- 1School of Aquatic and Fishery Sciences, University of Washington, Seattle, WA, United States
- 2U.S. Geological Survey Washington Cooperative Fish and Wildlife Research Unit, School of Aquatic and Fishery Sciences, University of Washington, Seattle, WA, United States
- 3Fish Ecology Division, Northwest Fisheries Science Center, National Marine Fisheries Service (NMFS), National Oceanic and Atmospheric Administration (NOAA), Seattle, WA, United States
Bivalves such as oysters and clams have been farmed in intertidal zones across the Puget Sound region of the Salish Sea for thousands of years. The variety of gear types used on bivalve farms creates complex vertical structure and attachment points for aquatic epiphytes and invertebrates which increases habitat structural complexity, but may alter eelgrass cover in areas where bivalve farms and eelgrass meadows overlap. Eelgrass meadows are highly productive and ecologically foundational nearshore habitats that provide valuable ecosystem services including the provision of nursery, refuge, and foraging habitat. Aquaculture has been a key feature of the environment in the Puget Sound for millennia, however, little is known about how well aquaculture practices are integrated into the system, and what services they provide to mobile species assemblages relative to unfarmed eelgrass meadows. We used stable isotope mixing models to estimate, for several species of nearshore fish and crab in two areas of North Puget Sound, Washington, the percent diet originating from either a natural bottom habitat (eelgrass meadows), farm habitat (oyster farms), or pelagic planktonic sources. Our results indicate that several species of nearshore fish such as surf perch and staghorn sculpin derive a significant proportion of their diets from farm areas, while crabs derive most of their diets from eelgrass habitat, and stickleback derive a significant proportion of their diets from planktonic sources. The results indicate that foraging habitat uses are species specific, and that several species that spatially overlap bivalve farms obtained a large percentage of their diets from adjacent bivalve farm habitat.
Introduction
Eelgrass meadows are highly productive nearshore ecosystems that have declined globally over the past several centuries (Lotze et al., 2006; Waycott et al., 2009). These protected intertidal meadows serve as nursery habitat for many nearshore species of fish and crab, including commercially and culturally important salmon species (Rubin et al., 2018) and Dungeness crab (Holsman et al., 2006). Eelgrass meadows are also highly productive ecosystems, fixing around one kilogram of carbon per square meter per year (McRoy, 1974), and providing about $1.9 trillion dollars globally in ecosystem services via nutrient cycling alone (Waycott et al., 2009). Eelgrass meadows also sequester carbon, though the native eelgrass species found in Washington Zostera marina sequesters lower levels of carbon compared to other seagrass systems elsewhere in the world (Poppe and Rybczyk, 2018; Postlethwaite et al., 2018).
In many areas of Washington State, eelgrass meadows overlap with bivalve farming activities (Washington State Department of Natural Resources, 2022), some of which have existed for thousands of years (Deur et al., 2015; Lepofsky et al., 2021). Indigenous peoples across the Pacific coast of North America historically modified intertidal areas using clam garden systems (Deur et al., 2015; Lepofsky et al., 2021), and continue to farm and harvest wild oysters, clams, and other bivalves in many areas. Most of the current oyster farming in Washington State is done either by growing oysters loosely on the bottom, or by using mesh bags or lines suspended above the benthic substrate to secure the oysters above the substrate.
There has been increasing interest in understanding how the potential value of artificial habitats to ecosystem functioning within nearshore coastal systems compares to unfarmed natural habitats. Bivalve-growing gear such as flipbags, clam nets, and loose oyster bottom culture modify tide flats by introducing complex vertical structure, while potentially reducing eelgrass cover through increased localized disturbances related to gear movement, crop out-planting, and harvesting etc. (Dumbauld et al., 2009; Ferriss et al., 2019). Interactions between eelgrass and bivalve aquaculture are complex (Ferriss et al., 2019) and continue to be a consideration for expansion of bivalve aquaculture. The complex vertical structure added to the tide flats creates attachment points for filamentous algae, aquatic macrophytes, and ideal habitat for epibenthic meiofauna such as amphipods (Hosack et al., 2006). Epibenthic meiofauna are important prey items for small nearshore fish (Alheit and Scheibel, 1982; Gee, 1989; Caine, 1991), and occur in higher densities around oyster flipbags and eelgrass meadows than in open, unstructured mudflats (Hosack et al., 2006). The vertical structure can be used both as predator refuge and foraging habitat for intertidal fishes and crabs.
Epiphyte density may also play a role in habitat function and usage, as surf perch (Embiotocidae) have a higher probability of foraging in areas containing high epiphyte cover compared to areas with no epiphyte cover (Veggerby et al., in press). Thus, bivalve aquaculture gear that has accumulated epiphyte cover may provide more habitat function than clean gear that has spent less time on the tide flat, or had biofouling removed. For example, Ferraro and Cole (2007) found that the diversity of benthic macrofauna was equal between oyster farm habitat and eelgrass meadows after the oysters were left to grow for 2 - 3 years without disturbance. Nevertheless, the degree to which aquaculture habitats are used for foraging, and how those habitats compare to unfarmed mudflats or eelgrass meadows, is not well understood (but see Dumbauld et al., 2015; Muething et al., 2020; Ferriss et al., 2021). Understanding the ecological role of habitat that has been modified and created by bivalve aquaculture in the intertidal food web is important for effective management of nearshore systems and the many ecological functions and services they provide.
Stable isotopes are a useful tool for studying food webs and habitat usage (Peterson and Fry, 1987; Fry, 2006). Stable isotope ratios such as carbon12/carbon13 and nitrogen14/nitrogen15 can be used to estimate diet sources when both the predator (i.e., consumer) and the potential prey (i.e., source) isotopic values are known (Fry, 2006). Nitrogen isotope ratios are commonly used to estimate trophic position, and carbon isotope ratios are commonly used to estimate nutrient flow through an ecosystem. When used together, carbon and nitrogen isotopes can reveal the contribution of different prey or habitat types towards the overall diet of a species of interest. By modeling how isotopically similar a group of consumers are to a suite of possible diet sources, the contributions of each candidate diet source towards a consumer’s overall diet can be estimated. As computational power has increased, more complex and computationally intensive Bayesian methods for modeling predators and prey using stable isotopes have become more accessible to researchers (Stock et al., 2018).
In this study we estimated the proportion of species’ diets derived from eelgrass meadows, pelagic planktonic sources, and oyster farm habitat types using a Bayesian stable isotope mixing model. We did not attempt to quantify feeding behavior as in Veggerby et al. (in press), but instead focused on habitat sources of carbon and nitrogen. Specifically, we sought to answer the following questions:
1. How much of each species diet came from eelgrass meadows versus pelagic planktonic sources versus oyster farm habitat?
2. Were diet sources consistent across farmed sites?
3. Were diet sources similar between bivalve aquaculture and unfarmed eelgrass habitat?
Methods
Study area
The Puget Sound is a large estuary located in Washington State, USA (Ruckelshaus and McClure, 2007). It forms the southern extent of the Salish Sea, which is a marginal sea located along the northwest coast of North America spanning the United States and Canada. Various types of bivalve aquaculture are present along the outer coast of Washington as well as within Puget Sound. Oysters are often grown either directly on the benthic substrate, or in buoyant mesh bags suspended just above the benthic substrate (Figure 1).
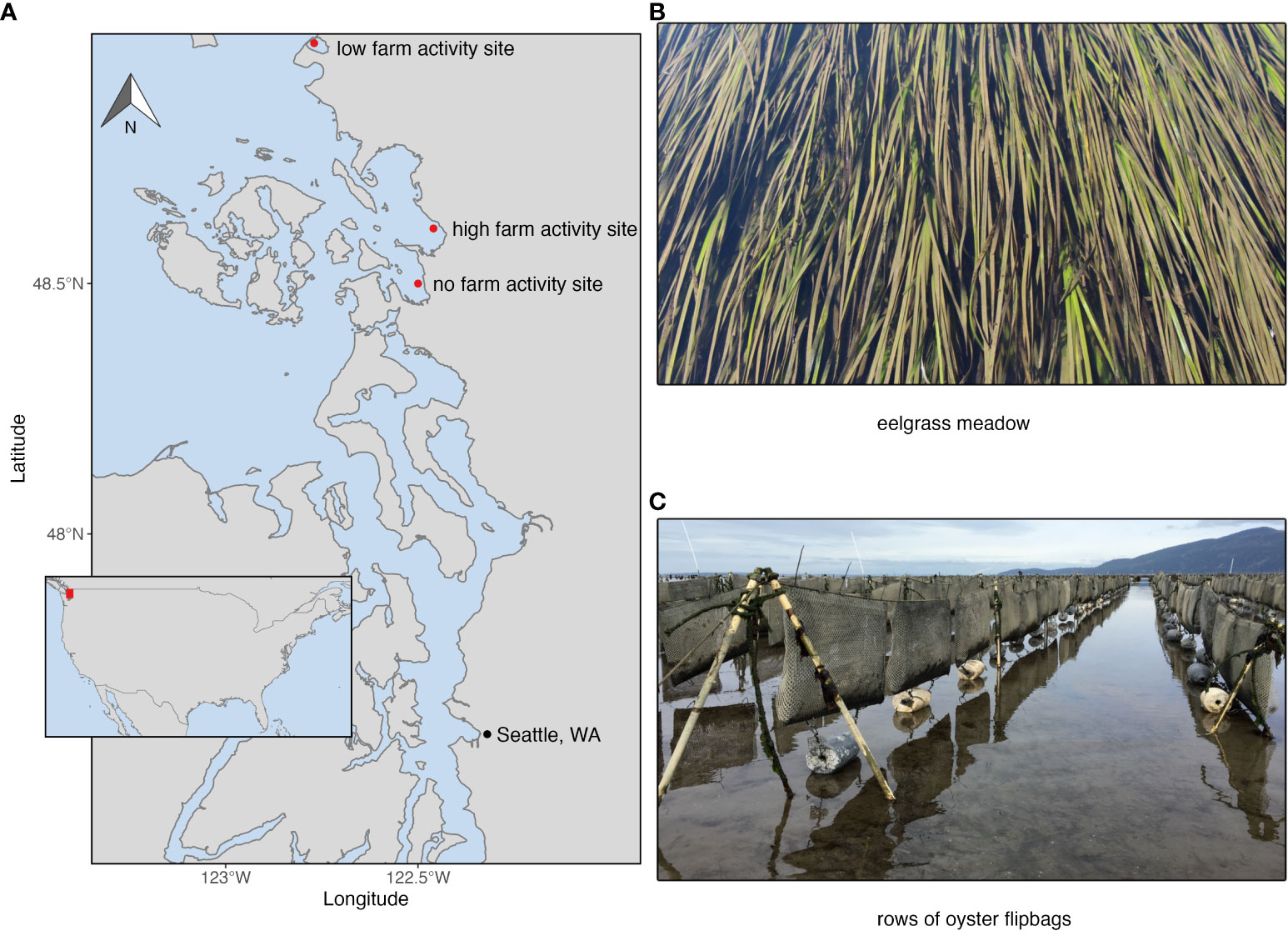
Figure 1 (A) Map of the North/central Puget Sound with locations of the two farm sites (low farming activity, Drayton Harbor; and high farming activity, Samish Bay) and one reference site (no farming activity, Padilla Bay). The two different benthic habitat types compared in the study are shown on the right: Eelgrass meadows (B) and oyster flipbags (C).
Within the North Puget Sound region there are extensive tracts of eelgrass meadows, bivalve farms, and mudflats, within different bays and inlets. Two examples of Puget Sound areas with bivalve farming are Samish Bay and Drayton Harbor, which are used for growing oysters and other bivalves. In Samish Bay, a large amount of tide flat area is dedicated to bivalve farming, where multiple shellfish companies have grown oysters, clams, and other bivalves using loose bottom culture, mesh bags, nets, and long lines across approximately 5,200 acres of tide flat since the early 1900’s (Steele, 1964; Washington State Department of Health, 2022). Drayton Harbor has comparatively much less aquaculture, with one oyster farm using less than two acres of tide flat for active farm operations. Both areas encompass extensive eelgrass meadows adjacent to, and often immediately surrounding farmed areas, with eelgrass, macroalgae, or bare mudflat substrates within the active farm area. There are approximately 650 acres of eelgrass in Drayton Harbor, and 5,100 acres that contain eelgrass in Samish Bay (Washington State Department of Natural Resources, 2022). However, eelgrass cover and density are not uniform across either site, with most of the farm area in Samish Bay overlapping the area that contains eelgrass (Washington State Department of Health, 2022; Washington State Department of Natural Resources, 2022). Other areas within North Puget Sound such as Padilla Bay National Estuarine Reserve serve as protected estuarine reserves with no bivalve farming activity. Padilla Bay has the second largest eelgrass meadow on North America’s Pacific coast with over 8,000 acres of eelgrass (Shull and Bulthuis, 2002). We chose our study sites to fall along a gradient of farming activity representative of the different site conditions in the North Puget Sound: Padilla Bay represented a site with no farming activity, Drayton Harbor represented a site with low farming activity surrounded by dense eelgrass meadows, and Samish Bay represented a site with high farming activity within a patchwork of thousands of acres of farms, eelgrass meadows, and mudflats. Specific percent cover of farm versus unfarmed area was not available for each site, so the sites were categorized as ‘no farming activity’, ‘low farming activity’, and ‘high farming activity’.
Sample collection
We conducted sampling in Padilla Bay, Samish Bay, and Drayton Harbor during July and August of 2020, 2021, and 2022, with most of the sampling conducted in the summer of 2022. Padilla Bay was used as a reference site in contrast to bays containing low levels of aquaculture (Drayton Harbor) and bays with higher levels of aquaculture (Samish Bay). Both farm sites we collected samples at grew Pacific Oysters in flipbags. We collected mobile species of nearshore fish and crabs that are common in both farmed and unfarmed intertidal areas: shiner perch (Cymatogaster aggregata), threespine stickleback (Gasterosteus aculeatus), Pacific staghorn sculpin (Leptocottus armatus), Dungeness crab (Metacarcinus magister), shore crab (Hemigrapsus nudus and H. oregonensis), and small juvenile flatfish comprising either English sole or starry flounder (Parophrys vetulus and Platichthys stellatus respectively). We chose these species because of their consistent abundance across these study sites, and because these species were the dominant species observed in underwater video collected in the same farm and eelgrass habitats in the same sites during a previous study (Ferriss et al., 2021).
We targeted nearshore species within and adjacent to oyster flipbag farmed areas with a 25-meter beach seine with 1.5 mm mesh, along with opportunistic hand collection of crabs when possible. We deployed crab pots concurrently with beach seining to try to trap additional crabs while we were at each site, and we timed seining at the same point in the tidal cycle. Seining and crab pot deployment occurred in both the centers and edges of farm sites to collect a representative sample of the fish species across a patch of farm. At Padilla Bay we sampled an eelgrass meadow that had similar tidal elevation to the farmed areas in the other bays. We counted fish and crabs caught in the seines, immediately released non-target species, and euthanized target species with tricaine mesylate (MS-222) and immediately placed them on ice. We transferred samples to a laboratory freezer for storage within a few hours of collection.
We used two species of snails as proxies for benthic isotopic baselines in lieu of direct benthic primary productivity measurements (Post, 2002). By using herbivorous snails instead of direct primary productivity measurements, we were able to characterize the base of the benthic food web in a temporally integrated manner at the small spatial scale of each habitat (Post, 2002). We accounted for individual differences by combining multiple individuals from each site into a single sample. Japanese mud snails (Batillaria attramentaria) and Japanese bubble snails (Haloa japonica) were ideal species to use as isotopic baselines because they were abundant and densely spread across all three of our sites. We collected 15 - 20 individuals of each snail species from the center of flipbag farmed areas near where we seined, as well as from adjacent eelgrass meadows approximately 30 meters away from the edge of the farmed site. We chose 30 meters distance to reduce potential environmental influences from the farmed areas, while maintaining similarity in environmental conditions between the farmed and unfarmed reference sites (Ferriss et al., 2021).
Pelagic sources of primary productivity were measured using Pacific oyster (Magallana gigas) gutballs. Oysters are filter feeders, so by dissecting their gutballs, we could quantify the recent planktonic isotopic signature in an area. Oysters were collected from the center of flipbag farmed areas where we seined, as well as from adjacent eelgrass meadows approximately 30 meters away from the edge of the farmed site. Oysters and snails were immediately placed on ice after collection. We transferred samples to a laboratory freezer for storage within a few hours of collection. We collected a total of 145 shiner perch, 116 stickleback, 154 staghorn sculpin, 92 juvenile flatfish, 65 Dungeness crab, and 25 shore crab across the three sites. To quantify primary productivity, we collected a total of 67 Japanese mud snails, 73 bubble snails, and 35 oyster gutballs across the three sites.
Sample processing
We extracted tissue samples from each individual for isotope analysis in the laboratory. Samples were allowed to partially thaw to make dissections easier. We cut dorsal muscle plugs from each fish, and we cut claw muscle plugs from each crab. We dissected oyster gut balls from each oyster and removed the whole body of snails from their shells. All samples were placed in 20 mL glass scintillation vials, labeled, and freeze dried for 24 hours to remove all moisture. Once samples were freeze dried, we pulverized them, weighed them into 5 mm by 9 mm tin capsules, and analyzed them on a Thermo-Fisher Delta V mass spectrometer at the Holtgrieve Ecosystem Ecology Lab at the University of Washington.
Stable isotope data went through the laboratory’s QAQC process: sample blanks were run periodically to check for incomplete combustion, and L-Glutamic acid and salmon standards run with the sample sets were used to estimate the precision and accuracy of the isotope ratio measurements. Commonly used standards like L-Glutamic acid are used to estimate the precision, accuracy, and drift of a mass spectrometer by comparing the known isotopic value of a standard to its measured isotopic value through repeated measurements of the standard throughout the course of a sample set. Based on L-Glutamic acid standards measured across runs, average nitrogen precision and accuracy was 0.12 ‰ and 0.05 ‰, respectively, across all sample sets run. Average carbon precision and accuracy was 0.06 ‰ and 0.04 ‰, respectively, across all sample sets run. We merged stable isotope data with all the collected metadata and combined it into a single large dataset for analysis (Veggerby et al., 2023).
Statistical analysis
We used a stable isotope mixing model to estimate the probability distribution of each habitat as a source for diets of fishes and crabs. This was not an attempt to quantify feeding behavior, or where feeding took place, but rather to quantify where the carbon and nitrogen within each species originated by comparing how isotopically similar each consumer species was to the three candidate source habitats. In other words, using a known starting value (the source) and known ending values (the consumer species) to estimate the mix ratio. Each species of consumer was modeled separately using the MixSIAR package in R (Stock and Semmens, 2018; Stock et al., 2022). We used data from Post (2002) to select the necessary trophic enrichment factor. We used 0.4 +/- 1.3 for δ 13C and 3.4 +/- 1.0 for δ 15N. Using a trophic enrichment factor corrects for isotopic fractionation in consumer tissue that results from preferential uptake of lighter or heavier isotopes when tissue from prey is digested and incorporated into the predator’s tissue. There are very few data on the percentage of diet that originates from eelgrass versus oyster farms for the species included in our study, so we used an uninformative prior in the mixing model, which assigned equal probability to each habitat type at the start of the model run. We used the best practices of stable isotope data analysis and mixing model outlined in Phillips et al. (2014).
We modeled each consumer species independently with a base model containing no added effects, as well as a model with aquaculture site as a fixed effect. Samples from the no farming site were modeled separately with no added effects. We classified diet sources as derived from eelgrass, flipbag farm habitat, or planktonic sources. There were not enough shore crabs collected at the low farming site for site comparisons, so we only fit a base model with no fixed effects for shore crabs. There were not enough staghorn sculpin or flatfish collected at the no farming site, so we did not fit a no farming site model for those two species.
We used several model diagnostics to ensure that the mixing models had converged properly. First, we examined isospace plots to ensure that source and consumer isotopic signatures overlapped in isospace. Consumer isotopic signatures that are outside of the source isospace polygon would yield nonsensical model results (Stock et al., 2018). For every mixing model we used three Markov chain Monte Carlo (MCMC) chains with a total chain length of 1,000,000, a burn-in of 500,000, and a thinning rate of 500, for a final chain length of 1,000 steps. The thinning rates are set by default by MixSIAR and not based on the measures of autocorrelation within any of the specific chains.
We calculated Gelman-Rubin statistics for each MCMC chain. Gelman-Rubin values should be around 1.0, with values above 1.1 indicating that the chains did not converge (Gelman et al., 1995). Gelman Rubin values compare the within-chain variation to the between-chain variation to assess if the chains have all reached the same stationary distribution, i.e., convergence. We also visually examined MCMC trace plots to confirm that the chains had converged. The chains were considered stationary if the distribution of points did not appear to change across the trace plot. Proper convergence was determined based on guidance from Gelman et al. (1995).
We conducted all statistical analyses in R version 4.2.2 (R Core Team, 2022). We also used the ‘here’ version 1.0.1 (Müller and Bryan, 2020), ‘tidyverse’ version 2.0.0 (Wickham, et al. 2019) and ‘RColorBrewer’ version 1.1-3 (Neuwirth, 2022) packages in this project. All data, code, and model diagnostics necessary to reproduce our analyses and results are available on GitHub at: https://github.com/veggerk/veggerby_2023_stable-iso_habitat.
Results
Carbon and nitrogen originating from bivalve farms made up a substantial portion of the diets of several species of nearshore fish collected in the vicinity of bivalve farms. Shiner perch and staghorn sculpin obtained about 80% of their diets from farmed areas, while juvenile English sole and starry flounder derived about 40% of their diets from farmed areas. Stickleback derived most of their diets from pelagic sources, and Dungeness crabs and shore crabs obtained most of their diets from eelgrass meadow habitat (Figure 2; Table 1).
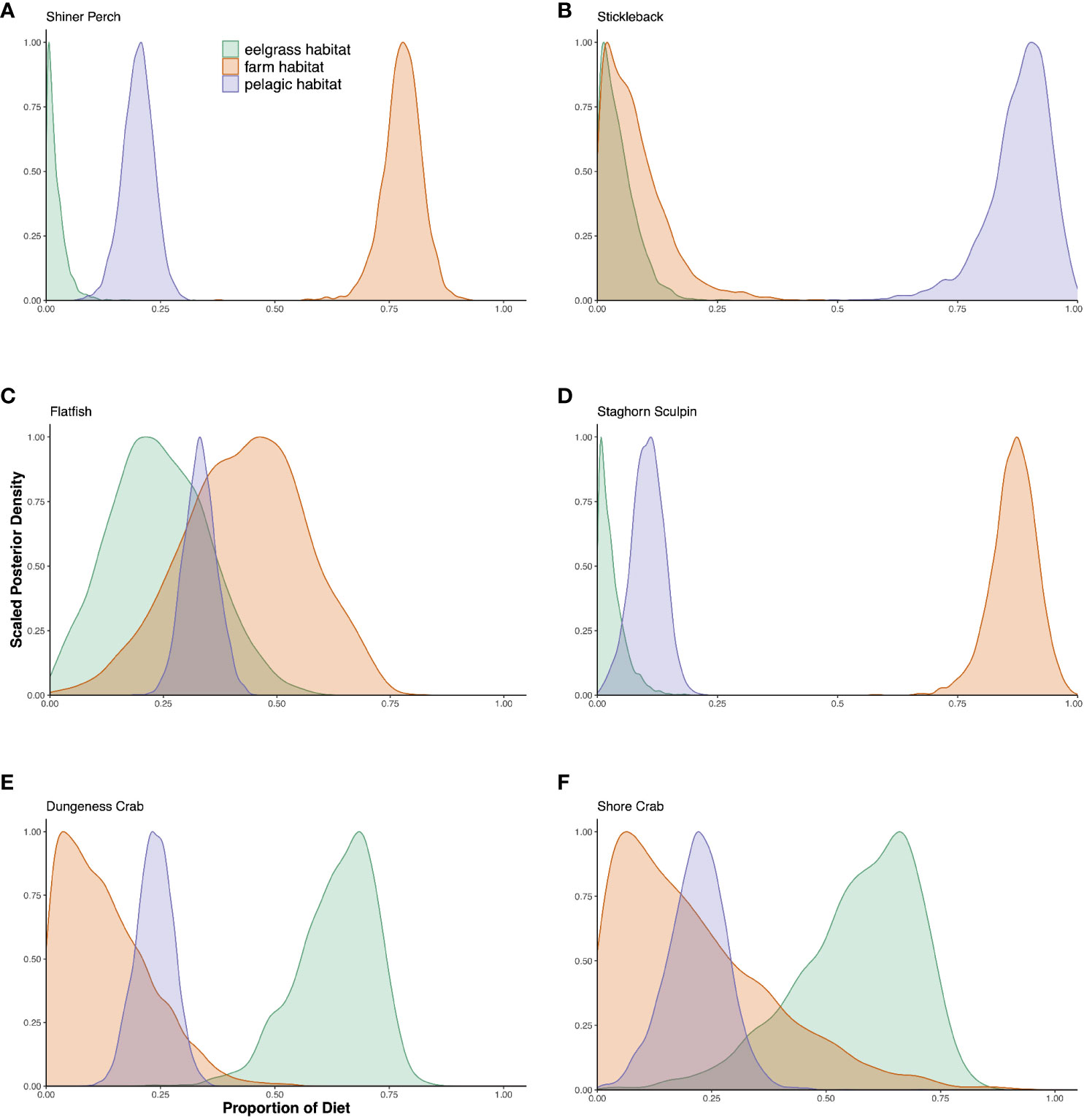
Figure 2 Posterior probability densities of estimated diet sources for each consumer species. (A) Shiner perch, (B) Stickleback, (C) Flatfish, (D) Staghorn sculpin, (E) Dungeness crab, (F) Shore crab. Light green represents the estimated diet percentage coming from eelgrass type habitat, light red represents the estimated diet percentage coming from farm type habitat, and light purple represents the estimated diet percentage coming from pelagic type habitat. The peaks for each diet source represent the highest probability diet percentage coming from that source.
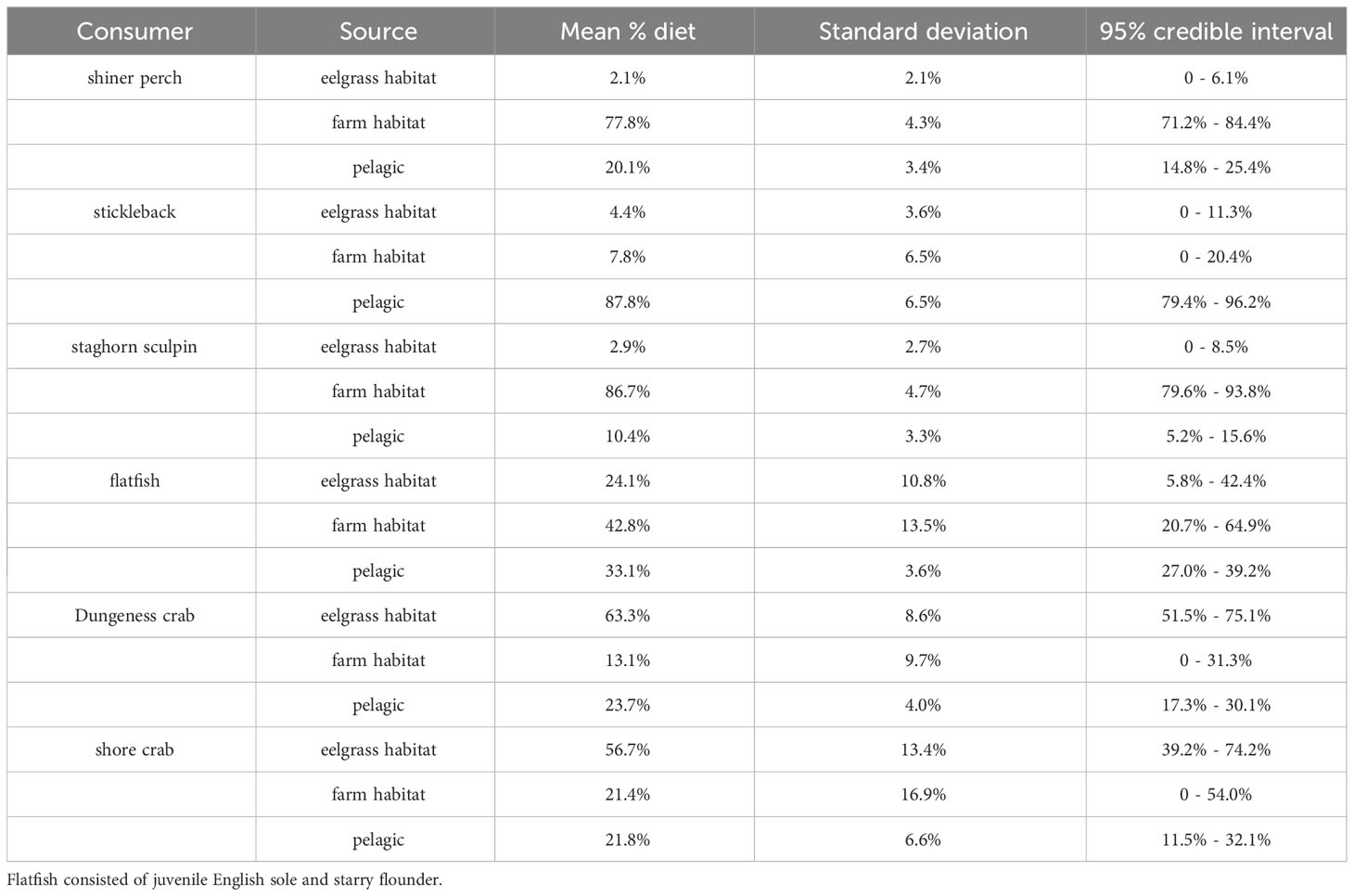
Table 1 Model estimates of percent diet coming from each habitat type from bivalve farm sites (high and low combined).
Estimates of diet source between the two farm sites were consistent for shiner perch, stickleback, and juvenile flatfish. Staghorn sculpin and Dungeness crab diet source estimates were different between the low farming activity and high farming activity site, with variation being driven by the percent diet derived from pelagic versus eelgrass sources. The percent diet derived from flipbag habitat was similar across the two sites. In the high farming activity site we estimated that Dungeness crabs derived about 70% of their diets from eelgrass meadows compared to about 37% in the low farming activity site (Table 2).
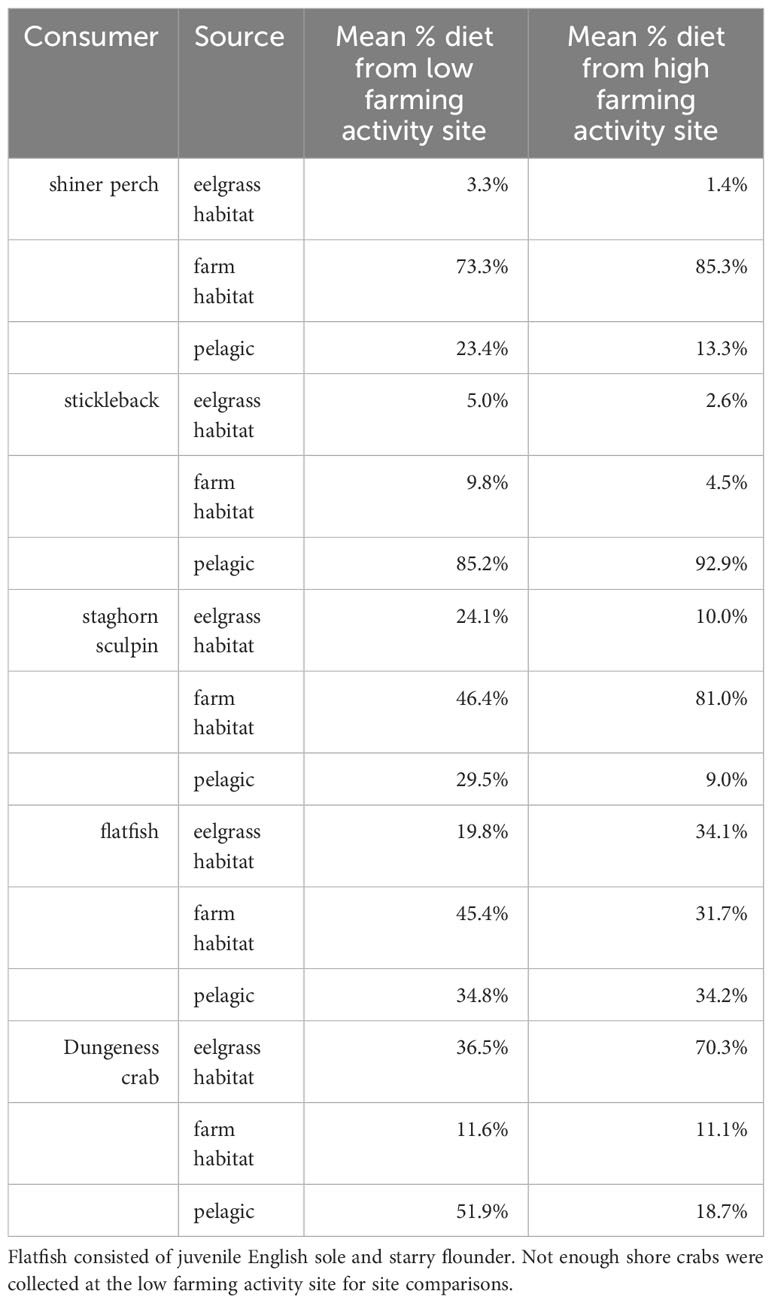
Table 2 Model estimates comparing percent diet of organisms from high farming activity vs. low farming activity sites.
Estimates of diet source derived from pelagic or combined benthic sources between the farm sites and the no-farming activity site were very similar for shiner perch, stickleback, and Dungeness crab, which were the three species where this comparison was possible. In the no-farming activity site, combined benthic sources were simply (exclusively) eelgrass meadows, whereas in the two farm sites, combined benthic sources included both farm and eelgrass habitat. Shiner perch, stickleback, and Dungeness crab fed on benthic versus pelagic derived nutrients in consistent proportions across sites with different characteristics and eelgrass abundances. In farmed sites where eelgrass cover was broken up by farmed plots, Dungeness crabs on average still fed on about 75% benthic-derived nutrients (Table 3).
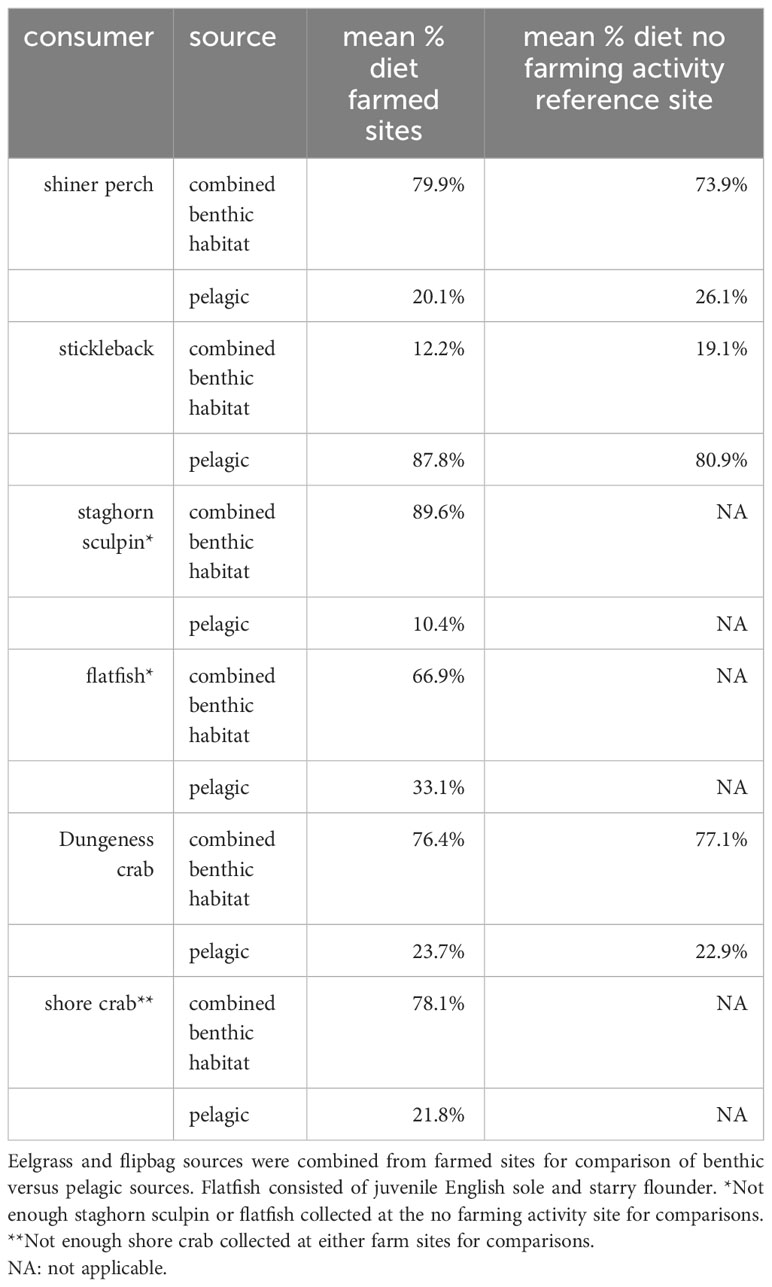
Table 3 Model estimates of the percent diet coming from pelagic and combined benthic sources between organisms from both farm sites and the no farming activity reference site.
Model diagnostics for all models indicated that all models had reached convergence. Gelman-Rubin values for all models except the shiner perch location effect model were below 1.1. The shiner perch location effect model had one out of 133 Gelman-Rubin values above 1.1. The other 132 Gelman-Rubin values were below 1.1, indicating that most of the chain steps after the burn-in period remained in a stationary distribution. Trace plots for the one Gelman-Rubin value above 1.1 showed that the first chain momentarily left the convergence area for a few chain steps before returning for the remainder of the chain. Trace plot inspection for all other models further indicated that MCMC chains had converged properly. The model diagnostics indicated that we could confidently proceed with interpreting the model outputs.
Discussion
Our analysis provides unique insights into the functional role of different nearshore habitat types for key nearshore consumers. Aquaculture habitat appeared to provide unique foraging opportunities for certain mobile species within this system. This, in turn, likely benefits the systems biodiversity in some capacity, despite the potential impact on eelgrass, which was also estimated to provide key foraging opportunities. All three potential habitat diet sources were estimated to be important for at least one species, which highlights the benefits of having a diverse array of habitat types within a nearshore area. Both Dungeness crabs and shore crabs derived most of their diet from eelgrass type habitat, whereas shiner perch and staghorn sculpin derived most of their diets from farm type habitat. Stickleback diets were predominantly derived from pelagic planktonic sources, and small flatfish appeared to have diets reflective of habitat generalists, with both eelgrass and farm type habitat both providing similar proportions of their diets.
For species such as shiner perch, staghorn sculpin, and juvenile flatfish, bivalve type habitat appeared to be an important foraging location. This finding is in line with previous research, indicating that bivalve farm habitat provides foraging opportunities for certain species of nearshore fish, particularly surf perch, juvenile flatfish, and sculpin (Veggerby et al., in press). Although our study did not explicitly examine the specific gut contents of consumers, which would be helpful for further resolving the importance of different habitats, another ongoing study is engaged in this work.
Eelgrass meadows are highly productive nearshore habitats (McRoy, 1974) that can export nutrients to adjacent mudflats, particularly in areas with high tidal and wave energy (Johannessen and Macdonald, 2016). In previous research, large cancer crabs including Dungeness crabs were observed foraging in mudflats at higher rates than bivalve farm habitat type (Veggerby et al., in press). However, bivalve farm habitat that did contain eelgrass supported high crab foraging (Veggerby et al., in press). Eelgrass also serves as nursery habitat for small Dungeness crabs (Williams, 1994; Holsman et al., 2006), as well as foraging and nursery habitat for a wide range of nearshore species (McDevitt-Irwin et al., 2016; Kennedy et al., 2018). Our findings indicate that Dungeness crabs likely receive valuable nutrients from eelgrass habitats, even when they move into open mudflats and deeper water to forage as they mature (Veggerby et al., in press). This nutrient transfer may be from detrital inputs to the benthos that then supports prey for crabs, or other production that end up in nearby habitats. Nutrient cycling is common between local-scale nearshore habitats, with strong linkages between adjacent bays, saltmarshes, and pelagic planktonic sources contributing to a mosaic of interconnected habitats (Conway-Cranos et al., 2015).
Several species derived their energy and nutrients primarily from flipbag habitats, which contain higher vertical structure than mudflats or eelgrass meadows. Previous studies also noted that demersal fishes like staghorn sculpin and small flatfish (Able et al., 2005) have been observed to feed primarily in flipbag and unstructured mudflat habitats, and pelagic species like stickleback (Ferriss et al., 2021) are abundant in both flipbag habitat and eelgrass meadows. It is also quite possible that the vertical structure created by flipbags offers these small fishes refuge from larger predators (Caine, 1991).
Filamentous algae, other aquatic macrophytes, and biofouling anchored to natural or artificial structure such as oyster flipbags or clam-growing nets may harbor high numbers of preferred prey items such as amphipods (Caine, 1991) for shiner perch and other structure-affiliated fish (Dumbauld et al., 2015). Shiner perch have previously been observed foraging in both farmed and unfarmed habitat types, particularly over heavily bio-fouled bivalve growing gear (Veggerby et al., in press). Thus, gear subjected to less disturbance or cleaning may provide increased foraging opportunities compared to gear subjected to more frequent disturbance.
This suggests that the local environmental impact of adding bivalve growing gear is not simply proportional to the size of the farmed area, but is also related to what kind of gear is used, and what farming practices are employed. In our study, Dungeness crab diets from the high farming activity site were more similar to the unfarmed eelgrass reference area than to the low farming activity site. Thus, between-site variability in Dungeness crab diet sources were mediated by factors beyond simply farm intensity. The diets of shiner perch, stickleback, and flatfish were notably similar across the two farm sites, despite orders of magnitude difference in the scale of farming. Staghorn sculpin were the only study species that had a much higher proportion of diet derived from flipbag habitat in the site with higher farming, compared to the site with low farming activity.
Bivalve farms within a larger intertidal habitat mosaic appear to provide ecosystem function in the form of foraging habitat for nearshore fish. In general, eelgrass is often considered key nursery habitat for some earlier life stages of these species (Holsman et al., 2006; Rubin et al., 2018). As subadults or adults, these species of nearshore fish derived a substantial proportion of their diets from bivalve farm habitat types. Other species such as Dungeness crabs and shore crabs appeared to derive most of their diets from unfarmed eelgrass meadows, either through directly foraging within eelgrass, or by consuming nutrients that originated from eelgrass meadows but were exported to adjacent farmed areas or mudflats. Bayesian stable isotope mixing models may be used in other study systems to partition diets by different habitat sources, provided there are large enough isotopic differences between the habitat types being studied.
Oyster flipbags modify habitat through the addition of benthic structure and disturbance via trampling, shading, or other mechanical damage, which can reduce eelgrass density within actively farmed areas (Dumbauld et al., 2009). This leads to the creation of a habitat patchwork of eelgrass meadows and either bare mudflats or sparsely vegetated farm plots with a variety of artificial structure types. These habitats may alter the species composition within a localized area to favor species that prefer the habitat characteristics created by a combination of artificial structure and disturbance from harvesting, crop out-planting, and other farm activities. Thus, localized shifts in the composition of the nearshore community may be expected if a mudflat or eelgrass meadow is converted into bivalve farm habitat.
Having a diverse set of habitat types within a nearshore area provides foraging and refuge habitat to a wide range of species. While farm habitats cause localized disturbance to native nearshore habitat, they also appear to avail a wide range of foraging opportunities. Our results also show that the patchwork of habitat types inside and adjacent to bivalve aquaculture sites will favor some species at the expense of others. For example, bivalve farm habitats within the larger nearshore ecosystem function as foraging habitat by generating organic matter that is used by several species of nearshore fish, while Dungeness and shore crabs primarily rely on eelgrass meadows for their nutrient needs. This may alter the local spatial distribution of nearshore species around a farm site, but did not appear to greatly alter the diet compositions of our study species. Bivalve farm impacts are related not just to farm size, but also disturbance frequency, extent of biofouling, and the gear type used. Future studies could consider the existing spatial distribution of bivalve farms and natural habitats across the entire Puget Sound seascape, and the benefits they provide, compared to those envisioned under an expansion of aquaculture activities when estimating the entirety of ecosystem services provided at spatial scales larger than single farms.
Data availability statement
The datasets presented in this study can be found in online repositories. The names of the repository/repositories and accession number(s) can be found below: https://doi.org/10.5281/zenodo.8118102.
Ethics statement
The animal study was approved by Office of Animal Welfare - University of Washington. The study was conducted in accordance with the local legislation and institutional requirements.
Author contributions
KV: Data curation, Formal Analysis, Investigation, Project administration, Validation, Visualization, Writing – original draft, Conceptualization, Methodology. MS: Conceptualization, Funding acquisition, Investigation, Project administration, Resources, Supervision, Writing – review & editing. BS: Conceptualization, Funding acquisition, Project administration, Resources, Supervision, Writing – review & editing. PK: Conceptualization, Funding acquisition, Investigation, Supervision, Writing – review & editing.
Funding
The author(s) declare financial support was received for the research, authorship, and/or publication of this article. This study was funded by the NMFS Office of Aquaculture and a Borman Research Grant.
Acknowledgments
This research was conducted in collaboration with support of shellfish aquaculture farms and Padilla Bay National Estuarine Reserve in Washington, USA. Thank you to Chelsea Wood and Bridget Ferriss for their insight and advice, Jessica Diallo for the use of her mass spectrometer data processing code, and Gordon Holtgrieve for the use of the Holtgrieve lab mass spectrometer. Thank you to two reviewers for their helpful suggestions to improve the manuscript. Any use of trade, firm, or product names is for descriptive purposes only and does not imply endorsement by the U.S. Government. This study was performed under the auspices of University of Washington protocol #4550-01.
Conflict of interest
The authors declare that the research was conducted in the absence of any commercial or financial relationships that could be construed as a potential conflict of interest.
Publisher’s note
All claims expressed in this article are solely those of the authors and do not necessarily represent those of their affiliated organizations, or those of the publisher, the editors and the reviewers. Any product that may be evaluated in this article, or claim that may be made by its manufacturer, is not guaranteed or endorsed by the publisher.
References
Able K. W., Neuman M. J., Wennhage H. (2005). “Ecology of juvenile and adult stages of flatfishes: Distribution and dynamics of habitat associations,” in Flatfishes: Biology and Exploitation. Ed. Gibson R. N. (Hoboken, New Jersey, USA: Blackwell Science Ltd), 164–184. doi: 10.1002/9780470995259.ch8
Alheit J., Scheibel W. (1982). Benthic harpacticoids as a food source for fish. Mar. Biol. 70, 141–147. doi: 10.1007/BF00397678
Caine E. A. (1991). Caprellid amphipods: Fast food for the reproductively active. J. Exp. Mar. Biol. Ecol. 148, 27–33. doi: 10.1016/0022-0981(91)90144-L
Conway-Cranos L., Kiffney P., Banas N., Plummer M., Naman S., MacCready P., et al. (2015). Stable isotopes and oceanographic modeling reveal spatial and trophic connectivity among terrestrial, estuarine, and marine environments. Mar. Ecol. Prog. Ser. 533, 15–28. doi: 10.3354/meps11318
Deur D., Dick A., Recalma-Clutesi K., Turner N. J. (2015). Kwakwaka’wakw “clam gardens”: Motive and agency in traditional Northwest coast mariculture. Hum. Ecol. 43, 201–212. doi: 10.1007/s10745-015-9743-3
Dumbauld B. R., Hosack G. R., Bosley K. M. (2015). Association of juvenile Salmon and estuarine fish with intertidal seagrass and oyster aquaculture habitats in a Northeast Pacific Estuary. Trans. Am. Fish Soc. 144, 1091–1110. doi: 10.1080/00028487.2015.1054518
Dumbauld B. R., Ruesink J. L., Rumrill S. S. (2009). The ecological role of bivalve shellfish aquaculture in the estuarine environment: A review with application to oyster and clam culture in West Coast (USA) estuaries. Aquaculture 290, 196–223. doi: 10.1016/j.aquaculture.2009.02.033
Ferraro S. P., Cole F. A. (2007). Benthic macrofauna-habitat associations in Willapa Bay, Washington, USA. Estuar. Coast. Shelf Sci. 71, 491–507. doi: 10.1016/j.ecss.2006.09.002
Ferriss B., Veggerby K., Bogeberg M., Conway-Cranos L., Hoberecht L., Kiffney P., et al. (2021). Characterizing the habitat function of bivalve aquaculture using underwater video. Aquac. Environ. Interact. 13, 439–454. doi: 10.3354/aei00418
Ferriss B. E., Conway-Cranos L. L., Sanderson B. L., Hoberecht L. (2019). Bivalve aquaculture and eelgrass: A global meta-analysis. Aquaculture 498, 254–262. doi: 10.1016/j.aquaculture.2018.08.046
Gee J. M. (1989). An ecological and economic review of meiofauna as food for fish. Zool. J. Linn. Soc. 96, 243–261. doi: 10.1111/j.1096-3642.1989.tb01830.x
Gelman A., Carlin J. B., Stern H. S., Dunson D. B., Vehtari A., Rubin D. B. (1995). Bayesian Data Analysis Third edition (with errors fixed as of 15 February 2021). (Boca Raton FL, USA: Chapman & Hall/CRC Press).
Holsman K. K., McDonald S. P., Armstrong D. A. (2006). Intertidal migration and habitat use by subadult Dungeness crab Cancer magister in a NE Pacific estuary. Mar. Ecol. Prog. Ser. 308, 183–195. doi: 10.3354/meps308183
Hosack G. R., Dumbauld B. R., Ruesink J. L., Armstrong D. A. (2006). Habitat associations of estuarine species: Comparisons of intertidal mudflat, seagrass (Zostera marina), and oyster (Crassostrea gigas) habitats. Estuaries Coasts 29, 1150–1160. doi: 10.1007/BF02781816
Johannessen S. C., Macdonald R. W. (2016). Geoengineering with seagrasses: Is credit due where credit is given? Environ. Res. Lett. 11. doi: 10.1088/1748-9326/11/11/113001
Kennedy L. A., Juanes F., El-Sabaawi R. (2018). Eelgrass as valuable nearshore foraging habitat for juvenile Pacific Salmon in the early marine period. Mar. Coast. Fisheries 10, 190–203. doi: 10.1002/mcf2.10018
Lepofsky D., Toniello G., Earnshaw J., Roberts C., Wilson L., Rowell K., et al. (2021). Ancient anthropogenic clam gardens of the Northwest coast expand clam habitat. Ecosystems 24, 248–260. doi: 10.1007/s10021-020-00515-6
Lotze H. K., Lenihan H. S., Bourque B. J., Bradbury R. H., Cooke R. G., Kay M. C., et al. (2006). Depletion, degradation, and recovery potential of estuaries and coastal seas. Sci. (1979) 312, 1806–1809. doi: 10.1126/science.1128035
McDevitt-Irwin J. M., Iacarella J. C., Baum J. K. (2016). Reassessing the nursery role of seagrass habitats from temperate to tropical regions: A meta-analysis. Mar. Ecol. Prog. Ser. 557, 133–143. doi: 10.3354/meps11848
McRoy C. P. (1974). Seagrass productivity: Carbon uptake experiments in eelgrass, Zostera marina. Aquaculture 4, 131–137. doi: 10.1016/0044-8486(74)90028-3
Muething K. A., Tomas F., Waldbusser G., Dumbauld B. R. (2020). On the edge: Assessing fish habitat use across the boundary between Pacific oyster aquaculture and eelgrass in Willapa Bay, Washington, USA. Aquac. Environ. Interact. 12, 541–557. doi: 10.3354/aei00381
Müller K., Bryan J. (2020). here: A Simpler Way to Find Your Files. R package version 1.0.1. Available at: https://CRAN.R-project.org/package=here.
Neuwirth E. (2022). RColorBrewer: ColorBrewer Palettes. R package version 1.1-3. Available at: https://CRAN.R-project.org/package=RColorBrewer.
Peterson B. J., Fry B. (1987). Stable isotopes in ecosystem studies. Annu. Rev. Ecol. Syst. 18, 293–320. doi: 10.1146/annurev.es.18.110187.001453
Phillips D. L., Inger R., Bearhop S., Jackson A. L., Moore J. W., Parnell A. C., et al. (2014). Best practices for use of stable isotope mixing models in food-web studies. Can. J. Zool. 92, 823–835. doi: 10.1139/cjz-2014-0127
Poppe K. L., Rybczyk J. M. (2018). Carbon sequestration in a Pacific northwest eelgrass (Zostera marina) meadow. Northwest Sci. 92, 80–91. doi: 10.3955/046.092.0202
Post D. M. (2002). Using stable isotopes to estimate trophic position: Models, methods, and assumptions. Ecology 83, 703–718. doi: 10.1890/0012-9658(2002)083[0703:USITET]2.0.CO;2
Postlethwaite V. R., McGowan A. E., Kohfeld K. E., Robinson C. L. K., Pellatt M. G. (2018). Low blue carbon storage in eelgrass (Zostera marina) meadows on the Pacific Coast of Canada. PloS One 13. doi: 10.1371/journal.pone.0198348
R Core Team (2022) R: A language and environment for statistical computing. Available at: https://www.r-project.org/.
Rubin S. P., Hayes M. C., Grossman E. E. (2018). Juvenile Chinook salmon and forage fish use of eelgrass habitats in a diked and channelized Puget Sound river delta. Mar. Coast. Fisheries 10, 435–451. doi: 10.1002/mcf2.10035
Ruckelshaus M. H., McClure M. M. (2007). Sound Science: Synthesizing ecological and socioeconomic information about the Puget Sound ecosystem (Seattle, Washington, USA: U.S. Department of Commerce, National Oceanic and Atmospheric Administration (NMFS), Northwest Fisheries Science Center).
Shull S., Bulthuis D. (2002). A methodology for mapping current and historical coverage of estuarine vegetation with aerial photography and ArcView (Washington, USA: Washington State Department of Ecology, Padilla Bay National Estuarine Research Reserve).
Steele E. N. (1964). The Immigrant Oyster (Ostrea gigas) Now Known as The Pacific Oyster. (Olympia, Washington, USA: Pacific Coast Oyster Growers Association Inc.).
Stock B. C., Jackson A. L., Ward E. J., Parnell A. C., Phillips D. L., Semmens B. X. (2018). Analyzing mixing systems using a new generation of Bayesian tracer mixing models. PeerJ 6, e5096. doi: 10.7717/peerj.5096
Stock B., Semmens B., Ward E., Parnell A., Jackson A., Phillips D. (2022) Package ‘MixSIAR.’. Available at: https://github.com/brianstock/MixSIAR/issues.
Veggerby K., Scheuerell M. D., Sanderson B., Kiffney P. (2023). Stable isotopes reveal intertidal fish and crabs use shellfish farms as foraging habitat in Puget Sound, Washington [Data set] (Meyrin, Switzerland: Zenodo). doi: 10.5281/zenodo.8118102
Veggerby K., Scheuerell M. D., Sanderson B. L., Kiffney P., Ferriss B. (in press). Shellfish aquaculture farms as foraging habitat for nearshore fishes and crabs. In Press.
Washington State Department of Health (2022) Samish Bay Annual Shellfish Growing Area Review. Available at: https://fortress.wa.gov/doh/oswpviewer/index.html (Accessed December 6, 2023).
Washington State Department of Natural Resources (2022) Seagrass Monitoring in Puget Sound, (2000-2020): What we have learned. Available at: https://storymaps.arcgis.com/stories/062778d5233542b19fc7f209c58637c2.
Waycott M., Duarte C. M., Carruthers T. J. B., Orth R. J., Dennison W. C., Olyarnik S., et al. (2009). Accelerating loss of seagrasses across the globe threatens coastal ecosystems. PNAS 106, 12377–12381. doi: 10.1073/pnas.0905620106
Wickham H., Averick M., Bryan J., Chang W., McGowan L. D., François R., et al. (2029). Welcome to the tidyverse. J. Open Source Softw. 4 (43), 1686. doi: 10.21105/joss.01686
Williams G. D. (1994) Effects of habitat modification on distribution and diets of intertidal fishes in Grays Harbor Estuary, Washington. Available at: www.lib.washington.edu/services/borrow/getit/reasons-for-cancellation.
Keywords: aquaculture, shellfish, habitat, feeding, intertidal, oysters, stable isotopes
Citation: Veggerby KB, Scheuerell MD, Sanderson BL and Kiffney PM (2024) Stable isotopes reveal intertidal fish and crabs use bivalve farms as foraging habitat in Puget Sound, Washington. Front. Mar. Sci. 10:1282225. doi: 10.3389/fmars.2023.1282225
Received: 23 August 2023; Accepted: 21 December 2023;
Published: 10 January 2024.
Edited by:
Elene Trujillo, State of Washington, United StatesReviewed by:
Kevin Alexander Hovel, San Diego State University, United StatesAl Alder, The University of Auckland, New Zealand
Copyright © 2024 Veggerby, Scheuerell, Sanderson and Kiffney. This is an open-access article distributed under the terms of the Creative Commons Attribution License (CC BY). The use, distribution or reproduction in other forums is permitted, provided the original author(s) and the copyright owner(s) are credited and that the original publication in this journal is cited, in accordance with accepted academic practice. No use, distribution or reproduction is permitted which does not comply with these terms.
*Correspondence: Karl B. Veggerby, dmVnZ2Vya0B1dy5lZHU=