- 1Eco-environmental Protection Research Institute, Shanghai Academy of Agricultural Sciences, Shanghai, China
- 2Institute for Research in Molecular Medicine, Universiti Sains Malaysia, Penang, China
- 3Freshwater Fisheries Research Institute of Jiangsu Province, Nanjing, China
- 4Nanjing Institute of Environmental Sciences, Ministry of Ecology and Environment, Nanjing, China
Nanoplastics (NPs) have emerged as contaminants in recent years and have attracted widespread attention because of their ecotoxicological effects. This study aimed to document the effects of different concentrations of NPs on the Monopterus albus. M. albus. M. albus were orally administered three different concentrations of 100 nm polystyrene NPs (0.05%, 0.5%, and 1% of the feed) for 35 days. The effects of different NPs concentrations on energy metabolism, enzyme biomarker responses, gene expression responses, and amino acid changes were investigated in M. albus after exposure. The results revealed that the gene expression of phosphoenolpyruvate carboxykinase and glucose-6-phosphatase was up-regulated after the ingestion of high concentrations of NPs. The gluconeogenic pathway was inhibited, lactic acid (LA) content was increased, anaerobic glycolysis was used to produce LA to power the organism, and the accumulation of NPs led to a decrease in total cholesterol and triglyceride levels in liver tissues. There were increases in the relative liver content of glutamine, glycine, and methionine, which may be due to antioxidation in the liver. The stress may caused by NPs leads to the formation of some glutamylated amino acids, which are converted into glutathione to play an antioxidant role. NPs also induced lipotoxicity of the liver organoid by increasing lipid accumulation, these include methyl tetradecanoate (myristate), pentadecanoic acid, eicosanoic acid (arachidic acid), heptadecanoic acid (margaric acid), 5,8,11,14-eicosatetraenoic acid, and doconexent (Docosahexaenoic acid). Interestingly, some immune-related metabolites, such as 9-octadecenoic acid (oleic acid) and 9,12-octadecadienoic acid (linoleic acid), were significantly reduced, and these changes were probably caused by disturbances in hepatic lipid metabolism following NPs exposure.
1 Introduction
The accumulation of plastic waste in the aquatic environment is one of the common and persistent ecological problems on Earth, with significant impacts on ecosystems, food safety, and human health. At present, microplastics (MPs) are accumulating in the global marine and freshwater ecosystems, and the environmental problem of pollution has become the focus of global attention(Lamichhane et al., 2023). MP particles (<5 mm diameter) are found ubiquitous, from the Arctic to the Antarctic, from thin air layers to deep dense seawater, and from remote land to the middle of the ocean (Rochman, 2018). Over time, the plastic disintegrates into MPs and nanoplastics (NP <0.1 μm diameter) (Smith et al., 2018). When NPs are ingested and accumulate in different tissues of aquatic organisms, they can affect their biological systems. NPs are bioaccumulated in fish liver and muscle and cause DNA damage after a chronic exposure (Issac and Kandasubramanian, 2021; Brandts et al., 2022) and may clog the digestive system (Van Dyck et al., 2016). NPs can also adhere and enter to the gills, transfer to various tissues (Li et al., 2022b).
Currently, studies on the effects of NPs on aquatic organisms have been conducted mainly in populations and individuals (Gong et al., 2023). Exposure of aquatic organisms to NPs can affect enzyme activity, as well as cholesterol, glucose, and fat levels (Brandts et al., 2018). NPs have been reported in bivalves, copepods, and fish to raise the levels of reactive oxygen species (ROS), cause the buildup of cellular oxidative damage, and affect the levels, activity, or expression of antioxidant biomolecules (Trevisan et al., 2022). Teng et al. found that the accumulation of NPs in the zebrafish gut caused intestinal inflammation and inhibited zebrafish growth and development (Teng et al., 2022). When mammals consume aquatic organisms containing NPs, they accumulate in the liver, kidneys, and intestines of the organism, causing energy and lipid metabolism disorders, as well as oxidative stress (Trevisan et al., 2022). However, few researches have been done on the effect of NPs on energy metabolism and amino acid changes in freshwater fish.
M. albus is a fish that lives in the coastal areas of China, Japan, and the Korean Peninsula (Shafland et al., 2009). In recent years, with the increasing use of various agricultural chemicals and the discharge of industrial pollutants, the habitat of wild M. albus has deteriorated. The number of M. albus is also greatly reduced due to overfishing, and MPs in the water of artificially raised M. albus and the wastewater of rice fields has increased significantly (Nuryadin et al., 2020; Zhu et al., 2023). MPs and NPs are associated with ecological risks in aquaculture, however there is very little research on their toxic effects on M. albus. As shown in Figure 1, we investigated the effects of adding different concentrations of NPs to feed on liver energy metabolism, as well as changes in amino acids and fatty acids. The results of this study provide valuable ecotoxicological data for better evaluating the impact of NPs on artificially cultured M. albus.
2 Materials and methods
2.1 Culture of M. albus
The experimental M. albus were obtained from the Shanghai Academy of Agricultural Sciences. All the M. albus were healthy individuals with an average weight of 22.7 ± 0.4 g and were bred from the same parent. During the temporary culture period, they were all raised in an 8 m × 6 m × 6 m cement pool, with water aerated continuously at a temperature of 28 ± 0.9°C and pH of 7.8 ± 0.3. The M. albus were fed with commercial feed (Hubei Zhaoliang Biotechnology Co., Ltd.) at 4 pm every day. The main ingredients of the feed were fish meal, fish oil, soybean meal, flour, yeast powder, multivitamins, and minerals. Approximately one-third of the total volume of water was replaced before feeding.
2.2 Polystyrene NPs
Polystyrene NPs were purchased from BaseLine Chromtech Research Centre in Tianjin, China. These NPs were monodisperse polystyrene microspheres with a diameter of 100 nm and a concentration of 10 mg/mL (5.32 × 1012 particles/mL). In this study, fish meal, soybean meal, and soybean protein concentrate were used as protein sources, α-starch was used as a binder and a source of carbohydrates, and fish oil was used as a lipid source. Additionally, all components were crushed into a fine powder and sieved through a 320 μm mesh for A, B, and C groups, where the NPs were 0.5, 5, and 10 g/kg, respectively. The materials for the experimental meals were completely blended with fish oil using a mixer, then stored in sample bags at −20°C until use.
2.3 Toxicity test
After temporary cultivation, the M. albus were divided into four groups, namely A, B, C, and CK (E). Each group consists of three repeated water tanks, with four M. albus fed in a 10 L water tank. The CK (E) group of M. albus received normal feed without NPs, while the A, B, and C groups received 1%, 0.5%, and 0.05% concentrations of polystyrene NPs in the feed. The water in each water tank was changed every 24 hours and the same weight (3 g) of feed was added every day. After 35 days of NPs exposure, the liver of M. albus was removed and stored in liquid nitrogen to prepare for the subsequent enzyme activity and transcriptome experiments.
2.4 Analysis of biomarkers
Nine M. albus were collected from each group, and their livers were used to prepare tissue homogenate. The method was as follows: the livers were homogenized in phosphate buffer (50 mM monopotassium phosphate; 50 mM monopotassium phosphate dibasic; 1 mM EDTA; pH 7.0) with an IKA homogenizer (Ultra Turrax IKA T10 basic), and then centrifuged at 10000 g for 20 minutes at 4°C to obtain the supernatant. Commercial kits (Nanjing Jiancheng Bioengineering Institute) were used to determine the levels of energy metabolism-related biochemical substances, total cholesterol (T-CHO), and CoA carboxylase (ACC) according to the manufacturer’s instructions. Levels of triglyceride (TG) were analyzed by the Bucolo et al. method (Bucolo and David, 1973), and fatty acid synthase (FAS) activity was analyzed by the procedure described by Tian et al. (Tian et al., 1985). All these parameters were measured using an enzyme plate spectrophotometer (BioTek Instruments).
2.5 Gene expression
Primer 5 software was used to design primers for energy metabolism-related genes, hexokinase (HK), protein kinases (PK), phosphoenolpyruvate carboxykinase (PCK), phosphofructokinase (PFK), maltase-glucoamylase (MGAM), glucose-6-phosphatase (G6PC), and citrate (Si)-synthase (SI). The primers used for qRT-PCR were synthesized by the Anhui General Biology Company. β-Actin was used as an internal reference gene. Total RNA extraction, RNA integrity analysis, cDNA synthesis, and qRT PCR were performed as previously described (Limbu et al., 2019). Detailed information is shown in Table 1.
2.6 Proximate composition analysis
The proximate composition of the samples in different groups was determined according to methods described previously (Taşbozan et al., 2016). The data were examined by Nanjing Innovation Biotechnology.
2.7 Amino acid analysis
The powdered sample (0.2 g) was added to 0.1 mol/L hydrochloric acid (1.0 mL) in a 10 mL centrifuge tube, shaken, and placed in ice water. The centrifuge tube containing the sample, ice water, and a rack were placed together into an ultrasonic instrument. The sample was extracted for 40 minutes and shaken three times halfway through the process. The sample was then centrifuged at 4°C for 10 minutes at high speed. The supernatant was retrieved and 1 ml of 0.1 mol/L hydrochloric acid was added. The sample was extracted once according to the above steps, and the supernatant was merged twice. The supernatant was placed into a 10 mL centrifuge tube and 750 µl of 0.1 mol/L PITC acetonitrile solution and 750 µl of 1 moL/L triethylamine acetonitrile solution were added, then vortexed for 1 minute. The sample was then placed in a water bath at 25°C for 40 minutes. Then, 6 mL of n-hexane was added, vortexed for 1–2 min, and let stand for 5 min. The sample was then subjected to high-speed centrifugation for 5 minutes and extracted twice. A syringe was used to absorb the lower layer of liquid, which was passed through a 0.22 µm filter membrane and transferred to a chromatographic flask to perform liquid phase analysis. The data was tested by Nanjing Innovation Biotechnology.
2.8 Related fatty acids analysis
A uniform sample was weighed and 500 mg was transferred into a 10 mL test tube with a stopper. Petroleum ether (2 ml) was added and 1 mL of potassium hydroxide methanol solution was added to the ether mixture as a methylating reagent. The mixture was subjected to vortex oscillation and then left stationary for 1 hour. Then vortexed again, 2 mL of deionized water was added and the mixture was left to stand for 30 minutes until layers formed. It was then centrifuged at 4500 g for 2 minutes and the supernatant was removed to be analyzed using gas chromatography-mass spectrometry. The data was tested by Nanjing Innovation Biotechnology.
2.9 Statistics
The mean and standard error (Mean ± SE) of all data from this experiment were calculated using SPSS. One-way ANOVA, followed by Tukey’s multiple comparisons, were used to analyze group differences. Differences with a p < 0.05 (*), p < 0.01 (**) were considered significant, whereas those with a p < 0.001 (***), p < 0.0001 (****) were considered highly significant. Graphs were generated using the R package and GraphPad Prism 10 program.
3 Results
3.1 Effects of NPs on the energy metabolism of M. albus
Figure 2 shows the effect of different NPs concentrations on energy metabolism in the liver of M. albus after 35 days of treatment with NPs. In the 1% concentration group the expression levels of PK, HK genes in liver tissue decreases (p < 0.05). At the same time, it was observed that the expression levels of the SI, PCK, MGAM, and G6PC genes increased (p < 0.05), especially in the 1% high-concentration group, where the expression levels were the highest.
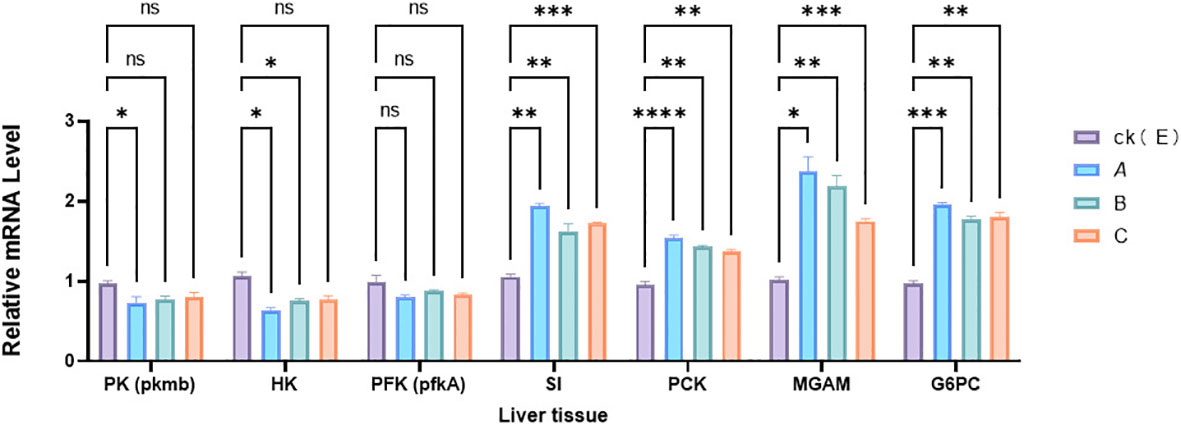
Figure 2 Effect of NPs on the expression of genes related to liver metabolism in M. albus. CK(E) indicates control samples A, B, and C groups received 1%, 0.5%, and 0.05% concentrations of polystyrene NPs in the feed. The data represents the average standard error (N=3 replicates). p < 0.001 (***), p < 0.01 (**), and p < 0.05 (*) are considered statistically significant differences, ns is no significance. Different asterisks indicate statistical differences between treatments.
To evaluate the changes in energy reserve substances in liver tissue caused by exposure to NPs, as shown in Figure 3, the levels of T-CHO and TG in liver tissue were detected. Changes occurred in T-CHO and TG liver content, especially in the 1% high-concentration group, where the content of T-CHO and TG was the lowest. At the same time, in the 1% and 0.5% concentration group, it was observed that the activity of FAS and ACC enzymes increased (p < 0.05), especially in the 1% high-concentration group, where ACC had the highest activity.
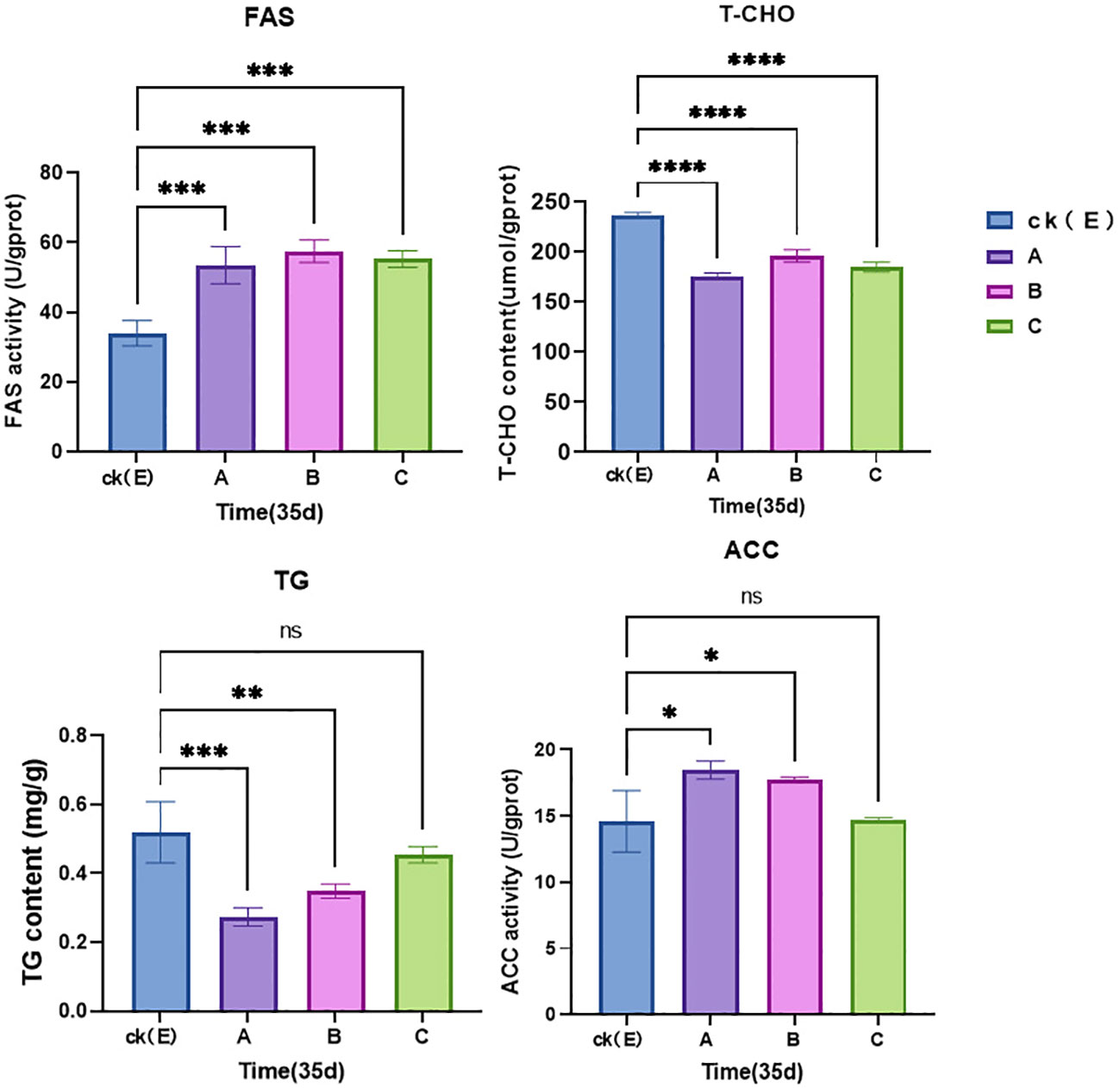
Figure 3 Relative levels of enzyme activities in the liver tissue of M. albus. CK(E) indicates control samples. A, B and C groups received 1%, 0.5%, and 0.05% concentrations of polystyrene NPs in the feed. Data represent the mean and standard errors (N = 3 replicate treatments). p < 0.0001 (****), p < 0.001 (***), p < 0.01 (**), and p < 0.05 (*) were considered statistically significant differences, ns is no significance. Different asterisks indicate statistical differences between groups.
3.2 Effects of NPs on lipid metabolism in the liver of M. albus
Significant changes in lipid metabolism in M. albus liver induced by NPs exposure were evident in Table 2. Eighteen fatty acids were found to accumulate in the liver. The levels of methyl tetradecanoate, pentadecanoic acid, eicosanoic acid, heptadecanoic acid, 5,8,11,14-eicosatetraenoic acid, and doconexent were significantly increased, and the levels of 9-octadecenoic acid and 9,12-octadecadienoic acid were significantly decreased in the A group than that in the CK(E) group (p ≤ 0.05). These data demonstrate that exposure to NPs induces the accumulation of fatty acids in the liver of M. albus and affects its immune function.
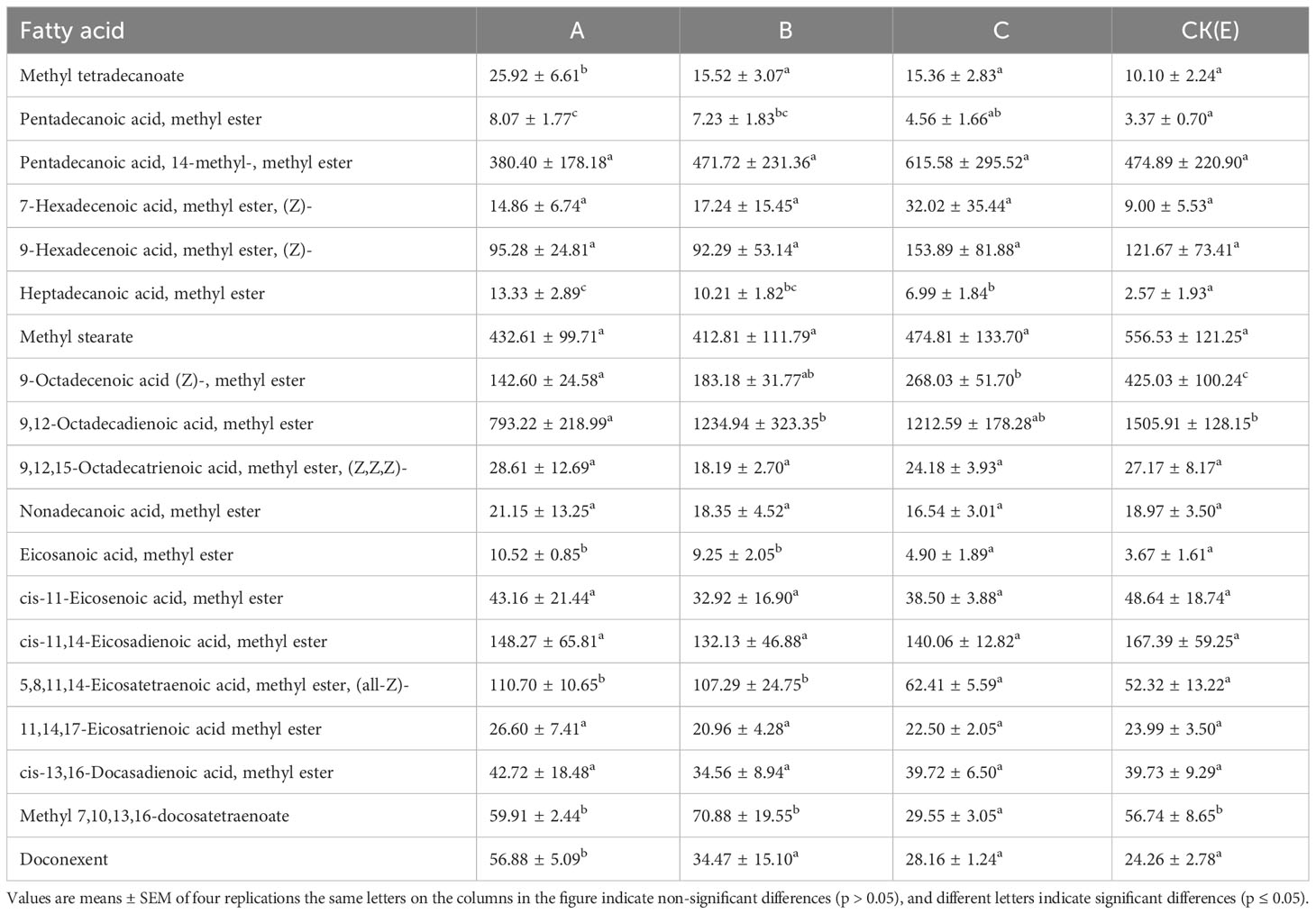
Table 2 Effects of different concentrations of NPs on fatty acid content in the liver and pancreas of M. albus (mg/kg dry matter).
3.3 Effects of NPs on the liver proximate composition and amino acids of M. albus
The content of water, crude protein, crude fat and ash in the liver of M. albus is shown in Table 3. NPs significantly increased the crude fat content, and the ash content also in the A group were significantly higher than that in the CK(E) group (p ≤ 0.05). In addition, NPs did not significantly affect the water and crude protein content of the liver and pancreas of M. albus(p > 0.05).
Eighteen amino acids in the liver of M. albus were analyzed and evaluated: Glutamate, asparagine, serine, glutamine, glycine, histidine, arginine, threonine, alanine, proline, tyrosine, valine, methionine, isoleucine, leucine, phenylalanine, and lysine. The effect of different concentrations of NPs on the amino acid content in the liver of M. albus is shown in Table 4. The levels of glutamine, glycine, and methionine increased significantly in the A group than that in the CK(E) group (p ≤ 0.05).
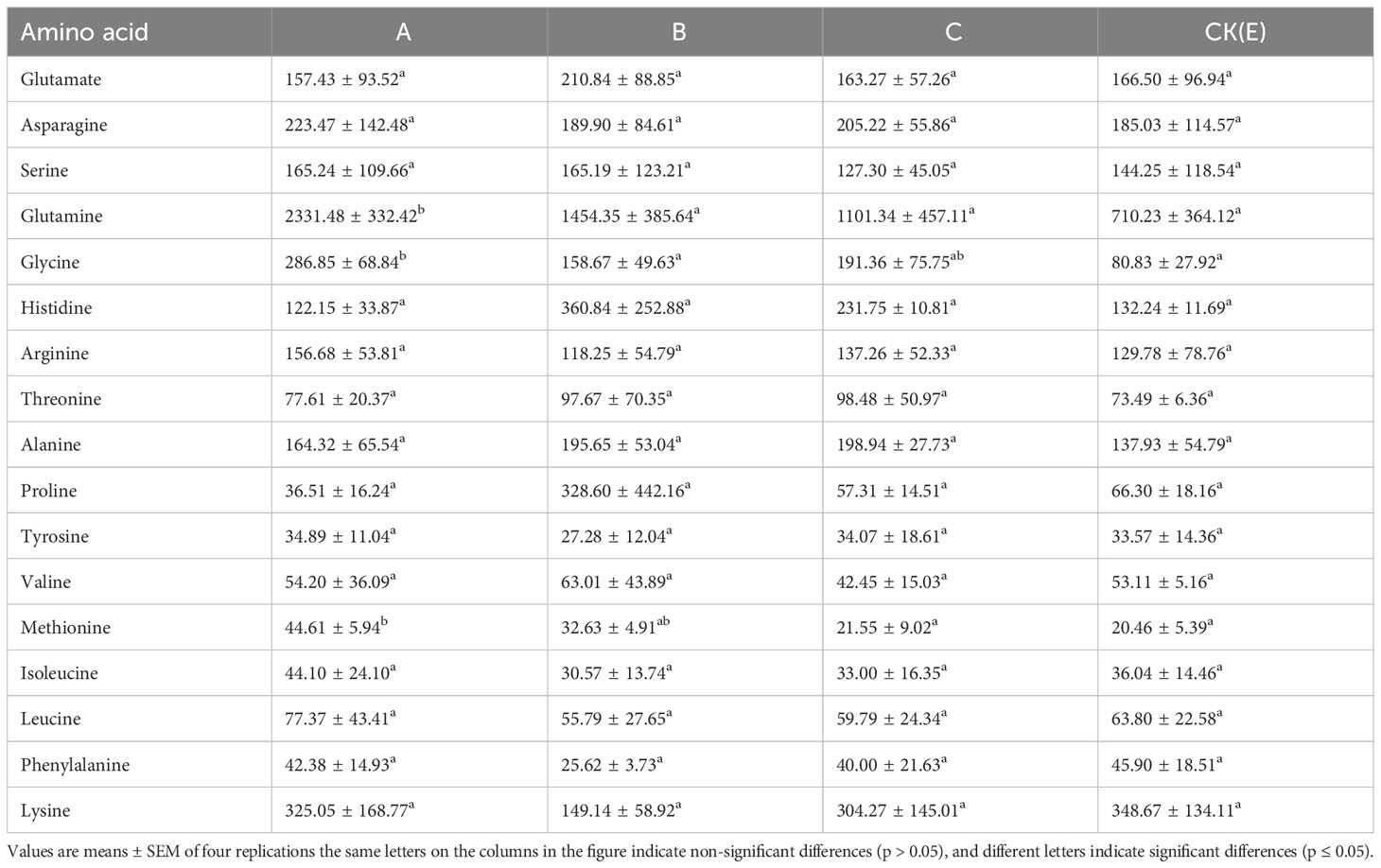
Table 4 Effect of different concentrations of NPs on amino acid content in the liver of M. albus (mg/kg dry matter).
4 Discussion
This study investigated how oral exposure to different concentrations of 100 nm plastic particles affected the liver of M. albus. Our results confirm that after 35 days of NPs ingestion, there are changes in the expression of energy metabolism enzyme biomarkers and related genes in the liver. M. albus may use increased glycolysis to provide energy to adapt to the oxidative damage of the liver after exposure to NPs. At the same time, NPs also changed the amino acid content in the liver. The experiment found that the concentration of NPs is closely related to disordered energy metabolism in the liver.
The glycolytic pathway is crucial for the metabolism of carbohydrates and glucose. When aerobic metabolism produces ATP, anaerobic glycolysis is used to produce lactic acid and provide energy for the organism (Li et al., 2022a). Previous studies have found that NPs may alter the energy related enzymes of Dicentrarchus labrax, reducing their energy reserves and nutritional quality (Barboza et al., 2018). As a limiting enzyme of glycolysis, PK catalyzes the conversion of phosphate groups from phosphoenolpyruvic acid to adenosine diphosphate (ADP), producing pyruvic acid and adenosine triphosphate (ATP) (Israelsen and Vander Heiden, 2015). HK promotes the phosphorylation of glucose to produce glucose 6-phosphate, which is the first step of the glycolysis pathway. PK and HK are important enzymes that control the direction and rate of glucose metabolism, and their levels can reflect the vitality of the body. By measuring the activities of energy metabolism related enzymes HK and PK, the effect of exposure to NPs on sugar metabolism in M. albus was studied. The results showed that with the increase of NPs concentration, the PK and HK activities in M. albus liver tissue generally decreased. Therefore, with the increase of NPs concentration, the glycolysis pathway is restricted, and the occurrence of anaerobic glucose metabolism increases. These results are consistent with a previous study conducted by Teng et al. (Teng et al., 2021). NPs may cause changes in metabolites and disrupt some mechanisms, including oxidative stress, immune regulation, and Trojan horse effects, and even energy metabolism(Li et al., 2021). A relevant research shows that zebrafish exposed to NPs increase glucose consumption, and have a shortage of energy supplies (Chen et al., 2020). Therefore, our results indicate that exposure to NPs can have a negative impact on glucose metabolism in M. albus significantly inhibits the glycolytic pathway and leads to a shift from aerobic metabolism to anaerobic metabolism.
Gluconeogenesis is the process by which fresh glucose is created in animals from common metabolites such as amino acids, glycerol, lactate, pyruvate, and intermediate tricarboxylic acid cycle metabolites (TeSlaa et al., 2021). Of these metabolites, only amino acids and glycerol lead to net glucose production. Lactate, the main gluconeogenic substrate, is synthesized from glucose but can be converted back to glucose using fuels made from fat (TeSlaa et al., 2021). So, during the process of energy metabolism, glucose content is in a steady state. This is also true of alanine, another important glycolysis byproduct. It is used to return amino groups from other organs to the liver. Glutamine, as an amino shuttle from other organs to the kidneys, can once again be quantitatively converted into glucose using energy from fat sources (Nuttall et al., 2008). PCK and G6PC are the limiting enzymes of the gluconeogenesis pathway (Tang et al., 2018). In this study, we found that when M. albus was exposed to NPs, the expression levels of PCK and G6PC genes were up-regulated, whereas the glucose production pathway was inhibited. The double enzyme (sucrase/maltase) system completes carbohydrate digestion. The digestion of carbohydrates such as sucrose and starch in aquatic organisms is believed to be widespread. In aquatic organisms, SI and MGAM can not only hydrolyze maltose but also starch (Diaz-Sotomayor et al., 2013). MGAM seems to have a higher activity to oligosaccharides with higher residue number, MGAM has higher activity to oligosaccharides with higher residue number than SI and has higher specificity to maltose (Lee et al., 2016). Therefore, the combined effect of SI and MGAM is crucial for digesting food sources of α-maltose. When M. albus was exposed to NPs for a short time, the expression of SI and MGAM genes increased, which helped to hydrolyze maltose and starch obtained from feed.
Fish must strictly regulate lipid storage and mobilization in order to adapt to their living environment and meet their energy needs for physiological activities. The lipids in feed are absorbed and transported to storage sites, usually in the form of TG combined with proteins to form chyle particles (Van de Pol et al., 2017). During lipid metabolism, stored TG is hydrolyzed in a stepwise manner into free fatty acids and glycerol. The hydrolysis reaction is catalyzed by different lipases (Althaher, 2022). Fish lipid metabolism is a very complex process, and the influencing factors are also diverse. Therefore, how to improve fish’s stress resistance and nutrient utilization efficiency through lipids, as well as the impact of certain fatty acids on exposure to NPs, is one of the future research directions that needs to be studied. Lipids are energy-rich compounds and are generally considered indicators to evaluate the nutritional status and health of aquatic animals (Filimonova et al., 2016). Lipids are an important source of energy reserve in crustaceans (Glover, 2019). In addition, lipid metabolism is the main pathway for providing energy in organisms and plays an important role in the response of crustaceans to environmental pressure (Teng et al., 2021). Various studies have shown that partial pressure of carbon dioxide, food quality, hunger, salinity, and chemical exposure can affect lipid metabolism in aquatic invertebrates (Yoon et al., 2022). As the main components of lipids, TG and T-CHO play key roles in the metabolism of organisms. Our research shows that NPs cause a decrease in T-CHO and TG levels in the liver of M. albus, which is probably due to lipid metabolism disorders caused by exposure to NPs. Similarly, lipid metabolism disorders were also observed in other fish exposed to NPs (Von Moos et al., 2012; Wan et al., 2019; Ye et al., 2021).
FAS and ACC activities can be used to evaluate the digestive and metabolic abilities of animals (Yue-qiang et al., 2010). The increase in ACC and FAS activity in M. albus exposed to NPs reflects an increase in the amount of fatty acids synthesized by the organism (Lv et al., 2023), this may be an important cause of liver lipid accumulation. TG can be hydrolyzed into glycerol and fatty acids, which can be oxidized to generate energy (Chen et al., 2020). In addition, fatty acids derived from TG hydrolysis play an important role as a source of phospholipids, especially in repairing oxidative damage to lipid membranes (Chan and Wang, 2018). In our study, the TG content in the liver of M. albus decreased significantly, possibly due to the pressure of the external NP environment promoting lipid accumulation, leading to an increase in the energy reserve of M. albus.
Currently, a research has shown that NPs can reduce the immune function of fish (Limbu et al., 2019). Some immune-related metabolites, 9-octadecenoic acid, 9,12-octadecadienoic acid, and arachidonic acid, were affected by NPs in our study. Linoleic acid and arachidonic acid belong to the linoleic acid family (Bieber and Fiol, 1986). Studies have confirmed that 9-octadecenoic acid, 9,12-octadecadienoic acid, and arachidonic acid can improve the immunity of fish (Calder, 2011). In our results, we found that the liver content of 9-octadecenoic acid and 9,12-octadecadienoic acid in M. albus decreased significantly in the A group than that in the CK(E) group (Table 2). Therefore, we speculate that this will lead to further interference of the immune system by NPs. After exposure to NPs, the level of 2-mono palmitic acid glyceride in the liver of Oryzias melastigma significantly increased, the synthesis of triglycerides increased, and the degradation of triglycerides decreased. In addition, methyl esters and ethyl esters also accumulate in the liver of Oryzias melastigma, which confirms the lipid disorder induced by NPs exposure in the liver (Ye et al., 2021). Our results showed that the liver content of methyl tetradecanoate, pentadecanoic acid, eicosanoic acid, heptadecanoic acid, 5,8,11,14-eicosatetraenoic acid, and doconexent significantly increased when M. albus were received 1% concentrations of polystyrene NPs in the feed, which caused the accumulation of fatty acids in the liver.
The liver plays a central role in the metabolism of amino acids in humans and other animals, This organ synthesizes many amino acids (including glutamic acid, glutamine, alanine, aspartic acid, asparagine, glycine, serine, and homoarginine), glucose, and glutathione (a major antioxidant) (Hou et al., 2020). In addition to the hydrolysis of arginine and glutamine, the oxidation of proline, and the gluconeogenesis of amino acids, the liver of fish also has a high rate of glutamate and glutamine oxidation to produce ATP, which controls the metabolic stability of glutamine (Vadlakonda et al., 2020). Under the action of NPs, on the one hand, glutamine catabolism is transformed into glutamic acid through glutaminase (GLS) in mitochondria, and the metabolism of GLS eventually leads to the increase of reduction potential, thus increasing levels of NADPH (Sun et al., 2023). However, on the other hand, some glutamylated amino acids are formed and converted into glutathione to exert antioxidant effects (Cruzat et al., 2018). Functional amino acids (such as methionine, cysteine, and glycine) can reduce or prevent oxidative stress and injury in fish liver. Methionine is an aliphatic sulfur-containing essential amino acid and is the precursor of succinyl-CoA, homocysteine, cysteine, creatine, and carnitine (Martínez et al., 2017). Recent studies have shown that methionine can regulate the metabolic process, innate immune system, and digestive function of mammals. It also interferes with lipid metabolism, activation of endogenous antioxidant enzymes, and biosynthesis of glutathione to counteract oxidative stress. In addition, methionine restriction can prevent changes in methionine/transmethylation metabolism, thereby reducing DNA damage and carcinogenic processes, and potentially preventing arterial, neuropsychiatric, and neurodegenerative diseases (Hou et al., 2020). Our results have similar findings. After exposure to NPs, the levels of functional amino acids such as glutamine, glycine, and methionine in the liver of M. albus significantly increase, which plays an active role in preventing oxidative damage to the liver caused by exposure to NPs. These findings provide basic data for assessing the ecological risks of NPs pollution to wild or artificially bred M. albus in the real environment.
5 Conclusion
Our results show that the NPs may interfere with the metabolism of amino acids and lipids in the liver of M. albus and provide a possible mechanism (Figure 4). After the intake of NPs, the activities of enzymes related to sugar metabolism (PK, HK, and PFK) are inhibited, this can explain that the intake of NPs lead to a decrease in M. albus energy reserves, possibly failed to maintain liver energy metabolism and provide ATP for other stress responses. The dihydroxyacetone phosphate produced by glycolysis is reduced to form glycerol, and the oxidative decarboxylation of pyruvic acid forms acetylCoA, which is the raw material for fatty acid synthesis, The increased activity of ACC and FAS on exposure to NPs simultaneously reflects an increase in the amount of fatty acids synthesized by the organism, which may play a positive role in the accumulation of fatty acids in the liver of M. albus. In addition, we also found that the down-regulation of some immune-related metabolites, such as 9-octadecenoic acid and 9,12-octadecadienoic acid, and the up-regulation of functional amino acids, such as methionine, cysteine, and glycine, may be the result of a defensive strategy by M. albus to combat oxidative damage to the liver and pancreas caused by NPs. The results of this research provide valuable ecotoxicological data for better evaluating the impact of NPs on artificially cultured M. albus.
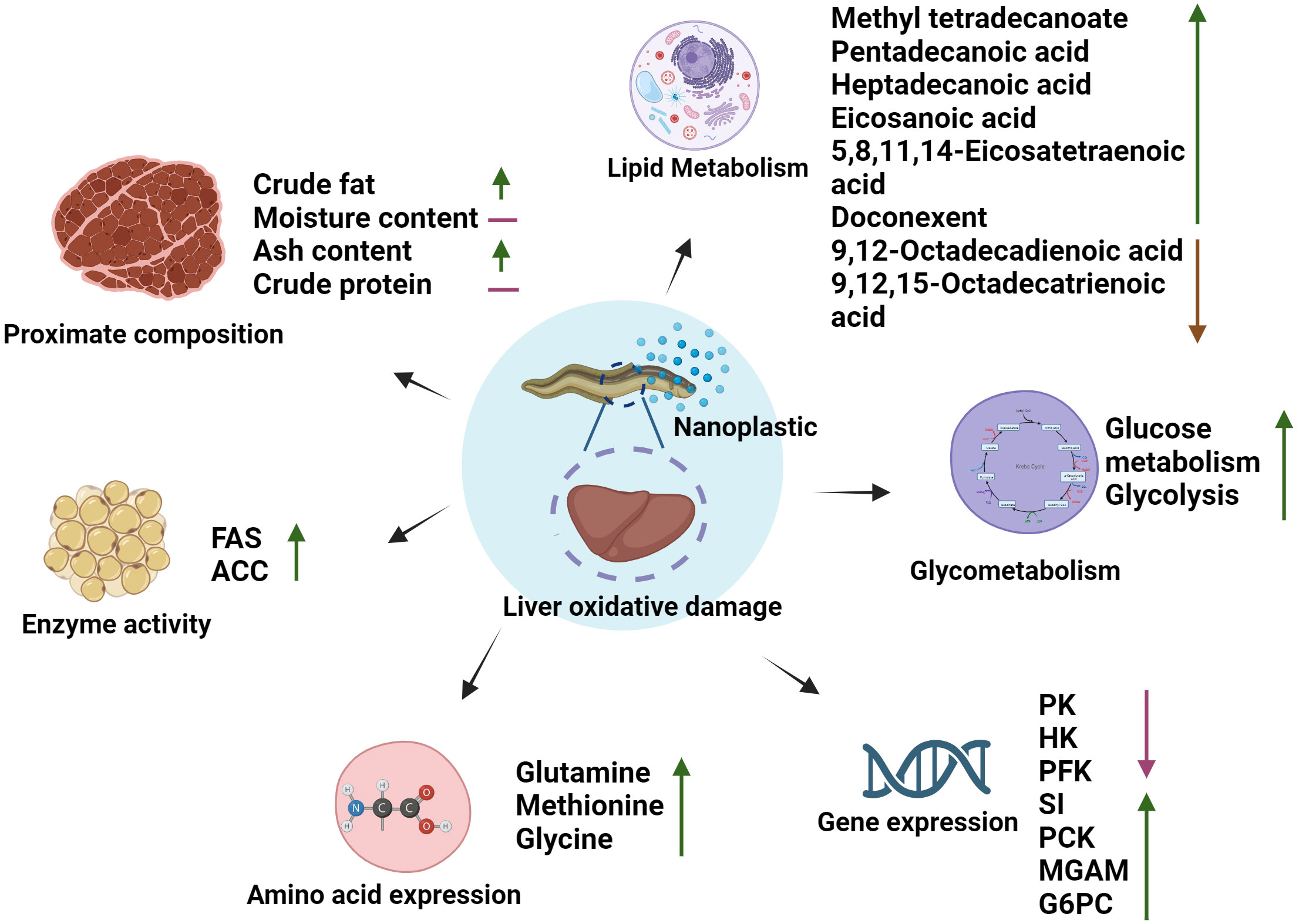
Figure 4 The potential mechanism of NPs interference with energy metabolism, amino acid, and lipid metabolism in the liver of M. albus.
Data availability statement
The raw data supporting the conclusion of this article will be made available by the authors, without undue reservation.
Ethics statement
The animal study was approved by Freshwater Fisheries Research Institute of Jiangsu Province. The study was conducted in accordance with the local legislation and institutional requirements.
Author contributions
HL: Data curation, Formal Analysis, Investigation, Methodology, Software, Writing – original draft. WZ: Methodology, Writing – original draft. ZZ: Data curation, Software, Writing – original draft. CY: Investigation, Writing – original draft. GT: Software, Writing – original draft. WL: Formal Analysis, Funding acquisition, Project administration, Resources, Supervision, Writing – review & editing. QJ: Formal Analysis, Funding acquisition, Project administration, Resources, Supervision, Visualization, Writing – review & editing.
Funding
The author(s) declare financial support was received for the research, authorship, and/or publication of this article. This work was funded by projects grants by the National Natural Science Foundation of China (42277273); Shanghai Municipal Science and Technology Commission (23N61900500); and the China Agriculture Research System of MOF and MARA (grant number CARS-46); and the Demonstration of RiceLoach-Eel Green Co-cultivation in Northern Jiangsu province [CX (20) 2031].
Conflict of interest
The authors declare that the research was conducted in the absence of any commercial or financial relationships that could be construed as a potential conflict of interest.
Publisher’s note
All claims expressed in this article are solely those of the authors and do not necessarily represent those of their affiliated organizations, or those of the publisher, the editors and the reviewers. Any product that may be evaluated in this article, or claim that may be made by its manufacturer, is not guaranteed or endorsed by the publisher.
References
Althaher A. R. (2022). An overview of hormone-sensitive lipase (HSL). Sci. World J. 2022, 1964684. doi: 10.1155/2022/1964684
Barboza L. G. A., Vieira L. R., Branco V., Figueiredo N., Carvalho F., Carvalho C., et al. (2018). Microplastics cause neurotoxicity, oxidative damage and energy-related changes and interact with the bioaccumulation of mercury in the European seabass, Dicentrarchus labrax (Linnaeus 1758). Aquat. Toxicol. 195, 49–57. doi: 10.1016/j.aquatox.2017.12.008
Bieber L., Fiol C. (1986). “[29] Purification and assay of carnitine acyltransferases.” in Methods enzymology. (Elsevier). 123, 276–284. doi: 10.1016/S0076-6879(86)23031-1
Brandts I., Cánovas M., Tvarijonaviciute A., Llorca M., Vega A., Farré M., et al. (2022). Nanoplastics are bioaccumulated in fish liver and muscle and cause DNA damage after a chronic exposure. Environ. Res. 212, 113433. doi: 10.1016/j.envres.2022.113433
Brandts I., Teles M., Tvarijonaviciute A., Pereira M., Martins M., Tort L., et al. (2018). Effects of polymethylmethacrylate nanoplastics on Dicentrarchus labrax. Genomics 110 (6), 435–441. doi: 10.1016/j.ygeno.2018.10.006
Bucolo G., David H. (1973). Quantitative determination of serum triglycerides by the use of enzymes. Clin. Chem. 19 (5), 476–482. doi: 10.1093/clinchem/19.5.476
Calder P. C. (2011). Fatty acids and inflammation: the cutting edge between food and pharma. Eur. J. Pharmacol. 668, S50–S58. doi: 10.1016/j.ejphar.2011.05.085
Chan C. Y., Wang W.-X. (2018). A lipidomic approach to understand copper resilience in oyster Crassostrea hongkongensis. Aquat. Toxicol. 204, 160–170. doi: 10.1016/j.aquatox.2018.09.011
Chen Q., Lackmann C., Wang W., Seiler T.-B., Hollert H., Shi H. (2020). Microplastics lead to hyperactive swimming behaviour in adult zebrafish. Aquat. Toxicol. 224, 105521. doi: 10.1016/j.aquatox.2020.105521
Cruzat V., Macedo Rogero M., Noel Keane K., Curi R., Newsholme P. (2018). Glutamine: metabolism and immune function, supplementation and clinical translation. Nutrients 10 (11), 1564. doi: 10.3390/nu10111564
Diaz-Sotomayor M., Quezada-Calvillo R., Avery S. E., Chacko S. K., Yan L.-Y., Lin A. H.-M., et al. (2013). Maltase-glucoamylase modulates gluconeogenesis and sucrase-isomaltase dominates starch digestion glucogenesis. J. Pediatr. Gastroenterol. Nutr. 57 (6), 704–712. doi: 10.1097/MPG.0b013e3182a27438
Filimonova V., Goncalves F., Marques J. C., De Troch M., Goncalves A. M. (2016). Biochemical and toxicological effects of organic (herbicide Primextra® Gold TZ) and inorganic (copper) compounds on zooplankton and phytoplankton species. Aquat. Toxicol. 177, 33–43. doi: 10.1016/j.aquatox.2016.05.008
Glover C. N. (2019). Cellular and molecular approaches to the investigation of piscine osmoregulation: current and future perspectives. Fish Osmoregulation 177–234. doi: 10.1201/9780429063909-7
Gong H., Li R., Li F., Guo X., Xu L., Gan L., et al. (2023). Toxicity of nanoplastics to aquatic organisms: Genotoxicity, cytotoxicity, individual level and beyond individual level. J. Hazardous Materials 443, 130266. doi: 10.1016/j.jhazmat.2022.130266
Hou Y., Hu S., Li X., He W., Wu G. (2020). Amino acid metabolism in the liver: nutritional and physiological significance. Amino Acids Nutr. Health: Amino Acids Syst. Funct. Health 21–37. doi: 10.1007/978-3-030-45328-2_2
Israelsen W. J., Vander Heiden M. G. (2015). “Pyruvate kinase: Function, regulation and role in cancer.” in: Semin. Cell Dev. Biol. (Elsevier), 43–51. doi: 10.1016/j.semcdb.2015.08.004
Issac M. N., Kandasubramanian B. (2021). Effect of microplastics in water and aquatic systems. Environ. Sci. pollut. Res. 28, 19544–19562. doi: 10.1007/s11356-021-13184-2
Lamichhane G., Acharya A., Marahatha R., Modi B., Paudel R., Adhikari A., et al. (2023). Microplastics in environment: global concern, challenges, and controlling measures. Int. J. Environ. Sci. Technol. 20 (4), 4673–4694. doi: 10.1007/s13762-022-04261-1
Lee B.-H., Rose D. R., Lin A. H.-M., Quezada-Calvillo R., Nichols B. L., Hamaker B. R. (2016). Contribution of the individual small intestinal α-glucosidases to digestion of unusual α-linked glycemic disaccharides. J. Agric. Food Chem. 64 (33), 6487–6494. doi: 10.1021/acs.jafc.6b01816
Li L., Wang M., Ma Q., Ye J., Sun G. (2022a). Role of glycolysis in the development of atherosclerosis. Am. J. Physiology-Cell Physiol. 323 (2), C617–C629. doi: 10.1152/ajpcell.00218.2022
Li Y., Liu Z., Jiang Q., Ye Y., Zhao Y. (2022b). Effects of nanoplastic on cell apoptosis and ion regulation in the gills of Macrobrachium nipponense. Environ. pollut. 300, 118989. doi: 10.1016/j.envpol.2022.118989
Li Y., Liu Z., Yang Y., Jiang Q., Wu D., Huang Y., et al. (2021). Effects of nanoplastics on energy metabolism in the oriental river prawn (Macrobrachium nipponense). Environ. pollut. 268, 115890. doi: 10.1016/j.envpol.2020.115890
Limbu S. M., Ma Q., Zhang M.-L., Du Z.-Y. (2019). High fat diet worsens the adverse effects of antibiotic on intestinal health in juvenile Nile tilapia (Oreochromis niloticus). Sci. Total Environ. 680, 169–180. doi: 10.1016/j.scitotenv.2019.05.067
Lv W., Gu H., He D., Liu Z., Yao C., Huang W., et al. (2023). Polystyrene nanospheres-induced hepatotoxicity in swamp eel (Monopterus albus): From biochemical, pathological and transcriptomic perspectives. Sci. Total Environ. 893, 164844. doi: 10.1016/j.scitotenv.2023.164844
Martínez Y., Li X., Liu G., Bin P., Yan W., Más D., et al. (2017). The role of methionine on metabolism, oxidative stress, and diseases. Amino Acids 49, 2091–2098. doi: 10.1007/s00726-017-2494-2
Nuryadin K., Rahim A. R., Aminin A. (2020). ANALISIS PENGGUNAAN LIMBAH ORGANIK YANG BERBEDA TERHADAP PERTUMBUHAN DAN KELANGSUNGAN HIDUP BELUT SAWAH (Monopterus albus). Jurnal Perikanan Pantura (JPP) 3 (1), 9–15. doi: 10.30587/jpp.v3i1.1396
Nuttall F. Q., Ngo A., Gannon M. C. (2008). Regulation of hepatic glucose production and the role of gluconeogenesis in humans: is the rate of gluconeogenesis constant? Diabetes/metabolism Res. Rev. 24 (6), 438–458. doi: 10.1002/dmrr.863
Rochman C. M. (2018). Microplastics research—from sink to source. Science 360 (6384), 28–29. doi: 10.1126/science.aar7734
Shafland P. L., Gestring K. B., Stanford M. S. (2009). An assessment of the Asian swamp eel (Monopterus albus) in Florida. Rev. Fisheries Sci. 18 (1), 25–39. doi: 10.1080/10641260903225542
Smith M., Love D. C., Rochman C. M., Neff R. A. (2018). Microplastics in seafood and the implications for human health. Curr. Environ. Health Rep. 5, 375–386. doi: 10.1007/s40572-018-0206-z
Sun Z., Wen Y., Zhang F., Fu Z., Yuan Y., Kuang H., et al. (2023). Exposure to nanoplastics induces mitochondrial impairment and cytomembrane destruction in Leydig cells. Ecotoxicology Environ. Saf. 255, 114796. doi: 10.1016/j.ecoenv.2023.114796
Tang Y., Zhang Y., Wang C., Sun Z., Li L., Cheng S., et al. (2018). Overexpression of PCK1 gene antagonizes hepatocellular carcinoma through the activation of gluconeogenesis and suppression of glycolysis pathways. Cell. Physiol. Biochem. 47 (1), 344–355. doi: 10.1159/000489811
Taşbozan O., Gökçe M. A., Erbaş C. (2016). The effect of different growing conditions to proximate composition and fatty acid profiles of rainbow trouts (Oncorhynchus mykiss). J. Appl. Anim. Res. 44 (1), 442–445. doi: 10.1080/09712119.2015.1091323
Teng J., Zhao J., Zhu X., Shan E., Zhang C., Zhang W., et al. (2021). Toxic effects of exposure to microplastics with environmentally relevant shapes and concentrations: Accumulation, energy metabolism and tissue damage in oyster Crassostrea gigas. Environ. Pollut. 269, 116169. doi: 10.1016/j.envpol.2020.116169
Teng M., Zhao X., Wang C., Wang C., White J. C., Zhao W., et al. (2022). Polystyrene nanoplastics toxicity to zebrafish: dysregulation of the brain–intestine–microbiota axis. ACS nano 16 (5), 8190–8204. doi: 10.1021/acsnano.2c01872
TeSlaa T., Bartman C. R., Jankowski C. S., Zhang Z., Xu X., Xing X., et al. (2021). The source of glycolytic intermediates in mammalian tissues. Cell Metab. 33 (2), 367–378.e365. doi: 10.1016/j.cmet.2020.12.020
Tian W. X., Hsu R., Wang Y. S. (1985). Studies on the reactivity of the essential sulfhydryl groups as a conformational probe for the fatty acid synthetase of chicken liver. Inactivation by 5, 5'-dithiobis-(2-nitrobenzoic acid) and intersubunit cross-linking of the inactivated enzyme. J. Biol. Chem. 260 (20), 11375–11387. doi: 10.1016/S0021-9258(17)39189-5
Trevisan R., Ranasinghe P., Jayasundara N., Di Giulio R. T. (2022). Nanoplastics in aquatic environments: impacts on aquatic species and interactions with environmental factors and pollutants. Toxics 10 (6), 326. doi: 10.3390/toxics10060326
Vadlakonda L., Indracanti M., Kalangi S. K., Gayatri B. M., Naidu N. G., Reddy A. B. (2020). The role of pi, glutamine and the essential amino acids in modulating the metabolism in diabetes and cancer. J. Diabetes Metab. Disord. 19, 1731–1775. doi: 10.1007/s40200-020-00566-5
Van de Pol I., Flik G., Gorissen M. (2017). Comparative physiology of energy metabolism: fishing for endocrine signals in the early vertebrate pool. Front. Endocrinol. 8, 36. doi: 10.3389/fendo.2017.00036
Van Dyck I. P., Nunoo F. K., Lawson E. T. (2016). An empirical assessment of marine debris, seawater quality and littering in Ghana. J. Geosci. Environ. Prot. 4 (5), 21–36. doi: 10.4236/gep.2016.45003
Von Moos N., Burkhardt-Holm P., Köhler A. (2012). Uptake and effects of microplastics on cells and tissue of the blue mussel Mytilus edulis L. after an experimental exposure. Environ. Sci. Technol. 46 (20), 11327–11335. doi: 10.1021/es302332w
Wan Z., Wang C., Zhou J., Shen M., Wang X., Fu Z., et al. (2019). Effects of polystyrene microplastics on the composition of the microbiome and metabolism in larval zebrafish. Chemosphere 217, 646–658. doi: 10.1016/j.chemosphere.2018.11.070
Ye G., Zhang X., Liu X., Liao X., Zhang H., Yan C., et al. (2021). Polystyrene microplastics induce metabolic disturbances in marine medaka (Oryzias melastigmas) liver. Sci. Total Environ. 782, 146885. doi: 10.1016/j.scitotenv.2021.146885
Yoon D.-S., Byeon E., Kim D.-H., Lee M.-C., Shin K.-H., Hagiwara A., et al. (2022). Effects of temperature and combinational exposures on lipid metabolism in aquatic invertebrates. Comp. Biochem. Physiol. Part C: Toxicol. Pharmacol. 262, 109449. doi: 10.1016/j.cbpc.2022.109449
Yue-qiang G., Li L., Hui-chun W., Zhi-li W. (2010). Effects of hypoxia on respiratory metabolism and antioxidant capability of Macrobrachium nipponense. J. Hebei Univ. (Natural Sci. Edition) 30 (3), 301–306. doi: 10.3969/j.issn.1000-1565.2010.03.017
Keywords: NPS, M. albus, energy metabolism, gene expression, antioxidant
Citation: Liu H, Zhou W, Zhou Z, Yu C, Tye GJ, Lv W and Jiang Q (2023) Effects of polystyrene nanoplastic exposure on energy metabolism, lipid metabolism, and amino acid changes in Monopterus albus. Front. Mar. Sci. 10:1285427. doi: 10.3389/fmars.2023.1285427
Received: 30 August 2023; Accepted: 16 October 2023;
Published: 23 November 2023.
Edited by:
Yi-Feng Li, Shanghai Ocean University, ChinaReviewed by:
Yao Zheng, Chinese Academy of Fishery Sciences (CAFS), ChinaAdnan H. Gora, Central Marine Fisheries Research Institute (ICAR), India
Hong Li, Nanjing Normal University, China
Copyright © 2023 Liu, Zhou, Zhou, Yu, Tye, Lv and Jiang. This is an open-access article distributed under the terms of the Creative Commons Attribution License (CC BY). The use, distribution or reproduction in other forums is permitted, provided the original author(s) and the copyright owner(s) are credited and that the original publication in this journal is cited, in accordance with accepted academic practice. No use, distribution or reproduction is permitted which does not comply with these terms.
*Correspondence: Qichen Jiang, qichenjiang@live.cn; Weiwei Lv, wwlv1986@sina.com
†These authors share first authorship