- IHCantabria - Instituto de Hidráulica Ambiental de la Universidad de Cantabria, Santander, Spain
Widespread generalist species, particularly the non-native invasive ones, are expected to be enhanced by climate change resulting in a biotic homogenization of ecosystems. The red seaweed Asparagopsis armata is a non-native opportunistic species, widely distributed in the European coasts of the North Atlantic, where it has been considered invasive. In this work, we examined the effects of temperature and irradiance on the vegetative propagation process of this species in a laboratory experiment. We considered vegetative propagation due to its implications in the invasion process (as it is considered one of the main sources of recruitment). In gametophytes, the process was characterized through the survival rates of hook-shaped specialized structures and the production and growth of new plantlets from hooks of 1-3cm in length. In tetrasporophytes, the growth and phycobiliprotein contents of previously excised tufts was analyzed. For gametophytes, results revealed how vegetative propagation for this species was conditioned by the low survival rates of hooks once detached from the main thallus. In our experiment, survival probabilities after 30 days of culture were always below 50%. Comparisons among environmental conditions showed lower survival rates under increasing levels of temperature and decreasing levels of irradiance. In fact, mass mortality was detected at 18°C and low irradiance, where almost any hook-shaped fragment survived. Nevertheless, patterns of appearance and growth of plantlets at different temperatures and irradiances were not clear. In the case of tetrasporophytes, only positive growth rates were registered at 15°C and 55-60 µmol/m2/s after 30 days of culture. Higher concentration of phycobiliproteins was detected at higher temperatures during the first days of culture, while not clear patterns were detected at the end. In the light of climate change, understanding these reproduction patterns is necessary in order to adopt better management actions in the future.
1 Introduction
Non-indigenous species are considered as a major driver of change for marine biodiversity, affecting the functions and services provided by native species. Macroalgae constitute a significant component of non-indigenous marine species which, in combination with other processes such as eutrophication or habitat degradation, represent a major threat to marine biodiversity (Schaffelke et al., 2006). Understanding the invasion process of those species, their ecology and their impacts is an important challenge for conservation and management of local communities in the context of climate change (Andreakis and Schaffelke, 2012).
The red seaweed Asparagopsis armata Harvey, 1855 is a clear example of non-indigenous seaweed in the Atlantic European coasts. Being endemic to the Indo-Pacific region, in the Southern Hemisphere (Dijoux et al., 2014), this species was firstly described outside its natural boundaries in France in 1923 (Svedelius, 1933). Currently, A. armata is spread in the north-eastern Atlantic from the British Isles to Senegal, including Canary Islands, Azores and the Mediterranean Sea (Guiry and Dawes, 1992). Marine transport through the Suez Canal seemed the most probable vector of introduction, though no direct evidences are available (Zanolla et al., 2022). In non-native locations, negative effects of this species on the abundance and taxonomic composition of native seaweed communities and their epifaunal assemblages have been reported (Rubal et al., 2018; Silva et al., 2021).
Life history of A. armata consisted of a triphasic life cycle with a gametophytic and a tetrasporophytic phase morphologically distinct (Feldmann and Feldmann, 1942). In fact, both phases were previously recognized as two different species, the tetrasporophyte being named Falkenbergia rufolanosa and the gametophyte being named A. armata. Phase alternation has been shown to be temperature, nutrient and photoperiod dependent. Indeed, tetrasporogenesis occurs within a narrow range of daylength and temperature conditions, which explains why sexual reproduction is limited in areas close to its biogeographical limits (Bonin and Hawkes, 1987; Guiry and Dawes, 1992; Zanolla et al., 2022). The absence of sexual reproduction indicators in such places and the observed differences in the distribution patterns of gametophytes and tetrasporophytes led to the hypothesis that both phases can reproduce independently through vegetative mechanisms (Dixon, 1965). For example, A. armata gametophytes produce specialized vegetative structures (morphologically similar to hooks) with the capacity to attach to new surfaces and to regenerate into new individuals once dislodged from the original thalli (Codomier et al., 1979). On the other hand, tetrasporophytes can also propagate through vegetative means by breaking their filamentous aggregations (Chihara, 1960).
Vegetative propagation is, actually, an important reproductive mechanism for many seaweeds within the Bonemaisoniales order (e.g. Asparagopsis taxiformis or Bonnemaisonia hamifera) (Breeman et al., 1988; Zanolla et al., 2018b) and others (e.g. Gelidium corneum, Gracilaria sp.) (Santelices and Doty, 1989; Juanes and Puente, 1993), being in some cases the main form of population maintenance. Environmental variables such as temperature, nutrients, photoperiod or irradiance may influence this process. The response to these variables seems to be different for each phase and may help to explain why gametophytes are characterized by seasonal patterns in some regions while tetrasporophytes can be found in the field all year round (Zanolla et al., 2022). However, this effects on the vegetative propagation process are still scarcely understood. For A. armata gametophytes, only the effects of medium composition (i.e. nutrients) (Codomier et al., 1981; Haslin and Pellegrini, 2001) and the type of substrata haven been explored (Wright et al., 2022). These studies showed how the production and growth of vegetative structures (plantlets) was promoted by substances such us iron sulfate and potassium bromide. Those plantlets are the result from the development of hook-like structures’ apical cells, opposite to the spines (Haslin and Pellegrini, 2001). On the other hand, mussel ropes were the preferred substrata to attach from the different materials and structures tested (polypropylene ropes and nylon mono and multifilament nets). More research has been done for tetrasporophytes, being temperature, nutrients and culture density among the most explored factors (e.g. Ní Chualáin et al., 2004; Mata et al., 2006; Mata et al., 2007; Zanolla et al., 2014).
Given that climate change is modifying the current ranges of those environmental variables, it is necessary to understand how key processes (e.g. vegetative propagation) will be affected by those changes in order to predict future impacts and adopt effective monitoring and management strategies. Indeed, predictive models on A. armata have already anticipated distributional changes for this species with contractions on the Atlantic coasts of Europe and shifts in the Mediterranean (Zanolla et al., 2018a). Considering all this information, the objective of the present study is to analyze the interactive effects of temperature and irradiance on the vegetative propagation process of gametophytes and tetrasporophytes of A. armata. Temperature and irradiance conditions were selected individually for each phase according to their ecology. Different response variables were also measured for each life cycle stage. The survival rates of hook-shaped specialized structures and the production of plantlets were analyzed for gametophytes. In the case of tetrasporophytes, the vegetative propagation process was characterized by the growth rates of tufts and the phycobiliprotein content.
2 Materials and methods
2.1 Collection of samples
Individuals of Asparagopsis armata were collected from a lower intertidal zone in the Cantabrian Sea (43°28’14”N, 3°46’33”W). This area is characterized by semi-exposed conditions and a combination of rocky and sandy bottom substrates. Both phases, the gametophyte and the tetrasporophyte, were detected at this place growing simultaneously during July and August 2022. Gametophyte individuals were collected first and transported to the laboratory in dark-cool boxes filled with seawater within 30 minutes after collection. Biased sampling procedures were used to ensure the collection of healthy, highly branched and low epiphyte plants. Samples were carefully removed from the substrata to avoid tissue damages. Tetrasporophyte individuals were collected 15 days later following the same methodology. Once in the laboratory, the collected material was inspected and carefully cleaned with filtered seawater to remove epiphytes and sediments. Finally, seaweeds were pre-conditioned in 10L tanks, at 15°C, in filtered and UV sterilized seawater with continuous aeration and low light conditions (~ 10-20 µmol/m2/s), under a 12:12 light:dark cycle for at least 10 days before the beginning of the experiments. Conditioning and experiments were set up in an isothermal walk-in growth chamber, with controlled temperature and light conditions.
2.2 Mesocosm experiments
2.2.1 Experiment 1: Survival and regrowth of gametophytes mediated by hook-like structures
This experiment aimed to test the effects of two factors on the survival and regrowth capacity of gametophyte hook-like structures (referred as hooks from now on). Factors considered were: Temperature (2 levels: 15°C and 18°C) and Photon Flux Density (PFD) as a proxy of irradiance (3 levels: 5-10 µmol/m2/s, 55-60 µmol/m2/s and 95-100 µmol/m2/s). Temperature conditions tried to simulate spring average conditions (15°C) (de la Hoz et al., 2018), matching the peak period of biomass and cover in the collection area (personal observation), and a hypothetical climate change scenario of spring conditions (18°C). According to its ecology and life cycle, higher temperatures (e.g. summer temperatures) were not considered for the analysis as summer periods at the collection area were characterized by biomass declines. Light treatments tried to simulate an increasing gradient of irradiance, from under-canopy conditions to high light availability (e.g. at the top of the canopy where they grow epiphytically). Experiment consisted of two phases based on previous laboratory experiences (unpublished data). The first phase included the first 30 days of culture and was considered a transition period as almost any hook showed vegetative propagules (see Supplementary Figure 1 to see an example of those propagules). During this phase, the survival rate of the hooks was measured every 5 days. Survival was estimated by assigning 0s to those hooks showing less than 60% of damaged tissues (“alive” state) and 1s to those showing more than 60% (“non-alive” state). A damaged hook was considered when it showed symptoms of tissue depigmentation (“bleaching”) or necrosis (black patches). Second phase included the period between 30 and 60 days of culture, when the first vegetative structures appeared. In this phase, the survival rate and the length of the new erect axes (vegetative propagules) growing from original hooks (regrowth capacity) was measured at the end of the experiment. Supported by the previously mentioned laboratory experiences, regrowth analysis was done separately for two size classes (Class 1: <5mm; Class 2: >5mm) to check for differences among early recruits and those appearing at the end of the experiment.
Each combination of temperature and photon flux density (referred as treatment from now on) was replicated 4 times. Experiment conditions were simulated in 24 (6 treatments x 4 replicates) translucent-white plastic tanks (2L) supplied with filtered and UV-sterilized seawater under continuous aeration. Water was changed every 7 days to avoid nutrient depletion. The coldest temperature of the experiment (15°C) was determined by the chamber temperature, while the other temperature (18°C) required a heating system consisted of 100W auto-regulated aquarium heaters (EHEIM®, Germany). Every tank was placed inside water baths to avoid temperature fluctuations. Heaters were placed strategically to achieve homogeneous temperatures over the water bath. Lighting was provided by a combination of cool-white fluorescents (Sylvania® F30W/835) and Sylvania® Grolux F30W fluorescent lamps placed over the tanks. The photon flux density conditions were recreated using light filters (5-10 µmol/m2/s: Dark-gray filter, 55-60 µmol/m2/s: Translucent-white filter, 95-100 µmol/m2/s: Non-filter treatment). Each tank was covered by one of the filters (except those with the non-filter treatment) which were randomly assigned. Other parameters such as the photoperiod, the air temperature or the salinity were kept constant for the whole experiment (12:12 light:dark cycle, 15°C and ~ 35 PSU). Salinity was adjusted by adding distilled (Milli-Q®) water when required.
A total of 288 gametophyte hooks were excised from the collected individuals, gently washed and, then, randomly assigned to experimental units by groups of four. Experimental units were made of concrete with high limestone content (emulating natural rocky conditions) and disc shape. Three discs were placed in each replicated tank (i.e 12 hooks per tank distributed in 3 artificial discs). Hooks were trapped in each disc using a transparent nylon net to avoid drifting. Artificial discs and nylon nets were kept in seawater for 10 days before the start of the experiment. Hooks were selected according to two criteria: size (individuals of ~1-3cm) and morphology (only dichotomous hooks were selected as they were predominant among the collected samples; see Supplementary Figure 2 to see an example). Furthermore, we discarded fragments with necrotic patches, tissue damages or high epiphyte loads.
2.2.2 Experiment 2: Growth and phycobiliprotein content of drifting tetrasporophytes
The second experiment characterized the survival and growth responses to temperature and irradiance of previously excised tetrasporophyte fragments of A. armata. Three temperature levels (15, 20 and 25°C) and two irradiance levels (Low irradiance: <5 µmol/m2/s, High irradiance: 55-60 µmol/m2/s) were tested. Temperature conditions tried to simulate spring conditions (15°C), summer conditions (20°C) and a climate change scenario of summer conditions (25°C). The first two levels represent averaged temperatures for those periods when abundance peaks have been observed in the field (according to personal observations and the review of Zanolla et al., 2022). Light treatments simulate a non-limiting scenario of irradiance conditions (55-60 µmol/m2/s), always over the light compensation point and below photoinhibition (according to Zanolla et al., 2014) and a limiting scenario of low irradiance (<10 µmol/m2/s). The experiment lasted 30 days and two variables were measured every 10 days: relative growth and phycobiliproteins content. First, absolute growth (or de-growth) was estimated as the difference between fresh weight (FW) at day 0 and days 10, 20 and 30 respectively. Fresh weights were estimated by weighing each individual tuft after 30s of centrifugation in a hand-operated centrifuge and 30s in blotting paper. FW was transformed intro dry weight (DW) using a ratio of 0.12. This ratio was determined by drying 30 samples of 1gFW during 24 hours at 70°C. Finally, the relative growth was estimated as the ratio between initial and final weights. Phycobiliproteins were extracted by adding 5ml of phosphate buffer (0.1 M, pH 6.5) to the previously grounded algal thalli (0.10-0.15g FW). Two samples were taken from each thallus. Analysis was not performed when biomass was lower than 0.15g. Same samples cultured at 20°C and 25°C with enough biomass to perform the analysis after 20 and 30 days were discarded due to failures during the extraction process. Once centrifugated, the supernatants obtained were read by a UV–visible spectrophotometer. Phycobiliprotein content was estimated according to Beer and Eshel (1985) equations and expressed as mg/g DW. Due to the destructive nature of this technique, four flasks were randomly selected for the analysis at each time interval.
Temperatures were controlled by heaters in water baths; and irradiances by applying light filters (as in experiment 1). Treatments were replicated 4 times. For the experiment, the collected individuals were gently washed, divided in 288 spherical tufts (with similar sizes) and then distributed in 72 flasks (6 treatments x 4 replicates x 3 sampling times) by groups of four (i.e. 4 tufts per flask). Flasks were filled with 200ml of filtered and UV-sterilized seawater enriched with Provasoli medium (Provasoli, 1966) and supplied with continuous aeration. Water medium was changed every 5 days to avoid nutrient depletion. Algae entangled with other species or showing necrotic patches or tissue damages were discarded.
2.3 Data analysis
The potential effects of temperature and irradiance on the survival of A. armata hooks during the first 30 days of culture were evaluated by using a mixed modelling approach. For this purpose, a Generalized Mixed Model (GLMM, Zuur et al., 2009) was fitted with a binomial distribution of error terms and a logit link function. Temperature and irradiance were considered fixed factors. As repeated measures were done every 5 days, time was also added as a fixed factor. Possible random effects generated by the experimental design were addressed by adding Tank as random factor. Similarly, the differences between the survival rate after 30 days (when the first vegetative structures appeared) and 60 days of culture (the end of the experiment) were tested following the same mixed modelling approach. On the other hand, the potential effects of those factors on the regrowth capacity were tested using a Linear Mixed Model (LMM) (suitable for continuous data). In this analysis, time was not considered as a factor because only one measure at the end of the experiment was taken. Possible random effects generated by the experimental design were addressed by adding the hook of origin (“Parent_Hook”) as random factor. Both kinds of models were applied using the nlme, the lme4 and the MuMIn packages (Pinheiro et al., 2012; Bates et al., 2015; Bartoń, 2019) in the R 3.6.2 software (R Core Team, 2023). On the other hand, the growth (or de-growth) and the phycobiliprotein content of A. armata tetrasporophytes were analyzed by applying Linear Models (LMs) as independence among replicates was guaranteed. Temperature and irradiance were considered fixed factors and 3 models were fitted using the data collected after 10, 20 and 30 days of culture as measurements were taken on different individuals at each time interval.
The modelling approach for both mixed and non-mixed models started with the definition of the full or “beyond optimal” structures (see Zuur et al. (2009) for details) which consisted of the fixed factors, the interaction between them and the random factors (the later only for mixed models). Once defined, a model selection was performed. In the case of mixed models, first the optimal random structure was analyzed using the “beyond optimal” fixed structure. The final random structure was selected from nested models built with restricted maximum likelihood estimation and by using the hypothesis testing approach (Likelihood ratio test) (Zuur et al., 2009). Once the random structure was defined (if required), the final fixed structure was selected. All possible combinations of fixed factors and its interactions were compared using the Akaike Information Criteria for small samples (AICc; Burnham and Anderson (2004)). Candidate models (those with similar performance) were those differing less than 2 units from the model scoring the better AICc. The structure scoring the lowest AICc from candidate models was finally selected. Additionally, the Akaike weights (ωi) were also estimated to determine the relative importance of explanatory variables. According to Burnham (2015) this relative importance could be considered as the sum of all the Akaike weights of the set of models containing each variable. Once the final structure was selected, post-hoc analyses were applied to analyze pairwise differences using the R packages emmeans (Lenth et al., 2021) and multcomp (Hothorn et al., 2008). A significance level of 0.05 was considered. Model assumptions were analyzed and checked by using both graphical techniques and statistical tests (i.e. Kolmogórov-Smirnov and Levene’s tests). R packages dharma (Hartig, 2021), car (Fox and Weisberg, 2019), rcompanion (Mangiafico, 2016) and Stats were used.
3 Results
3.1 Survival of gametophytes’ hook-like structures
Survival percentages were low for every treatment after 30 days of culture. In fact, they were always below 50% at this moment (Figure 1). The evolution over time of survival was similar for treatments with low temperature (15°C) and intermediate/high irradiances (55-60/95-100 µmol/m2/s, respectively). At this temperature, low lighting levels (5-10 µmol/m2/s) implied lower survival rates at each time interval, being similar to the rates of the highest temperature (18°C) and intermediate/high irradiance levels. In the mixed modelling approach, the survival within the first 30 days of culture was mainly influenced by the interactive effects of temperature, irradiance and time (Table 1A; relative importance of Te x Ir x Tm = 0.940). This model structure was the only selected in the subset of candidate models (Δi < 2), suggesting a strong effect of the interaction of those fixed variables on the response variable.
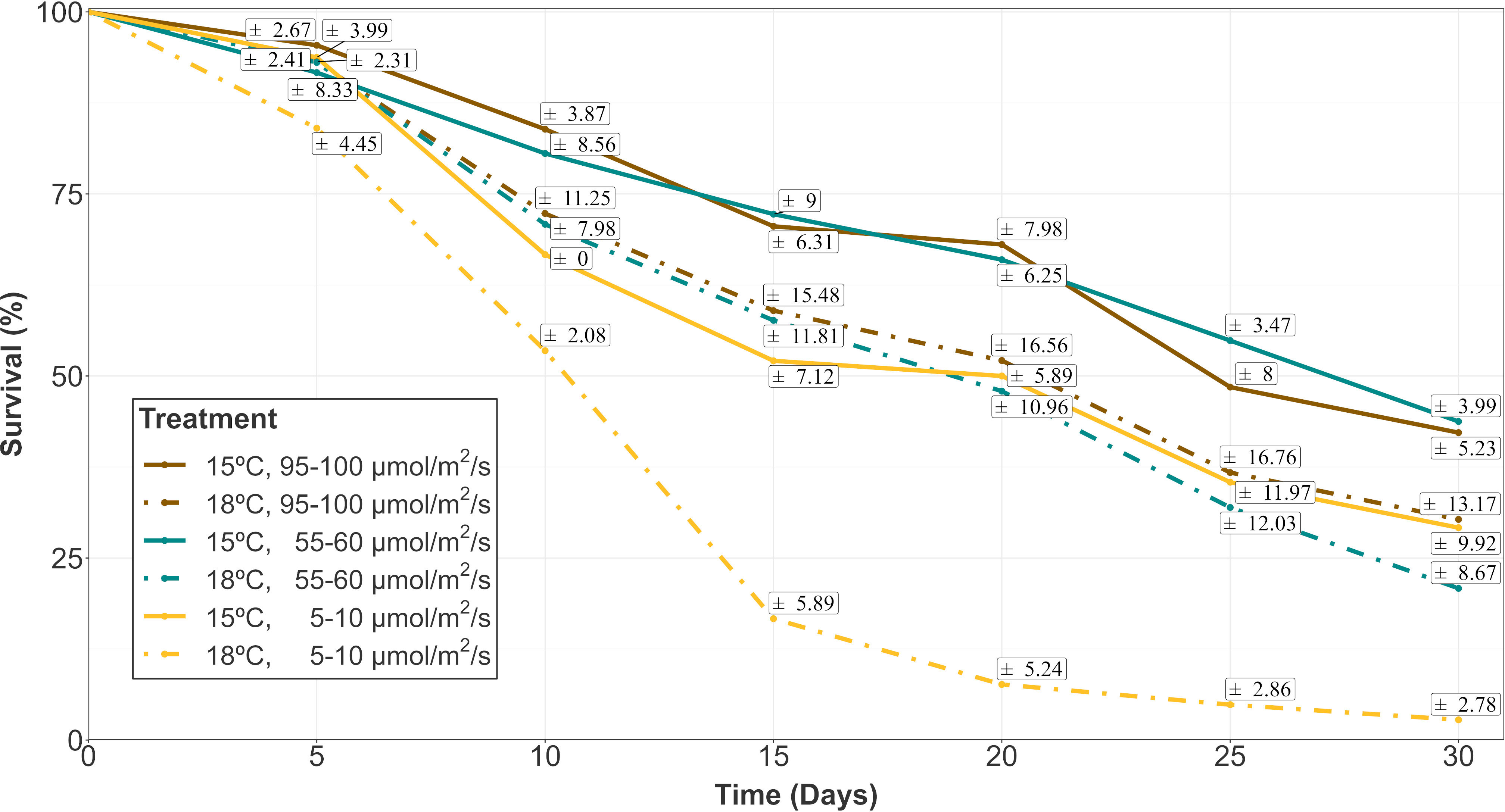
Figure 1 Survival evolution of gametophyte hooks of A. armata within the first 30 days of culture. Mean values are shown with standard errors represented in white boxes (n=4 at each time). Different colors represent different irradiances. Dashed and continuous lines reprepresent the different temperatures (18 and 15°C respectively).
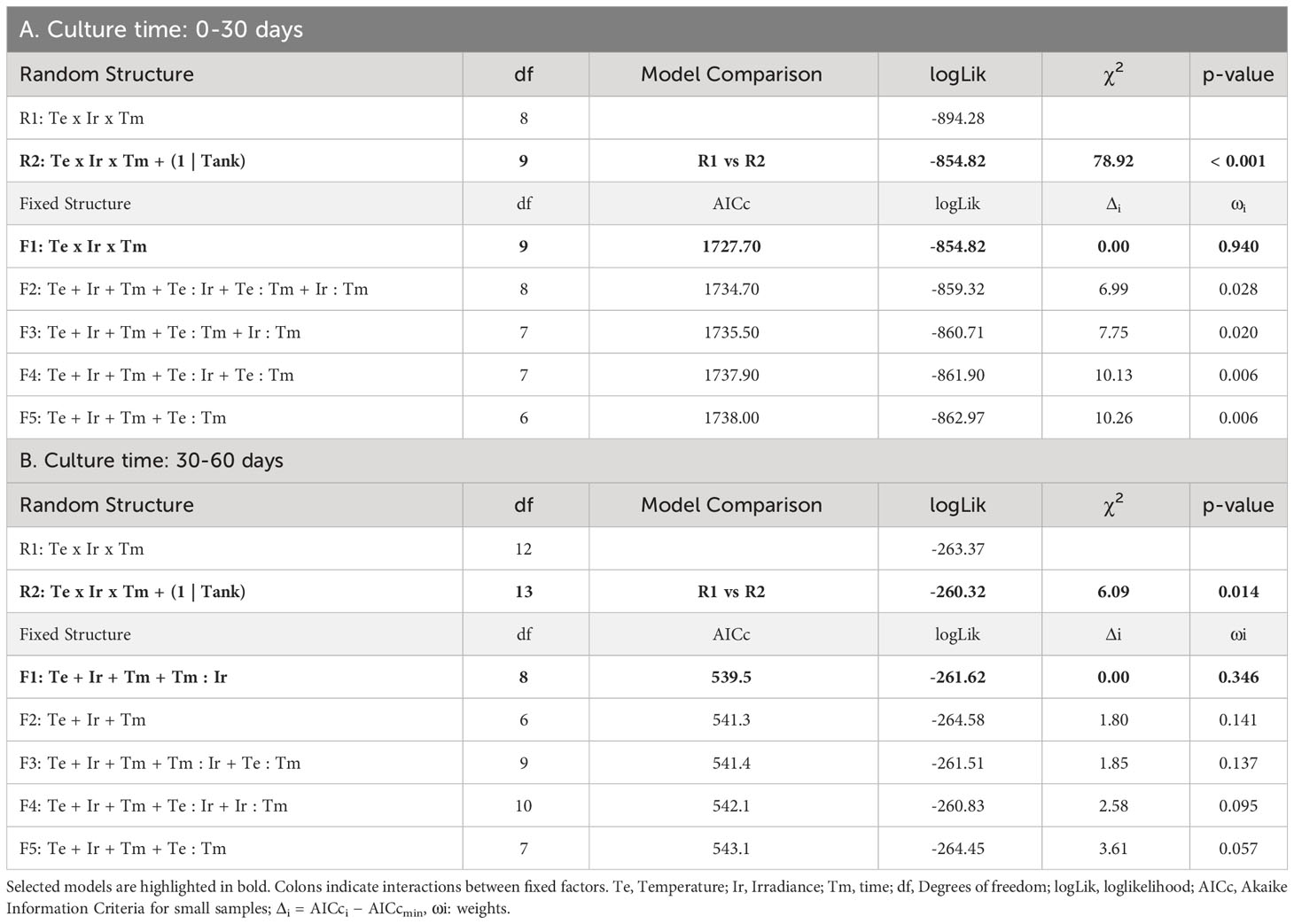
Table 1 Selection of random and fixed structures of GLMMs for the survival of hooks: A) during the first phase of culture (0-30 days), B) during the second phase of culture, once vegetative structures appeared (30-60 days).
At day 30 (the end of the first phase of the experiment), the lowest survival capacity (close to zero) was measured for the highest level of temperature (18°C) and low irradiance (5-10 µmol/m2/s) with a mean value of 2.78 ± 2.78% (Mean ± SE). Mean survival rates between 20 and 30% were obtained for the other irradiances at this temperature (Mean ± SE: 20.83 ± 8.67% at 55-60 µmol/m2/s; 30.30 ± 13.17% at 95-100 µmol/m2/s). A similar percentage was registered at 15°C and low irradiance (Mean ± SE: 29.17 ± 9.92%), while higher irradiances were associated to slightly higher survival percentages (Mean ± SE: 43.75 ± 3.99% at 55-60 µmol/m2/s; 42.22 ± 5.23% at 95-100 µmol/m2/s).
Survival after 30 days of culture (once the first vegetative shoots were observed) and 60 days of culture was also compared. In this analysis, the survival response variable was mainly influenced by the additive effects of temperature, irradiance, time and the interaction between time and irradiance (Table 1B; relative importance of Te, Ir and Tm = 0.624, Tm : Ir = 0.483). Among the candidate models, structures including the interaction between temperature and time were also identified suggesting a possible (but marginal) effect on the survival capacity (relative importance of Tm : Te = 0.137) after the appearance of vegetative propagules. After 60 days of culture, the survival capacity decreased for every treatment compared to that observed after 30 days, with the exception of the low irradiance cultures at 18°C where the few surviving hooks after 30 days remained alive (Figure 2). The main changes in survival were observed at 15°C and 95-100 µmol/m2/s where percentages were reduced from 42.22 ± 5.23% to 15.69 ± 3.43% (mean ± SE). At this temperature, higher percentages were registered after 60 days at the other irradiances, although differences were not significant (Tukey’s test, p > 0.05). At 18°C, mean survival was lower at almost every irradiance level being only significantly lower (Tukey’s test, p < 0.05) the survival percentage registered at the lowest level of irradiance.
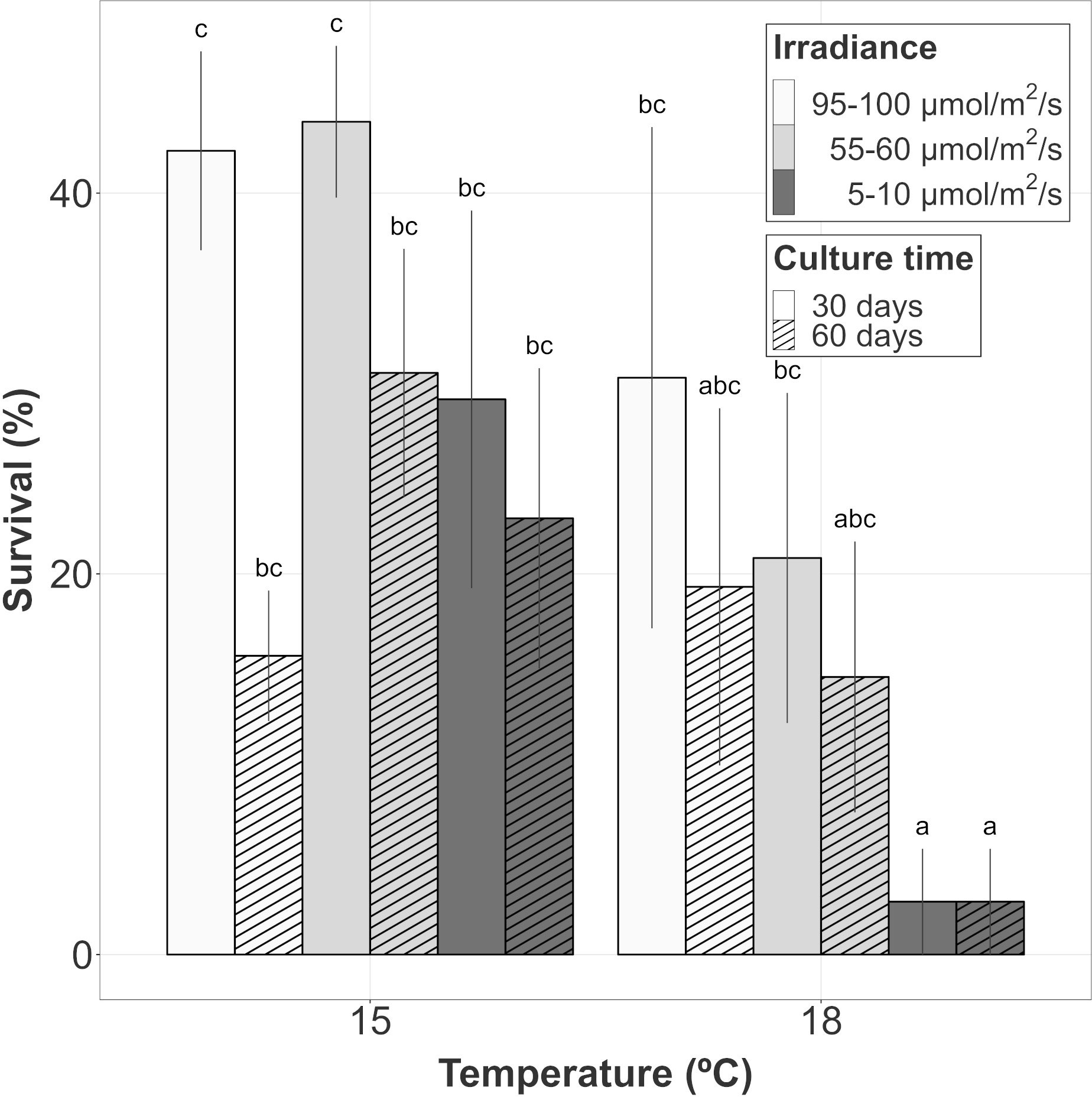
Figure 2 Survival evolution of gametophyte hooks of A. armata within the last 30 days of culture. Mean values ± SE are shown (n=4 at each time). Darker colors represent lower irradiances, while brighter colors represent higher irradiances. Dashed lines represent the number of culture days at the moment of measurements. Different letters represent significant differences (p<0.05) among treatments.
3.2 Regrowth capacity of gametophytes through vegetative structures
After 60 days of culture, vegetative structures appeared in every treatment, but not on every hook. At 15°C, the proportion of surviving hooks showing vegetative plantlets was almost 60% at intermediate and low levels of irradiance. However, the proportion for the hooks cultured at the highest irradiance was below 15%. At 18°C, the proportion was close to 60% at intermediate and high irradiances. Only one hook survived at low irradiance and 18°C after 60 days but showed vegetative structures (Figure 3). Unfortunately, the low number of surviving hooks in each replicate did not allow us to statistically test the difference among treatments.
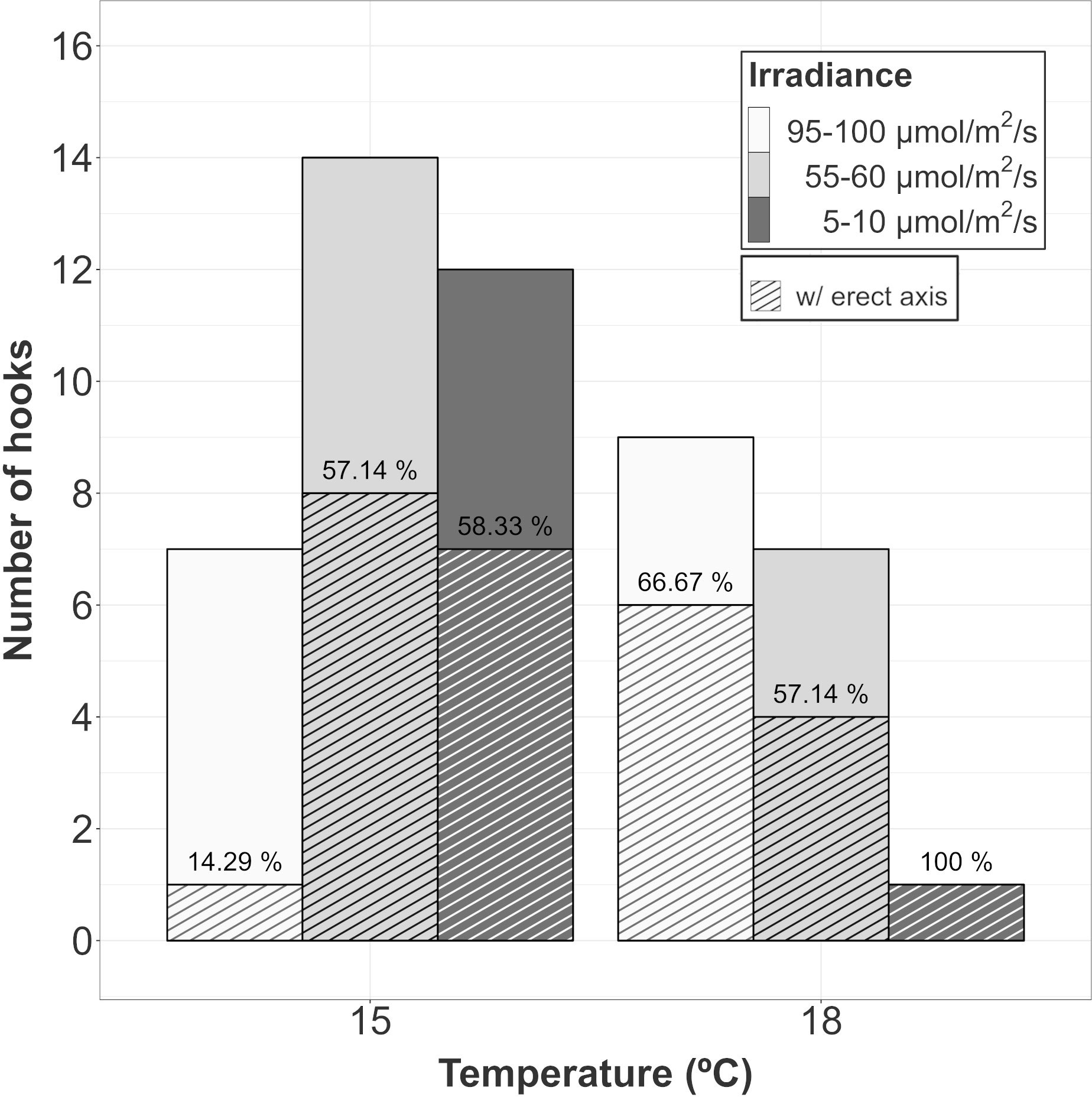
Figure 3 Proportion of surviving hooks in A. armata gametophytes showing vegetative structures after 60 days of culture. Top bars represent the number of surviving hooks at the end of the experiment and dashed bars represent the number of hooks showing vegetative structures. The ratio between both of them (as percentage) has been placed over dashed bar.
From those hooks showing vegetative shoots, a high proportion of class 1 recruits (0-5mm) was observed for almost every combination of temperature and irradiance. The length of those new recruits seemed to be influenced mainly by the additive effects of temperature and irradiance (Table 2A; relative importance of Te and Ir = 0.989). The interaction of temperature and irradiance was included in some models from the subset of candidates, suggesting a possible effect on growth (relative importance of T x L = 0.367). Mean length at intermediate irradiance was over 2mm regardless of the temperature treatment, while low irradiances were below but being significantly lower only at 18°C (Figure 4A). No recruits of that size were identified at 15°C and high irradiance.
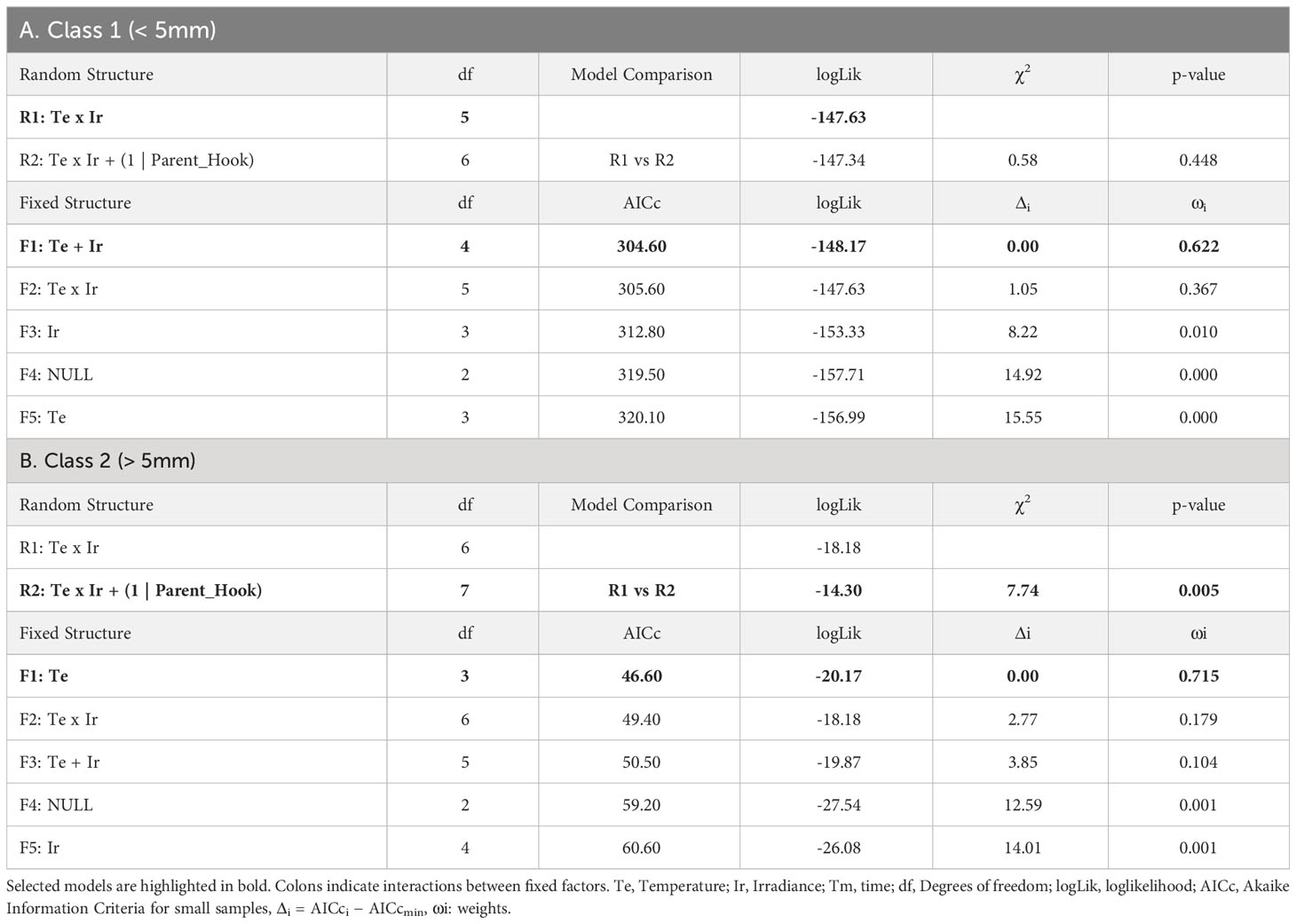
Table 2 Selection of random and fixed structures of LMMs for the growth of recruits: A) Class 1 (<5mm length), B) Class 2 (>5mm length).
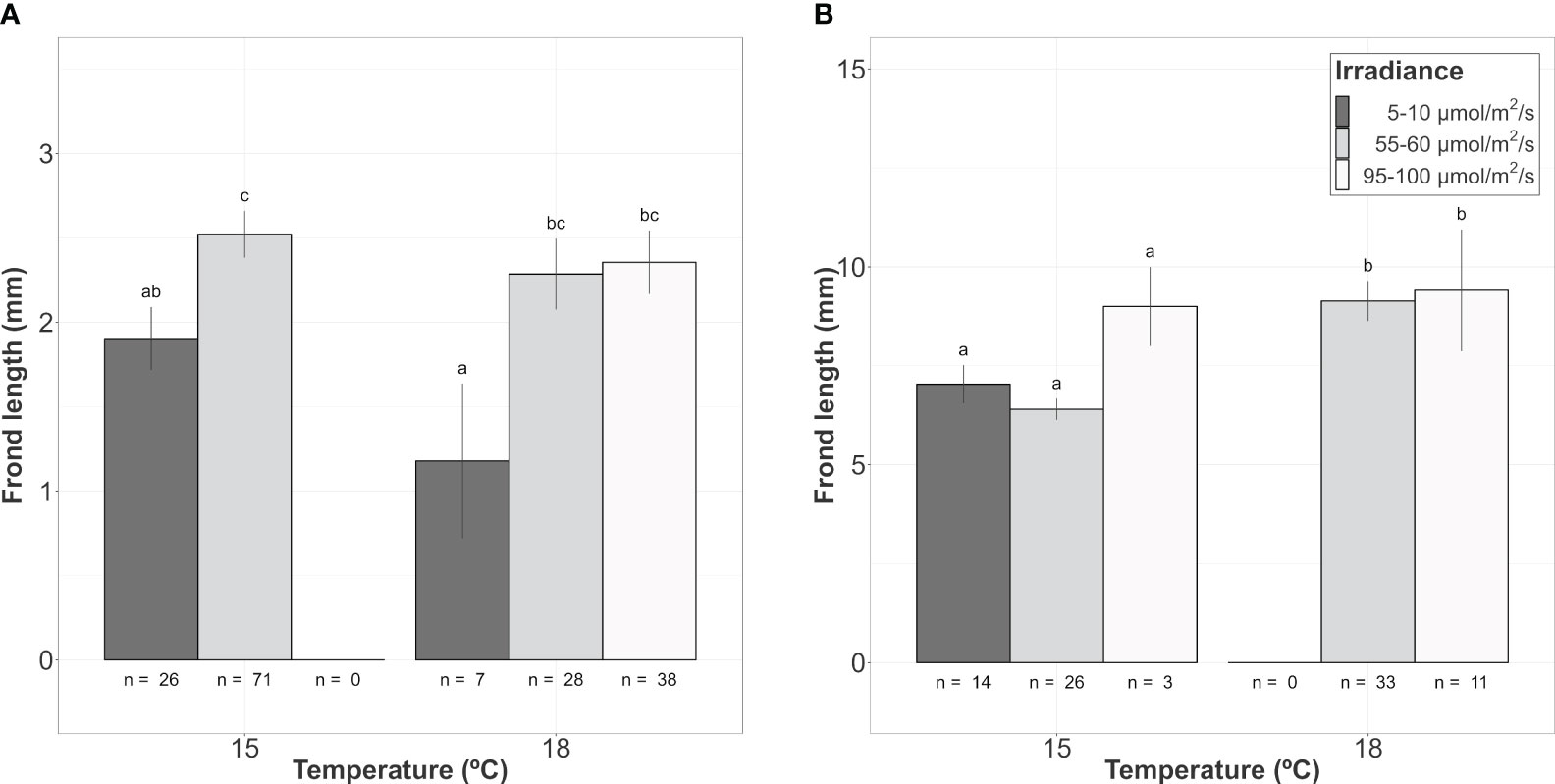
Figure 4 Mean frond length of A. armata gametophyte recruits growing from the hook’s surface after 60 days of culture. (A) Class 1 (<5mm length). (B) Class 2 (>5mm length). Mean values ± SE are shown. Number of recruits (n) to calculate mean values are shown below the bars. Darker colors represent lower irradiances, while brighter colors represent higher irradiances. Different letters represent significant differences (p<0.05) among treatments.
As mentioned before, the number of class 2 recruits (>5mm) was lower. For them, the differences in length observed after 60 days of culture were mainly explained by temperature as shown in the modelling approach (Table 2B, relative importance of Te = 0.715). Mean length was significantly higher at 18°C compared to the values registered at 15°C (mean values ± SE: 9.20 ± 0.53mm at 18°C and 6.79 ± 0.25mm at 15°C). Only the few vegetative propagules found at 15°C and 95-100 µmol/m2/s (n = 3) registered a length closer to the mean values registered at 18°C (9.00 ± 1.00 mm; mean value ± SE). No recruits larger than 5mm were identified at 18°C and low irradiance (Figure 4B).
3.3 Tetrasporophytes’ growth
After 10 days of culture, mean positive values of growth were only found at 20°C (regardless of the incident irradiance) and 15°C and 55-60 µmol/m2/s. However, even with a positive mean, both negative and positive values were registered for tetrasporophytes growing at 20°C and a low level of irradiance (Figure 5). At this time, growth was influenced by the additive effects of temperature and irradiance (Table 3A; relative importance of Te = 0.853 and Ir = 0.853). The highest relative growth was detected at 15°C and 55-60 µmol/m2/s, though differences were not significant compared to the values registered at 20°C. For those treatments registering degrowth rates, weight losses ranged from the 14.23 ± 6.14%, at 15°C and low irradiance, to 57.22 ± 3.60% at 25°C and low irradiance.
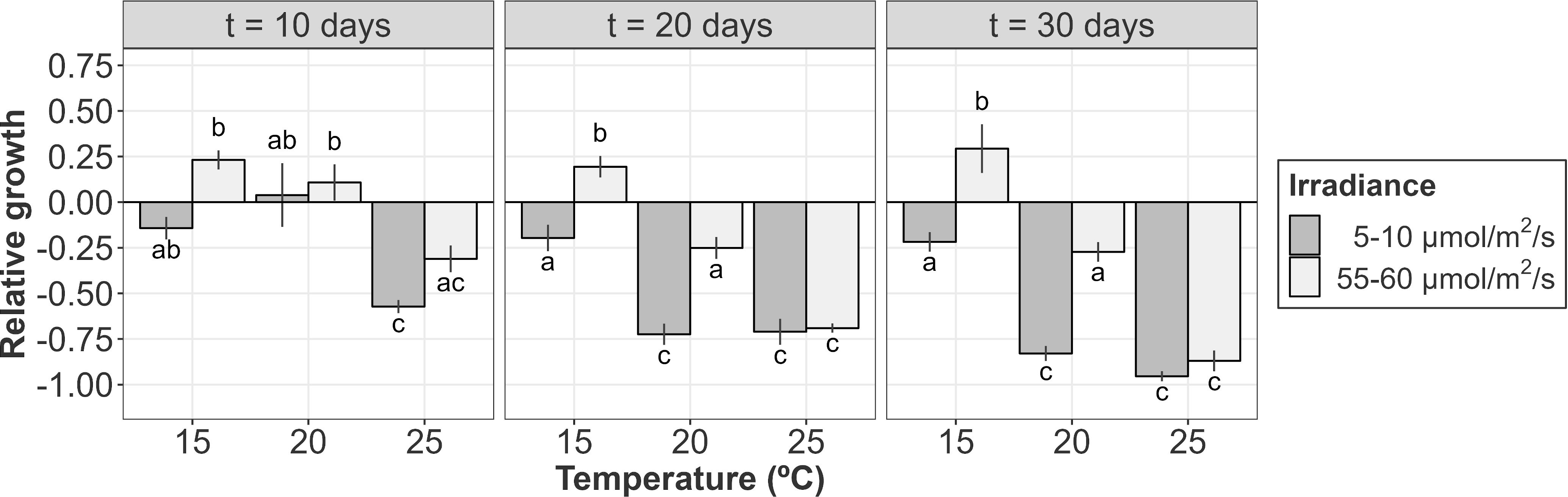
Figure 5 Relative growth (or de-growth) of A. armata tetrasporophytes after 10, 20 and 30 days of culture. Mean values ± SE are shown (n = 4). Darker colors represent lower irradiances, while brighter colors represent higher irradiances. Different letters represent significant differences (p<0.05) among treatments.
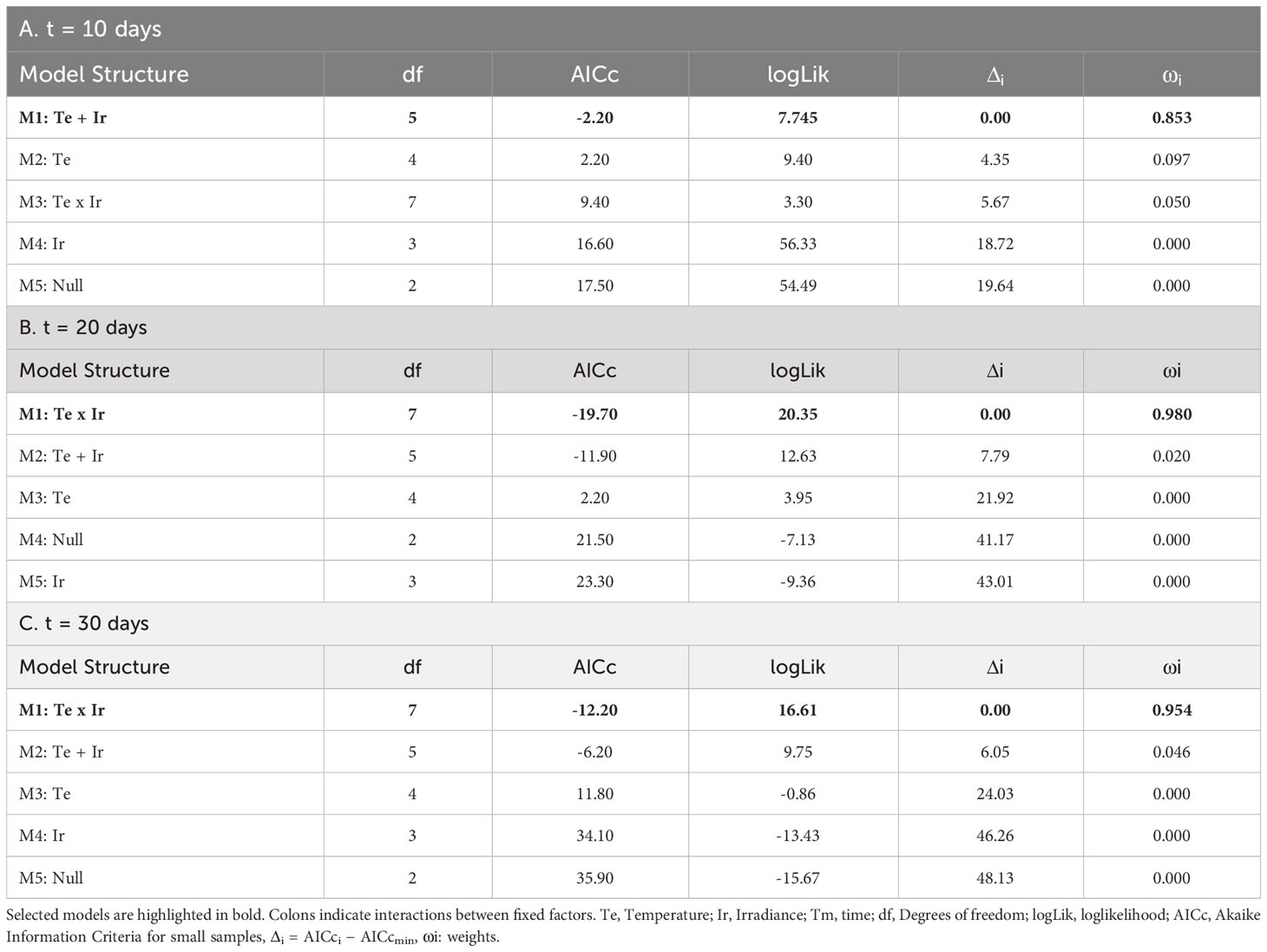
Table 3 Selection of fixed structures of LMs for the growth of tetrasporophytes after: A) 10 days; B) 20 days; and C) 30 days.
Relative growth after 20 days of culture was only detected at 15°C and 55-60 µmol/m2/s. The other treatments were characterized by biomass losses during the second half of the experiment (Figure 5). Increasing rates of tufts growing under these conditions were close to the 25% of the initial weight, a similar value to that obtained after 10 days of culture. Mean positive rates at 20°C became negative after 20 days of culture, being significantly higher for the tufts receiving less irradiance. At higher irradiances, weight losses were lower and similar to that obtained at 15°C and 5-10 µmol/m2/s. This interactive effect of temperature and irradiance over the relative growth of tetrasporophytes after 20 days of culture was capture by the linear model (Table 3B; relative importance of Te x Ir = 0.980), being the only candidate model to fit the data.
After 30 days of culture, the relative growth was again mainly influenced by the interactive effects of temperature and irradiance (Table 3C; relative importance of Te x Ir = 0.954). In fact, the pattern obtained was very similar to that obtained after 20 days of culture. Mean weight losses were higher at 25°C (regardless of the irradiance) and at 20°C and low irradiances with values over the 75%, reaching almost the 100% at 25°C and 5-10 µmol/m2/s. Losses at 20°C/55-60 µmol/m2/s and 15°C/5-10 µmol/m2/s were similar (~25%) and kept constant compared to the results obtained after 20 days of culture. Again, the only gaining in weight was detected at 15°C and 55-60 µmol/m2/s. Gaining was slightly over the 25% of the initial weight, a value similar to that obtained after 10 and 20 days of culture (Figure 5).
3.4 Phycobiliprotein content of tetrasporophytes
The phycobiliprotein content was mainly influenced by temperature and irradiance after 10 days of culture (Table 4A; relative importance of Te =0.676 and Ir = 0.676). In general, at this stage, higher temperatures and lower irradiances were associated to higher phycobiliprotein contents. Phycobiliprotein contents were always higher at low irradiances within the same temperature, though differences were not significative in the pairwise comparisons. Within the same irradiance level, phycobiliprotein content at 25°C was significantly higher than values registered at 15°C, though differences were not significant when compared to 20°C (Figure 6).
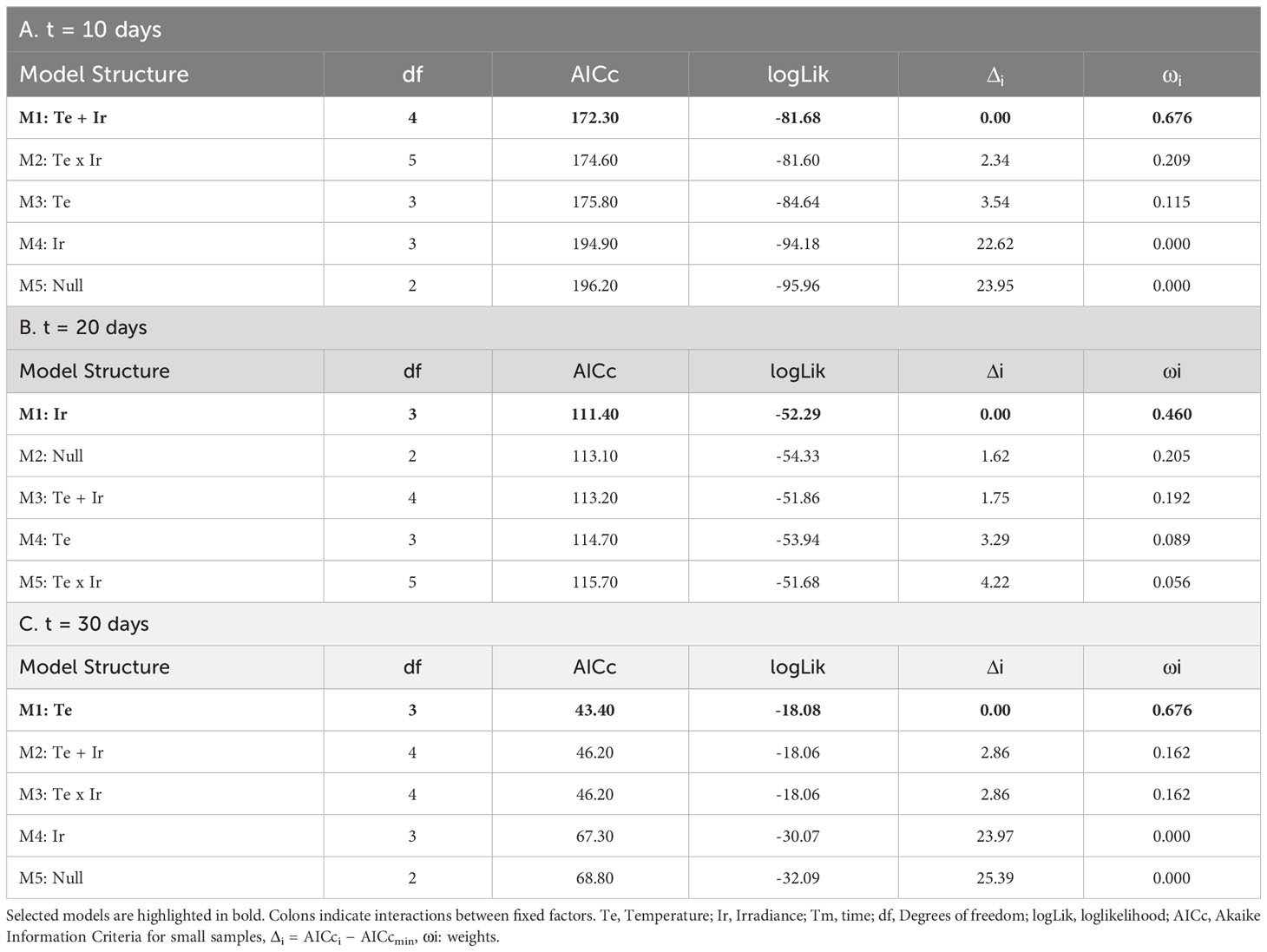
Table 4 Selection of fixed structures of LMs for the Phycobiliprotein content (mg/g DW) of tetrasporophytes after: A) 10 days; B) 20 days; and C) 30 days.
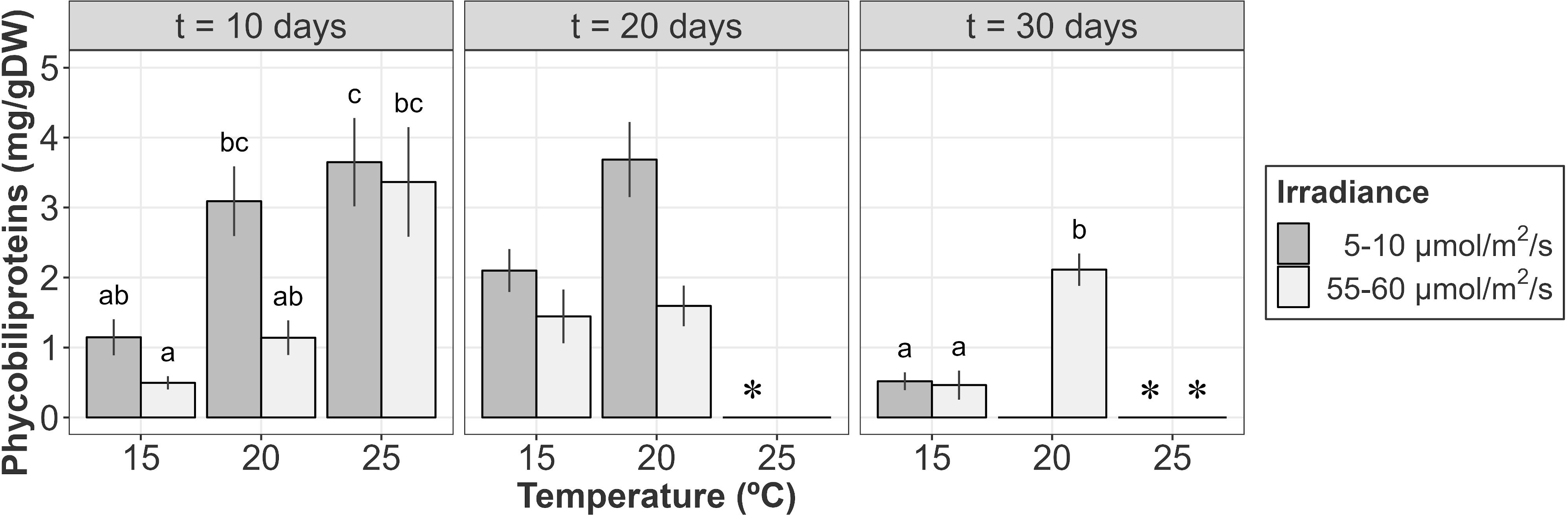
Figure 6 Phycobiliprotein content (mg/g DW) of A. armata tetrasporophytes after 10, 20 and 30 days of culture. Mean values ± SE are shown (n = 6-8). Darker colors represent lower irradiances, while brighter colors represent higher irradiances. * Phycobiliprotein analysis were not performed at 25°C/5-10 µmol/m2/s after 20 days and 25°C (both irradiances) after 30 days of culture due to the lack of biomass. Different letters represent significant differences (p<0.05) among treatments.
After 20 days of culture, weight losses at 25°C were associated to red pigment degradation. Visual inspection of samples at this temperature revealed that red pigments disappeared after 20 days. Tufts at this moment were characterized by a green/yellowish color. However, as the biomass for some samples was not enough to measure phycobiliprotein content, this treatment was not included in LMs. For the remaining treatments, differences seemed to be mainly influenced by the irradiance received, with higher phycobiliprotein contents at lower irradiances (Figure 6, Table 4B; relative importance of Ir = 0.652). However, pairwise comparisons revealed no significant differences between treatments.
Biomass losses continued until the end of the experiment at 20 and 25°C. At that point, the remaining biological tissues at 20°C/5-10 µmol/m2/s and 25°C (both irradiances) were not enough to measure the pigment content for almost every sample. From the other treatments, higher phycobiliprotein contents were detected for those tufts cultured at 20°C during 30 days (Figure 6). Differences were mainly influenced by temperature, as phycobiliprotein content at 15°C was statistically equal for both irradiances (Figure 6 and Table 4C; relative importance of Te = 0.676).
4 Discussion
Vegetative propagation responses for gametophytes and tetrasporophytes of A. armata were influenced by temperature and irradiance. For gametophytes, the survival rates of the hooks after 30 days of culture were lower (though not always significant) under increasing levels of temperature and decreasing levels of irradiance. However, patterns of appearance of plantlets at different temperature and irradiance conditions were not clear. In the case of tetrasporophytes, only positive growth rates were registered at 15°C and 55-60 µmol/m2/s after 30 days of culture. Higher concentration of phycobiliproteins was detected at higher temperatures during the first days of culture, while not clear patterns were detected at the end. The results for this phase indicated that temperature is the main factor controlling the survival of broken pompon-like structures when irradiance is not a limiting factor.
Study findings showed how the development of new fronds from single hooks collected in northern Spain (1-3cm length) is possible. These findings differ to those found for A. armata in New Zealand and Tasmania where regrowth from single hooks was not possible (Bonin and Hawkes, 1987; Wright et al., 2022), but are in close agreement to those found in France where regrowth occurred (Codomier et al., 1979; Haslin and Pellegrini, 2001). The lack of regrowth in New Zealand and South Australia has been suggested to be attributed to seasonal effects or the existence of genetically different strains (Wright et al., 2022). In fact, there are genetic evidences of different clades of A. armata clearly linked to its geographic distribution (Dijoux et al., 2014; Preuss et al., 2022) and differences among these strains have been previously reported for other phenological responses (e.g. Ní Chualáin et al., 2004).
In our study, vegetative propagation through hooks was conditioned by the low survival rates of those structures, being always below 50% after 60 days of culture. The low survival of those specialized structures and the weakness of the attachment bundles produced by a single hook (Wright et al., 2022) may explain why they are produced in high numbers, occurring every 3-4cm along the thallus (Wright et al., 2022). Both temperature and irradiance seemed to have influenced the survival rates, being the rates higher at increasing levels of irradiance and decreasing temperatures. In fact, temperatures over 18°C seemed to be lethal for excised hooks. In a preliminary study, these structures were cultured also at 20 and 25°C resulting in 100% mortality rates within the first ten days of culture (unpublished data). These results were congruent with the annual summer biomass decline observed in the field for this species at the collection area. According to Ní Chualáin et al. (2004), A. armata can grow at temperatures between 9 and 21-23°C, with a wider range of survival (up to 25-28°C). Presented results suggest that the vegetative propagation through hooks may be limited to a narrower range as a consequence of its survival capacity, being 18°C the upper limit. Temperatures below 15°C were not tested, so the lower limit for this process is still to be determined. Regarding light availability, lower irradiances resulted in lower survival rates. For A. armata gametophytes the light compensation point (Ic) was set at a mean value of 20 µmol photons/m2/s (Flores-Moya et al., 1996). Being continuously under this compensation point was critical for hooks growing at 18°C, as only a 3% of the initial hooks survived after 60 days of culture, but not for those growing at 15°C. According to this, it seems that Ic may be influenced by temperature as it has been shown for tetrasporophytes (Zanolla et al., 2014). Those results contrast to that found for other red seaweeds such as Gelidium sp., where more vegetative structures were produced under low levels of irradiance (Fonck et al., 2007; Otaíza et al., 2018; Sainz-Villegas et al., 2023). In fact, Lüning et al. (1990) stated that germlings of red algae growing under canopy achieve its maximum growth rates at very low levels of incident light (approx. 4-5 µmol/m2/s). However, as shown by the results, it seems that the under-canopy development of A. armata may be scarce. In fact, germlings grow mainly on top of other species (as an epiphyte) or over complex surfaces where the specialized structures (hooks) get entangled (personal observation) in the field.
Regarding regrowth, hooks developing new plantlets were registered in every treatment after 60 days of culture but a clear response pattern to temperature and/or irradiance was not detected. The ratio recruit size/hook size was always between 0-2. These results differ from those reported by Haslin and Pellegrini (2001) were ratios scored values between 0-3.5 after one month of culture. In fact, the first vegetative structures in our study did not show until the 30th day of culture, while in Haslin and Pellegrini (2001) appeared after 10 days. Temperature and irradiance conditions in that study were close to the ranges explored in our study, suggesting nutrients to be the main factor influencing those differences.
It is well known that many red algae can produce structures that allow attachment through asexual reproduction (Cecere et al., 2011). Here, after 60 days of culture, attachment structures were not identified for any of the cultured hooks, despite contact between tissues and substrata was assured with a plastic mesh. Instead, a complex system of axes through the different threads of the net was observed, preventing the original thalli from moving. In fact, it was impossible to remove the hooks from the mesh without breaking any biological tissue. Nevertheless, no solid points/discs of attachment nor a rhizomatous system were found. These results suggest that it is not necessary the attachment of the hook to another object to induce vegetative propagation as it has been previously suggested (Svedelius, 1933). Actually, it seems that the entanglement, and thus, the attachment is promoted by the growing vegetative axis of the new recruits. In contrast, some field collected individuals presented morphologically different hooks with bigger and rounded spines not seen in the laboratory experiments. These hooks seemed to be attached to other species, but it was difficult to discern if there was through attachment structures or not due to the entanglement degree. In some cases, those modified spines seemed to grow and trap the other species tissue (personal observation), but this require a more detailed analysis to be confirmed.
A. armata tetrasporophytes are also capable of reproducing through vegetative propagation by the breakage of the pompom-like structures into different tufts (Zanolla et al., 2022). Previous studies on the life cycle of this species showed how phase alternation is temperature, light and nutrient dependent. In fact, sporogenesis occurs only at a narrow range of temperatures ( ± 4-6°C) and daylengths below 9 hours (Oza, 1977; Guiry and Dawes, 1992; Ní Chualáin et al., 2004). Temperature ranges vary among strains, being 13-17° C for Australian individuals, 15-21°C for the Irish strain and 17-21°C for Italian seaweeds (Guiry and Dawes, 1992). In this study, we have shown that the vegetative propagation process is also influenced by temperature and light conditions. Actually, both the growth and the phycobiliprotein content were affected by temperature and/or irradiance at some point during the 30 days of culture.
Regarding tetrasporophyte growth, gaining of biomass was only registered at 15°C and 55-60 µmol/m2/s after 30 days of culture. At 20°C, a weak increase of biomass was observed during the first 10 days of culture followed by biomass losses after 20 and 30 days. At 25°C biomass losses were registered at every time interval. Previous studies on A. armata tetrasporophytes established a survival range from 3 to 25°C and a growing range from 7°C to 23°, being wider or narrower depending on the strain considered (Ní Chualáin et al., 2004; Mata et al., 2006). In Ní Chualáin et al. (2004), the survival range of the strain from the site closest to our collection area was 5-25°C and the growth range was 9-21°C. In our research, once divided into tufts, A. armata tetrasporophytes were not able to grow at 20°C after 30 days of culture. However, when the light levels were between 55-60 µmol/m2/s, biomass losses were around 25% after 20 days and remained stable after 30 days. In addition, survival at 25°C was either not possible, as almost 100% of the initial weight was lost after 30 days. Many possible factors could explain those differences. However, we have to consider that 20°C and 25°C are close or at the top limits of growing (21°C) and survival (25°C) respectively. In this sense, differences in the environmental conditions previous to the collection dates (e.g. hotter summer stressful conditions, lower nutrient levels, etc.) or even the stress induced during the sample processing may have affected the results.
Considering light, it has been shown how the lowest irradiance level (5-10 µmol/m2/s) induced higher biomass losses. Previous studies on the photosynthetic plasticity of A. armata tetrasporophytes found low light compensation points (Ic) values which were temperature dependent (e.g. Mata et al., 2006; Zanolla et al., 2014). For example, Ic values at 15°C were around ~5-6 µmol/m2/s. This means that one of our treatments was close to the light compensation point during the whole experiment and may explain why a stable biomass loss (15-25%) was observed at each time interval. At 20°C, direct estimations of Ic were not carried out, but considering the closest temperatures, the Ic should be between 7.5 and 12.5 µmol/m2/s. At 25°C, Ic values should be in the range 12.5-15 µmol/m2/s. According to this, being continuously under the compensation point at 25°C led to biomass losses clearly visible during the first days of culture (50% of the biomass was lost within the first 10 days). In this sense, short extreme marine heatwaves may be lethal for those tetrasporophytes growing under canopy, epiphytically at a certain depth or floating in high density biomass aggregations. On the other hand, photoinhibition was not a problem in our study as it is induced at an irradiance level of 150 µmol/m2/s, regardless of the temperature considered (Zanolla et al., 2014).
The analysis of phycobiliproteins was carried out in order to quantitatively evaluate the bleaching or depigmentation process. Although chlorophyll-a is considered an essential pigment for photosynthesis due to its role in the capture of light and its function as an electron donor in the electron transport chain (Raven et al., 2005), we focused the analysis on phycobiliproteins, considered accessory pigments (Häder and Figueroa, 1997), because they are directly linked to that bleaching process. This process has been previously considered as a morphological “health” indicator for red seaweeds growing under stressful conditions (e.g. Quintano et al., 2017; Quintano et al., 2018). Bleaching is the consequence of a physiological response to stress which result in a complete degradation of photosynthetic pigments. In our study, frond bleaching did not show until the second half of the experiment. In fact, after 10 days of culture no depigmentation was registered as shown by the phycobiliprotein analysis. Even at 25°C and low irradiance, where 50% of the biomass was lost, depigmentation was not visible. Actually, phycobiliprotein content was higher at lower irradiances at that time. The increasing of photosynthetic pigments to compensate low light availability is a common strategy of seaweeds and it has been previously reported for other red macroalgae (Ramus et al., 1976; Xu and Gao, 2008). However, the following ten days were critical for tetrasporophytes growing at 25°C. During this time, some metabolic thresholds were probably exceeded leading to frond bleaching in almost every thallus. At this moment, depigmentation was also observed for some tetrasporophytes growing at 20°C and 5-10 µmol/m2/s, as reflected by the slight variance increase at the pigment analysis. After 30 days, depigmentation was generalized over the replicates. This loss of pigments is usually linked to a reduction in growth, thallus weakening and death (Martone et al., 2010). However, this was not entirely supported by our results. At 25°C, depigmentation and weight losses were clearly linked and evidenced tetrasporophytes death. Although depigmentation was clearly visible, it was not confirmed by the pigment analysis as it was impossible to perform it due to the lack of biomass at this temperature. The same pattern was observed at 20°C and low irradiance, but bleaching was not detected at 55-60 µmol/m2/s despite registering weight losses. At 15°C, depigmentation was not observed though the pigment analysis reflected a clear reduction in the content of red pigments. However, conclusions derived from the red pigment analysis should be taken carefully as tests were clearly limited by biomass losses. More research is still needed on the photosynthetic responses of vegetatively grown tetrasporophytes, from the analysis of a wider pool of pigments to its relationship with photosynthetic parameters.
Vegetative propagation has been previously identified as the major form of population maintenance for other species in the genus Asparagopsis (Zanolla et al., 2018b). Considering this, it is easy to find synergies between the studies predicting changes in A. armata distributions under projected temperature scenarios (e.g. Zanolla et al., 2018a) and our study, where temperature was the main factor limiting propagation. But not only at biogeographical scales, it might be also linked to changes in local processes such as seasonality. Cantabrian Sea populations were characterized by four peaks of abundance and biomass throughout the year, corresponding to four different cohorts (Aranda et al., 1984). According to our results, the number and duration of cohorts and the peak values may be affected by temperature changes that limit the vegetative propagation process particularly in late spring and summer. In fact, A. armata gametophytes seemed to have already changed at the collection area, as only one peak of biomass in spring has been observed in the last years (personal observation). Similar patterns have been previously reported for other populations (e.g. Azores populations, Neto, 2000). Understanding this patterns of change is crucial from a management perspective, as the negative impacts of this invasive seaweed on native ecosystems are well documented. For example, it has been associated to reductions in macroalgal species richness and abundance (e.g. Silva et al., 2021) or negative impacts on native epifauna as a consequence of the production of toxic exudates (Guerra-García et al., 2012; Rubal et al., 2018; Silva et al., 2020). In this sense, the results presented here have important implications for the analysis of invasion risk and the detection of potentially threatened areas as it has been shown that the spread of the species by vegetative means is dependant on temperature and irradiance. A possible way to include this information on risk analysis is through the use of Hybrid Species Distribution Models (SDMs), which integrate the vegetative propagation responses in correlative models enhancing a better delimitation of vulnerable areas and conservation/protection zones.
Data availability statement
The original contributions presented in the study are included in the article/Supplementary Material, further inquiries can be directed to the corresponding author/s.
Author contributions
SS-V: Conceptualization, Data curation, Formal analysis, Investigation, Methodology, Writing – original draft, Writing – review & editing. BS-A: Formal analysis, Investigation, Writing – review & editing. AP: Conceptualization, Formal analysis, Funding acquisition, Project administration, Writing – review & editing. JJ: Conceptualization, Formal analysis, Funding acquisition, Project administration, Writing – review & editing.
Funding
The author(s) declare financial support was received for the research, authorship, and/or publication of this article. This work was funded by the National Plan for Research in Science and Technological Innovation from the Spanish Government 2017-2020 [grant number C3N-pro project PID2019-105503RB-I00] and co-funded by the European Regional Development’s funds. Samuel Sainz-Villegas and Begoña Sánchez-Astráin acknowledge the financial support received under predoctoral grants from the Spanish Ministry of Science, Innovation and Universities [grant numbers: FPU18/03573 and PRE2020-096255, respectively]. This work is part of the PhD project of Samuel Sainz-Villegas.
Conflict of interest
The authors declare that the research was conducted in the absence of any commercial or financial relationships that could be construed as a potential conflict of interest.
Publisher’s note
All claims expressed in this article are solely those of the authors and do not necessarily represent those of their affiliated organizations, or those of the publisher, the editors and the reviewers. Any product that may be evaluated in this article, or claim that may be made by its manufacturer, is not guaranteed or endorsed by the publisher.
Supplementary material
The Supplementary Material for this article can be found online at: https://www.frontiersin.org/articles/10.3389/fmars.2023.1343353/full#supplementary-material
References
Andreakis N., Schaffelke B. (2012). “Invasive marine seaweeds: Pest or prize? BT - Seaweed Biology: Novel Insights into Ecophysiology, Ecology and Utilization,” in Seaweed Biology. Eds. Wiencke C., Bischof K. (Berlin: Springer), 235–262. doi: 10.1007/978-3-642-28451-9_12
Aranda J., Niell F. X., Fernández J. A. (1984). Production of Asparagopsis armata (Harvey) in a thermally-stressed intertidal system of low tidal amplitude. J. Exp. Mar. Bio. Ecol. 84, 285–295. doi: 10.1007/s100520050387
Bartoń K. (2019) “MuMIn”: Multi-Model Inference. Available at: https://cran.r-project.org/web/packages/MuMIn.
Bates D., Mächler M., Bolker B., Walker S. (2015). Fitting linear mixed-effects models using “lme4”. J. Stat. Software 67, 1–48. doi: 10.18637/jss.v067.i01
Beer S., Eshel A. (1985). Determining phycoerythrin and phycocyanin concentrations in aqueous crude extracts of red algae. Aust. J. Mar. Freshw. Res. 36, 785–792. doi: 10.1071/MF9850785
Bonin D. R., Hawkes M. W. (1987). Systematics and life histories of New Zealand Bonnemaisoniaceae (Bonnemaisoniales, Rhodophyta): I. The genus Asparagopsis. New Zeal. J. Bot. 25, 577–590. doi: 10.1080/0028825X.1987.10410088
Breeman A. M., Meulenhoff E. J. S., Guiry M. D. (1988). Life history regulation and phenology of the red alga Bonnemaisonia hamifera. Helgoländer Meeresuntersuchungen 42, 535–551. doi: 10.1007/BF02365625
Burnham K. P. (2015) Multimodel inference: understanding AIC relative variable importance values. Available at: http://warnercnr.colostate.edu/kenb/pdfs/KenB/AICRelativeVariableImportanceWeights-Burnham.pdf.
Burnham K. P., Anderson D. R. (2004). Multimodel Inference: Understanding AIC and BIC in model selection. Sociol. Methods Res. 33, 261–304. doi: 10.1177/0049124104268644
Cecere E., Petrocelli A., Verlaque M. (2011). Vegetative reproduction by multicellular propagules in Rhodophyta: an overview. Mar. Ecol. 32, 419–437. doi: 10.1111/j.1439-0485.2011.00448.x
Chihara M. (1960). On the germination of tetraspores of Falkenbergia hillebrandii (Bornet) Falkenberg. Japanese J. Bot. 35, 181–199.
Codomier L., Segot M., Combaut G., Teste J. (1981). Sur le développement d’Asparagopsis armata (Rhodophycée, Bonnemaisoniale) en culture. Effets de certaines substances azotées ou phosphorées inorganiques ou organiques. Bot. Mar. 24, 43–49. doi: 10.1515/botm.1981.24.1.43
Codomier L., Segot M., Teste J. (1979). Sur le developpement d’Asparagopsis armata (Rhodophycée, Bonnemaisoniale) en culture: effet des phytohormones sur la croissance des frondes. Botanica Marina 22, 153–158. doi: 10.1515/botm.1979.22.3.153
de la Hoz C. F., Ramos E., Acevedo A., Puente A., Losada Í.J., Juanes J. A. (2018). OCLE: A European open access database on climate change effects on littoral and oceanic ecosystems. Prog. Oceanogr. 168, 222–231. doi: 10.1016/j.pocean.2018.09.021
Dijoux L., Viard F., Payri C. (2014). The more we search, the more we find: Discovery of a new lineage and a new species complex in the genus Asparagopsis. PloS One 9, 1–13. doi: 10.1371/journal.pone.0103826
Dixon P. S. (1965). Perennation, vegetative propagation and algal life histories, with special reference to Asparagopsis and other Rhodophyta. Bot. Gothobg. 3, 67–74.
Feldmann J., Feldmann G. (1942). Recherches sur le Bonnemaisoniaces leur alternate de generations. Ann. Des. Sci. Nat. Bot. Série 11, 75–175.
Flores-Moya A., Fernández J. A., Niell F. X. (1996). Growth pattern, reproduction, and self-thinning in seaweeds. J. Phycol. 32, 767–769. doi: 10.1111/j.0022-3646.1996.00767.x
Fonck E., Martínez R., Vásquez J., Bulboa C. (2007). Factors that affect the re-attachment of Chondracanthus chamissoi (Rhodophyta, Gigartinales) thalli. J. Appl. Phycol. 20, 311. doi: 10.1007/s10811-007-9251-y
Fox J., Weisberg S. (2019) “car”: An R Companion to Applied Regression. Available at: https://socialsciences.mcmaster.ca/jfox/Books/Companion/.
Guerra-García J. M., Ros M., Izquierdo D., Soler-Hurtado M. M. (2012). The invasive Asparagopsis armata versus the native Corallina elongata: Differences in associated peracarid assemblages. J. Exp. Mar. Bio. Ecol. 416–417, 121–128. doi: 10.1016/j.jembe.2012.02.018
Guiry M. D., Dawes C. J. (1992). Daylength, temperature and nutrient control of tetrasporogenesis in Asparagopsis armata (Rhodophyta). J. Exp. Mar. Bio. Ecol. 158, 197–217. doi: 10.1016/0022-0981(92)90227-2
Häder D. P., Figueroa F. L. (1997). Photoecophysiology of marine macroalgae. Photochem. Photobiol. 66 (1), 1–14. doi: 10.1515/BOT.2001.004
Hartig L. L. F. (2021). “DHARMa”: Residual diagnostics for hierarchical (multi-level/mixed) regression Models. Available at: https://cran.r-project.org/web/packages/DHARMa/.
Haslin C., Pellegrini M. (2001). Culture medium composition for optimal thallus regeneration in the red alga Asparagopsis armata Harvey (Rhodophyta, Bonnemaisoniaceae). Bot. Mar. 44, 23–30. doi: 10.1515/BOT.2001.004
Hothorn T., Bretz F., Westfall P. (2008). Simultaneous inference in general parametric models. Biometrical J. 50, 346–363. doi: 10.1002/bimj.200810425
Juanes J. A., Puente A. (1993). Differential reattachment capacity of isomorphic life history phases of Gelidium sesquipedale. Hydrobiologia 260, 139–144. doi: 10.1007/BF00049012
Lenth R. V., Buerkner P., Herve M., Love J., Miguez F., Riebl H., et al. (2021) “emmeans”: Estimated Marginal Means, aka Least-Squares Means. Available at: https://cran.r-project.org/web/packages/emmeans.
Lüning K., Yarish C., Kirkman H. (1990). Seaweeds: their environment, biogeography, and ecophysiology (New York, USA: John Wiley & Sons). doi: 10.1002/aqc.3270010208
Mangiafico S. (2016) “rcompanion”: Functions to Support Extension Education Program Evaluation. Available at: https://cran.r-project.org/web/packages/rcompanion.
Martone P., Alyono M., Stites S. (2010). Bleaching of an intertidal coralline alga: untangling the effects of light, temperature, and desiccation. Mar. Ecol. Prog. Ser. 416, 57–67. doi: 10.3354/meps08782
Mata L., Silva J., Schuenhoff A., Santos R. (2006). The effects of light and temperature on the photosynthesis of the Asparagopsis armata tetrasporophyte (Falkenbergia rufolanosa), cultivated in tanks. Aquaculture 252, 12–19. doi: 10.1016/j.aquaculture.2005.11.045
Mata L., Silva J., Schuenhoff A., Santos R. (2007). Is the tetrasporophyte of Asparagopsis armata (Bonnemaisoniales) limited by inorganic carbon in integrated aquaculture?1. J. Phycol. 43, 1252–1258. doi: 10.1111/j.1529-8817.2007.00421.x
Neto A. I. (2000). Observations on the biology and ecology of selected macroalgae from the littoral of São Miguel (Azores). Bot. Mar. 43, 483–498. doi: 10.1515/BOT.2000.049
Ní Chualáin F., Maggs C. A., Saunders G. W., Guiry M. D. (2004). The invasive genus Asparagopsis (Bonnemaisoniaceae, Rhodophyta): Molecular systematics, morphology, and ecophysiology of Falkenbergia isolates. J. Phycol. 40, 1112–1126. doi: 10.1111/j.1529-8817.2004.03135.x
Otaíza R. D., Rodríguez C. Y., Cáceres J. H., Sanhueza Á.G. (2018). Fragmentation of thalli and secondary attachment of fragments of the agarophyte Gelidium lingulatum (Rhodophyta, Gelidiales). J. Appl. Phycol. 30, 1921–1931. doi: 10.1007/s10811-018-1391-8
Oza R. M. (1977). Culture studies on induction of tetraspores and their subsequent development in the red alga Falkenbergia rufolanosa (Harvey) Schmitz. Bot. Mar. 20, 29–32. doi: 10.1515/botm.1977.20.1.29
Pinheiro J., Bates D., DebRoy S., Sarkar D., R Core Team (2012) ‘nlme’: Linear and Nonlinear Mixed Effects Models. Available at: https://cran.r-project.org/package=nlme.
Preuss M., Nelson W. A., D’Archino R. (2022). Cryptic diversity and phylogeographic patterns in the Asparagopsis armata species complex (Bonnemaisoniales, Rhodophyta) from New Zealand. Phycologia 61, 89–96. doi: 10.1080/00318884.2021.2015223
Provasoli L. (1966). “Media and prospects for the cultivation of marine algae,” in Proceedings of the US–Japan conference (Hakone, Japan: Japanese Society for Plant Physiology), 63–75.
Quintano E., Díez I., Muguerza N., Figueroa F. L., Gorostiaga J. M. (2017). Bed structure (frond bleaching, density and biomass) of the red alga Gelidium corneum under different irradiance levels. J. Sea Res. 130, 180–188. doi: 10.1016/j.seares.2017.02.008
Quintano E., Díez I., Muguerza N., Figueroa F. L., Gorostiaga J. M. (2018). Depth influence on biochemical performance and thallus size of the red alga Gelidium corneum. Mar. Ecol. 39, 1–10. doi: 10.1111/maec.12478
Ramus J., Beale S. I., Mauzerall D., Howard K. L. (1976). Changes in photosynthetic pigment concentration in seaweeds as a function of water depth. Mar. Biol. 37, 223–229. doi: 10.1007/BF00387607
Raven P. H., Evert R. F., Eichhorn S. E. (2005). “Photosynthesis, light and life,” in Biology of Plants. Eds. Raven P. H., Evert R. F., Eichhorn S. E., Freeman W. H. (San Francisco, USA).
R Core Team (2023) R: A language and environment for statistical computing. Available at: https://www.r-project.org/.
Rubal M., Costa-Garcia R., Besteiro C., Sousa-Pinto I., Veiga P. (2018). Mollusc diversity associated with the non-indigenous macroalga Asparagopsis armata Harvey 1855 along the Atlantic coast of the Iberian Peninsula. Mar. Environ. Res. 136, 1–7. doi: 10.1016/j.marenvres.2018.02.025
Sainz-Villegas S., Sánchez-Astráin B., Puente A., Juanes J. A. (2023). Characterization of Gelidium corneum’s (Florideophyceae, Rhodophyta) vegetative propagation process under increasing levels of temperature and irradiance. Mar. Environ. Res. 187, 105966. doi: 10.1016/j.marenvres.2023.105966
Santelices B., Doty M. S. (1989). A review of Gracilaria farming. Aquaculture 78, 95–133. doi: 10.1016/0044-8486(89)90026-4
Schaffelke B., Smith J. E., Hewitt C. L. (2006). Introduced macroalgae – a growing concern. J. Appl. Phycol. 18, 529–541. doi: 10.1007/s10811-006-9074-2
Silva C. O., Lemos M. F. L., Gaspar R., Gonçalves C., Neto J. M. (2021). The effects of the invasive seaweed Asparagopsis armata on native rock pool communities: Evidences from experimental exclusion. Ecol. Indic. 125, 107463. doi: 10.1016/j.ecolind.2021.107463
Silva C. O., Novais S. C., Soares A. M. V. M., Barata C., Lemos M. F. L. (2020). Impacts of the invasive seaweed Asparagopsis armata exudate on energetic metabolism of rock pool invertebrates. Toxins 13. doi: 10.3390/toxins13010015
Svedelius N. (1933). On the development of Asparagopsis armata Harv. and Bonnemaisonia asparagoides (Woodw.) Ag.: A contribution to the cytology of the haplobiontic Rhodophyceae. Nov. Acta Regiae Soc Sci. Ups. Ser. IV, 1–61.
Wright J. T., Kennedy E. J., de Nys R., Tatsumi M. (2022). Asexual propagation of Asparagopsis armata gametophytes: fragmentation, regrowth and attachment mechanisms for sea-based cultivation. J. Appl. Phycol. 34, 2135–2144. doi: 10.1007/s10811-022-02763-6
Xu J., Gao K. (2008). Growth, pigments, UV-absorbing compounds and agar yield of the economic red seaweed Gracilaria lemaneiformis (Rhodophyta) grown at different depths in the coastal waters of the South China Sea. J. Appl. Phycol. 20, 681–686. doi: 10.1007/s10811-007-9247-7
Zanolla M., Altamirano M., Carmona R., de la Rosa J., Sherwood A., Andreakis N. (2014). Photosynthetic plasticity of the genus Asparagopsis (Bonnemaisoniales, Rhodophyta) in response to temperature: implications for invasiveness. Biol. Invasions 17, 1341–1353. doi: 10.1007/s10530-014-0797-8
Zanolla M., Altamirano M., Carmona R., de la Rosa J., Souza-Egipsy V., Sherwood A., et al. (2018a). Assessing global range expansion in a cryptic species complex: insights from the red seaweed genus Asparagopsis (Florideophyceae). J. Phycol. 54, 12–24. doi: 10.1111/jpy.12598
Zanolla M., Altamirano M., de la Rosa J., Niell F. X., Carmona R. (2018b). Size structure and dynamics of an invasive population of lineage 2 of Asparagopsis taxiformis (Florideophyceae) in the Alboran Sea. Phycol. Res. 66, 45–51. doi: 10.1111/pre.12189
Zanolla M., Carmona R., Mata L., de la Rosa J., Sherwood A., Barranco C. N., et al. (2022). Concise review of the genus Asparagopsis Montagne 1840. J. Appl. Phycol. 34, 1–17. doi: 10.1007/s10811-021-02665-z
Keywords: Asparagopsis, propagation, asexual reproduction, regrowth, invasive, climate change
Citation: Sainz-Villegas S, Sánchez-Astráin B, Puente A and Juanes JA (2024) Exploring the effects of temperature and light availability on the vegetative propagation processes of the non-native species Asparagopsis armata. Front. Mar. Sci. 10:1343353. doi: 10.3389/fmars.2023.1343353
Received: 23 November 2023; Accepted: 18 December 2023;
Published: 10 January 2024.
Edited by:
Ricardo A. Melo, University of Lisbon, PortugalReviewed by:
Mirko Mutalipassi, Stazione Zoologica Anton Dohrn, ItalyAjit Kumar Mohanty, Indira Gandhi Centre for Atomic Research (IGCAR), India
Copyright © 2024 Sainz-Villegas, Sánchez-Astráin, Puente and Juanes. This is an open-access article distributed under the terms of the Creative Commons Attribution License (CC BY). The use, distribution or reproduction in other forums is permitted, provided the original author(s) and the copyright owner(s) are credited and that the original publication in this journal is cited, in accordance with accepted academic practice. No use, distribution or reproduction is permitted which does not comply with these terms.
*Correspondence: Araceli Puente, YXJhY2VsaS5wdWVudGVAdW5pY2FuLmVz