- Institute for Marine and Antarctic Studies, University of Tasmania, Hobart, TAS, Australia
Temperate rocky reefs are complex habitats that support high levels of biodiversity and productivity. However, the sessile benthic communities attached to these reefs are vulnerable to climate change and local human impacts due to their limited capacity to rapidly avoid unfavourable environments. In southeastern Australia, high latitude rocky reefs face increasing threats from rapid warming, surpassing the global average. Existing management efforts for these reefs rely heavily on research from tropical coral ecosystems and shallow temperate algal-dominated rocky reefs, where sufficient data allows for reliable predictions on the future state of the systems. Recent evidence from seabed mapping programs indicates that mesophotic reefs (~30-150 m depth) are equally or more extensive than shallow systems in temperate waters. They provide novel ecosystem functions and could potentially act as climate refuges for some components of shallow water communities that extend across depth strata. However, despite their importance, very little is known about the threats faced by non-algal sessile organisms in either shallow water or mesophotic systems in many parts of the world, including temperate Australia. This review examined the current state of knowledge regarding the direct impact of physical disturbances, such as extreme weather events, ocean warming and destructive fishing practices, on sessile mesophotic communities in general. We then used this to infer the likely drivers of change in temperate mesophotic systems. We found that Australia’s temperate mesophotic ecosystems were less likely to be impacted by fishing, anchoring and lost fishing gears compared to the northern hemisphere due to lower population density. Instead, we identified present and future pulsed warming events and severe storms as major threats to Australia’s mesophotic systems. This conclusion is based on the lack of evidence supporting the existence of depth refuges from climate events in the current literature and the potential implications of the predicted future increases in high wind-wave energy and warming extending into the mesophotic depths. Drawing from observations globally, changes in the distribution of growth forms, size or diversity of sponges may be useful indicators of heat stress and seabed disturbances in temperate mesophotic systems.
1 Introduction
Almost 90% of the world’s oceans have been impacted by human activities (Jones et al., 2018). The global rise in greenhouse gas emissions, ocean warming, acidification, and intensification of storms are threatening marine ecosystems at multiple fronts and large spatial scales (Halpern et al., 2008; Bhatia et al., 2022). At local and regional scales, key human stressors such as commercial and recreational fishing, shipping, introduced species, and terrestrial-related organic and non-organic pollution further exacerbate ecosystem degradation (Halpern et al., 2019; Gissi et al., 2021).
The changing climate has brought about considerable changes in the distribution and behaviour of warm-water mobile species. In several instances, this has led to the local extinction of endemic species, while also prompting the range shift of these mobile species towards the poles (Poloczanska et al., 2013). Additionally, as a way of adapting to the warming conditions, some of these species have expanded their depth range (Pinsky et al., 2020). However, many native mobile and sessile species may be unable to acclimatise to increasing temperatures or shift their distribution rapidly enough to align with their preferred thermal conditions at current rates of warming. This can lead to potential competition from range-shifting species, which, in turn, can cause ecosystem instability due to the introduction of novel assemblages, often resulting in the local extinction and replacement of these native species (Johnson et al., 2011; McLean et al., 2021). Such changes may have cascading trophic effects if important keystone or ecosystem engineering species contract or extend their range (Johnson et al., 2011; Pinsky et al., 2020).
Barriers to latitudinal dispersal, including habitat suitability and ocean currents for larval transport, act as limiting factors for sessile taxa to range shift at the same rate as mobile species. As a result, they are being directly and disproportionately affected by climate and human pressures (Smale et al., 2019). As such, research efforts are now focused on the potential of upper mesophotic reef systems (30 – 60 m depth) to act as an additional recruitment source for a range of shallow coral and fish species after significant environmental events impact shallow populations (Bongaerts et al., 2010). However, despite the suggestion that some coral species may at times benefit from a mesophotic refuge, evidence is generally lacking for other benthic taxa, particularly in temperate regions (Laverick et al., 2018). The current consensus of the depth range of the mesophotic zone is between 30 m and 150 m (Hinderstein et al., 2010). However, there may also be the need to consider regional variations in light attenuation and changes in community assemblage from photosynthetic communities to non-light-dependent species and species richness when defining mesophotic depth boundaries (Micaroni et al., 2021; Castellan et al., 2022; Pérez-Castro et al., 2022). For example, non-light-dependent corals in the Eastern Tropical Pacific can start to dominate as shallow as 13 m depth or from 45 m depth, depending on the seasonal changes in water clarity (Pérez-Castro et al., 2022); whereas temperate mesophotic ecosystems in Ireland are found in depths between 12 and 40 m (Micaroni et al., 2021).
The potential for a depth refugia for shallow water species may be limited by the differences in community composition along the depth gradient, and the complex, dynamic outcomes of species interactions as a function of prevailing environmental conditions. For example, diversity hotspots like the Great Barrier Reef (GBR) have a higher proportion of mesophotic-depth specialist species, resulting in lower community overlap and connectivity with the shallow water communities (Laverick et al., 2018). Moreover, species exhibit differences in their susceptibility to a range of stressors and intensities, leading to distinct species-specific recovery patterns and resulting in a divergence in community structure with depth (Hernandez-Agreda et al., 2021). Importantly, fluctuating environmental variables such as temperature, nutrient levels, and anthropogenic stressors can trigger dynamic shifts in direct and indirect species interactions, encompassing competition, predation, mediation, and symbiosis (Johnson et al., 2011; Mauro et al., 2022). Due to these complexities, it may be prudent to implement targeted management approaches for resource extraction and conservation purposes tailored to the unique characteristics of each community (Halpern et al., 2019).
1.1 Australian context
Australia offers an illustrative case study that showcases the application of ecosystem-based management practices to coastal habitats. Australia’s marine estate spans approximately 14 million square kilometres and has a variety of habitats ranging from estuarine mangroves to some of the world’s largest coral and rocky reef systems (Bennett et al., 2015; Smith et al., 2017). To manage this diverse marine environment, state/territorial government agencies such as fisheries (e.g., Victorian Fisheries Authority, the Department of Natural Resources and Environment Tasmania, Northern Territory Department of Industry Tourism and Trade, Queensland Department of Agriculture and Fisheries, New South Wales Department of Primary Industries, and Western Australia Primary Industries and Regional Development) and marine park authorities (e.g., Tasmania Parks and Wildlife Service, Parks Victoria, and National Parks and Wildlife Service South Australia) are responsible for management of various aspects of the estate and sectors inside three nautical miles from the coast. Waters beyond this limit but within Australia’s Exclusive Economic Zone are under the Commonwealth government’s jurisdiction and jointly managed by Commonwealth agencies including Parks Australia (for the Australian Marine Park (AMP) network) and the Australian Fisheries Management Agency for remaining waters, including fisheries operating within the marine parks where permitted. An exception to this is the GBR where Queensland state government and the Commonwealth government have overlapping jurisdictional arrangements (Smith et al., 2017).
Most of the mesophotic ecosystems in Australian waters are found within Commonwealth waters, and the primary form of conservation-protection of these is within the newly established AMP network (Hayes et al., 2021). These marine parks were established to represent Australia’s offshore bioregions, each accompanied by management plans, with new management plans for the network implemented in 2018. These plans identify monitoring priorities for conservation and pressures across seven regionally divided AMP networks (Australian Marine Parks, 2023). Despite this recent conservation initiative, there are suggestions that Australia’s marine ecosystems are regressing, partly due to uncontrollable stressors like acute weather disturbances (Evans et al., 2016). The ecosystem health may worsen as many new AMPs have recently had proposed protection levels downgraded, with many now permitting most forms of commercial fishing in previously designated ‘no-take’ zones (Albrecht et al., 2021). As the majority of the AMP network is relatively new, essential data elements necessary for effective management such as knowledge of the distribution/presence of species and the extent of key habitats and ecosystem functioning, are often lacking (Australian Marine Parks, 2023). However, since the advent of remote sensing platforms and vehicles (Verfuss et al., 2019), the speed of knowledge acquisition for Australia’s shallow and mesophotic ecosystems is improving rapidly (Williams et al., 2010; Warnock et al., 2016; James et al., 2017). As a result, management plans have the potential to be constantly revised to incorporate the new knowledge derived from these advancements (Hayes et al., 2021).
The GBR Marine Park, overseen by the Great Barrier Reef Marine Park Authority (GBRMPA), an independent Australian Government agency, operates independently from the AMP network. With its long establishment and management history, along with an extensive range of monitoring programs, the GBR is recognised as a data-rich system. The integrated management and monitoring framework developed by the GBRMPA is now one of the world’s most comprehensive ecosystem-based management programs to date (GBRMPA, 2023). As a result of multi-decadal monitoring, the current GBR adaptive plan (Commonwealth of Australia, 2021) addresses many threats to the reef, including expansion of its Crown-of-Thorns starfish, Acanthaster planci, control program, and improving land management practices, aiming to reduce sediment runoff that can amplify coral mortality and compromising their recovery from climate disturbances (De’ath et al., 2012). Monitoring of water quality to ensure that nutrient loading and pollution are promptly identified and addressed is also part of the strategic updates in the adaptive plan intended to preserve biodiversity and resilience of the GBR ecosystem (Leverington et al., 2019).
Compared to the GBR with a length of 2300 km, Australia’s temperate reefs have much less extensive temporal and spatial monitoring (Barrett et al., 2014), despite spanning over 8000 km of the coastline and having comparable social, ecological and economic value (Bennett et al., 2015). At present, Australia’s temperate ecosystem research and management is mainly focused on kelp-covered reefs and coastal shallow systems that are approximately 5.5 km from shore. This emphasis is due to the proximity of the foundation kelp species like Ecklonia radiata to human pressures (Bennett et al., 2015). For example, in the southeastern region of Australia, rapid ocean warming has caused significant changes such as range shifts and increased influence of nutrient-poor waters. These changes have led to the rapid reorganisation of Tasmania’s temperate shallow rocky reef assemblages along the north-eastern coastline, where the colonisation by the range-shifting long-spined sea urchin, Centrostephanus rodgersii, has replaced kelp beds with urchin-grazed barrens (Johnson et al., 2011; Vergés et al., 2019). In response to this transformation, various management strategies have been implemented, particularly to protect commercial fisheries that rely on kelp habitat. Multi-pronged approaches to mitigate the destructive grazing of this species include boosting lobster stocks (a predator of urchins) on the east coast of Tasmania to greater than 20% of the original biomass, and encouraging and incentivising commercial harvesting of C. rodgersii (Dubecki, 2020; Westcott et al., 2020).
Most research and monitoring to date has focussed on nearshore temperate kelp systems. Hence, the full extent of biodiversity or conservation values present in other productive temperate habitat-forming groups (e.g., sponges, soft-bodied cnidarians, bryozoans, ascidians) in both shallow and mesophotic shelf systems in Australia remains unclear (Wood et al., 2012; De Goeij et al., 2013). Furthermore, there is a limited understanding of the types and magnitude of threats these habitats face due to the significant lack of baseline or monitoring data (Hedge et al., 2022). Apart from shallow kelp-dominated systems, there is scant information available to support context-specific management approaches for such pressures, particularly in mesophotic systems in Australia (Australian Marine Parks, 2023).
To address the knowledge gap in ecosystem-based management of temperate mesophotic systems, we need to develop both conceptual and empirically based predictive models that can help estimate the potential ecological impacts resulting from cumulative pressures. These models should initially be built upon existing knowledge, then validated through field sampling, and continuously refined through ongoing monitoring. This approach can help 1) identify spatial and temporal patterns, 2) untangle the influence of individual pressures and 3) measure responses to management actions (Dietze et al., 2018). Despite the scarcity of data for temperate mesophotic systems, it is imperative that managers promptly undertake initial precautionary actions to enhance ecosystem resilience. Valuable insights can be drawn from previous research conducted on tropical mesophotic and temperate shallow systems. This allows anticipation of potential threats, prediction of the nature of their interactions, and development of hypotheses regarding their possible mechanisms of action. Such an approach may help expedite decision-making regarding the most critical factors for on-ground monitoring and undertaking empirical validation of mesophotic reef species distribution and temporal trends.
This review serves as the initial step in assessing possible threats and the mesophotic system’s responses to anthropogenic stressors. We aim to explore: 1) where our current knowledge is at with respect to the health and functioning of temperate mesophotic systems globally; 2) whether temperate mesophotic communities are either more, less, or equally as vulnerable to localised stressors and climate disturbances as those in the tropical mesophotic systems or shallow systems, where applicable; and 3) identify how threats are likely to affect Australia’s temperate mesophotic systems. Here, we focus on sessile species as they are typically more susceptible to climate and human pressures than motile benthic taxa, and hence are good indicators of subtle environmental change (Smale et al., 2019). Furthermore, direct observations of their response to pressures are lacking compared to pelagic species (Poloczanska et al., 2016). Likely, cascading ecosystem effects from fishing and climate change may also be occurring in the mesophotic systems. We focus here on the direct impacts of these anthropogenic stressors due to the current limited knowledge of mesophotic systems.
2 Methods
A search strategy and method of synthesis for each objective were assigned to each question (Table 1). To address these, a systematic bibliographic search for peer-reviewed articles published until the end of 2022 was performed using the Web of Science and Scopus databases in accordance with the PRISMA (Preferred Reporting Items for Systematic Reviews and Meta Analyses) guidelines (Page et al., 2021). This search strategy yielded 3,733 full-text articles written in English. We excluded papers that were theoretical, riverine-, estuary-, terrestrial- or paleo-based, chemical analyses of sediments, microbial, genomics, human health or social science management and planning, and articles without a case study relating to anthropogenic stressors (i.e., reviews, commentary, editorials, perspectives, and letters) (Supplementary Figure 1). The bibliography resulting from the full screen and the data from the coding strategy below are in Supplementary File 1.
2.1 Data coding strategy
2.1.1 Mesophotic literature review themes
We examined each review paper for recurring topics. Each topic must have at least one paragraph of critique to be included in the count and were then grouped into key themes (Table 2). Using the ‘vegan’ package in R (Oksanen et al., 2017), redundancy analysis was performed on presence-absence of each theme (Hellinger transformed) and research gaps against publication year and geographical coverage of the review papers.
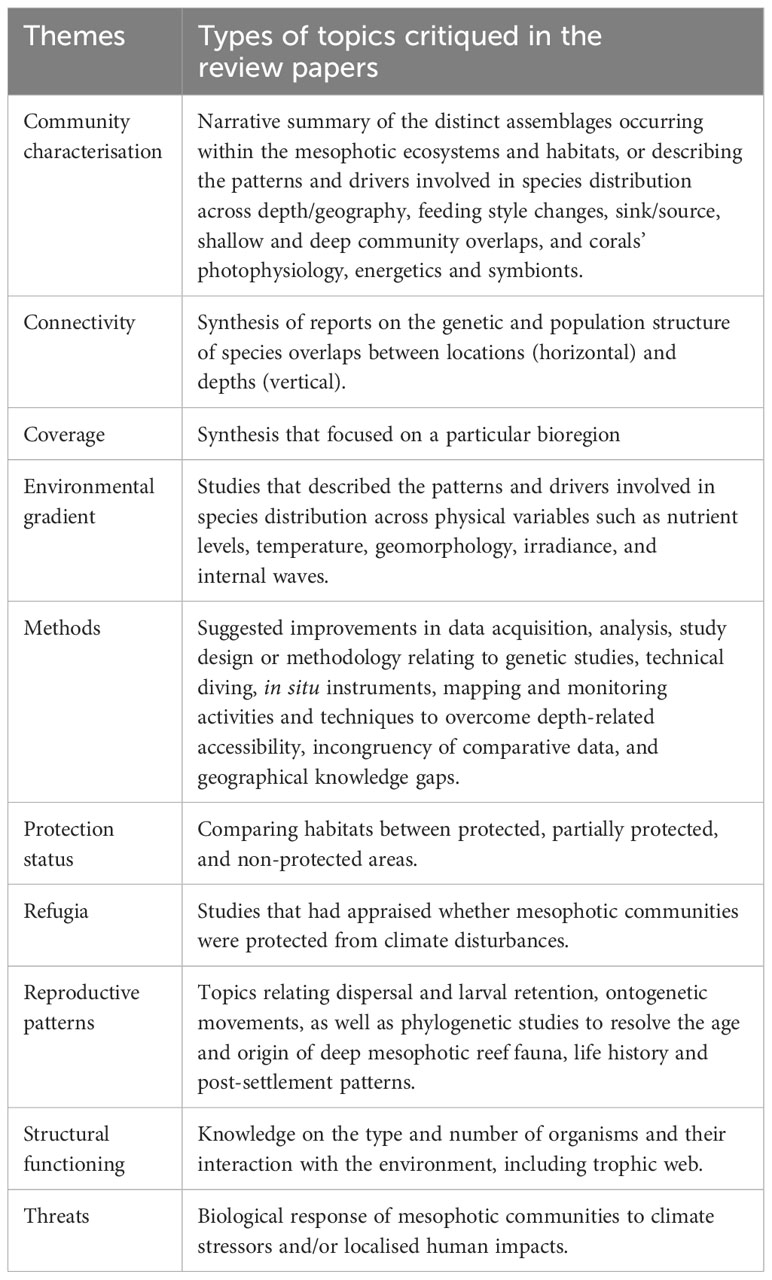
Table 2 Descriptions of the type of works critiqued in the review papers as classified under each theme.
2.1.2 Stressor themes between tropical and temperate bioregions
Case studies describing benthic sessile invertebrates’ responses to various stressors were grouped into six themes (Table 3). Here, the mesophotic zone was defined by a depth range of 30 – 150 m (Hinderstein et al., 2010). Recognising that some shallow system surveys were conducted over depths beyond 30 m, we also included these papers in our analysis. To do this, the depth range was not applied to the first screening. Abstracts were screened for relevancy to our review. For case reports, we retained articles that directly assessed or modelled the response of reefs’ biota to stressors obtained through observations in the field. If ambiguity remained, we examined the full text for its suitability. We scrutinised each non-mesophotic specific paper relating to weather disturbances to determine the depth range surveyed, ensuring that the survey depths extended beyond 30 m for thoroughness. The minimum depth criteria of 30 m to be included in the analysis did not apply to papers on seabed impacts.
To assess whether depth serves as an effective barrier against the impact of pulsed warming or severe storm events, we examined the range of surveyed depths and categorised the response of organisms or communities (positive, no difference or negative) based on the reported results and the authors’ interpretation.
To compare marine bioregions, each paper was differentiated into tropical or temperate according to their survey sites (Beger et al., 2014). In most cases, tropical reefs lie between the Tropic of Cancer and the Tropic of Capricorn. Whilst there is no consensus on the latitudinal cut-off for sub-tropical or warm-temperate biome, this transition zone is usually characterised by an overlap between tropical and temperate biota. Along Australia’s coastline, the cold-temperate bioregion starts in areas where the average water temperature is less than 22° C (Kahng et al., 2012) and is dominated by macrophytes habitat formers such as kelp and seagrass (Wernberg et al., 2011). Where ambiguous, we used the latitudes of 23.5° to 40° north and south as the cut-off for the warm temperate bioregion, and between 40° and 70° north and south for the cold-temperate.
2.1.3 Seabed impacts
There are several meta-analyses on the direct disturbance of bottom fishing on the benthos (Sciberras et al., 2018), the rate of ecosystem recovery (Kaiser et al., 2006; Hiddink et al., 2017), and comparisons of the response and recovery of benthic communities to various fishing gear (Collie et al., 2000). These reviews included studies on specific phyla, functional groups, or benthic communities in general. Here, we examined the direct seabed impact on the response and recovery specific to benthic communities that must include sessile species. For each metric examined, either an ‘increase’, ‘decrease’ or ‘no change’ was assigned according to the findings. If unclear, the direction of the response was determined based on the authors’ interpretation. The count data was then weighted by the number of papers for each type of seabed impact. We ran a Canonical Correspondence Analysis (CCA) using the ‘vegan’ package in R (Oksanen et al., 2017) to explore the relationships between the direction of response and types of seabed impacts.
2.2 Ranking of relative threats to mesophotic systems
We quantified the magnitude of threat impacts on mesophotic systems reported in the literature and conducted a comparative analysis of these threats in the context of temperate Australia. Seven threats were identified from our analysis of the literature: warming, floods, storms, fishing impacts on soft and hard substrate, lost fishing gear, and anchoring. We evaluated the vulnerability of the systems by considering the occurrence, recurrence, extent, impact, and longevity of each threat. We included a measure of uncertainty that allowed the ranks for each threat to be weighted by the current state of knowledge (see Table 4 for definitions). The vulnerability and uncertainty weighting scores were multiplied to give a single score, which was adjusted by square root transformation to enhance the interpretability of the scores. The magnitude of the threat was categorised as mild, moderate, high, or severe using quartiles based on the maximum score of 28.
3 Results and discussion
3.1 Temporal trends in mesophotic research
Until recently, review publications examining mesophotic research focussed entirely on the impact of thermal disturbances on the ecosystem. This focus was primarily driven by interest in exploring the validity of the ‘deep reef refuge’ hypothesis, coined in 2010 (Bongaerts et al., 2010), developed around the potential of deeper reefs to provide climate refuge for some species in tropical coral reef systems. However, there was a shift in focus for review papers starting in 2018, moving away from the refugia hypothesis and towards understanding the broader environmental drivers and ecological implications of threats for mesophotic communities (Figure 1A). The most recent review paper in this space presented a broad management framework tailored to temperate mesophotic rocky reef systems based on potential drivers for their community structure and response to large-scale and localised stressors (Bell et al., 2022).
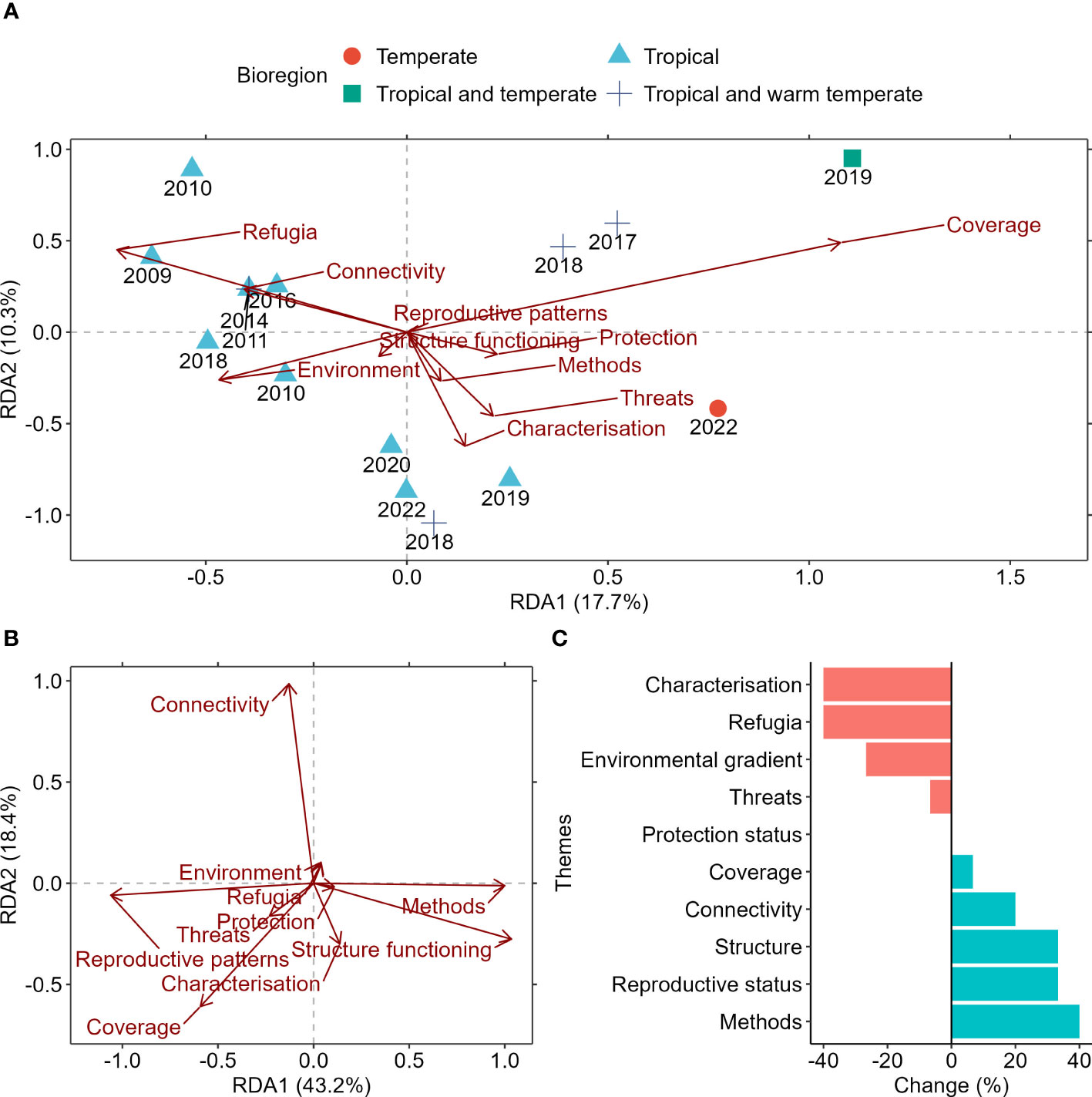
Figure 1 (A) Redundancy analysis (RDA) of the relationships between presence-absence of key research themes and bioregions identified from review papers on mesophotic systems and the year of publication. (B) RDA of presence-absence in research gaps identified for each theme. Arrows indicate the direction and magnitude of variables. (C) Percent change in the temporal trends of knowledge gaps identified. A negative change in trend would suggest a reduced emphasis for that theme over time because either knowledge in the field has improved, or the emphasis has changed. Likewise, a positive change would imply a priority for future research to improve knowledge in the area.
The effect of conservation, reproductive patterns of organisms, structural functioning of mesophotic ecosystems and critique of methods were the least reviewed themes (short vector lengths in Figure 2A) and remained as an identified research gap since 2009 (long vector lengths in Figure 1B). The lack of knowledge advancement in the above-identified themes highlighted a significant handicap in the field’s capacity to generate critical knowledge to keep pace with the rapidly changing environment. Importantly, the effect of fully protected marine protected areas (MPAs) and the impacts of stressors on mesophotic ecosystems were understudied and had not been listed as a research priority (Figure 1C). The latter was surprising considering the global trends of increasing threats on both systems (Figure 1B and see our results henceforth). In the context of tropical systems, a large volume of knowledge has been generated due to the imperative to preserve coral populations heavily impacted by bleaching events. However, this does not imply that coral and non-coral communities at higher latitudes are unaffected by climate disturbances. Furthermore, the lack of studies investigating the impact of protection on mesophotic systems can be attributed, in part, to data deficiency which led to the limited understanding of the role, distribution, and significance of mesophotic ecosystems. Historical exclusion of mesophotic systems from fully protected MPAs and MPA-related research may have also contributed to this knowledge gap.
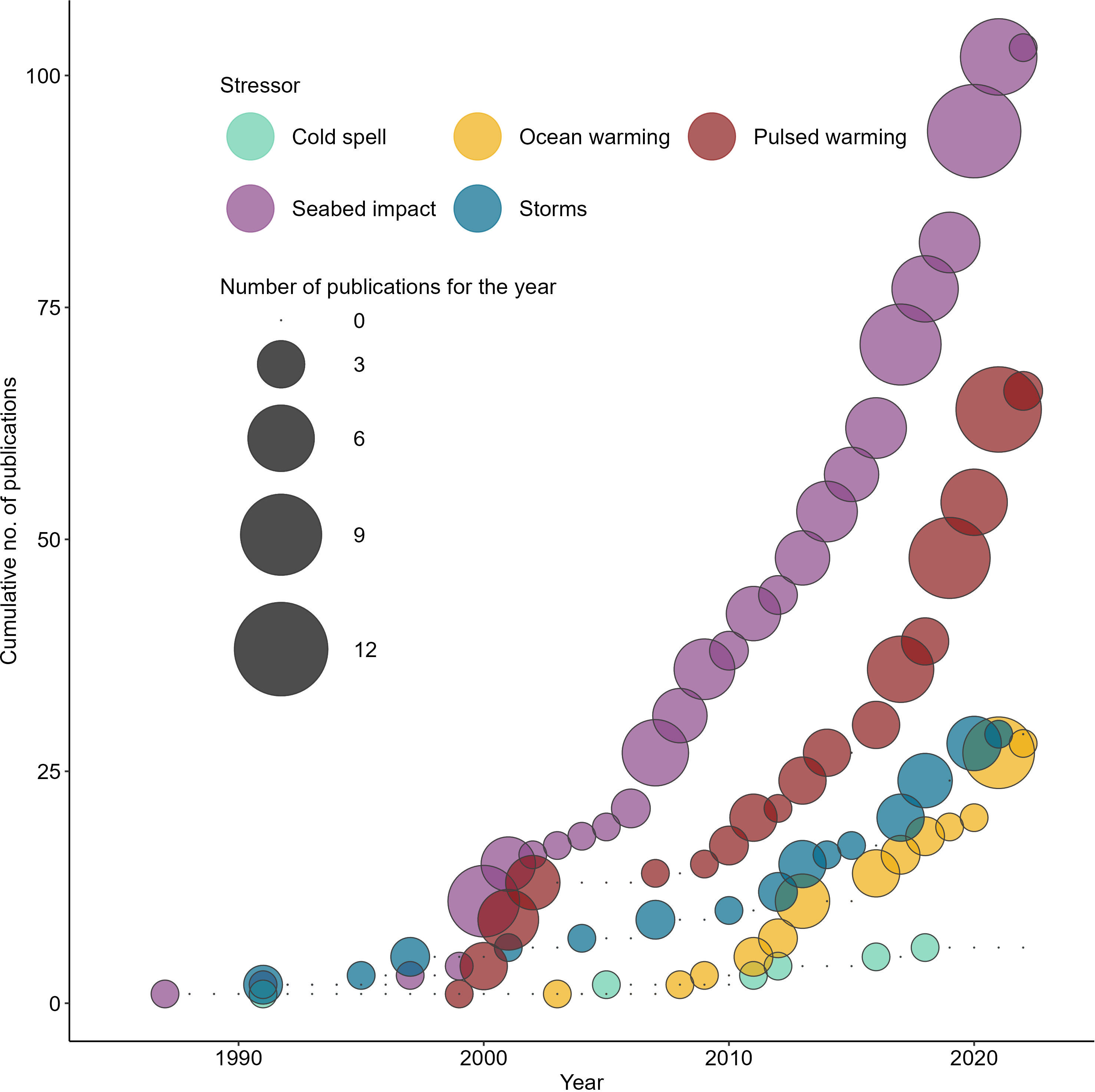
Figure 2 Temporal trend for publications according to the assigned stressor themes. ‘Seabed impact’ refers to physical disturbances to the seafloor as result of fishing or boating related activities.
It is important to note that 13 out of 15 review papers were narrative reviews and two papers were systematic reviews (Turner et al., 2017; Bell et al., 2022). Because narrative reviews allow for a deeper understanding of the topic, they often cover only one or two themes, which might have contributed to the low per cent variation explained by the constrained axes (Figure 1).
3.2 Response to seabed impacts
Of the 262 papers retained, the number of publications relating to seabed impact was the highest (n=103), with most case studies reported from the cold-temperate regions (n=79) (Supplementary Figure 2). Whilst multi-national efforts have been made to restrict, minimise, and mitigate destructive fishing practices (Halpern et al., 2019), the number of publications per year that characterised seabed impacts from fishing activities has remained steady since 1987, reflecting the persistence of this stressor as a driver of research (Figure 2).
Within ‘seabed impact’, the proportion of papers on trawling was overwhelming (Figure 3A). Due to the lack of historical ecological data, 42.7% of papers were a one-time assessment of the state of the habitat at a specific point in time, which might not capture trends or changes over a longer timeframe. The heterogeneity in resampling intervals between studies, type of seabed, and lack of comparable reference sites may have underestimated the full impact and recovery prediction of benthic communities (Figure 3B). The spatial and temporal heterogeneity in species abundance and composition between treatment and control sites (Lindegarth et al., 2000) might also have contributed to the lack of meaningful change in short-lived experiments (Figure 3C) and an underestimation of the true footprint (Gall et al., 2020). Regardless, site closure may have a positive effect on species’ recovery but was only evident after at least 10 years (Figure 3B) and broadly limited to soft sediment communities (Figure 3D).
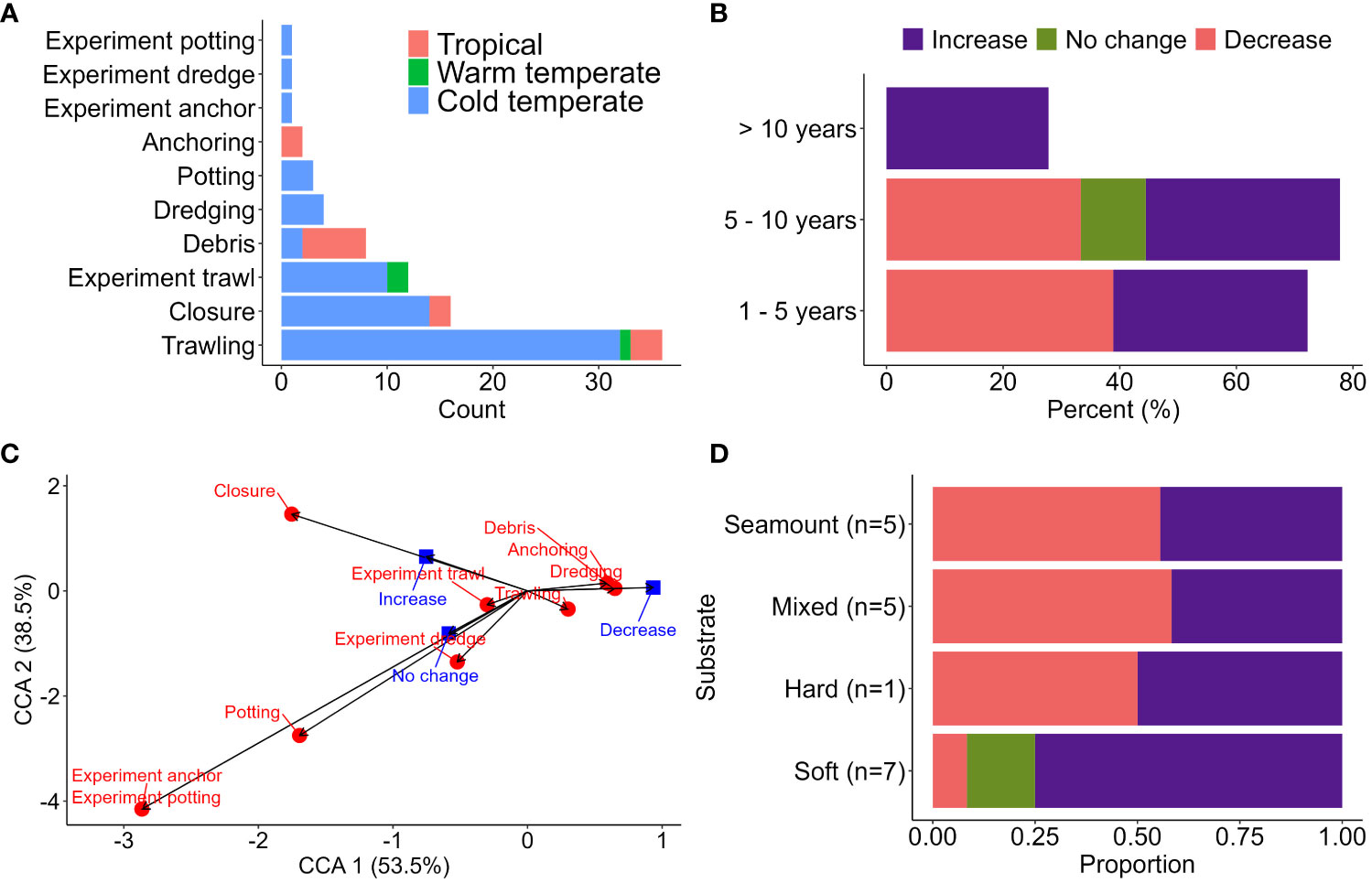
Figure 3 (A) Number of papers documenting the effects of various stressors on the seabed communities by bioregions. (B) Effect of fishery closure on benthic communities based on lag time since closure. (C) Canonical correspondence analysis (CCA) comparing the documented biological response (blue squares) in response to various stressors on the seabed (red circles). The type of responses included abundance, diversity, size class, density, community structure, trophic structure, richness, biomass, and structural complexity. (D) Response of benthic communities after site closure by the dominant substrate type at fished sites (number of studies for each substrate type in brackets).
The impacts on sessile epifauna in hard bottom or rocky reef habitats vary between trawling (Clark et al., 2015; Clark et al., 2019; Williams et al., 2020) and potting (Coleman et al., 2013; Stephenson et al., 2017). Benthic sessile invertebrates with an erect morphology (e.g., habitat-forming corals Solenosmilia variabilis and Madrepora oculata (Clark and Rowden, 2009) and sponges (Morrison et al., 2020) tend to be most affected by the sweeping action of trawling gear. The benthic communities on shallower seamounts (< 950 m deep) are more affected due to higher fishing intensity (Williams et al., 2020), with limited evidence of the recovery of benthic assemblage composition even after 15 years of fishing cessation (Clark et al., 2019). In contrast, experimental pot fishing at a high intensity, exceeding local commercial pot fisheries, showed little change in benthic faunal and algal abundance and community assemblage between lightly fished and heavily fished experiment sites (Stephenson et al., 2017). Similarly, sites closed to fishing for five years had comparable abundance of erect epifauna to fished sites (Coleman et al., 2013). It is important to note that the existing (and limited) studies on potting focussed on structure-forming species (Stevens, 2020), but potting may have substantial impacts on other sessile and motile epifauna including octocorals, hydroids and bryozoans. These organisms are critical epibenthic food sources and habitat providers and play an important role in regulating the primary and secondary productions within the food chain (Gili and Coma, 1998).
Mesophotic erect invertebrates between 40 and 120 m depth were also most susceptible to derelict fishing gear (e.g., lines and nets) including macro litter (Consoli et al., 2019; Mulochau et al., 2020). The spatial distribution of lost lines and nets is hard to predict as they drift with currents, posing a challenge for management. Lost pots and traps are less problematic because when wedged in rocky and hard bottom reefs they may form another habitat like an artificial reef serving as a refuge for small-bodied organisms (Goodman et al., 2021). However, if moved by strong currents, the damage footprint may be substantial (Lewis et al., 2009).
The existing body of published evidence on anchoring impacts primarily focuses on recreational anchoring’s effects on benthic communities in shallow tropical waters (< 30 m) (Figure 3). However, there is a growing awareness regarding the potential impact of anchoring by large vessels on sensitive habitats, although these impacts have not yet been quantified (Davis et al., 2022). Despite this, the lack of published case studies in the temperate and tropical mesophotic regions for ocean-going vessels (e.g., cruise ships and container vessels) may not be considered as ‘evidence of absence’ of an impact because anchors from these vessels can be deployed into mesophotic depths and are likely to cause extensive damage to the habitats (Davis et al., 2016; Davis et al., 2022). Strong wind and currents can cause the anchor chain to oscillate, an action likened to trawling or pot hauling, displacing large volumes of sediments and smothering filter feeders and small-bodied or encrusting fauna in neighbouring reefs (Kutti et al., 2015). Physical recovery of the seabed from anchor chain scour can take more than four years (Watson et al., 2022). Differences in recovery potential exist between taxa (Broad et al., 2020). For example, fast-growing seagrass beds (e.g., Halodule wrightii) made full recovery within nine months after anchor scour simulation (Creed and Amado Filho, 1999). In contrast, live coral cover, reef structural complexity, and fish density remained lower than those at undamaged sites a decade after an accidental anchoring event by a cruise liner on a coral reef (Forrester et al., 2016). Ultimately, the size of the ecological footprint is contingent on the confluence of factors, including the composition of the seafloor, the type of anchor and frequency of deployment over the same area, and the diversity in stress tolerance among different species.
3.3 Implications of seabed impact for temperate Australia
Many of the various seabed stressors identified from global literature are less relevant for temperate Australia (Table 5). For example, trawling activities for all of Australia are declining from historical highs (Pitcher et al., 2018), and they are currently amongst the lowest in the world compared to areas in Europe where shelf productivity is significantly higher (Amoroso et al., 2018). However, the correlation between trawling and potting footprint and actual impact on the seabed communities is unknown due to insufficient data on benthic habitats and their distribution. Nonetheless, a predictive model of impact has highlighted the potential ramifications of high-intensity fishing in areas overlapping sensitive and rare benthic communities (Pitcher et al., 2018). Ideally, the focus should be on assessing impacts in intensively trawled areas compared to strategically placed fully protected MPAs that can serve as appropriate reference areas with no fishing activity.
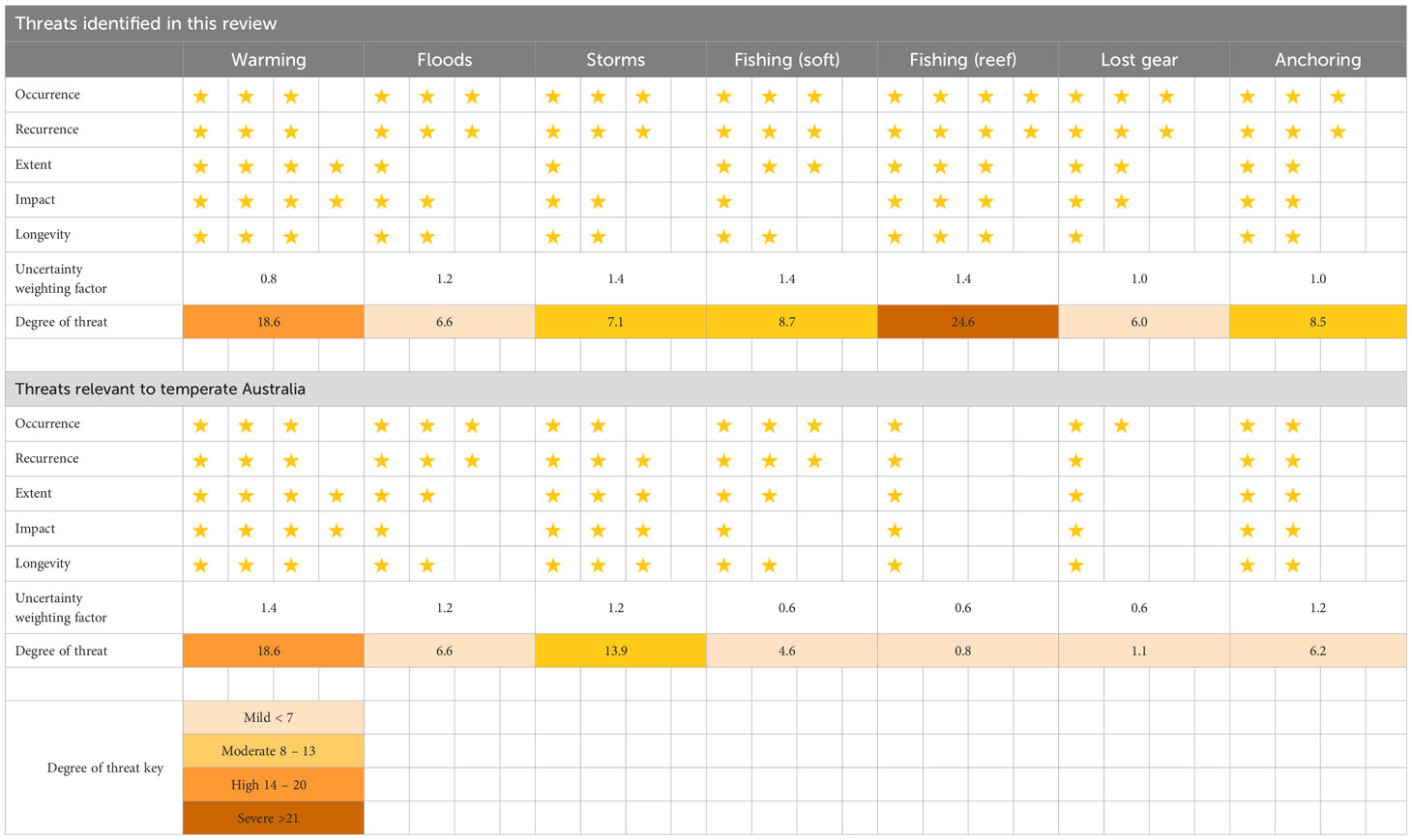
Table 5 Semi-quantitative assessment of the relative threats to mesophotic systems reviewed in the literature and their applicability to temperate Australia. Each quality was assigned a value, represented by number of stars (see Table 4), and the values and the uncertainty weighting factor were multiplied, then squared root to calculate the degree of pressure and threat.
Non-trawling fishing remains high in temperate regions (Trebilco et al., 2021), and derelict fishing gears have had negative interactions with megafauna in Australian waters (Commonwealth of Australia, 2018). The impact of locally lost fishing gear on the benthos in temperate Australia has not been assessed. Likewise, the relative footprint of anchoring activities in Australia is unlikely to be extensive due to the sheer size of the continent, despite an upward trajectory in shipping and vessel traffic (Commonwealth of Australia, 2016) (Table 3). Moreover, hotspots for anchor drops in Australia may only be concentrated in small offshore non-designated anchoring areas outside port limits, particularly in mesophotic depths near hub ports in major cities such as New South Wales, Melbourne, and Western Australia (Davis et al., 2016).
3.4 Mesophotic communities’ responses to thermal stress and severe storms
Pulsed warming events on tropical reef systems were the next largest stressor examined (n=66) and the number of papers has risen sharply since 2010 (Figure 2). In 2021 alone, there were 10 and seven published titles under pulsed warming and ocean warming respectively, which was more than any other years represented in this review. Within tropical reef systems, nine studies out of 19 papers relating to acute and chronic warming and severe storms had surveyed beyond 30 m depth, but only two single studies were designed specifically to assess mesophotic fauna’s response to pulsed warming events (Smith et al., 2016; Eyal et al., 2022).
Whilst there were relatively fewer papers on pulsed warming impacts on temperate mesophotic systems than in the tropics (30% of the total of 66 papers included), 36% of the studies in the temperate regions were conducted exclusively within mesophotic depths. There is a 10-year gap in publication between 2000 and 2009, and from 2009 to 2019. Since 2019, one paper per year on the impact of pulsed warming on temperate mesophotic fauna has been published (Figure 4). The recent increase in the number of case studies on the impact of pulsed warming on mesophotic communities is likely due to improvement in the imaging capabilities of mesophotic reefs (Armstrong et al., 2019) rather than the absence of pulsed warming events.
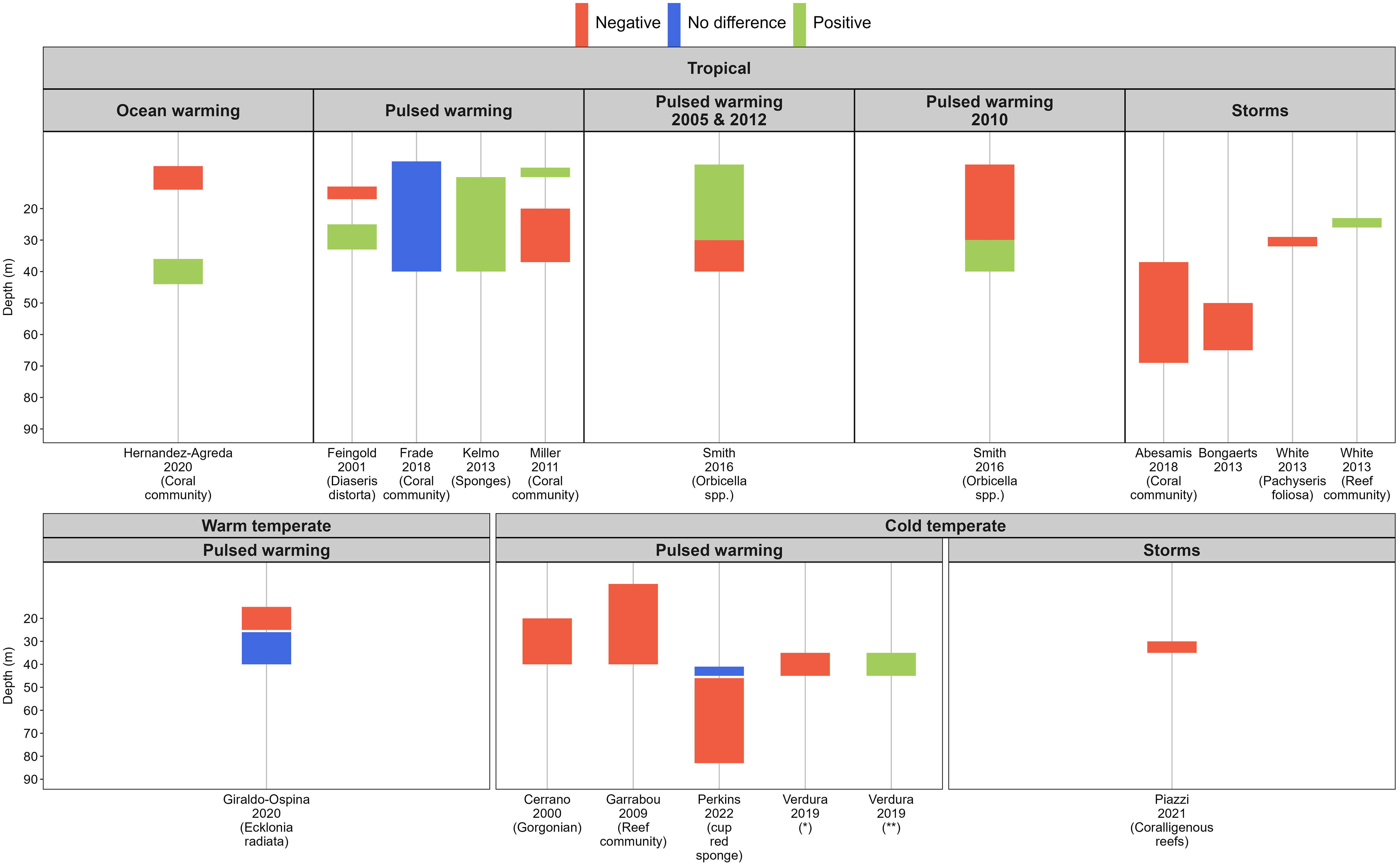
Figure 4 Biological responses of various benthic organisms and communities in the mesophotic zone following extreme weather disturbances across the survey depth gradient by bioregions and stressors, indicated by the first author, year of publication and organisms studied. “Ocean warming” included pulsed warming events and cyclones. ‘Positive’ (in green) indicates comparatively less severe response than other corresponding depths within the study; vice versa for ‘Negative (in red)’. ‘No difference’ (in blue) signifies no difference in the response between reference depths or in a before-after study design. *Axinella damicornis and Crambe crambe (sponge), Alcyonium acaule (cnidarian), Pseudosistoma cyrnusense (tunicate), Scrupocellaria mamillata (bryozoan). ** Small invertebrates and algae, Dictyopteris sp. and Carpomitra costa (erect algae), Savignyella (bryozoan).
Based on the papers included in the analysis, we found no support for the concept of depth refugia from pulsed warming or extreme storm events for mesophotic non-algal sessile communities in the warm and cold temperate regions (Figure 4). We acknowledge that our results are influenced by the search strategy and the theoretical depth threshold of 30 meters for the mesophotic zone. It is worth noting that the refinement of mesophotic habitat definitions, considering factors like community composition or photosynthetically active radiation measurements, is a recent development in the field. Many papers we reviewed had not reported such details at the time of their publication. Nonetheless, we observed species-specific responses to pulsed warming events within the community assemblage. For example, tropical mesophotic sponges were more resilient to warming than hard corals, bryozoans or ascidians (Kelmo et al., 2013) (Figure 4), and as such sponges are hypothesised to be the sessile species with higher resilience to warming under future climate change scenarios (Bell et al., 2018). Likewise, the extent of mortality of temperate sponges in the upper mesophotic depth (< 40 m) was comparatively lower than cnidarians and tunicates following a single pulsed warming event in the northwestern Mediterranean region (Cerrano et al., 2000; Garrabou et al., 2009; Verdura et al., 2019). However, the mortality patterns between sponges and gorgonians were not uniform amongst sites and were related to the synergistic effects of warming and opportunistic pathogens (Cerrano et al., 2000). The virulence of sponge diseases induced by acute warming conditions is believed to have contributed to the disappearance of a branching sponge in Long Island Sound, in the northwest Atlantic (Stefaniak et al., 2014). Regardless, more direct observation studies are needed in both regions to determine whether there is a consistent pattern in sponge resilience to warming. It is worth noting that gorgonians and black corals are the primary ecosystem engineers in the Mediterranean’s mesophotic systems (Gori et al., 2017), whilst sponges are the dominant phylum in temperate Australia (James et al., 2017), and their abundance increases with depth (Bell et al., 2022). Hence, the response and potential ecosystem impact of warming on temperate Australia’s mesophotic invertebrate communities is likely to differ from the Mediterranean.
Sessile species in shallow waters, which exhibit depth distributions extending into the mesophotic zone (i.e., depth-generalists), are less affected by pulsed warming events. This resilience is primarily attributed to the cooler temperatures found in deeper waters (Feingold, 2001; Frade et al., 2018). Furthermore, physiological adaptations to low-light conditions and frequent exposure to high-temperature variability are among the factors contributing to heat stress tolerance in mesophotic organisms. For instance, habitat-forming seaweeds in deeper waters (around 40 meters) in the warm temperate regions of West Australia have demonstrated this resilience (Giraldo-Ospina et al., 2020).
In contrast, depth-specialised species are less likely to have acclimated to sudden temperature changes, making them more vulnerable to heat stress when the thermocline deepens (Eyal et al., 2022). However, it’s essential to consider local environmental conditions, such as the availability of hard-substrate habitat, light, nutrient availability, and food sources, as well as changes in species interactions, when assessing the potential of depth refugia as a short-term buffer against thermal stress (Witman et al., 2023). Even if mesophotic communities are not directly impacted by discrete warming events, there are knock-on consequences of acute seawater temperature increases, including the proliferation of opportunistic pathogens that can lead to tissue necrosis or disease in temperate sessile invertebrates (Cerrano et al., 2001; Stefaniak et al., 2014; Corinaldesi et al., 2022).
3.5 Implications of future pulsed warming events in temperate Australia
In the context of ocean warming, the frequency and intensity of marine heatwaves (i.e., pulsed warming events) around Australia are increasing due to climate change. When compared to the tropical north, the number of Marine Heatwave Days categorised as strong or severe has been notably higher over the past two decades for Western Australia (22° to 32.5°S) and South Australia (31° to 40°S). In southeastern Australia (37° to 46°S), Marine Heatwave Days exceeding 100 days in those categories have been annual since 2014 (Kajtar et al., 2021). Pulsed warming events in southeast Australia can be partially attributable to two main factors: the strengthening of the southward extension of the East Australian Current (EAC), a western branch of the South Pacific subtropical gyre (Oliver et al., 2017), as well as the increased poleward displacement of warm, nutrient-poor waters caused by heightened eddy activities (Cetina-Heredia et al., 2014). This prevailing circulation feature has amplified surface warming and oligotrophic conditions beyond 28°S (Cetina-Heredia et al., 2014), which in turn, correlates with decreased frequency and density in biological aggregations near the surface (Phillips et al., 2022). Additionally, this circulation feature has enabled the migration of viable planktonic larvae from the east coast of Australia, facilitating their settlement into new habitats at higher latitudes. Collectively, these factors have contributed to the decline in kelp habitats in coastal systems along southeast Australia (Johnson et al., 2011). These factors can also potentially impact the mesophotic shelf community structure, but at present, our lack of knowledge of the ecology is hindering our capacity to predict response to climate change.
The limited effect of a ‘depth buffer’ from pulsed warming in tropical mesophotic systems (Figure 4) offers key lessons that are relevant to temperate mesophotic systems: temperate mesophotic fauna may be at risk of 1) prolonged exposure to above-average temperatures due to reduced heat dissipation in the water column after a pulsed warming event (Miller et al., 2011; Frade et al., 2018); and 2) regional oceanographic changes (e.g., deepening of mixed layer depth, flow reversal of bottom currents or unseasonal upwelling) that can cause abnormally high water temperatures (Cerrano et al., 2000; Guihen et al., 2012; Smith et al., 2016). Sub-surface warming in the cold temperate region of Australia has been documented to reach depths of up to 200 m (Oliver et al., 2018). Notably, this phenomenon can manifest without a concurrent surface warming signature (Porter et al., 2021), and may persist at depth for as long as two years (Jackson et al., 2018). Furthermore, the extent of accelerated warming, both at the surface and bottom over the last 20 years, has exhibited variability across different depths and latitudes along the coastal sites within the EAC southern extension region (Hemming et al., 2023). This intricate variability poses a significant challenge in predicting the spatial and temporal reach of regional marine heatwaves. The years 2010, 2013, and 2014 saw a series of pulsed warming events in southeast Tasmania, Australia, which resulted in bleaching of red cup sponges below 50 m depth, although mortality rates of sponges and other sessile mesophotic species following the pulsed warming events were not monitored (Perkins et al., 2022). Given current knowledge, temperate mesophotic sponges may not be as resilient to warming as previously hypothesised under future climate change conditions. Thus, subsurface warming due to oceanographic abnormalities warrants recognition as a genuine threat to deep reef habitats.
The intensity and frequency of pulsed warming events directly south and east of Tasmania are projected to increase greater than other temperate regions in Australia (Misra et al., 2021). This may not allow sufficient time for species to recover to a pre-disturbance state between warming pulses (Verdura et al., 2019). By 2060, the current assemblage of warmer species of mesophotic octocorals in southeast Australia may extend southward, whilst broadly and locally distributed sponge species particularly in south and east of Tasmania are less likely to persist (Marzloff et al., 2018). Range-extending herbivorous sea urchins that are affecting the shallow systems in southeast Australia presently (Johnson et al., 2011) may also extend to upper mesophotic depths (Perkins et al., 2015), potentially impacting sponge-dominated habitats that may have cascading consequences for benthic productivity (Bart et al., 2021).
The mechanisms of biological adaptation to repeated pulsed warming of temperate mesophotic species are currently unknown. Available evidence comes from the northern hemisphere showing that the temperate mesophotic habitat remained in a simplified state characterised by a high cover of turf-like mix of small invertebrates and algae two years after a pulsed warming event (Figure 4); although longer-term data will be required to resolve whether the phase shift is transient or permanent (Verdura et al., 2019). Although there is a lack of comparative data in temperate Australia, in the GBR the findings are nonetheless promising. They indicate that in this tropical mesophotic system, the structural complexity has been maintained, even amidst multiple weather stressor events (Hernandez-Agreda et al., 2021). This preservation of complexity is attributed to a shift in the hard coral assemblage, favouring branching and plating morphologies over massive, encrusting, and foliose-type species (Hernandez-Agreda et al., 2021). Monitoring of bleaching incidence in photosymbiotic sponges or signs of necrosis associated with heat-related opportunistic pathogens in deeper azooxanthellate species may be useful indicators for heat stress (Cerrano et al., 2000). Also, expanding long-term monitoring of fine-scale oceanographic parameters may help refine understanding of climate change impact hotspots.
3.6 Under-recognised threats from severe storms
Next to warming, the projected increase in the intensity and frequency of severe storms in temperate Australia from 40° S by 2081 may be another high-level stressor (Wandres et al., 2017) (Table 3). Mesophotic habitats were equally as vulnerable as the shallow water communities to the direct impacts of severe tropical storms and intense rainfall (Figure 4). Storm swells at 100 km from the reef and the path of Cyclone Yasi (category 5 on the Australian scale) were powerful enough to break off and overturn hard corals at 65 m deep on the GBR (Bongaerts et al., 2013). Hence, secondary to direct warming, impacts on warm temperate systems may be compounded by another large-scale warming-related stressor, as the paths of tropical severe storms are projected to shift poleward (Sharmila and Walsh, 2018).
Australian coastlines between latitudes of 40° and 50° S are exposed to one of the highest wave energies in the world (Hemer et al., 2017). This is due to persistent strong westerly winds over extremely long fetches across the Southern Ocean that are capable of generating more frequent and higher energy swells (wavelength of more than 200 m) than those in the northern hemisphere (Wang et al., 2016) or during destructive tropical cyclones (Tang et al., 1997). Significant wave heights of up to 10 m and wave periods of 15 s have been recorded on the southern coast of Australia during a storm in 2021 (Liu et al., 2022), which had sufficient energy to generate a bottom current velocity of 17 m.s-1 at 100 m depth. This was estimated to be 22 times higher than the recorded bottom current velocities from tropical cyclones (Tang et al., 1997).
Depending on the geomorphology of the seabed and sediment type, wave-exposed mesophotic systems may experience underwater turbulence that is high enough to displace infauna and small organisms, remove larger sessile invertebrates, and resuspend soft sediments which can smother encrusting filter feeding invertebrates and impair feeding efficiency of suspension feeders (Paavo et al., 2011; Larkin et al., 2021). In addition, the heterogeneity in the width of Australia’s continental shelf gives rise to regional differences in wave energy exposure (i.e., higher wave energy on a narrower shelf) (Seamap Australia, 2023). The anticipated increased frequency in pulse-type seabed disturbance may hinder the ecosystem from regaining its pre-disturbance equilibrium, perpetuating a persistent transient state of lower diversity assemblages consisting of small-bodied invertebrates and fast-growing species (Walker et al., 2008), a community assemblage similar to that found post pulse-warming events (Verdura et al., 2019). Selecting representative species (e.g., sponges that have predictable patterns of size and growth form shaped by a given level of wave energy exposure) may be a useful bioindicator for monitoring changes in hydrodynamics within different geographical regions (Schönberg, 2021).
The projected increase in extreme rainfall events, ~ 25% by 2081 under Representative Concentration Pathway 8.5, is yet another compounding stressor for southern Australia, (CSIRO & Bureau of Meteorology, 2015). Insights gleaned from tropical regions have shown extensive loss of seagrass meadows because of the intense rainfall accompanying destructive storms upon landfall. This loss is attributed to the large volume and high flow rate of terrestrial run-off, which transports sediments, nutrients, debris, and pollutants to shallow nearshore systems (Kendrick et al., 2019). Riverine food plumes can be transported some distance offshore, influenced by wind and Coriolis forcing, which has the potential to expose mid and outer-shelf marine ecosystems to terrestrial pollutants and silt resulting from poor land use (Devlin and Schaffelke, 2009). An example is the 2022-2023 Murray River flood event in southeastern Australia, brought about by persistent heavy rain. Through underwater imagery, it was observed that hypersaline silt originating from the Murray River extended as far as 80 km offshore (Mosley et al., 2023). This phenomenon was facilitated by a combination of gravity, current, and wind, which collectively contributed to the dispersal of the Murray River flood plume across the continental shelf seabed (Monk, J 2023, pers comm, 11 August 23).
Community composition in temperate mesophotic reefs has been recorded to shift towards turf-algae dominance as the abundance of structural forming invertebrates declined where flood plumes have extended to 35 m depth (Piazzi et al., 2021). The top-down sediment deposition associated with river plumes and rainfall events may also impact photosynthetic-dependent corals, sponges and macroalgae, and azooxanthellate passive suspension and filter feeders at upper mesophotic depths (Larkin et al., 2021), although investigation into species-specific response is warranted. As extreme rain events are likely to become more common under future climate projections (CSIRO & Bureau of Meteorology, 2015), mesophotic habitats in regions with high-flowing river systems (i.e., east and southeast Australia) may be at greater risk from top-down sedimentation. Drawing from lessons learned from the cascading impacts of chronic terrestrial nutrient input and coral reef degradation in the GBR (Kroon et al., 2016), anticipatory actions to manage water quality issues in light of this risk may be highly valuable.
4 Conclusion
Our review has outlined the vulnerability of sessile biota in mesophotic systems to various large-scale and local stressors that can, directly and indirectly, impact the ecosystem. Existing evidence on tropical and warm temperate mesophotic systems is largely centred on the characterisation and reproductive potential for mesophotic hard coral species to reseed shallow water populations to preserve reef complexity (Figure 1). A significant knowledge gap exists in whether temperate mesophotic reefs have similar ecosystem functioning as their tropical counterparts because temperate mesophotic assemblages are often distinct from temperate shallow water and tropical mesophotic communities. Regardless, mesophotic communities in both regions are susceptible to weather and localised pressures but their responses to threats vary between taxa and habitat types and are likely to depend on factors such as type, magnitude and duration of disturbance and rate of occurrence, as well as the combination of pressures.
To date, it appears there is little evidence indicating that depth per se can effectively serve as a physical barrier against pulsed warming events, severe storms, or intense rainfall, especially in the case of temperate mesophotic systems. Consequently, it appears that the temperate mesophotic reef habitats in Australia are equally, if not more, susceptible to significant weather disturbances compared to tropical mesophotic systems. Unlike some temperate regions elsewhere in the world, the deep shelf soft-sediment benthic communities in temperate Australia are suggested to be typically less impacted by trawling or anchoring activities at present (although these observations are backed by few empirical studies, and more are needed). Nonetheless, the predicted escalation of severe storm surges under future global warming scenarios has the potential to increase sediment resuspension from the bottom-up, while also exacerbating localised top-down sediment load from land-based runoff. However, following disturbance, existing evidence suggests that soft-sediment populations are likely to recover faster than mixed sediment or reef habitats (Figure 3). Overall, though, the potential impacts of multiple stressors on soft-sediment communities need to be better assessed.
In the context of climate change, Australia’s temperate mesophotic reef habitats are likely to experience the greatest impacts from pulsed warming and severe storms in the immediate future. The accelerated rate of warming and pulsed warming events in southeast of Australia are a cause for concern and pose an urgent risk. Based on existing knowledge, evidence of depth refuge from weather disturbance is very limited, but temperate mesophotic sponges may be more resilient than other sessile habitat formers to pulsed warming. Currently, we cannot infer the consequence of pulsed warming events and long-term warming for Australia’s temperate mesophotic systems from existing knowledge because of the heterogeneity in community assemblages. Moreover, the speed of warming is likely to cause the continual reshuffling of assemblages and novel interactions will arise with unpredictable ecological impacts. This points to a pressing need for research to focus on better understanding natural variability, species interactions, adaptation to local conditions and phenotypic plasticity to identify pathways for increasing resilience to warming. It will require tailored monitoring programs to track population and community trends and develop site-specific adaptive management solutions. These monitoring datasets should be collected from multiple agencies and cover sufficient temporal and spatial scales, like the monitoring programs on the GBR. Finally, identifying potential climate and human pressure hotspots and refugia habitats at multiple scales can improve the positioning of current and new MPAs that have full protection and enhance the clarity of existing management plans in terms of adaptive strategies and actions taken to reduce climate change impacts.
Author contributions
RW: Conceptualization, Data curation, Formal Analysis, Writing – original draft. JM: Supervision, Writing – review & editing. NP: Supervision, Writing – review & editing. NB: Supervision, Writing – review & editing.
Funding
The author(s) declare that no financial support was received for the research, authorship, and/or publication of this article.
Conflict of interest
The authors declare that the research was conducted in the absence of any commercial or financial relationships that could be construed as a potential conflict of interest.
Publisher’s note
All claims expressed in this article are solely those of the authors and do not necessarily represent those of their affiliated organizations, or those of the publisher, the editors and the reviewers. Any product that may be evaluated in this article, or claim that may be made by its manufacturer, is not guaranteed or endorsed by the publisher.
Supplementary material
The Supplementary Material for this article can be found online at: https://www.frontiersin.org/articles/10.3389/fmars.2023.1276072/full#supplementary-material
References
Albrecht R., Cook C. N., Andrews O., Roberts K. E., Taylor M. F. J., Mascia M. B., et al. (2021). Protected area downgrading, downsizing, and degazettement (PADDD) in marine protected areas. Mar. Policy 129, 104437. doi: 10.1016/j.marpol.2021.104437
Amoroso R. O., Pitcher C. R., Rijnsdorp A. D., McConnaughey R. A., Parma A. M., Suuronen P., et al. (2018). Bottom trawl fishing footprints on the world’s continental shelves. Proc. Natl. Acad. Sci. 115, E10275–E10282. doi: 10.1073/pnas.1802379115
Armstrong R. A., Pizarro O., Roman C. (2019). “Underwater Robotic Technology for Imaging Mesophotic Coral Ecosystems,” in Mesophotic Coral Ecosystems. Eds. Loya Y., Puglise K. A., et al (Cham: Springer International Publishing), pp. 973–988.
Australian Marine Parks (2023) Management plans for marine parks. Available at: https://parksAustralia.gov.au/marine/management/plans/.
Barrett N., Bates A., Beger M., Syms C., Holbrook N., Knott N., et al. (2014). Adaptive management of temperate reefs to minimise effects of climate change: Developing new effective approaches for ecological monitoring and predictive modelling (Australia: Hobart).
Bart M. C., Hudspith M., Rapp H. T., Verdonschot P. F. M., de Goeij J. M. (2021). A deep-sea sponge loop? Sponges transfer dissolved and particulate organic carbon and nitrogen to associated fauna. Front. Mar. Sci. 8. doi: 10.3389/fmars.2021.604879
Beger M., Sommer B., Harrison P. L., Smith S. D. A., Pandolfi J. M. (2014). Conserving potential coral reef refuges at high latitudes. Diversity Distributions 20, 245–257. doi: 10.1111/ddi.12140
Bell J. J., Bennett H. M., Rovellini A., Webster N. S. (2018). Sponges to be winners under near-future climate scenarios. BioScience 68, 955–968. doi: 10.1093/biosci/biy142
Bell J. J., Micaroni V., Harris B., Strano F., Broadribb M., Rogers A. (2022). Global status, impacts, and management of rocky temperate mesophotic ecosystems. Conserv. Biol., e13945. doi: 10.1111/cobi.13945
Bennett S., Wernberg T., Connell S. D., Hobday A. J., Johnson C. R., Poloczanska E. S. (2015). The ‘Great Southern Reef’: social, ecological and economic value of Australia’s neglected kelp forests. Mar. Freshw. Res. 67, 47–56. doi: 10.1071/MF15232
Bhatia K., Baker A., Yang W., Vecchi G., Knutson T., Murakami H. (2022). A potential explanation for the global increase in tropical cyclone rapid intensification. Nat. Commun. 13, 6626. doi: 10.1038/s41467-022-34321-6
Bongaerts P., Muir P., Englebert N., Bridge T. C. L., Hoegh-Guldberg O. (2013). Cyclone damage at mesophotic depths on Myrmidon Reef (GBR). Coral Reefs 32, 935–935. doi: 10.1007/s00338-013-1052-y
Bongaerts P., Ridgway T., Sampayo E. M., Hoegh-Guldberg O. (2010). Assessing the ‘deep reef refugia’ hypothesis: focus on Caribbean reefs. Coral Reefs 29, 309–327. doi: 10.1007/s00338-009-0581-x
Broad A., Rees M. J., Davis A. R. (2020). Anchor and chain scour as disturbance agents in benthic environments: trends in the literature and charting a course to more sustainable boating and shipping. Mar. pollut. Bull. 161, 111683. doi: 10.1016/j.marpolbul.2020.111683
Castellan G., Angeletti L., Montagna P., Taviani M. (2022). Drawing the borders of the mesophotic zone of the Mediterranean Sea using satellite data. Sci. Rep. 12, 5585. doi: 10.1038/s41598-022-09413-4
Cerrano C., Bavestrello G., Bianchi C. N., Cattaneo-vietti R., Bava S., Morganti C., et al. (2000). A catastrophic mass-mortality episode of gorgonians and other organisms in the Ligurian Sea (North-western Mediterranean), summer 1999. Ecol. Lett. 3, 284–293. doi: 10.1046/j.1461-0248.2000.00152.x
Cerrano C., Magnino G., Sarà A., Bavestrello G., Gaino E. (2001). Necrosis in a population of Petrosia ficiformis (Porifera, Demospongiae) in relation with environmental stress. Ital. J. Zoology 68, 131–136. doi: 10.1080/11250000109356397
Cetina-Heredia P., Roughan M., van Sebille E., Coleman M. A. (2014). Long-term trends in the East Australian Current separation latitude and eddy driven transport. J. Geophysical Research: Oceans 119, 4351–4366. doi: 10.1002/2014JC010071
Clark M. R., Althaus F., Schlacher T. A., Williams A., Bowden D. A., Rowden A. A. (2015). The impacts of deep-sea fisheries on benthic communities: a review. ICES J. Mar. Sci. 73, i51–i69. doi: 10.1093/icesjms/fsv123
Clark M. R., Bowden D. A., Rowden A. A., Stewart R. (2019). Little evidence of benthic community resilience to bottom trawling on seamounts after 15 years. Front. Mar. Sci. 6. doi: 10.3389/fmars.2019.00063
Clark M. R., Rowden A. A. (2009). Effect of deepwater trawling on the macro-invertebrate assemblages of seamounts on the Chatham Rise, New Zealand. Deep Sea Res. Part I: Oceanographic Res. Papers 56, 1540–1554. doi: 10.1016/j.dsr.2009.04.015
Coleman R. A., Hoskin M. G., von Carlshausen E., Davis C. M. (2013). Using a no-take zone to assess the impacts of fishing: Sessile epifauna appear insensitive to environmental disturbances from commercial potting. J. Exp. Mar. Biol. Ecol. 440, 100–107. doi: 10.1016/j.jembe.2012.12.005
Collie J. S., Hall S. J., Kaiser M. J., Poiner I. R. (2000). A quantitative analysis of fishing impacts on shelf-sea benthos. J. Anim. Ecol. 69, 785–798. doi: 10.1046/j.1365-2656.2000.00434.x
Commonwealth of Australia (2018). Threat Abatement Plan for the impacts of marine debris on the vertebrate wildlife of Australia’s coasts and oceans. (Commonwealth of Australia, Canberra, Australia).
Commonwealth of Australia (2021). Reef 2050 long-Term Sustainability Plan 2021-2025 (Canberra, Australia: Department of Agriculture, Water and the Environment).
Commonwealth of Australia (2016). Trends: transport and Australia’s development to 2040 and beyond. (Canberra, Australia: Commonwealth of Australia). Available at: https://www.infrastructure.gov.au/sites/default/files/migrated/infrastructure/publications/files/Trends_to_2040.pdf.
Consoli P., Romeo T., Angiolillo M., Canese S., Esposito V., Salvati E., et al. (2019). Marine litter from fishery activities in the Western Mediterranean sea: The impact of entanglement on marine animal forests. Environ. pollut. 249, 472–481. doi: 10.1016/j.envpol.2019.03.072
Corinaldesi C., Varrella S., Tangherlini M., Dell'Anno A., Canensi S., Cerrano C., et al. (2022). Changes in coral forest microbiomes predict the impact of marine heatwaves on habitat-forming species down to mesophotic depths. Sci. Total Environ. 823, 153701. doi: 10.1016/j.scitotenv.2022.153701
Creed J. C., Amado Filho G. M. (1999). Disturbance and recovery of the macroflora of a seagrass (Halodule wrightii Ascherson) meadow in the Abrolhos Marine National Park, Brazil: an experimental evaluation of anchor damage. J. Exp. Mar. Biol. Ecol. 235, 285–306. doi: 10.1016/S0022-0981(98)00188-9
CSIRO & Bureau of Meteorology (2015). Climate Change in Australia information for Australia’s Natural Resource Management Regions: Technical Report. Eds. Marie Ekström C. G., Grose M., Bhend J., Webb L., Risbey J. (Canberra, Australia: Commonwealth Science Industrial Research Organisation and Bureau of Meteorology Australia).
Davis A. R., Broad A., Gullett W., Reveley J., Steele C., Schofield C. (2016). Anchors away? The impacts of anchor scour by ocean-going vessels and potential response options. Mar. Policy 73, 1–7. doi: 10.1016/j.marpol.2016.07.021
Davis A. R., Broad A., Small M., Oxenford H. A., Morris B., Ingleton T. C. (2022). Mapping of benthic ecosystems: Key to improving the management and sustainability of anchoring practices for ocean-going vessels. Continental Shelf Res. 247, 104834. doi: 10.1016/j.csr.2022.104834
De’ath G., Fabricius K. E., Sweatman H., Puotinen M. (2012). The 27–year decline of coral cover on the Great Barrier Reef and its causes. Proc. Natl. Acad. Sci. 109, 17995–17999. doi: 10.1073/pnas.1208909109
De Goeij J. M., Van Oevelen D., van Oevelen D., Vermeij M. J. A., Osinga R., Middelburg J. J., de Goeij A. F. P. M., et al. (2013). Surviving in a marine desert: the sponge loop retains resources within coral reefs. Sci. (New York N.Y.) 342, 108–110. doi: 10.1126/science.1241981
Devlin M., Schaffelke B. (2009). Spatial extent of riverine flood plumes and exposure of marine ecosystems in the Tully coastal region, Great Barrier Reef. Mar. Freshw. Res. - Mar. Freshw. Res. 60, 1109–1122. doi: 10.1071/MF08343
Dietze M. C., Fox A., Beck-Johnson L. M., Betancourt J. L., Hooten M. B., Jarnevich C. S., et al. (2018). Iterative near-term ecological forecasting: Needs, opportunities, and challenges. Proc. Natl. Acad. Sci. 115, 1424–1432. doi: 10.1073/pnas.1710231115
Dubecki L. (2020) A coalition of research, government and industry partners is working to bring invasive sea urchins under control in Tasmania, with a mix of biological and market solutions. Available at: https://www.frdc.com.au/fish-vol-28-2/multi-pronged-strategy-targets-invasive-urchins.
Evans K., Bax N. J., Smith D. C. (2016). “Marine environment: Marine regions,” in Australia state of the environment 2016 (Canberra: Energy, A. G. D. o. t. E. a).
Eyal G., Laverick J. H., Ben-Zvi O., Brown K. T., Kramer N., Tamir R., et al. (2022). Selective deep water coral bleaching occurs through depth isolation. Sci. Total Environ. 844. doi: 10.1016/j.scitotenv.2022.157180
Feingold J. (2001). Responses of three coral communities to the 1997-98 El Niño-Southern Oscillation: Galápagos Islands, Ecuador. Bull. Mar. Sci. 69, 61–77.
Forrester G. E., Flynn R. L., Forrester L. M., Jarecki L. L. (2016). Episodic Disturbance from Boat Anchoring Is a Major Contributor to, but Does Not Alter the Trajectory of, Long-Term Coral Reef Decline. PloS One 10, e0144498. doi: 10.1371/journal.pone.0144498
Frade P. R., Bongaerts P., Englebert N., Rogers A., Gonzalez-Rivero M., Hoegh-Guldberg O. (2018). Deep reefs of the Great Barrier Reef offer limited thermal refuge during mass coral bleaching. Nat. Commun. 9, 1–8. doi: 10.1038/s41467-018-05741-0
Gall S. C., Rodwell L. D., Clark S., Robbins T., Attrill M. J., Holmes L. A., et al. (2020). The impact of potting for crustaceans on temperate rocky reef habitats: Implications for management. Mar. Environ. Res. 162, 105134. doi: 10.1016/j.marenvres.2020.105134
Garrabou J., Coma R., Bensoussan N., Bally M., Chevaldonne P., Cigliano M., et al. (2009). Mass mortality in Northwestern Mediterranean rocky benthic communities: effects of the 2003 heat wave. Global Change Biol. 15, 1090–1103. doi: 10.1111/j.1365-2486.2008.01823.x
Gili J.-M., Coma R. (1998). Benthic suspension feeders: their paramount role in littoral marine food webs. Trends Ecol. Evol. 13, 316–321. doi: 10.1016/S0169-5347(98)01365-2
Giraldo-Ospina A., Kendrick G. A., Hovey R. K. (2020). Depth moderates loss of marine foundation species after an extreme marine heatwave: could deep temperate reefs act as a refuge? Proc. R. Soc. B-Biological Sci. 287. doi: 10.1098/rspb.2020.0709
Gissi E., Manea E., Mazaris A. D., Fraschetti S., Almpanidou V., Bevilacqua S., et al. (2021). A review of the combined effects of climate change and other local human stressors on the marine environment. Sci. total Environ. 755, 142564. doi: 10.1016/j.scitotenv.2020.142564
Goodman A. J., Mcintyre J., Smith A., Fulton L., Walker T. R., Brown C. J. (2021). Retrieval of abandoned, lost, and discarded fishing gear in Southwest Nova Scotia, Canada: Preliminary environmental and economic impacts to the commercial lobster industry. Mar. pollut. Bull. 171, 112766. doi: 10.1016/j.marpolbul.2021.112766
Gori A., Bavestrello G., Grinyó J., Dominguez-Carrió C., Ambroso S., Bo M. (2017). Animal forests in deep coastal bottoms and continental shelf of the Mediterranean Sea. Mar. Anim. Forests: Ecol. benthic biodiversity hotspots, 207–233. doi: 10.1007/978-3-319-21012-4_5
Great Barrier Reef Marine Park Authority (2023) Reef 2050 Integrated Monitoring and Reporting Program. Available at: https://reefknowledgesystem.gbrmpa.gov.au/about/reef-2050-integrated-monitoring-and-reporting-program (Accessed 01 July 2023).
Guihen D., White M., Lundälv T. (2012). Temperature shocks and ecological implications at a cold-water coral reef. Mar. Biodiversity Records 5, e68. doi: 10.1017/S1755267212000413
Halpern B. S., Frazier M., Afflerbach J., Lowndes J.S., Micheli F., O’Hara C., et al. (2019). Recent pace of change in human impact on the world’s ocean. Sci. Rep. 9, 11609. doi: 10.1038/s41598-019-47201-9
Halpern B. S., Walbridge S., Selkoe K. A., Kappel C. V., Micheli F., D'Agrosa C., et al. (2008). A global map of human impact on marine ecosystems. Science 319, 948–952. doi: 10.1126/science.1149345
Hayes K. R., Dunstan P., Woolley S., Barrett N., Howe S. A., Samson C. R., et al. (2021). Designing a targeted Monitoring Program to Support Evidence Based Management of Australian Marine Parks: A Pilot on the South-East Marine Parks Network. Report to Parks Australia and the National Environmental Science Program. Marine Biodiversity Hub (Hobart, Australia: Parks Australia, University of Tasmanian and CSIRO).
Hedge P., Souter D., Jordan A., Trebilco R., Ward T., van Ruth P., et al. (2022). Establishing and supporting a national marine baselines and monitoring program: Advice from the Marine Baselines and Monitoring Working Group (Australia: Prepared for Australia’s National Marine Science Committee).
Hemer M. A., Zieger S., Durrant T., O'Grady J., Hoeke R. K., McInnes K. L., et al. (2017). A revised assessment of Australia’s national wave energy resource. Renewable Energy 114, 85–107. doi: 10.1016/j.renene.2016.08.039
Hemming M. P., Roughan M., Malan N., Schaeffer A. (2023). Observed multi-decadal trends in subsurface temperature adjacent to the East Australian Current. Ocean Sci. 19, 1145–1162. doi: 10.5194/os-19-1145-2023
Hernandez-Agreda A., Sahit F., Englebert N., Hoegh-Guldberg O., Bongaerts P. (2022). Hidden in the deep: distinct benthic trajectories call for monitoring of mesophotic reefs. Conserv. Lett. 15, 454664. doi: 10.1111/conl.12875
Hiddink J. G., Jennings S., Sciberras M., Szostek C.L., Hughes K.M., Ellis N., et al. (2017). Global analysis of depletion and recovery of seabed biota after bottom trawling disturbance. Proc. Natl. Acad. Sci. 114, 8301–8306. doi: 10.1073/pnas.1618858114
Hinderstein L. M., Marr J. C. A., Martinez F. A., Dowgiallo M. J., Puglise K. A., Pyle R. L., et al. (2010). Theme section on “Mesophotic coral ecosystems: characterization, ecology, and management”. Coral Reefs 29, 247–251. doi: 10.1007/s00338-010-0614-5
Jackson J. M., Johnson G. C., Dosser H. V., Ross T. (2018). Warming from recent marine heatwave lingers in deep british columbia fjord. Geophysical Res. Lett. 45, 9757–9764. doi: 10.1029/2018GL078971
James L. C., Marzloff M. P., Barrett N., Friedman A., Johnson C. R. (2017). Changes in deep reef benthic community composition across a latitudinal and environmental gradient in temperate Eastern Australia. Mar. Ecol. Prog. Ser. 565, 35–52. doi: 10.3354/meps11989
Johnson C. R., Banks S. C., Barrett N. S., Cazassus F., Dunstan P. K., Edgar G. J., et al. (2011). Climate change cascades: Shifts in oceanography, species’ ranges and subtidal marine community dynamics in eastern Tasmania. J. Exp. Mar. Biol. Ecol. 400, 17–32. doi: 10.1016/j.jembe.2011.02.032
Jones K. R., Klein C. J., Halpern B. S., Venter O., Grantham H., Kuempel C. D., et al. (2018). The location and protection status of earth’s diminishing marine wilderness. Curr. Biol. 28, 2506–2512.e3. doi: 10.1016/j.cub.2018.06.010
Kahng S. E., Wagner D., Lantz C, Vetter O, Gove J., Merrifield M. (2012). “Temperature related depth limits of warm-water corals. 9C Ecology of mesophotic coral reefs 2012 Cairns, Australia,” in Proceedings of the 12th International Coral Reef Symposium. Cairns, Australia.
Kaiser M. J., Clarke K. R., Hinz H., Austen M. C. V., Somerfield P. J., Karakassis I. (2006). Global analysis of response and recovery of benthic biota to fishing. Mar. Ecol. Prog. Ser. 311, 1–14. doi: 10.3354/meps311001
Kajtar J. B., Holbrook N. J., Hernaman V. (2021). A catalogue of marine heatwave metrics and trends for the Australian region. J. South. Hemisphere Earth Syst. Sci. 71, 284–302. doi: 10.1071/ES21014
Kelmo F., Bell J. J., Attrill M. J. (2013). Tolerance of sponge assemblages to temperature anomalies: resilience and proliferation of sponges following the 1997-8 el-nino southern oscillation. PloS One 8. doi: 10.1371/journal.pone.0076441
Kendrick G. A., Nowicki R. J., Olsen Y. S., Strydom S., Fraser M. W., Sinclair E. A., et al. (2019). A systematic review of how multiple stressors from an extreme event drove ecosystem-wide loss of resilience in an iconic seagrass community. Front. Mar. Sci. 6. doi: 10.3389/fmars.2019.00455
Kroon F. J., Thorburn P., Schaffelke B., Whitten S. (2016). Towards protecting the Great Barrier Reef from land-based pollution. Global Change Biol. 22, 1985–2002. doi: 10.1111/gcb.13262
Kutti T., Bannister R. J., Fosså J. H., Krogness C. M., Tjensvoll I., Søvik G. (2015). Metabolic responses of the deep-water sponge Geodia barretti to suspended bottom sediment, simulated mine tailings and drill cuttings. J. Exp. Mar. Biol. Ecol. 473, 64–72. doi: 10.1016/j.jembe.2015.07.017
Larkin M. F., Davis T. R., Harasti D., Cadiou G., Poulos D. E., Smith S. D. A. (2021). The rapid decline of an Endangered temperate soft coral species. Estuarine Coast. Shelf Sci. 255, 107364. doi: 10.1016/j.ecss.2021.107364
Laverick J. H., Piango S., Andradi-Brown D. A., Exton D. A., Bongaerts P., Bridge T. C. L., et al. (2018). To what extent do mesophotic coral ecosystems and shallow reefs share species of conservation interest? A systematic review. Environ. Evidence 7, 15. doi: 10.1186/s13750-018-0127-1
Leverington A., Hockings M., Leverington F., Trinder C., Polglaze J. (2019). Independent assessment of management Effectiveness for the Great Barrier Reef Outlook Report 2019 (Australia: Townville).
Lewis C. F., Slade S. L., Maxwell K. E., Matthews T. R. (2009). Lobster trap impact on coral reefs: Effects of wind-driven trap movement. New Z. J. Mar. Freshw. Res. 43, 271–282. doi: 10.1080/00288330909510000
Lindegarth M., Valentinsson D., Hansson M., Ulmestrand M. (2000). Interpreting large-scale experiments on effects of trawling on benthic fauna: an empirical test of the potential effects of spatial confounding in experiments without replicated control and trawled areas. J. Exp. Mar. Biol. Ecol. 245, 155–169. doi: 10.1016/S0022-0981(99)00158-6
Liu J., Meucci A., Liu Q., Babanin A. V., Ierodiaconou D., Young I. R. (2022). The wave climate of Bass Strait and South-East Australia. Ocean Model. 172, 101980. doi: 10.1016/j.ocemod.2022.101980
Marzloff M. P., Oliver E. C. J., Barrett N. S., Holbrook N. J., James L., Wotherspoon S. J., et al. (2018). Differential vulnerability to climate change yields novel deep-reef communities. Nat. Climate Change 8, 873–87+. doi: 10.1038/s41558-018-0278-7
Mauro A. A., Shah A. A., Martin P. R., Ghalambor C. K. (2022). An integrative perspective on the mechanistic basis of context- dependent species interactions. Integr. Comp. Biol. 62, 164–178. doi: 10.1093/icb/icac055
Mclean M., Mouillot D., Maureaud A. A., Hattab T., MacNeil M. A., Goberville E., et al. (2021). Disentangling tropicalization and deborealization in marine ecosystems under climate change. Curr. Biol. 31, 4817–4823.e5. doi: 10.1016/j.cub.2021.08.034
Micaroni V., Mcallen R., Turner J., Strano F., Morrow C., Picton B., et al. (2021). Vulnerability of Temperate Mesophotic Ecosystems (TMEs) to environmental impacts: Rapid ecosystem changes at Lough Hyne Marine Nature Reserve, Ireland. Sci. Total Environ. 789. doi: 10.1016/j.scitotenv.2021.147708
Miller M. W., Piniak G. A., Williams D. E. (2011). Coral mass bleaching and reef temperatures at Navassa Islan. Estuar. Coast. Shelf Sci. 91, 42–50. doi: 10.1016/j.ecss.2010.10.005
Misra R., Sérazin G., Meissner K. J., Sen Gupta A. (2021). Projected changes to Australian marine heatwaves. Geophysical Res. Lett. 48, e2020GL091323. doi: 10.1029/2020GL091323
Morrison K. M., Meyer H. K., Roberts E. M., Rapp H. T., Colaço A., Pham C. K. (2020). The first cut is the deepest: trawl effects on a deep-sea sponge ground are pronounced four years on. Front. Mar. Sci. 7. doi: 10.3389/fmars.2020.605281
Mosley L. M., Priestley S., Brookes J., Dittmann S., Farkaš J., Farrell M., et al. (2023). Extreme eutrophication and salinisation in the Coorong estuarine-lagoon ecosystem of Australia’s largest river basin (Murray-Darling). Mar. Pollut. Bull. 188, 114648. doi: 10.1016/j.marpolbul.2023.114648
Mulochau T., Lelabousse C., Séré M. (2020). Estimations of densities of marine litter on the fringing reefs of Mayotte (France – South Western Indian Ocean) - impacts on coral communities. Mar. pollut. Bull. 160, 111643. doi: 10.1016/j.marpolbul.2020.111643
Oksanen F. J., Blanchet F. G., Kindt R., Legendre P., Minchin P. R., O’Hara R. B., et al. (2017). Vegan: community ecology package. R package Version 2.6-4.
Oliver E. C. J., Benthuysen J. A., Bindoff N. L., Hobday A .J., Holbrook N. J., Mundy C. N., et al. (2017). The unprecedented 2015/16 Tasman Sea marine heatwave. Nat. Commun. 8, 16101. doi: 10.1038/ncomms16101
Oliver E. C. J., Lago V., Hobday A. J., Holbrook N. J., Ling S. D., Mundy C. N. (2018). Marine heatwaves off eastern Tasmania: Trends, interannual variability, and predictability. Prog. Oceanography 161, 116–130. doi: 10.1016/j.pocean.2018.02.007
Paavo B., Jonker R., Thrush S., Probert P. K. (2011). Macrofaunal community patterns of adjacent coastal sediments with wave-reflecting or wave-dissipating characteristics. J. Coast. Res. 27, 515–528. doi: 10.2112/JCOASTRES-D-10-00001.1
Page M. J., Mckenzie J. E., Bossuyt P. M., Boutron I., Hoffmann T. C., Mulrow C. D., et al. (2021). The PRISMA 2020 statement: an updated guideline for reporting systematic reviews. BMJ 372, n71. doi: 10.1136/bmj.n71
Pérez-Castro M.Á., Schubert N., Ang-Montes de Oca G., Leyte-Morales G. E., Eyal G., Hinojosa-Arango G. (2022). Mesophotic Coral Ecosystems in the Eastern Tropical Pacific: The current state of knowledge and the spatial variability of their depth boundaries. Sci. Total Environ. 806, 150576. doi: 10.1016/j.scitotenv.2021.150576
Perkins N. R., Hill N. A., Foster S. D., Barrett N. S. (2015). Altered niche of an ecologically significant urchin species, Centrostephanus rodgersii, in its extended range revealed using an Autonomous Underwater Vehicle. Estuarine Coast. Shelf Sci. 155, 56–65. doi: 10.1016/j.ecss.2015.01.014
Perkins N. R., Monk J., Soler G., Gallagher P., Barrett N. S. (2022). Bleaching in sponges on temperate mesophotic reefs observed following marine heatwave events. Climate Change Ecol. 3, 100046. doi: 10.1016/j.ecochg.2021.100046
Phillips L. R., Malan N., Roughan M., Harcourt R., Jonsen I., Cox M., et al. (2022). Coastal seascape variability in the intensifying East Australian Current Southern Extension. Front. Mar. Sci. 9. doi: 10.3389/fmars.2022.925123
Piazzi L., Cecchi E., Cinti M. F., Ceccherelli G. (2021). Extreme events and conservation of subtidal habitats: Effects of a rainfall flood on coralligenous reefs. Mar. pollut. Bull. 165, 112106. doi: 10.1016/j.marpolbul.2021.112106
Pinsky M. L., Selden R. L., Kitchel Z. J. (2020). Climate-driven shifts in marine species ranges: scaling from organisms to communities. Annu. Rev. Mar. Sci. 12, 153–179. doi: 10.1146/annurev-marine-010419-010916
Pitcher C. R., Rochester W., Dunning M., Courtney T., Broadhurst M., Noell C., et al. (2018). Putting potential environmental risk of Australia’s trawl fisheries in landscape perspective: exposure of seabed assemblages to trawling, and inclusion in closures and reserves — FRDC Project No 2016-039 (Brisbane: CSIRO Oceans & Atmosphere).
Poloczanska E. S., Brown C. J., Sydeman W. J., Kiessling W., Schoeman D. S., Moore P. J., et al. (2013). Global imprint of climate change on marine life. Nat. Climate Change 3, 919–925. doi: 10.1038/nclimate1958
Poloczanska E. S., Burrows M. T., Brown C.J., García Molinos J., Halpern B. S., Hoegh-Guldberg O., et al. (2016). Responses of marine organisms to climate change across oceans. Front. Mar. Sci. 3. doi: 10.3389/fmars.2016.00062
Porter S. N., Sink K. J., Schleyer M. H. (2021). The third global coral bleaching event on the marginal coral reefs of the southwestern Indian ocean and factors that contribute to their resistance and resilience. Diversity 13, 464. doi: 10.3390/d13100464
Schönberg C. H. L. (2021). No taxonomy needed: Sponge functional morphologies inform about environmental conditions. Ecol. Indic. 129, 107806. doi: 10.1016/j.ecolind.2021.107806
Sciberras M., Hiddink J. G., Jennings S., Szostek C. L., Hughes K. M., Kneafsey B., et al. (2018). Response of benthic fauna to experimental bottom fishing: A global meta-analysis. Fish Fisheries 19, 698–715. doi: 10.1111/faf.12283
Seamap Australia (2023) Seabed disturbance (wave/current). Available at: https://seamapAustralia.org/map/#ec0444de-b07b-4719-975f-632df5b2250c (Accessed 09 June 2023).
Sharmila S., Walsh K. J. E. (2018). Recent poleward shift of tropical cyclone formation linked to Hadley cell expansion. Nat. Climate Change 8, 730–736. doi: 10.1038/s41558-018-0227-5
Smale D. A., Wernberg T., Oliver E. C. J., Thomsen M., Harvey B. P., Straub S. C., et al. (2019). Marine heatwaves threaten global biodiversity and the provision of ecosystem services. Nat. Climate Change 9, 306–30+. doi: 10.1038/s41558-019-0412-1
Smith D. C., Fulton E. A., Apfel P., Cresswell I. D., Gillanders B. M., Haward M., et al. (2017). Implementing marine ecosystem-based management: lessons from Australia. ICES J. Mar. Sci. 74, 1990–2003. doi: 10.1093/icesjms/fsx113
Smith T. B., Gyory J., Brandt M. E., Miller W. J., Jossart J., Nemeth R. S. (2016). Caribbean mesophotic coral ecosystems are unlikely climate change refugia. Global Change Biol. 22, 2756–2765. doi: 10.1111/gcb.13175
Stefaniak L. M., Auster P. J., Babb I. G. (2014). Loss of an erect sponge on a rock reef in Long Island Sound (north-west Atlantic). Mar. Biodiversity Records 7, e115. doi: 10.1017/S1755267214001109
Stephenson F., Mill A. C., Scott C. L., Polunin N. V. C., Fitzsimmons C. (2017). Experimental potting impacts on common UK reef habitats in areas of high and low fishing pressure. Ices J. Mar. Sci. 74, 1648–1659. doi: 10.1093/icesjms/fsx013
Stevens B. G. (2020). The ups and downs of traps: environmental impacts, entanglement, mitigation, and the future of trap fishing for crustaceans and fish. ICES J. Mar. Sci. 78, 584–596. doi: 10.1093/icesjms/fsaa135
Tang Y. M., Holloway P., Holloway P., Grimshaw R. (1997). A numerical study of the storm surge generated by tropical cyclone jane. J. Phys. Oceanography 27, 963–976. doi: 10.1175/1520-0485(1997)027<0963:ANSOTS>2.0.CO;2
Trebilco R., Fischer M., Hunter C., Hobday A., Thomas L., Evans K. (2021). Australia state of the environment 2021: marine, independent report to the Australian Government Minister for the Environment (Canberra: Commonwealth of Australia).
Turner J. A., Babcock R. C., Hovey R., Kendrick G. A. (2017). Deep thinking: a systematic review of mesophotic coral ecosystems. Ices J. Mar. Sci. 74, 2309–2320. doi: 10.1093/icesjms/fsx085
Verdura J., Linares C., Ballesteros E., Coma R., Uriz M. J., Bensoussan N., et al. (2019). Biodiversity loss in a Mediterranean ecosystem due to an extreme warming event unveils the role of an engineering gorgonian species. Sci. Rep. 9. doi: 10.1038/s41598-019-41929-0
Verfuss U. K., Aniceto A. S., Harris D. V., Gillespie D., Fielding S., Jiménez G., et al. (2019). A review of unmanned vehicles for the detection and monitoring of marine fauna. Mar. pollut. Bull. 140, 17–29. doi: 10.1016/j.marpolbul.2019.01.009
Vergés A., Mccosker E., Mayer-Pinto M., Coleman M. A., Wernberg T., Ainsworth T., et al. (2019). Tropicalisation of temperate reefs: Implications for ecosystem functions and management actions. Funct. Ecol. 33, 1000–1013. doi: 10.1111/1365-2435.13310
Walker S. J., Degnan B. M., Hooper J. N. A., Skilleter G. A. (2008). Will increased storm disturbance affect the biodiversity of intertidal, nonscleractinian sessile fauna on coral reefs? Global Change Biol. 14, 2755–2770. doi: 10.1111/j.1365-2486.2008.01644.x
Wandres M., Pattiaratchi C., Hemer M. A. (2017). Projected changes of the southwest Australian wave climate under two atmospheric greenhouse gas concentration pathways. Ocean Model. 117, 70–87. doi: 10.1016/j.ocemod.2017.08.002
Wang X., Jiang H., Chen G., Yu F. (2016). Identifying storm-induced wave origins using SAR wave mode data. Sci. China Earth Sci. 59, 1971–1980. doi: 10.1007/s11430-015-5478-3
Warnock B., Harvey E. S., Newman S. J. (2016). Remote drifted and diver operated stereo–video systems: A comparison from tropical and temperate reef fish assemblages. J. Exp. Mar. Biol. Ecol. 478, 45–53. doi: 10.1016/j.jembe.2016.02.002
Watson S. J., Ribó M., Seabrook S., Strachan L. J., Hale R., Lamarche G. (2022). The footprint of ship anchoring on the seafloor. Sci. Rep. 12, 7500. doi: 10.1038/s41598-022-11627-5
Wernberg T., Thomsen M. S., Tuya F., Kendrick G. A. (2011). Biogenic habitat structure of seaweeds change along a latitudinal gradient in ocean temperature. J. Exp. Mar. Biol. Ecol. 400, 264–271. doi: 10.1016/j.jembe.2011.02.017
Westcott D. A., Fletcher C. S., Chades I. (2020). Strategies for the management of Centrostephanus rodgersii in Tasmanian Waters Australia: CSIRO. (Canberra, Australia: Commonwealth Science Industrial Research Organisation)
Williams A., Althaus F., Maguire K., Green M., Untiedt C., Alderslade P., et al. (2020). The fate of deep-sea coral reefs on seamounts in a fishery-seascape: what are the impacts, what remains, and what is protected? Front. Mar. Sci. 7. doi: 10.3389/fmars.2020.567002
Williams S. B., Pizarro O., Webster J. M., Beaman R. J., Mahon I., Johnson-Roberson M., et al. (2010). Autonomous underwater vehicle–assisted surveying of drowned reefs on the shelf edge of the Great Barrier Reef, Australia. J. Field Robotics 27, 675–697. doi: 10.1002/rob.20356
Witman J. D., Pershing A. J., Bruno J. F. (2023). Smooth and spiky: the importance of variability in marine climate change ecology. Annu. Rev. Ecology Evolution Systematics 54, 129–149. doi: 10.1146/annurev-ecolsys-022323-082123
Keywords: deep reef, ocean warming, pulsed warming, storms, seabed impacts, refugia, animal forest, high latitude
Citation: Wong RHX, Monk J, Perkins NR and Barrett NS (2023) A systematic review on the anthropogenic stressors on sessile benthic mesophotic reef communities: implications for temperate reef management in Australia. Front. Mar. Sci. 10:1276072. doi: 10.3389/fmars.2023.1276072
Received: 11 August 2023; Accepted: 27 November 2023;
Published: 13 December 2023.
Edited by:
Susana Carvalho, King Abdullah University of Science and Technology, Saudi ArabiaReviewed by:
Peter J. Auster, Mystic Aquarium, United StatesSandra Brooke, Florida State University, United States
Copyright © 2023 Wong, Monk, Perkins and Barrett. This is an open-access article distributed under the terms of the Creative Commons Attribution License (CC BY). The use, distribution or reproduction in other forums is permitted, provided the original author(s) and the copyright owner(s) are credited and that the original publication in this journal is cited, in accordance with accepted academic practice. No use, distribution or reproduction is permitted which does not comply with these terms.
*Correspondence: Rachel H. X. Wong, UmFjaGVsLndvbmdAdXRhcy5lZHUuYXU=