- 1Ocean Systems (OCS), Royal Netherlands Institute for Sea Research (NIOZ), Texel, Netherlands
- 2Acoustics & Underwater Warfare Division, Netherlands Organization for Applied Scientific Research (TNO), The Hague, Netherlands
- 3Physics of Fluid Group, University of Twente, Enschede, Netherlands
- 4Research & Development, Maritime Research Institute Netherlands (MARIN), Wageningen, Netherlands
- 5Faculty of Applied Sciences, Delft University of Technology, Delft, Netherlands
- 6Department of Earth Sciences – Faculty of Geosciences, Utrecht University, Utrecht, Netherlands
Since the industrial revolution the ocean has become noisier. The global increase in shipping is one of the main contributors to this. In some regions, shipping contributed to an increase in ambient noise of several decibels, especially at low frequencies (10 to 100 Hz). Such an increase can have a substantial negative impact on fish, invertebrates, marine mammals and birds interfering with key life functions (e.g. foraging, mating, resting, etc.). Consequently, engineers are investigating ways to reduce the noise emitted by vessels when designing new ships. At the same time, since the industrial revolution (starting around 1760) greenhouse gas emissions have increased the atmospheric carbon dioxide fraction x(CO2) by more than 100 μmol mol-1. The ocean uptake of approximately one third of the emitted CO2 decreased the average global surface ocean pH from 8.21 to 8.10. This decrease is modifying sound propagation, especially sound absorption at the frequencies affected by shipping noise lower than 10 kHz, making the future ocean potentially noisier. There are also other climate change effects that may influence sound propagation. Sea surface warming might alter the depth of the deep sound speed channel, ice melting could locally decrease salinity and more frequent storms and higher wind speed alter the depth of the thermocline. In particular, modification of the sound speed profile can lead to the appearance of new ducts making specific depths noisier. In addition, ice melting and the increase in seawater temperature will open new shipping routes at the poles increasing anthropogenic noise in these regions. This review aims to discuss parameters that might change in the coming decades, focusing on the contribution of shipping, climate change and economic and technical developments to the future underwater soundscape in the ocean. Examples are given, contrasting the open ocean and the shallow seas. Apart from the changes in sound propagation, this review will also discuss the effects of water quality on ship-radiated noise with a focus on propeller cavitation noise.
1 Introduction
The ocean is naturally noisy because of wind, rain, breaking waves, cracking polar ice, subsea earthquakes, volcanoes and marine life. The natural soundscape is altered by anthropogenic activities such as shipping, transport, oil and gas, defense, tourism, fishing, offshore wind and water energy and on- and near-shore construction (Richardson et al., 2013; Duarte et al., 2021). Among these the main anthropogenic noise source in the oceans is shipping. This noise source dominates the soundscape in the low-frequency range (10 Hz to 1 kHz, Wenz, 1962). In this, low-frequency (<1 kHz) range, noise experiences less attenuation and can potentially propagate over large distances. The noise generated in the mid- to high-frequency range (>1 kHz) does not propagate as far because it is more strongly attenuated. Therefore, low frequency-shipping noise is the main source of ambient underwater noise (Hildebrand, 2009). Under 0.3 kHz this effect increased in the past 50-60 years because regions exposed to intense ship traffic have experienced an increase in ambient noise. In these regions ambient noise increased by 3 dB decade-1 until 2000 (Andrew et al., 2002; Andrew et al., 2011; Chapman and Price, 2011; Miksis-Olds et al., 2013; Miksis-Olds and Nichols, 2016; Erbe et al., 2019), resulting in an absolute sound increase of 15 to 20 dB (Andrew et al., 2002; McDonald et al., 2006; McKenna et al., 2012). A likely explanation of this increase is the rise in the number of ships, which is estimated to have doubled in the period between 1965 to 2000 (from approximately 44000 to 88000, Hildebrand, 2009). This rise in vessel number is also visible by satellite, with Tournadre (2014) observing an increase by a factor of 4 between 1992 to 2012, with an increase of 6% yr-1 until 2002 and later 10% yr-1. However, the final effect on the ambient noise is more complicated than just the number of vessels. Ambient noise also depends on the vessel class, speed, size and load (Erbe et al., 2019; MacGillivray and de Jong, 2021). This increase appears to have stopped since the beginning of the 21st century (Frisk, 2012), but estimates for future developments suggest that with the current rate of growth in ship traffic and economic trading, ambient noise might rise again, especially in the Arctic and Africa (United Nations, 2021).
The rise in ambient noise is a growing concern due to the adverse effects on marine life, in particular on marine mammals (Southall et al., 2008, 2019; Richardson et al., 2013), invertebrates (Murchy et al., 2019), fishes (Slabbekoorn et al., 2010; Popper et al., 2014; Cox et al., 2018) and birds (Anderson Hansen et al., 2020). Impacts are pervasive and affect individual animals as well as populations (Pirotta et al., 2018; Soudijn et al., 2020), at all taxonomic and trophic levels. The realization that marine life needs protection has increased the effort to monitor the ocean’s soundscape. In 2010, the European Commission produced new detailed criteria and indicators to help member states to implement the Marine Strategy Framework Directive (MFSD). In this framework two criteria where created to monitor and reduce the noise energy: 1) the proportion and distribution of days in which anthropogenic noise exceeds levels that might impact marine animals and 2) trends in ambient noise in specific low-frequency bands (63 and 125 Hz, Van der Graaf et al., 2012). After the introduction of the MSFD, many programs such as the Baltic Sea Information on the Acoustic Soundscape (BIAS), the Joint Framework for Ocean Noise in the Atlantic Seas (JOMOPANS), QuietMed and QuietMed2 in the Mediterranean Sea and Quiet Seas in the Mediterranean and Black Seas started to monitor underwater noise (Thomsen et al., 2021). However, quantifying a trend in ambient noise is challenging due to the lack of baseline information and the necessity to monitor over long periods (Merchant et al., 2016). These monitoring programs ultimately aim to contribute to the reduction of shipping noise. However, it is difficult to identify a single action that is equally effective in the reduction of emitted noise for all the vessels because in the same ship’s class, the source level may vary by 20-40 dB due to variability in design, size, maintenance and operational parameters (e.g. speed) (Simard et al., 2016; Joy et al., 2019). In general, actions that can reduce shipping noise include a myriad of options, varying in effects and costs. The easiest action to decrease shipping noise is reducing the vessel’s speed (Leaper et al., 2014; Joy et al., 2019; MacGillivray et al., 2019). Other projects are trying to design quieter vessels focusing on the reduction of cavitation noise by optimizing propeller load, ensuring a water flow into propellers as uniform as possible and a careful selection of the propeller characteristics such as diameter, blade number, pitch, skew and sections (Spence and Fischer, 2016). These measures can also be applied to existing ships by retrofitting a quieter propeller (Spence and Fischer, 2016).
Climate change is also altering the ocean’s soundscape. Since the industrial revolution, burning fossil fuels is increasing the atmosphere’s carbon dioxide concentration. Approximately one third of the added atmospheric CO2 is absorbed by the ocean, decreasing oceanic surface pH by more than 0.1 (Doney et al., 2009). One consequence of this ocean acidification is a reduction of sound absorption (α), which in the next 300 years could decrease up to 60% (Ilyina et al., 2010). This effect is strongest at frequencies <10 kHz which are the same frequencies associated with shipping activities. Despite the large decrease in sound absorption, studies have suggested that the absolute change might be relatively small (Joseph and Chiu, 2010; Rouseff and Tang, 2010; Udovydchenkov et al., 2010), because absorption is generally not the dominant mechanism limiting propagation at these frequencies. The ocean soundscape is also affected by other climate change processes including: ocean warming, wind speed increase, enhanced storm intensity and frequency, increased sea-ice melting, decreases in salinity and consequently, changes in the sound speed profile (Andrew et al., 2002; Munk, 2011; Young et al., 2011; Ainslie et al., 2021; Duarte et al., 2021; Possenti et al., 2023). Currently, the consequences of these changes are not well understood and exact impacts cannot be accurately quantified. This is partly due to a lack of attention for the effect of climate change on the ocean’s soundscape compared to other climate impacts. In fact, the last assessment by the IPCC on climate change impacts (Pachauri et al., 2014) did not acknowledge that climate change is influencing the ocean’s soundscape. Instead, the recent IPCC report on oceans and the cryosphere acknowledged noise only in the context of increased human operations in the Arctic Ocean (Poloczanska et al., 2018).
Based on recent literature, this paper aims to present and quantify the expected changes in the ocean soundscape. We focus on the main sources of these changes, including technological developments and noise generation as a function of economic development and the direct effect of climate change on sound propagation. The first section of the manuscript explains the different components of shipping noise and its diffusion in shallow and deep seas. Subsequently, we discuss technological improvements aimed at reducing shipping noise. In the shipping noise components section, we also explain propeller cavitation and the consequence of the presence of bubbles on near-field sound propagation. Later, we discuss the effects of changes in the bubble spectra on propeller cavitation, ambient noise and sound attenuation. We also discuss the most recent studies that focused on the impacts of climate change (ocean acidification, warming and wind) on sound propagation and generation. We explain these processes, their impacts and the knowledge gaps that need to be filled to accurately quantify the expected changes. In the last section, we integrate the analyses of the various sources and propagation effects and discuss which will likely contribute most to changes in the future soundscape.
2 Current and future ship sound propagation
2.1 Shipping noise
The underwater radiated noise generated by a ship has different sources such as machinery noise, propeller noise and flow noise (Ross, 1979; Arveson and Vendittis, 2000; Fischer and Collier, 2007; Bosschers et al., 2017). Machinery noise is related to all main and auxiliary machinery equipment installed on the ship including gearboxes and diesel generators. The airborne noise and structural vibrations are generated by the ship’s equipment and then transferred to the outer ship’s hull where they are radiated underwater. The force to move a ship is generated by a propulsor, for which we assume here that it consists of a propeller, and depending on the type of propeller the noise signals generated by individual vessels differ. The noise generated by the propeller may also excite the ship’s hull and the resulting vibrations may contribute to the underwater noise. The same holds for the hull vibrations due to the propeller force and moment variations that are transferred to the ship by the propeller shaft. Another noise source is flow noise that is caused by the water flow over the hull, including hull openings. The generated noise includes the hull vibrations by this flow and the entrainment of air bubbles by the surface waves generated by the ship.
Propeller and flow noise are strongly related to the vessel’s speed while machinery noise is only weakly dependent on the speed (or engine power). Also, the ships dominating noise sources depend on the type and installation method of machinery equipment and propeller and hull design. In the same vessel class, with similar machinery equipment, the largest variability of emitted noise is usually due to differences in hull and propeller design and installation of machinery equipment. Arveson and Vendittis (2000) have presented a typical example of the emitted noise by a merchant vessel at different speeds (from 8 to 16 knots) which is shown in Figure 1. Their narrowband data of the radiated noise shows high-level tonal frequencies from the ship’s diesel generator, main engine firing rate, and blade harmonics due to propeller cavitation. At 16 knots the blade rate at 9 Hz reached the peak of 174 dB re 1 μPa m. Still, recent studies have shown that the noise by vessels when anchored can also affect marine life (Ivanova et al., 2020; Murchy et al., 2022) as the ambient sound pressure level between 20 to 24000 Hz can increase by 2 to 8 dB.
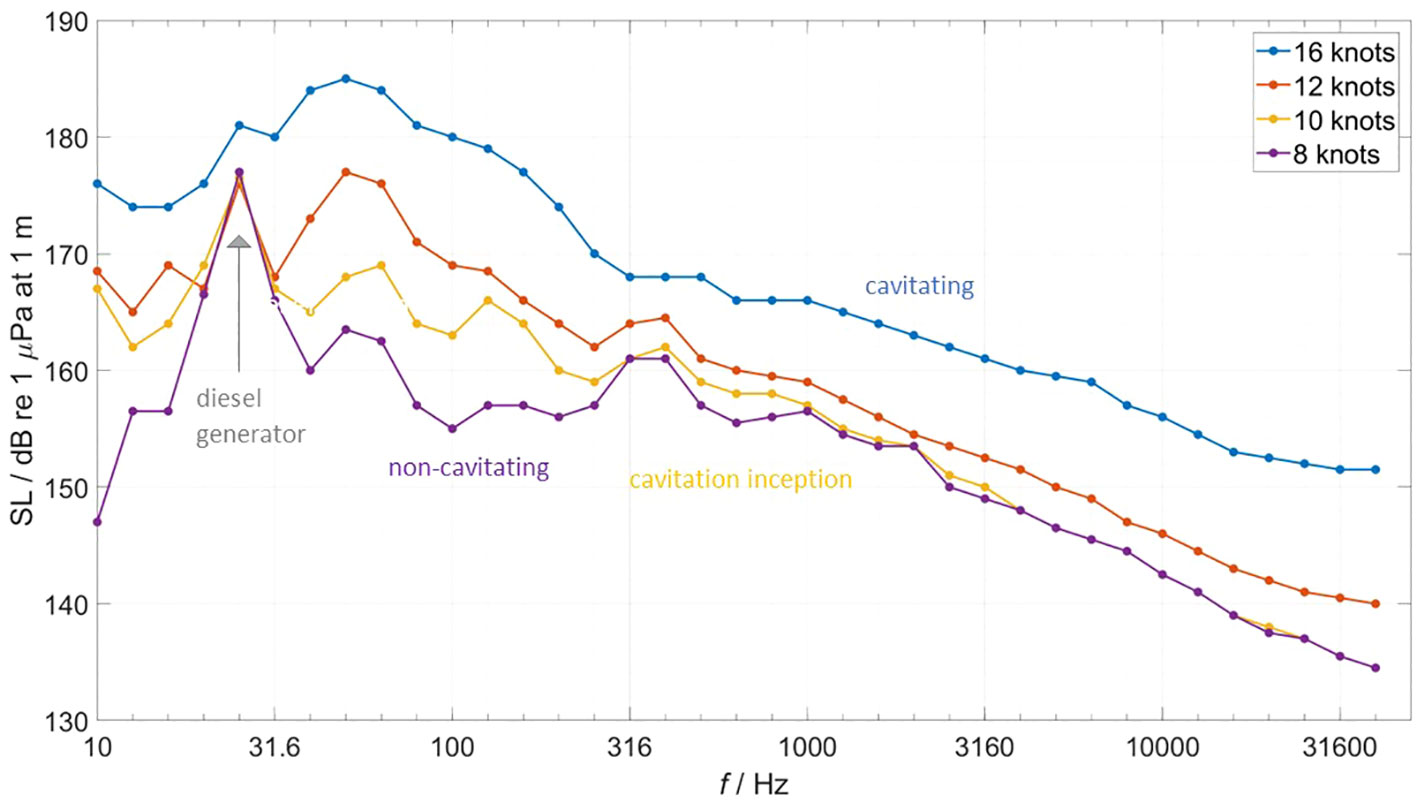
Figure 1 Example of the noise spectrum of a merchant vessel sailing at different speeds, data taken from Arveson and Vendittis (2000), dominated by machinery noise at 8 knots and by propeller cavitation noise at 16 knots.
2.1.1 Propeller noise
For propeller noise one can make a distinction between the noise related to the non-cavitating flow over the propeller and the noise by cavitation (Blake, 2017). If merchant vessels are not cavitating, the noise that can be identified are from the blade passage frequencies and, if present, due to flow-excited propeller blade vibrations (‘propeller singing’). These tones may occur for wide range of frequencies depending on propeller size and shaft rotation rate: Fischer (2008) shows examples in which the frequency of singing varied between 180 and 1800 Hz.
Carlton (2018) describes cavitation showing that it is an important source of shipping noise. In the presence of nuclei such as small gas bubbles, cavitation occurs when the cavity’s volume is immediately transferred downstream with the flow after formation (Figure 2). This typically occurs when the minimum pressure on the blade occurs relatively far from the leading edge of the blade. As bubble cavitation can be very erosive, most propellers are designed such that bubble cavitation is not present. When at the leading edge of the blade the pressure drops below the vapor pressure, a small region of flow separation occurs upstream of the cavity allowing the cavity to grow into a sheet cavity rather than individual bubbles. This sheet cavity can break-up into a cloud of bubbles and vortex cavities or it can merge with the tip-vortex cavitation. The minimum pressure may also occur in the flow due to the centrifugal force exerted on the flow within a vortex (Bosschers, 2018). Cavitation then starts in the center of the vortex and it is then referred to as vortex cavitation. The loading on a propeller blade varies when the blade rotates through the ship’s wake and this typically leads to the inception, growth and collapse of a cavity on the blade within each revolution. Even though any change in cavity volume leads to noise emission, it is especially the volume acceleration during the collapse phase that contributes to the underwater radiated noise (Ross, 1979; Franc and Michel, 2006). The cavity collapse is a very efficient noise source as it is a monopole in contrary to other (non-cavitating) flow noise sources which are either dipoles or quadrupoles.
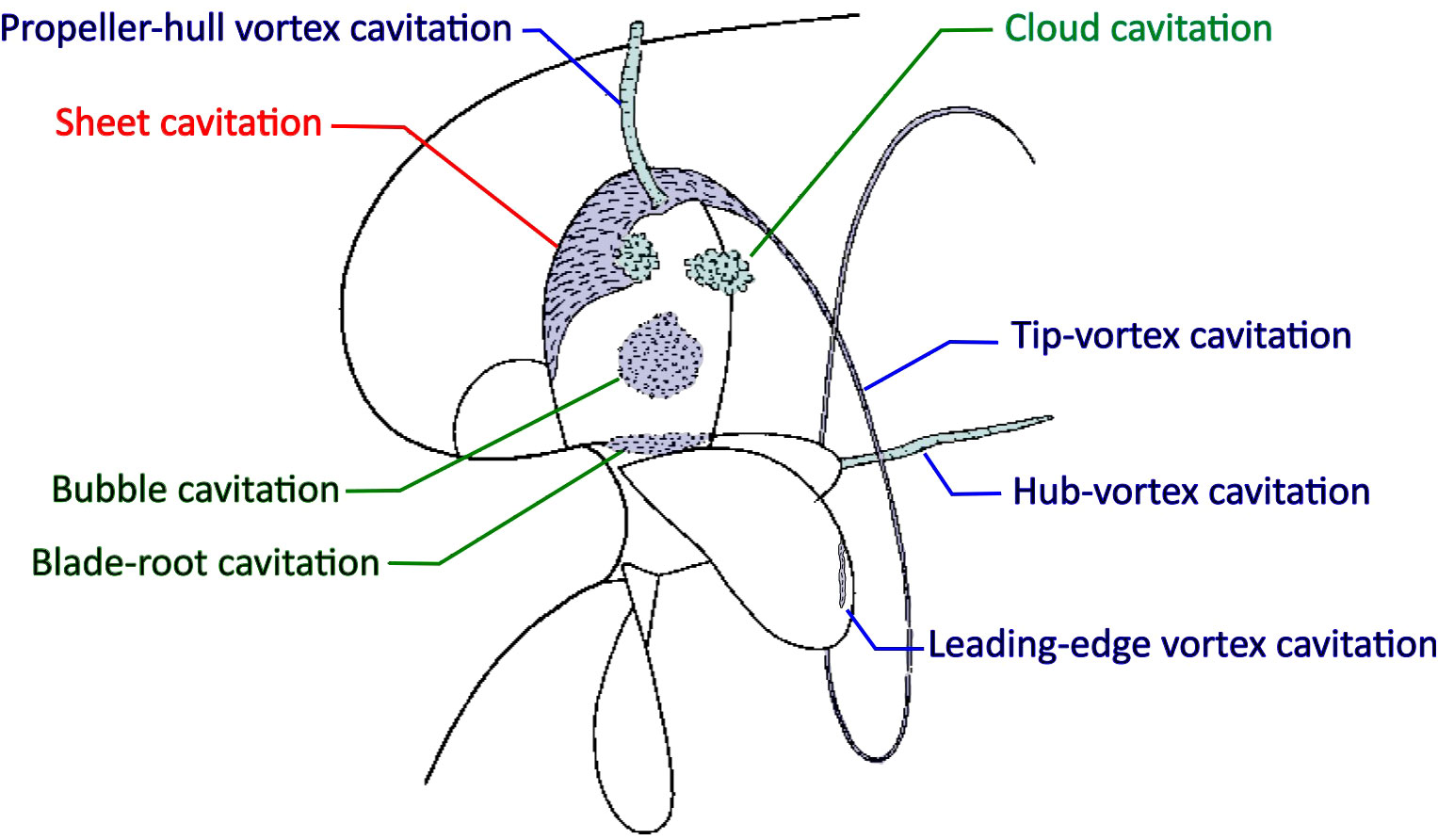
Figure 2 Various cavitation patterns that may occur on marine propellers with in red sheet cavitation, in green bubble cavitation and in blue vortex cavitation. The figure has been adapted fro ITTC procedure 7.5-02-03-03.2.
Water quality can affect nuclei and therefore the onset of cavitation and the resulting cavitation noise (Brandner et al., 2022). Nuclei typically consist of very small free gas bubbles generated in the ocean by breaking waves and their sizes and numbers decrease with depth (Atlar et al., 2002). The effect of nuclei variations in the ocean on cavitation inception is considered small (Gowing and Shen, 2001) but there is very limited data available on this topic. Beyond inception, the growth and collapse of cavitation is also affected by bubbles identified as non-condensable gas. By diffusion and coalescence, the cavity volume and the growth rate increase with air content (Brennen, 1969; Briançon-Marjollet and Merle, 1996; Nanda et al., 2022). This happens especially for cavitation in separated flow or in vortices, which can increase the radiated sound due to larger cavity volume. However, in the collapse phase, the compressibility of the non-condensable gas dampens the collapse of the cavity thereby reducing the radiated sound (Moss et al., 2000; Trummler et al., 2021). The collapse and rebounds of the large cavity structure are considered responsible for sound at low frequencies ranging from blade passage frequency up to a few hundred Hertz, with the upper frequency depending on the amount of cavitation (Bosschers, 2018). At higher frequencies, it is the cloud of resonating bubbles generated by the collapse of the larger cavity structure that is considered responsible for the radiated sound. The presence of bubbles will also attenuate sound. In the top 10 m of the water column, sound is attenuated by bubbles generated by breaking waves that are connected to wind speed (Thorpe and Humphries, 1980).
Other variables such as water temperature, salinity and CO2 may affect the amount and size of cavitation bubbles. Water temperature may affect cavitation because an increase in water temperature leads to an increase in vapor pressure. At 15°C, a 1°C temperature rise increases vapor pressure by 7%, but the resulting change in inception speed and emitted noise is negligible (<0.1%, ITTC, 2011). Temperature also affects the bubble clouds generated by breaking waves via air entrainment processes. In particular, a minimum critical temperature is needed for air entrainment (10°C for a water jet) and the bubble penetration depth increases with increasing temperature up to 19°C (Hwang et al., 1991). The effect of salinity on cavitation and the resulting acoustic emission has been analyzed by Ceccio et al. (1997) for an axisymmetric head form (a modified ellipsoidal shape) in a small water tunnel. They found that in saline water event rate and size of bubble cavitation were both reduced compared to those in fresh water, which was explained by a suppression of nuclei distribution in salt water. Bubbles of similar size showed comparable noise levels, independent of water salinity (Ceccio et al., 1997). However, smaller bubbles produced larger noise levels than larger bubbles, and therefore the noise of cavitation on the head form was about 10 dB larger for smaller bubbles. In the future other parameters affecting water quality such as surfactants (e.g. plankton) and the seawater CO2 content, might need to be explored to better understand and estimate impact on cavitation. These parameters will also change due to climate change and are therefore relevant to predict future noise levels. Li et al., (2021) found that in an industrial setup varying the solution pH from 4.4 to 9.5, the cavitation inception number increases in a more acidic solution showing that hydrodynamic cavitation is easier at low pH. The effect of pH needs to be verified with a specific experiment at the relevant surface ocean pH interval (8.00 to 8.25, Jiang et al., 2019).
2.2 Shipping noise propagation in the ocean
Shipping noise is one of the main sources of noise in the ocean between 10 to 1000 Hz (Figures 3, 4). In general, sound propagation in the ocean is affected by several factors and one of these is sound speed (c). In seawater, sound speed is approximately 0.3% faster than in distilled water. The average sound speed is 1528 m s-1 and it varies with temperature (T), salinity (S) and pressure (p, Wong and Zhu, 1995). Of these effects, the temperature has the largest impact; an increase of 1°C results in a rise of sound speed by 3.5 m s-1. Pressure contributes less to the total variability in sound speed since an increase of 105 Pa enhances sound speed by 1.7 m s-1. Lastly, an increase in salinity by 1 unit leads to an increase in sound speed by 2.49 m s-1 (Wong and Zhu, 1995). After its generation, the sound pressure level (SPL) decreases over space due to propagation loss (PL) and the SPL at the receiver is defined by the difference between source level (SL) and PL. Several processes such as scattering, absorption, refraction, reflection and geometrical spreading contribute to PL. Examples of geometrical spreading are spherical and cylindrical spreading (Urick, 1979). Equation 1 shows that spherical spreading assumes that sound propagates away from a source in all directions uniformly:
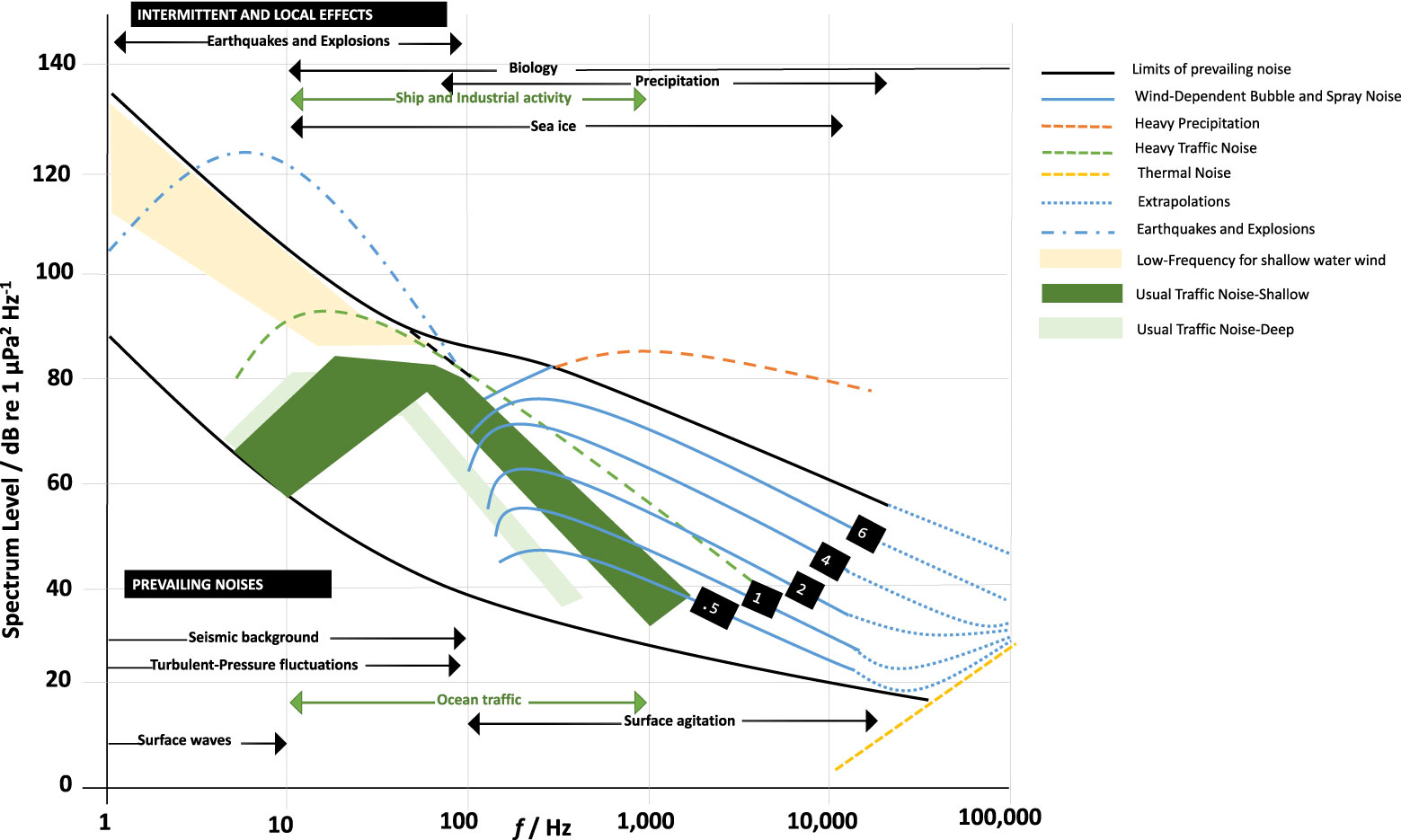
Figure 3 Generalized ocean ambient noise spectral levels for a deep-water site. The x-axis covers five decades of frequency from 1 to 100 kHz. The y-axis shows the noise spectrum level from 0 to 140 dB re µPa2 Hz-1/ Source: Reprinted with permission from Figure 13 of Wenz, G. M. (1962). 'Acoustic ambient noise in the ocean: spectra and sources'. J. Acoustical Society America 34 (12). Copyright 1962, 2005, Acoustic Society of America.
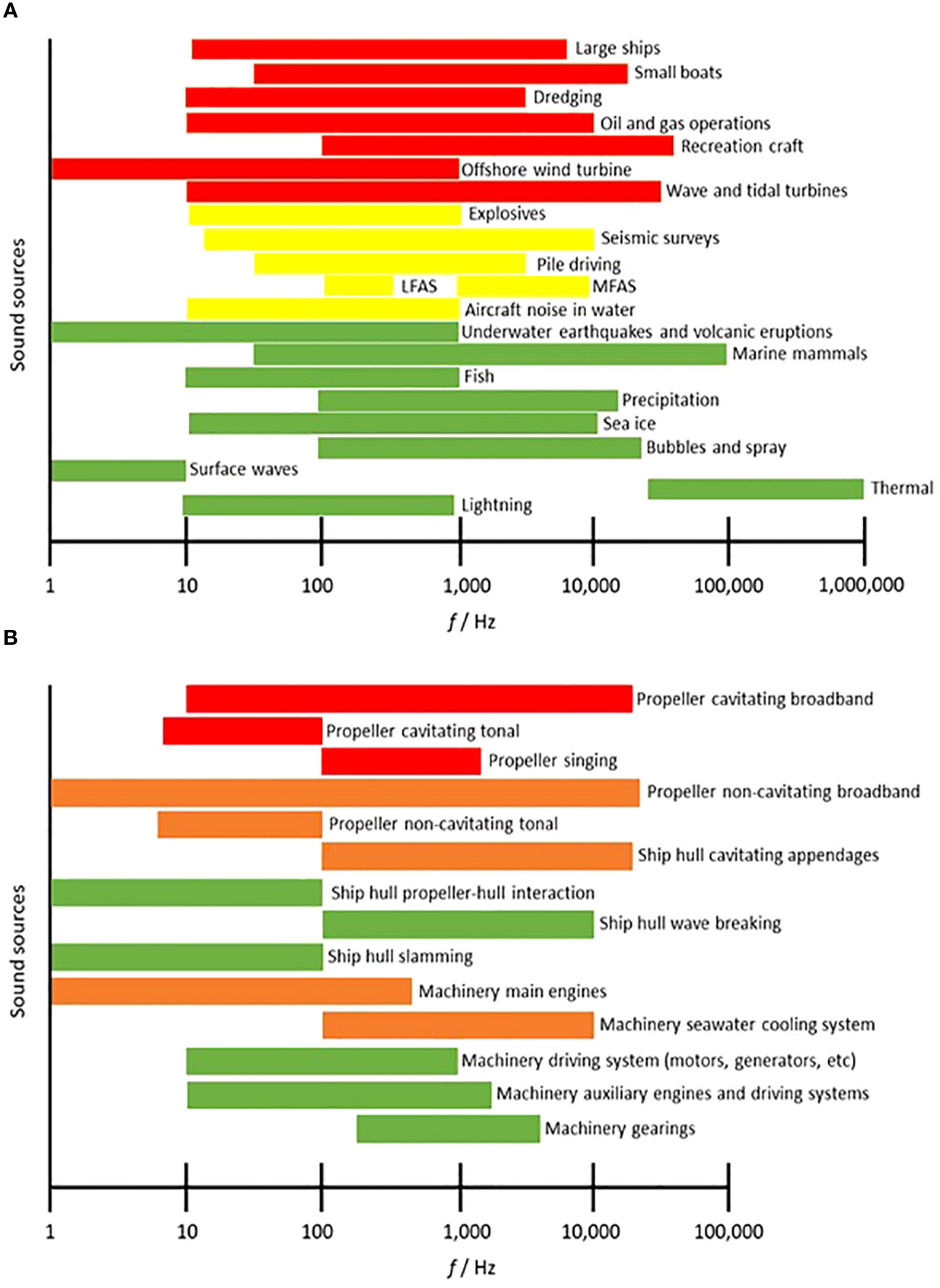
Figure 4 Comparison of different ocean sound sources (A) and shipping noise sources (B) at different frequencies. In (A) we show in red the continuous sound sources, in yellow the impulsive sources and in green the natural sources. In (B) we show the sources of shipping noise colored by importance at typical design speed, with red as most important, orange mid-importance and green least important.
where r is the distance in meters from the source and r0 is the reference distance, typically r0 = 1 m. Equation 2 shows that in shallow water, spherical spreading (at a short distance from a source) generally develops into cylindrical spreading:
where z is the water depth in meters and ψ the seabed critical angle. The transformation into cylindrical spreading occurs after a horizontal distance of one or two water depths when r > z/2ψ (for ψ between 0.25 and 0.5). Another process affecting sound propagation is refraction, which is governed by Snell’s law. Refraction happens when sound interacts at the boundary of two layers with different sound speeds (e.g. two different water masses, seawater-seafloor, seawater-atmosphere, sound speed gradient over depth, etc.) and it can be calculated using Equation 3:
where θ is the local angle of the ray with the vertical/horizontal. One important consequence of this law is that assuming a constant sound speed profile results in a ray having the same θ at all water depths. Another consequence is that sound waves are always refracted toward the region with the lowest sound speed. Low-frequency sound experiences less absorption (Francois and Garrison, 1982b, Figures 5, 6), from 30 Hz to 300 kHz sound absorption increases by 4 orders of magnitude. The same principles apply to the propagation of wind noise. Wind generated sound dominates the 2-10 kHz frequency band (Vagle et al., 1990; Anagnostou et al., 2008) and the strong correlation between wind driven SPL and wind speeds allow the calculation of wind speed from SPL (Vagle et al., 1990).
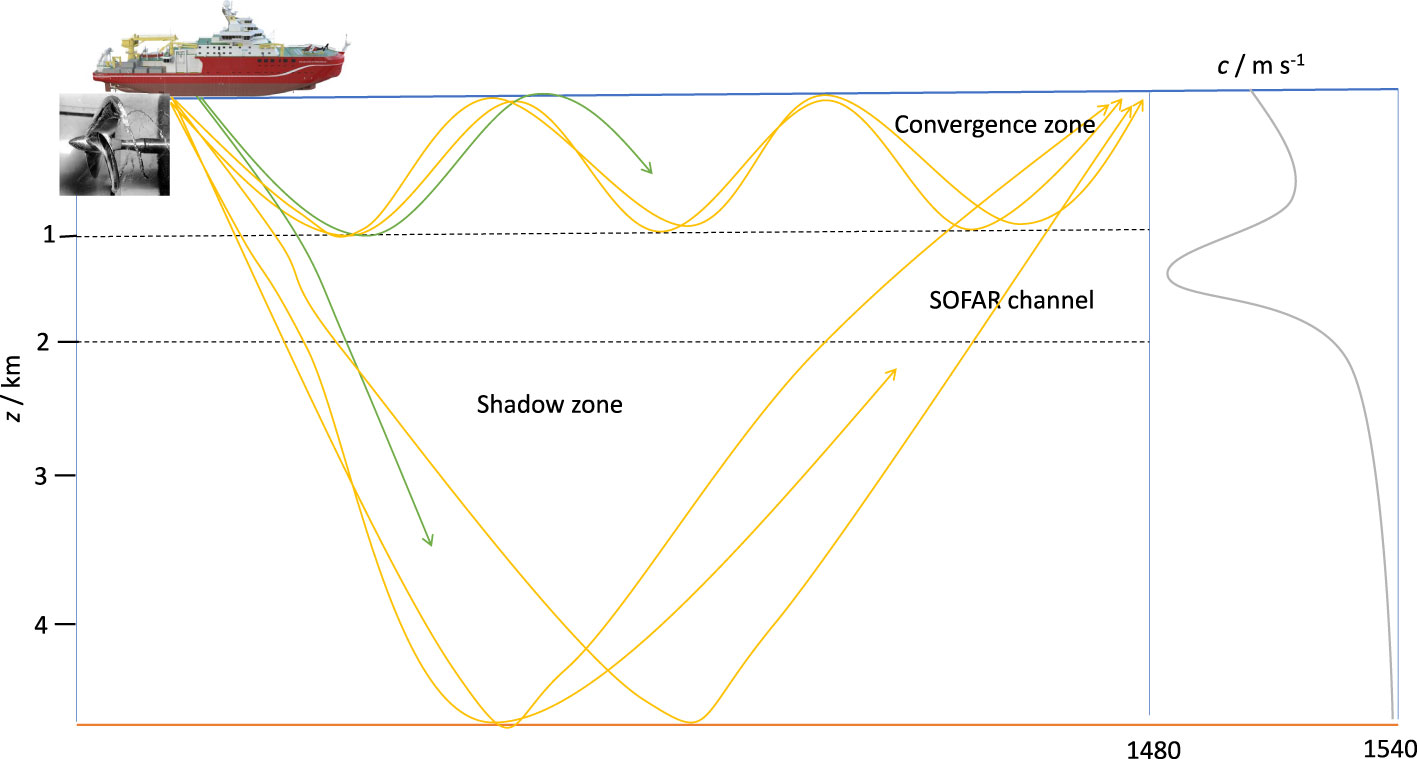
Figure 5 diagram showing the propagation of shipping noise in the open ocean on an indefinite distant and a bathymetry >4 km. On the right side in grey the sound speed profile in m s-1 with a SOFAR channel (see section 4.1.2) between 1 and 2 km of water depth. The yellow arrows show low frequency noise (<1 kHz) mainly generated by propeller cavitation and in the green higher frequency noise (>10 kHz). The low frequency sound can reach larger distances due to the lower sound absorption.
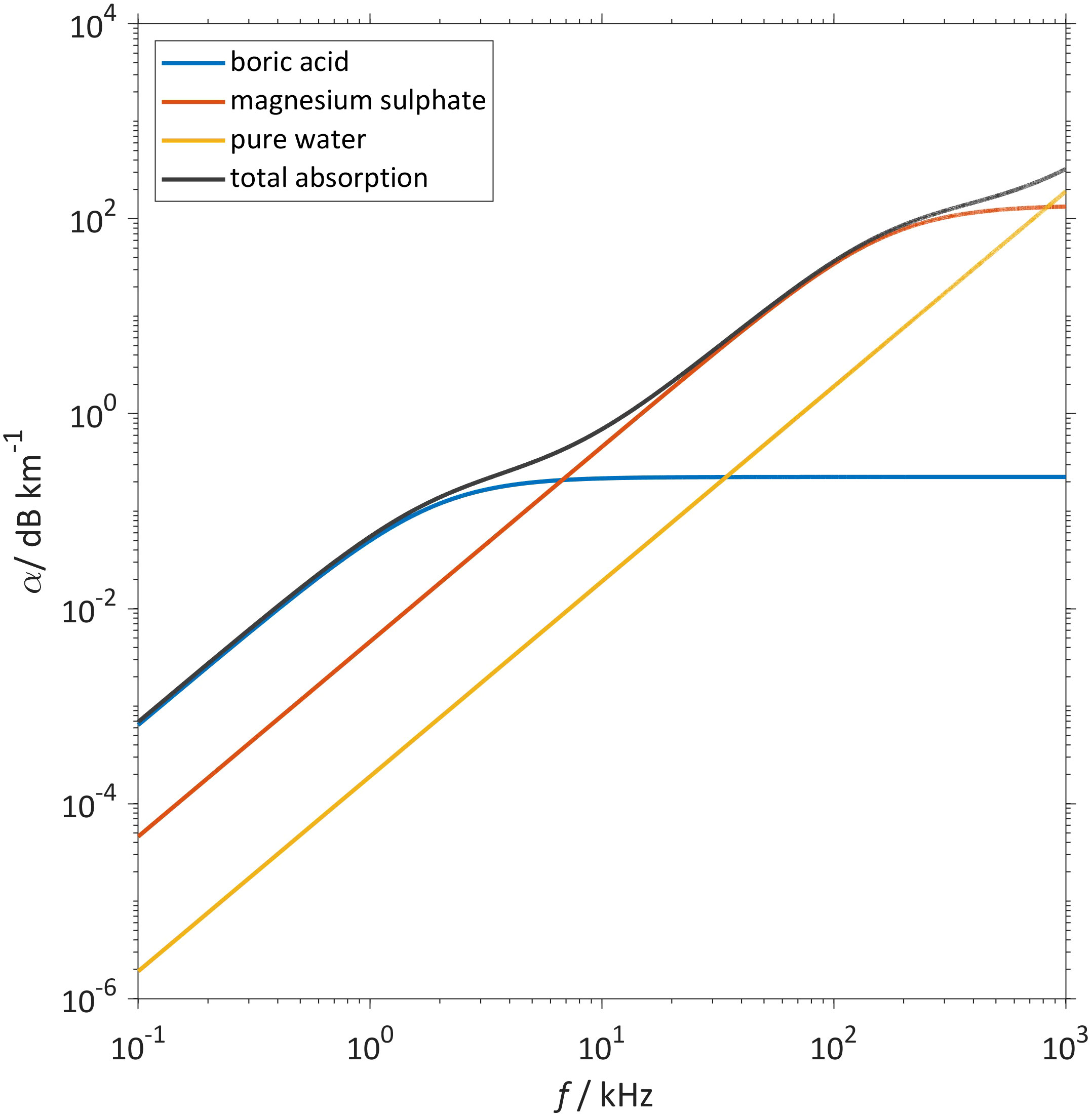
Figure 6 sound absorption (α) calculated at different frequencies (f) using the algorithm of (Francois and Garrison, 1982b) for the contribution of boric acid (blue), magnesium sulphate (red), pure water (yellow) and the sum of the three components (black).
2.2.1 Shallow waters (<200 m)
The term “shallow water” implies an acoustic environment such as a continental shelf shallower than 200 m. Despite shallow waters covering only 8% of the total sea areas, they host a large part of the total anthropogenic activities, including the associated noise generated. The interactions with the sea surface and seafloor make the PL in shallow waters larger and more complicated than in deep waters (Figure 7, Urick, 1979; Jensen, 1981). In general, shallow waters act as steep high-pass filters (Forrest et al., 1993), where low-frequency sound propagates poorly or not at all. For that reason, despite the large presence of broadband sources, such as shipping noise, the medium- to high-frequency contribution is larger than in the open ocean (Noise, 2003). In these environments, the major contributors to PL are cylindrical spreading, bottom attenuation and scattering due to roughness at the surface and bottom. Therefore the sound does not propagate over large distances because it loses energy after interacting with the sea surface and seafloor. The short propagation distance for low-frequency noise in shallow waters makes the contribution of sound absorption in seawater negligible but may still be important in the sediments.
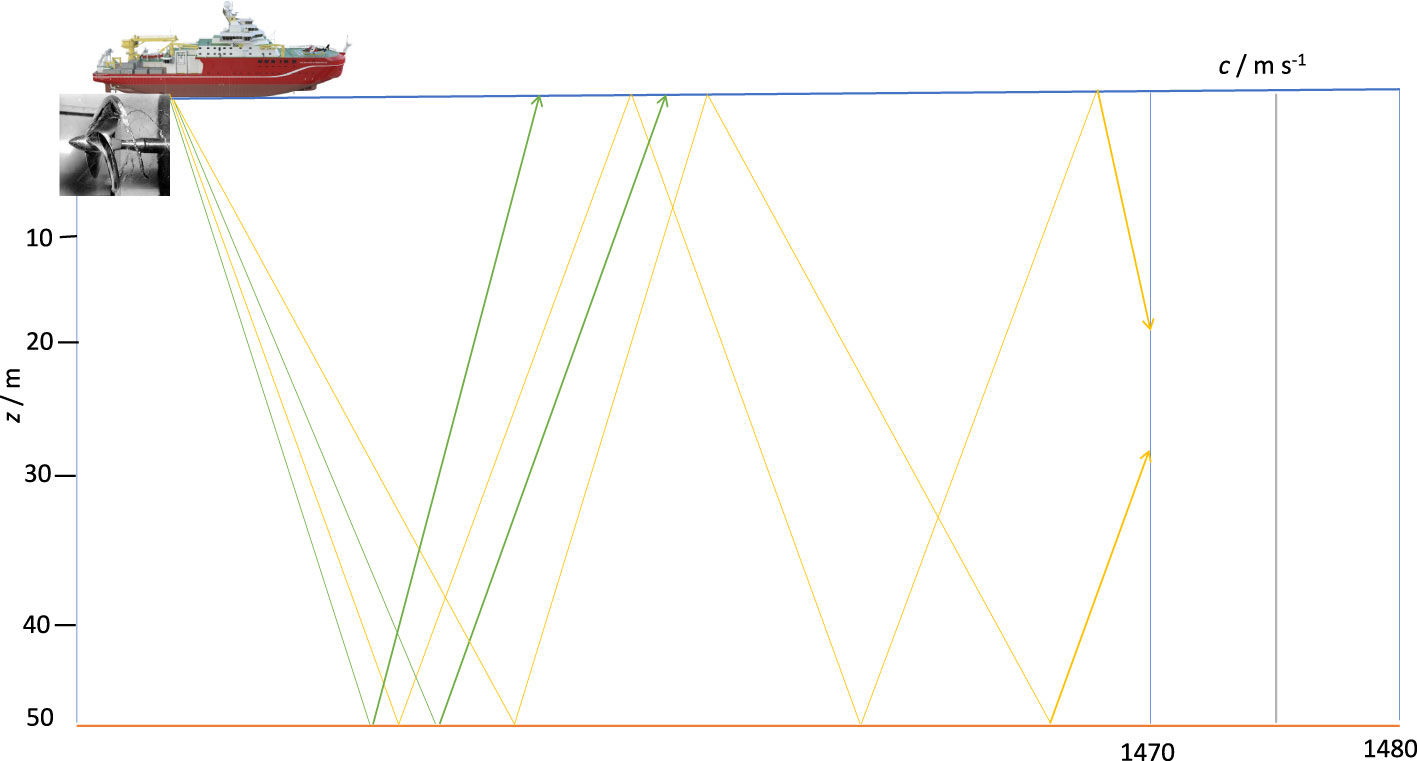
Figure 7 diagram showing the propagation of shipping noise in shallow sea on an indefinite distant and a bathymetry of 50 m. On the right side in grey a constant sound speed profile in m s-1, typical of a fully mixed sea. The yellow arrows show low frequency noise (<1 kHz) mainly generated by propeller cavitation and in the green higher frequency noise (>10 kHz).
The propagation of sound in shallow waters varies with season. During winter, shallow seas are generally well-mixed by wind and waves, leading to homogenous temperatures and salinities over the depth of the water column. As a result, sound speed can be considered uniform over depth and the propagation channel covers the entire water column from the sea surface to the seafloor. The constant sound speed also implies that sound travels straight until it interacts and it is reflected by the sea surface and seafloor. The seafloor does not reflect all sound but depending on the angle of incidence absorbs a portion of it (Urick, 1979). In this environment, high sound frequencies are largely attenuated by absorption and scattering by the seafloor (Kuperman and Ingenito, 1977). Since sound scattering increases with seafloor roughness, estimating PL is particularly challenging in shallow seas. An accurate estimation of the seafloor contribution to the PL therefore requires a specific survey to derive the seafloor sound speed, density and depth.
The consequent PL is connected to the frequencies of the sound source. At low frequencies, PL is largely controlled by attenuation at the seafloor where sound can leave the water column with the excitation of shear waves. However, low-frequency sound can only propagate if the effective water depth is larger than half the sound’s wavelength. The attenuation depends on the sediment properties (Kuperman and Ingenito, 1977; Urick, 1979) and the propagation of sound into the seafloor depends on critical angle ψ that is calculated using Equation 4 from the sound speed difference between the two layers:
where cw and cs are the sound speed in seawater and in the seafloor, respectively. In general, cs is larger than cw and it varies with the seafloor composition (for example, ψ for coarse silt is around 22° and for medium sand 30°). When sound travels at lower angles than ψ, sound is almost perfectly reflected. Instead, when sound travels at grazing angles greater than ψ, some of the sound is absorbed by the seafloor, reducing the reflected sound. In practice, to simplify the PL calculations the seafloor is generally considered a fluid with properties not too different from seawater. Therefore, after the interaction with the seafloor the sound field is not subject to drastic changes (Jensen, 1981). The other propagation limit is the sea surface which is considered a pressure release (Jensen, 1981) contributing to large PLs for receiver positions close to the sea surface, especially at low frequencies at<800 Hz the PL at 1 m depth is 25 dB higher than at mid-depth.
During the warmer season, water columns in temperate regions can be stratified with a warmer surface layer separated from a deeper cool layer by the thermocline. In this environment, noise generated at certain frequencies can propagate for many kilometers with the main PL given by the frequent interaction with the sea surface and seafloor (Jensen, 1981). The sound speed gradient in the surface layer changes the angles of the steeper rays into less steep angles, reducing the reflection loss at both sea surface and seafloor. This happens because steeper rays have higher losses than less steep rays. Another parameter affecting PL is the sound frequency that depends on the sound source. The PL for low frequency sound, defined as above the low-frequency cut off of the shallow water channel, is generally smaller because of the decrease in sound absorption and scattering at the surface (Francois and Garrison, 1982b; Kuperman and Roux, 2007). Snell’s law is frequency independent, however for a layered seafloor the reflectivity has a complicated frequency dependence affecting the reflection loss (Kuperman, 2019).
2.2.2 Open ocean
Deep seas are characterized by a minimum sound speed, of which the depth varies per region. At mid-latitudes the lowest sound speed is located at approximately 1000 m below the water surface. The depths adjacent to this minimum are known as the SOFAR or deep sound channel. The refraction in the SOFAR channel allows low-frequency noise to propagate over long distances (in some cases more than 1000 km) with no interactions with the sea surface and seafloor (Figure 8, Thorp, 1965; Chow and Turner, 1982). Consequently the largest contributor to PL here is absorption. However, at very low frequencies (e.g.< 10 Hz) noise cannot propagate in the SOFAR channel because the wavelength is larger than the SOFAR’s channel vertical extension. The propagation in the SOFAR channel happens because noise is always refracted or bent back towards the minimum sound speed (following Snell’s law). Below the SOFAR channel, temperature and salinity are generally constant and sound speed increases with depth causing the refraction of noise back to the SOFAR channel. In the SOFAR channel, PL is mainly driven by cylindrical spreading and absorption. Instead, the contribution of absorption increases with frequency, limiting the propagation distance at higher frequencies, for example contributing 36 dB km-1 to the PL at 100 kHz (Francois and Garrison, 1982a). Noise is trapped in the SOFAR channel when it is originated within the channel’s depth or when sound originates on the continental slope and part of it enters the SOFAR channel after reflection by the seafloor (Qin et al., 2014). At high latitudes, the SOFAR channel is replaced by a surface duct formed by freshwater coming from ice melting. As at mid-latitudes, under this surface duct salinity and temperature are constant for the entire water column and sound speed increases due to pressure, causing the refraction of noise back to the surface. The continuous interaction with the sea surface does not allow the propagation of noise to the same distances as happens in the SOFAR channel (Urick, 1979).
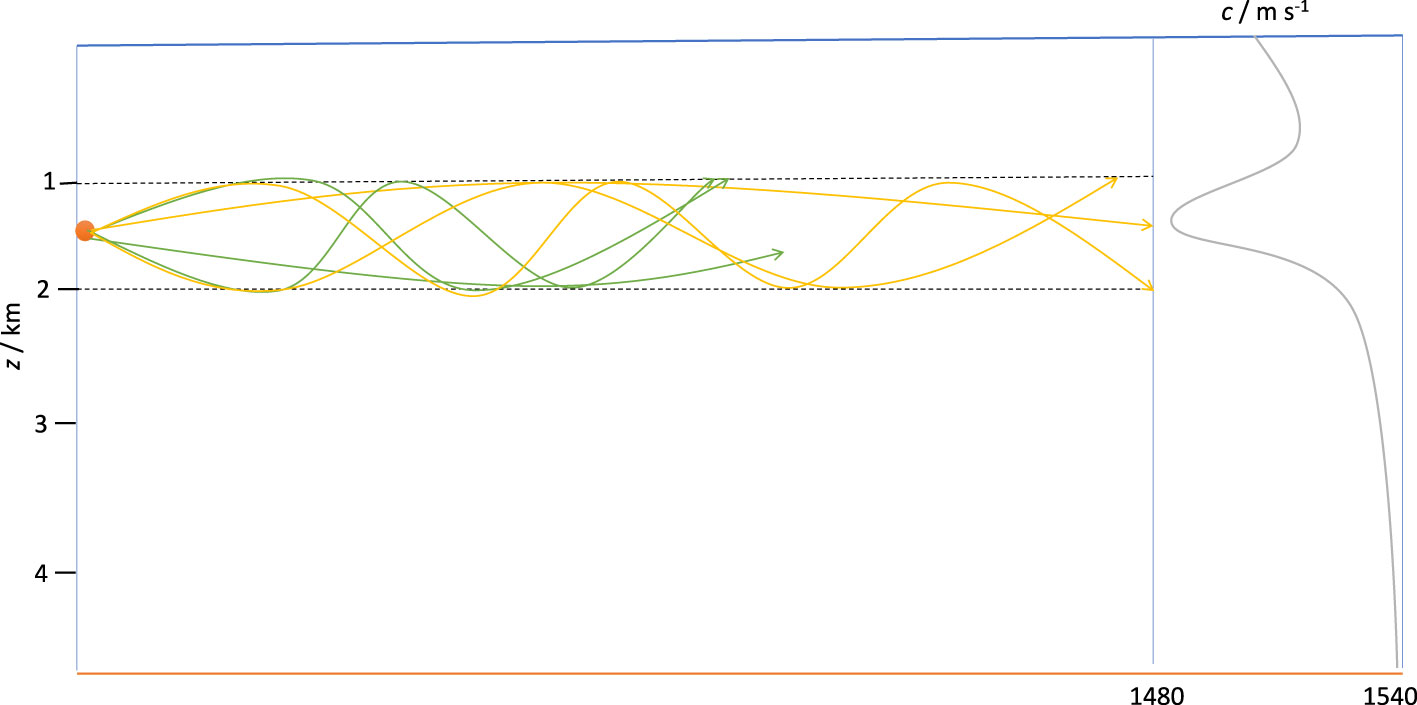
Figure 8 diagram showing the propagation of a source located in the SOFAR channel in the open ocean on an indefinite distant and a bathymetry >4 km. On the right side in grey the sound speed profile in m s-1 with a SOFAR channel (see section 4.1.2) between 1 and 2 km of water depth. The yellow arrows show low frequency noise (<1 kHz) and in the green higher frequency noise (>10 kHz). The low frequency sound can reach larger distances due to the lower sound absorption.
In most cases, a sound source located at the surface does not propagate into the SOFAR channel. In this case, noise is attenuated by the same phenomena of shallow seas but with a smaller PL due to fewer interactions with the sea surface and seafloor. A sound ray that leaves the source horizontally is refracted downward at steeper and steeper angles until it crosses the axis of the SOFAR channel. Under the SOFAR channel, the ray is refracted upward until it is horizontal and may reach the surface. Rays that start more vertically can reach larger depths and may be refracted back toward the surface. The refraction of noise downward leads to a rapid decrease in sound intensity in the horizontal direction. Figure 5 shows that noise refraction creates three different zones: 1) shadow zone, 2) convergence zone located at the surface (a narrow region of very high noise level) and 3) a wider region of lower noise level. The shadow zones are characterized by no noise. Frosch (1964) showed that the typical distance between convergence zones is between 48 to 56 km and they have been observed in the Atlantic and Pacific Oceans at more than 650 km from the source. However, in the Arctic, the range of convergence zones is generally very short or even absent (Urick, 1979) and sound mainly propagate in the top 100 m (Figure 9).
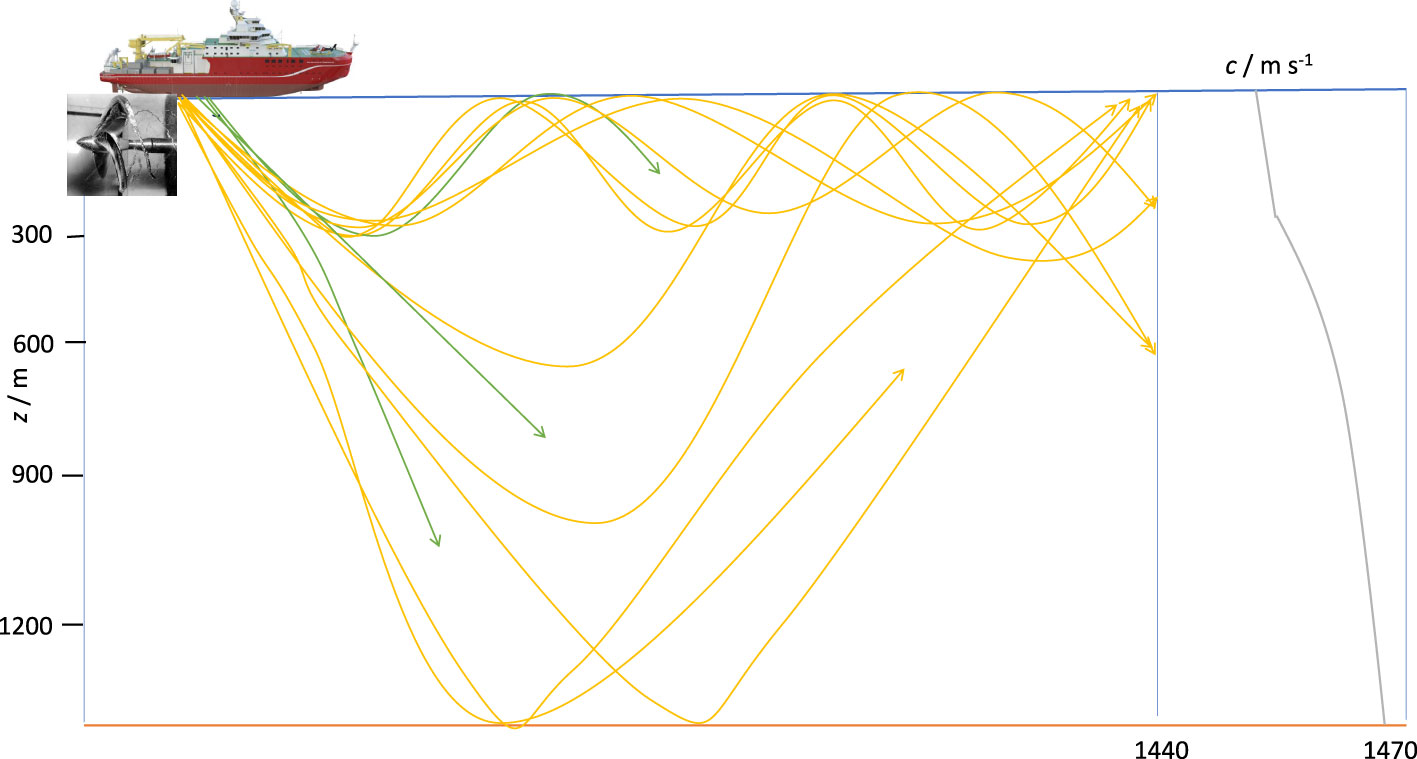
Figure 9 diagram showing the propagation of shipping noise in the Arctic Ocean on an indefinite distant and a bathymetry >4 km. On the right side in grey the sound speed profile in m s-1 with a SOFAR channel (see section 4.1.2) between 1 and 2 km of water depth. The yellow arrows show low frequency noise (<1 kHz) mainly generated by propeller cavitation and in the green higher frequency noise (>10 kHz). The low frequency sound can reach larger distances due to the lower sound absorption.
2.3 Anthropogenic changes
2.3.1 Ocean acidification
The ocean carbon system has been changing since the industrial revolution (starting around 1760) by the increase of atmospheric x(CO2) by more than 100 μmol mol-1. This resulted in a decrease of the global ocean pH average from 8.21 to 8.10, corresponding to a 29% increase in H+ activity (Fabry et al., 2008; Doney et al., 2009). The increase of x(CO2) is driven by human activities such as fossil fuel combustion and deforestation (Doney and Schimel, 2007). Future projections suggest that in the next decades, the ocean CO2 uptake will continue, further decreasing the surface ocean’s pH.
Ocean acidification is affecting the ocean environment by lowering the calcium carbonate saturation state (Ωcarbonate) and carbonate ion concentration. This impacts shell-forming organisms such as plankton, benthic mollusks, echinoderms and corals (Doney et al., 2009; Hofmann et al., 2011). Another effect is the reduction of α at low frequencies (<10 kHz, Hester et al., 2008). The climate projections predict by the middle of this century a decrease of pH up to 0.3 (Brewer, 1997), leading to a decrease in α by almost 40% (Hester et al., 2008). Figure 6 shows that at frequencies between 10 kHz to 100 kHz α is not affected by pH because α changes are controlled by the chemical relaxation of magnesium sulphate. At frequencies higher than 100 kHz α is driven just by water viscous absorption.
The most applied algorithm to calculate α was derived by Francois and Garrison (1982a) and its inputs are sound frequency, temperature, salinity, depth and pH. The pH dependency at low frequencies is influenced by a pH-dependent chemical relaxation between B(OH)3/ (Simmons and Fisher, 1975). Acoustic relaxations occur due to pressure-dependent volume changes. When a sound wave encounters a charged molecule such as borate a resonance can occur and the sound energy is lost. After the passage of the sound wave, the molecule returns to its normal state. In this case the molecule is bigger than B(OH)3 and because of its charge is associated with water molecules as a loose assemblage. A sound wave can temporarily compress this weak complex into B(OH)3 which is a lower-volume form (Brewer and Hester, 2009). The ratio between B(OH)3 and is set by seawater pH (Zeebe and Wolf-Gladrow, 2001), therefore in a more acidic environment will decrease in favor of B(OH)3 leading to a smaller α.
For simplicity, most of the α algorithms (Francois and Garrison, 1982b; Ainslie and McColm, 1998; van Moll et al., 2009) consider at low frequencies (<10 kHz) just the chemical relaxations between B(OH)3/. However, other studies have shown that at these frequencies α is also affected by other chemical species. Mellen et al. (1980) proposed a simple mechanism that involves the chemical relaxations between B(OH)3/ and /. The final algorithm was not satisfactory because the inclusion of these two mechanisms suggested a lower α than expected. In a later study, Mellen et al. (1983) hypothesized a four-state exchange including Ca2+ but they suggested that more research is needed to quantify the effect of Ca2+ and other ions on sound adsorption. Fischer (1979) hypothesized another mechanism involving the relaxation of MgCO3 and Mg(HCO3)+. All these hypotheses have not led to a final equation that relates seawater chemistry to sound absorption. Therefore, further research is necessary to identify all the chemical species affecting α and combine these dependencies within one single algorithm.
Several studies analyzed the effect of ocean acidification on PL showing a final effect of <2 dB (Joseph and Chiu, 2010; Udovydchenkov et al., 2010; Ainslie, 2011). For example, Hester et al. (2008) used the Global Ocean Data Analysis Project (GLODAP) (Key et al., 2004; Sabine et al., 2005) to estimate the decrease of α at 0.44 kHz since the industrial revolution. They found that in parts of the North Atlantic Ocean in the top 400 m α decreased by over 15% and by more than 10% in other parts of the Atlantic and Pacific Oceans. In the same study, they applied a conservative pH decrease of 0.15 for the coming decades to calculate sound absorption, they found a decrease of α by over 20% that increases to 60% for a pH decrease of 0.6. A similar decrease in α was found by Ilyina et al. (2010) that using climate models found a decrease in α up to 60% between 0.1 to 10 kHz for a decrease of ocean pH by 0.6. These changes in α depend on the region considered since ocean acidification has smaller effects at high latitudes (e.g. North Atlantic Ocean and Arctic Oceans) and regions of deep-water formation (Ilyina et al., 2010). Despite the projections showing a significant reduction in α the absolute change at >100 km from the source are projected to be less than 2 dB (Joseph and Chiu, 2010; Reeder and Chiu, 2010; Udovydchenkov et al., 2010; Ainslie, 2011). Sound absorption is not the only effect contributing to PL, it acts together with refraction, seabed attenuation, stratification, surface scattering and geometrical loss (spherical or cylindrical) (see section 3.1.1 and 3.1.2). In shallow seas, the changes in α will not be visible as PL is dominated by the interactions with the sea surface and seafloor. The contribution of α is larger in the deep sound channel where the largest components are geometrical spreading and α. Initially, the main mechanism controlling PL is geometrical spreading but at a certain distance (cross-over range) α becomes important. α has a frequency-squared dependence and therefore the cross-over distance is smaller with the increase in frequency.
The final effect has been quantified by Udovydchenkov et al. (2010) that applied a simple propagation model to predict underwater sound in the coming 100 years. Udovydchenkov et al. (2010) quantified that propagation loss will decrease by 1.5 dB at 500 Hz and about 2 dB at 1000 Hz. This happens because the absolute contribution of α at low frequencies is small (at <100 Hz is less than 0.002 dB km-1). They found that a sound increase due to decreasing α will only be detectable in quiet regions far away from shipping lanes and other anthropogenic activities.
The changes in α are important when sound is trapped in a sound channel (duct) without interacting with the ocean boundaries (seafloor and sea surface). In the Arctic Ocean where in the next 30-50 years pH is projected to decrease by 0.2 (from 8.1 to 7.9, Ciais et al., 2013), sound can be trapped in a duct located at around 150 m (Duda, 2017). Considering a sound source in the Beaufort Sea’s duct, a 0.2 pH decrease is expected to allow the propagation of 900 Hz sound 38% further (100-300 km) leading to an increase in sound of 7 dB at 200 km. However, most sound is generally not trapped in a duct and a 1 kHz sound source outside the duct is expected to increase by 0.5 dB only. All these studies estimated the increase in sound level assuming a constant pH decrease for the entire water column. However, the decrease in pH is expected to be larger at the surface compared to the deeper waters (Caldeira and Wickett, 2005). This implies that the projected changes in α might generally be overestimated.
To conclude, further research is needed to refine Francois and Garrison (1982b)’s algorithm. The inaccurate pH values used to derive the current algorithm results in potentially large uncertainties in the calculated α. To improve the uncertainty to less than 15%, pH needs to be measured using the proper pH scale (total scale) with an accuracy of <0.05 (Brewer et al., 1995). Also, these studies need to elucidate which seawater components contribute to α and include these components in the new algorithm. Even if the effect of OA in the future soundscape is expected to be small (<2 dB) the new algorithm could help to elucidate the size of this change.
2.3.2 Climate change
2.3.2.1 Sea surface warming and stratification
One effect of climate change is the increase of atmospheric temperature, 90% of this heat is absorbed by the oceans (von Schuckmann et al., 2023) causing the world’s oceans to warm. This temperature increase is contributing to lower α, although this effect is smaller than the contribution by the lower pH due to ocean acidification. At 1 kHz Hester et al. (2008) quantified a decrease in α between 5 to 10% for a rise in surface temperature of 3°C. At higher frequencies (200 kHz) the effect is expected to be the opposite and α will increase by more than 10%. At frequencies lower than 10 kHz Ilyina et al. (2010) predicted in 2100 a global surface warming up to 4.4°C decreasing α by 8%.
The largest temperature increase is expected at the high latitudes. This temperature increase is reducing the surface ice pack, forming surface ducts with low salinity characterized by a lower sound speed than the water below (Lynch et al., 2018). In their review, Lynch et al. (2018) also mentioned that at mid-latitudes, the propagation will change due to greater spatial variability in the presence of oceanic fronts and their gradients. Generally, the increase in sea-surface temperature will increase refraction (Ainslie, 2011). Surface heating decreases the proportion of radiated power trapped in the ocean and consequently decreases the global mean square sound pressure. The temperature will also decrease ψ, reducing the proportion of radiated power trapped in the ocean. Ainslie (2011) reported that an increase in surface temperature by 0.1°C gives an 8% reduction in the noise level. Ainslie et al. (2021) found that in the northeast Pacific Ocean temperature fluctuations can lead to variability of sound levels up to ±1.2 dB. This effect is mainly controlled by the amount of sound trapped in the deep sound channel. Climate change is also modifying the sound speed profile because of changes in stratification and temperature. For example, the surface sound speed is expected to increase, reducing scattering loss (Ainslie, 2005).
Sound absorption is in addition to changes in temperature also affected by the predicted decrease of surface salinity in mid- and high-latitudes and an increase at low latitudes. These salinity changes will be driven by a shift in global evaporation and precipitation patterns (Solomon et al., 2007). The magnitude of these changes is uncertain but the effect on α is expected to be minor. At 3 kHz a change of surface salinity by ±0.1 would contribute to a change in α of ±0.5% (Ilyina et al., 2010).
Another process that might alter sound propagation is the decrease of sea-ice. Menze et al. (2017) hypothesized that the predicted reduction of sea-ice will increase ambient sound levels and the propagation of shipping noise in polar regions. Sea-ice effectively attenuates acoustic waves and decouples ambient sound from local wind speeds and distant sources. A polar region with less sea-ice will be largely dominated by distant sources, but local sources will increase because ships will be able to navigate in these currently ice-dominated regions (Duarte et al., 2021). Considering sea-ice melting, Roth et al. (2012) hypothesized in the next decades that ambient noise in the Arctic will increase between 5 to 20 dB.
The changes in temperature and salinity will lead to different sound speeds compared to today (Affatati et al., 2022). Considering a “business-as-usual” climate scenario (Representative Concentration Pathway 8.5) they found an increase in sound speed up to 1.5% (around 20 m s-1) and a larger seasonal variability in sound speed. The expected changes will be mostly limited to the upper 500 m, except for a few regions such as the north-west Atlantic Ocean. The only regions showing a decrease in sound speed are the Labrador Sea and the north Atlantic Ocean. Affatati et al. (2022) hypothesized a relationship between this decrease and a future shift in the Gulf Stream. Also, the future sound speed will increase and changes in stratification could lead to the formation of new ducts. Possenti et al. (2023) found that in the coming years a new surface duct will be present around a depth of 100 m in the north-west Atlantic Ocean. In general, the effect of temperature on the future soundscape has not been studied in detail and although the processes that will be altered by ocean warming have been identified, studies are needed to quantify the final contribution to the future soundscape.
Temperature and salinity may affect formation and the content of ocean bubbles altering propeller cavitation as discussed in Section 2.2. However, from the studies reported it is difficult to judge the impact of climate change on the noise emitted by a cavitating propeller. A final conclusion has not been reached because the mechanisms are not well understood. Some authors reported a decrease in air-bubble production with a temperature increase (Slauenwhite and Johnson, 1999; Salter et al., 2014), while others showed an increase in the entrained air due to the increasing number of large bubbles (Callaghan et al., 2014). The field study by Callaghan, Stokes and Deane (2014) found that the surface white foam coverage is a good indicator for the amount of air entrained in seas and oceans. This is in line with the study of Christiansen et al. (2019) showing that between 6 and 10°C sea spray aerosol production, and therefore whitecap foam coverage, is at minimum leading to the conclusion of Lewis et al. (2004) that the whitecap foam coverage is generally greater at higher temperatures.
Thorpe et al. (1992) modelled the transport of bubbles in combination with the bubbles gas exchange with the surrounding water and tested it for changing environmental factors. They observed that the number of bubbles at a depth of 4 m to roughly halved every 10°C increase, contradicting the earlier observation based on whitecap foam coverage. The lack of a changing bubble size distribution (BSD) with varying temperature and the omission of the air entrainment process in this modelling exercise could explain the different results of both studies. Applicability of these results with respect to bubble presence therefore remains limited. Generally speaking, more bubbles leads to more sound attenuation. Following the field observations, related to whitecap foam coverage, seas already warmer than the minimum whitecap coverage temperature might experience marginally more attenuation at the surface. However, the effect of environmental parameters on the amount and even more on the size of bubbles remains largely unknown. Therefore, this subject needs more research before a conclusion to its effect on the underwater soundscape can be made.
2.3.3 Changes in storminess and wind
Climate change is globally increasing wind speeds. Young et al. (2011) used 17 years (1991-2008) of satellite altimeter measurements and observed a wind speed increase of 0.25 to 0.5% per year. This increase in wind speed is affected by the rise in the number of extreme events by at least 0.75% per year. The increase in wind speed will be larger in the Southern than in the Northern Hemisphere. At the sea surface, the rise in wind speed will increase PL due to rough surface scattering loss and the interaction with near-surface bubble clouds (Ainslie, 2005). The bubbles produced by wind play an important role, not just by scattering or absorbing sound but also by refracting the sound upwards of the sea surface. This refraction enhances the scattering loss associated with the rough air-sea boundary. Therefore, an increase in wind speed will lead to an increase in ambient noise but the PL is also expected to increase. Driven by wind speed the largest increase in ambient noise is expected in the tropics (Duarte et al., 2021). Also, the increase in wind speed will enhance the generation of more bubbles due to breaking waves (Thorpe and Humphries, 1980) with consequences for propeller noise, sound speed and PL. The PL is expected to increase and the sound speed to decrease but the size of these changes has not been quantified yet. Hence, the effects of wind and storms on PL require further research. The effect has not been studied in detail, making it challenging to quantify the contribution to the future soundscape.
3 Discussion
In the next decades, the oceans might become noisier because of changes in anthropogenic activities and possibly in sound propagation related to climate change. To reduce the increase of anthropogenic impacts, policymakers and the shipping industry are trying to reduce the generated noise by considering new regulations and new technologies. In section 3 we discussed the effect of climate change on sound propagation. Considering the available literature we can conclude that when the sound is not trapped in a duct the effect of climate change will be <2 dB. This increase can still be considered as appreciable because it is close to doubling of energy, which is seen at 3 dB but more research need to be done to fully understand the impact of this change. In other cases when sound is trapped in a duct the impact can be more relevant with an expected sound increase of >5 dB (Duda, 2017). However, studies investigating the impact of climate change on sound propagation were characterized by large uncertainties. For example, the mechanisms responsible for sound absorption are not well understood, leading to uncertainties in the applied algorithm (Francois and Garrison, 1982b).
Stratification in the water column will be also altered by climate change. However, few studies investigated the changes in sound propagation due to changes in stratification related to the increase of storms and changes in ocean circulation. Changes in ocean circulation are already visible in the North Atlantic Ocean where the Atlantic Meridional Overturning Circulation (AMOC) is slowing down (Delworth and Dixon, 2000; Visbeck et al., 2001; Bryden et al., 2005; Lynch-Stieglitz, 2017; Boers, 2021) and its impact is expected to increase. This impact might be significant due to the formation of new ducts making absorption a relevant parameter in many regions (Possenti et al., 2023).
In the next decades, loss of ice melting will allow safe navigation in the Arctic for a large part of the year. At the moment the Northern Sea Route is used by a small number of ships only during the summer (46 in 2012 and 71 in 2013, Lasserre, 2014). This new route will shift this region’s ambient noise under 1 kHz from wind noise-dominated to shipping noise-dominated (Worley and Walker, 1982; Zakarauskas et al., 1990; Cato and McCauley, 2002; Hatch et al., 2008). However, sea-ice irregularity will pose significant problems for shipping and these routes will likely start to grow just in the mid to late century (Lasserre and Pelletier, 2011; Lasserre, 2014).
Globally, the effect of climate change will coincide with an expected increase in shipping traffic driven by population growth and increased global trading. The Organisation for Economic Co-operation and Development OECD (2016) expects a doubling of ocean-based economy contribution to the global gross domestic product by 2030. In general, global maritime traffic is expected to increase between 240 to 1209% by 2050, especially in middle-income countries and particularly in Northeast Asia (Sardain et al., 2019). In another study, International Civil Aviation Organization ICAO (2016) quantified the annual growth in global passenger and freight traffic between 2012 and 2042 by 4.5 and 4.2%, respectively. The largest changes are predicted for Central Southwest Asia (up to 10%). In addition to shipping as a source for underwater noise, other activities are expected to affect the soundscape, including offshore construction, decommissioning of oil and gas infrastructure, deep-sea mining, widespread use of autonomous vehicles and new acoustic communication systems (e.g. underwater Wi-Fi) (Figure 5, Duarte et al., 2021; Thomsen et al., 2021). In the future autonomous vehicles will replace ships in many ocean monitoring applications, which may contribute to reduce ambient noise because autonomous vehicles can operate substantially quieter than manned ships (Griffiths et al., 2001; Cauchy et al., 2023). In general, predictions should be taken as rough estimates because models cannot accurately quantify economic growths and they cannot predict adverse economic events such as wars, pandemics and economic crises. During the COVID-19 pandemic the marine shipping density decreased by 54.8% (March et al., 2021) and consequently resulted in a major decrease in SPL (Thomson and Barclay, 2020). However, despite the noted uncertainties an increase in anthropogenic impacts on the marine environment due to increasing human activities at sea is almost certain.
Due to the concern about the impact of shipping noise on marine life, several studies have reviewed mitigation measures (Renilson et al., 2013; Williams et al., 2019; Burnham et al., 2021; Smith and Rigby, 2022; Vard Marine Inc, 2023). New technologies can be implemented in new and existing vessels for example by changing the propeller/propulsor design, modification of the wake flow, reduction of machinery noise and modification of the hull. The impact on fuel consumption is also an important aspect to consider in view of International Maritime Organization (IMO) regulations on the energy efficiency of existing and new ships. Vard Marine Inc. (2023) emphasizes that the shipping industry has not yet identified optimal solutions for this task and that new technologies are still under development. Various technologies are available to reduce machinery noise characterized by different costs, impact on frequency ranges and noise reductions. For example, resilient mounts can significantly reduce machinery noise in all vessels. A more costly option is the use of an electric power plant that efficiently reduces machinery noise. This is an effective solution to reduce noise emissions when propeller cavitation is not the dominant source. Measurements on small electric vessels show that these emit less noise than traditional vessels (Parsons et al., 2020, 2021) by 10 to 25 dB below 500 Hz (Parsons et al., 2020). However, at the moment this option is only feasible for short voyages such as short distance ferries. Other systems still under development for commercial shipping are air bubble systems that can reduce either machinery noise or propeller cavitation noise (Lloyd et al., 2024). The effectiveness of these systems depends on the location where the bubbles are injected in the flow. Overall, there is a lack of quantitative sea-trial data to quantify the effectiveness of mitigation measures for commercial vessels, especially for propeller cavitation.
Along with technological advancements policymakers are implementing new regulations and recommendations to reduce and monitor shipping noise. The first action was taken in 2008 by the IMO which set up a group to develop non-mandatory technical guidelines on ship noise control strategies. This resulted in the “IMO Guidelines for the reduction of underwater noise from commercial shipping to address the adverse impact on marine life” (IMO, 2014). These guidelines were revised in 2023 (IMO, 2023). In particular, the Scientific Committee of the International Whaling Commission (IWC) has endorsed the goal of reducing ambient noise by 3 dB in the next decade and 10 dB over the next 30 years.
The European Commission recognized noise as an ocean pollutant and produced a set of detailed criteria and indicators to help member states to monitor trends in ambient noise (63 and 125 Hz) (Van der Graaf et al., 2012). Over the past 10 years, ship classification societies such as Det Norske Veritas, Bureau Veritas, Registro Navale Italiano (RINA), American Bureau of Shipping and Lloyd’s Register have developed specific class rules to encourage the reduction of underwater radiated noise.
Together with the ongoing political discussion to reduce shipping noise, some regions are enforcing mitigation measures to protect key marine habitats. One of these ongoing measures consisting of a two-months voluntary vessel slowdown to 11 knots, during which the Vancouver Fraser Port Authority’s Enhancing Cetacean Habitat and Observation (ECHO) observed a decrease in SL up to 11.5 dB (Joy et al., 2019; MacGillivray et al., 2019). This maximum decrease of 11.5 dB was related to containerships, but smaller for cruise vessels (10.5 dB), vehicle carriers (9.3 dB), tankers (6.1 dB) and bulkers (5.9 dB). However, the final reduction in the received level was just 3.1 dB between 10 to 100 Hz and 0.3 dB between 10 to 100 kHz. Similar initiatives are only feasible for limited areas and requires the implementation of economic incentives. An enforced necessity to reduce speed to arrive in port, causing a possible delay, however might push ship owners to replace their fleet with quieter vessels. The main challenge will be the selections of critical regions and the required work to set site specific noise limits and enforce regulations.
4 Conclusions
In the coming decades, the ocean sound sources are expected to increase due to economic growth and the emerging of new ocean activities (e.g. deep-sea mining, offshore construction, etc.). However, the major source of anthropogenic noise is and will be shipping which is expected to double compared to 2014 before 2050 (Sardain et al., 2019). To reduce this impact, the shipping industry is evaluating the implementation of various technologies but the lack of international regulations and incentives is slowing the shift towards less noisy vessels. An immediate solution can be the application of speed reduction. This solution gave an effective result in reducing the single ship SL, though it led to a less satisfactory reduction of ambient noise (Joy et al., 2019).
At the same time, climate change is altering the marine environment and as a consequence sound propagation, ambient noise and sound absorption. Previous studies quantified the expected effect of climate on ambient sound as <2 dB however these studies did not fully elucidate the mechanisms behind the changes in sound propagation. For example, the mechanisms responsible for sound absorption are not well understood and very few studies looked in detail at the changes in ambient noise related to climate change. From the current knowledge, the climate change effect considering single mechanisms can be considered negligible even though the sum of all the mechanisms can become significant. These changes will be especially visible in remote regions (e.g., Arctic and Antarctica), where ice melting will alter the propagation of sound but also open new navigation routes increasing the anthropogenic noise in the region with potential changes larger than 20 dB. Therefore, more attention and research is necessary to fully understand the impact of climate change on the future soundscape. The additional research can help policymakers implementing new effective measures to reduce the impact of anthropogenic noise on the marine environment.
Author contributions
All authors contributed to the conception and design of the review. LP wrote the first draft of the manuscript and SB and RS wrote one section of the manuscript. All authors contributed to the article and approved the submitted version.
Funding
The author(s) declare financial support was received for the research, authorship, and/or publication of this article. This publication is part of the SOUND-2 project with project number P17-07 of the research program AQUA which is (partly) financed by the Dutch Research Council (NWO).
Conflict of interest
The authors declare that the research was conducted in the absence of any commercial or financial relationships that could be construed as a potential conflict of interest.
Publisher’s note
All claims expressed in this article are solely those of the authors and do not necessarily represent those of their affiliated organizations, or those of the publisher, the editors and the reviewers. Any product that may be evaluated in this article, or claim that may be made by its manufacturer, is not guaranteed or endorsed by the publisher.
References
Affatati A., Scaini C., Salon S. (2022). Ocean sound propagation in a changing climate: Global sound speed changes and identification of acoustic hotspots. Earth’s Future 10, e2021EF002099. doi: 10.1029/2021EF002099
Ainslie M. A. (2005). Effect of wind-generated bubbles on fixed range acoustic attenuation in shallow water at 1–4 kHz. J. acoustical Soc. America 118, 3513–3523. doi: 10.1121/1.2114527
Ainslie M. (2011). “Potential causes of increasing low frequency ocean noise levels,” in Proceedings of Meetings on Acoustics 161ASA. 70004 (Seatte United States: Acoustical Society of America). 12(1).
Ainslie M. A., Andrew R. K., Howe B. M., Mercer J. A. (2021). Temperature-driven seasonal and longer term changes in spatially averaged deep ocean ambient sound at frequencies 63–125 Hz. J. Acoustical Soc. America 149, 2531–2545. doi: 10.1121/10.0003960
Ainslie M. A., McColm J. G. (1998). A simplified formula for viscous and chemical absorption in sea water. J. Acoustical Soc. America 103, 1671–1672. doi: 10.1121/1.421258
Anagnostou M. N., Nystuen J. A., Anagnostou E. N., Nikolopoulos E. I., Amitai E. (2008). Evaluation of underwater rainfall measurements during the Ionian Sea rainfall experiment. IEEE Trans. Geosci. Remote Sens. 46, 2936–2946. doi: 10.1109/TGRS.2008.2000756
Anderson Hansen K., Hernandez A., Mooney T. A., Rasmussen M. H., Sørensen K., Wahlberg M. (2020). The common murre (Uria aalge), an auk seabird, reacts to underwater sound. J. Acoustical Soc. America 147, 4069–4074. doi: 10.1121/10.0001400
Andrew R. K., Howe B. M., Mercer J. A., Dzieciuch M. A. (2002). Ocean ambient sound: comparing the 1960s with the 1990s for a receiver off the California coast. Acoustics Res. Lett. Online 3, 65–70. doi: 10.1121/1.1461915
Andrew R. K., Howe B. M., Mercer J. A. (2011). Long-time trends in ship traffic noise for four sites off the North American West Coast. J. Acoustical Soc. America 129, 642–651. doi: 10.1121/1.3518770
Arveson P. T., Vendittis D. J. (2000). Radiated noise characteristics of a modern cargo ship. J. Acoustical Soc. America 107, 118–129. doi: 10.1121/1.428344
Atlar M., Billet M, Briancon-Marjollet L., Ceccio S., Oshima A., Semionicheva E., et al. (2002). “The Specialist Committee on Water Quality and cavitation, Final Report and recommendations to the 23rd ITTC,” in 23rd International Towing Tank Conference (ITTC) (Newcastle University).
Blake W. K. (2017). Mechanics of flow-induced sound and vibration, Volume 2 (London, United Kingdom: Complex flow-structure interactions. Academic press).
Boers N. (2021). Observation-based early-warning signals for a collapse of the Atlantic Meridional Overturning Circulation. Nat. Climate Change 11, 680–688. doi: 10.1038/s41558-021-01097-4
Bosschers J., Choi G. H., Hyundai H. I., Farabee K. T., Fréchou D., Korkut E., et al. (2017). Specialist committee on hydrodynamic noise. In: Final Report and Recommendations to the 28th International Towing Tank Conference Specialist Committee on Hydrodynamic Noise, Vol 45.
Bosschers J. (2018). 'Propeller tip-vortex cavitation and its broadband noise' (Enschede, the Netherlands: PhD thesis University of Twente).
Brandner P. A., Venning J. A., Pearce B. W. (2022). Nucleation effects on cavitation about a sphere. J. Fluid Mechanics 946, A1. doi: 10.1017/jfm.2022.511
Brennen C. (1969). The dynamic balances of dissolved air and heat in natural cavity flows. J. Fluid Mechanics 37, 115–127. doi: 10.1017/S0022112069000449
Brewer P. G. (1997). Ocean chemistry of the fossil fuel CO2 signal: The haline signal of “business as usual”. Geophysical Res. Lett. 24, 1367–1369. doi: 10.1029/97GL01179
Brewer P. G., Glover D. M., Goyet C., Shafer D. K. (1995). The pH of the North Atlantic Ocean: Improvements to the global model for sound absorption in seawater. J. Geophys. Res.: Oceans 100, 8761–8776. doi: 10.1029/95JC00306
Brewer P. G., Hester K. (2009). Ocean acidification and the increasing transparency of the ocean to low-frequency sound’. Oceanography 22, 86–93. doi: 10.5670/oceanog.2009.99
Briançon-Marjollet L., Merle L. (1996). Inception, development and noise of a tip vortex cavitation,” in 21st Symp. on Naval Hydrodynamics, Trondheim, Norway.
Bryden H. L., Longworth H. R., Cunningham S. A. (2005). Slowing of the Atlantic meridional overturning circulation at 25 N. Nature 438, 655–657. doi: 10.1038/nature04385
Burnham R. E., Vagle S., O’Neill C., Trounce K. (2021). The efficacy of management measures to reduce vessel noise in critical habitat of Southern Resident killer whales in the Salish Sea. Front. Mar. Sci. 8, 664691. doi: 10.3389/fmars.2021.664691
Caldeira K., Wickett M. E. (2005). Ocean model predictions of chemistry changes from carbon dioxide emissions to the atmosphere and ocean. J. Geophysical Res.: Oceans. 110, 1–12. doi: 10.1029/2004JC002671
Callaghan A. H., Stokes M. D., Deane G. B. (2014). The effect of water temperature on air entrainment, bubble plumes, and surface foam in a laboratory breaking-wave analog. J. Geophysical Research: Oceans 119, 7463–7482. doi: 10.1002/2014JC010351
Cato D. H., McCauley R. D. (2002). Australlan research in ambient sea noise. Acoustics Aust. (Oxford, United Kingdom). 30, 13–20.
Cauchy P., Heywood K. J., Merchant N. D., Risch D., Queste B. Y., Testor P. (2023). Gliders for passive acoustic monitoring of the oceanic environment. Front. Remote Sens. 4, 1106533. doi: 10.3389/frsen.2023.1106533
Ceccio S., Gowing S., Shen Y. T. (1997). The effects of salt water on bubble cavitation. J. Fluids Engineering. 119 (1), 155–163. doi: 10.1115/1.2819102
Chapman N. R., Price A. (2011). Low frequency deep ocean ambient noise trend in the Northeast Pacific Ocean. J. Acoustical Soc. America 129, EL161–EL165. doi: 10.1121/1.3567084
Chow R. K., Turner R. G. (1982). Attenuation of low-frequency sound in the Northeast Pacific Ocean. J. Acoustical Soc. America 72, 888–891. doi: 10.1121/1.388168
Christiansen S., Salter M. E., Gorokhova E., Nguyen Q. T., Bilde M. (2019). Sea spray aerosol formation: Laboratory results on the role of air entrainment, water temperature, and phytoplankton biomass. Environ. Sci. Technol. 53, 13107–13116. doi: 10.1021/acs.est.9b04078
Ciais P., Sabine C., Bala G., Bopp L., Brovkin V., Canadell J., et al. (2013). Carbon and other biogeochemical cycles. Climate Change 2013 - Phys. Sci. Basis, 465–570. doi: 10.1017/CBO9781107415324.015
Cox K., Brennan L. P., Gerwing T. G., Dudas S. E., Juanes F. (2018). Sound the alarm: A meta-analysis on the effect of aquatic noise on fish behavior and physiology. Global Change Biol. 24, 3105–3116. doi: 10.1111/gcb.14106
Delworth T. L., Dixon K. W. (2000). Implications of the recent trend in the Arctic/North Atlantic Oscillation for the North Atlantic thermohaline circulation. J. Climate 13, 3721–3727. doi: 10.1175/1520-0442(2000)013<3721:IOTRTI>2.0.CO;2
Doney S. C., Fabry V. J., Feely R. A., Kleypas J. A. (2009). Ocean acidification: the other CO 2Problem. Annu. Rev. Mar. Sci. 1, 169–192. doi: 10.1146/annurev.marine.010908.163834
Doney S. C., Schimel D. S. (2007). ‘Carbon and climate system coupling on timescales from the Precambrian to the Anthropocene’, Annu. Rev. Environ. Resour. 32, 31–66. doi: 10.1146/annurev.energy.32.041706.124700
Duarte C. M., Chapuis L., Collin S. P., Costa D. P., Devassy R. P., Eguiluz V. M., et al. (2021). The soundscape of the Anthropocene ocean. Science. 371 (6529). doi: 10.1126/science.aba4658
Duda T. F. (2017). Acoustic signal and noise changes in the Beaufort Sea Pacific Water duct under anticipated future acidification of Arctic Ocean waters. J. Acoustical Soc. America 142, 1926–1933. doi: 10.1121/1.5006184
Erbe C., Marley S. A., Schoeman R. P., Smith J. N., Trigg L. E., Embling C. B. (2019). The effects of ship noise on marine mammals—a review. Front. Mar. Sci. 6, 606. doi: 10.3389/fmars.2019.00606
Fabry V. J., Seibel B. A., Feely R. A., Orr J. C. (2008). Impacts of ocean acidification on marine fauna and ecosystem processes. ICES J. Mar. Sci. 65, 414–432. doi: 10.1093/icesjms/fsn048
Fischer R. (2008). Singing propellers—solutions and case histories. Mar. Technol. SNAME News 45, 221–227. doi: 10.5957/mt1.2008.45.4.221
Fischer R., Collier R. D. (2007). “Noise prediction and prevention on ships,” in Handbook of noise and Vibration Control, 1216–1232.
Fisher F. H. (1979). Sound absorption in sea water by a third chemical relaxation. J. Acoustical Soc. America 65, 1327–1329. doi: 10.1121/1.382752
Forrest T. G., Miller G. L., Zagar J. R. (1993). Sound propagation in shallow water: implications for acoustic communication by aquatic animals. Bioacoustics 4, 259–270. doi: 10.1080/09524622.1993.10510437
Franc J.-P., Michel J.-M. (2006). Fundamentals of cavitation (Dordrecht, the Netherlands: Springer science & Business media).
Francois R. E., Garrison G. R. (1982a). Sound absorption based on ocean measurements: Part I: Pure water and magnesium sulfate contributions. J. Acoustical Soc. America 72, 896–907. doi: 10.1121/1.388170
Francois R. E., Garrison G. R. (1982b). Sound absorption based on ocean measurements. Part II: Boric acid contribution and equation for total absorption. J. Acoustical Soc. America 72, 1879–1890. doi: 10.1121/1.388673
Frisk G. V. (2012). Noiseonomics: The relationship between ambient noise levels in the sea and global economic trends. Sci. Rep. 2, 1–4. doi: 10.1038/srep00437
Frosch R. A. (1964). Underwater Sound: Deep-Ocean Propagation: Variations of temperature and pressure have great influence on the propagation of sound in the ocean. Science 146, 889–894. doi: 10.1126/science.146.3646.889
Gowing S., Shen Y. T. (2001) Nuceli Effects on Tip Vortex Cavitation Scaling. Available online at: http://resolver.caltech.edu/cav2001:sessionA6.005.
Griffiths G., Enoch P., Millard N. W. (2001). On the radiated noise of the Autosub autonomous underwater vehicle. ICES J. Mar. Sci. 58, 1195–1200. doi: 10.1006/jmsc.2001.1120
Hatch L., Clark C., Merrick R., Van Parijs S., Ponirakis D., Schwehr K., et al. (2008). ‘Characterizing the relative contributions of large vessels to total ocean noise fields: a case study using the Gerry E. Studds Stellwagen Bank National Marine Sanctuary’. Environ. Manage. 42, 735–752. doi: 10.1007/s00267-008-9169-4
Hester K. C., Peltzer E. T., Kirkwood W. J., Brewer P. G. (2008). Unanticipated consequences of ocean acidification: A noisier ocean at lower pH. Geophysical Res. Lett. 35, 19. doi: 10.1029/2008GL034913
Hildebrand J. A. (2009). Anthropogenic and natural sources of ambient noise in the ocean. Mar. Ecol. Prog. Ser. 395, 5–20. doi: 10.3354/meps08353
Hofmann G. E., Smith J. E., Johnson K. S., Send U., Levin L. A., Paytan A., et al. (2011). High-frequency dynamics of ocean pH : A multi- ecosystem comparison . PloS one. 6 (12), e28983. doi: 10.1371/journal.pone.0028983
Hwang PA, Poon Y-K, Wu J. (1991). Temperature effects on generation and entrainment of bubbles induced by a water jet. J. Phys. Ocean. 21, 1602–5.
ICAO. (2016). ICAO Long-Term Traffic Forecasts Passenger and Cargo. Available at: https://www.icao.int/safety/ngap/NGAP8%20Presentations/ICAO-Long-Term-Traffic-Forecasts-July-2016.pdf.
Ilyina T., Zeebe R. E., Brewer P. G. (2010). Future ocean increasingly transparent to low-frequency sound owing to carbon dioxide emissions. Nat. Geosci. 3, 18–22. doi: 10.1038/ngeo719
IMO M. E. (2014). Guidelines for the reduction of underwater noise from commercial shipping to address adverse impacts on marine life. MEPC. (London, United Kingdom).
IMO (2023). “Revised guidelines for the reduction of underwater radiated noise from shipping to address adverse impacts on marine life,” in MEPC.1/Circ. 906. International Towing Tank Committee (ITTC) procedure 7.5-02-01-03(2011). (London, United Kingdom).
ITTC Specialist Committee on Hydrodynamic Noise (2017). “Final report and recommendations to the 27th ITTC,” in Proceedings of the 27th International Towing Tank Conference, Wuxi, China. Available at: https://www.ittc.info/media/7837/17-sc-hydrodynamic-noise-compressed.pdf.
Ivanova S. V., Kessel S. T., Espinoza M., McLean M. F., O’Neill C., Landry J., et al. (2020). Shipping alters the movement and behavior of Arctic cod (Boreogadus saida), a keystone fish in Arctic marine ecosystems. Ecol. Appl. 30, e02050. doi: 10.1002/eap.2050
Jensen F. B. (1981). Sound propagation in shallow water: a detailed description of the acoustic field close to surface and bottom. J. Acoustical Soc. America 70, 1397–1406. doi: 10.1121/1.387130
Jiang L.-Q., Carter B. R., Feely R. A., Lauvset S. K., Olsen A. (2019). Surface ocean pH and buffer capacity: past, present and future. Sci. Rep. 9, 1–11. doi: 10.1038/s41598-019-55039-4
Joseph J. E., Chiu C.-S. (2010). A computational assessment of the sensitivity of ambient noise level to ocean acidification. J. Acoustical Soc. America 128, EL144–EL149. doi: 10.1121/1.3425738
Joy R., et al. (2019). Potential benefits of vessel slowdowns on endangered southern resident killer whales. Front. Mar. Sci. 6, 344. doi: 10.3389/fmars.2019.00344
Key R. M., Kozyr A., Sabine C. L., Lee K., Wanninkhof R., Bullister J. L., et al. (2004). A global ocean carbon climatology : Results from Global Data Analysis Project (GLODAP ). Global Biogeochem. Cycles. 18, 1–23. doi: 10.1029/2004GB002247
Kuperman W. A., Ingenito F. (1977). Attenuation of the coherent component of sound propagating in shallow water with rough boundaries. J. Acoustical Soc. America 61, 1178–1187. doi: 10.1121/1.381417
Kuperman W., Roux P. (2007). Underwater acoustics (New York, United States: Springer Handbook of Acoustics), 149–204.
Lasserre F. (2014). Case studies of shipping along Arctic routes. Analysis and profitability perspectives for the container sector. Transportation Res. Part A: Policy Pract. 66, 144–161. doi: 10.1016/j.tra.2014.05.005
Lasserre F., Pelletier S. (2011). Polar super seaways? Maritime transport in the Arctic: an analysis of shipowners’ intentions. J. Transport Geogr. 19, 1465–1473. doi: 10.1016/j.jtrangeo.2011.08.006
Leaper R., Renilson M., Ryan C. (2014). Reducing underwater noise from large commercial ships: current status and future directions. J. Ocean Technol. 9 (1), 51.
Lewis E. R., Schwartz S. E. (2004). Sea salt aerosol production: mechanisms, methods, measurements, and models. (Washington, United States: American geophysical union).
Li M., Manica R., Xiang B., Liu Q. (2021). Effect of NaCl and CO2 on the inception control of hydrodynamic cavitation by gas solubility change. Chem. Eng. Sci. 246, 116997. doi: 10.1016/j.ces.2021.116997
Lloyd T, Lafeber F. H., Bosschers J. (2024). ‘Ship URN mitigation by air injection: model-scale experiments and application to full-scale measurement data’. Eight International Symposium on Marine Propellers, Berlin, Germany.
Lynch J. F., Gawarkiewicz G. G., Lin Y.-T., Duda T. F., Newhall A. E. (2018). Impacts of ocean warming on acoustic propagation over continental shelf and slope regions. Oceanography 31, 174–181. doi: 10.5670/oceanog.2018.219
Lynch-Stieglitz J. (2017). The Atlantic meridional overturning circulation and abrupt climate change. Annu. Rev. Mar. Sci. 9 (585), 83–104. doi: 10.1146/annurev-marine-010816-060415
MacGillivray A., de Jong C. (2021). A reference spectrum model for estimating source levels of marine shipping based on Automated Identification System data. J. Mar. Sci. Eng. 9, 369. doi: 10.3390/jmse9040369
MacGillivray A. O., Li Z., Hannay D. E., Trounce K. B., Robinson O. M. (2019). Slowing deep-sea commercial vessels reduces underwater radiated noise. J. Acoustical Soc. America 146, 340–351. doi: 10.1121/1.5116140
March D., Metcalfe K., Tintoré J., Godley B. J. (2021). Tracking the global reduction of marine traffic during the COVID-19 pandemic. Nat. Commun. 12, 1–12. doi: 10.1038/s41467-021-22423-6
McDonald M. A., Hildebrand J. A., Wiggins S. M. (2006). Increases in deep ocean ambient noise in the Northeast Pacific west of San Nicolas Island, California. J. Acoustical Soc. America 120, 711–718. doi: 10.1121/1.2216565
McKenna M. F., Ross D., Wiggins S. M., Hildebrand J. A. (2012). Underwater radiated noise from modern commercial ships. J. Acoustical Soc. America 131, 92–103. doi: 10.1121/1.3664100
Mellen R. H., Browning D. G., Simmons V. P. (1980). Investigation of chemical sound absorption in seawater by the resonator method: Part I. J. Acoustical Soc. America 68, 248–257. doi: 10.1121/1.384632
Mellen R. H., Browning D. G., Simmons V. P. (1983). Investigation of chemical sound absorption in sea water. Part IV’. J. Acoustical Soc. America 74, 987–993. doi: 10.1121/1.389845
Menze S., Zitterbart D. P., van Opzeeland I., Boebel O. (2017). The influence of sea ice, wind speed and marine mammals on Southern Ocean ambient sound. R. Soc. Open Sci. 4, 160370. doi: 10.1098/rsos.160370
Merchant N. D., Brookes K. L., Faulkner R. C., Bicknell A. W.J., Godley B. J., Witt M. J. (2016). Underwater noise levels in UK waters. Sci. Rep. 6, 1–10. doi: 10.1038/srep36942
Miksis-Olds J. L., Bradley D. L., Maggie Niu X. (2013). Decadal trends in Indian Ocean ambient sound. J. Acoustical Soc. America 134, 3464–3475. doi: 10.1121/1.4821537
Miksis-Olds J. L., Nichols S. M. (2016). Is low frequency ocean sound increasing globally? J. Acoustical Soc. America 139, 501–511. doi: 10.1121/1.4938237
Moss W. C., Levatin J. L., Szeri A. J. (2000). A new damping mechanism in strongly collapsing bubbles’, Proceedings of the Royal Society of London. Ser. A: Mathematical Phys. Eng. Sci. 456, 2983–2994. doi: 10.1098/rspa.2000.0649
Munk W. (2011). The sound of climate change. Tellus A: Dynamic Meteorology Oceanography 63, 190–197. doi: 10.1111/j.1600-0870.2010.00495.x
Murchy K. A., Davies H., Shafer H., Cox K., Nikolich K., Juanes F. (2019). “Impacts of noise on the behavior and physiology of marine invertebrates: A meta-analysis,” in Proceedings of Meetings on Acoustics (The Hague, the Netherlands: AIP Publishing).
Murchy K. A., Vagle S., Juanes F. (2022). Anchored bulk carriers have substantial impacts on the underwater soundscape in Cowichan Bay, British Columbia. Mar. pollut. Bull. 182, 113921. doi: 10.1016/j.marpolbul.2022.113921
Nanda S., Westerweel J., van Terwisga T., Elsinga G. (2022). Mechanisms for diffusion-driven growth of cavitating wing-tip vortices. Int. J. Multiphase Flow 156, 104146. doi: 10.1016/j.ijmultiphaseflow.2022.104146
Pachauri R. K., Allen M. R., Barros V. R., Broome J., Cramer W., Christ R., et al. (2014). Climate change 2014: synthesis report. Contribution of Working Groups I, II and III to the fifth assessment report of the Intergovernmental Panel on Climate Change (Geneva, Switzerland: Ipcc).
Parsons M. J. G., Duncan A. J., Parsons S. K., Erbe C. (2020). Reducing vessel noise: An example of a solar-electric passenger ferry. J. Acoustical Soc. America 147, 3575–3583. doi: 10.1121/10.0001264
Parsons M. J. G., Erbe C., Meekan M. G., Parsons S. K. (2021). A review and meta-analysis of underwater noise radiated by small (< 25 m length) vessels. J. Mar. Sci. Eng. p, 827. doi: 10.3390/jmse9080827
Pirotta E., Booth C. G., Costa D. P., Fleishman E., Kraus S. D., Lusseau D., et al. (2018). Understanding the population consequences of disturbance. Ecol. Evol. 8, 9934–9946. doi: 10.1002/ece3.4458
Poloczanska E., Mintenbeck K., Portner H. O., Roberts D., Levin L. A. (2018). “The IPCC special report on the ocean and cryosphere in a changing climate’,” in 2018 Ocean Sciences Meeting (Portland, United States: AGU).
Popper A. N., Hawkins A. D., Fay R. R., Mann D. A., Bartol S., Carlson T. J., et al. (2014). “Sound exposure guidelines,” in ASA S3/SC1. 4 TR-2014 Sound Exposure Guidelines for Fishes and Sea Turtles: A Technical Report prepared by ANSI-Accredited Standards Committee S3/SC1 and registered with ANSI (Richmond, United States: Springer), 33–51.
Possenti L., Reichart G.-J., de Nooijer L., Lam F.-P., de Jong C., Colin M., et al. (2023). Predicting the contribution of climate change on North Atlantic underwater sound propagation. PeerJ 11, e16208. doi: 10.7717/peerj.16208
Qin J. X., Zhang R. H., Luo W. Y., Peng Z. H., Liu J. J., Wang D. (2014). Sound propagation from the shelfbreak to deep water? Sci. China Physics Mechanics Astronomy 57, 1031–1037. doi: 10.1007/s11433-013-5297-9
Reeder D. B., Chiu C.-S. (2010). Ocean acidification and its impact on ocean noise: Phenomenology and analysis. J. Acoustical Soc. America 128, EL137–EL143. doi: 10.1121/1.3431091
Renilson M., Leaper R., Boisseau O. (2013). “‘Hydro-acoustic noise from merchant ships–impacts and practical mitigation techniques’,” in Proceedings of the third international symposium on marine propulsors, smp. 201–208.
Richardson W. J., Greene C. R. Jr., Malme C. I., Thomson D. H. (2013). Marine mammals and noise (San Diego, United States: Academic press).
Ross D. (1979). Mechanics of underwater noise. (New York, United States: Elsevier Science & Technology Books).
Roth E. H., Hildebrand J. A., Wiggins S. M., Ross D. (2012). Underwater ambient noise on the Chukchi Sea continental slope from 2006–2009’. J. Acoustical Soc. America 131, 104–110. doi: 10.1121/1.3664096
Rouseff D., Tang D. (2010). Internal waves as a proposed mechanism for increasing ambient noise in an increasingly acidic ocean. J. Acoustical Soc. America 127, EL235–EL239. doi: 10.1121/1.3425741
Sabine C. L., Key R. M., Kozyr A., Feely R. A., Wanninkhof R., Millero F. J., et al. (2005). “Global ocean data analysis project: Results and data,” in ORNL/CDIAC-145, NDP-083, Carbon Dioxide Information Analysis Center, Oak Ridge National Laboratory. (Oak Ridge, Tennessee: US Department of Energy).
Salter M. E., Nilsson E. D., Butcher A., Bilde M. (2014). On the seawater temperature dependence of the sea spray aerosol generated by a continuous plunging jet. J. Geophysical Research: Atmospheres 119, 9052–9072. doi: 10.1002/2013JD021376
Sardain A., Sardain E., Leung B. (2019). Global forecasts of shipping traffic and biological invasions to 2050. Nat. Sustainability 2, 274–282. doi: 10.1038/s41893-019-0245-y
Simard Y., Roy N., Gervaise C., Giard S. (2016). ‘Analysis and modeling of 255 source levels of merchant ships from an acoustic observatory along St. Lawrence Seaway’. J. Acoustical Soc. America 140, 2002–2018. doi: 10.1121/1.4962557
Simmons V. P., Fisher F. H. (1975). Low-frequency (1 kHz) sound absorption in sea water as measured in the laboratory using a spherical resonator. J. Acoustical Soc. America 57, S56–S56. doi: 10.1121/1.1995306
Slabbekoorn H., Bouton N., van Opzeeland I., Coers A., ten Cate C., Popper A. N. (2010). A noisy spring: the impact of globally rising underwater sound levels on fish. Trends Ecol. Evol. 25, 419–427. doi: 10.1016/j.tree.2010.04.005
Slauenwhite D. E., Johnson B. D. (1999). Bubble shattering: Differences in bubble formation in fresh water and seawater. J. Geophysical Research: Oceans 104, 3265–3275. doi: 10.1029/1998JC900064
Smith T. A., Rigby J. (2022). Underwater radiated noise from marine vessels: A review of noise reduction methods and technology. Ocean Eng. 266, 112863. doi: 10.1016/j.oceaneng.2022.112863
Solomon S., Manning M., Marquis M., Qin D. (2007). Climate change 2007-the physical science basis: Working group I contribution to the fourth assessment report of the IPCC (Cambridge, United Kingdom: Cambridge university press).
Soudijn F. H., van Kooten T., Slabbekoorn H., de Roos A. M. (2020). Population-level effects of acoustic disturbance in Atlantic cod: a size-structured analysis based on energy budgets’. Proc. R. Soc. B 287, 20200490. doi: 10.1098/rspb.2020.0490
Southall B. L., Bowles A. E., Ellison W. T., Finneran J. J., Gentry R. L., Greene C. R. Jr., et al. (2008). Marine mammal noise-exposure criteria: initial scientific recommendations. Bioacoustics 17, 273–275. doi: 10.1080/09524622.2008.9753846
Southall B. L., Finneran J. J., Reichmuth C., Nachtigall P. E., Ketten D. R., Bowles A. E., et al. (2019). Marine mammal noise exposure criteria: updated scientific recommendations for residual hearing effects. Aquat. Mammals 45 (2), 125–232. doi: 10.1578/AM.45.2.2019.125
Spence J. H., Fischer R. W. (2016). Requirements for reducing underwater noise from ships. IEEE J. Oceanic Eng. 42, 388–398. doi: 10.1109/JOE.2016.2578198
Thomsen F., Mendes S., Bertucci F., Breitzke M., Ciappi E., Cresci A., et al. (2021). Addressing underwater noise in Europe: Current state of knowledge and future priorities. (European Marine Board Ostend, Belgium). doi: 10.5281/zenodo.5534224
Thomson D. J. M., Barclay D. R. (2020). Real-time observations of the impact of COVID-19 on underwater noise. J. Acoustical Soc. America 147, 3390–3396. doi: 10.1121/10.0001271
Thorp W. H. (1965). Deep-ocean sound attenuation in the sub-and low-kilocycle-per-second region. J. Acoustical Soc. America 38, 648–654. doi: 10.1121/1.1909768
Thorpe S. A., Bowyer P., Woolf D. K. (1992). Some factors affecting the size distributions of oceanic bubbles. J. Phys. oceanography 22, 382–389. doi: 10.1175/1520-0485(1992)022<0382:SFATSD>2.0.CO;2
Thorpe S. A., Humphries P. N. (1980). Bubbles and breaking waves. Nature 283, 463–465. doi: 10.1038/283463a0
Tournadre J. (2014). Anthropogenic pressure on the open ocean: The growth of ship traffic revealed by altimeter data analysis. Geophysical Res. Lett. 41, 7924–7932. doi: 10.1002/2014GL061786
Trummler T., Schmidt S. J., Adams N. A. (2021). Numerical investigation of non-condensable gas effect on vapor bubble collapse. Phys. Fluids 33, 96107. doi: 10.1063/5.0062399
Udovydchenkov I. A., Duda T. F., Doney S. C., Lima I. D. (2010). Modeling deep ocean shipping noise in varying acidity conditions. J. Acoustical Soc. America 128, EL130–EL136. doi: 10.1121/1.3402284
Urick R. J. (1979). “Sound propagation in the sea,” in Darpa. (Washington, United States: Defense Advanced Research Projects Agency DARPA).
Vagle S., Large W. G., Farmer D. M. (1990). An evaluation of the WOTAN technique of inferring oceanic winds from underwater ambient sound. J. atmospheric oceanic Technol. 7, 576–595. doi: 10.1175/1520-0426(1990)007<0576:AEOTWT>2.0.CO;2
Van der Graaf A. J., Ainslie M. A., André M., Brensing K., Dalen J., Dekeling R. P.A., et al. (2012). European Marine Strategy Framework Directive-Good Environmental Status (Msfd Ges): Report of the Technical Subgroup on Underwater Noise and Other Forms of Energy. Brussels: TSG Noise Milieu Ltd.
van Moll C. A. M., Ainslie M. A., van Vossen R. (2009). A simple and accurate formula for the absorption of sound in seawater. IEEE J. Oceanic Eng. 34, 610–616. doi: 10.1109/JOE.2009.2027800
Vard Marine Inc (2023). Ship energy efficiency and underwater radiated noise Report 545-000-02, rev. 3.
Visbeck M. H., Hurrell J. W., Polvani L., Cullen H. M. (2001). The North Atlantic Oscillation: past, present, and future. Proc. Natl. Acad. Sci. 98, 12876–12877. doi: 10.1073/pnas.231391598
von Schuckmann K., Minière A., Gues F., Cuesta-Valero F. J., Kirchengast G., Adusumili S., et al. (2023). Heat stored in the Earth system 1960–2020: where does the energy go? Earth System Sci. Data 15, 1675–1709. doi: 10.5194/essd-15-1675-2023
Wenz G. M. (1962). Acoustic ambient noise in the ocean: spectra and sources. J. Acoustical Soc. America 34, 1936–1956. doi: 10.1121/1.1909155
Williams R., Veirs S., Veirs V., Ashe E., Mastick N. (2019). Approaches to reduce noise from ships operating in important killer whale habitats. Mar. pollut. Bull. 139, 459–469. doi: 10.1016/j.marpolbul.2018.05.015
Wong G. S. K., Zhu S. (1995). Speed of sound in seawater as a function of salinity, temperature, and pressure. J. Acoustical Soc. America 97, 1732–1736. doi: 10.1121/1.413048
Worley R. D., Walker R. A. (1982). Low-frequency ambient ocean noise and sound transmission over a thinly sedimented rock bottom. J. Acoustical Soc. America 71, 863–870. doi: 10.1121/1.387565
Young I. R., Zieger S., Babanin A. V. (2011). Global trends in wind speed and wave height. Science 332, 451–455. doi: 10.1126/science.1197219
Zakarauskas P., Chapman D. M. F., Staal P. R. (1990). Underwater acoustic ambient noise levels on the eastern Canadian continental shelf. J. Acoustical Soc. America 87, 2064–2071. doi: 10.1121/1.399333
Keywords: climate change, shipping, underwater acoustics, soundscape, ocean noise
Citation: Possenti L, de Nooijer L, de Jong C, Lam F-P, Beelen S, Bosschers J, van Terwisga T, Stigter R and Reichart G-J (2024) The present and future contribution of ships to the underwater soundscape. Front. Mar. Sci. 11:1252901. doi: 10.3389/fmars.2024.1252901
Received: 04 July 2023; Accepted: 06 February 2024;
Published: 08 March 2024.
Edited by:
Cathryn Murray, Fisheries and Oceans Canada (DFO), CanadaReviewed by:
Rianna Burnham, University of Victoria, CanadaKelsie Murchy, University of Victoria, Canada
Copyright © 2024 Possenti, de Nooijer, de Jong, Lam, Beelen, Bosschers, van Terwisga, Stigter and Reichart. This is an open-access article distributed under the terms of the Creative Commons Attribution License (CC BY). The use, distribution or reproduction in other forums is permitted, provided the original author(s) and the copyright owner(s) are credited and that the original publication in this journal is cited, in accordance with accepted academic practice. No use, distribution or reproduction is permitted which does not comply with these terms.
*Correspondence: Luca Possenti, bHVjYS5wb3NzZW50aUB3dXIubmw=
†Present address: Luca Possenti, Wageningen Marine Research, Wageningen University and Research, IJmuiden, Netherlands