- 1Climate Change Research Centre, University of New South Wales, Sydney, NSW, Australia
- 2The Australian Centre for Excellence in Antarctic Science, University of Tasmania, Hobart, TAS, Australia
- 3Institute for Marine and Antarctic Studies and Australian Antarctic Program Partnership, University of Tasmania, Hobart, TAS, Australia
In the context of past and present climate change, the Southern Ocean (SO) has been identified as a crucial region modulating the concentration of atmospheric CO2. The sustained upwelling of carbon-rich deep waters and inefficient nutrient utilization at the surface of the SO leads to an outgassing of natural CO2, while anthropogenic CO2 is entrained to depth during the formation of Antarctic Bottom water (AABW), Antarctic intermediate water (AAIW) and sub-Antarctic mode water (SAMW). Changes to the SO circulation resulting from both dynamic and buoyancy forcing can alter the rate of upwelling as well as formation and subsequent transport of AABW, AAIW and SAMW, thus impacting the air-sea CO2 exchange in the SO. Models of all complexity robustly show that stronger southern hemispheric (SH) westerlies enhance SO upwelling, thus leading to stronger natural CO2 outgassing, with a sensitivity of 0.13 GtC/yr for a 10% increase in SH westerly windstress. While the impact of changes in the position of the SH westerly winds was previously unclear, recent simulations with high-resolution ocean/sea-ice/carbon cycle models show that a poleward shift of the SH westerlies also enhances natural CO2 outgassing with a sensitivity of 0.08GtC/yr for a 5° poleward shift. While enhanced AABW transport reduces deep ocean natural DIC concentration and increases surface natural DIC concentration, it acts on a multi-decadal timescale. Future work should better constrain both the natural and anthropogenic carbon cycle response to changes in AABW and the compound impacts of dynamic and buoyancy changes on the SO marine carbon cycle.
1 Introduction
Despite covering only about 25% of the world’s ocean surface, the Southern Ocean (SO, south of 35°S) is one of today’s largest sink of both anthropogenic carbon and excess heat, accounting for ∼40% of the oceanic anthropogenic carbon uptake and ∼72% of the excess heat uptake (Sabine et al., 2004; Mikaloff-Fletcher et al., 2006; Shi et al., 2018). This oversized role is due to the unique overturning circulation of the SO that brings deep water up to the surface and leads to the formation of deep and intermediate water masses. A key driver of this overturning are the strong westerly winds that peak in strength around 55°S and are bounded by easterly winds on their Antarctic and subtropical flanks. These winds create a divergent surface flow and steeply tilted isopycnals that outcrop south of the maximum westerly wind stress to allow old, nutrient rich Circumpolar Deep Water (CDW) to upwell from mid-depths to the surface (Figure 1A). Due to Ekman transport within the westerly wind belt, Antarctic surface waters move equatorwards, freshen and warm. As a result, there is an outgassing of natural CO2 south of the sub-Antarctic front and particularly south of the polar front. As CDW equilibrates with the atmosphere, its dissolved inorganic carbon (DIC) is consumed by phytoplankton, thus decreasing the surface water pCO2, leading to an oceanic uptake of atmospheric CO2, a process referred to as the biological pump (Talley, 2013).
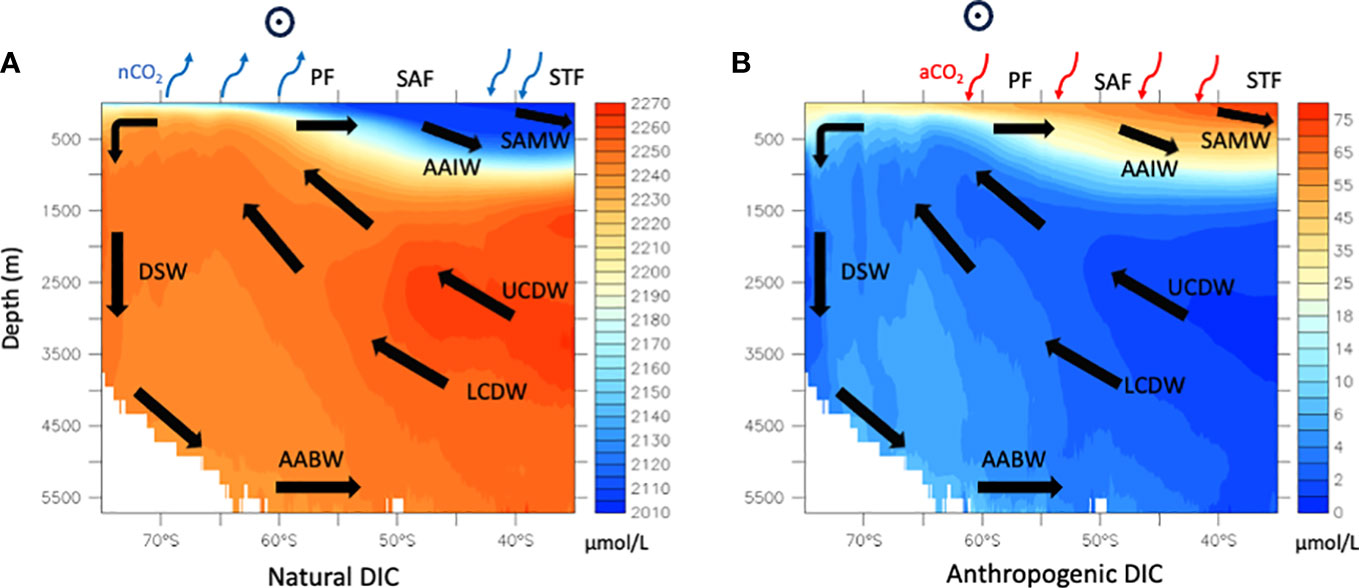
Figure 1 Schematic showing zonally averaged Southern Ocean (A) natural DIC and (B) anthropogenic DIC concentration (µmol/L) averaged over years 2017-2021 in the ACCESS-OM2-01 simulation. Blue and red arrows at the ocean’s surface represent the natural and anthropogenic CO2 fluxes, respectively, and the westerly wind is represented by a circle above. PF, SAF and STF are the positions of the Polar Front, Subantarctic Front and Subtropical Front, respectively. Thick black arrows in the ocean interior represent the circulation of water masses: Dense Shelf Water (DSW), Antarctic Bottom Water (AABW), Lower Circumpolar Deep Water (LCDW), Upper Circumpolar Deep Water (UCDW), Antarctic Intermediate Water (AAIW), and Subantarctic Mode Water (SAMW).
The CDW that rises to the SO surface is replaced by downward flows of surface waters in other regions. In the current context of elevated atmospheric CO2 concentration, these downward flows entrain carbon, and particularly anthropogenic carbon, as well as heat in the SO interior (Frölicher et al., 2015; Gruber et al., 2019), primarily within Antarctic Intermediate Water (AAIW), but also within Antarctic Bottom Water (AABW) and sub-Antarctic Mode Water (SAMW) (Figure 1B). AAIW subducts along isopycnals into the SO interior equatorward of the sub-Antarctic front where wind driven surface flows converge. Winter deepening of the mixed layer north of the Antarctic Circumpolar Current (ACC) leads to the formation of SAMW, which overlays AAIW (Morrison et al., 2022). Closer to Antarctica, dense shelf waters, the precursor of AABW are formed on the Antarctic continental shelf in the Ross Sea, Weddell Sea, Prydz Bay, and Adelie Land (Purkey et al., 2018), through brine rejection and ocean/ice-shelf interactions.
Observational products suggest that the SO total (sum of anthropogenic and natural) CO2 fluxes exhibit large decadal-scale variability (LeQuéré et al., 2007; Matear and Lenton, 2008; Landschützer et al., 2015; Bushinsky et al., 2019; Gruber et al., 2019; Keppler and Landschützer, 2019; Gruber et al., 2023), with a lower than expected total CO2 uptake in the 1990s and early 2000s (LeQuéré et al., 2007; Landschützer et al., 2015; Gruber et al., 2019) and probably during the mid-2010s (Bushinsky et al., 2019; Gruber et al., 2019; Keppler and Landschützer, 2019; Landschützer et al., 2020). The main hypothesis to explain the stagnation in the CO2 uptake observed in the 1990s and early 2000s is the concurrent positive SAM trend (LeQuéré et al., 2007; Lovenduski et al., 2008; Resplandy et al., 2015; Gruber et al., 2023), which corresponds to a strengthening and poleward shift of the SH westerlies. As the CO2 uptake re-invigorated, particularly between 2002 and 2012, other hypotheses to explain the variability emerged such as regional wind variability associated with the zonal wave number 3 pattern (Keppler and Landschützer, 2019), solubility effects (Landschützer et al., 2015) and changes in atmospheric CO2 growth rate (McKinley et al., 2020). While hindcast experiments support a dominant role of the SAM in modulating the SO CO2 fluxes (Gruber et al., 2019; Hauck et al., 2020; Menviel et al., 2023), they underestimate the observed SO CO2 flux variability. This could be due to an under-estimation of Southern Ocean stratification (de Lavergne et al., 2014), potentially linked to enhanced Antarctic basal melt rates (Adusumilli et al., 2020), which would weaken AABW formation and transport (Li, 2023). The individual and compound roles of changes in SH westerly wind strength and position, and AABW formation and transport on the marine carbon cycle need to be fully understood to constrain changes in SO CO2 fluxes.
While overall the SO has mitigated the rise in atmospheric CO2 since at least the 1980s, SO CO2 outgassing has been suggested to induce an increase in atmospheric CO2 during deglaciations as well as past abrupt climate change events (Anderson et al., 2009; Skinner et al., 2010; Watson et al., 2015; Rae et al., 2018; Shuttleworth et al., 2021; Gray et al., 2023). SO stratification during glacial periods, potentially arising from a large sea-ice cover, weaker and/or equatorward shifted SH westerlies would allow a carbon accumulation in the deep ocean (Toggweiler et al., 2006; Menviel et al., 2017). The breakdown of this stratification during deglaciations and Antarctic warm events, i.e. millennial-scale warm periods over Antarctica and the Southern Ocean (Buizert and Members, 2015), would lead to a SO CO2 outgassing and an increase in atmospheric CO2 of up to 20 ppm (Ahn and Brook, 2008; Marcott et al., 2014; Menviel et al., 2018; Bauska et al., 2021). An improved understanding of the interplay between SO circulation and marine carbon cycle is however needed to refine our understanding of these past climate change events.
Here we provide a perspective on past, present and future relationship between changes in atmospheric CO2 and SO circulation arising from both changes in surface wind and buoyancy. We also present recent advances and future work.
2 Southern hemispheric westerly winds
There is a general consensus that stronger SH westerly winds enhance SO natural CO2 outgassing by increasing the upward transport of DIC-rich CDW, thus leading to an increase in atmospheric CO2 (Gottschalk et al., 2020). This DIC increase mostly arises from the advective upwelling of CDW along isopycnals into the mixed layer, although increased vertical diffusion at the base of the mixed layer could also significantly contribute to the higher surface DIC (Dufour et al., 2013). Enhanced upwelling of CDW can also enhance primary production, which acts to increase the export of organic carbon to depth, thus lowering atmospheric CO2 (Menviel, 2008; Hauck et al., 2013). However, most studies found this negative feedback to only have a small impact on the CO2 fluxes (Dufour et al., 2013) and to mostly impact carbon uptake north of the sub-Antarctic front (Menviel et al., 2023).
Depending on the pCO2 of the upwelled water, the magnitude of the negative biological feedback as well as the resolution of the ocean model used, which can impact the outcrop area of deep water and SO circulation response, a 10% strengthening of the SH westerly wind stress has been found to lead to a 2.7 ppm +/- 0.5 ppm CO2 increase (Menviel et al., 2008; Menviel et al., 2015; Huiskamp et al., 2016; Lauderdale et al., 2017; Menviel et al., 2018; Gottschalk et al., 2019). While Toggweiler et al. (2006) hypothesized that a poleward shift of the SH westerly should enhance the SO CO2 outgassing, simulations performed with coarse resolution models led to mixed results, with a poleward SH westerly shift leading to both an increase and a decrease in atmospheric CO2 depending on the model used (Gottschalk et al., 2019).
Most modelling studies that assessed the impact of SH westerly changes on the marine carbon cycle were performed with models that could not resolve mesoscale eddies (Lenton and Matear, 2007; Lovenduski et al., 2007; Lovenduski et al., 2008; Menviel, 2008; Tschumi et al., 2011; Dufour et al., 2013; Hauck et al., 2013; Völker and Köhler, 2013; Huiskamp et al., 2016; Lauderdale et al., 2017). Mesoscale eddies are prevalent in the SO, particularly downstream of topographic features that intercept the path of the Antarctic Circumpolar Current (ACC) (Sokolov and Rintoul, 2009; Frenger et al., 2015). In the SO, eddy induced transports oppose the Ekman-driven overturning transport via eddy compensation. Eddy-permitting and eddy-rich models have thus highlighted the role of eddy compensation in mitigating the Southern Ocean overturning change due to increased wind stress (Meredith et al., 2012; Morrison and Hogg, 2013). It has further been suggested that climate models that did not use a variable eddy parametrization coefficient most likely overestimated the wind-driven response (Gent, 2016).
A recent study (Menviel et al., 2023) assessed the impact of changes in the strength and position of the SH westerlies on SO CO2 fluxes, associated with a positive trend of the SAM over the last 42 years in a global eddy-rich model (ACCESS-OM2-01, 0.1° resolution, 75 vertical levels) forced by the 55-year Japanese Reanalysis for driving oceans (JRA55-do) (Tsujino et al., 2018). This simulation shows that positive phases of the SAM (stronger and poleward shifted SH westerlies) lead to enhanced SO CO2 outgassing south of the polar front, and reduced CO2 uptake north of the sub-Antarctic front. The CO2 outgassing is maximum over topography features such as over the eastern part of the Southeast Indian Ridge, east of the Drake Passage and over the Southwest Indian Ridge, which could be due to enhanced eddy mixing over topography linked to the merging of multiple jets.
Here, we synthesize these results and merge them with other experiments performed with the suite of ACCESS-OM2 models. This includes the ACCESS-OM2 (1° and 50 vertical levels) and ACCESS-OM2-01 (0.1° and 75 vertical levels) forced by the JRA55-do reanalysis over the period 1958 to 2021 (Tsujino et al., 2018; Kiss et al., 2020) as well as four idealized perturbation experiments performed with the ACCESS-OM2-025 (0.25° and 50 vertical levels), in which the SH near surface wind speeds are abruptly modified (Hogg et al., 2017). The zonally uniform and temporally steady perturbations are applied to the CORE-NYF 6-hourly wind field between 27°S and 70°S with smoothing within 5° latitude of the perturbation boundaries. The perturbations include i) a 20% increase in SH westerly windstress between 32°S and 65°S, ii) a 20% increase with a 4° poleward shift, iii) a ∼16% decrease, and iv) a ∼4° equatorward shift of the SH westerly windstress. The first three idealized experiments are integrated for 42 years, while the last one is run for 125 years (Gray et al., 2023).
In all the simulations performed with the suite of ACCESS-OM2 models, stronger and poleward shifted SH westerlies lead to enhanced CO2 outgassing south of the polar front (Figure 1). A robust linear relationship is obtained between the natural SO CO2 flux and mean SO windstress (Figure 2A). The SO CO2 outgassing increases by 13 ± 3 GtC/yr per N/m2 (therefore ∼0.13 GtC/yr per 10% windstress increase). These results show that an eddy-rich model displays a similar sensitivity to changes in SO upwelling than a coarse-resolution model with a varying isopycnal thickness diffusivity (Kiss et al., 2020).
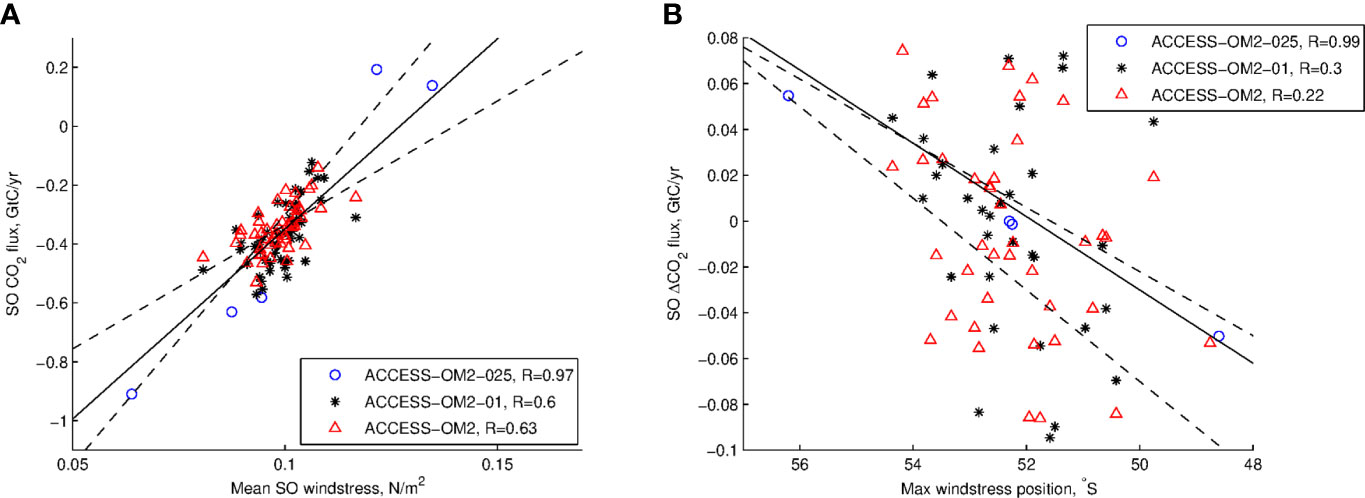
Figure 2 Relationship between SO natural CO2 flux (south of 35°S) and SO windstress as simulated in experiments performed with ACCESS-OM2 models at 0.1° (black stars, ACCESS-OM2-01), 0.25° (blue circles, ACCESS-OM2-025) and 1° (red triangles, ACCESS-OM2) resolution. (left) SO natural CO2 flux as a function of SO windstress (averaged between 35°S-65°S), with a slope of 13 ± 3 GtC.m2/N across all experiments and R values of 0.97, 0.6 and 0.63 for the experiments performed with the ACCESS-OM2-025, ACCESS-OM2-01 and ACCESS-OM2, respectively. (right) SO residual natural CO2 flux as a function of location of the maximum SO windstress with a slope of 0.016 ± 0.04 GtC/° latitude. The R values are of 0.99, 0.3 and 0.22 for the experiments performed with the ACCESS-OM2-025, ACCESS-OM2-01 and ACCESS-OM2, respectively. The residual natural CO2 flux was calculated by subtracting the impact of changes in the strength of the westerly windstress as per the relationship defined on the left.
While the relationship between the position of the maximum SO windstress and SO CO2 fluxes is weak, there is still a significant relationship, with enhanced SO CO2 outgassing as the SH westerlies shift poleward, with a sensitivity of 0.016 ± 0.04 GtC/° latitude (Figure 2B). As the SH westerlies move poleward, so does the divergence, which leads to a steepening of heavier isopycnals, thus increasing the upwelling pathway of carbon-rich deep-waters (Toggweiler et al., 2006; Gray et al., 2023).
While many modelling studies assessed the impact of SH westerly changes on natural CO2 fluxes, the impact on anthropogenic CO2 fluxes has been less studied. Nevertheless, the results suggest that stronger SH westerlies also enhance the uptake of anthropogenic CO2 in the SO, but the amplitude of this enhanced SO anthropogenic CO2 uptake is only one third of the associated enhanced natural CO2 outgassing (Lovenduski et al., 2008; Menviel et al., 2023). As such stronger SH westerlies reduce the total SO CO2 uptake.
3 Antarctic bottom water transport
AABW is the densest large-scale water mass in the global ocean and it fills roughly 35% of the global ocean volume. As AABW moves northward in the abyss, it upwells through diapycnal mixing in the sub-tropics and tropics into deep waters (Talley, 2013). These deep waters, i.e. North Atlantic Deep Water (NADW), Indian Deep Water (IDW) and Pacific Deep Water (PDW) are then often upwelled at the surface of the Southern Ocean as CDW. Changes in AABW formation and transport, can arise from changes in SH westerlies, but also changes in sea-ice cover and polynya opening. For example, a poleward strengthening of the SH westerlies in the ACCESS-OM2-025 enhances the northward transport of sea-ice, thus leading to enhanced AABW formation and transport (Hogg et al., 2017; Menviel et al., 2018). On the contrary, stronger polar easterlies increase the build-up of sea ice over the continental shelf, which reduces AABW formation (Jeong et al., 2022; Schmidt et al., 2023). Changes in buoyancy forcing resulting from changes in sea-ice formation can also affect AABW formation and transport. Polynya openings in the Antarctic coastal zone trigger large deep-ocean convection events, which strengthen the lower overturning cell (Hogg et al., 2017; Jeong et al., 2022). On the other hand, reduced sea-ice formation and associated warmer conditions can decrease the density of surface waters, thus increasing stratification and decreasing the formation of AABW (Choudhury et al., 2022; Zhou et al., 2023).
In turn, changes in AABW formation and transport affect the oceanic DIC concentration and atmospheric CO2. Figure 3 shows zonally averaged DIC anomalies in two experiments which feature stronger AABW transport, but one is generated by surface SO salinification in an Earth system model of intermediate complexity, LOVECLIM (Menviel et al., 2015) (△AABW=+14 Sv, Figures 3A, B) and the other by a poleward strengthening of the SH westerlies in the ACCESS-OM2-025 (△AABW=+11 Sv, Figures 3C–F). In both cases, enhanced AABW transport leads to a natural DIC decrease in the deep ocean (Figures 3A, C). This arises i) from a decrease in remineralized DIC (Figures 3B, D), i.e. a decrease in the amount of carbon that can accumulate in the deep ocean resulting from a lower residence time of AABW; and ii) from a larger proportion of southern sourced waters with lower DIC concentration being advected to the deep North Pacific. The deep North Pacific indeed host the oldest watermass with the highest DIC concentration. As this enhanced lower overturning cell reduces deep ocean DIC concentration, it leads to an increase in DIC concentration above 1500m depth north of 40°S and in the mixed layer of the Southern Ocean (Figures 3A, C), thus resulting in a weaker vertical DIC gradient.
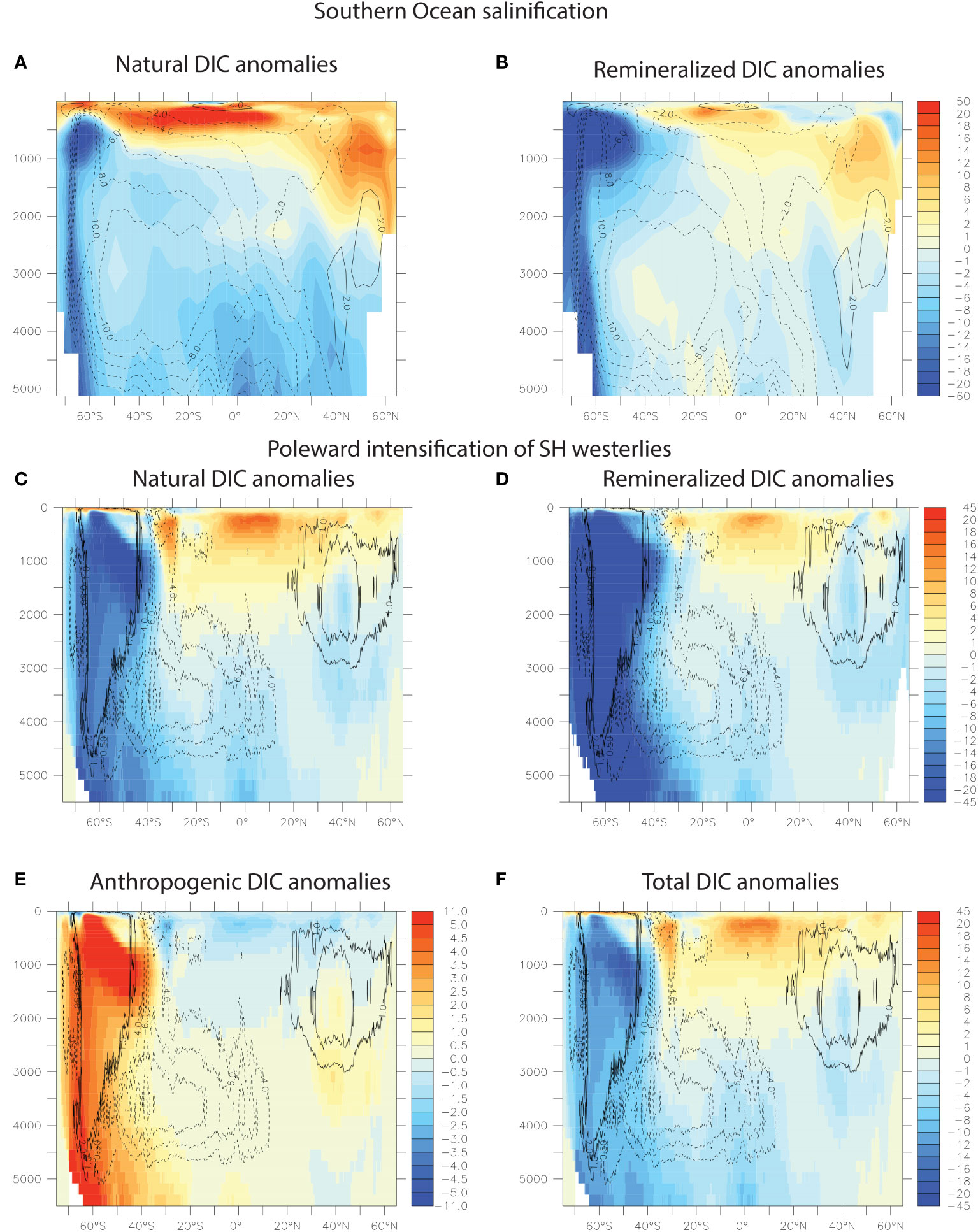
Figure 3 Zonally-averaged (A) natural DIC and (B) remineralized DIC anomalies (mmol/m3) after 100 years for the SO salinification experiment performed with the Earth system model of intermediate complexity LOVECLIM (Menviel et al., 2015). Zonally-averaged (C) natural DIC, (D) remineralized DIC, (E) anthropogenic DIC and (F) total DIC anomalies (mmol/m3) after 50 years for the poleward intensified SH westerlies (+20% and 4° poleward shift) experiment performed with the ACCESS-OM2-025 (Menviel et al., 2018). Overlaid are the global overturning streamfunction anomalies (Sv).
The differences in simulated DIC anomalies between the SO salinification and SH westerly poleward strengthening experiments could be attributed i) to the different length of the experiments (100 years vs 50 years): the DIC anomaly needs more than 50 years to reach the deep North Pacific; ii) the poleward strengthening of the winds enhance the upper overturning cell (Hogg et al., 2017) (Figure 3C); and iii) the poleward strengthening of the SH westerlies leads to the opening of a polynya, which triggers a large deep-ocean convection event in SO.
Even though the preformed carbon entrained in the downwelling branch of AABW can increase, thus also leading to a significant increase in anthropogenic DIC concentration in the Southern Ocean (Figure 3E), this cannot compensate for the loss of natural DIC (Figure 3C) thus leading to negative total DIC anomalies (Figure 3F).
4 Discussion
Both dynamical and buoyancy changes can impact SO circulation and thus the SO CO2 air-sea gas exchange, as well as the oceanic DIC distribution. As changes in the strength and position of the SH westerlies modulate the upwelling of carbon-rich deep-waters to the surface of the SO, it has long been suggested that changes in the SH westerlies could modulate the atmospheric CO2 concentration (Russell et al., 2006; Toggweiler et al., 2006). While coarse resolution models consistently showed that stronger SH westerlies enhance the SO natural CO2 outgassing (Gottschalk et al., 2019), the lack of representation of mesoscale eddies within these modelling framework cast doubts on this result. In addition, due to the misrepresentation of AABW formation and the coarse resolution of the models used, the impact of changes in the position of SH westerlies on SO CO2 flux was debated (Gottschalk et al., 2019). New results obtained with an eddy-rich model, which correctly forms dense-shelf water, show that mesoscale eddies do not significantly diminish the carbon cycle response to the wind-driven circulation and that poleward shifted SH westerlies enhance the SO natural CO2 outgassing (Menviel et al., 2023).
In line with this, present-day hindcast simulations have suggested that changes in SH westerlies associated with the SAM were the dominant factor modulating the SO air-sea CO2 exchange (Lenton and Matear, 2007; Lovenduski et al., 2007; Lovenduski et al., 2008). However, these simulations have not been able to reproduce the observational-based estimates contemporary CO2 fluxes in SO (Lequere et al., 2018; Gruber et al., 2019; Hauck et al., 2020), even with eddy-rich ocean models (Menviel et al., 2023) forced with atmospheric reanalyses. Gloege et al. (2021) found that the decadal-scale variability in SO contemporary CO2 flux could be overestimated by 30% due to undersampling. On the other hand, hindcast simulations could also underestimate the variability due to an overestimation of negative feedbacks (e.g. biological). The impact of changes in the strength and position of the SH westerlies should be further studied in eddy-permitting or eddy-resolving models that include complex ecosystem models.
The underestimation of the decadal-scale CO2 variability could also be due to a poor representation of changes in buoyancy forcing, for example arising from enhanced Antarctic basal melt rates (Adusumilli et al., 2020), that could weaken AABW (Li, 2023). Buoyancy gain on the Antarctic shelf through either increased meltwater or warming will weaken the formation of AABW, and its subsequent transport (Menviel et al., 2010; Bintanja et al., 2013; Li, 2023). As a water mass ages, it accumulates remineralized nutrients. Weaker AABW transport increases the remineralized nutrient content in the deep ocean, which has been shown to increase the vertical DIC gradient and reduce SO natural CO2 outgassing (Ito and Follows, 2005; Menviel et al., 2015). Future hindcast simulations should thus also include changes in Antarctic basal melt rates, with the caveat that the present-day carbon cycle could still be responding to changes that occurred decades ago.
In addition, as most models used to study the impact of changes in the oceanic circulation form AABW through deep-ocean convection, the impact of changes in dense-shelf water formation on the marine carbon cycle needs to be studied. In particular, the transport pathway of carbon from the abyss into deep waters and back to the surface as a function of bottom and deep water masses transport and volume should be better constrained. While both changes in SO upwelling strength and AABW formation can impact atmospheric CO2, the marine carbon cycle response timescales are different: the SH westerlies impact the SO CO2 outgassing within just a few months (Lenton and Matear, 2007; Menviel et al., 2023), while changes in AABW formation and transport only significantly impact air-sea CO2 fluxes on a multi-decadal timescale (Menviel et al., 2018).
Finally, buoyancy and dynamic SO forcing can oppose each other, thus leading to a complex time-evolving oceanic circulation and thus marine carbon cycle response. In the context of past, present and future climate change, the individual and compound effects of changes in SH westerly, sea-ice formation and melt, formation of SO watermasses and the marine carbon cycle need to be better constrained to understand the resultant changes in atmospheric CO2.
Data availability statement
The datasets presented in this study can be found in the Dryad repository https://datadryad.org/stash/share/E21b0EPD0x4ZXYb5JUt41sM42fEqhdA40hl8bnC1-2Y.
Author contributions
LM: Conceptualization, Formal Analysis, Investigation, Methodology, Writing – original draft. PS: Conceptualization, Methodology, Writing – review & editing.
Funding
The author(s) declare financial support was received for the research, authorship, and/or publication of this article. This project was supported by the Australian Research Council (ARC), including grants FT180100606, FT190100413, and SR200100008.
Acknowledgments
The authors thank the Consortium for Ocean-Sea Ice Modelling in Australia (COSIMA; http://www.cosima.org.au) for making the ACCESS-OM2 suite of models available at https://github.com/COSIMA/accessom2. Model runs were undertaken with the assistance of resources from the National Computational Infrastructure (NCI), which is supported by the Australian Government.
Conflict of interest
The authors declare that the research was conducted in the absence of any commercial or financial relationships that could be construed as a potential conflict of interest.
Publisher’s note
All claims expressed in this article are solely those of the authors and do not necessarily represent those of their affiliated organizations, or those of the publisher, the editors and the reviewers. Any product that may be evaluated in this article, or claim that may be made by its manufacturer, is not guaranteed or endorsed by the publisher.
References
Adusumilli S., Fricker H., Medley B., Padman L., Siegfried M. (2020). Interannual variations in meltwater input to the Southern Ocean from Antarctic ice shelves. Nat. Geosciences 13, 616–620. doi: 10.1038/s41561-020-0616-z
Ahn J., Brook E. (2008). Atmospheric CO2 and climate on millennial time scales during the last glacial period. Science 322, doi:10.1126/science.1160832.
Anderson R. F., Ali S., Bradtmiller L., Nielsen S., Fleisher M., Anderson B., et al. (2009). Wind-driven upwelling in the Southern Ocean and the deglacial rise in Atmospheric CO2. Science 323, 1443–1448.
Bauska T., Brook E., Marcott S. (2021). Abrupt changes in the global carbon cycle during the last glacial period. Nat. Geosci. 14, 91–96. doi: 10.1038/s41561-020-00680-2
Bintanja R., van Oldenborgh G., Drijfhout S. (2013). Important role for ocean warming and increased ice-shelf melt in antarctic sea-ice expansion. Nat. Geosci. 6, 376–379.
Buizert C., Members W. D. P. (2015). Precise interpolar phasing of abrupt climate change during the last ice age. Nature 520, 661–665. doi: 10.1038/nature14401
Bushinsky S. M., Landschützer P., Rödenbeck C., Gray A. R., Baker D., Mazloff M. R., et al. (2019). Reassessing southern ocean air-sea CO2 flux estimates with the addition of biogeochemical float observations. Global Biogeochemical Cycles 33, 1370–1388. doi: 10.1029/2019GB006176
Choudhury D., Menviel L., Meissner K. J., Yeung N. K. H., Chamberlain M., Ziehn T. (2022). Marine carbon cycle response to a warmer southern ocean: the case of the last interglacial. Climate Past 18, 507–523. doi: 10.5194/cp-18-507-2022
de Lavergne C., Palter J., Galbraith E., Bernardello R., Marinov I. (2014). Cessation of deep convection in the open Southern Ocean under anthropogenic climate change. Nat. Climate Change 4, 278–282. doi: 10.1038/NCLIMATE2132
Dufour C. O., Sommer J. L., Gehlen M., Orr J. C., Molines J.-M., Simeon J., et al. (2013). Eddy compensation and controls of the enhanced sea-to-air CO2 flux during positive phases of the Southern Annular Mode. Global Biogeochemical Cycles 27, 950–961. doi: 10.1002/gbc.20090
Frenger I., Münnich M., Gruber N., Knutti R. (2015). Southern Ocean eddy phenomenology. J. Geophysical Research: Oceans 120, 7413–7449. doi: 10.1002/2015JC011047
Frölicher T. L., Sarmiento J. L., Paynter D. J., Dunne J. P., Krasting J. P., Winton M. (2015). Dominance of the southern ocean in anthropogenic carbon and heat uptake in CMIP5 models. J. Climate 28, 862–886. doi: 10.1175/JCLI-D-14-00117.1
Gent P. R. (2016). Effects of southern hemisphere wind changes on the meridional overturning circulation in ocean models. Annu. Rev. Mar. Sci. 8, 79–94. doi: 10.1146/annurev-marine-122414-033929
Gloege L., McKinley G. A., Landschützer P., Fay A. R., Frölicher T. L., Fyfe J. C., et al. (2021). Quantifying errors in observationally based estimates of ocean carbon sink variability. Global Biogeochemical Cycles 35, e2020GB006788. doi: 10.1029/2020GB006788
Gottschalk J., Battaglia G., Fischer H., Frölicher T. L., Jaccard S. L., Jeltsch-Thömmes A., et al. (2019). Mechanisms of millennial-scale atmospheric co2 change in numerical model simulations. Quaternary Sci. Rev. 220, 30–74. doi: 10.1016/j.quascirev.2019.05.013
Gottschalk J., Skinner L. C., Jaccard S. L., Menviel L., Nehrbass-Ahles C., Waelbroeck C. (2020). Southern Ocean link between changes in atmospheric CO2 levels and northern-hemisphere climate anomalies during the last two glacial periods. Quaternary Sci. Rev. 230, 106067. doi: 10.1016/j.quascirev.2019.106067
Gray W. R., de Lavergne C., Jnglin Wills R. C., Menviel L., Spence P., Holzer M., et al. (2023). Poleward shift in the southern hemisphere westerly winds synchronous with the deglacial rise in co2. Paleoceanography Paleoclimatology 38, e2023PA004666. doi: 10.1029/2023PA004666
Gruber N., Bakker D., DeVries T., Gregor L., Hauck J., Landschützer P., et al. (2023). Trends and variability in the ocean carbon sink. Nat.Rev Earth Environ. 4, 119–134. doi: 10.1038/s43017-022-00381-x
Gruber N., Landschützer P., Lovenduski N. (2019). The variable Southern Ocean carbon sink. Annu. Rev. Mar. Sci. 11, 159–186.
Hauck J., Völker C., Wang T., Hoppema M., Losch M., Wolf-Gladrow D. A. (2013). Seasonally different carbon flux changes in the southern ocean inresponse to the southern annular mode. Global Biogeochemical Cycles 27, 1236–1245. doi: 10.1002/2013GB004600
Hauck J., Zeising M., Le Quéré C., Gruber N., Bakker D. C. E., Bopp L., et al. (2020). Consistency and challenges in the ocean carbon sink estimate for the global carbon budget. Front. Mar. Sci. 7. doi: 10.3389/fmars.2020.571720
Hogg A., Spence P., Saenko O., Downes S. (2017). The energetics of southern ocean upwelling. J. Phys. Oceanogr. 47, 135–153.
Huiskamp W. N., Meissner K. J., d’Orgeville M. (2016). Competition between ocean carbon pumps in simulations with varying Southern Hemisphere westerly wind forcing. Climate Dynamics 46, 3463–3480. doi: 10.1007/s00382-015-2781-0
Ito T., Follows M. (2005). Preformed phosphate, soft tissue pump and atmospheric CO2. J. Mar. Res. 63, 813–839.
Jeong H., Turner A. K., Roberts A. F., Veneziani M., Price S. P., Asay-Davis X. S., et al. (2022). Southern ocean polynyas and dense water formation in a high-resolution, coupled earth system model. Cryosphere Discussions 2022, 1–32. doi: 10.5194/tc-2022-133
Keppler L., Landschützer P. (2019). Regional wind variability modulates the southern ocean carbon sink. Sci. Rep. 9, 7384. doi: 10.1038/s41598-019-43826-y
Kiss A. E., Hogg A. M., Hannah N., Boeira Dias F., Brassington G. B., Chamberlain M. A., et al. (2020). ACCESS-OM2 v1.0: a global ocean–sea ice model at three resolutions. Geoscientific Model. Dev. 13, 401–442. doi: 10.5194/gmd-13-401-2020
Landschützer P., Gruber N., Bakker D. (2020). An observation-based global monthly gridded sea surface pCO2 product from 1982 onward and its monthly climatology (NCEI Accession 0160558). (NOAA: NOAA National Centers for Environmental Information). doi: 10.7289/V5Z899N6
Landschützer P., Gruber N., Haumann F. A., Rödenbeck C., Bakker D. C. E., van Heuven S., et al. (2015). The reinvigoration of the Southern Ocean carbon sink. Science 349, 1221–1224. doi: 10.1126/science.aab2620
Lauderdale J. M., Williams R. G., Munday D. R., Marshall D. P. (2017). The impact of Southern Ocean residual upwelling on atmospheric CO2 on centennial and millennial timescales. Climate Dynamics 48, 1611–1631. doi: 10.1007/s00382-016-3163-y
Lenton A., Matear R. (2007). Role of the southern annular mode (SAM) in southern ocean CO2 uptake. Global Biogeochemical Cycles 21. doi: 10.1029/2006GB002714
Lequere C., Andrew R. M., Friedlingstein P., Sitch S., Hauck J., Pongratz J., et al. (2018). Global carbon budget 2018. Earth System Sci. Data 10, 2141–2194. doi: 10.5194/essd-10-2141-2018
LeQuéré C., Rödenbeck C., Buitenhuis E., Conway T., Langenfelds R., Gomez A., et al. (2007). Saturation of the Southern Ocean CO2 sink due to recent climate change. Science 316, 1735–1738.
Li E. M. H. A. S. R. A. M. (2023). Abyssal ocean overturning slowdown and warming driven by Antarctic meltwater. Nature 615, 841–847. doi: 10.1038/s41586-023-05762-w
Lovenduski N., Gruber N., Doney S. (2008). Toward a mechanistic understanding of the decadal trends in the Southern Ocean carbon sink. Global Biogeochemical Cycles 22GB3016. doi: 10.1029/2007GB003139
Lovenduski N., Gruber N., Doney S., Lima I. (2007). Enhanced CO2 outgassing in the Southern Ocean from a positive phase of the Southern Annular Mode. Global Biogeochemical Cycles 21. doi: 10.1029/2006GB002900
Marcott S., Bauska T., Buizert C., Steig E., Rosen J., Cuffey K., et al. (2014). Centennial-scale changes in the global carbon cycle during the last deglaciation. Nature 514, 616–619. doi: 10.1038/nature13799
Matear R., Lenton A. (2008). Impact of historical climate change on the southern ocean carbon cycle. J. Climate 21, 5820–5834. doi: 10.1175/2008JCLI2194.1
McKinley G. A., Fay A. R., Eddebbar Y. A., Gloege L., Lovenduski N. S. (2020). External forcing explains recent decadal variability of the ocean carbon sink. AGU Adv. 1, e2019AV000149. doi: 10.1029/2019AV000149
Menviel L. (2008). Climate-carbon cycle interactions on millennial to glacial timescales as simulated by a model of intermediate complexity, LOVECLIM. Ph.D. thesis (University of Hawai’i).
Menviel L., Mouchet A., Meissner K., Joos F., England M. (2015). Impact of oceanic circulation changes on atmospheric δ13CO2. Global Biogeochemical Cycles 29, 1944–1961. doi: 10.1002/2015GB005207
Menviel L. C., Spence P., Kiss A. E., Chamberlain M. A., Hayashida H., England M. H., et al. (2023). Enhanced Southern Ocean CO2 outgassing as a result of stronger and poleward shifted southern hemispheric westerlies. EGUsphere 2023, 1–27. doi: 10.5194/egusphere-2023-390
Menviel L., Spence P., Yu J., Chamberlain M., Matear R., Meissner K., et al. (2018). Southern Hemisphere westerlies as a driver of the early deglacial atmospheric CO2 rise. Nat. Commun. 92503. doi: 10.1038/s41467-018-04876-4
Menviel L., Timmermann A., Mouchet A., Timm O. (2008). Climate and marine carbon cycle response to changes in the strength of the southern hemispheric westerlies. Paleoceanography 23. doi: 10.1029/2008PA001604
Menviel L., Timmermann A., Mouchet A., Timm O. (2010). Climate and biogeochemical response to a rapid melting of the West-Antarctic Ice Sheet during interglacials and implications for future climate. Paleoceanography 25, doi:10.1029/2009PA001892.
Menviel L., Yu J., Joos F., Mouchet A., Meissner K., England M. (2017). Poorly ventilated deep ocean at the Last Glacial Maximum inferred from carbon isotopes: a data-model comparison study. Paleoceanography 32, 2–17. doi: 10.1002/2016PA003024
Meredith M. P., Garabato A. C. N., Hogg A. M., Farneti R. (2012). Sensitivity of the overturning circulation in the southern ocean to decadal changes in wind forcing. J. Climate 25, 99–110. doi: 10.1175/2011JCLI4204.1
Mikaloff-Fletcher S., Gruber N., Jacobson A., Doney S., Dutkiewicz S., Gerber M., et al. (2006). Inverse estimates of anthropogenic CO2 uptake, tranport, and storage by the ocean. Global Biogeochemical Cycles 20, GB2002. doi: 10.1029/2005GB002530
Morrison A., Hogg A. (2013). On the relationship between southern ocean overturning and ACC transport. J. Phys. Oceanogr. 43, 140–148. doi: 10.1175/JPO-D-12-057.1
Morrison A. K., Waugh D. W., Hogg A. M., Jones D. C., Abernathey R. P. (2022). Ventilation of the southern ocean pycnocline. Annu. Rev. Mar. Sci. 14, 405–430. doi: 10.1146/annurev-marine-010419-011012
Purkey S. G., Smethie W. M., Gebbie G., Gordon A. L., Sonnerup R. E., Warner M. J., et al. (2018). A synoptic view of the ventilation and circulation of antarctic bottom water from chlorofluorocarbons and natural tracers. Annu. Rev. Mar. Sci. 10, 503–527. doi: 10.1146/annurev-marine-121916-063414
Rae J., Burke A., Robinson L., Adkins J., Chen T., Cole C., et al. (2018). CO2 storage and release in the deep Southern Ocean on millennial to centennial timescales. Nature 562, 569–573. doi: 10.1038/s41586-018-0614-0
Resplandy L., Séférian R., Bopp L. (2015). Natural variability of CO2 and O2 fluxes: What can we learn from centuries-long climate models simulations? J. Geophysical Research: Oceans 120, 384–404. doi: 10.1002/2014JC010463
Russell J. L., Dixon K. W., Gnanadesikan A., Stouffer R. J., Toggweiler J. R. (2006). The southern hemisphere westerlies in a warming world: Propping open the door to the deep ocean. J. Climate 19, 6382–6390. doi: 10.1175/JCLI3984.1
Sabine C., Feely R., Gruber N., Key R., Lee K., Bullister J., et al. (2004). The oceanic sink of anthropogenic CO2. Science 305, 367–371.
Schmidt C., Morrison A. K., England M. H. (2023). Wind- and sea-ice-driven interannual variability of antarctic bottom water formation. J. Geophysical Research: Oceans 128, e2023JC019774. doi: 10.1029/2023JC019774
Shi J.-R., Xie S.-P., Talley L. D. (2018). Evolving relative importance of the southern ocean and north atlantic in anthropogenic ocean heat uptake. J. Climate 31, 7459–7479. doi: 10.1175/JCLI-D-18-0170.1
Shuttleworth R., Bostock H., Chalk T., Calvo E., Jaccard S., Pelejero C., et al. (2021). Early deglacial co2 release from the sub-antarctic atlantic and pacific oceans. Earth Planetary Sci. Lett. 554, 116649. doi: 10.1016/j.epsl.2020.116649
Skinner L., Fallon S., Waelbroeck C., Michel E., Barker S. (2010). Ventilation of the deep Southern Ocean and deglacial CO2 rise. Science 328, 1147–1151.
Sokolov S., Rintoul S. R. (2009). Circumpolar structure and distribution of the antarctic circumpolar current fronts: 1. mean circumpolar paths. J. Geophysical Research: Oceans 114. doi: 10.1029/2008JC005108
Talley L. D. (2013). Closure of the global overturning circulation through the Indian, pacific, and southern oceans: Schematics and transports. Oceanography 26, 80–97.
Toggweiler J., Russell J., Carson S. (2006). Midlatitude westerlies, atmospheric CO2, and climate change during ice ages. Paleoceanography 21, PA2005. doi: 10.1029/2005PA001154
Tschumi T., Joos F., Gehlen M., Heinze C. (2011). Deep ocean ventilation, carbon isotopes, marine sedimentation and the deglacial CO2 rise. Climate Past 7, 771–800. doi: 10.5194/cp-7-771-2011
Tsujino H., Urakawa S., Nakano H., Small R. J., Kim W. M., Yeager S. G., et al. (2018). JRA-55 based surface dataset for driving ocean–sea-ice models (JRA55-do). Ocean Model. 130, 79–139. doi: 10.1016/j.ocemod.2018.07.002
Völker C., Köhler P. (2013). Responses of ocean circulation and carbon cycle to changes in the position of the Southern Hemisphere westerlies at Last Glacial Maximum. Paleoceanography 28, 726–739. doi: 10.1002/2013PA002556
Watson A., Vallis G., Nikurashin M. (2015). Southern Ocean buoyancy forcing of ocean ventilation and glacial atmospheric CO2. Nat. Geosci. 8, 861–864. doi: 10.1038/ngeo2538
Keywords: Southern Ocean, carbon cycle, atmospheric CO2, Antarctic Bottom Water (AABW), Southern hemisphere westerlies
Citation: Menviel L and Spence P (2024) Southern Ocean circulation’s impact on atmospheric CO2 concentration. Front. Mar. Sci. 10:1328534. doi: 10.3389/fmars.2023.1328534
Received: 26 October 2023; Accepted: 08 December 2023;
Published: 08 January 2024.
Edited by:
Zhifang Xiong, Ministry of Natural Resources, ChinaCopyright © 2024 Menviel and Spence. This is an open-access article distributed under the terms of the Creative Commons Attribution License (CC BY). The use, distribution or reproduction in other forums is permitted, provided the original author(s) and the copyright owner(s) are credited and that the original publication in this journal is cited, in accordance with accepted academic practice. No use, distribution or reproduction is permitted which does not comply with these terms.
*Correspondence: Laurie Menviel, bC5tZW52aWVsQHVuc3cuZWR1LmF1