- Institut des Substances et Organismes de la Mer (ISOMer), Nantes Université, Nantes, France
Macroalgae offshore cultivation and sinking is considered a potentially practical approach for ocean-based carbon dioxide removal. However, several considerations need to be resolved to assess the effectiveness and sustainability of this approach. Currently, several studies focus on the area required for climate-relevant carbon sequestration through macroalgae cultivation and sinking without considering realistic, global spatial limitations. This study uses a spatially-explicit suitability assessment model for optimised open-ocean afforestation and sinking site designation. By applying specific maritime, ecological and industrial constraints, two maps are produced: a) suitable areas for macroalgae offshore cultivation and sinking, and b) suitable areas for macroalgae sinking only (for sequestration purposes). These data provide a more realistic approach to quantifying the ocean surface (including the corresponding depths) required for macroalgae offshore cultivation and sinking within a spatially sustainable framework. The resulting maps estimate the respective suitability areas within the EEZs of the world countries. A total area suitable for macroalgae offshore cultivation and sinking is calculated at 10.8M km2, whereas sinking-only areas account for 32.8M km2 of the offshore ocean. The implications of spatial suitability patterns at global and national levels are discussed. We suggest that the concept of ‘grow nearshore, sink offshore’ should be explored as an alternative to offshore cultivation.
Introduction
Current international climate agreements require a rapid and extensive restriction of carbon dioxide (CO2) emissions so that the global average temperature rise is kept below 1.5°C by the year 2100 (UNFCCC, 2015; IPCC, 2022). Several approaches for reducing atmospheric CO2 levels have been proposed to achieve this ambitious goal. These approaches include a) energy efficiency, b) diversification of energy resources (renewables), and c) limiting fossil fuel consumption (Hassan et al., 2022; IPCC, 2022), as well as more aggressive strategies, focusing on CO2 removal (hereafter Carbon Dioxide Removal, CDR) (Duarte et al., 2017; IPCC, 2022). Industrial-type CDR strategies (aka ‘carbon capture and storage’, CCS) mainly refer to extracting CO2 at the emission spots or directly from the atmosphere and storing it in the subsurface as pressurised liquid (Leung et al., 2014). Additionally, nature-based CDR solutions, such as: enhancing carbon absorption by restoring or expanding natural habitats such as forests, wetlands, mangroves, seagrasses, and macroalgae, have also been proposed or implemented (Chung et al., 2013; Smith and Torn, 2013; Gao et al., 2022; Kuwae et al., 2022; Fujita et al., 2023; O’Dell et al., 2023). Recently, particular interest has focused on CDR through the geoengineering approach of ocean afforestation, also called macroalgae open-ocean mariculture and sinking (MOS) (Wu et al., 2023). The MOS approach aims at inducing a sustained, artificial deficit in oceanic CO2 so that the ocean responds by absorbing more CO2 from the atmosphere. Macroalgae (or seaweeds) are capable of absorbing more CO2 than other ‘blue carbon’ species, which is further sequestered to the deep sea by natural processes (Krause-Jensen and Duarte, 2016; Duarte et al., 2017; Queirós et al., 2019) To achieve this CO2 deficit in the ocean, large-scale, massive cultivation of macroalgae is required. Macroalgae build biomass by consuming nutrients and absorbing CO2 for photosynthesis, and thus when cultivated over vast areas it is suggested that they could accelerate the carbon pump in the ocean (Krause-Jensen et al., 2018; Siegel et al., 2021). The basis of MOS is not only to cultivate macroalgae but also to remove their biomass on time and quickly from the ocean surface and isolate it on the deep seafloor where carbon remineralisation can take place over extended time-scales (Krause-Jensen and Duarte, 2016; Siegel et al., 2021; Wu et al., 2023) and thus avoiding the dissolved carbon to re-enter the upper parts of the ocean and subsequently the atmosphere. Specifically, Siegel et al. (2021) estimated that more than half of sequestered carbon in the deep sea should remain stored for at least 400 years in most oceanic basins. Effective MOS-CDR operation requires large, open-ocean areas where macroalgae can grow and get directly sunk in the deep sea once a sufficient biomass level is reached. However, due to lack of updated scientific evidence, MOS is considered a highly controversial approach, being currently under criticism about its potential impact on coastal and deep sea ecosystems (Bach et al., 2021; Ricart et al., 2022; Ross et al., 2022; Wu et al., 2023), as well as its sustainability considering the amount of energy and resources required for its implementation (Krause-Jensen et al., 2018; Ross et al., 2022). Thus, several feasibility aspects of MOS implementation need to be resolved before such a practice becomes operational, including life cycle assessment of macroalgae cultivation for carbon dioxide removal (Ricart et al., 2022; Ross et al., 2022; Lian et al., 2023). One of the most important downturns is the excessive consumption of nutrients (Sheppard et al., 2023) which is predicted to lead to competition with phytoplankton (Bach et al., 2021; Sheppard et al., 2023; Wu et al., 2023). Similarly, there are serious concerns about the ecological effects that may result from MOS operations and which are expecting to impact the upper ocean layer and deep seafloor as well (Bach et al., 2021; Ross et al., 2022; Wu et al., 2023). Following, another major concern is overall efficiency of MOS given the carbon footprint required for developing, monitoring and sustaining MOS operations at a global scale (Krause-Jensen et al., 2018; Ross et al., 2022).
Although the MOS concept is still in its infancy, it seems to be gaining more attention due to the effectiveness of macroalgae in absorbing more CO2 compared to other ‘blue carbon’ resources and the potential scalability of existing macroalgae farming industry (Krause-Jensen and Duarte, 2016; Lehahn et al., 2016; Froehlich et al., 2019; Coleman et al., 2022; Ross et al., 2022). Several recent studies tried to estimate how much space is necessary for effective CDR through MOS operation. Arzeno-Soltero et al. (2023) estimated that 1M km2 of MOS area is required to cultivate various broad taxa (their study modeled temperate brown algae, and tropical red macroalgae) at oceanic areas with optimal nutrient concentration to absorb 1 GtCyear−1, which is relevant for CDR purposes. Wu et al. (2023) suggested a global MOS area of 70M km2 is required for removing ca. 13 GtCyear−1, using offshore areas that are not bounded by strict spatial criteria. However, these studies do not answer the question: how much area in the global ocean is suitable for MOS operations? Froehlich et al. (2019) estimated that ca. 48M km2 was suitable for macroalgae aquaculture using sea surface temperature and nutrient availability for building a suitability model. To our knowledge, no study has focused so far on spatial suitability assessment for cultivating macroalgae (i.e.: kelp, Sargassum) for MOS operations.
In practice, MOS planning requires the identification of suitable offshore areas where: a) MOS can be operated and monitored in a sustainable way, ensuring its CDR efficiency is not affected by operational CO2 emissions, b) it does not overlap with other activities (e.g.: shipping, submarine infrastructures), and c) it does not overlap with ecologically significant areas (such as marine protected areas and hydrothermal vents) and d) is located in open-ocean areas with sufficient nutrient concentrations and adequate depth for carbon sequestration. This study aims to provide a spatially explicit suitability assessment for MOS and sink-only areas of the global ocean. These areas can be used as a base map for MOS planning and for obtaining more realistic approximations about CDR metrics such as biomass production and CO2 absorption over different time scales and CDR scenarios. Spatial layers were processed to produce a global map of MOS- and sink-suitable areas and to calculate the percentage of these areas within national jurisdictions (Exclusive Economic zones, EEZ). In addition, the potential MOS-CDR efficiency of selected countries is discussed along with various scenarios focusing on the feasibility of MOS in achieving the 2100 climate goals. The sink-only suitability map is a novel dataset that addresses the multidisciplinary aspects and synergistic effects of various geospatial components that could be complementary to MOS approach.
Methodology
The global geospatial layers used in this study (Table 1) were based on a detailed set of criteria for MOS area selection, as suggested in a recent report by Ocean Visions (2022). The global datasets were initially divided into potential and unsuitable areas depending on their role in MOS, and data processing was based on a similar concept applied in spatial multi-criteria evaluation (Barillé et al., 2020). Datasets of the potential areas were transformed to represent suitability scores. The most critical factors for MOS potential areas include a) bathymetry, b) distance to the nearest port and c) nutrient (nitrogen-phosphorus ratio, N:P hereafter) availability. These factors are considered to have a direct impact on financial and ecological feasibility of MOS implementation (Froehlich et al., 2019; Arzeno-Soltero et al., 2023). In addition, potential areas were identified either for MOS or sinking operations. Potential MOS areas should satisfy all three criteria. In contrast, potential sinking areas should satisfy only the bathymetry and distance to port criteria, given that macroalgae could be grown nearshore and transported in these areas.
Unsuitable areas
The following global datasets were used to exclude sensitive areas. Marine Protected Areas (MPAs) were applied without any buffer as they already contain appropriate buffer zones. The operation of MOS sites may involve increased ship traffic for management purposes, potentially resulting in marine mammal disturbance. Sustainable, ecologically-informed, large-scale MOS planning should further exclude areas important for marine mammals (e.g.: migration corridors, and reproduction sites) (Ocean Visions, 2022). Hydrothermal vents host fragile and complex ecosystems which rely on particular types of microbial communities. These communities should not be disturbed or altered by potential interactions during macroalgae remineralisation (Ocean Visions, 2022). As macroalgae accumulations start to decompose, they may develop their microbial communities, which are expected to interact with local benthos (Campbell et al., 2019; Harbour et al., 2021). MOS sites should not overlap with major shipping lanes (Ocean Visions, 2022; DeAngelo et al., 2023) and should be sufficiently distant from submarine installations such as cables or pipelines (Ocean Visions, 2022). Here, we applied a 1 km buffer distance from submarine cables only, considering that open-ocean pipelines are limited and could be considered during fine-tuning of the MOS designation. Depths shallower than 1000 m and deeper than 5000 m were also excluded (see next paragraph).
Suitable areas
Once all exclusion areas were applied to potential areas, two types of suitability areas resulted: a) MOS-suitable areas, where sufficiently deep areas exist within sustainable proximity to ports and open-ocean nutrient availability is at acceptable levels for macroalgae mariculture and sinking, and b) Sink-suitable areas where open-ocean mariculture is not advised due to nutrient limitations, but these areas could be targeted for macroalgae sinking only, due to their proximity to nearest ports and appropriate sequestration depths. It should be noted that native seaweed types should be preferred for growing at each oceanic zone; i.e.: kelp in subarctic, Sargassum in temperate and Eucheumatoids in tropic waters (Froehlich et al., 2019; Ross et al., 2022; Arzeno-Soltero et al., 2023).
The main concept of MOS projects assumes that cultivation and sinking occur at the same location. In this way, the transport of cultivated biomass is avoided since it can be directly and quickly sunk and deposited on the seafloor below (Ross et al., 2022; Wu et al., 2023). This practice is supposed to lower the MOS costs and minimise the project’s carbon footprint. Thus, the GEBCO bathymetry grid was used for selecting areas with appropriate depths for macroalgae biomass sequestration. Coleman et al. (2022) suggested 1000 meters as an upper depth limit (sequestration horizon), as dissolved carbon (a by-product of macroalgae mineralization) may be leaked to the upper ocean layers if the deposition is not sufficiently deep. Wu et al. (2023) proposed that a seafloor deeper than 3000 meters was required to ensure that mineralized carbon will not re-enter the atmosphere in the next 100 years. However, areas deeper than 3000 m are located further offshore, requiring more time and cost (and thus having a larger footprint) for MOS deployment and monitoring. According to Siegel et al. (2021) the different basins of the global ocean hold specific ocean-ventilation times (time required by deep water to reach the top layer), meaning that MOS-suitable depth should vary across the global ocean. According to findings from Siegel et al. (2021), most carbon sequestered at depths of 1500-3500 m should not reach the atmosphere for at least 500 years. Thus, a Gaussian distribution function was fitted to GEBCO bathymetry to highlight areas in the 1500-3500 m range and assign lower scores to areas beyond this range (Table 1; Supplementary Figure 1). This bathymetry range is suggested as optimal for either MOS or Sink operations, as shallower areas may pose a risk due to carbon leakage in the long term, and deeper areas could render CDR operations inefficient. The proximity of potential MOS/Sink areas to the shore is important not only for nutrient availability but also for optimizing the necessary logistics required for project implementation. Combining information from the economic feasibility studies of (Lehahn et al., 2016; Coleman et al., 2022; DeAngelo et al., 2023) a one-way distance to the nearest port of 1000 km was considered as maximum acceptable. A vessel suitable for such operations with an average speed of 15 knots, containing 100k metric tons of fresh seaweed biomass would need approximately 72 h to cover a 2000 km distance (two-way) which results in a 2-20% reduction in CO2 efficiency due to emissions from fuel consumption (Lehahn et al., 2016; Bach et al., 2021). Therefore, an empirical Gaussian distribution function was applied to the Distance2port dataset (Table 1; Supplementary Figure 1) in order to assign high scores to areas closest to nearest ports, while introducing rapid score decrease with increasing distance beyond the 1000 km fuzzy boundary. The function was applied to distances corresponding to areas with a depth greater than the depth of sequestration horizon (1000 m).
The N:P ratio in the surface water is a significant criterion for establishing an offshore ocean afforestation area. Different macroalgae species have their own optimal ratios, but 30:1 has been proposed as an average, with the range between 10:1 and 60:1 describing the overall variability of nutrient preferences (Harrison and Hurd, 2001; Froehlich et al., 2019; Sheppard et al., 2023). In this study, we applied an empirical Gaussian function, with the highest scores assigned to a 30:1 nutrient ratio (Supplementary Figure 1). The global potential area, resulting from the sum of thresholded data, was thinned according to the ecological and industrial activity criteria shown in Table 1. Bathymetry and distance to port were thresholded using a cut-off score of 0.75, and for nutrient ratio, a 0.5 score was used for selecting a more comprehensive range of optimal areas. These threshold values ensure that only areas with the most favorable/sustainable conditions are considered for further analyses.
It has to be noted that the surface area results provided here is calculated as an upper limit of suitable space available for MOS. The actual surface areas in future, real-world implementations could be smaller, since detailed fine-tuning may exclude areas: a) with steep seafloor or areas where sinking is not advised due to geo-hazards (Ocean Visions, 2022) and b) where there is potential conflict between MOS with localized maritime activities.
Results
Global and EEZ-scale coverage
According to the spatial suitability model developed in this study, the total surface area of the global ocean which is suitable for MOS is 10.8M km2 (Figure 1). Many of the global MOS-suitable areas are located in sub-arctic and temperate zones. On the other hand, the global ocean area, which is sink-suitable (i.e., sinking only), is significantly larger, covering 32.8M km2 approximately (Figure 1). Considering that global efforts toward CDR are expected to rely mainly on national-level initiatives (Froehlich et al., 2019), the amount of MOS- and Sink-suitable areas within the national EEZs was calculated (Table 2). The total MOS-suitable area within the national EEZs is ca. 4.8M km2. Most countries with the largest MOS-suitable surface area in absolute numbers are in sub-arctic and temperate zones. At the same time, there are also a few tropical countries located along the western coast of Africa (Mauritania), the Caribbean Sea (Cuba, Jamaica) and the eastern and western coasts of South America (Brazil, Chile, Ecuador). Norway has the largest surface of MOS-suitable area (593616 km2) in its EEZ, followed by Denmark (453805 km2) and the US (390911 km2). The total Sink-suitable area falling within the national EEZs is ca. 18.4M km2. The countries with the most significant Sink-suitable areas within their EEZ are located worldwide, with more than half covering the tropical and subtropical regions. It is essential to highlight the sinking-area capacity of the top 10 macroalgae farming countries (Table 3). With a combined surface area of ca. 2.2 M km2, they occupy more than half of the area of the top 20 countries with the largest MOS-suitable area. Indonesia is by far the country with the largest Sink-suitable area (764836 km2) in its EEZ, followed by Japan (518978 km2) and the Philippines (266236 km2).
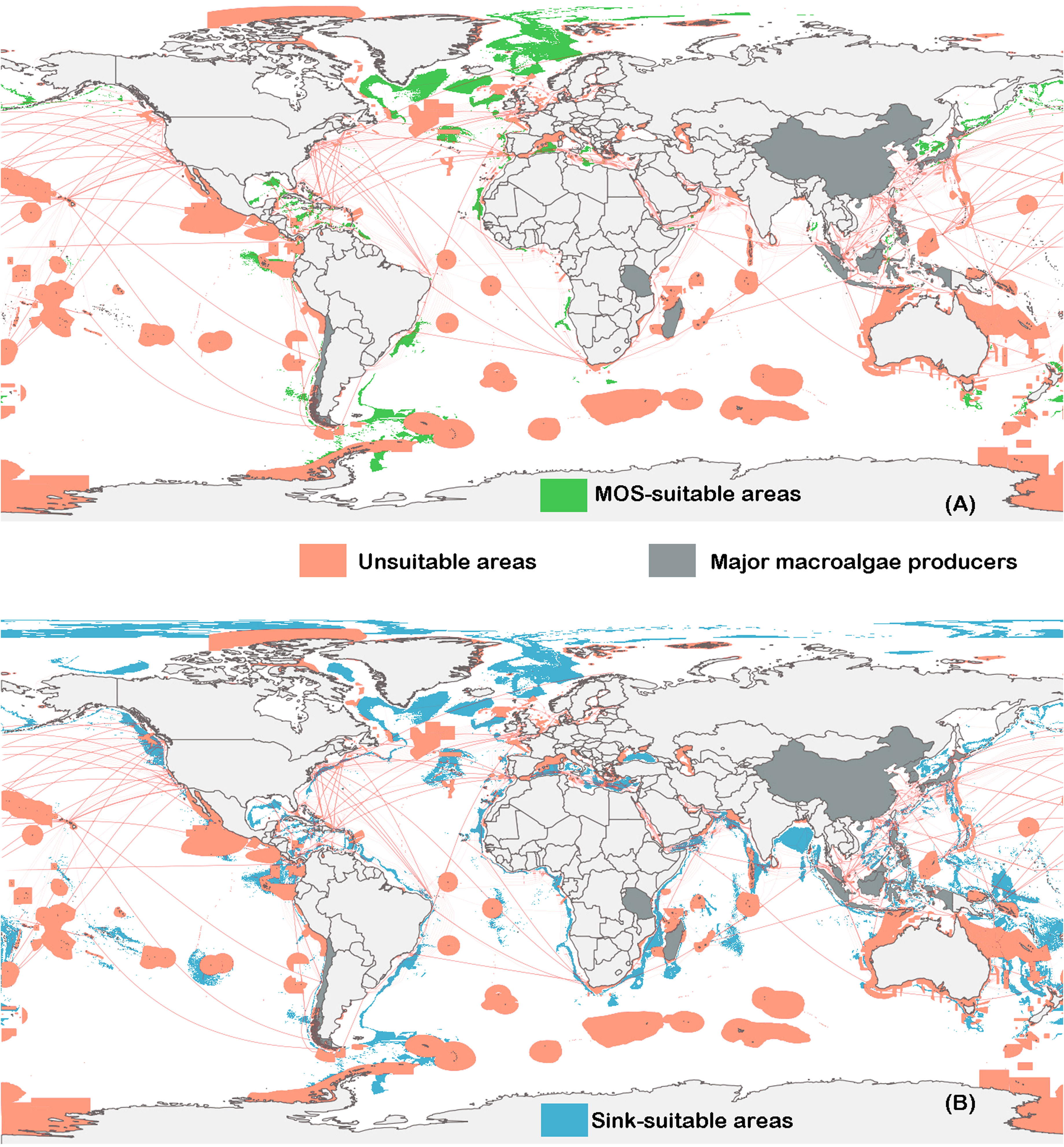
Figure 1 Global suitability map for: (A) Macroalgae offshore cultivation and sinking (MOS) and (B) sinking-only.
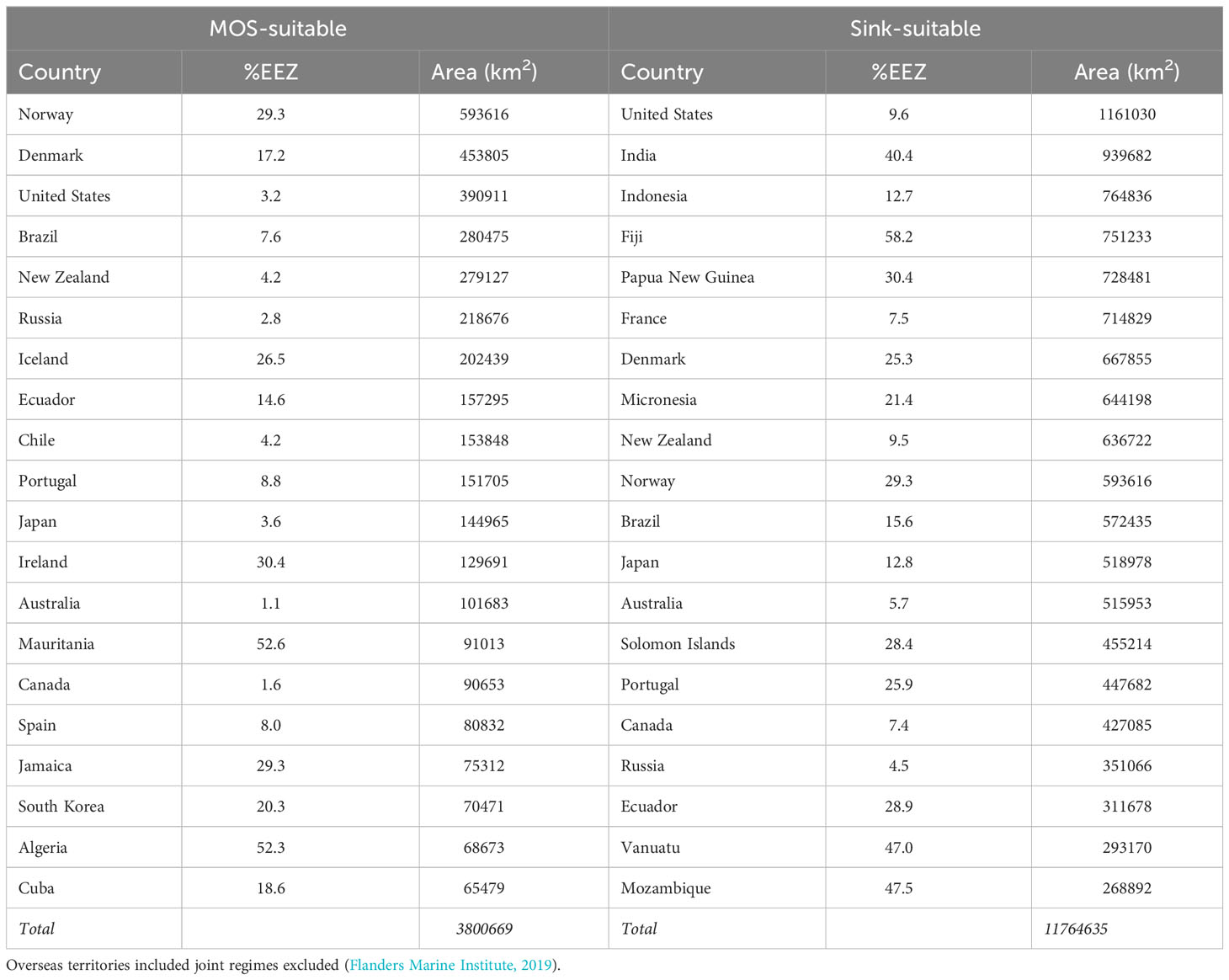
Table 2 Top 20 countries with a) largest MOS-suitable surface area within their EEZ; b) largest Sink-suitable surface area within their EEZ.
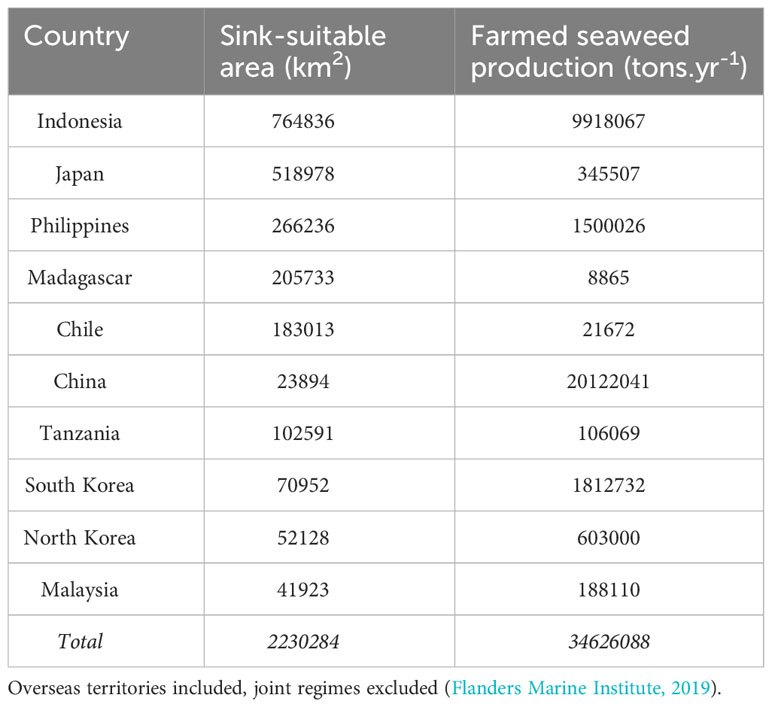
Table 3 Sinking area capacity of top-10 major producers of cultivated macroalgae (Cai, 2021) within their EEZ.
Discussion
MOS and sinking-only spatial extents
The resulting global suitability maps for MOS estimated in this study was 10.8M km2, a smaller surface than suggested previously. Wu et al. (2023) tested a global model which provided a potential MOS area of 69.6 × 106 km2 (∼ 19.7% of the world ocean), while Lehahn et al. (2016) estimated an area of 35× 106 km2 (∼ 10% of the world ocean). Froehlich et al. (2019) calculated an area of ∼ 48 × 106 km2 suitable for ocean macroalgae aquaculture based on nutrient availability and temperature but did not relate it to the sinking process. Adding constraints such as distance to the port, depth, wave energy, shipping traffic, and marine protected areas, Spillias et al. (2023) obtained a smaller estimation of ∼ 6.5× 106 km2. The MOS suitability maps obtained in this study combined simple nutrient availability criteria for growing macroalgae with additional physical, ecological and industrial constraints related to the sinking process. The discrepancy with the MOS estimations from previous studies is mainly due to the setting of strict sinking criteria for optimal depth range (~1500-3500 m) and maximum sustainable distance (<1000 km) from the nearest port. These two limitations are crucial for increasing the cost- and time-effectiveness of MOS operations by reducing the total transit time required (for development and monitoring) and, hence, the carbon footprint of the MOS project itself. Depositing macroalgae on the seafloor in the depth range used in this study reduces the risk of CO2 leakage back to the surface (Siegel et al., 2021). The global extent of sink-suitable area estimated in this study is 32.8M km2. This is a new type of dataset that is expected to provide a new dimension in the discussion about open-ocean afforestation and sinking for CDR purposes.
Carbon estimations
The resulting global maps present the necessary spatial context that should be utilized for spatially restricting economic and biogeochemical models for MOS operations, allowing more realistic estimations/extrapolations of carbon removal to be obtained. Additionally, the resulting suitability maps are expected to contribute to the marine spatial planning of MOS activities by a) exploiting the carbon removal and sequestration potential of particular areas and b) minimizing the costs and effort required for MOS site selection and MOS operation/monitoring (Ross et al., 2022). Arzeno-Soltero et al. (2023) calculated that a 1M km2 area (corresponding to 1Gt of fresh macroalgae biomass) with optimal nutrient availability should be cultivated intensively (5 cycles.year-1) for capturing 1 Gt CO2.yr-1, whereas Ross et al. (2022) estimated an area of 0.4M km2 for 1 GtCO2 fixation. Both estimates fall in the lower level of CO2 quantity that must be removed by 2100 to keep global temperatures lower than 1.5°C (Bach et al., 2021). Upscaling the results from Bach et al. (2021), who considered various uncertainties, a 3M km2 cultivation area (with 3 cycles.year-1) is required for capturing a climate-effective amount of CO2 by the end of the century. Considering the above estimations and that international climate goals require at least 5 GtCO2.yr-1 to be removed from the atmosphere by midcentury (Arzeno-Soltero et al., 2023), it is understood that the entire MOS-suitable area within the national EEZs should become operational. Although such a scenario is not realistic for the near future, this study showed significant MOS potential areas within the countries’ EEZs. The vast MOS-suitable areas in Canada, the USA, Japan, Russia and Australia occupy less than 5% of their EEZ surface (Table 2), allowing them to pioneer the MOS-CDR development due to spatial opportunities. Considering only the sinking process for the top 10 major producers of cultivated macroalgae, Indonesia has the most significant potential with 0.76 M km2. These countries are further highlighted by (Liu et al., 2023) as having the greatest nearshore area (without considering sinking in the deep sea) suitable for macroalgae farming. Particularly, according to estimations from Gentry et al., 2017) regarding Indonesia, it was found that there is enough space available for upscaling nearshore aquaculture without impacting the existing production of biomass. MOS/sink-only areas could be seen as a new type of valuable resource that could influence carbon policy and decision making at both national and trans-national levels. At national level, the presented suitability maps could assist countries in defining their specific net-zero strategies, whereas new economic relationships could be developed between different countries on the base of voluntary carbon offset markets (Froehlich et al., 2019; Ross et al., 2022), as suitable areas tend to be disproportionally distributed in some parts of the globe.
Implications on MOS feasibility assessment
The typical MOS concept is based on ‘grow and sink offshore’, which is challenged by various technical feasibility aspects. One of the biggest challenges of MOS is related to the logistics and energy required for building and keeping MOS operations running. As large-scale, open-ocean areas are required for effective CDR through MOS, the sustainability of such operations is questionable, given their large carbon footprint (Ross et al., 2022). In addition, the cultivation of macroalgae at different oceanic conditions (temperature, salinity, nutrients) and the effect of various environmental factors (e.g., storms) would cause the biomass (and hence CO2 absorption) to diverge substantially between MOS areas. On the other hand, the potential of sink-suitable areas within sustainable distance from ports assists in rethinking the operational design of MOS. Thus, the ‘grow nearshore, sink offshore’ concept may be explored as an alternative to MOS, particularly for countries such as Indonesia, the second macroalgae producer in the world, with the largest sink-suitable area. It is therefore suggested that macroalgae cultivation could take place in nearshore areas, and wet biomass should then be transported to adjacent sink-suitable areas for deposition. This practice would not require vast and complex offshore installations and would not be subjected to harsh ocean conditions, a strong constraint for offshore aquaculture (Buck and Langan, 2017; Buck and Grote, 2018). Additionally, growing macroalgae nearshore, could improve water quality by targeting primarily eutrophic coastal areas (Froehlich et al., 2019; Weerakkody et al., 2023). However, this concept requires further consideration of various factors such as environmental, ethical, and economic issues (more details in the next section). One of the main downturns is the environmental footprint resulting from the continuous transport of wet biomass to sinking sites. It needs to be more accurately assessed considering that it could be mitigated by relying on ‘green’ fuels (e.g., ethanol) for shipping, produced by part of the cultivated macroalgae biomass (Lehahn et al., 2016).
The fact that the top ten macroalgae-producing countries occupy large open-ocean areas suitable for sinking-only (Table 3), further emphasizes the potential of these countries in leading global initiatives on ‘grow nearshore, sink offshore’ operations. The growing demand for macroalgae products, drives a subsequent need for upscaling the macroalgae farming industry (Lehahn et al., 2016; Froehlich et al., 2019; Ross et al., 2022; Liu et al., 2023; Spillias et al., 2023). However, countries outside the tropics, with large suitable space availability have a negligible macroalgae farming development due to various socio-economic reasons (Liu et al., 2023). Incorporating macroalgae farming for ocean-based CDR along with the use for bioenergy/bio-products production could potentially accelerate the upscaling of current farms globally (Lehahn et al., 2016; Froehlich et al., 2019).
MOS uncertainties
This study highlights the substantial opportunities for ocean-based CDR by identifying significant MOS and sinking-only areas in the global ocean and within countries’ EEZs. However, spatial planning is only one aspect of establishing such operations, and several legal, economic and ecological uncertainties must be accounted for. Large-scale MOS is currently lacking a detailed legal framework that regulates multiple user interactions in the open ocean (O’Dell et al., 2023) and ensures compliance with the London (1972) Convention on the Prevention of Marine Pollution by Dumping of Wastes and Other Matter (IMO, n.d.). Macroalgae cultivation and sinking are currently subject of feasibility scrutiny, particularly in terms of economic viability as such operations involve huge costs and associated effort. Detailed economic estimates from (DeAngelo et al., 2023) and (Froehlich et al., 2019) place ocean afforestation costs toward the upper range of costs required for terrestrial afforestation. However, upscaling the macroalgae farming sector for large-scale carbon offsetting and production of biofuels could lower the overall costs (Froehlich et al., 2019). Monitoring the CDR efficiency of ocean afforestation includes the challenging tasks of verifying additionality and permanence (Ross et al., 2022; Hurd et al., 2023; Pessarrodona et al., 2023). Additionality refers to the amount of carbon removed from the ocean due to MOS implementation. At the same time, permanence is the amount of MOS-captured carbon that is securely sequestered for sufficient timescales. Verification of the actual CO2 uptake from the ocean – as a result of macroalgae growth – is a complex task, however ongoing research focuses on the novel concept of air-sea CO2 equilibration (Hurd et al., 2023) for measuring the effectiveness of ocean-based CDR. Last but not least, important environmental and ecological uncertainties need to be resolved and quantified to prioritize further MOS research (Campbell et al., 2019). Both macroalgae cultivation and sinking are expected to influence in various ways the upper ocean layers and deep sea habitats, respectively. Open ocean macroalgae cultivation is associated with massive nutrient consumption, leading to competition with phytoplankton (Sheppard et al., 2023). This may cause a decrease in carbon that could be otherwise fixed by phytoplankton (Bach et al., 2021; Ross et al., 2022). Furthermore, macroalgae may host calcifying organisms that reduce seawater alkalinity and subsequently the capacity of the ocean to absorb CO2 (Ross et al., 2022; Wu et al., 2023). Additional uncertainties and complications are related to the fate of macroalgae and carbon after sinking and their impact on deep sea habitats. Thick accumulations of macroalgae on the seafloor could create new hypoxic zones (Ross et al., 2022; Wu et al., 2023) however the exact influence on benthic fauna remains quite unclear. Therefore, continuing simulations and real-world experiments are required to better constrain the overall benefits of MOS operations.
Data availability statement
The original contributions presented in the study are included in the article/Supplementary Material. Further inquiries can be directed to the corresponding author.
Author contributions
EA: Conceptualization, Data curation, Formal analysis, Investigation, Methodology, Software, Validation, Visualization, Writing – original draft, Writing – review & editing. LB: Methodology, Project administration, Resources, Supervision, Validation, Writing – review & editing.
Funding
The author(s) declare financial support was received for the research, authorship, and/or publication of this article. This work was supported by the Erasmus+: Key Action 1 – Erasmus Mundus Joint Master Degrees (EMJMD) (Grant No. 599111-EPP-1-2018-1-EL-EPPKA1-JMD-MOB) for the EMJMD in Aquaculture, Environment and Society PLUS (ACES+).
Conflict of interest
The authors declare that the research was conducted in the absence of any commercial or financial relationships that could be construed as a potential conflict of interest.
Publisher’s note
All claims expressed in this article are solely those of the authors and do not necessarily represent those of their affiliated organizations, or those of the publisher, the editors and the reviewers. Any product that may be evaluated in this article, or claim that may be made by its manufacturer, is not guaranteed or endorsed by the publisher.
Supplementary material
The Supplementary Material for this article can be found online at: https://www.frontiersin.org/articles/10.3389/fmars.2023.1320642/full#supplementary-material
References
Arzeno-Soltero I. B., Saenz B. T., Frieder C. A., Long M. C., DeAngelo J., Davis S. J., et al. (2023). Large global variations in the carbon dioxide removal potential of seaweed farming due to biophysical constraints. Commun. Earth Environ. 4, 1–12. doi: 10.1038/s43247-023-00833-2
Bach L. T., Tamsitt V., Gower J., Hurd C. L., Raven J. A., Boyd P. W. (2021). Testing the climate intervention potential of ocean afforestation using the Great Atlantic Sargassum Belt. Nat. Commun. 12, 2556. doi: 10.1038/s41467-021-22837-2
Barillé L., Le Bris A., Goulletquer P., Thomas Y., Glize P., Kane F., et al. (2020). Biological, socio-economic, and administrative opportunities and challenges to moving aquaculture offshore for small French oyster-farming companies. Aquaculture 521. doi: 10.1016/j.aquaculture.2020.735045
Buck B. H., Grote B. (2018). “Seaweed in high-energy environments: Protocol to move Saccharina cultivation offshore,” in Protocols for Macroalgae Research (CRC Press, Taylor&Francis Group).
Buck B. H., Langan R. (Eds.) (2017). Aquaculture Perspective of Multi-Use Sites in the Open Ocean (Cham: Springer International Publishing). doi: 10.1007/978-3-319-51159-7
Cai J. (2021). “Seaweeds and microalgae: an overview for unlocking their potential in global aquaculture development,” in FAO Fisheries and Aquaculture Circular (Rome, Italy: FAO). doi: 10.4060/cb5670en
Campbell I., Macleod A., Sahlmann C., Neves L., Funderud J., Øverland M., et al. (2019). The environmental risks associated with the development of seaweed farming in Europe - prioritizing key knowledge gaps. Front. Mar. Sci. 6. doi: 10.3389/fmars.2019.00107
Chung I. K., Oak J. H., Lee J. A., Shin J. A., Kim J. G., Park K.-S. (2013). Installing kelp forests/seaweed beds for mitigation and adaptation against global warming: Korean Project Overview. ICES J. Mar. Sci. 70, 1038–1044. doi: 10.1093/icesjms/fss206
Coleman S., Dewhurst T., Fredriksson D. W., St. Gelais A. T., Cole K. L., MacNicoll M., et al. (2022). Quantifying baseline costs and cataloging potential optimization strategies for kelp aquaculture carbon dioxide removal. Front. Mar. Sci 9. doi: 10.3389/fmars.2022.966304
DeAngelo J., Saenz B. T., Arzeno-Soltero I. B., Frieder C. A., Long M. C., Hamman J., et al. (2023). Economic and biophysical limits to seaweed farming for climate change mitigation. Nat. Plants 9, 45–57. doi: 10.1038/s41477-022-01305-9
Duarte C. M., Wu J., Xiao X., Bruhn A., Krause-Jensen D. (2017). Can seaweed farming play a role in climate change mitigation and adaptation? Front. Mar. Sci. 4. doi: 10.3389/fmars.2017.00100
Flanders Marine Institute. (2019). IMIS [WWW document] (Vlaams Inst. Voor Zee). Available at: https://www.vliz.be/en/imis (Accessed 9.28.23).
Froehlich H. E., Afflerbach J. C., Frazier M., Halpern B. S. (2019). Blue growth potential to mitigate climate change through seaweed offsetting. Curr. Biol. 29, 3087–3093.e3. doi: 10.1016/j.cub.2019.07.041
Fujita R., Augyte S., Bender J., Brittingham P., Buschmann A. H., Chalfin M., et al. (2023). Seaweed blue carbon: Ready? Or Not? Mar. Policy 155. doi: 10.1016/j.marpol.2023.105747
Gao G., Beardall J., Jin P., Gao L., Xie S., Gao K. (2022). A review of existing and potential blue carbon contributions to climate change mitigation in the Anthropocene. J. Appl. Ecol. 59, 1686–1699. doi: 10.1111/1365-2664.14173
Gentry R.R., Froehlich H.E., Grimm D., Kareiva P., Parke M., Rust M., et al. (2017). Mapping the global potential for marine aquaculture. Nat. Ecol. Evol. 1, 1317–1324. doi: 10.1038/s41559-017-0257-9
Harbour R. P., Smith C. R., Simon-Nutbrown C., Cecchetto M., Young E., Coral C., et al. (2021). Biodiversity, community structure and ecosystem function on kelp and wood falls in the Norwegian deep sea. Mar. Ecol. Prog. Ser. 657, 73–91. doi: 10.3354/meps13541
Harrison P., Hurd C. (2001). Nutrient physiology of seaweeds: Application of concepts to aquaculture. Cah. Biol. Mar. 42, 71–82. doi: 102.100.100/576353
Hassan T., Song H., Khan Y., Kirikkaleli D. (2022). Energy efficiency a source of low carbon energy sources? Evidence from 16 high-income OECD economies. Energy 243, 123063. doi: 10.1016/j.energy.2021.123063
Hurd C. L., Gattuso J.-P., Boyd P. W. (2023). Air-sea carbon dioxide equilibrium: Will it be possible to use seaweeds for carbon removal offsets? J. Phycol. doi: 10.1111/jpy.13405
IMO. Convention on the prevention of marine pollution by dumping of wastes and other matter. Available at: https://www.imo.org/en/OurWork/Environment/Pages/London-Convention-Protocol.aspx (Accessed 10.11.23).
IPCC. (2022). Global Warming of 1.5°C: IPCC Special Report on Impacts of Global Warming of 1.5°C above Pre-industrial Levels in Context of Strengthening Response to Climate Change, Sustainable Development, and Efforts to Eradicate Poverty. 1st ed (Cambridge: Cambridge University Press). doi: 10.1017/9781009157940
IUCN MMPATF. (2022). Global Dataset of Important Marine Mammal Areas (IUCN IMMA). November, 2022. Made available under agreement on terms and conditions of use by the IUCN Joint SSC/WCPA Marine Mammal Protected Areas Task Force and accessible via the IMMA e-Atlas. Available at: http://www.marinemammalhabitat.org/imma-eatlas/.
Krause-Jensen D., Duarte C. M. (2016). Substantial role of macroalgae in marine carbon sequestration. Nat. Geosci. 9, 737–742. doi: 10.1038/ngeo2790
Krause-Jensen D., Lavery P., Serrano O., Marbà N., Masque P., Duarte C. M. (2018). Sequestration of macroalgal carbon: the elephant in the Blue Carbon room. Biol. Lett. 14. doi: 10.1098/rsbl.2018.0236
Kuwae T., Watanabe A., Yoshihara S., Suehiro F., Sugimura Y. (2022). Implementation of blue carbon offset crediting for seagrass meadows, macroalgal beds, and macroalgae farming in Japan. Mar. Policy 138, 104996. doi: 10.1016/j.marpol.2022.104996
Lehahn Y., Ingle K. N., Golberg A. (2016). Global potential of offshore and shallow waters macroalgal biorefineries to provide for food, chemicals and energy: feasibility and sustainability. Algal Res. 17, 150–160. doi: 10.1016/j.algal.2016.03.031
Leung D. Y. C., Caramanna G., Maroto-Valer M. M. (2014). An overview of current status of carbon dioxide capture and storage technologies. Renew. Sustain. Energy Rev. 39, 426–443. doi: 10.1016/j.rser.2014.07.093
Lian Y., Wang R., Zheng J., Chen W., Chang L., Li C., et al. (2023). Carbon sequestration assessment and analysis in the whole life cycle of seaweed. Environ. Res. Lett. 18. doi: 10.1088/1748-9326/acdae9
Liu Y., Cao L., Cheung W. W. L., Sumaila U. R. (2023). Global estimates of suitable areas for marine algae farming. Environ. Res. Lett. 18, 064028. doi: 10.1088/1748-9326/acd398
Ocean Visions. (2022). Framework to guide research on seaweed cultivation and sinking for carbon dioxide removal. Ocean Vis. Available at: https://oceanvisions.org/wp-content/uploads/2022/10/Ocean-Visions-Sinking-Seaweed-Report_FINAL.pdf.
O’Dell A., Canvin M., de Bettignies F., Filbee-Dexter K., Grisenthwaite R., Hughes A., et al. (2023). BLUE FORESTS A review of carbon offset strategies with seaweed aquaculture -feasibility, current knowledge, and suggestions for future research. The Scottish Association for Marine Science, Oban, UK. Report prepared for the Scottish Government. Available at: https://www.sams.ac.uk/.
Pessarrodona A., Franco-Santos R. M., Wright L. S., Vanderklift M. A., Howard J., Pidgeon E., et al. (2023). Carbon sequestration and climate change mitigation using macroalgae: a state of knowledge review. Biol. Rev. Brv. 98, 12990. doi: 10.1111/brv.12990
Queirós A. M., Stephens N., Widdicombe S., Tait K., McCoy S.J., Ingels J., et al. (2019). Connected macroalgal-sediment systems: blue carbon and food webs in the deep coastal ocean. Ecol. Monogr. 89, e01366. doi: 10.1002/ecm.1366
Ricart A. M., Krause-Jensen D., Hancke K., Price N. N., Masqué P., Duarte C. M. (2022). Sinking seaweed in the deep ocean for carbon neutrality is ahead of science and beyond the ethics. Environ. Res. Lett. 17, 081003. doi: 10.1088/1748-9326/ac82ff
Ross F., Tarbuck P., Macreadie P. I. (2022). Seaweed afforestation at large-scales exclusively for carbon sequestration: Critical assessment of risks, viability and the state of knowledge. Front. Mar. Sci. 9. doi: 10.3389/fmars.2022.1015612
Sheppard E. J., Hurd C. L., Britton D. D., Reed D. C., Bach L. T. (2023). Seaweed biogeochemistry: Global assessment of C:N and C:P ratios and implications for ocean afforestation. J. Phycol. 59, 879–892. doi: 10.1111/jpy.13381
Siegel D. A., DeVries T., Doney S. C., Bell T. (2021). Assessing the sequestration time scales of some ocean-based carbon dioxide reduction strategies. Environ. Res. Lett. 16, 104003. doi: 10.1088/1748-9326/ac0be0
Smith L. J., Torn M. S. (2013). Ecological limits to terrestrial biological carbon dioxide removal. Clim. Change 118, 89–103. doi: 10.1007/s10584-012-0682-3
Spillias S., Valin H., Batka M., Sperling F., Havlík P., Leclère D., et al. (2023). Reducing global land-use pressures with seaweed farming. Nat. Sustain. 6, 380–390. doi: 10.1038/s41893-022-01043-y
UNEP-WCMC. (2019). User Manual for the World Database on Protected Areas and world database on other: 1.6. (Cambridge, UK: UNEP-WCMC). Available at: http://wcmc.io/WDPA_Manual.
UNFCCC. (2015). The Paris Agreement (UNFCCC). Available at: https://unfccc.int/process-and-meetings/the-paris-agreement (Accessed 9.20.23).
Weerakkody W. S., Ling K. H., Hsieh H.-H., Abedneko V. G., Shyu J.-F., Lee T.-M., et al. (2023). Carbon capture by macroalgae Sarcodia suae using aquaculture wastewater and solar energy for cooling in subtropical regions. Sci. Total Environ. 855, 158850. doi: 10.1016/j.scitotenv.2022.158850
Keywords: macroalgae, ocean afforestation, carbon dioxide removal, suitability, marine planning, mariculture, carbon sequestration
Citation: Alevizos E and Barillé L (2023) Global ocean spatial suitability for macroalgae offshore cultivation and sinking. Front. Mar. Sci. 10:1320642. doi: 10.3389/fmars.2023.1320642
Received: 12 October 2023; Accepted: 16 November 2023;
Published: 30 November 2023.
Edited by:
Michael Yu Roleda, University of the Philippines Diliman, PhilippinesReviewed by:
Yushun Lian, Hohai University, ChinaAlan T. Critchley, The Verschuren Centre (VC), Canada
Copyright © 2023 Alevizos and Barillé. This is an open-access article distributed under the terms of the Creative Commons Attribution License (CC BY). The use, distribution or reproduction in other forums is permitted, provided the original author(s) and the copyright owner(s) are credited and that the original publication in this journal is cited, in accordance with accepted academic practice. No use, distribution or reproduction is permitted which does not comply with these terms.
*Correspondence: Evangelos Alevizos, RXZhbmdlbG9zLkFsZXZpem9zQHVuaXYtbmFudGVzLmZy