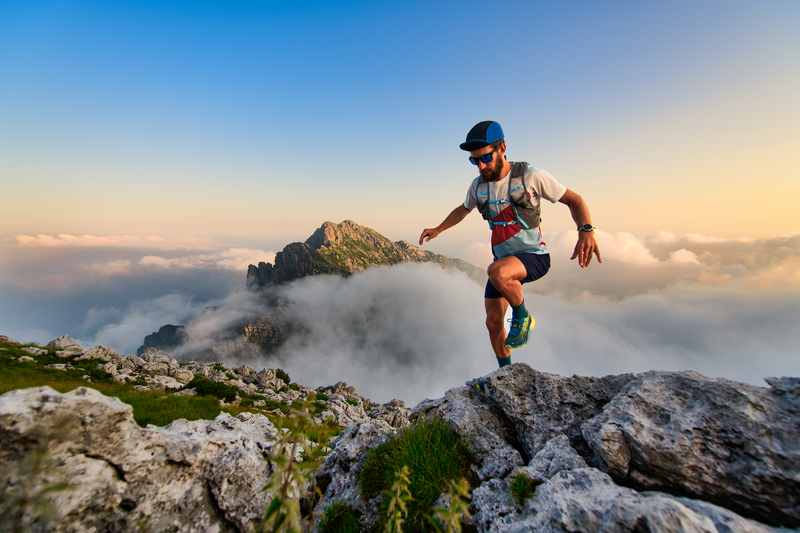
95% of researchers rate our articles as excellent or good
Learn more about the work of our research integrity team to safeguard the quality of each article we publish.
Find out more
ORIGINAL RESEARCH article
Front. Mar. Sci. , 06 February 2023
Sec. Marine Fisheries, Aquaculture and Living Resources
Volume 10 - 2023 | https://doi.org/10.3389/fmars.2023.992179
This article is part of the Research Topic Sustainable Seaweed Aquaculture: Current Advances and its Environmental Implications View all 6 articles
Production of sugar kelp, Saccharina latissima, has the potential of extracting nutrients and carbon from the seawater and returning it to land as a bioresource. The production thereby acts as an emission capture and utilization instrument contributing to mitigation of eutrophication and climate change. To achieve higher biomass yields improving the effects on climate and environment, and the economic feasibility of the production, cultivation techniques need to be optimized. In Denmark so far, S. latissima production yields have been lower than yields documented from Norway and the Faroe Islands. Use of alternative cultivation infrastructure designs with a higher line density per area, and better understanding the effects of annual and seasonal variations in abiotic parameters on growth, could lead the way towards a future higher yield of the S. latissima production. In this study, S. latissima was cultivated in a Danish commercial scale cultivation site for two consecutive seasons comparing the yields of different cultivation techniques: cultivation on nets and a multi-layer single line system, while also testing the possibility of coppicing the blades instead of applying a full harvest. Biomass yields of 5.0 - 6.8 kg FW m-1 cultivation line year-1, and 10.9 – 30.4 kg FW m-2 net structure were achieved. Biomass production, nutrient and carbon extraction potentials up to 91.3 t FW, 110 kg N, 13.1 kg P and 5.1 t C ha-1 were obtained using net cultivation systems and a 1.5 years growth period. Depth of cultivation had a significant effect on yield, but no effect on dry matter, C, N or P contents of S. latissima in a multi-layered line system. Biomass yields from the same system and site varied with a factor of four between years. The use of coppicing enabled multiple harvests of S. latissima, providing however biomass of reduced quality in the second year. This study demonstrates that the production yield of S. latissima can be increased by optimizing cultivation infrastructure, and indicates that net systems, or other cultivation systems with a high line density in the upper water column, can be a means to increase the areal yield of S. latissima.
The production of kelp is expanding worldwide, mainly driven by an increase in production from aquaculture in Asia (Chopin and Tacon, 2020). As the production of kelp is not competing for arable land, does not use freshwater for growth, and has the nature of taking up carbon dioxide (CO2) and nutrients directly from the ocean as it grows, kelp production aligns with several UN Sustainable Development Goals (Duarte et al., 2022). In Europe, sugar kelp, Saccharina latissima (Linnaeus) C.E.Lane, C.Mayes, Druehl & G.W.Saunders, is the most widely cultivated species, and multiple research and development (R & D) activities are directed towards the upscaling of its cultivation (Araújo et al., 2022). The three primary drivers for scaling up production are 1) bio-resource production; 2) counteracting eutrophication and 3) climate change mitigation. In Europe, the interest is increasing for using kelp as a bioresource for food and cosmetics, but also as a raw material for feed (Araújo et al., 2021). At the same time, European countries make great efforts in meeting the goals of the EU Water Framework Directive, in which mitigation of eutrophication is one of the major foci (EU 2000). As elevated nutrient concentrations cause eutrophication in marine coastal areas, as e.g. in inner Danish waters (Hansen and Høgslund, 2021), the use of S. latissima as an emission capture and utilization instrument to extract and re-use nitrogen and phosphorous, and hereby supporting the circular bioeconomy, is on the agenda of governmental institutions and the European Commission (European commision, 2022). Nutrient extraction potentials using S. latissima as a mitigation tool, has previously been reported in the range of 3 – 46 kg N ha-1 (Grebe et al., 2021). Through photosynthesis, seaweeds assimilate carbon (C) in the forms of CO2 and HCO3-, which is either incorporated into structural tissue or subsequently lost as dissolved or particulate organic carbon (DOC or POC) (Parke, 1948; Maberly, 1990; Abdullah and Fredriksen, 2004; Paine et al., 2021), of which only a fraction is sequestered (Krause-Jensen and Duarte, 2016; Hurd et al., 2022). The downstream use of the kelp biomass will affect how fast the C will reenter the atmosphere, and therefore how the production will affect the CO2 content of the atmosphere (Chung et al., 2011). If the seaweed biomass is used for purposes which substitute or reduce the use of fossil fuels, this could lead to a decrease in atmospheric CO2 (Chung et al., 2011). The Chinese production of Laminaria sp. has been calculated to remove an average of 8.59 t C ha-1 y-1 corresponding to 31.53 t CO2 ha-1 y-1 (Zheng et al., 2019). Recent cultivation trials of S. latissima in Danish waters have documented a C extraction equivalent to 2.40 t CO2 ha-1 y-1, and an overall carbon negative production during a 10 year production period in a full lifecycle analysis of the production (Zhang et al., 2022).
In order to enable the cultivation of S. latissima as a bioresource and a nutrient and carbon extraction tool, there is a need to produce it profitably at large scale. Current yields obtained in inner Danish waters are typically 10-50% of the yields reported from other European countries such as Norway, Scotland, The Faroe Islands or Spain, potentially due to differences in environmental parameters at the cultivation sites, genetic differences between cultivars, or use of less efficient infrastructure designs (Peteiro and Freire, 2013b; Bak et al., 2018; Forbord et al., 2020; Kerrison et al., 2020). The cultivation systems presently used in Denmark are longline systems with cultivation lines attached to horizontal longlines as discrete vertical lines (droppers) or as continuous loops. In an experimental setup, a yield of 1.6 kg fresh weight (FW) m-1 was obtained from vertical cultivation lines (deployed from 0 – 2 m depth, identical to this study) from the Limfjorden, Denmark after 9 months of growth, with an areal yield of 1.6 t FW ha-1 (Boderskov et al., 2021a). The highest reported yield from Denmark was derived from a vertical dropper system where cultivation lines were deployed from 1 – 5 m depth. Here, yields of 0.4 and 3.2 kg FW m-1 cultivation line were obtained after 5 and 18 month of cultivation, respectively, with a maximal estimated areal yield of 7.1 t FW ha-1 (Marinho et al., 2015). In comparison, yields of 16.1 kg m-1 and 40.2 t FW ha-1, were obtained with a horizontal line cultivation system suspended from 0 – 3 m depth in Northern Spain, at the North Atlantic coast (Peteiro and Freire, 2013b), a yield of 7.2 kg m-1 was achieved from vertical cultivation lines at 1 – 2 m depth in Norway (Forbord et al., 2020), a yield around 7.5 kg m-1 from a horizontal cultivation line system at 1.5 m depth in Scotland (Kerrison et al., 2020) and a yield of 0.33 kg dry weight (DW) m-1 corresponding to 3.3 kg FW m-1 (if using a 10% DW to FW ratio) was obtained from vertical cultivation lines deployed from 0 – 10 m depth in the Faroe Islands (Bak et al., 2018). The cultivation in the Faroe Islands allows for coppicing (applying multiple harvests) with up to four harvests of the same seeded structures during two consecutive cultivation seasons (two years), resulting in a total areal yield of 2.9 t dry matter (DM) ha-1 or 29 t FW ha-1 (Bak et al., 2018).
The aim of this study was to increase biomass yields of cultivated S. latissima in inner Danish waters by optimizing the cultivation infrastructure design, and at the same time to document the nutrient and carbon extraction potential of the production. In this study, the focus is on the nutrient and carbon ‘extraction’ potential, not the ‘carbon sequestration’ potential which is defined as ‘the secure storage of carbon-containing molecules for >100 years’ (Hurd et al., 2022). In contrast, the ‘extraction potential’ is defined as the nutrients and the carbon removed from the marine environment, harvested in the biomass and made available for re-use in the bio-economic system on land. The study was conducted over two consecutive cultivation seasons, comparing the yields from three systems: vertical lines, a horizontal multi-layer setup, and a net system, including a test of coppicing of S. latissima.
We hypothesized that 1) the areal biomass yield could be increased significantly by increasing the line density and hence stocking density per area, using either a net structure or a horizontal 5-line system, 2) biomass yields would vary interannually, due to variations in environmental parameters, 3) nutrient and carbon extraction potentials will primarily be driven by biomass yields, and 4) early-summer biofouling of the biomass would preclude coppicing and re-harvest.
Data on light attenuation, seawater temperature, salinity, and nutrient concentrations (NO3/NO2-N, NH4/NH3-N and PO4-P) was obtained from a nearby marine environmental monitoring station (VEJ0006870, Figure 1) maintained by the Danish National Monitoring Programme (NOVANA). In addition, Photosynthetically Active Radiation (PAR) and temperature loggers (Odyssey ®), were deployed at the cultivation site, at 2.5 m depth, with an additional PAR logger at 4.5 m depth. Water sampling for nutrient and salinity measurements was performed at 1 m depth with, in average, 17 day intervals (min. 7 and max. 74 days) from 10 October 2018 to 11 June 2020. At the same occasions, the PAR and temperature loggers were cleaned.
Figure 1 Location of the seaweed and mussel cultivation sites and the national environmental monitoring station, from where the environmental information was extracted (NOVANA station: VEJ0006870).
All deployments were made in the outer part of Horsens Fjord (Figure 1). The line systems were deployed at the Hjarnø Hage seaweed cultivation site (100 ha) belonging to Hjarnø Havbrug A/S. The net cultivation structure was deployed at a nearby mussel cultivation site in As Vig (As Vig mussel cultivation site) (Figure 1) as the existing anchors in the seaweed site were not dimensioned to secure the net structure (see description in section 2.4).
The following infrastructure design systems were tested during this study (Table 1):
1. Vertical cultivation (Figures 2A, B).
Figure 2 Overview of cultivation infrastructure designs used in this study. Line cultivation systems were based on using longlines of a length of 200 m. (A) the longline system seen from above; (B) the vertical line setup; (C) the horizontal 5-line setup; (D) the net cultivation system seen from above; and (E) the net cultivation setup. (A–C) are previously published as part of Boderskov et al. (2021a) and Zhang et al. (2022).
2. Horizontal multi-layer (5-line) cultivation (Figures 2A, C)
3. Net cultivation (Figures 2D, E)
To follow the seasonal variation in growth and to test the ability to harvest the same lines in consecutive seasons (coppicing), cultivation lines were deployed in October/November 2018, February 2019 and October 2019 (Table 1). A vertical cultivation design, where 2 m line sections of a 2 mm seeded line were coiled around a 10 mm carrier line, were deployed from 0-2 m depth. Nylon line (2 mm) was wound onto spools made from gabions of 55 x 7 cm (BIO-BLOK®, EXPO-NET, Denmark) with 50 m of nylon line spool-1 which were soaked and washed in tap water before used for seeding.
The 5-line system was introduced to evaluate the possibilities of increasing the areal yield by positioning multiple horizontal carrier lines above each other, and to evaluate the technique of seeding and growing directly on a 4 mm Polyethylene line. Polyethylene lines (4 mm) were wound onto spools made from gabions of 55 x 7 cm (BIO-BLOK®, EXPO-NET, Denmark), with approximately 20 m of line spool-1, which were soaked and washed in tap water before used for seeding.
The net cultivation trial was made on a single novel net structure developed by Hjarnø Havbrug A/S, being compatible with the tube-net cultivation systems used for cultivation of mussels (Mytilus edulis) (Taylor et al., 2019). The net was 100 m long, 3 m deep, and made of Polyethylene (PE), with a mesh size of 10 cm, a line width of 1.5 cm and with loops at the top every 0.5 m for attachment to a floating 200 mm PE pipe, which carries the net system at sea (Figure 2D).
For all cultivation trials made in this study, sporophytes from Middelfart Old Harbour, Denmark (55°30’33.7’’N, 9°43’12.4E) were used for the production of spores. For all seeding events, a minimum of 10 sporophytes was used for spore extraction. The sporophytes were collected during May – July 2018 and 2019, and artificially induced to produce spores in darkness in an intermediate bulk container (IBC) of 1000 L with up to 50 sporophytes per tank, according to Boderskov et al. (2021b). The spores were released from fertile tissue, and counted in a Thoma hemocytometer counting chamber prior to seeding.
Seawater from Snaptun Harbour was used for all seeding trials. All seeding and hatchery trials were carried out according to the EU legislation regarding organic cultivation of algae (EU, 2018). The organic nutrient source used to achieve a concentration of 100 µM N (ammonium-N) in the water of the hatchery tanks was degassed manure from an organic biogas plant (Kroghsminde Biogas, Ølgod, Denmark).
Due to commercial optimization of the hatchery at Hjarnø Havbrug A/S, the seeding procedures were slightly modified from 2018 to 2019, as described in detail below.
The seeding lines were seeded on 23 August 2018 and 4 July 2019 using traditional spore seeding techniques (Boderskov et al., 2021a). The lines were seeded with spore concentrations in the range of 1,500 - 7,500 spores mL-1. The spools were placed vertically in an IBC container (cut in the middle, and therefore with no top, containing 600 L) in nutrient enriched water with a N concentration around 100 µM ammonium-N, at 10°C and 60 µmol photons m-2 s-1, and with a 50% water exchange after 40-48 days.
Seeded lines in excess after the first deployment, were kept on spools arranged vertically in an identical open IBC container (containing app. 600 L) at Snaptun Harbour, Horsens, Denmark from 5 November 2018 until deployment 7 February 2019. The container was supplied with a semi-continuous flow of seawater, pumped in from 1 m depth in Snaptun Harbour, exchanging the water in periods of 15 min h-1 with a flow rate of app. 200 L min-1.
Due to foam gathering in the top of the container, lines seeded on 4 July 2019 experienced a period of reduced light availability, and therefore the growth of the sporophytes was delayed from this seeding. Despite this minor delay in growth, the resulting quality of sporophytes from this seeding was good.
The seeding lines for the horizontal 5-line system were seeded on 3 and 4 September 2019 in a rectangular tank containing 500 L of seawater and 88 line-wrapped spools. The lines on the spools were seeded on the one side with a spore concentration of app. 850 spores mL-1, by pouring the spores into the water above the spools (200 spores mL-1 for the total water volume). The spools were left for the spores to settle for one day before being rotated 180 degrees and seeded again on the opposite side of the spool with 480 spores mL-1 in the water above the spools (120 spores mL-1 for the total water volume) on the second day. Aeration was started after 4 h, lights after one day (40-45 µmol photons m-2 s-1, 16:8 h light:dark cycle), and water circulation of 2000 L h-1 after 5 days. The N concentration was 55 µM ammonium-N at the start of the seeding period. After 20 days, 50% of the water was renewed with new water containing 45 µM ammonium-N. The spools were manually rotated once every day during the hatchery period to ensure sufficient light for growth on all sides of the spools.
The seaweed net was seeded in an outdoor tank of 6000 L on 7 September 2018, as the net was too large to be accommodated in the hatchery. The tank was 3.5 x 2 x 1 m, and custom made of steel with glass sides. The net was suspended in the tank, hanging from two pipes resting on the top of the tank. The net was seeded with spores from a total of 50 sporophytes. The spore solution (40 L) was added to the tank containing 5000 L of seawater to reach a concentration of 1,933 spores mL-1 in the tank. The tank was connected to a cooler (Aquamedic Titan 1500 Cooler), to keep the water temperature around 10°C. The cooler however, was not of sufficient capacity and consequently the temperature ranged between 12 and 18°C (Figure 3A). To prevent bleaching of the emerging gametophytes, the light intensity was controlled with a shading net during the first five days of the hatchery period. Temperature and PAR loggers (Odyssey®), one of each, were deployed in the glass tank for monitoring of light and temperature. Towards the end of the hatchery period, the amount of incoming light in the tank was reduced considerably due to growth of microalgae on the inner glass surfaces (Figure 3B).
Figure 3 (A) Temperature and (B) light development in the outdoor tank, where the 100 m net was seeded and kept during a nursery period from 7 September 2018 to 15 October 2018. Data are shown as box-plots including the median with no outliers.
Although not quantified, a visual inspection of the net on 2 October 2018, confirmed presence of gametophytes. Only few juvenile sporophytes however were observed on the net prior to deployment.
The lines seeded in 2018 were deployed on 26 October 2018 and 7 February 2019. Upon deployment, the juvenile sporophytes had a size around 0.5 and 5-10 cm, respectively. The lines seeded in 2019 were deployed on 3 October 2019, with the juvenile sporophytes measuring around 0.5 cm upon deployment. Upon deployment, the 2 mm seeded nylon line was wound around carrier lines consisting of 2 m sections of 10 mm polypropylene line. The lines were suspended vertically in the water, attached to a header line in the bottom and a 4 L buoy in the top and positioned at least 2 m apart to prevent shading and tangling of individual vertical lines (Figure 2B). Five vertical 2 m lines were deployed in October 2018, twelve lines in February 2019 and six lines in October 2019 (Table 1).
The horizontal 5-line system was deployed on 16 October 2019, and the juvenile sporophytes had a size of < 0.5 cm upon deployment. The seeded lines were deployed as a horizontal 5-line system with five parallel lines arranged with a 0.5 m vertical distance (Figure 2C). One day before deployment, the lines were prepared by tightening the horizontal lines to vertical connector lines. The vertical connector lines were interspaced with a 10 m distance. Continuously, as the 5-line system was assembled, the system was transferred to a 600 L tank which - after preparation - was filled with seawater and stored at 10°C before deployment on the following day. The whole 5-line system was 200 m long and each horizontal line was made by connecting the lines from the spools. On the following day, the water was drained from the 600 L tank, and the lines were transported, covered with a lid, to the cultivation site. At the cultivation site, the lines were deployed by connecting the vertical connector lines (spaced with 10 meters between each), to a vertical longline (mainline) in the top, and a 10 L concrete weight followed by a 2.5 kg iron weight in the bottom. For every 10 L concrete weight, an 18 L buoy was attached to the longline (Figure 2C). The horizontal 5-line system was attached with a distance of 1 m to the mainline, which was suspended app. 0.5 meters below the surface, so in all, the system was suspended from 1.5 – 3.5 m depth.
The net was transported to the seaweed cultivation site on 15 October 2018, hanging in the cargo of a ship, not submerged in water, but protected from desiccation by covering the net with plastic. The net was deployed by dragging it onto a 115 m long and 200 mm wide PE pipe, fastening the net in each end to a screw anchor, and in the middle to the pipe. On 26 November, the net was moved (dragged) to a final destination at the As Vig mussel cultivation site, approximately 6 km south west of the Hjarnø Hage seaweed cultivation site (Figure 1), in order to prevent damage to the cultivation system, as the anchoring structures at the seaweed site were not dimensioned for carrying the weight of the heavier net system.
The sporophyte length growth on the lines was measured on a routine basis once every second month from November 2018 to May 2019 and again monthly from October 2019 to June 2020. The length and width of the six longest sporophytes was measured, and the density of sporophytes (number of sporophytes 10 cm-1) of each of the five replicate line was registered (regardless of the position on the line). From the deployment in February 2019, only three replicate lines were measured upon each visit. Biomass from the lines deployed in October 2018 was harvested on 28 May 2019 by coppicing the sporophytes approximately 15 cm above the meristem, in order to measure possible regrowth in the following year, where a full harvest was made on 3 June 2020. Biomass from the three lines deployed in February 2019 was harvested on 28 May 2019, 3 October 2019 and 3 June 2020. Biomass from all lines deployed in October 2019 was harvested 3 June 2020. Upon harvest, the linear yield (kg m-1) was recorded and the presence of fouling organisms was registered together with the routine measurements of length, width and sporophyte density.
Once every month, three sections of 1 m of cultivation line was stripped/sampled from the cultivation line in the top (1.5 m depth) as well as from the cultivation line in the bottom (3.5 m depth). Samples were kept cool until the following day, where the following parameters were measured for each sample: Total wet weight per sample, length, width and weight of 12 randomly selected individuals, and a visual inspection of the presence of fouling. The lines were harvested on 28 April 2020, were the measurements were expanded to include all 5 lines in the system.
On 28 May 2019, 3 October 2019 and 3 June 2020, sporophytes from the net were sampled to follow the growth of the biomass. Between 6 June and 29 September 2018, the net was lowered from the surface to 5 - 8 m depth, to prevent the sporophytes from experiencing bleaching and high temperatures during summer. At each sampling event, three biomass samples from the top and three samples from the bottom of the net were collected. Each sample consisted of the biomass from 20 net squares (0.2 m2) and the length, width, wet weight of 25 randomly sampled sporophytes, and the total yield and number of sporophytes per sample was measured the following day.
On 17 June 2020, an extra sampling was made from a larger surface area per sample to verify the biomass density on the net. At this sampling, four samples were taken, each from 50 net squares (0.5 m2) of net surface, and only biomass yields were recorded for these samples.
The DM content of the biomass samples was determined by drying the samples at 105°C until achieving a stable dry weight (DW). The DM was hereafter calculated as % of fresh biomass: DW/FW × 100. Hereafter the dry samples were finely milled and homogenized before further analysis.
For determination of ash content, a known amount of DM was combusted at 550°C for 2 h, and the ash fraction was calculated as % of DM. Tissue concentrations of carbon (C) and nitrogen (N) were determined by Pregl-Dumas ignition in pure oxygen atmosphere followed by chromatographic separation of C and N with detection of the individual elements by thermal conductivity (Marcó et al., 2002). Total phosphorus (P) content was determined spectrophotometrically following standard methods (Grasshoff et al., 1999). Prior to analysis, the dried and homogenized samples were heated at 550°C for 2 h, autoclaved with 2 M hydrogen chloride (HCl) (20 mg DM for 7 mL acid), and finally filtered through GFF filters (Whatman).
Data for all systems were extrapolated to 1000 m of cultivation structure ha-1, equivalent to 10 nets ha-1 (100 m nets spaced 10 m apart) and 5 longlines (mainlines) ha-1 (200 m long lines spaced 10 m apart), as this is the arrangement generaly used at present for net and longline mussel cultivation systems in Denmark (Petersen et al., 2020). Consequently, using the infrastructure design suggested from this study, the extrapolated net cultivation covered; 3 x 1000 m net structure = 3000 m2 of net substrate ha-1, and the horizontal 5-line system; 5 x 1000 m = 5000 m seeded lines ha-1. The C uptake was calculated as CO2 uptake by multiplying the C content by ~3.66 (M weight of CO2/M weight of C).
All data analyses were performed in RStudio, version 1.3.1073 (RStudio Team, 2020). RStudio: Integrated Development for R. RStudio, PBC, Boston, MA URL http://www.rstudio.com/). All datasets were tested for normality using Shapiro Wilks test, and for homoscedasticity using Levene’s test. If the data did not meet the assumptions of normality or homoscedasticity, a log transformation (ln) was applied before analysis. Data meeting the assumptions of normality and homoscedasticity were analysed using a one-way ANOVA (AN) followed by Tukey’s HSD post hoc test (TUKEY). If the assumptions were not met, a Kruskall Wallis (KW) test was used followed by a Wilcoxon (W) test to test for significant differences between groups using the Benjamini Hochberg (BH) adjustment method. A significance level of p < 0.05 was used for all analyses.
The temperature varied from 3 to 20°C, and the salinity from 15 to 29 ppt during the study period. Both temperature and salinity data from the site measurements were similar to the data from the NOVANA monitoring station, confirming that the monitoring station data were representative for the cultivation site (Figures 4A, B). The light extinction coefficient (Kd) varied between 0.2 and 0.5 m-1 during the two cultivation seasons with several peaks during the years (Figure 4C). The Kd measured at the cultivation site was higher than the Kd measured at the monitoring station, and showed a pattern with peaks (indicating high turbidity) in both spring 2019 and spring 2020. In both years, the dissolved inorganic nitrogen, (DIN) concentrations, including nitrate, nitrite and ammonium, peaked from January to February and remained low from March to October (Figure 4D). The measured concentrations of nitrate-N were highest during winter 2019/2020, where the concentrations reached > 10 µM nitrate-N, whereas during winter 2018/2019, only a concentration of < 7 µM nitrate-N was measured. The concentrations of ammonium-N measured at the cultivation site, were generally higher than what was measured at the monitoring station, especially during the winter 2019/2020, where the concentration reached 8.08 µM ammonium-N at the cultivation site as compared to only 1.93 µM ammonium-N at the monitoring station. The concentrations of dissolved inorganic phosphorus (DIP) were generally low during summer, although the DIP concentration increased earlier (August) than the concentration of DIN (Figure 4E). The measured orthophosphate-P (DIP) concentrations were generally higher in the season 2019/2020 as compared to the 2018/2019 season, reaching 1.17 and 0.71 µM orthophosphate-P in the respective seasons.
Figure 4 Environmental parameters registered during the full cultivation period (October 2018 – July 2020): (A) Temperature, (B) salinity, (C) light extinction coefficient (Kd), (D) concentration of dissolved inorganic nitrogen (DIN – nitrate+nitrite and ammonium+ammonia-N) and (E) concentration of dissolved inorganic phosphate (DIP – orthophosphate-P). Closed symbols represent monitoring data obtained from the nearby national environmental monitoring station (VEJ0006870, Figure 1) maintained by the Danish National Monitoring Programme (NOVANA). Open symbols represent measurements made at the cultivation site as part of this study. Data are shown as single measurements, and nutrients/salinity/temperature were measured at 1-3 m depth.
There was a significant difference in Saccharina latissima biomass yield between the two sequential cultivation seasons 2018/2019 and 2019/2020 (Table 2), as the yields were significantly higher for all deployed vertical cultivation lines upon harvest in 2020, as compared to the harvest in 2019. Additionally, a deployment in autumn (October 2018) resulted in a significantly higher yield than a deployment in spring (February 2019) (Table 2). Lines deployed in October 2018, which were harvested in May 2019, by coppicing the sporophytes 15 cm above the meristem, gave a yield of 1.47 ± 0.04 kg m -1 in the first year (2019) and a yield of 7.36 ± 0.54 kg m-1 following the second year of growth (2020). If taking into account that the lines deployed in October 2018 were harvested twice over two seasons, the total yield from these lines was 8.83 kg m-1. Upon harvest in 2020, the biomass yield varied from 5.0 ± 0.67 - 7.36 ± 0.54 kg m-1 with the highest yield from the lines deployed in October 2018 and coppiced in October 2019. A new deployment from October 2019 gave a yield of 5.0 ± 0.67 kg m-1, almost two-fold the yield obtained from the same cultivation period the year before. The C content from the biomass on lines deployed in February 2019, was significantly lower, and the N content significantly higher, when harvested in October 2019 as compared to the biomass harvested in May 2019 (Table 2). Although, not significantly different, the N content was three times higher from biomass harvested in 2020 as compared to biomass harvested in 2019. There were no significant differences neither in the DM nor in the P content of the biomass from the different deployments upon harvest in June 2020.
Table 2 The linear biomass yield and tissue content of DM, C, N and P of S. latissima on vertical cultivation lines deployed in October 2018 and February/October 2019.
The maximal average frond length was also obtained in 2020, as the maximal frond length in 2020 was about twice the length obtained in 2019. The maximum frond length in June 2020: 231.2 ± 6.1 cm, was obtained from biomass from the lines deployed in October 2018 and coppiced in May 2019 (Figure 5).
Figure 5 Maximum lengths of S. latissima sporophytes grown on vertical lines deployed in October 2018 (Oct-18), February 2019 (Feb-19) and October 2019 (Oct-19). Quartiles are calculated including the median. The median is marked with a line, the average with a cross and the max/min values with whiskers in top/bottom of each box (n = 18-36). The maximum sporophyte length was measured as the six longest sporophytes on each line (n=18-36). Letters indicate significant differences in length between the different deployments on the final harvest day in June 2020.
During spring 2020, the average sporophyte density was measured as 200 ± 20, 250 ± 30 and 420 ± 34 sporophytes m-1 line from the lines deployed in October 2018, February 2019 and October 2019, respectively.
From November 2019 until harvest in April 2020, the biomass yield and average sporophyte length was significantly larger at 1.5 m depth, than at 3.5 meters depth. In April 2020, the biomass yield and average length was 6.8 ± 0.48 kg m-1 and 72.9 ± 7.3 cm respectively, at 1.5 m depth and 1.75 ± 0.07 kg m-1 and 46.8 ± 5.2 cm respectively, at 3.5 m depth (Figures 6A, B).
Figure 6 (A) Biomass yields and (B) sporophyte length (cm) of S. latissima sporophytes at 1.5 and 3.5 m cultivation depth in the horizontal 5-line system from October 2020 - April 2020. (C) Biomass yields at 1.5-3.5 m depth upon harvest in April in the horizontal 5-line cultivation system. *and letters mark significant differences between depths. In panel A and C, data are shown as average ± SE, n = 3. In panel B, quartiles are calculated including the median, and the median is marked with a line, the average with a cross and the max/min with whiskers in top/bottom of each box (n = 36-74).
In April 2020, the final biomass yields were measured from all consecutive lines, and the yields decreased exponentially as a function of cultivation depth, being significantly different between lines down to three m depth (Figure 6C). The average sporophyte density was 306 ± 29 sporophytes m-1 from lines at 1.5 m depth and 180 ± 23 sporophytes m-1 from lines at 3.5 m depth.
Generally, only minor differences were observed in the biomass composition of biomass from 1.5 and 3.5 m depth (Figures 7A-D). The DM varied between 6.3-9.6% DM of FW, and the C content between 26-32% C of DM, being significantly highest in the biomass from 1.5 m depth, upon harvest in April 2020. The tissue content of N peaked in February 2020 at 3.5 m depth, being 5.98 ± 0.02% N of DM, and the P content in January 2020 at 3.5 m depth, being 0.89% P of DM (n = 1). Thereafter, the N and P contents decreased to the lowest level at harvest in April 2020, being 1.79 ± 0.06% N of DM at 3.5 m depth, and 0.18 ± 0.02% P of DM at 1.5 m depth. Upon harvest in April 2020, the N and P contents were not significantly different between biomass from lines at 1.5 and 3.5 m depth.
Figure 7 Composition of S. latissima from 1.5 and 3.5 m cultivation depth: (A) DM (% of FW), (B) C (% of DM), (C) N (% of DM) and (D) P (% of DM) contents from the horizontal layer cultivation system. * mark significant differences between biomass cultivated at different depths. Data are shown as average ± SE. n = 1 in January at 3.5 meters depth, otherwise n = 3.
The biomass yield on the net system, was significantly higher upon harvest after two years compared to after one year, although the biomass density decreased significantly in the same period (Table 3).
Table 3 The biomass yield, tissue content of DM, N and P of the S. latissima growing on the large cultivation net deployed in October 2018.
In June 2020, two different methods were used for estimating the biomass yield from the net structures, which showed a significantly lower yield when using a larger proportion of the net for biomass determination, than when using a smaller proportion of the net, giving a yield of 10.9 ± 1.7 and 30.4 ± 4.3 kg m-2, respectively. The tissue DM and C contents of S. latissima cultivated on the net, did not vary significantly between sporophytes sampled at different periods of the year. The highest tissue DM content was 15.1 ± 1.1% DM of FW, measured in May 2019 and June 2020. The C content was lowest in October 2019 (30.5 ± 2.5% of DM), and highest in June 2020 (37.1 ± 0.5% C of DM). The N content varied significantly with the highest measured N content in October 2019 being 4.2 ± 0.69% N of DM.
The length of the sporophytes on the net increased slowly during the first year of cultivation, but during the second cultivation year, sporophyte lengths were comparable to what was found on the line systems, with an average of 117.2 ± 4.9 cm (Figure 8).
Figure 8 Sporophyte lengths on the 100 m long cultivation net (n=150). Box plots are calculated including the median, the median is marked with a line, the average with a cross and the max/min with whiskers in top/bottom of each box.
In May 2019, when the fronds were coppiced from the vertical lines deployed in 2018, the lines and the biomass was almost free from epiphytes, but speckled with minute colonies of bryozoans (Figures 9A, B). After being left in the water over the summer, in October 2019, when the lines were brought back to the surface again, the sporophytes were covered with several epiphytes such as bryozoans, barnacles, filamentous red algae, starfish and tunicates (Figures 9C, D). Before harvest in April 2020, the S. latissima fronds were again almost clean, however, some epiphytes, especially bryozoans, had not disappeared completely during the growth season. Small sporophytes in particular, which had not regrown to a significant size, were still speckled with bryozoans (Figures 9E, F). In contrast, the S. latissima growing on lines deployed in October 2019, were clean of epiphytes in April 2020 (Figure 9G), but also had small colonies of bryozoans upon harvest in June.
Figure 9 Pictures of the vertical lines deployed in October 2018: (A) Line before coppicing in May 2019, (B) same line just after coppicing in May 2019, (C, D) line upon measurement in October 2019, (E, F) line upon measurement in April 2020. (G) Picture of the vertical lines deployed in October 2019 upon measurement in April 2020.
The sporophytes from lines deployed in the horizontal 5-line system were clean of epiphytes until harvest in April 2020, where only minute colonies of bryozoans of few mm in size were present (Figure 10A). Identical lines, harvested one month later in May (19 May 2020 and 28 May 2020), showed an increase in the size of the bryozoan colonies, and a clear pattern was observed of more bryozoans on sporophytes growing at 3.5 m depth than at 1.5 m depth (Figures 10B, C).
Figure 10 Saccharina latissima biomass from the horizontal 5-line system (A) upon harvest in April (2020), (B) on similar lines from 1.5 m depth harvested later in May (2020), and (C) similar lines from 3.5 m depth, also harvested in May (2020). Arrows mark colonies of bryozoans.
The S. latissima growing on the net cultivation system was pale upon measurements in May 2018, however with an allmost clean biomass, and only minute bryozoan colonies as observed on the vertical lines (Figure 11A). Upon the final measurement of the biomass on the net in June 2020, the biomass had regained color and appeared clean, but had colonies of both bryozoans, barnacles, and single individuals of blue mussels (Figures 11B-D). It did however, appear as if the sporophytes on the net had less epiphytes than the sporophytes on the line cultivation systems. Underneath the seaweed, mussels were growing on the net surface, and the density of sporophytes on the net appeared lower than what could be possible (Figure 11D).
Figure 11 Biomass S. latissima on the net system (A) in May 2019, (B) June 2020, and (C, D) a close up of the biomass on the net in June 2019.
From the biomass yields and tissue contents obtained, the area specific biomass yield, and nutrient and carbon extraction potentials were calculated for the different infrastructure design systems used in this study (Table 4).
Table 4 Extrapolated biomass yields (t FW ha-1) and nutrient and carbon extraction potentials (kg N, P and t C ha-1) from the three infrastructure design systems, with the biomass stocking density in the farm taken into account.
The potential biomass yield and the N and P extraction potential (kg ha-1) was generally highest for the net cultivation system, even though only small sporophytes were obtained during the first season, and the sporophyte density was low. The estimated biomass yield per ha obtained after 1.5 year, from the net structures was 32.6 – 91.3 t FW ha-1 as compared to an estimated 21.4 t FW ha-1, obtained after 7 month of cultivation, from the horizontal 5-line system. As the vertical line systems had a low stocking density, as compared to the net system, an areal yield of only 7.4 t ha-1 after 1.5 years cultivation was achieved, from the lines deployed in October 2018, even though a high linear yield m-1 was obtained from these line systems.
The N extraction potential was markedly highest on the cultivation nets after 1.5 years. From the net system, the N extraction potential varied from 39.3 to 110.5 kg N ha-1, and the P extraction potential from 4.7 – 13.1 kg P ha-1. The N extraction potential of the net system increased from 3.9 to 44.7 kg N ha-1 from May to October 2019, primarily due to an increase in N content of the biomass and potentially also biofouling of the biomass. The N and P extraction potential from the horizontal 5-line system was 34.8 kg N ha-1 and 3.3 kg P ha-1 from a clean biomass harvested in late April 2020, after 7 months of cultivation, which was the highest nutrient extraction potential measured from the line systems after one season. Notably, the N and P extraction potential was 33.4 kg N ha-1 and 3.2 kg P ha-1 in March, being allmost the same as in April despite an increase in biomass from 10.4 t ha-1 in March to 21.4 t ha-1 in April.
The C extraction potential was also highest on the net structures, and varied between 1.8 – 5.1 t C ha-1 in June after 1.5 years of growth, driven by a combination of high yield and a high carbon content of this biomass. The highest C extraction potential from the lines was 0.5 t C ha-1 from the horizontal 5-line cultivation system, after 7 months of cultivation. This was only marginally higher than what was measured from the vertical cultivation lines, due to a consistently low DM and C content in this biomass.
Biomass yields from the net cultivation system resulted in an estimated areal yield of 39.5 – 110.5 t FW ha-1 following a 1.5 year cultivation cycle, which is 1 - 2.75 times higher than the previously extrapolated highest areal yield reported from Europe (40 t FW ha-1) (Peteiro and Freire, 2013b). This confirms that net cultivation designs hold a considerable potential for increasing the areal biomass yields of S. latissima. The low yield and poor growth of S. latissima on the net during the first cultivation season, possibly resulted from a suboptimal seeding, and future studies would need to address seeding of large net structures to achieve the full production potential of this type of infrastructure design.
As an alternative to the net structure, the horizontal 5-line system gave a harvest potential of 21.3 t FW ha-1, following 7 months of cultivation, which is lower than from the net structure, but 3 times higher than areal yields previously reported for Danish waters (7 t FW ha-1) (Marinho et al., 2015). A significant effect on the biomass yield of the cultivation depth was observed in the 5-line cultivation system, as the lines at 3.5 m depth had a final yield of only 26% of the yield achieved on the lines on 1.5 m depth. Most likely a combination of turbidity of the water and self-shading resulted in the reduction in biomass yield on the deeper lines. In less turbid marine waters i.e. at the Faroe Islands, where the light attenuation coefficient is generally lower than in Danish waters (Lund-Hansen et al., 2009), the biomass yield at 3.5 m only decreases to 88% of the yield on 1.5 m using a vertical cultivation line (Bak et al., 2018). In this study, the horizontal 5-line system was suspended 1.5 m below the water surface to avoid tangling with the mainline. In future setups, cultivation lines should be positioned higher in the water column, optimally placed in a close horizontal layer e.g. like the cultivation design using catenary lines, recently described by Kite-Powell et al. (2022).
Major differences in yields were seen between the two seasons, as the yield obtained on the vertical lines during the second cultivation season (2019-2020) was 3-5 times higher than during the first cultivation season (2018-2019) using the same cultivation setup. Several factors may contribute to explaining the differences including differences in important environmental factors - ambient nitrogen concentrations, seawater temperature and salinity: During the second cultivation season (2019-2020), the ambient N concentrations were significantly higher during spring than in the first season, resulting in a tissue N-content of 1.22 ± 0.27% N of DM at harvest in June for algae deployed in October 2019, which was ~2 times higher than the year before for algae also deployed in October and harvested in May. As the growth rate of kelps is known to decrease, when the intercellular N content is below 1.5% N of DM, the N availability could have been a factor driving the differences in yield between the two seasons (Jevne et al., 2020). Another explanatory factor, could be that the sea temperature fluctuations were more modest in the season of 2019-2020, with temperatures constantly above 5°C, and the growth rate of S. latissima is known to decrease with temperature decreasing below 5°C (Bolton and Lüning, 1982). Salinity is another important driving factor affecting growth of S. latissima (Spurkland and Iken, 2011). As inner Danish waters are part of the estuary formed by the transition zone between the Baltic Sea and the North Sea, major temporal and spatial variations in salinity from >30 ppt in the Skagerrak region to <10 ppt in the Baltic Sea area are implicit for the region. Broch et al. (2019) describes the effect of salinity on growth of S. latissima in a step-wise function, where salinities <25 ppt result in increasingly impaired growth of S. latissima. During the cultivation trials in this study, the salinity varied between 15 – 29 ppt, meaning that the salinity could have been restricting growth, and explanatory for the 50 – 70% lower yield found in this study, as compared to yields documented from Spain, Scotland and Norway, where higher salinities prevail (Peteiro and Freire, 2013b; Forbord et al., 2020; Kerrison et al., 2020). Since salinity did not differ markedly between the two seasons however, salinity was not expected to contribute to the differences in yield between the two seasons. Since this study is based on commercial large-scale cultivation trials, it was not possible to harmonize all variables in the cultivation designs between years. Thus, in addition to the environmental factors, other intrinsic factors could have contributed to the observed differences in yields, including differences in seeding density between seasons, slight differences in deployment schedules between years and differences in local hydrodynamics – again potentially affected by the different cultivation designs. Including these complex interactions in the statistical analyses was not possible. Since seeding method and environmental factors are well correlated with final biomass yields as compared to seeding density and minor differences in deployment schedules (Forbord et al., 2020; Boderskov et al., 2021a), we expect the impact of seeding densities and deployment schedules to be of minor importance.
Another way of achieving higher yields, or reducing costs associated with deployment, is coppicing of the algae above the meristem at harvest, giving the possibility to obtain multiple harvests from the same lines, as S. latissima is able to regrow to full size following coppicing (Parke, 1948). Coppicing has previously been shown to improve both yields and economy in the cultivation of S. latissima, giving the possibility to harvest twice during a year with only one seeding (Bak et al., 2018; Skjermo et al., 2021). In the present study after coppicing, the sporophytes needed a whole season of regrowth before being ready to harvest, as they did not grow during summer after coppicing, in contrast to what is reported from the Faroe Islands. In Faroese waters the constant temperatures and salinities are optimal for the growth of S. latissima, and in contrast to conditions in inner Danish waters, also a constantly higher nitrate concentration during summer supports the growth during summer (Steingrund and Gaard, 2005; Mortensen et al., 2017; Bak et al., 2019). In this study, the biomass harvested in the year following coppicing was almost clean, but still had minute colonies of epiphytes, which could compromise the use of the biomass for consumption.
When using the biomass for downstream processing and sales for food, feed or other purposes, it is important that the biomass is clean and free of epiphytes (Lüning and Mortensen, 2015). As seen previously in the Limfjorden, Denmark, and in this study, the biofouling from bryozoans can start as early as April in inner Danish waters, thereby restricting the growth period at sea and reducing the potential biomass yield (Bruhn et al., 2016). The early onset of fouling in Denmark is earlier than what has been described from Norway, where biofouling typically onsets in June (Handå et al., 2013; Matsson et al., 2019). Although not quantitatively documented, there was a clear indication from this study that the biofouling pressure was highest on the deepest horizontal lines at 3.5 m depth. This may be explained by differences in exposure, as the deep lines were less exposed to waves than the lines in the top – and exposure is documented to be inversely correlated to biofouling (Peteiro and Freire, 2013a; Matsson et al., 2019; Visch et al., 2020). Therefore, future cultivation practices in Denmark could focus on having lines exposed to waves, as this could potentially reduce the biofouling pressure on the biomass, and potentially increase yield, as S. latissima grows well in wave exposed conditions (Mortensen et al., 2017). With more exposed cultivation practices however, there will be trade-offs with the risk of loss of biomass and equipment, and potentially with growth.
The highest nutrient extraction potential found in this study of 39.3 - 110.1 kg N ha-1 and 4.7 – 13.1 kg P ha-1 from the net structure, and 34.8 kg N ha-1 in March from the horizontal 5-line system, was in the high range of what has previously been reported from Europe/western countries: 3 – 46 kg N ha-1 (Grebe et al., 2021). In China, where the currently largest production of kelp is taking place, production estimates support an up to ten-fold higher nutrient extraction potential of 1.20 t N and 0.17 t P ha-1 y-1 extracted from marine waters by producing kelps, demonstrating the large nutrient extraction potential for the production under optimized conditions (Zheng et al., 2019). Current nutrient emission reduction goals for Danish marine waters under the EU Water Framework Directive are 13,100 t N y-1 towards 2027 (Miljø og landbrugsministeriet, 2021), which therefore would correspond to ~10,917 ha of seaweed production, assuming Chinese production standards, if the entire N reduction should be accomplished through seaweed cultivation. Assuming the nutrient extraction potential from this study, the area needed for seaweed cultivation would need to be ten-fold larger. The nutrient extraction potential from cultivation of mussels in Danish waters, using the same infrastructure design as used for the net cultivation trial in this study, has been reported to be 1.63 – 3.01 t N ha-1 and 0.1 – 0.17 t P ha-1 (Taylor et al., 2019), which is significantly higher than what is found using the same net system for cultivation of S. latissima in this study.
In order to optimize the nutrient extraction of the production, it is important to find the optimal time for harvest, where ideally both tissue nutrient contents and biomass yields are maximal. The tissue N and P contents of S. latissima followed the general pattern known for S. latissima, as internal nutrient concentrations were high during winter and low during summer (Black, 1950; Nielsen et al., 2014; Marinho et al., 2015). The N and P extraction potential was almost equal in March and April on the horizontal 5-line system even though the biomass doubled. Therefore, in the cultivation season of 2019-2020, there was a proportional decrease in N and P concentration as a function of the biomass increase, emphasizing that the N and P-extraction potentials of the production can be the same if harvesting in March or April. If harvesting for N and P extraction or if using the biomass for protein production, the March harvest could be preferred, as only half of the biomass volume should be handled to achieve the same N or protein yield. However, if a biomass with biofouling can be handled in the downstream processing of the biomass, our results suggest that leaving the biomass in the water through summer into autumn, would optimize the nutrient extraction, as the biomass yield increases during summer, and the nutrient content of the biomass is increased, possibly due to the tissue of the fouling organisms also accumulating nutrients (Marinho et al., 2015). The maximal N content documented during this study was 5.98 ± 0.02% N of DM in February, which is higher than what has previously been reported for S. latissima from Kattegat, Denmark (Nielsen et al., 2014), but in line with what has been found from other – more eutrophic locations, such as the Limfjorden, Denmark characterized by having high ambient N concentrations (Bruhn et al., 2016). The high N concentrations of the biomass most likely reflected the relatively high concentrations of nitrate in the water during the season 2019–2020 (Manns et al., 2017).
As the growth of macroalgae, such as S. latissima, take up CO2, during the production, another goal for the production could be to optimize the CO2 uptake of the production. This study found C extraction potentials of up to 5.1 t C ha-1 following a 1.5 year production cycle on the net structure, and a C extraction potential of up to 0.5 t C ha-1 from the horizontal 5-line system after 7 month of cultivation, and therefore the maximal CO2 extraction potential found in this study was 18.7 t CO2 ha-1 following a 1.5 year production cycle on the net structure. This is in line with the reported average CO2 extraction of seaweed production, which is 1.5 t CO2 ha-1 y-1 (Duarte et al., 2017). In perspective, current global CO2 emissions are 6.65 t CO2 per capita y-1 (https://www.worldometers.info/co2-emissions/co2-emissions-per-capita/), corresponding to 0.4 ha of S. latissima cultivation in 1.5 years for extracting and making available for recycling the CO2 emissions per capita, if using the highest CO2 extraction estimate found in this study. The highest tissue C content was found upon harvest in May/June in this study, however, leaving the biomass longer in the water, into summer, could potentially optimize both yield and C content, but would again compromise the biomass quality, as biofouling would increase. The Chinese production of Laminaria sp. is the largest in the world, and in 2015, the production of Laminaria sp. in China extracted a total of 374,626.67 t C from the atmosphere, corresponding to 137.11 kt CO2 y-1 (Zheng et al., 2019).
When considering the use of S. latissima cultivation as a climate change mitigation tool, there are several aspects to take into account (Hurd et al., 2022). As mentioned in the introduction, the downstream use of the biomass will determine how fast the carbon will reenter the atmosphere as CO2. Moreover, as species such as S. latissima loose a significant part of the structural tissue through erosion as particulate organic carbon (POC), loss of dissolved organic carbon (DOC) (Parke, 1948; Abdullah and Fredriksen, 2004) and volatile organic C (VOC) (Tokarek et al., 2019), the total C uptake of the production is higher, than what is accounted for in the harvested biomass, as the DOC, POC and VOC is not included. The DOC and POC may enter the marine food web by bacterial uptake or animal degradation, and an unknown fraction of this is expected to be buried in sediments or transported to the deep sea, and hence potentially sequestered from the atmosphere (Azam et al., 1983; Duarte and Cebrián, 1996; Krause-Jensen and Duarte, 2016). The climate impact of the various VOCs is not yet fully understood (Tokarek et al., 2019). Also the C invested in the production and processing of the seaweed needs to be accounted for, and hence a full life cycle analysis approach is necessary for estimating the true carbon footprint of S. latissima cultivation (Zhang et al., 2022).
As seen in this study, the use of cultivation infrastructure systems with a high density of substrate in the uppermost part of the water column, increases both yield, nutrient extraction and carbon uptake potentials of kelp cultivation. Therefore, systems with a high line density placed in close proximity to the surface, would be beneficial for future upscaling scenarios. There are however, significant barriers to overcome regarding the seeding and harvesting techniques to apply to multi-layer line systems, or net cultivation systems. The seeding techniques used in the present study, constitute a clear barrier for the efficient cultivation of S. latissima on net structures, as the initial development of the sporophytes was not sufficiently controlled. Therefore, development of seeding methods targeting large-scale structures, are evidently needed to make this production feasible both considering the direct seeding techniques and the storage/nursery of the large structures. Harvesting machines have been developed for mussel farming on nets, which could be of advantage, if these could be modified to accommodate seaweed harvest from the same net structures. The horizontal 5-line system presented in this paper, presents one solution of coupling traditional seeding techniques to generate larger 2D structures, enhancing the potential areal yield.
As seen in this, and previous studies, there is a possibility to increase tissue nitrogen concentrations, and thereby nutrient extraction potentials, significantly, if postponing harvest from late spring until autumn, but at the same time this involves a risk of compromising the biomass quality because of biofouling (Marinho et al., 2015). The second year harvest carried out as part of this study, was consistently biofouled, compromising the market value of the biomass. Perhaps future scenarios could make use of various qualities of seaweed biomass, where the first year biomass could be harvested for consumption, and the latter harvests for lower grade purposes. However, there is a need to develop the market and biorefinery processing if the use of lower quality biomasses should result in a positive business case (Araújo et al., 2021). For inner Danish waters in particular, future climate change could improve growth conditions for the growth of S. latissima through winter, as the combination of increased nutrient run-off from land due to increased precipitation, and elevated sea temperatures, could favor the production of S. latissima, as seen in this study.
During the best cultivation season, the biomass yield of S. latissima, was comparable to what is reported from other cultivation areas in Europe, with biomass yields of 5.0 – 6.8 kg m-1 cultivation line year-1. The biomass yields however, varied with a factor of four between two consecutive years, possibly driven by differences in nitrate concentrations and water temperature between years. A net cultivation system resulted in higher areal yields than all line systems tested, but further improvements of seeding and harvest technology are needed for the net system to be fully implemented for up-scaling. Multiple harvests could be applied to the same seeded lines over two consecutive years, potentially minimizing costs and resources for seeding, but the biomass quality of the second year harvest was compromised due to epiphytes. The highest nutrient extraction and carbon uptake potentials were achieved from the net cultivation systems driven by the high biomass yield. Harvesting in autumn implies a trade-off between nutrient extraction potential and biomass quality, as autumn harvest potentially increases nutrient extraction potentials, but biofouling compromises the autumn biomass quality. Future cultivation infrastructure designs should focus on near surface cultivation systems with a high substrate density, such as net systems, to maximize areal biomass yields, nutrient extraction and carbon uptake potentials.
The raw data supporting the conclusions of this article will be made available by the authors, without undue reservation.
TB, AB, and MR contributed to conception and design of the study. TB and MR collected data. TB and AB analyzed the data. TB made the statistical analysis. TB wrote the first draft. AB wrote sections of the manuscript. All authors contributed to the article and approved the submitted version.
The work was supported by the Innovation Fund Denmark (Grant no. 7038-00133B) and Hjarnø Havbrug A/S, through the commercial PhD project “Organic Sugar kelp – industrial production of a new Danish bioresource”,the VELUX FOUNDATION through the Tang.nu project (Grant no. 13744), and The Danish Agricultural Agency through the GUDP project, SMART TANG (Grant no. 34009-21-1963).
We thank Kitte Linding Gerlich for analysing samples for C, N and P contents, and Tinna Christensen for creating the final version of the figures.
Author TB was employed by the company Hjarnø Havbrug A/S. The remaining authors declare that the research was conducted in the absence of any commercial or financial relationships that could be construed as a potential conflict of interest.
The authors declare that this study received funding from Hjarnø Havbrug A/S. The funder was not involved in the study design, collection, analysis, interpretation of data, the writing of this article or the decision to submit it for publication.
All claims expressed in this article are solely those of the authors and do not necessarily represent those of their affiliated organizations, or those of the publisher, the editors and the reviewers. Any product that may be evaluated in this article, or claim that may be made by its manufacturer, is not guaranteed or endorsed by the publisher.
Abdullah M. I., Fredriksen S. (2004). Production, respiration and exudation of dissolved organic matter by the kelp Laminaria hyperborea along the west coast of Norway. J. Mar. Biol. Assoc. United Kingdom 84, 887–894. doi: 10.1017/S002531540401015Xh
Araújo R., Vázquez C. F., Sánchez L. J., Azevedo I. C., Bruhn A., Fluch S., et al. (2021). Current status of the algae production industry in Europe: An emerging sector of the blue bioeconomy. Front. Mar. Sci. 7. doi: 10.3389/fmars.2020.626389
Azam F., Fenchel T., Field J. G., Gray J. S., Mayer-Reil L. A., Thingstad F. (1983). The ecological role of water-column microbes in the Sea. Mar. Ecol. Prog. Ser. 10, 257–263. doi: 10.3354/meps010257
Bak U. G., Mols-Mortensen A., Gregersen O. (2018). Production method and cost of commercial-scale offshore cultivation of kelp in the Faroe Islands using multiple partial harvesting. Algal Res. 33, 36–47. doi: 10.1016/j.algal.2018.05.001
Bak U. G., Nielsen C. W., Marinho G. S., Gregersen Ó, Jónsdóttir R., Holdt S. L. (2019). The seasonal variation in nitrogen, amino acid, protein and nitrogen-to-protein conversion factors of commercially cultivated Faroese Saccharina latissima. Algal Res. 42, 101576. doi: 10.1016/j.algal.2019.101576
Black W. A. P. (1950). The seasonal variation in weight and chemical composition of the common British Laminariacea. Scottish Seaweed Res. Assoc. UK 29, 45–72. doi: 10.1017/S0025315400056186
Boderskov T., Nielsen M. M., Rasmussen M., Balsby T. J. S., Macleod A., Holdt S. L., et al. (2021a). Effects of seeding method, timing and site selection on the production and quality of sugar kelp, Saccharina latissima: a Danish case study. Algal Res. 53. doi: 10.1016/j.algal.2020.102160
Boderskov T., Rasmussen M. B. B., Bruhn A. (2021b). Obtaining spores for the production of Saccharina latissima: Seasonal limitations in nature, and induction of sporogenesis in darkness. J. Appl. Phycol 33, 1035-1046. doi: 10.1007/s10811-020-02357-0
Bolton J. J., Lüning K. (1982). Optimal growth and maximal survival temperatures of Atlantic Laminaria species (Phaeophyta) in culture. Mar. Biol. 66, 89–94. doi: 10.1007/BF00397259
Broch O. J., Alver M. O., Bekkby T., Gundersen H., Forbord S., Handå A., et al. (2019). The kelp cultivation potential in coastal and offshore regions of Norway 5, 529. doi: 10.3389/fmars.2018.00529
Bruhn A., Tørring D. B., Thomsen M., Canal-Vergés P., Nielsen M. M., Rasmussen M. B., et al. (2016). Impact of environmental conditions on biomass yield, quality, and bio-mitigation capacity of Saccharina latissima. Aquac Environ. Interact. 8, 619–636. doi: 10.3354/aei00200
Chopin T., Tacon A. G. J. (2020). Importance of seaweeds and extractive species in global aquaculture production. Rev. Fish Sci. Aquac 0, 1–10. doi: 10.1080/23308249.2020.1810626
Chung I. K., Beardall J., Mehta S., Sahoo D., Stojkovic S. (2011). Using marine macroalgae for carbon sequestration: A critical appraisal. J. Appl. Phycol 23, 877–886. doi: 10.1007/s10811-010-9604-9
EU. (2018). Regulation (EU) 2018/848 of the European parliament and of the council of 30 may 2018 on organic production and labelling of organic products and repealing council regulaiton (EC) no 834/2007. Off J. Eur. Union, 1–92.
Duarte C. M., Bruhn A., Krause-Jensen D. (2022). A seaweed aquaculture imperative to meet global sustainability targets. Nat. Sustain 5, 185–193. doi: 10.1038/s41893-021-00773-9
Duarte C. M., Cebrián J. (1996). The fate of marine autotrophic production. Limnol Oceanogr 41, 1758–1766. doi: 10.4319/lo.1996.41.8.1758
Duarte C. M., Wu J., Xiao X., Bruhn A., Krause-Jensen D. (2017). Can seaweed farming play a role in climate change mitigation and adaptation? Front. Mar. Sci. 4. doi: 10.3389/fmars.2017.00100
European commision. (2022) Communication from the commission to the European parliament, the council, the European economic and social committee and the committee of the regions. Available at: https://eur-lex.europa.eu/legal-content.
EU. (2000). “(Directive 2000/60/EC of the European parliament and of the council of 23 October 2000 establishing a framework for community action in the field of water policy),” in EU Water framework directive, vol. 32722122000 . , 1–73. Available at: https://eur-lex.europa.eu/legal-content/EN/ALL/?uri=celex%3A32000L0060.
Forbord S., Steinhovden K. B., Solvang T., Handå A., Skjermo J. (2020). Effect of seeding methods and hatchery periods on sea cultivation of Saccharina latissima (Phaeophyceae): a Norwegian case study. J. Appl. Phycol 32, 2201–2212. doi: 10.1007/s10811-019-01936-0
Grasshoff K., Ehrhardt M., Kramling K. (1999). Methods of sea-water analysis (Weinheim: Verlag Chemie).
Grebe G. S., Byron C. J., Brady D. C., Geisser A., Brennan K. (2021). The nitrogen bioextraction potential of nearshore Saccharina latissima cultivation and harvest in the Western gulf of Maine. J. Appl. Phycol 33(3):1741-1757. doi: 10.1007/s10811-021-02367-6
Handå A., Forbord S., Wang X., Broch O. J., Dahle S. W., Størseth T. R., et al. (2013). Seasonal- and depth-dependent growth of cultivated kelp (Saccharina latissima) in close proximity to salmon (Salmo salar) aquaculture in Norway. Aquaculture 414–415, 191–201. doi: 10.1016/j.aquaculture.2013.08.006
Hansen J. W., Høgslund S. (2021). Marine områder 2019 (DCE – Nationalt Center for Miljø og Energi: NOVANA. Aarhus Universitet). 174 s. - Videnskabelig rapport fra DCE nr. 418. http://dce2.au.dk/pub/SR418.pdf
Hurd C. L., Law C. S., Bach L. T., Britton D., Hovenden M., Paine E. R., et al. (2022). Forensic carbon accounting: Assessing the role of seaweeds for carbon sequestration. J. Phycol. 58, 347–363. doi: 10.1111/jpy.13249
Jevne L. S., Forbord S., Olsen Y. (2020). The effect of nutrient availability and light conditions on the growth and intracellular nitrogen components of land-based cultivated Saccharina latissima (Phaeophyta). Front. Mar. Sci. 7. doi: 10.3389/fmars.2020.557460
Kerrison P. D., Innes M., Macleod A., McCormick E., Elbourne P. D., Stanley M. S., et al. (2020). Comparing the effectiveness of twine- and binder-seeding in the laminariales species Alaria esculenta and Saccharina latissima. J. Appl. Phycol 32, 2173–2181. doi: 10.1007/s10811-020-02069-5
Kite-Powell H., Erick A., Augyte S., Bailey D., Decker J., Goudey C., et al. (2022). Estimating production cost for large-scale seaweed farms. Appl. Phycol 3, 435–445. doi: 10.1080/26388081.2022.2111271
Krause-Jensen D., Duarte C. M. (2016). Substantial role of macroalgae in marine carbon sequestration. Nat. Geosci 9, 737–742. doi: 10.1038/ngeo2790
Lüning K., Mortensen L. (2015). European Aquaculture of sugar kelp (Saccharina latissima) for food industries: iodine content and epiphytic animals as major problems. Bot. Mar. 58, 449–455. doi: 10.1515/bot-2015-0036
Lund-Hansen L. C., Gaard E., Debes H. (2009). Optical properties and optical constituents of the Faroe Islands shelf and off-shelf waters, north East Atlantic. Hydrobiologia 636 (1), 285–293. doi: 10.1007/s10750-009-9957-8
Maberly S. C. (1990). Exogenous sources of inorganic carbon for photosynthesis by marine macroalgae. J. Phycol 26, 439–449. doi: 10.1111/j.0022-3646.1990.00439.x
Manns D., Nielsen M. M., Bruhn A., Saake B., Meyer A. S. (2017). Compositional variations of brown seaweeds Laminaria digitata and Saccharina latissima in Danish waters. J. Appl. Phycol 29, 1493–1506. doi: 10.1007/s10811-017-1056-z
Marcó A., Rubio R., Compañó R., Casals I. (2002). Comparison of the Kjeldahl method and a combustion method for total nitrogen determination in animal feed. Talanta 57, 1019–1026. doi: 10.1016/S0039-9140(02)00136-4
Marinho G. S., Holdt S. L., Birkeland M. J., Angelidaki I. (2015). Commercial cultivation and bioremediation potential of sugar kelp, Saccharina latissima, in Danish waters. J. Appl. Phycol 27, 1963–1973. doi: 10.1007/s10811-014-0519-8
Matsson S., Christie H., Fieler R. (2019). Variation in biomass and biofouling of kelp, Saccharina latissima, cultivated in the Arctic, Norway. Aquaculture 506, 445–452. doi: 10.1016/j.aquaculture.2019.03.068
Miljø og landbrugsministeriet (2021). Miljørapport over målrettet kvælstofregulering 2021 (Denmark: Landbrugsstyrelsen, Nyropsgade 30, 1780 København V).
Mortensen M. M., Ortind E. G., Jacobsen C., Holdt S. L. (2017). Variation in growth, yield and protein concentration in Saccharina latissima (Laminariales, phaeophyceae) cultivated with different wave and current exposure in the Faroe Islands. J. Appl. Phycol 29, 2277–2286. doi: 10.1007/s10811-017-1169-4
Nielsen M. M., Krause-Jensen D., Olesen B., Thinggaard R., Christensen P. B., Bruhn A. (2014). Growth dynamics of Saccharina latissima (Laminariales, phaeophyceae) in Aarhus Bay, Denmark, and along the species’ distribution range. Mar. Biol. 161, 2011–2022. doi: 10.1007/s00227-014-2482-y
Paine E. R., Schmid M., Boyd P. W., Diaz-Pulido G., Hurd C. L. (2021). Rate and fate of dissolved organic carbon release by seaweeds: A missing link in the coastal ocean carbon cycle. J. Phycol 57, 1375–1391. doi: 10.1111/jpy.13198
Parke M. (1948). Studies on British Laminariaceae. Mar. Biol. Assoc. 27, 651–709. doi: 10.1017/S0025315400056071
Peteiro C., Freire O. (2013a). Epiphytism on blades of the edible kelps Undaria pinnatifida and Saccharina latissima farmed under different abiotic conditions. J. World Aquac Soc. 44, 706–715. doi: 10.1111/jwas.12065
Peteiro C., Freire Ó (2013b). Biomass yield and morphological features of the seaweed Saccharina latissima cultivated at two different sites in a coastal bay in the Atlantic coast of Spain. J. Appl. Phycol 25, 205–213. doi: 10.1007/s10811-012-9854-9
Petersen J. K., Taylor D., Bergström P., Buer A.-L., Darecki M., Filippelli R., et al. (2020). Policy guidelines for implementation of mussel cultivation as a mitigation measure for coastal eutrophication in the Western Baltic Sea (DTU Aqua). DTU Aqua-rapport No. 362-2020. doi: 10.11581/dtu:00000079. https://backend.orbit.dtu.dk/ws/portalfiles/portal/210255337/optimus_policy_dtuaqua.pdf
RStudio Team (2020). RStudio: Integrated development for r (Boston, MA: RStudio, PBC). Available at: http://www.rstudio.com/.
Skjermo J., Broch O. J., Forbord S., Sarno A., Handå A., Lona A., et al. (2021) GENIALG: Report on yield optimisation including strain selection, coppicing and IMTA. Available at: https://genialgproject.eu/wp-content/uploads/2021/07/D3.2-Deliverable-Report_Yield-Optimisation-Report.pdf.
Spurkland T., Iken K. (2011). Salinity and irradiance effects on growth and maximum photosynthetic quantum yield in subarctic Saccharina latissima (Laminariales, laminariaceae). Bot. Mar. 54, 355–365. doi: 10.1515/bot.2011.042
Steingrund P., Gaard E. (2005). Relationship between phytoplankton production and cod production on the Faroe Shelf. ICES J. Mar. Sci. 62, 163–176. doi: 10.1016/j.icesjms.2004.08.019
Taylor D., Saurel C., Nielsen P., Petersen J. K. (2019). Production characteristics and optimization of mitigation mussel culture. Front. Mar. Sci. 6. doi: 10.3389/fmars.2019.00698
Tokarek T. W., Brownsey D. K., Jordan N., Garner N. M., Ye C. Z., Osthoff H. D. (2019). Emissions of C9 – C16 hydrocarbons from kelp species on Vancouver island: Alaria marginata (winged kelp) and Nereocystis luetkeana (bull kelp) as an atmospheric source of limonene. Atmos Environ. 2, 100007. doi: 10.1016/j.aeaoa.2019.100007
Visch W., Nylund G. M., Pavia H. (2020). Growth and biofouling in kelp aquaculture (Saccharina latissima): the effect of location and wave exposure. J. Appl. Phycol 32 (5):3199-3209. doi: 10.1007/s10811-020-02201-5
Zhang X., Boderskov T., Bruhn A., Thomsen M. (2022). Blue growth and bioextraction potentials of Danish Saccharina latissima aquaculture — a model of eco-industrial production systems mitigating marine eutrophication and climate change. Algal Res. 64, 102686. doi: 10.1016/j.algal.2022.102686
Keywords: sugar kelp (Saccharina latissima), production, nitrogen, phosphorous, infrastructure design, biofouling, lines, nets
Citation: Boderskov T, Rasmussen MB and Bruhn A (2023) Upscaling cultivation of Saccharina latissima on net or line systems; comparing biomass yields and nutrient extraction potentials. Front. Mar. Sci. 10:992179. doi: 10.3389/fmars.2023.992179
Received: 12 July 2022; Accepted: 10 January 2023;
Published: 06 February 2023.
Edited by:
Matthias Schmid, Trinity College Dublin, IrelandReviewed by:
Nora Diehl, University of Bremen, GermanyCopyright © 2023 Boderskov, Rasmussen and Bruhn. This is an open-access article distributed under the terms of the Creative Commons Attribution License (CC BY). The use, distribution or reproduction in other forums is permitted, provided the original author(s) and the copyright owner(s) are credited and that the original publication in this journal is cited, in accordance with accepted academic practice. No use, distribution or reproduction is permitted which does not comply with these terms.
*Correspondence: Teis Boderskov, dGVib0BlY29zLmF1LmRr
Disclaimer: All claims expressed in this article are solely those of the authors and do not necessarily represent those of their affiliated organizations, or those of the publisher, the editors and the reviewers. Any product that may be evaluated in this article or claim that may be made by its manufacturer is not guaranteed or endorsed by the publisher.
Research integrity at Frontiers
Learn more about the work of our research integrity team to safeguard the quality of each article we publish.