- 1Department of Mechanical Engineering, University of Victoria, Mechanical Engineering, Victoria, BC, Canada
- 2Division of Medical Sciences, University of Victoria, Victoria, BC, Canada
- 3Centre for Advanced Materials and Technology, University of Victoria, Victoria, BC, Canada
- 4School of Biomedical Engineering, University of British Columbia, Vancouver, BC, Canada
Introduction: Coastal ecosystems, including reefs, are becoming increasingly threatened as anthropogenic development continues to encroach on intertidal habitats with little initiative to establish ecologically considerate infrastructure. Submerging human-made, shelter-providing structures known as artificial reefs (AR) can contribute to the preservation of these ecosystems. ARs are historically used for promoting the abundance and biodiversity of marine species for aquaculture, conservation, and ecotourism; and are typically made of concretes or metal structures. An AR’s success correlates to its ability to establish a surface layer of microorganisms, such as microalgae and bacteria, known as a biofilm. The productivity of the biofilm can be influenced by material surface properties. It is hypothesized that material pH and porosity affect the rate of biofilm formation.
Methods: Here - a range of concrete mixtures were cast and submerged in circulating seawater and mass per surface area of biofilm accumulation was measured to evaluate this theory. These mixtures included standard Portland Cement (PC), PC with admixtures of diatomaceous earth (PDC) and limestone (PLC), fine-aggregate high-performance concrete (DUC), and terra cotta (TER). ARs were manufactured as 38mm tall cylinders, 76mm in diameter, and submerged in circulating seawater to evaluate mass per surface area of biofilm accumulation.
Results: Our results indicate that biofilm formation is directly affected by surface porosity and less-so by pH, as determined by measuring material properties after submersion. We found that the PDC samples were most successful in forming a biofilm despite being more fragile than other concrete samples.
Discussion: This preliminary study provides insight into how different material properties influence the accumulation of biofilm as a starting point for designing ARs. Future work will investigate the long-term performance of such samples in relevant conditions.
1 Introduction
As human development continues to increase, coastal communities are frequently building more infrastructure near oceans and seas. With this development comes the trade-off of preserving natural areas and habitats. As cities expand their coastlines, marine habitats begin to decline. It is estimated that Europe established one kilometer of coastline per day between 1960 and 1995, destroying over 50% of the coastal wetlands and seagrasses in the area. Anthropogenic stressors such as climate change and infrastructure have caused significant habitat loss, which is characterized as the loss of resident species, food resources, and ecosystem function. With a decline in species richness, organisms, such as shellfish and seagrasses, providing structural stability, shelter, and carbon sequestration may decline as well, further damaging the ecosystems around it. Habitats with established reefs and aquatic vegetation play key roles in maintaining coastlines and protecting them from the ocean’s hydrodynamic forces and erosion. This loss of habitat creates a gradual decline into biotic homogenization; where diversity and structural complexity is reduced, weakening the ecosystem in question (Airoldi et al., 2008).
One of the more at-risk habitats are biogenic temperate reefs, which are cold water ecosystems made up of rocky shores harbouring bivalves and macroalga. Canada’s coastlines often consist of these temperate habitats (Airoldi et al., 2008). These areas are part of the littoral, or intertidal, zone which are coastal ecosystems connecting the ocean and shore. This region can be submerged at high tide, but exposed to air when the tide is low, resulting in considerable species diversity and providing a buffer from the ocean’s forces (Cefalì et al., 2016). While these areas are ecologically significant, they are often threatened by anthropogenic development, ocean acidification, and general climate change. Man-made structures such as docks and breakwaters infringe on the intertidal zone, displacing marine life while providing little in return for the species that inhabit these shores. Although these developments can provide habitats for more stress-resistant species such as Mediterranean mussels and Ulvales, organisms which have more specific habitat requirements like some forms of coralline red-algae are unsuccessful in colonizing this infrastructure (Cefalì et al., 2016).
One of the methods for habitat restoration and ecological preservation is the development of man-made structures known as artificial reefs, structures placed in a marine environment with the goal of increasing local biomass by providing favourable conditions for organisms to inhabit (Seaman, 2000). ARs can promote ecological restoration, aquaculture production, and ecotourism. The materials and shapes of an AR can vary greatly from sunken naval ships, blocks of concrete, to heaps of tires (Seaman, 2000). Beyond habitat establishment or aquaculture organism accumulation, ARs can benefit coastal communities by reducing erosion and easing wave height as these hard structures can break up hydrodynamic forces similar to the structural functions of marshes and mangrove forests. A historically applied method of foreshore protection is the use of breakwaters and other hard marine structures. However, the goal of this infrastructure is often community protection instead of environmental protection or habitat restoration (Nitsch et al., 2021). Ideally, an artificial reef or foreshore structure can provide protection from coastal erosion while still restoring habitats and promoting sustainable biodiversity. Some projects have explored methods of improving the ecological benefits of hard coastal protection and promoting marine growth on seawalls. Most of these projects have been largely successful in creating micro-habitats by using panels to increase surface complexity (channels, holes, textures, etc.) to better resemble natural environments (Strain et al., 2018). Other methods of environmentally friendly intertidal development have been the installation of biodegradable, starch and shellfish-based reef blocks to reduce wave height and protect salt marshes from edge erosion (Marin-Diaz et al., 2021); and the transplantation of reef biota such as macroalgae onto seawalls to establish new food sources and shelter for intertidal organisms (Ng et al., 2015). As 3D printing technologies improve and enable the rapid fabrication of complex structures using a wide range of materials, there is significant potential for marine restoration to benefit from the abilities of 3D printers. In recent years, there has been numerous projects utilizing this technology to design more effective ARs (Levy et al., 2022).
It is important to understand the biological interactions that occur when a structure is submerged in the ocean. An underwater AR surface is almost immediately colonized by microorganisms, forming a layer of organic material referred to as a biofilm. This biofilm typically consists of bacteria and phytoplankton such as diatoms (Salta et al., 2013). For marine engineering applications where biofilms are seen to hinder performance or are considered undesirable, this formation is often referred to as biofouling (Salta et al., 2013). In a different perspective, biofilms can improve the adhesion of larger organisms such as tubeworms, mussels, barnacles, and macroalgae onto colonized surfaces (Salta et al., 2013). For an artificial reef, the formation of biofilms may aid in promoting greater surface biodiversity and create a more favourable habitat for other species in different trophic positions such as invertebrates and fishes. Once an AR has been colonized by biofilm, a complex structure forms and enables multicellular organisms to inhabit the surface through epibiosis. While there is limited information on characterizing nutritional value of biofilms and its function in the trophic network, it is accepted that the formation of these films is essential to supporting biodiversity in ARs (Riera et al., 2018).
While ARs have been produced from a range of materials, one of the most common is Portland cement (PC) - the primary ingredient of standard concrete - as it can be used to develop near-limitless shapes and geometries due to its fluidic nature prior to curing (McManus et al., 2018). While concretes are often used for ARs, there is limited information as to why it is preferred apart from its rocky characteristics and commercial availability. With concrete’s workability and proven success in AR applications, future development of artificial reefs made from concrete appear to be the evident path for marine restoration and coastal infrastructure. A large concern with concrete as an AR material is the surface conditions produced during the curing process. Most concretes are made with the base ingredient of PC consisting of calcined limestone and other calcium-based minerals. Limestone is an alkaline material, resulting in fresh concrete typically having a surface pH around 12 (Guilbeau et al., 2003). Over time, the concrete cures and undergoes a process known as carbonation where carbon dioxide reacts with the surface to produce calcium carbonate; this process gradually penetrates deeper into the bulk of the material over several months, increasing material strength and slowly neutralizing the material pH as carbonation continues. With high surface pH and slow carbonation times, it can take upwards of 6 months for a concrete artificial reef to equalize with the ocean’s pH of roughly 8.3 (Guilbeau et al., 2003). Additionally, concrete production contributes to approximately 9% of global CO2 emissions through the manufacturing of aggregate and calcination of limestone, resulting in 1 tonne of CO2 being released for every tonne of concrete produced (Monteiro et al., 2017). To resolve the issues of surface alkalinity and high emissions, many AR studies examine the effectiveness of different admixtures and alternative cements to produce more environmentally friendly alternatives to typical Portland cements (Mat Jusoh et al., 2018).
Among these solutions, admixtures such as diatomaceous earth (DE) and powdered limestone (LS) have been proposed as more sustainable ingredients to offset the volume of PC used for each batch of concrete (Li et al., 2019). DE is a naturally porous sedimentary rock produced by the fossilized structures of diatoms; having an average porosity upwards of 90%, DE is frequently used for insulation, filters, fillers, and adsorbents. LS, a highly abundant rock, can easily be broken down into a powdered form to offset cement volumes. Adding LS to a concrete mixture improves early-age properties, such as setting time and water demand, and improves mechanical strength (Li et al., 2019). In addition to admixtures, other forms of concretes with finer aggregate sizes and fiber reinforcements have been developed which promote considerably higher mechanical strengths and lower water requirements, a potential step towards reducing global emissions and waste produced by the concrete industry (LaFarge Holcim, n.d). This form of concrete is typically referred to as ultra-high-performance concrete (UHPC) with a leading brand in the industry being LaFarge Holcim’s product, Ductal, which uses recycled plastic fibers mixed into the uncured concrete to reinforce the material and improve resistance against crack propagation (LaFarge Holcim, n.d). Terracotta, a common form of pottery clay which has a red or orange colour, is often used for sculpting, tiling, and plant pots. It has a low heat requirement for firing and the final product during production is a porous ceramic material (Hansen, n.d). Terracotta has been used as a substrate for studying coral attachment due to its abundant and inexpensive nature (Burt et al., 2009). This current study aims to test the viability of readily available ceramic materials as solutions to manufacturing artificial reefs and other foreshore structures with the goal of promoting biodiversity and conserving coastal marine environments. Existing research on concrete used for marine infrastructure suggests that over sufficient time, film formation and total coverage by marine organisms occurs regardless of surface conditions (Lapinski et al., 2022). With that in mind, this experiment focused on examining the short-term effects of material properties during the initial stages of recruitment. We compare each material’s ability to accumulate algae and other microscopic species in forming initial biofilms as an indicator of potential success as a reef building material. Surface pH and porosity were also used to characterize the performance of testing materials in relation to biofilm growth.
2 Materials and methods
5 common building materials were tested using the Outdoor Aquatic Unit (OAU) at the University of Victoria to observe the growth of biofilms on the selected materials. The goal of using these materials was to perform the test using low-cost materials while also exploring alternatives to plain concrete by using limestone and diatomaceous earth admixtures; terra cotta clay was also tested as an alternative to concrete entirely (Table 1, Figure 1). Each concrete sample was manufactured using concrete test molds to create 76mm diameter cylinders which provided a dimensionally consistent surface area when measuring algae growth (Paragon Products, n.d). The samples were manufactured using the 152mm high cylinder molds and cut using a tile saw into sample pucks approximately 38mm in height. By creating smaller pucks, the number of molds and overall volume of materials required for testing can be reduced to limit waste and minimize cost. During the curing process of concrete, carbonation occurs and forms a calcium carbonate layer on the surface of the samples. To clean excess carbonation powder from the surface and to reduce surface alkalinity, the concrete was scrubbed with muriatic acid in a process known in the concrete industry as an efflorescent wash; this wash uses acid to neutralize exposed surfaces and remove buildup (Ecosi, n.d). The terra cotta samples were acquired separately from the concrete as there was significant difficulty in sourcing a pottery studio or kiln to make proper samples with. The alternative to this was in the form of terracotta plant pot saucers; not only were the materials simple to come by, the diameters of the saucers were also similar to that of the concrete cylinders at 87mm and 76mm respectively.
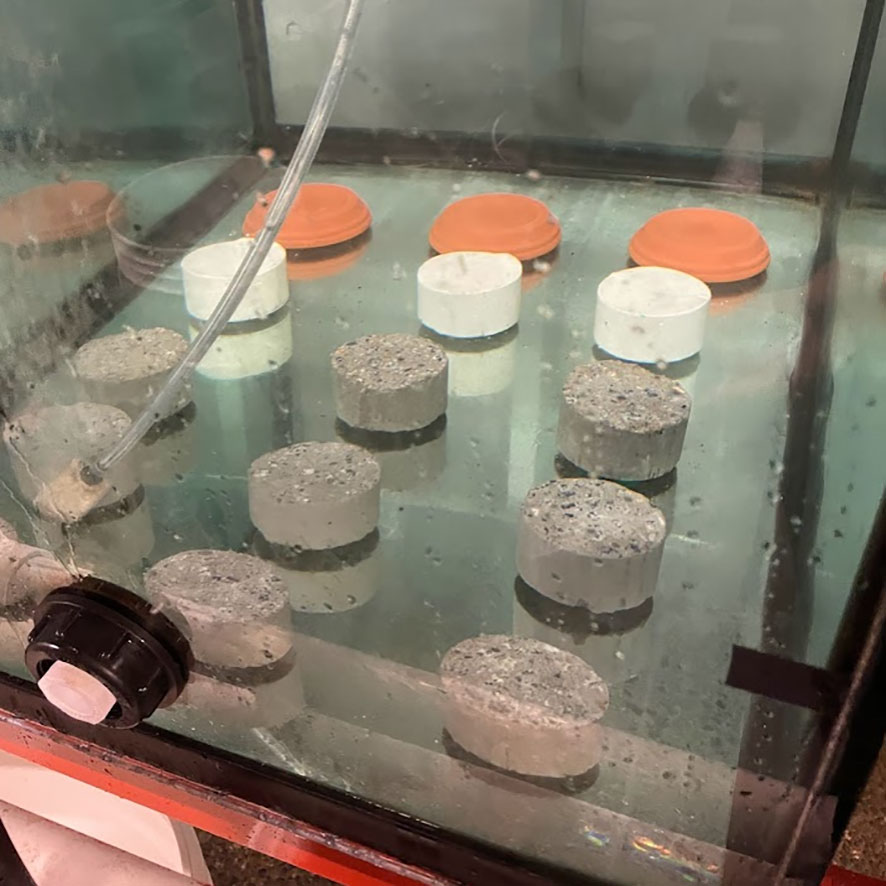
Figure 1 Image showing gray samples, including pre-mixed Portland Concrete (PC), 70% PC and 30% powered limestone (PLC), 70% PC and 30% diatomaceous earth (PDC), ductal UHPC (DUC), and Terra Cotta (TER) at the beginning of underwater testing.
A set of each material was subjected to 3 separate conditions:
● Green Sample Testing Conditions (n=3): Submerged in circulated seawater in a 400L outdoor painted fibreglass tank pre-populated with algae and rocks to monitor biofilm formation across materials. The samples were exposed to direct sunlight during this period.
● Gray Sample Testing Conditions (n=3): Submerged in circulated seawater in a 175L indoor glass tank without any algae to monitor any change to material bulk in seawater without biofilms (Figure 1). The samples were not exposed to any natural light.
● Dry Sample Testing Conditions (n=3): Kept in an ambient state out of water as the control set.
The materials were weighed, and approximate density was calculated based on volume displacement in water prior to testing. Surface pH was taken by placing distilled water on the material surfaces, leaving it stagnant for 1 minute, then measuring the water pH with litmus strips. Seawater for testing was collected from the Pacific Ocean near Victoria, BC and was provided by the OAU. Seawater is collected by the OAU which is then recirculated through facility testing tanks. Water at the facility is filtered, aerated, and chilled during recirculation. Temperatures were recorded daily within a range of 11-12°C; however, flowrate data was unavailable. The grey and green samples were submerged for 78 days from November 24, 2021 to February 10, 2022; each set of samples were monitored weekly to observe and visually document algae growth. Following seawater testing, the materials were removed from the water and the grey samples were weighed to measure the amount of water absorbed during submersion. The wet masses of the grey samples were then used to calculate an approximate value of material porosity (Equation 1).
Equation 1: Equation for calculating material porosity.
The green samples were removed from the water and film growth from each sample was scraped into aluminum weigh boats and dried at 60°C for 44 hours; after drying, the films were weighed to acquired values for dried biomass per unit of surface area. Figure 2 shows a comparison of the green sample tank on the first day of testing beside the final testing day. Surface pH after submersion was measured using the same procedure shown above to observe alkalinity change over the 2-month test period and to compare differences in pH across the 3 test conditions. The data collected from biomass, porosity, and pH was then compiled to determine if the two material properties had any affect on biofilm growth.
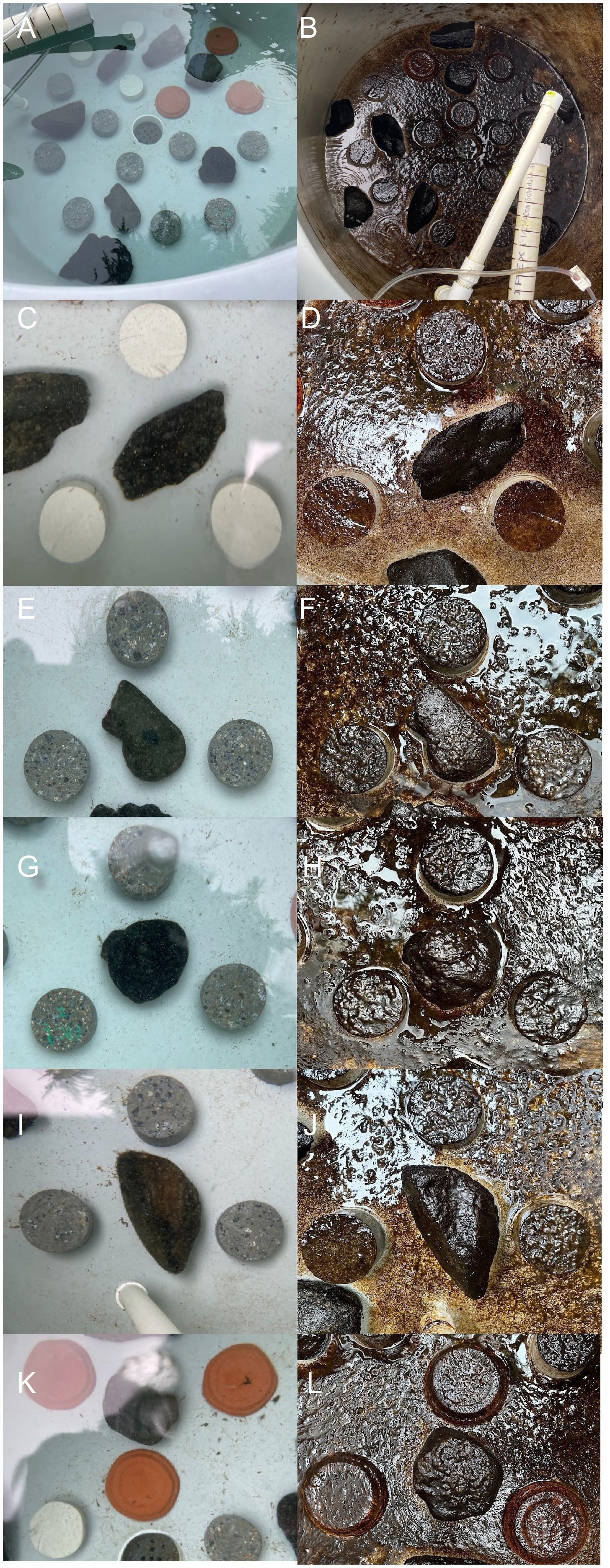
Figure 2 Images showing before (A) and after (B) pictures of biofilm growth testing over 78 days for all groups. Before (C) and after (D) pictures of biofilm growth on DUC. Before (E) and after (F) pictures of biofilm growth on PC. Before (G) and after (H) pictures of biofilm growth on PDC. Before (I) and after (J) pictures of biofilm growth on PLC. Before (K) and after (L) pictures of biofilm growth on TER.
3 Results
Samples were manufactured as described in Methods and they can be seen in Figure 1. Biofilm was observed to spread from the transplanted rocks onto surrounding samples over the two-month test period (Figure 2). Photo documentation was used to visually track progress of each sample. Qualitatively, PC and PDC samples accepted the growth most readily and were the quickest to form layers of biofilm, followed by PLC, DUC, and finally TER. Table 2 contains the data for post-submersion pH, porosity, and biomass with Table 3 representing the averages of each dataset. Using dried biomass data, linear regressions were created to visualizing any correlation between biofilm success and porosity or surface pH. There appeared to be a correlation of porosity to biomass where an increase in porosity produced greater amounts of biofilm on the test surfaces (Figure 3). Figure 4 uses a linear regression to visualize data for biofilm success and pH for both pre- and post-submersion. For post-submersion surface pH, the mass of the biofilm appeared to decrease on more alkaline surfaces; however, there was significant variance in biomass from samples with similarly measured post-pH values of 8.5 which could also imply that other factors are greater influences on mass than alkalinity. Figure 5 visualizes the change in pH after submersion for each material.
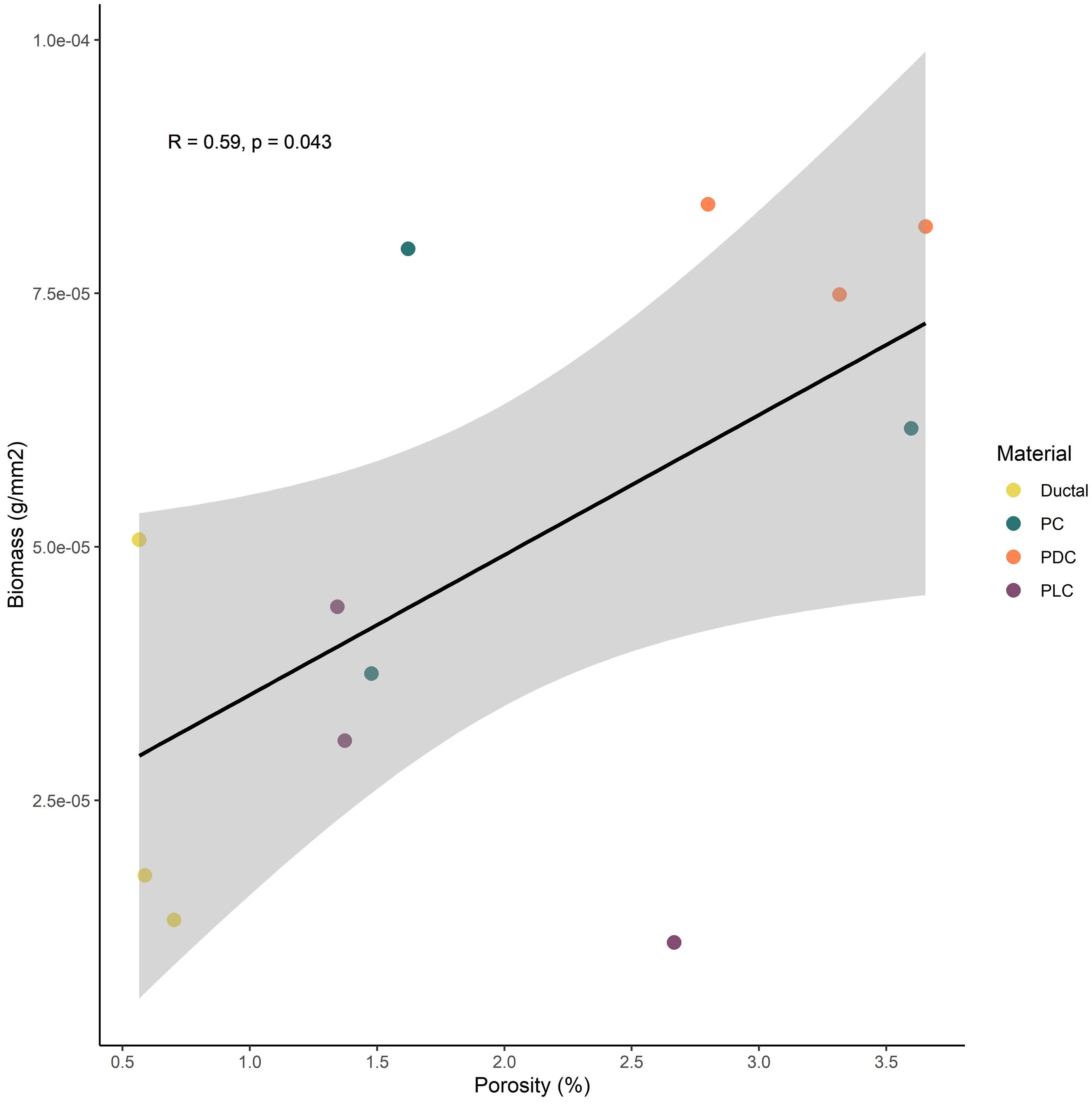
Figure 3 Linear regression of biomass in comparison to material porosity based on material composition.
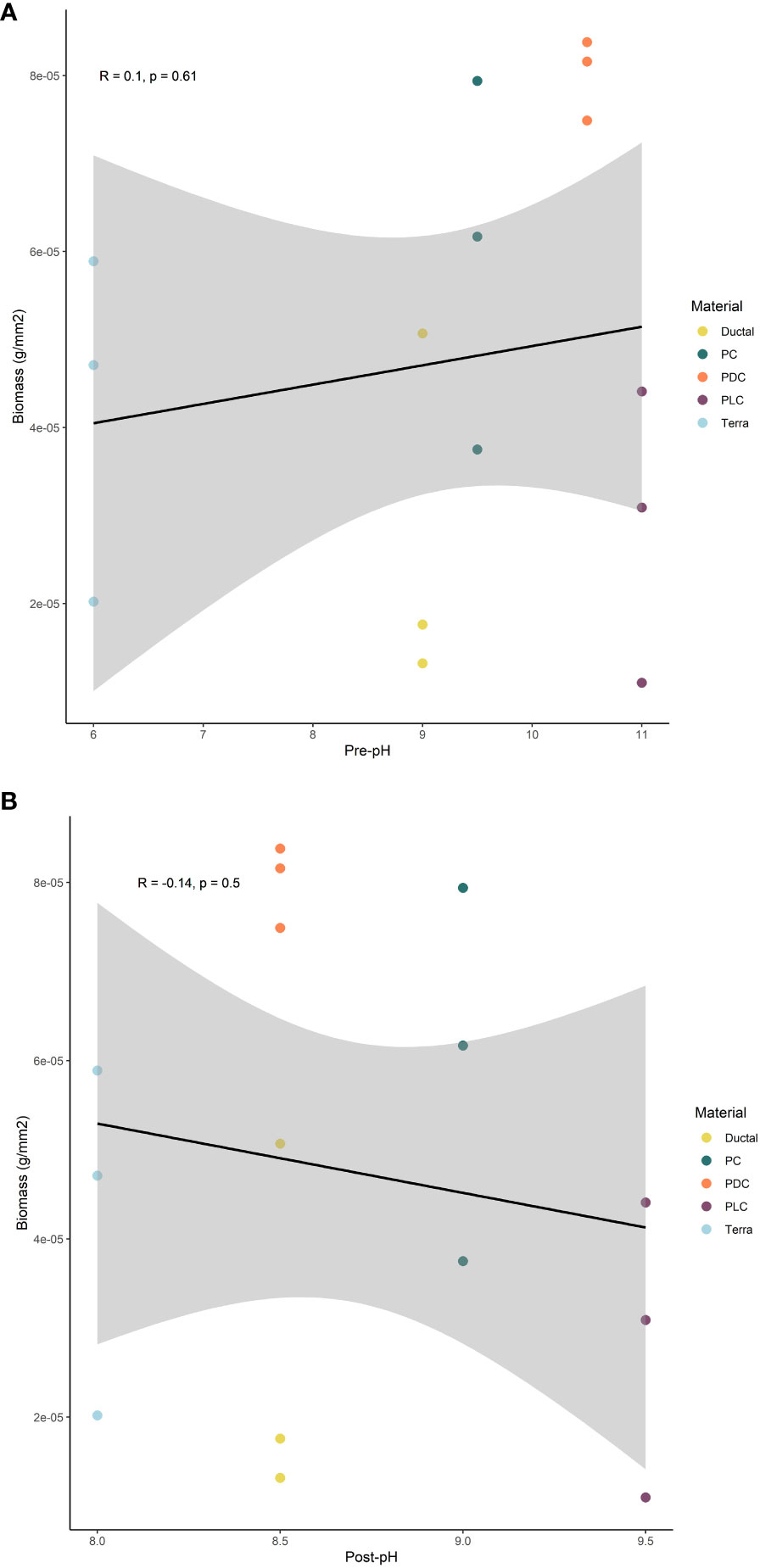
Figure 4 Linear regression of biomass in comparison to surface pH before submersion (A) and after (B) based on material composition.
All statistical analyses were performed in R version 4.1.2 (R Core Team, 2023). We tested the relationship between porosity and biomass using a Pearson correlation test, and between pH and biomass using a Kendall rank correlation test. Samples of terra cotta were excluded from the comparison of porosity and biomass due to strong outlying porosity values in comparison to other materials (off by a magnitude of 10). We found a significant positive relationship between porosity and biomass (p = 0.04) but no relationship between pre- or post-pH and biomass.
Material degradation was observed through measuring the mass of the gray samples after drying in ambient air for 2-months after testing (Table 4). The average concrete sample degradation was 1.02% with a standard deviation of 1.15%, with PLC samples degrading the most and DUC samples experiencing negligible differences in mass of 0.1%; terracotta samples all retained water at an average mass increase of 2.3% and standard deviation of 0.7%. While terracotta samples consistently retained water, likely due to amount of water absorbed during testing, most of the concrete samples experienced some amount of degradation throughout the test period. Concrete degradation was less than 2.8% for all cases which may be attributed to damage and flaking during transportation; some samples did measure a mass greater than the initial dry mass, implying they were not completely dried after the 2 months following the test period.
4 Discussion
4.1 Manufacturing of samples
Manufacturing limitations resulted in a loss of precision when cutting concrete pucks. The saw used did not have a large enough blade to cleanly cut through the sample, causing ridges and slightly angled surfaces on the testing face. These blemishes were assumed to be insignificant to the overall surface area of the test face, and biofilm formation was observed to have no noticeable differences between the edges and smooth cuts. There was significant difficulty to source pottery studios or even gain kiln access for manufacturing the terra cotta samples. Eventually, the plan to test custom terra cotta pucks was abandoned and clay plant pot trays purchased from Canadian Tire were used instead. Fortunately, the plant trays had a similar diameter to the desired sample size and was deemed a suitable substitute since biomass is measured as mass per unit surface area, making the diametral differences negligible. In the future, 3D printing of ceramic materials would be an effective method to generate more consistent samples. It also enables the possibility of making structures that resemble the structure of the coral reefs found in the ocean.
4.2 Testing arrangement factors
Here we discuss some of the limitations of our testing methods. The OAU is located behind UVic’s engineering buildings and the outdoor tanks receive direct, overhead sunlight for only a few hours per day. This effect in combination with the orientation of the seawater tanks relative to the sunlight resulted in terra cotta and Ductal samples receiving less direct sunlight than PDC, PC, and PLC samples. The level of impact the non-uniform sunlight irradiation had on testing is unknown, although Figure 6 shows a distinct band of growth on the side of the testing tank most exposed to sunlight which may suggest samples placed on the side with more sunlight experienced larger amounts of biofilm formation. Another potential cause of the difference in growth on opposite sides of the cylinder wall maybe be from the water circulation system which pumps small streams of water into the tank through the PVC tubes seen in the figure; the water streams are directed to one side of the tank, directly in front of the area of lower growth. This flow could create pooling of biofilm organisms on the western side of the tank, further from the water inlet area which would create an uneven distribution of growth. Experimental design of this project was partially driven by timeline restrictions, testing was limited to a roughly 2-month window during the academic year; further tests should be conducted over multiple years or use staggered start-dates for multiple tests to examine the differences in film growth across varying seasons. Future testing would be best conducted on a larger scale, using one tank per material to have a more consistent test case while also being able to directly study the effects of water turbidity and sunlight on each material by observing differences from the east and west sides of the tanks. Following the controlled large-scale testing, an additional test should be conducted by submerging samples in a coastal field site to achieve a better representation of what organisms interact with the materials, and how currents and other disturbances may affect AR success.
4.3 Material property assessment
The pH of each material was measured before and after testing, and the alkalinity dropped significantly for all concretes while the terra cotta samples increased from their initial acidic state. Overall, the materials’ surface pH approached a value around 8.0, near the alkalinity of seawater. As the materials equalize with their environment during submersion, they create a more favourable growing surface over time. PDC and TER samples appeared to be most influenced by the equalization, with PDC going from approximately 10.5 down to 8.5 and TER rising from 6.0 up to 8.0; the pH of DUC changed the least dropping from 9.0 to 8.5. Although PC and PLC samples had higher final pH values, they also had some of the highest initial conditions; given enough time, the samples could equalize further towards the water’s pH level. While all materials neutralized with the surroundings, materials that neutralize more readily would be better suited for artificial reef applications to quickly create favourable growing conditions for biofilms. Existing research confirms that algae attachment is encouraged on surfaces that have a lower pH while also suggesting that organisms such as barnacles favour higher pH environments (Guilbeau et al., 2003). With the variation in desirable pH in mind, consideration should be given to what organisms are to be promoted in each environment and how different material combinations may accommodate growth.
Surface porosity was measured by comparing the dry mass prior to testing against the saturated mass immediately after the 2-month submersion period; while this was effective as a quick and rudimentary measurement of porosity by estimating volume of water retained, it was widely inconsistent across samples with some material sets having a standard deviation of 43% and others with 5% deviation. TER samples were omitted from the porosity regression analysis due to their high measured porosity which should be expected as terracotta is known to allow water to pass through its bulk relatively easily. More accurate methods of measuring surface porosity may provide insight into relations between biofilm formation; however, manufacturing inconsistencies could have attributed to the considerable variation in porosity since vibration is often used to remove air bubbles produced in the mixing and pouring of concrete, a procedure which was not available during the sample preparation. The samples were observed through SEM to view their microstructure at 30x and 8000x magnification; however, with concrete’s non-homogenous nature due to containing aggregate of numerous materials, it is difficult to provide useful assessment from such a small area of the test material’s surfaces (Figure 7).
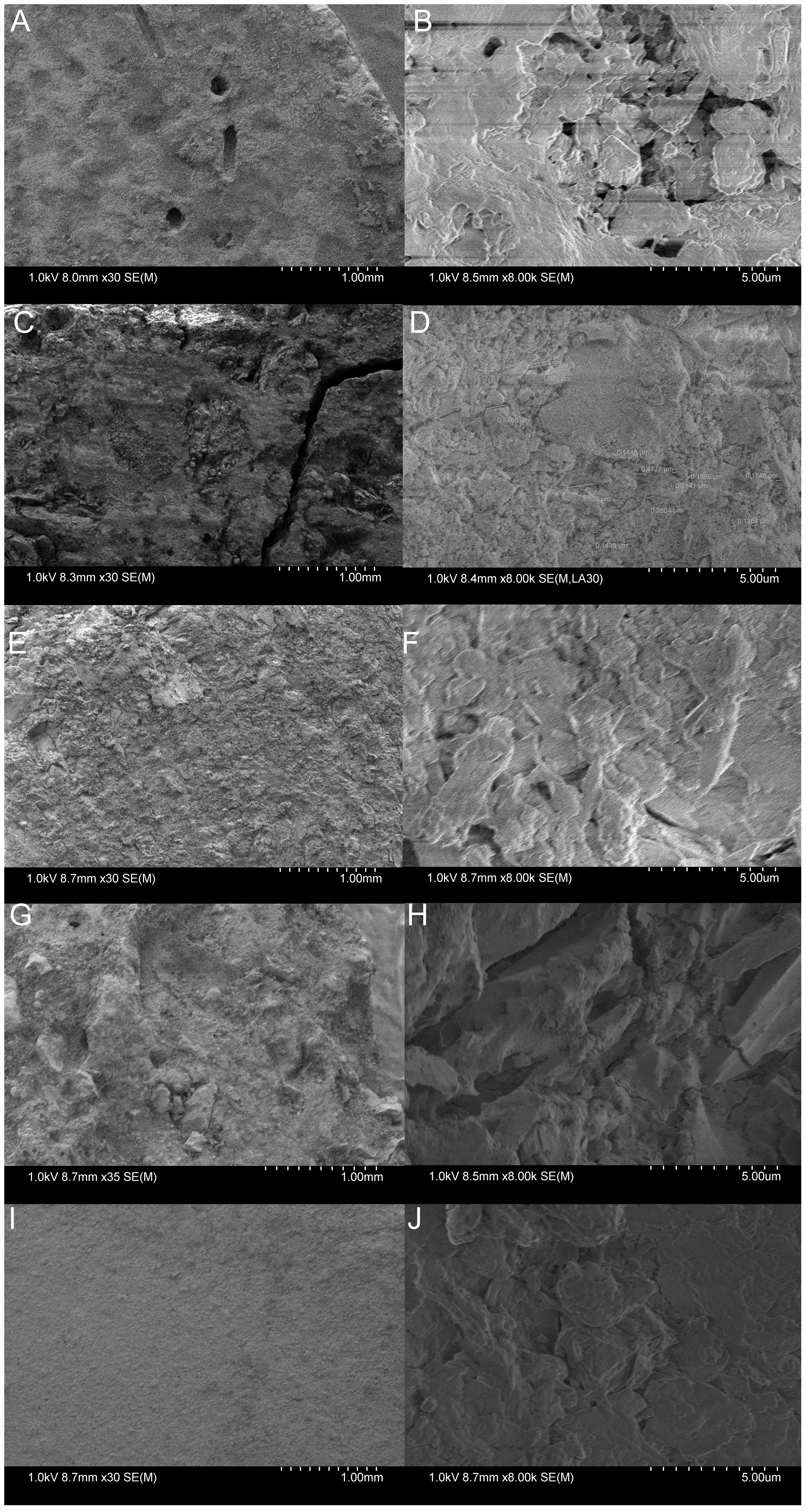
Figure 7 Scanning Electron Microscopy performed on the different samples. (A) DUC at 30x magnification. Note the lack of large aggregates due to DUC’s powdery cement when dry. (B) DUC at 8,000x magnification. Signification charging occurred while viewing the sample. (C) PC at 30x magnification. Aggregates of varying size can be seen, contributing to surface roughness. (D) PC at 8,000X magnification. (E) PDC at 30x magnification. (F) PDC at 8,000x magnification. Note the looser-packed substrate potentially contributing to greater measured porosity. (G) PLC at 30x magnification. (H) PLC at 8000x magnification. (I) TER at 30x magnification. Surface is notably smoother than concrete samples. (J) TER at 8,000x magnification.
Flow simulations suggest that algal spores are more likely to settle on artificial reef surfaces in areas of lower turbidity; rougher surfaces and grooved features can affect the boundary layers to potentially promote an increase in algal growth (Jiang et al., 2021). Grooves or recesses can provide shelter to smaller organisms, increase surface area, and reduce turbulent flow to allow for better biofilm formation. Given the significant effects large surface features have on artificial reefs, it may result in porosity being a somewhat negligible design consideration provided the surface is at least compatible with the biofilm organisms.
4.4 Future work
While material properties associated with biofilm success were the focus of the report, other properties affecting their usefulness as an artificial reef material such as compressive strength, exposure to sunlight or chemical leeching were not examined. Though PDC samples were most successful in forming a biofilm, it was noticeably more fragile than other concrete samples; furthermore, Ductal concrete samples were the least porous material but provide considerably higher strength than the other test materials. Porosity of concrete can be influenced by its manufacturing method through the removal or introduction of air bubbles, in addition to the use of porous or water-soluble aggregate to offset the amount of non-porous admixtures and Portland cement. With an understanding of what features are crucial to AR development, suitable materials can be chosen based on structural or environmental requirements and then modified through manufacturing processes to achieve the desired porosity and surface pH. Beyond material selection, artificial reefs require design complexity to provide enrichment and shelter to organisms through recesses and caverns; further testing should involve the development of scalable and easy reproduced structures which could be assessed based on their ability to attract local organisms or promote an increase in population of target species. Given the time required for materials to neutralize with the environment and form a biofilm, it is likely that a study of this size would require several months or years to examine long-term success. However, it could provide useful information on how existing marine infrastructure can be adapted to better support coastal ecosystems.
5 Conclusion
Through the analysis of biomass accumulated on test samples over a 2-month period, porosity may influence the success of biofilm formation while surface pH appears to be a lesser factor. Using this information may be beneficial when indicating a material’s suitability as an artificial reef material. With biomass increasing with porosity, and decreasing with alkalinity, an ideal reef building material would likely be very porous with a surface alkalinity near that of seawater’s pH or one that can neutralize with its environment in a short period of time. The results of this study were consistent with existing research and while the study was effective in determining some of the factors affecting artificial reef success through biofilm formation, more work should be conducted regarding the application of ecologically supportive infrastructure and methods for enhancing the biodiversity of developed coastal areas.
Data availability statement
The original contributions presented in the study are included in the article/supplementary material. Further inquiries can be directed to the corresponding author.
Ethics statement
The manuscript presents research on animals that do not require ethical approval for their study.
Author contributions
LO: Conceptualization, Data curation, Formal analysis, Investigation, Methodology, Project administration, Writing – original draft, Writing – review & editing. SW: Conceptualization, Funding acquisition, Methodology, Project administration, Resources, Supervision, Writing – review & editing.
Funding
The author(s) declare financial support was received for the research, authorship, and/or publication of this article. This work was funded by an NSERC Discovery Grant held by by Dr. Stephanie Willerth and the Canada Research Chairs program.
Conflict of interest
The authors declare that the research was conducted in the absence of any commercial or financial relationships that could be construed as a potential conflict of interest.
The author(s) declared that they were an editorial board member of Frontiers, at the time of submission. This had no impact on the peer review process and the final decision.
Publisher’s note
All claims expressed in this article are solely those of the authors and do not necessarily represent those of their affiliated organizations, or those of the publisher, the editors and the reviewers. Any product that may be evaluated in this article, or claim that may be made by its manufacturer, is not guaranteed or endorsed by the publisher.
References
Airoldi L., Balata D., Beck M. W. (2008). The Gray Zone: Relationships between habitat loss and marine diversity and their applications in conservation. J. Exp. Mar. Biol. Ecol. 366 (1-2), 8–15. doi: 10.1016/j.jembe.2008.07.034
Burt J., Bartholomew A., Bauman A., Saif A., Sale P. F. (2009). Coral recruitment and early benthic community development on several materials used in the construction of artificial reefs and breakwaters. J. Exp. Mar. Biol. Ecol. 373 (1), 72–78. doi: 10.1016/j.jembe.2009.03.009
Cefalì M. E., Cebrian E., Chappuis E., Pinedo S., Terradas M., Mariani S., et al. (2016). Life on the boundary: Environmental factors as drivers of habitat distribution in the littoral zone. Estuarine Coast. Shelf Sci. 172, 81–92. doi: 10.1016/j.ecss.2016.01.043
Ecosi Products - brisolve. Available at: https://www.ecosi.ca/produits/ (Accessed 13 August 2023).
Guilbeau B. P., Harry F. P., Gambrell R. P., Knopf F. C., Dooley K. M., et al. (2003). Algae attachment on carbonated cements in fresh and brackish waters—preliminary results. Ecol. Eng. 20 (4), 309–319. doi: 10.1016/S0925-8574(03)00026-0
Hansen T. Terra cotta. Available at: https://digitalfire.com/glossary/terra+cotta (Accessed 25 January 2022).
Jiang Z., Zhu L., Mei J., Guo Z., Cong W., Liang Z. (2021). Preliminary study on the boundary layer of an artificial reef with regular grooves surface structure. Ocean Eng. 219, 108355. doi: 10.1016/j.oceaneng.2020.108355
LaFarge Holcim Ductal: about. Available at: https://www.ductal.com/en (Accessed 25 January 2022).
Lapinski M., Perrot M., Sauleau P. (2022). “Can port concrete infrastructures be optimized to promote algal and macrofaunal colonisation in the marine intertidal zone? Case study of Port Haliguen (Brittany, France),” in IOP Conference Series: Materials Science and Engineering, Vol. 1245.
Levy N., Berman O., Yuval M., Loya Y., Treibitz T., Tarazi E., et al. (2022). Emerging 3D technologies for future reformation of coral reefs: Enhancing biodiversity using biomimetic structures based on designs by nature. Sci. Total Environ. 830, 154749. doi: 10.1016/j.scitotenv.2022.154749
Li J., Zhang W., Li C., Monteiro P. J. (2019). Green concrete containing diatomaceous earth and limestone: Workability, mechanical properties, and life-cycle assessment. J. Cleaner Production 223. doi: 10.1016/j.jclepro.2019.03.077
Marin-Diaz B., Fivash G. S., Nauta J., Temmink R. J.M., Hijner N., Reijers V. C., et al. (2021). On the use of large-scale biodegradable artificial reefs for intertidal foreshore stabilization. Ecol. Eng. 170, 106354. doi: 10.1016/j.ecoleng.2021.106354
Mat Jusoh S., Ghazali C. M.R., Mat Amin K. A., Zin Z. M., Wan Nik W. M.N., Mohamad N., et al. (2018). Innovative uses of recycle waste materials as an artificial concrete reef for estuarine ecosystem. IOP Conf. Series: Materials Sci. Eng. 374, 012088. doi: 10.1088/1757-899X/374/1/012088
McManus R. S., Archibald N., Comber S., Knights A. M., Thompson R. C., Firth L. B. (2018). Partial replacement of cement for waste aggregates in concrete coastal and marine infrastructure: A foundation for ecological enhancement? Ecol. Eng. 120, 665–667. doi: 10.1016/j.ecoleng.2017.06.062
Monteiro P. J. M., Miller S. A., Horvath A. (2017). Towards sustainable concrete. Nat. Materials 16 (7), 698–699. doi: 10.1038/nmat4930
Ng C. S. L., Lim S. C., Ong J. Y., Teo L. M.S., Chou L. M., Chua K. E., et al. (2015). Enhancing the biodiversity of coastal defence structures: transplantation of nursery-reared reef biota onto intertidal seawalls. Ecol. Eng. 82, 480–486. doi: 10.1016/j.ecoleng.2015.05.016
Nitsch C. K., Walters L. J., Sacks J. S., Sacks P. E., Chambers L. G. (2021). Biodegradable material for oyster reef restoration: first-year performance and biogeochemical considerations in a coastal lagoon. Sustainability 13 (13), 1–23. doi: 10.3390/su13137415
Paragon Products Concrete test cylinders. Available at: https://www.paragonproducts-ia.com/concrete-test-cylinders-c-293.html (Accessed 13 August 2023).
R Core Team (2023). R: A language and environment for statistical computing (Vienna, Austria). https://www.r-project.org/
Riera E., Lamy D., Goulard C., Francour P., Hubas C. (2018). Biofilm monitoring as a tool to assess the efficiency of artificial reefs as substrates: Toward 3D printed reefs. Ecol. Eng. 120, 230–237. doi: 10.1016/j.ecoleng.2018.06.005
Salta M., Wharton J. A., Blache Y., Stokes K. R., Briand J.-F. (2013). Marine biofilms on artificial surfaces: structure and dynamics. Environ. Microbiol. 8, 2879–2893. doi: 10.1111/1462-2920.12186
Seaman W. (2000). Artificial reef evaluation: with application to natural marine habitats (Gainesville: CRC Press).
Keywords: marine restoration, sustainable infrastructure, biocompatibility, material science, artificial reef, aquaculture, biodiversity, marine infrastructure
Citation: O’Reilly LM and Willerth SM (2024) Evaluating the biocompatibility of ceramic materials for constructing artificial reefs. Front. Mar. Sci. 10:1292584. doi: 10.3389/fmars.2023.1292584
Received: 11 September 2023; Accepted: 14 December 2023;
Published: 09 January 2024.
Edited by:
Yakun Guo, University of Bradford, United KingdomReviewed by:
Nadav Shashar, Ben-Gurion University of the Negev, IsraelTetsu Mieno, Shizuoka University, Japan
Copyright © 2024 O’Reilly and Willerth. This is an open-access article distributed under the terms of the Creative Commons Attribution License (CC BY). The use, distribution or reproduction in other forums is permitted, provided the original author(s) and the copyright owner(s) are credited and that the original publication in this journal is cited, in accordance with accepted academic practice. No use, distribution or reproduction is permitted which does not comply with these terms.
*Correspondence: Stephanie M. Willerth, d2lsbGVydGhAdXZpYy5jYQ==