 10.3354/ame030263
Berg G. M., Glibert P. M., Lomas M. W., Burford M. (1997). Organic nitrogen uptake and growth by the chrysophyte Aureococcus anophagefferens during a brown tide event. Mar. Biol. 129, 377–387. doi: 10.1007/s002270050178
Berman T., Bronk D. A. (2003). Dissolved organic nitrogen: a dynamic participant in aquatic ecosystems. Aquat. Microb. Ecol. 31, 279–305. doi: 10.3354/ame031279
Bronk D. A., Sanderson M. P., Mulholland M. R., Heil C. A., O'Neil J. M. (2004). “Organic and inorganic nitrogen uptake kinetics in field populations dominated by Karenia brevis,” in Harmful Algae. Eds. Steidinger K., Vargo G. A., Heil C. A. (St. Petersburg, FL: Florida Fish and Wildlife Conservation Commission, Florida Institute of Oceanography and Intergovernmental Oceanographic Commission of UNESCO), 2002 80–82.
Burford M. A., Carey C. C., Hamilton D. P., Huisman J., Paerl H. W., Wood S. A., et al. (2020). Perspective: Advancing the research agenda for improving understanding of cyanobacteria in a future of global change. Harmful Algae 91, 101601. doi: 10.1016/j.hal.2019.04.004
Burkholder J. M., Dickey D. A., Kinder C. A., Reed R. E., Mallin M. A., McIver M. R., et al. (2006). Comprehensive trend analysis of nutrients and related variables in a large eutrophic estuary; A decadal study of anthropogenic and climatic influences. Limnol. Oceanogr. 51 (1), 462–487. doi: 10.4319/lo.2006.51.1_part_2.0463
Burthold M., Shumann R. (2020). Phophorus dynamics in a eutrophic lagoon: Uptake and utilization of nutrient pulses by phytoplankton. Front. Mar. Sci. 7, 281. doi: 10.3389/fmars.2020.00281
Chan C. X., Reyes-Prieto A., Bhattacharya D. (2011). Red and green algal origin of diatom membrane transporters: Insights into environmental adaptation and cell evolution. PLoS One 6, e29138. doi: 10.1371/journal.pone.0029138
Cira E., Palmer T., Wetz M. (2021). Phytoplankton dynamics in a low-inflow estuary (Baffin Bay, TX) during drought and high-rainfall conditions associated with an El Niño event. Estuar. Coast. 44, 1752–1764. doi: 10.1007/s12237-021-00904-7
Cochlan W. P., Harrison P. J. (1991). Inhibition of nitrate uptake by ammonium and urea in the eukaryotic picoflagellate Micromonas pusilla (Butcher) Manton et Parke. J. Exp. Mar. Biol. Ecol. 153, 143–152. doi: 10.1016/0022-0981(91)90221-H
Cochlan W. P., Herndon J., Kudela R. M. (2008). Inorganic and organic nitrogen uptake by the toxigenic diatom Pseudo-nitzschia australis (Bacillariophyceae). Harmful Algae 8, 111–118. doi: 10.1016/j.hal.2008.08.008
Collos Y., Bec B., Jauzein C., Abadie E., Laugier T., Lautier J., et al. (2009). Oligotrophication and emergence of picocyanobacteria and a toxic dinoflagellate in Thau lagoon, southern France. J. Sea Res. 61 (1-2), 68–75. doi: 10.1016/j.seares.2008.05.008
Collos Y., Gagne C., Laabir M., Vaquer A., Cecchi P., Souchu P. (2004). Nitrogenous nutrition of Alexandrium catenella (Dinophyceae) in cultures and in Thau Lagoon, Southern France. J. Phycol. 40 (1), 96–103. doi: 10.1046/j.1529-8817.2004.03034.x
Conley D. J., Paerl H. W., Howarth R. W., Boesch D. F., Seitzinger S. P., Havens K. E., et al. (2009). Controlling eutrophication: Nitrogen and phosphorus. Science 323 (5917), 1014–1015. doi: 10.1126/science.116775
Cunningham B. R., John S. G. (2017). The effect of iron limitation on cyanobacteria major nutrient and trace metal stoichiometry. Limnol. Oceanogr. 62, 846–858. doi: 10.1002/lno.10484
Dames N. R., Wallschuss S., Rocke E., Pitcher G., Rybicki E., Pfaff M., et al. (2023). Short-term dynamics of nano- and picoplankton production ein an embayment of the Benguela upwelling regionCoastal. Shelf Sci. 284, 108285. doi: 10.1016/j.ecss.2023.108285
Davidson K., Gowen R. J., Harrison P. J., Fleming L. E., Hoagland P., Moschonas G. (2014). Anthropogenic nutrients and harmful algae in coastal waters. J. Environ. Manage. 146, 206–216. doi: 10.1016/j.jenvman.2014.07.002
Delgard M. L., Deflandre B., Deborde J., Richard M., Charbonnier C., Anschutz P. (2013). Changes in nutrient biogeochemistry in response to the regression of Zostera notii meadows in the Arcachon Bay (France). Aquat. Geochem. 19 (3), 241–259. doi: 10.1007/s10498-013-9192-9
De Philippis R., Vincenzini M. (1998). Exocellular polysaccharides from cyanobacteria and their possible applications. FEMS Microbiol. Rev. 22 (3), 151–175. doi: 10.1016/S0168-6445(98)00012-6
DeYoe H. R., Buskey E. J., Jochem F. J. (2007). Physiological responses of Aureoumbra lagunensis and Synechococcus sp. to nitrogen addition in a mesocosm study. Harmful Algae 6, 48–55. doi: 10.1016/j.hal.2006.06.001
Duan S., Bianchi T. S. (2007). Particulate and dissolved amino acids in the lower Mississippi and Pearl Rivers (USA). Mar. Chem. 107, 214–229. doi: 10.1016/j.marchem.2007.07.003
Dugdale R. C., Goering J. J. (1967). Uptake of new and regenerated forms of nitrogen in primary productivity. Limnol. Oceanogr. 12 (2), 196–206. doi: 10.4319/lo.1967.12.2.0196
Dyhrman S. T., Ruttenberg D. C. (2006). Presence and regulation of alkaline phosphatase activity in eukaryotic phytoplankton from the coastal ocean: implications for dissolved organic phosphorus remineralization. Limnol. Oceanogr. 53, 1381–1390. doi: 10.4319/lo.2006.51.3.1381
Edwards K. F., Thomas M. K., Klausmeier C. A., Litchman E. (2012). Allometric scaling and taxonomic variation in nutrient utilization traits and maximum growth rate of phytoplankton. Limnol. Oceanogr. 57, 554–566. doi: 10.4319/lo.2012.57.2.0554
EPA. (1993b). Method 353.2: Determination of nitrate nitrogen by semi-automated colorimetry, Revision 2.0.
EPA. (Environmental Protection Agency) (1993a). Method 350.1: Determination of ammonia nitrogen by semi-automated colorimetry, Revision 2.0.
Fan C., Glibert P. M., Burkholder J. M. (2003). Characterization of the affinity for nitrogen, uptake kinetics, and environmental relationships for Prorocentrum minimum in natural blooms and laboratory cultures. Harmful Algae 2, 283–299. doi: 10.1016/S1568-9883(03)00047-7
Flynn K. J. (2010). Ecological modelling in a sea of variable stoichiometry: Dysfunctionality and the legacy of Redfield and Monod. Prog. Oceanogr. 84 (1-2), 52–65. doi: 10.1016/j.pocean.2009.09.006
Flynn K. J., Mitra A., Anestis K., Anschütz A. A., Calbet A., Ferreira G. D., et al. (2019). Mixotrophic protists and a new paradigm for marine ecology: where does plankton research go now? J. Plankt. Res. 41 (4), 375–391. doi: 10.1093/plankt/fbz026
Flynn K. J., Stoecker D. K., Mitra A., Raven J. A., Glibert P. M., Hansen P. J., et al. (2013). Misuse of the phytoplankton–zooplankton dichotomy: the need to assign organisms as mixotrophs within plankton functional types. J. Plankton Res. 35, 3–11. doi: 10.1093/plankt/fbs062
Friksen O., Follows M. J., Aksnes D. L. (2013). Trait-based models of nutrient uptake in microbes extend the Michaelis-Menten framework. Limnol. Oceanogr. 58 (1), 193–202. doi: 10.4319/lo.2013.58.1.0193
Funkey C. P., Conley D. J., Reuss N. S., Humborg C., Jilbert T., Slomp C. P. (2014). Hypoxia sustains cyanobacteria blooms in the Baltic Sea. Environ. Sci. Technol. 48 (5), 2598–2602. doi: 10.1021/es404395a
Galimany E., Lunt J., Freeman C. J., Houl J., Sauvage T., Santos L., et al. (2020). Bivalve feeding responses to microalgal bloom species in the Indian river lagoon: the potential for top-down control. Estuar. Coast. 43, 1519–1532. doi: 10.1007/s12237-020-00746-9
Geider R. J., La Roche J. (2002). Redfield revisited: Variability of C:N:P in marine microalgae and its biochemical basis. Eur. J. Phycol. 37, 1–17. doi: 10.1017/S0967026201003456
German D. P., Weintraub M. N., Grandy A. S., Lauber C. L., Rinkes Z. I., Allison S. D. (2011). Optimization of hydrolytic and oxidative enzyme methods for ecosystem studies. Soil Biol. Biochem. 43, 1387–1397. doi: 10.1016/j.soilbio.2011.03.017
Gladfelter M. F., Buley R. P., Belfiore A. P., Fernandez-Figueroa E. G., Gerovac B. L., Baker N. D., et al. (2022). Dissolved nitrogen form mediates phycocyanin content in cyanobacteria. Fresh. Biol. 67, 954–964. doi: 10.1111/fwb.13892
Glibert P. M. (2012). Ecological stoichiometry and its implications for aquatic ecosystem sustainability. Curr. Opin. Environ. Sustain. 4 (3), 272–277. doi: 10.1016/j.cosust.2012.05.009
Glibert P. M. (2020). Harmful algae at the complex nexus of eutrophication and climate change. Harmful Algae 91, 101583. doi: 10.1016/j.hal.2019.03.001
Glibert P. M., Allen J. I., Bouwman A. F., Brown C. W., Flynn K. J., Lewitus A. J., et al. (2010). Modeling of HABs and eutrophication: Status, advances, challenges. J. Mar. Syst. 83 (3-4), 262–275. doi: 10.1016/j.jmarsys.2010.05.004
Glibert P. M., Harrison J., Heil C., Seitzinger S. (2006). Escalating worldwide use of urea: A global change contributing to coastal eutrophication. Biogeochemistry 77 (3), 441–463. doi: 10.1007/s10533-005-3070-5
Glibert P. M., Heil C. A., Hollander D., Revilla M., Hoare A., Alexander J., et al. (2004). Evidence for dissolved organic nitrogen and phosphorus uptake during a cyanobacterial bloom in Florida Bay. Mar. Ecol. Prog. Ser. 280, 73–83. doi: 10.3354/meps280073
Glibert P. M., Heil C. A., Madden C. J., Kelly S. P. (2023). Dissolved organic nutrients at the interface of fresh and marine waters: flow regime changes, biogeochemical cascades and picocyanobacterial blooms—the example of Florida Bay, USA. Biogeochmistry 164, 229–255. doi: 10.1007/s10533-021-00760-4
Glibert P. M., Magnien R., Lomas M. W., Alexander J., Fan C., Haramoto E., et al. (2001). Harmful algal blooms in Chesapeake and Coastal Bays of Maryland, USA: comparisons of 1997, 1998, and 199 events. Estuaries 24, 875–883. doi: 10.2307/1353178
Glibert P. M., Seitzinger S., Heil C., Burkholder J. M., Parrow M. W., Codispoti L. A., et al. (2005). The role of eutrophication in the global proliferation of harmful algal blooms. Oceanography 18, 198–209. doi: 10.5670/oceanog.2005.54
Glibert P. M., Wilerson F. P., Dudgale R. C., Raven J. A., Dupont C. L., Leavitt P. R., et al. (2016). Pluses and minuses of ammonium and nitrate uptake and assimilation by phytoplankton and implactions for productivity and community composition, with emphasis on nitrogen-enriched conditions. Limnol. Oceanogr. 61, 165–197. doi: 10.1002/lno.10203
Gobler C. J., Burson A., Koch F., Tang Y., Mulholland M. R. (2012). The role of nitrogenous nutrients in the occurrence of harmful algal blooms caused by Cochlodinium polykrikoides in New York estuaries (USA). Harmful Algae 7, 64–74. doi: 10.1016/j.hal.2012.03.001
Gobler C. J., Koch F., Kang Y., Berry D. L., Tang Y. Z., Lasi M., et al. (2013). Expansion of harmful brown tides caused by the pelagophyte Aureoumbra lagunensis DeYoe et Sockwell, to the US East Coast. Harmful Algae 27, 29–41. doi: 10.1016/j.hal.2013.04.004
Gowen R. J., Tett P., Bresnan E., Davidson K., McKinney A., Harrison P. J., et al. (2012). Anthropogenic nutrient enrichment and blooms of harmful phytoplankton. Oceanography Mar. Biol.: Annu. Rev. 50, 65–126. doi: 10.1201/b12157-3
Hagemann M., Möke F., Springer A., Westermann L., Frank M., Wasmund N., et al. (2019). Cyanobacterium Nodularia spumigena strain CCY9414 accumulates polyphosphate under long-term P-limiting conditions. Aquat. Microb. Ecol. 82, 265–274. doi: 10.3354/ame01896
Hagström Å., Zweifel U. L., Sundh J., Osbeck C. M. G., Bunse C., Sjöstedt J., et al. (2021). Composition and seasonality of membrane transporters in marine picoplankton. Front. Microbiol. 12. doi: 10.3389/fmicb.2021.714732
Healey F. P. (1980). Slope of the Monod equation as an indicator of advantage in nutrient competition. Microb. Ecol. 5, 281–286. doi: 10.1007/BF02020335
Heiser J., Glibert P. M., Burkholder J. M., Anderson D. M., Cochlan W. (2008). Eutrophication and harmful algal blooms: A scientific consensus. Harmful Algae 8, 3–13. doi: 10.1016/j.hal.2008.08.006
Herndon J., Cochlan W. P. (2007). Nitrogen utilization by the raphidophyte Heterosigma akashiwo: uptake kinetics in laboratory cultures. Harmful Algae 6, 260–270. doi: 10.1016/j.hal.2006.08.006
Howarth R. W., Marino R. (2006). Nitrogen as the limiting nutrient for eutrophication in coastal marine ecosystems: Evolving views over three decades. Limnol. Oceanogr. 51 (1, part 2), 364–376. doi: 10.4319/lo.2006.51.1_part_2.0364
Hu Z. H., Duan S., Xu N., Mulholland M. R. (2014). Growth and nitrogen uptake kinetics in cultured Prorocentrum donghaiense. PLoS One 9 (7), e94030. doi: 10.1371/journal.pone.0094030
Ignatiades L., Gotsis-Skretas O., Metaxatos A. (2007). Field and culture studies on the ecophysiology of the toxic dinoflagellate Alexandrium minutum (Halim) present in Greek coastal waters. Harmful Algae 6, 153–165. doi: 10.1016/j.hal.2006.04.002
Inglett P. W., Reddy K. R. (2006). Investigating the use of macrophyte stable C and N isotopic ratios as indicators of wetland eutrophication: patterns in the P-affected everglades. Limnol. Oceanogr. 51, 2380–2387. doi: 10.4319/lo.2006.51.5.2380
Inglett P. W., Reddy K. R., McCormick P. V. (2004). Periphyton chemistry and nitrogenase activity in a northern Everglades ecosystem. Biogeochemistry 67, 213–233. doi: 10.1023/B:BIOG.0000015280.44760.9a
Inglett P. W., Reddy K. R., Newman S., Lorenzen B. (2007). Increased soil stable nitrogen isotopic ratio following phosphorus enrichment: historical patterns and tests of two hypotheses in a phosphorus-limited wetland. Oecologia 153, 99–109. doi: 10.1007/s00442-007-0711-5
Ivancic I., Godrijan J., Pfannkuchen M., Maric D., Gasparovic B., Djakovac T., et al. (2012). Survival mechanisms of phytoplankton in conditions of stratification-induced deprivation of orthophosphate: Northern Adriatic case study. Limnol. Oceanogr. 57 (6), 1721–1731. doi: 10.4319/lo.2012.57.6.1721
Ivey J. E., Wolny J. L., Heil C. A., Murasko S. M., Brame J. A., Parks A. A. (2020). Urea inputs drive picoplankton Blooms in Sarasota Bay, Florida, USA. Water 12, 2755. doi: 10.3390/w12102755
Jauzein C., Collos Y., Garcés E., Vila M., Maso M. (2008a). Short-term temporal variability of ammonium and urea uptake by Alexandrium catenella (Dinophyta) in cultures. J. Phycol. 44 (5), 1136–1145. doi: 10.1111/j.1529-8817.2008.00570.x
Jauzein C., Labry C., Youenou A., Quéré J., Delmas D., Collos Y. (2010). Growth and phosphorus uptake by the toxic dinoflagellate Alexandrium catenella (dinophyceae) in response to phosphate limitation. J. Phycol. 46 (5), 926–936. doi: 10.1111/j.1529-8817.2010.00878.x
Jauzein C., Loureiro S., Garcés E., Collos Y. (2008b). Interactions between ammonium and urea uptake by five strains of Alexandrium catenella (Dinophyceae) in culture. Aquat. Microb. Ecol. 53, 271–280. doi: 10.3354/ame01249
Kang L. K., Chang J. (2014). Sequence diversity of ammonium transporter genes in cultures and natural species of marine phytoplankton. J. Mar. Sci. Tech. (Taiwan) 22, 89–96. doi: 10.6119/JMST-013-0412-1
Kang Y., Koch F., Gobler C. (2015). The interactive roles of nutrient loading and zooplankton grazing in facilitating the expansion of harmful algal blooms caused by the pelagophyte, Aureoumbra lagunensis, to the Indian River Lagoon, FL, USA. Harmful Algae 49, 162–173. doi: 10.1016/j.hal.2015.09.005
Kang Y., Kang Y. H., Kim J. K., Kang H. Y., Kang C. K. (2020). Year-to-year variation in phytoplankton biomass in an anthropogenically polluted and complex estuary: a novel paradigm for river discharge influence. Mar. pollut. Bull. 161, 111756. doi: 10.1016/j.marpolbul.2020.111756
Kathuria S., Martiny A. C. (2011). Prevalence of a calcium-based alkaline phosphatase associated with the marine cyanobacteria Prochlorococcus and other ocean bacteria. Environ. Microbiol. 13, 74–83. doi: 10.1111/j.1462-2920.2010.02310.x
Killberg-Thoreson L., Mulholland M. R., Heil C. A., Sanderson M. P., O’Neil J. M., Bronk D. A. (2014). Nitrogen uptake kinetics in field populations and cultured strains of Karenia brevis. Harmful Algae 38, 73–85. doi: 10.1016/j.hal.2014.04.008
Klausmeier C. A., Litchman E., Daufresne T., Levin S. (2004). Optimal nitrogen-to-phosphorus stoichiometry of phytoplankton. Nature 429, 171–174. doi: 10.1038/nature02454
Kudela R. M., Cochlan W. P. (2000). Nitrogen and carbon uptake kinetics and the influence of irradiance for a red tide bloom off southern California. Aquat. Microb. Ecol. 21, 31–47. doi: 10.3354/ame021031
Kudela R. M., Lane J. Q., Cochlan W. P. (2008a). The potential role of anthropogenically derived nitrogen in the growth of harmful algae in California, USA. Harmful Algae 8, 103–110. doi: 10.1016/j.hal.2008.08.019
Kudela R., Ryan J., Blakely M., Lane J., Peterson T. (2008b). Linking the physiology and ecology of Cochlodinium to better understand harmful algal bloom events: a comparative approach. Harmful Algae 7, 278–292. doi: 10.1016/j.hal.2007.12.016
Kudela R. M., Seeyave S., Cochlan W. P. (2010). The role of nutrients in regulation and promotion of harmful algal blooms in upwelling systems. Prog. Oceanogr. 85, 122–135. doi: 10.1016/j.pocean.2010.02.008
LaPointe B. E., Herren L. W., Debortoli D. D., Vogel M. A. (2015). Evidence of sewage-driven eutrophication and harmful algal blooms in Florida’s Indian River Lagoon. Harmful Algae 43, 8–102. doi: 10.1016/j.hal.2015.01.004
Lee J., Parker A. E., Wilkerson F. P., Dugdale R. C. (2015). Uptake and inhibition kinetics of nitrogen in Microcystis aeruginosa: Results from cultures and field assemblages collected in the San Francisco Bay Delta, CA. Harmful Algae 47, 126–140. doi: 10.1016/j.hal.2015.06.002
Lewis W. M., Wutsbaugh W. A., Paerl H. W. (2011). Rationale for control of anthropogenic nitrogen and phosphorus to reduce eutrophication of inland waters. Environ. Sci. Technol. 45 (24), 10300–10305. doi: 10.1021/es202401p
Li J., Glibert P. M., Zhou M. (2010). Temporal and spatial variability in nitrogen uptake kinetics during harmful dinoflagellate blooms in the East China Sea. Harmful Algae 9, 531–539. doi: 10.1016/j.hal.2010.03.007
Liao X., Inglett P. W., Inglett K. S. (2014). Vegetation and microbial indicators of nutrient status: testing their consistency and sufficiency in restored calcareous wetlands. Ecol. Indic. 46, 358–366. doi: 10.1016/j.ecolind.2014.07.001
Lima M. J., Barbosa A. B., Correia C., Matos A., Cravo A. (2023). Patterns and predictors of phytoplankton assemblage structure in a coastal Lagoon: species-specific analysis needed to disentangle anthropogenic pressures from ocean processes. Water 15, 4238. doi: 10.3390/w15244238
Lindehoff E., Granéli E., Glibert P. M. (2011). Nitrogen uptake kinetics of Prymnesium parvum (Haptophyte). Harmful Algae 12, 70–76. doi: 10.1016/j.hal.2011.09.001
Lindroth P., Mopper K. (1979). High performance liquid chromatographic determination of subpicomole amounts of amino acids by precolumn fluorescence derivatization with o-phthaldialdehyde. Anal. Chem. 51, 1667–1674. doi: 10.1021/ac50047a019
Liu H., Laws E., Villareal T. A., Buskey E. (2001). Nutrient-limited growth of Aureoumbra lagunensis (Pelagophyceae), with implications for its capability to outgrow other phytoplankton species in phosphate-limited environments. J. Phycol. 37 (4), 500–508. doi: 10.1046/j.1529-8817.2001.037004500.x
Liu C., Shi X., Fan F., Wu F., Lei J. (2019). N:P ratio influences the competition of Microcystis with its picophytoplankton counterparts, Mychonastes and Synechococcus, under nutrient enrichment conditions. J. Freshw. Ecol. 34, 445–454. doi: 10.1080/02705060.2019.1622604
Lomas M. W., Bonachela J. A., Levin S. A., Martiny A. C. (2014). Impact of ocean phytoplankton diversity on phosphate uptake. Proc. Natl. Acad. Sci. 111 (49), 17540–17545. doi: 10.1073/pnas.1420760111
Lomas M. W., Glibert P. M. (1999a). Interactions between NH4+ and NO3- uptake and assimilation: Comparison of diatoms and dinoflagellates at several growth temperatures. Mar. Biol. 133, 541–551. doi: 10.1007/s002270050494
Lomas M. W., Glibert P. M. (1999b). Temperature regulation of nitrate uptake: a novel hypothesis about nitrate uptake and reduction in cool-water diatoms. Limnol. Oceanogr. 44, 556–572. doi: 10.4319/lo.1999.44.3.0556
Lopez C. B., Tilney C. L., Mulbach E., Bouchard J. N., Villac M. C., Henschen K. L., et al. (2021). High-resolution spatiotemporal dynamics of harmful algae in the Indian River Lagoon (Florida)—A case study of Aureoumbra lagunensis, Pyrodinium bahamense, and Pseudo-nitzschia. Front. Mar. Sci. 8. doi: 10.3389/fmars.2021.769877
Loureiro S., Jauzein C., Garcés E., Collos Y., Camp J., Vaqué D. (2009). The significance of organic nutrients in the nutrition of Pseudo-nitzschia delicatissima (Bacillariophyceae). J. Plankton. Res. 31 (4), 399–410. doi: 10.1093/plankt/fbn122
Maguer J., L’Helguen S., Madec C., Labry C., Le Corre P. (2007). Nitrogen uptake and assimilation kinetics in Alexandrium minutum (Dynophyceae): effects of N-limited growth rate on nitrate and ammonium interactions. J. Phycol. 43, 295–303. doi: 10.1111/j.1529-8817.2007.00334.x
Martiny A. C., Vrugt J., Lomas M. (2014). Concentrations and ratios of particulate organic carbon, nitrogen, and phosphorus in the global ocean. Sci. Data 1, 140048. doi: 10.1038/sdata.2014.48
Marx M. C., Wood M., Jarvis S. C. (2001). A microplate fluorimetric assay for the study of enzyme diversity in soils. Soil Biol. Biochem. 33, 1633–1640. doi: 10.1016/S0038-0717(01)00079-7
McCarthy J. J., Taylor W. R., Taft J. L. (1977). Nitrogenous nutrition of the plankton in the Chesapeake Bay, 1. Nutrient availability and phytoplankton preferences. Limnol. Oceanogr. 22, 996–1011. doi: 10.4319/lo.1977.22.6.0996
McGlathery K. J., Sundbäck K., Anderson I. C. (2007). Eutrophication in shallow coastal bays and lagoons: the role of plants in the coastal filter. Mar. Ecol. Prog. Ser. 348, 1–18. doi: 10.3354/meps07132
Morris L. J., Hall L. M., Jacoby C. A., Chamberlain R. H., Hanisak M. D., Miller J. D., et al. (2022). Seagrass in a changing estuary, the Indian River Lagoon, Florida, United States. Front. Mar. Sci. 8, 789818. doi: 10.3389/fmars.2021.789818
Moschonas G., Gowen R., Paterson R., Mitchell E., Stewart B., McNeill S., et al. (2017). Nitrogen dynamics and phytoplankton community structure: the role of organic nutrients. Biogeochemistry 134, 125–145. doi: 10.1007/s10533-017-0351-8
Mulholland M. R., Lee C. (2009). Peptide hydrolysis and the uptake of dipeptides by phytoplankton. Limnol. Oceanogr. 54 (3), 856–868. doi: 10.4319/lo.2009.54.3.0856
Muñoz-Marín M. C., Gómez-Baena G., López-Lozano A., Díez J., García-Fernández J. M. (2020). Mixotrophy in marine picocyanobacteria: use of organic compounds by Prochlorococcus and Synechococcus. ISME J. 14, 1065–1073. doi: 10.1038/s41396-020-0603-9
Murphy J., Riley J. P. (1962). A modified single solution method for the determination of phosphate in natural waters. Anal. Chim. Acta. 27, 31–36. doi: 10.1016/S0003-2670(00)88444-5
Nicholson D., Dyhrman S., Chavex F., Paytan A. (2006). Alkaline phosphatase activity in the phytoplankton communities of Monterey Bay and San Francisco Bay. Limnol. Oceangr. 51 (2), 874–883. doi: 10.4319/lo.2006.51.2.0874
Paerl H. W., Scott J. T., McCarthy M. J., Newell S. E., Gardner W. S., Havens K. E., et al. (2016). It takes two to tango: When and where dual nutrient (N & P) reductions are needed to protect lakes and downstream ecosystems. Environ. Sci. Technol. 50, 10805–10813. doi: 10.1021/acs.est.6b02575
Phlips E. J., Badylak S., Grosskopf T. (2002). Factors affecting the abundance of phytoplankton in a restricted subtropical lagoon, the Indian River Lagoon, Florida, USA. Estuarine. Coast. Shelf Sci. 55, 385–402. doi: 10.1006/ecss.2001.0912
Phlips E. J., Badylak S., Lasi M. A., Chamberlain R., Green W. C., Hall L. M., et al. (2015). From red tides to green and brown tides: Bloom dynamics in a restricted subtropical lagoon under shifting climatic conditions. Estuar. Coast. 38, 886–904. doi: 10.1007/s12237-014-9874-6
Phlips E. J., Badylak S., Lynch T. L. (1999). Blooms of the picoplanktonic cyanobacterium Synechococcus in Florida Bay. Limnol. Oceanogr. 44, 1166–1175. doi: 10.4319/lo.1999.44.4.1166
Phlips E. J., Badylak S., Nelson N. G., Hall L. M., Jacoby C. A., Lasi M. A., et al. (2021). Cyclical patterns and a regime shift in the character of phytoplankton blooms in a restricted sub-tropical lagoon, Indian River Lagoon, Florida, United States. Front. Mar. Sci. 8, 730934. doi: 10.3389/fmars.2021.730934
Phlips E. J., Badylak S., Nelson N., Havens K. (2020). Hurricanes, El Niño and harmful algal blooms in two sub-tropical Florida estuaries: Direct and indirect impacts. Sci. Rep. 10, 1910. doi: 10.1038/s41598-020-58771-4
Phlips E. J., Love N., Badylak S., Hansen P., John C. V., Gleeson R. (2004). A comparison of water quality and hydrodynamic characteristics of the Guana Tolomato Matanzas National Estuarine Research Reserve and the Indian River Lagoon in Florida. J. Coast. Res. Special Issue 45, 93–109. doi: 10.2112/SI45-093.1
Phlips E. J., Zeman C., Hansen P. (1989). Growth, photosynthesis, nitrogen fixation and carbohydrate production by a unicellular cyanobacterium, Synechococcus sp. (Cyanophyta). J. Appl. Phycol. 1, 137–145. doi: 10.1007/BF00003876
Quigg A., Irwin A., Finkel Z. (2011). The evolutionary inheritance of elemental stoichiometry in phytoplankton. Proc Sci. 278, 526–534. doi: 10.1098/rspb.2010.1356
Raven J. A. (1998). The twelfth Tansley Lecture. Small is beautiful: the picophytoplankton. Funct. Ecol. 12, 503–513. doi: 10.1046/j.1365-2435.1998.00233.x
Reinl K., Harris T., Elfferich I., Coker A., Zhan Q., Domis L., et al. (2022). The role of organic nutrients in structuring freshwater phytoplankton communities in a rapidly changing world. Water Res. 219, 118573. doi: 10.1016/j.watres.2022.118573
Rothenberger M. B., Calomeni A. J. (2016). Complex interactions between nutrient enrichment and zooplankton in regulating estuarine phytoplankton assemblages: Microcosm experiments informed by an environmental dataset. J. Exp. Mar. Biol. Ecol. 48, 62–73. doi: 10.1016/j.jembe.2016.03.015
Sanz-Luque E., Bhaya D., Grossman A. R. (2020). Polyphosphate: a multifunctional metabolite in cyanobacteria and algae. Front. Plan Sci. 11, 938. doi: 10.3389/fpls.2020.00938
Seeyave S., Probyn T. A., Pitcher G. C., Lucas M. I., Purdie D. A. (2009). Nitrogen nutrition in assemblages dominated by Pseudo-nitzschia spp., Alexandrium catenella and Dinophysis acuminata off the west coast of South Africa. Mar. Ecol. Prog. Ser. 379, 91–107. doi: 10.3354/meps07898
Sharma K., Inglett P. W., Reddy K. R., Ogram A. V. (2005). Microscopic examination of photoautotrophic and phosphatase-producing organisms in phosphorus-limited Everglades periphyton mats. Limnol. Oceanogr 50 (6), 2057–2062. doi: 10.4319/lo.2005.50.6.2057
Sigua G. C., Steward J. S., Tweedale W. A. (2000). Water-quality monitoring and biological integrity assessment in the Indian River Lagoon, Florida: Status, trends, and loadings, (1988-1994). Environ. Manage. 25 (2), 199–209. doi: 10.1007/s002679910016
Sigua G. C., Tweedale W. A. (2003). Watershed scale assessment of nitrogen and phosphorus loadings in the Indian River Lagoon basin, Florida. J. Environ. Manage 67, 363–372. doi: 10.1016/S0301-4797(02)00220-7
Smayda T. J., Trainer V. L. (2010). Dinoflagellate blooms in upwelling systems: Seeding, variability, and contrasts with diatom bloom behavior. Prog. Oceanogr. 85, 92–107. doi: 10.1016/j.pocean.2010.02.006
Smith R. E. H., Kalff J. (1982). Size-dependent phosphorus uptake kinetics and cell quota in phytoplankton. J. Phycol. 18 (2), 275–284. doi: 10.1111/j.1529-8817.1982.tb03184.x
Smith S. L., Merico A., Wirtz K. W., Pahlow M. (2014). Leaving misleading legacies behind in plankton ecosystem modelling. J. Plankton Res. 36, 612–620. doi: 10.1093/plankt/fbu011
Smith L. S., Yamanaka Y., Pahlow M., OsChiles A. (2009). Optimal uptake kinetics: Physiological acclimation explains the observed pattern of nitrate uptake by phytoplankton in the ocean. Mar. Ecol. Prog. Ser. 384, 1–12. doi: 10.3354/meps08022
Song B., Ward B. B. (2007). Molecular cloning and characterization of high-affinity nitrate transporters in marine phytoplankton. J. Phycol. 43, 542–552. doi: 10.1111/j.1529-8817.2007.00352.x
Suzumura S. (2008). Persulfate chemical wet oxidation method for the determination of particulate phosphorus in comparison with a high-temperature dry combustion method. Limnol. Oceanogr. Methods 6 (11), 619–629. doi: 10.4319/lom.2008.6.619
Taylor G. T., Gobler C. J., Sañudo-Wilhelmy S. A. (2006). Speciation and concentrations of dissolved nitrogen as determinants of brown tide Aureococcus anophagefferens bloom initiation. Mar. Ecol. Prog. Ser. 312, 67–83. doi: 10.3354/meps312067
Townsend D. W., Cammen L. M., Holligan P. M., Campbell D. E., Pettigrew N. R. (1994). Causes and consequences of variability in the timing of spring phytoplankton blooms. Deep Sea Res. Part 1 Oceanogr. Res. Pap. 41 (5-6), 747–765. doi: 10.1016/0967-0637(94)90075-2
Twining B. S., Nuñez-Milland D., Vogt S., Johnson R., Sedwick P. (2010). Variations in Synechococcus cell quotas of phosphorus, sulfur, manganese, iron, nickel, and zinc within mesoscale eddies in the Sargasso Sea. Limnol. Oceanogr 55, 492–506. doi: 10.4319/lo.2009.55.2.0492
Vahtera E., Conley D. J., Gustafsson B. G., Kuosa H., Pitkänen H., Savchuk O. P., et al. (2007). Internal ecosystem feedback enhance nitrogen-fixing cyanobacteria blooms and complicate management in the Baltic Sea. Ambio 36 (2-3), 186–194. doi: 10.1579/0044-7447(2007)36[186:IEFENC]2.0.CO;2
Van Mooy B. A. S., Fredricks H. F., Pedler B. E., Dyhrman S. T., Karl D. M., Koblizek M., et al. (2009). Phytoplankton in the ocean use non-phosphorus lipids in response to phosphorus scarcity. Nature 458, 69–72. doi: 10.1038/nature07659
Varona-Cordero F., Gutierrez-Mendieta F., Rivera-Monroy V. H. (2014). In situ response of phytoplankton to nutrient additions in a tropical coastal lagoon, (La Mancha, Veracruz, Mexico). Estuar. Coast. 37 (6), 1353–1375. doi: 10.1007/s12237-014-9806-5
Wafar M., L’Helgeun S., Raikar V., Maguer J., Corre P. (2004). Nitrogen uptake by sizepfractionated plankton in permanently well-mixed temperate coastal waters. J. Plank. Res. 26, 1207–1218. doi: 10.1006/ecss.1996.0051
Weber T. S., Deutsch C. (2010). Ocean nutrient ratios governed by plankton biogeography. Nature 467, 550–554. doi: 10.1038/nature09403
Yamamoto T., Oh S. J., Kataoka Y. (2004). Growth and uptake kinetics for nitrate, ammonium and phosphate by the toxic dinoflagellate Gymnodinium catenatum isolated from Hiroshima Bay, Japan. Fisheries Sci. 70, 108–115. doi: 10.1111/j.1444-2906.2003.00778.x
Yelton A. P., Acinas S. G., Sunagawa S., Bork P., Pedrós-Alió C., Chisholm S. W. (2016). Global genetic capacity for mixotrophy in marine picocyanobacteria. ISME J. 10, 2946–2957. doi: 10.1038/ismej.2016.64
Keywords: nitrogen uptake, phosphorus uptake, kinetics, harmful algal blooms, picocyanobacteria, nanoeukaryote, estuary
Citation: Papacek JR, Inglett PW, Phlips EJ and Lasi MA (2024) Nitrogen and phosphorus uptake kinetics in cultures of two novel picoplankton groups responsible for a recent bloom event in a subtropical estuary (Indian River Lagoon, Florida). Front. Mar. Sci. 11:1256901. doi: 10.3389/fmars.2024.1256901
Received: 11 July 2023; Accepted: 08 January 2024;
Published: 25 January 2024.
Edited by:
Rhena Schumann, University of Rostock, GermanyReviewed by:
Frederico Pereira Brandini, University of São Paulo, BrazilHendrik Schubert, University of Rostock, Germany
Copyright © 2024 Papacek, Inglett, Phlips and Lasi. This is an open-access article distributed under the terms of the Creative Commons Attribution License (CC BY). The use, distribution or reproduction in other forums is permitted, provided the original author(s) and the copyright owner(s) are credited and that the original publication in this journal is cited, in accordance with accepted academic practice. No use, distribution or reproduction is permitted which does not comply with these terms.
*Correspondence: Patrick W. Inglett, cGluZ2xldHRAdWZsLmVkdQ==