- 1Department for Marine Aquaculture, Institute of Animal Breeding and Husbandry, Kiel University, Kiel, Germany
- 2Fraunhofer Research Institution for Individualized and Cell-Based Medical Engineering IMTE, Aquaculture and Aquatic Resources, Büsum, Germany
- 3Department of Safety and Quality of Milk and Fish Products, Max Rubner-Institut, Kiel, Germany
- 4Fish Genetics Unit, Institute of Genome Biology, Research Institute for Farm Animal Biology (FBN), Dummerstorf, Germany
- 5Faculty of Agriculture and Environmental Sciences, University of Rostock, Rostock, Germany
- 6Institute of Toxicology and Pharmacology for Natural Scientists, University Medical School Schleswig-Holstein, Kiel, Germany
Microalgae are increasingly being investigated as functional feed additives in a variety of fish species, but our knowledge on how microalgae supplementation affects Atlantic salmon remains limited. We hypothesized that microalgae inclusion of 8% in the feed would improve performance, fatty acid and pigment deposition as well as health and immunity of Atlantic salmon reared in recirculating aquaculture systems (RAS). We fed Atlantic salmon smolts with five different microalgae enriched diets containing Tetraselmis chuii (TC), Arthrospira platensis (AP), Schizochytrium limacinum (SL) or Chlorella vulgaris, either intact (CVI) or as broken cell wall derivative (CVB) or a control diet (CD). After eight weeks of feeding in brackish water (13 psu), all groups were transferred to seawater (32 psu) for additional two weeks. Our results indicate that CVB improved feed conversion and protein retention, but reduced condition factor (p < 0.05) compared to fish fed with a control diet. Voluntary feed intake decreased in seawater, but was similar among diet groups. The amount of docosahexaenoic acid was particularly high in SL-fed fish and alpha-linolenic acid was enriched in fish fed CVI, CVB and TC (p < 0.05). Following seawater transfer, fat content and monounsaturated fatty acids decreased in the muscle, while polyunsaturated fatty acids increased. Lutein was present in all muscle samples, but highest concentrations were found in CVB-, CVI- and TC-fed fish. In the anterior intestine, microalgae supplementation induced differentially regulated trout protein 1 (drtp1) expression in CVI- and CVB-fed fish, but reduced the expression of interleukin 1 and 10 receptor (il1r2 & il10rb) in CVI-fed fish. In the liver, feeding CVI and SL induced complement C1q like 2 (c1ql2) expression, while reducing serum amyloid A5 (saa5) expression. Superoxide-dismutase protein concentration was induced in the liver of fish fed SL, while myeloperoxidase was reduced in most microalgae-fed groups. In conclusion, we show that commercially relevant microalgae can be used as functional feed additives for Atlantic salmon promoting different health aspects without negatively affecting their growth performance when cultivated in RAS.
1 Introduction
Feed in aquaculture is a finite resource, which provides nutrients but should also promote the growth and health of farmed fish. Consequently, interest is growing in developing functional feeds that guarantee good fish health, improve performance and mitigate farming related stressors. Although a variety of compounds have been investigated as functional ingredients in fish feed, microalgae have only recently been considered. These single cell algae contain different types of polysaccharides, sulfolipids, polyunsaturated fatty acids and pigments (Riccio and Lauritano, 2020). Green algae such as Chlorella sp. and Tetraselmis sp. are rich in pigments such as chlorophylls and carotenoids, while heterotrophic Schizochytrium (herein considered as microalgae) contain high amounts of docosahexaenoic acid (DHA; Nakahara et al., 1996; Ren et al., 2010).
The diverse chemical composition of microalgae holds potential for a variety of biological activities, including antioxidant (Carballo et al., 2018; Teimouri et al., 2019), antimicrobial (Guzmán et al., 2019) as well as anti-inflammatory activities (Fujii, 2000; Guzmán et al., 2003). Feeding microalgae to different fish species was found to affect their health and immunity. Including Chlorella vulgaris into the feed, for instance, counteracted soy-bean meal-induced intestinal inflammation in Atlantic salmon Salmo salar (Grammes et al., 2013) and zebrafish Danio rerio (Bravo-Tello et al., 2017). Dietary administration of Chlorella sorokiniana further stimulated humoral innate immunity in rainbow trout Oncorhynchus mykiss (Chen et al., 2021), while Tetraselmis chuii and Phaedactylum tricornutum increased phagocytotic and complement activity in gilthead seabream Sparus aurata (Cerezuela et al., 2012a).
Although health and immune promoting effects are described for different microalgae species, the majority of studies in fish has investigated microalgae as a source to replace fishmeal or fish oil in the feed (Carvalho et al., 2020; Kousoulaki et al., 2020; Sarker et al., 2020a). Studies evaluating the functional properties of different microalgae species under challenging environmental conditions are currently missing.
Such challenging environmental conditions can occur during the production of Atlantic salmon in recirculating aquaculture systems (RAS). Production of Atlantic salmon in RAS is globally expanding (Bergheim et al., 2009; Davidson et al., 2021). This is because these systems allow a controlled production environment with a high level of biosecurity and a significantly reduced discharge of waste products into the aquatic environment (Dalsgaard et al., 2013; Ahmed and Turchini, 2021). However, the RAS environment is considered particularly challenging for fish health due to higher stocking densities (Calabrese et al., 2017), accumulation of waste products (Ruyet et al., 2008), as well as water disinfection treatment (Soleng et al., 2019; Stiller et al., 2020). Dietary mitigation under these conditions may be a promising strategy to improve overall health and performance of salmon cultivated in RAS. Currently the use of a brackish water phase in RAS before seawater transfer is investigated as an alternative to shorten the production time in the sea (Ytrestøyl et al., 2020; Ytrestøyl et al., 2023). While it is well established that transfer to seawater of Atlantic salmon is associated with a drastic stress-related reduction in appetite (Usher et al., 1991), little is known about whether microalgae, which contain different amino and fatty acids, could potentially increase feed intake in this critical time period.
A thorough assessment of the fish’s health and immune status in functional feeding studies requires investigating both transfer of functional components from feed to fish and their subsequent effects in vivo. The former is usually performed using analytical chemistry to trace fatty acids, pigments and other functional compounds. The latter requires investigating different aspects of the fish’s health and immune status. Functional feeds are expected to provoke a local response in the intestine (Bae et al., 2020; López Nadal et al., 2020), but also systemic effects may occur. These are reflected in physiological alterations of the blood plasma, the spleen and the liver, being constantly exposed to antigens from the bloodstream (Bayne et al., 2001; Wu et al., 2016).
In this study, we aimed to elucidate whether functional diets enriched with different commercially relevant microalgae species at 8% inclusion in the feed can improve performance, health and immune status of Atlantic salmon reared in RAS. Since seawater transfer of salmon smolts is a particular critical time period following land-based rearing in RAS (Usher et al., 1991; Karlsen et al., 2018), we evaluated diet dependent effects during this time-period in addition. Alongside with performance indicators, fatty acid and pigment profiles, a set of putative biomarkers on gene and protein level in plasma, liver, intestine and spleen were used to evaluate the health and immune status of the salmon.
2 Materials and methods
2.1 Feed formulation
Six isonitrogenous and isoenergetic (on dry matter basis; see Table 1) experimental diets were formulated based on the nutrient requirements of Atlantic salmon (National Research Council, 2011). The diets were designed to include one of the following microalgae: Chlorella vulgaris, Tetraselmis chuii (TC), Arthrospira platensis (AP) or Schizochytrium limacinum (SL) at an inclusion level of 8%. Two different Chlorella vulgaris were included in the experimental design, one had an intact (CVI) and the other a broken cell wall (CVB). The microalgae were obtained from a commercial supplier and were cultivated in both open and closed bioreactors under commercial settings (Supplementary Table 1). All microalgae were spray-dried after harvesting. Inclusion of the microalgae was done in exchange for wheat starch, wheat gluten and canola oil. In contrast to other studies basal feed components (e.g. fish meal and fish oil) were kept constant in the diet formulation and hence allowed to evaluate the direct effect of every microalgae ingredient. The experimental diets were pelletized (Type 14U175, Amandus Kahl, Hamburg, Germany) at temperatures below 60°C to pellets with 4 mm diameter and stored at 4°C in the dark before and during the trial.
2.2 Experimental setup
The experiment was conducted at the facilities of the Fraunhofer IMTE, Büsum, Germany. Atlantic salmon smolts were obtained from Jurassic Salmon, Poland and were acclimated for two months in a recirculating aquaculture system. During acclimation, the fish were fed a commercial salmon diet (Aller Aqua, Denmark). Water treatment of the RAS (7.6 m3, turnover rate 4 times h−1) consisted of a moving bed biofilter, a bead filter (PolyGeyser, Model DF-6, Aquaculture Systems Technologies, L.L.C., New Orleans, LA, USA), a protein skimmer and UV-light disinfection. Water quality parameters were measured on a daily basis (NH4+ and NO2- biweekly) and kept in a suitable range for Atlantic salmon (13.5 ± 0.4°C, 7.3 ± 0.1 pH, 10.3 ± 0.2 mg/L O2, 0.2 ± 0.1 mg/L NH4+, 0.2 ± 0.04 mg/L NO2-, (Microquant test kit for NH4+ and NO2-, Merck, Darmstadt, Germany). Salinity was set to 13.0 ± 0.8 psu (HI 96822 Seawater Refractometer, Hanna Instruments Inc., Woonsocket-RI, USA) by mixing freshwater and seawater. Light was provided for 24 h throughout the experimental period. Prior to the start of the experiment Atlantic salmon smolts (mean body weight 82.32 ± 1.96 g) were randomly divided into six different groups in triplicate, each consisting of 28 fish and stocked into 18 tanks (300 L) of the RAS (Figure 1). Following rearing the fish for eight weeks in brackish water, all of the fish were transferred into a new RAS system, which was identical to the other, but operated with full strength seawater (salinity of 31.8 ± 0.5 psu). Water parameters for this system were as followed: 13.4 ± 0.3°C, 7.2 ± 0.1 pH, 10.4 ± 0.3 mg/L O2, 0.2 ± 0.1 mg/L NH4+, 0.2 ± 0.07 mg/L NO2-. The fish were kept under these conditions for additional two weeks before the experiment was terminated. The fish were fed manually twice per day (8 a.m. and 2 p.m.) until apparent satiation during the entire experiment. Leftover pellets were collected, counted and used to calculate feed intake.
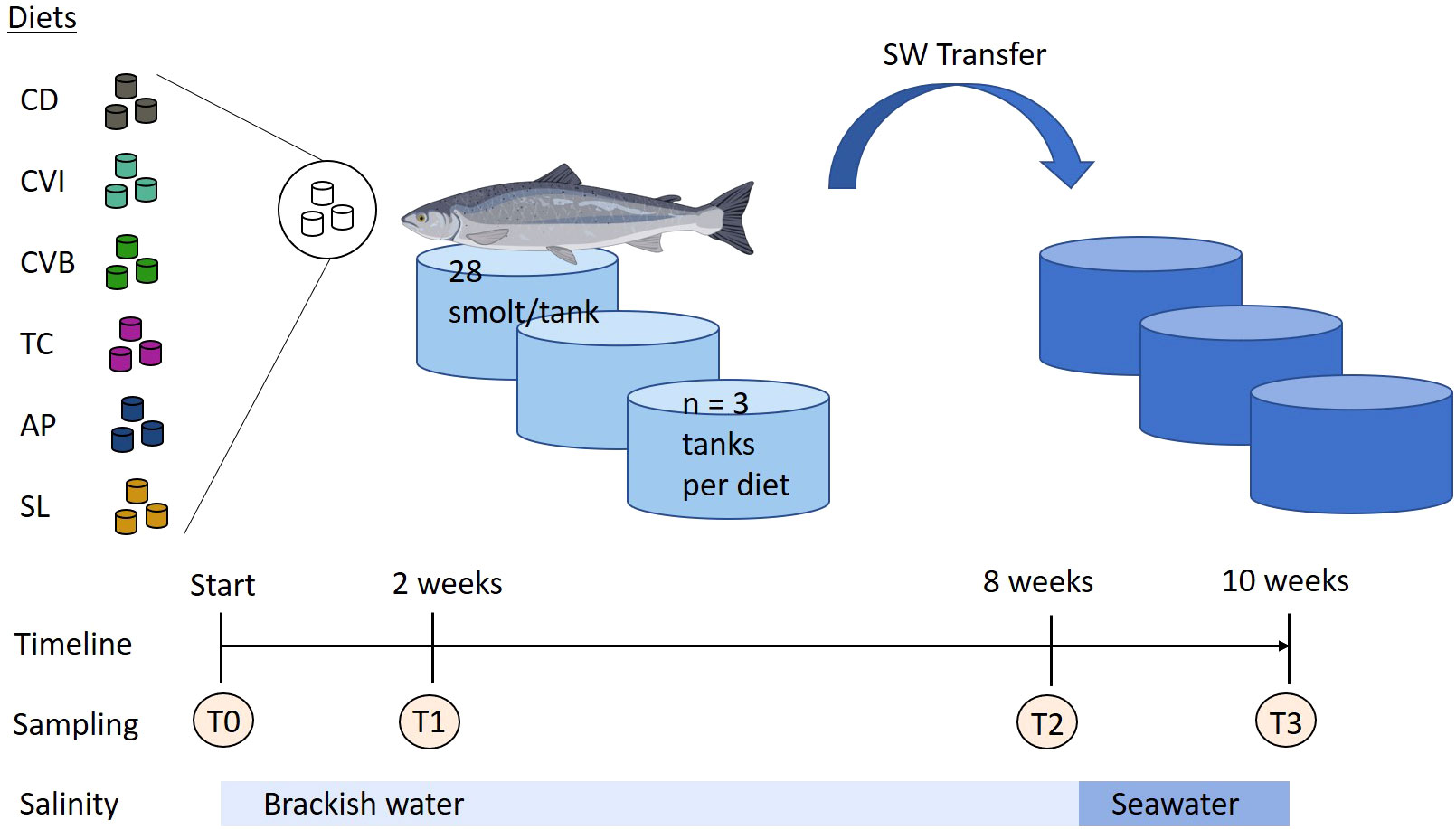
Figure 1 Experimental design of feeding trial with microalgae enriched diets. Atlantic salmon were first reared in brackish water and after eight weeks transferred to seawater (SW transfer) for additional two weeks. Throughout the trial the fish received six different experimental diets: control (CD), Chlorella vulgaris intact (CVI), Chlorella vulgaris broken (CVB), Tetraselmis chuii (TC), Arthrospira platensis (AP) and Schizochytrium limacinum (SL) at an inclusion level of 8%. Atlantic salmon image was created with BioRender.com.
2.3 Fish sampling
Samples were collected before the onset of the experiment (T0), after two weeks (T1) and eight weeks of feeding the experimental diets in brackish water (T2) and two weeks following transfer into seawater (T3; Figure 1). At each sampling nine fish per treatment (three per tank) were randomly sampled. The fish were quickly netted from the experimental tanks and euthanized by an overdose of buffered MS-222 (0.3 mg/L). For each fish total length and total weight was recorded. 2 ml of blood was collected in heparinized syringes by caudal vein puncture. The blood was transferred into 2 ml Eppendorf tubes and centrifuged at 4000 g for 8 min. Aliquots of the plasma were flash-frozen on dry ice and stored at −80°C for the determination of plasma metabolites, total carotenoid content, and enzyme activities.
The liver and spleen were carefully removed and weighed for the calculation of organ specific indices. At the end of the brackish water phase (T2) a piece of the liver, anterior intestine and the spleen was placed in an RNase free tube and flash frozen in liquid nitrogen for gene expression analysis. In addition, after two (T1) and eight weeks (T2) of feeding the experimental diets a piece of the liver was flash-frozen on dry ice for later protein analysis using western blots.
At T0, T2 and T3 both fillets from every fish were taken, de-skinned, homogenized by means of a knife-mill (Grindomix GM200, Retsch GmbH, Haan, Germany) and stored at −40°C for later analysis of proximate composition, fatty acid profile, as well as carotenoid content. At T0 and T2 three additional fish per tank were sampled and pooled for the analysis of whole-body proximate composition.
2.4 Proximate composition of whole body and diets
Proximate composition was analyzed in microalgae (Supplementary Table 2), diets (Table 2) and whole-body homogenates in duplicates using the same methods. Whole body samples were freeze-dried (Alpha 1-2 LD plus and Alpha 1-4 LSC, Martin Christ Gefriertrocknungsanlagen GmbH, Osterode am Harz, Germany) until a stable weight was achieved and homogenized using a knife mill (GM 200, Retsch GmbH). Nutrients and gross energy were analyzed according to EU guideline (EC) 152/2009. Dry matter content was determined following drying of samples at 103°C in a drying oven for 4 h (ED 53, Binder GmbH, Tuttlingen, Germany). Ash content was determined after combustion in a combustion oven at 550°C (P300, Nabertherm, Lilienthal, Germany). Crude protein content was analyzed following the Kjeldahl method (InKjel 1225M, WD30, Behr, Düsseldorf, Germany). Crude lipid content was extracted with petroleum ether in a Soxhlet extraction system (Soxtherm, Hydrotherm, Gerhardt Königswinter, Germany) and quantified gravimetrically. Gross energy was determined using a bomb calorimeter (C 200, IKA, Staufen, Germany).
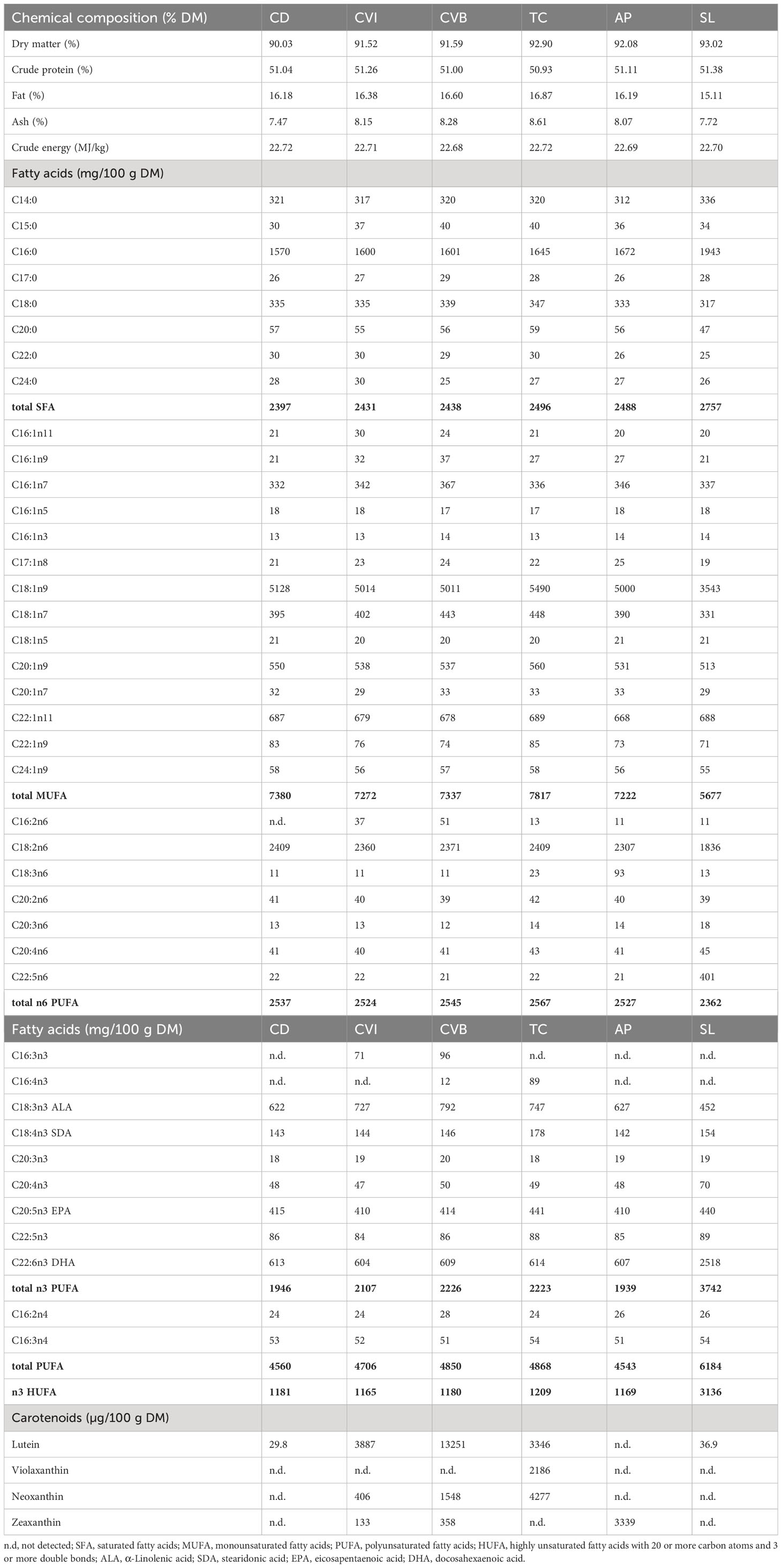
Table 2 Crude composition (percent dry matter) as well as fatty acid composition (mg/100g dry matter) and pigment composition (µg/100g dry matter) of the experimental diets given as mean of two and pigment concentrations as mean of four replicate analyses.
2.5 Diet and microalgae fatty acid composition
To physically break down the material, 10 g of each diet and microalgae (Supplementary Table 3) was ground with a mortar, mixed with 20 ml of distilled water and homogenised for 2 min with an Ultra-Turrax disperser (IKA). The slurry was lyophilised and mortared again. To determine the fatty acid composition, 20 mg sample (weighed to the nearest 0.1 mg) was then subjected to direct transesterification according to Griffiths et al. (2010). However, boron trifluoride-methanol was replaced by 3 M methanolic HCl (Sigma-Aldrich, Taufkirchen, Germany) and distilled water by 1 M aqueous NaCl (Merck, Germany). All other reagents were from Sigma-Aldrich. Each diet was transesterified in triplicate and the fatty acid methyl ester (FAME) extracts used for separate gas chromatography (GC) analysis. Fatty acid contents were calculated as mg FAME/100 g dry matter (Table 2). For GC conditions, see the section on muscle proximate and fatty acid composition.
2.6 Proximate and fatty acid composition of muscle
Moisture and ash content were determined by drying samples of around 5 g for 12 h at 105°C, followed by ashing at 550°C. The nitrogen content was measured by Dumas using a LECO TruSpecN (Leco Instruments GmbH, Mönchengladbach, Germany). Lipids were extracted according to Smedes (1999) using cyclohexane and 2-propanol (VWR, Darmstadt, Germany) with modifications by Karl et al. (2012). For GC analysis of fatty acids, lipids were obtained in a separate extraction without final drying at 105°C and transesterified into FAME using methanolic potassium hydroxide (ISO-IDF, 2002).
Fatty acid analysis was performed using a 7890A gas chromatograph (Agilent Technologies, Santa Clara, CA, USA) equipped with a 7683B autosampler, a split injection port (injection volume 1 µL, split 1:100), flame ionisation detection and a 100 m x 0.25 mm i.d. x 0.20 µm - CP-Sil 88 column (Agilent Technologies). Hydrogen was used as the carrier gas with a constant flow of 1.6 mL min-1. Two min after injection, the initial oven temperature of 175°C was increased by 1°C per minute to 190°C, held constant for 1 min, then increased by 5°C per minute to 225°C, held constant for 7 min, and finally increased by 1°C per minute to 237°C (1 min constant). Chromatograms were evaluated using EZChrom Elite 3.3.2 (Agilent Technologies). Identification of individual FAME was achieved by comparison to known standards (Supelco™ 37 Component FAME mix, PUFA No. 1, PUFA No. 3; all obtained from Sigma-Aldrich) in the range from C14:0 to C22:6n3. Fatty acid contents for fillet samples were calculated as weight percentage (g FA/100 g FA) and are given as means of duplicate analyses.
2.7 Carotenoid content
Carotenoids were extracted from the diets and microalgae with methanol using an Ultra-Turrax (IKA) at 24,000 rpm for two cycles of 45 s (Schüler et al., 2020). Samples were centrifuged and the supernatants of three repetitions were combined. During extraction the samples were kept on ice. The extraction of the fish fillet homogenate was done according to Ostermeyer and Schmidt (2004). After an initial evaluation of carotenoids in individual fish samples (n = 18), muscle samples were pooled on a tank level (n = 3).
For the quantitative determination of the carotenoids an aliquot of the extract was evaporated and the residue dissolved in mobile phase. HPLC was carried out using a C30 analytical column (5 µm, 250 x 4.6 mm i.d., YMC Europe, Dinslaken, Germany) preceding by a C30 guard column (5 µm, 10 x 4.0 mm i.d.) and a gradient of methyl tert-butyl ether, methanol with a small amount of an ammonium acetate buffer (pH 4.6), similar to (Rasmussen et al., 2012). A flow rate of 1.0 mL min-1 at 25°C with an injection volume of 100 µL was used. The detection was performed with a photodiode array detector (UV 6000 LP; Thermo Finnigan, San Jose, CA, USA) at 450 nm and 470 nm. Peaks were identified by comparison of the retention times and the absorption spectra (between 380 nm and 700 nm) with those of synthetic standards. The carotenoids were quantified using an external standard containing lutein, fucoxanthin, violaxanthin, neoxanthin, astaxanthin, zeaxanthin and canthaxanthin (ChromaDex, Irvine, CA, USA; Sigma-Aldrich; Dr. Ehrenstorfer, Augsburg, Germany).
2.8 Plasma metabolites and enzyme activities
Plasma glucose, triglycerides, total protein, alkaline phosphatase (ALP), aspartate aminotransferase (AST) and alanine amino-transferase (ALT) activity were measured on a Fuji Dry Chem NX500i (Fujifilm, Ratingen, Germany) using commercial kits and following the manufacturer´s instructions.
Total carotenoid content in plasma samples (Supplementary Figure 1) was measured after Donaldson (2012) with slight modifications. Briefly, 100 µL of plasma was mixed with 100 µL of 70% ethanol in a 1.5 mL reaction tube wrapped with aluminium foil and vortexed for one minute to precipitate the proteins. Then 300 µL of n-heptane (Roth, Karlsruhe, Germany) was added and the mixture was vortexed for four minutes at maximum speed. The mixture was centrifuged at 2000 g for two minutes. Following separation of both layers 290 µL of the heptane layer including the dissolved carotenoids were decanted and added to a 10 mm quartz cuvette (type 104-QS, Hellma, Müllheim, Germany) and further diluted with 410 µL of heptane. The absorbance was measured at 448 nm using a spectrophotometer (SPECORD210, Analytik Jena GmbH, Jena, Germany). The carotenoid content was then calculated according to Donaldson (2012). Spectral profiles of every sample (300 – 600 nm) confirmed the presence of a carotenoid peak at ~ 448 nm (Supplementary Figure 1B). Preparation of extracts and measurements were performed under reduced light conditions to minimize pigment degradation in the samples.
2.9 Western blots of liver proteins
Total protein from liver samples (n = 3 pool per tank) was extracted with Radioimmunoprecipitation (RIPA) lysis buffer according to the manufacturer’s protocol (RIPA Lysis Buffer System, Santa Cruz Biotechnology, Dallas, Texas, USA). The proteins Cu, Zn superoxide dismutase (Sod1) and myeloperoxidase (Mpo) were analyzed in the salmon liver per SDS-PAGE and Western Blot. The protein ß-actin served as loading control. A no template control and one positive control per antibody were included, Danio rerio liver for Mpo, Bovine liver for Sod1 and HEK-293 cells for ß-actin. 20 µg total protein was processed in reducing conditions with SDS sample and reducing buffer (both TruPAGE, Sigma-Aldrich, Schnelldorf, Germany) at 70°C for 10 min. SDS-PAGE was performed in a Xcell SureLock Mini-Cell (Thermo Fisher Scientific, Waltham, Massachusetts, USA) using precast 4-12% gradient gels, TruPAGE running buffer and antioxidant (Sigma-Aldrich). Proteins were electro-transferred to a PVDF membrane. For parallel protein detection of Mpo and Sod1 the membrane was horizontally cut. Primary antibody incubation, with Mpo antibody (ab210563, Abcam, Cambridge., UK) in 1:5000 dilution in PBS-T containing 2.5% skim milk and Sod1 antibody (NBP2-24915, Novus Biologicals, Bio-Techne Ltd., Abingdon, UK) in 1:500 dilution in PBS-T containing 2.5% skim milk, was performed at 4°C overnight. Secondary antibody anti-rabbit IgG conjugated HRP (sc-2357, Santa Cruz Biotechnology) was incubated in a 1:5000 dilution for 90 min at room temperature. Detection was performed using ECL detection reagents (Amersham, Global Life Sciences Solutions USA LLC, Marlborough, MA, USA) and chemiluminescence film (Amersham, GE Healthcare Ltd, Little Chalfont, UK) with 40 sec exposure time for both Mpo and Sod1. For the subsequent detection of ß-actin the antibodies were stripped using 100 mM Glycin buffer (pH 2.5). The membrane was incubated in 1:5000 dilution of β-actin antibody (NB600-503, Novus Biologicals, Bio-Techne Ltd., Abingdon, UK) at 4°C overnight. Quantification of protein expression was done following densitometric analysis of the protein bands using GIMP and normalized to housekeeping (ß-actin) protein expression.
2.10 Gene expression in liver, spleen and intestine
Total RNA was extracted using TRIzol (ThermoFisher Scientific, Waltham, MA, USA) and further purified with the ISOLATE II RNA Micro Kit (Meridian Bioscience Inc., Cincinnati, OH, USA). The concentration and integrity of the extracted RNA was measured by NanoDrop One (Thermo Fisher Scientific). Subsequently, cDNA synthesis was performed using Reverse Transcription Master Mix (Fluidigm, San Francisco, CA, USA). The samples were preamplified by the PreAmp Master Mix (Fluidigm) and at last treated with exonuclease I (New England BioLabs, Frankfurt/Main, Germany). All steps have been carried out according to the manufacturer’s instructions.
45 genes with tissue specific regulation were selected from an established gene set composed of key immune and stress regulated genes in Atlantic salmon (Krasnov et al., 2020; Lund et al., 2022; Supplementary Table 4). We extended this set by three immunogene-specific primers derived for hamp, saa5 and sod1 (Supplementary Table 4). The same 48 genes (45 target and 3 reference genes) were measured in the tissue anterior intestine, liver and spleen.
The 48.48 gene expression biochips were primed in the MX IFC Controller (Standard BioTools, San Francisco, CA, USA). The pre-amplified cDNA samples were pipetted to the sample inlets and the primers were loaded on the assay and finally, analyzed with the Biomark HD using the manufacturer’s thermal protocol “GE Fast 48 × 48 PCR+Melt v2.pcl” (application type: gene expression; passive reference: ROX; assay: single probe).
The raw qPCR Ct values were obtained using the Fluidigm real-time PCR analysis software v. 3.0.2 (Munich, Germany). Relative expression was calculated based on ΔΔCt where three reference genes coding for b-actin, ribosomal protein L4 and ribosomal protein S20 (actb, rpl4, rps20) were used as internal normalisers. The mean Ct per gene for all samples was used as a calibrator during the calculation. Relative expression values were log2 transformed prior to statistical analysis. Four individuals with abnormal phenotypic signs and subsequently abnormally high gene expression profiles were removed from the dataset. The genes cxcl8 and il1b in the liver and cxcl8 in the spleen were removed from the dataset, as to many missing values hampered analysis of the data.
2.11 Statistical analysis
Statistical analysis and data visualization were conducted using the software R (R version 4.1.0) in the environment RStudio. For all test α = 0.05 was used as the level of significance. Data is presented as mean ± standard error of mean (SEM). For the performance parameters, protein concentrations in the liver as well as carotenoid concentrations in the muscle an appropriate statistical model based on generalized least squares was defined (Carroll and Ruppert, 1988) which included the factor diet as well as timepoint for the latter two. The residuals were assumed to be normally distributed and to be heteroscedastic, which was based on a graphical residual analysis. Analysis of variance (ANOVA) was conducted, followed by multiple contrast tests for heteroscedastic data (Hasler and Hothorn, 2008) in order to compare the several diets with the control diet. For plasma parameters, proximate and fatty acid composition of the muscle, as well as gene expression data, mixed effect models (Laird and Ware, 1982; Carroll and Ruppert, 1988; Pinheiro and Bates, 2000) were used. The model included diet, timepoint and their interaction as fixed factors and tank as a random factor. The residuals were assumed to be normally distributed and to be heteroscedastic. Based on this model, a Pseudo R2 was calculated (Nakagawa and Schielzeth, 2013) and an ANOVA was conducted, followed by multiple contrast tests in order to compare the several diets with the control diet, and the timepoints, respectively. If the factors diet and timepoint had no significant interaction, then corresponding multiple contrast tests were pooled over the levels of the remaining factor. Spearman correlation analysis was employed to relate muscle lutein and plasma carotenoid concentrations, since muscle lutein concentrations were not normally distributed. Pearson correlation analysis was applied to relate the increase in DHA with a decrease in fat content in the muscle.
3 Results
3.1 Fish performance and proximate body composition
Feeding the experimental diets for eight weeks to the salmon in brackish water revealed no difference in growth and feed intake among groups (Table 3). Feed conversion ratio (FCR) was highest in the control group (CD), and including broken C. vulgaris (CVB) in the feed significantly improved the feed conversion ratio (p = 0.03). Furthermore, feeding CVB slightly improved the protein efficiency ratio (p = 0.11) but reduced body condition (p = 0.01). Hepatosomatic index and spleen somatic index were not affected by the diet (Table 3). Mortality was low and not different among diet groups. Furthermore, the diet did not affect proximate whole-body composition of the salmon (Table 4) but feeding CVB slightly increased ash content (p = 0.06). After the fish were transferred to full strength seawater voluntary feed intake decreased to one third of the levels prior transfer but was not different among groups (Table 3).
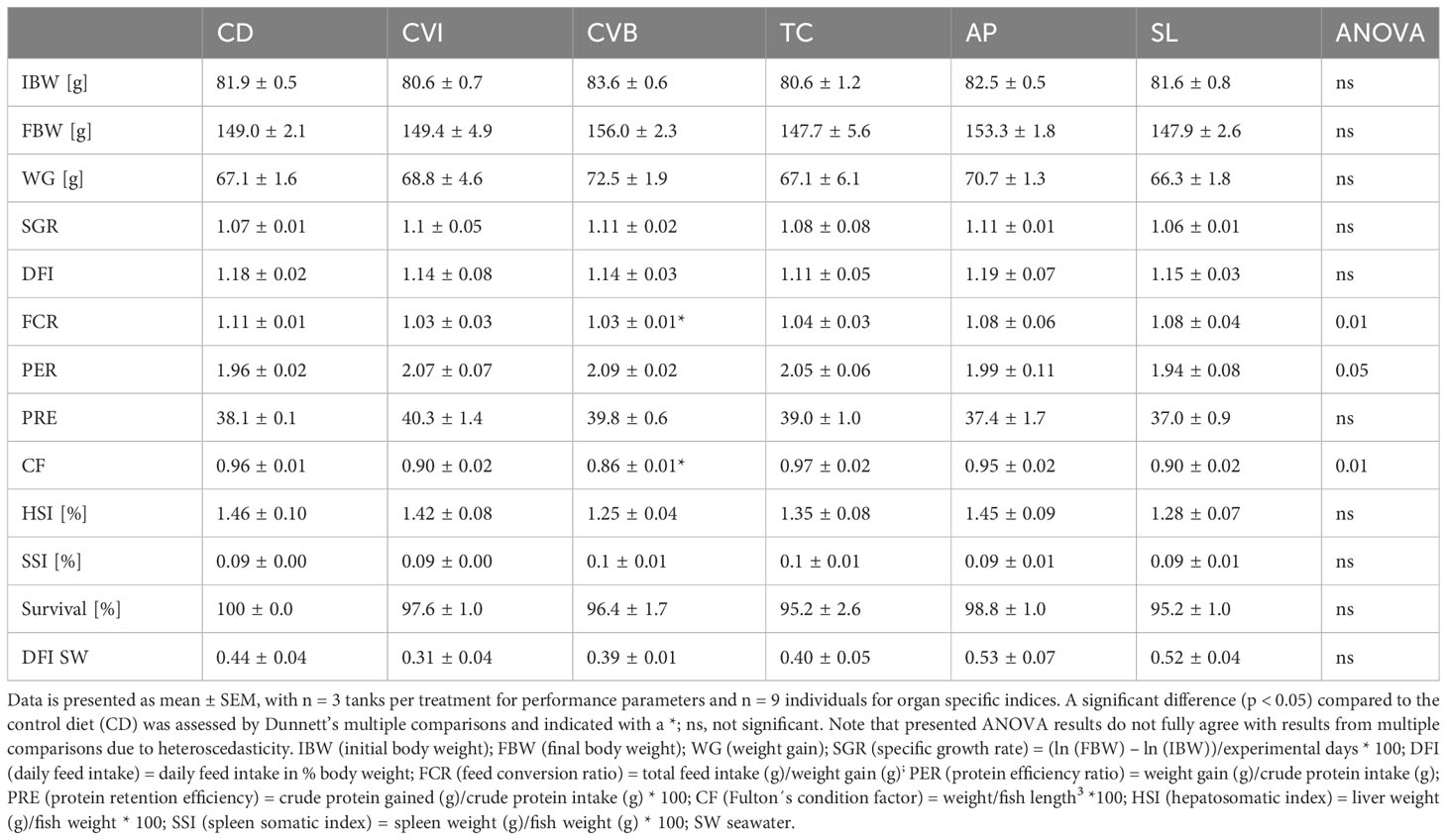
Table 3 Growth performance and organ specific indices of Atlantic salmon after eight weeks of feeding the experimental diets in brackish water and feed intake for the period of two weeks in seawater.
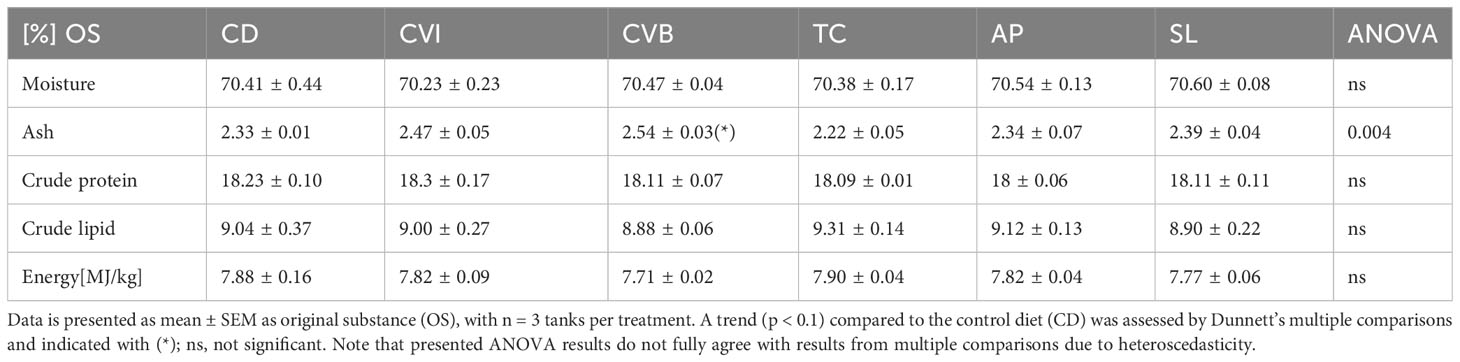
Table 4 Proximate body composition (percent OS) of Atlantic salmon after eight weeks of feeding the experimental diets.
3.2 Proximate and fatty acid composition of muscle
The diet did not affect protein, water and ash content of the muscle in brackish water (T2) and following transfer to seawater (T3; Tables 5; S5). Fat content was significantly reduced in brackish water in fish fed A. platensis (AP; 12% reduction) and S. limacinum (SL; 13.5% reduction) compared to CD (Table 5). Fat content decreased on average by 29% after transfer to seawater across all groups (Table 5). It decreased most in groups receiving intact and broken C. vulgaris, CVI (38%) and CVB (32.9%), but markedly less in fish fed AP (18.5%).
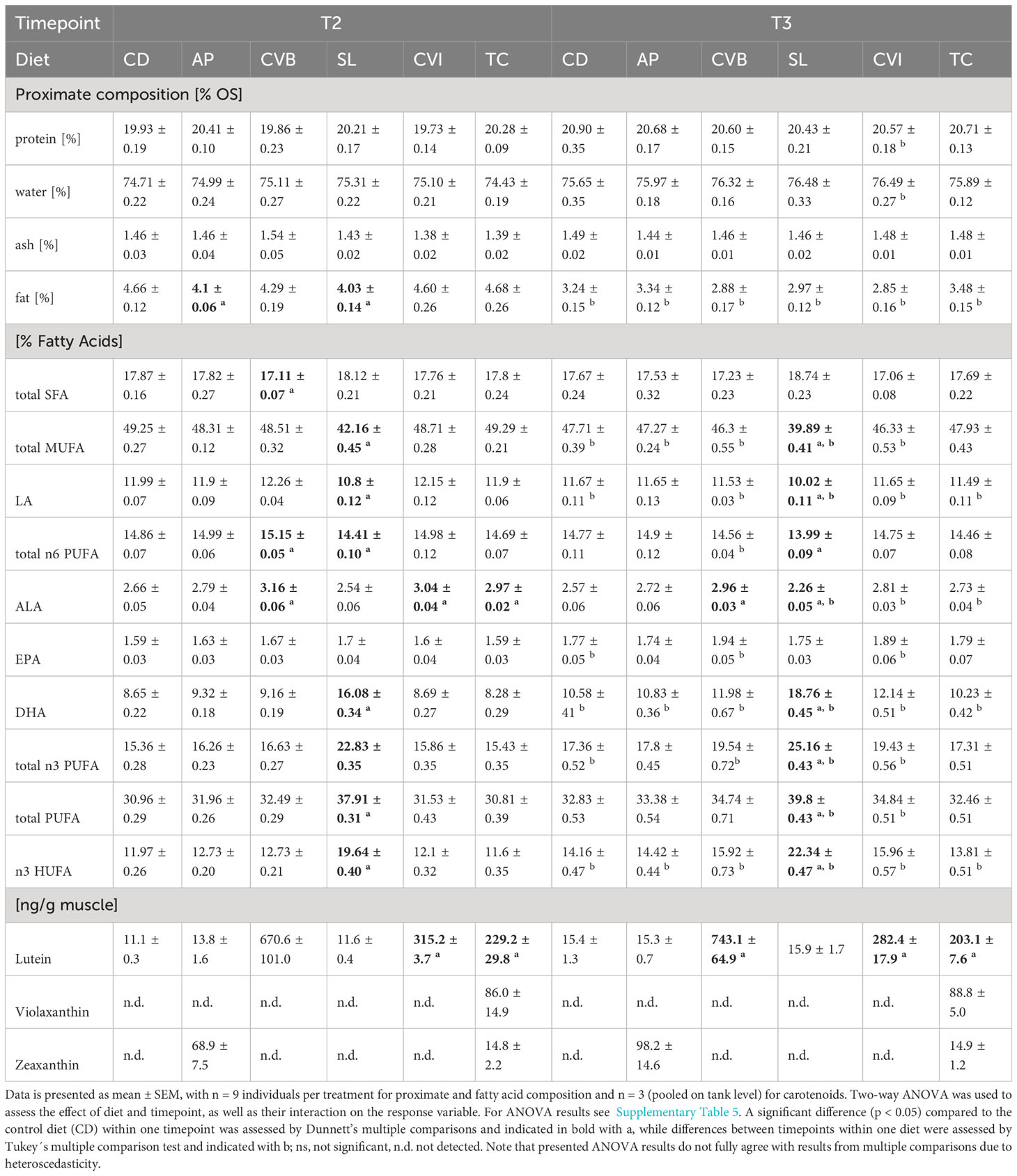
Table 5 Proximate, fatty acid and carotenoid composition of Atlantic salmon muscle fed microalgae enriched diets eight weeks in brackish water (T2) and two weeks following seawater transfer (T3).
Generally, fatty acid composition in the muscle lipids reflected that of the diet (Table 5; Table 2). Both diet and timepoint significantly influenced the fatty acid composition, however, an interaction of both factors was absent in most cases (Tables 5; S5). Alpha-linolenic acid (LA) was significantly enriched in muscle lipids of CVB, CVI and T. chuii (TC) fed fish in brackish water and in CVB and CVI fed fish after transfer to seawater (Table 5). Steraidonic acid (SDA) content was higher in TC compared to CD at both timepoints. Eicosapentaenoic acid (EPA) levels were affected by an interaction of diet and timepoint and levels increased in CD by 11.3%, CVB by 16.2% and CVI 18.1% following transfer to seawater (Tables 5; S5). Docosahexaenoic acid (DHA) levels were significantly increased in SL compared to CD fed fish at both timepoints and relative levels increased following transfer to seawater in all groups by 23.8% (Figure 2A, Table 5). A significant negative correlation between the relative reduction in total fat content in the muscle and the relative increase in DHA based on diet group means was detected (R = 0.89, p < 0.001; Figure 2B).
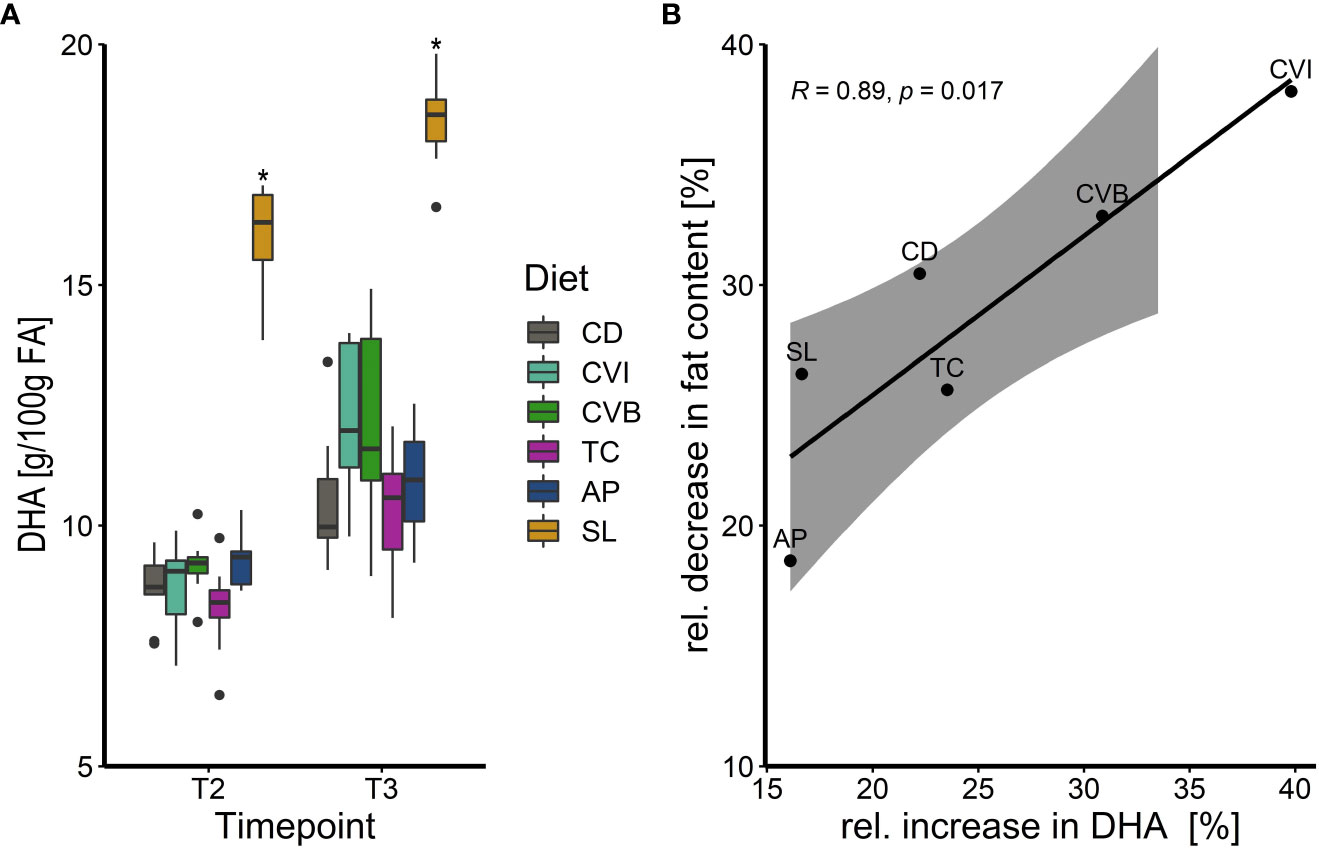
Figure 2 (A) Boxplot of docosahexaenoic acid (DHA) concentration in g/100g fatty acids (FA) of Atlantic salmon muscle fat following feeding the experimental diets for eight weeks in brackish water (T2) and transferred to seawater for two weeks (T3), n = 9. The fish received six different experimental diets: control (CD), Chlorella vulgaris intact (CVI), Chlorella vulgaris broken (CVB), Tetraselmis chuii (TC), Arthrospira platensis (AP) and Schizochytrium limacinum (SL) at an inclusion level of 8%. A significant difference (p < 0.05) compared to the control diet (CD) was assessed by Dunnett’s multiple comparisons and indicated with a * (B) Linear relationship between the relative increase in muscle lipid DHA content in % in relation to the relative decrease (%) of muscle fat content following transfer to seawater (T3). Note that for Pearson´s correlation analysis group means per diet were used (n = 6) and diet groups are indicated next to each datapoint.
3.3 Muscle and plasma carotenoid content
Lutein was the main carotenoid present in all groups and significantly enriched in the muscle at both timepoints of fish fed CVI, CVB and TC (Table 5) reflecting the content of lutein in the respective algae (Supplementary Table 3). Lutein content in fish fed CVB was more than two times higher than in fish fed CVI and TC. Zeaxanthin was detected in muscle of fish fed AP and TC and violaxanthin was only detected in fish fed TC (Table 5).
The total carotenoid content in plasma of CVB fed fish was two times higher than in fish fed CVI (p = 0.08) and TC (p = 0.05; Figure 3A), which was also visible when comparing plasma samples of the respective groups directly (Supplementary Figure 1A). No carotenoid content was detectable in groups fed CD and SL (Supplementary Figures 1A, B), while values for AP were below the calculated standard curve and were subsequently excluded from further analysis. Muscle lutein concentrations significantly correlated with total carotenoid concentration in plasma samples (Spearman R = 0.52, p = 0.03; Figure 3B).
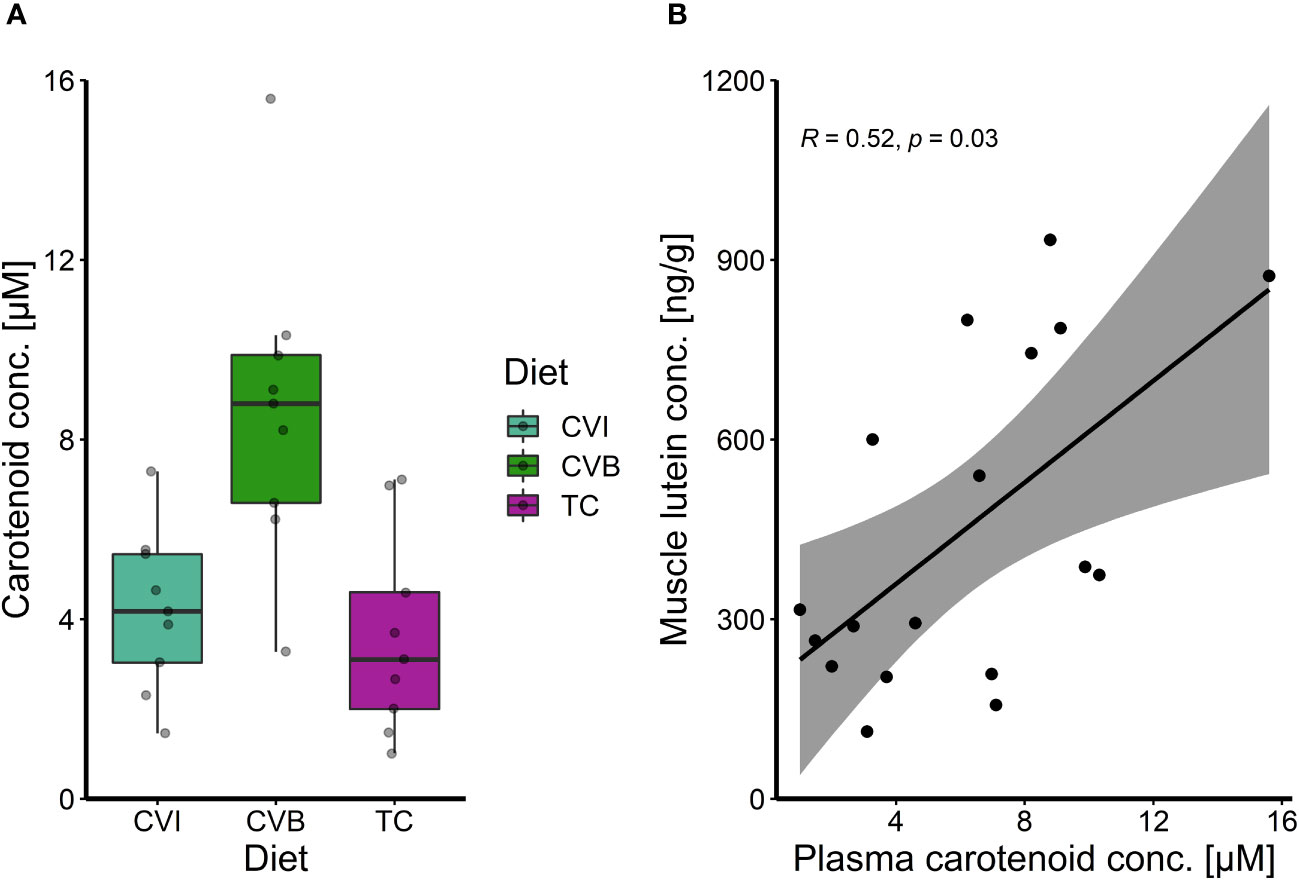
Figure 3 (A) Boxplot of carotenoid concentration in plasma of Atlantic salmon after eight weeks of feeding experimental diets enriched with C. vulgaris intact (CVI), broken (CVB) or T. chuii (TC) and (B) spearman correlation among plasma total carotenoid concentration and muscle lutein concentration after eight weeks of feeding experimental diets for fish where both parameters were measured on an individual level (n = 18).
3.4 Plasma metabolites and enzyme activities
CVI inclusion significantly lowered aspartate aminotransferase and alanine aminotransferase activity in microalgae fed groups compared to CD (p ≤ 0.046; Figures 4A, B). Furthermore, SL lowered aspartate aminotransferase activity (p = 0.06; Figure 4A). The highest variance in aspartate aminotransferase and alanine aminotransferase activity was found among fish fed CD, while it was lowest among fish fed CVI. Total plasma protein and alkaline phosphatase activity were not influenced by the diet but showed an overall increase between T1 and T2 (p < 0.001; Supplementary Figure 2). Total cholesterol increased significantly between the first two samplings for fish fed CVI, CVB and TC (p < 0.05; Supplementary Figure 2). Glucose furthermore increased over time only in fish fed SL (p = 0.02; Supplementary Figure 2).
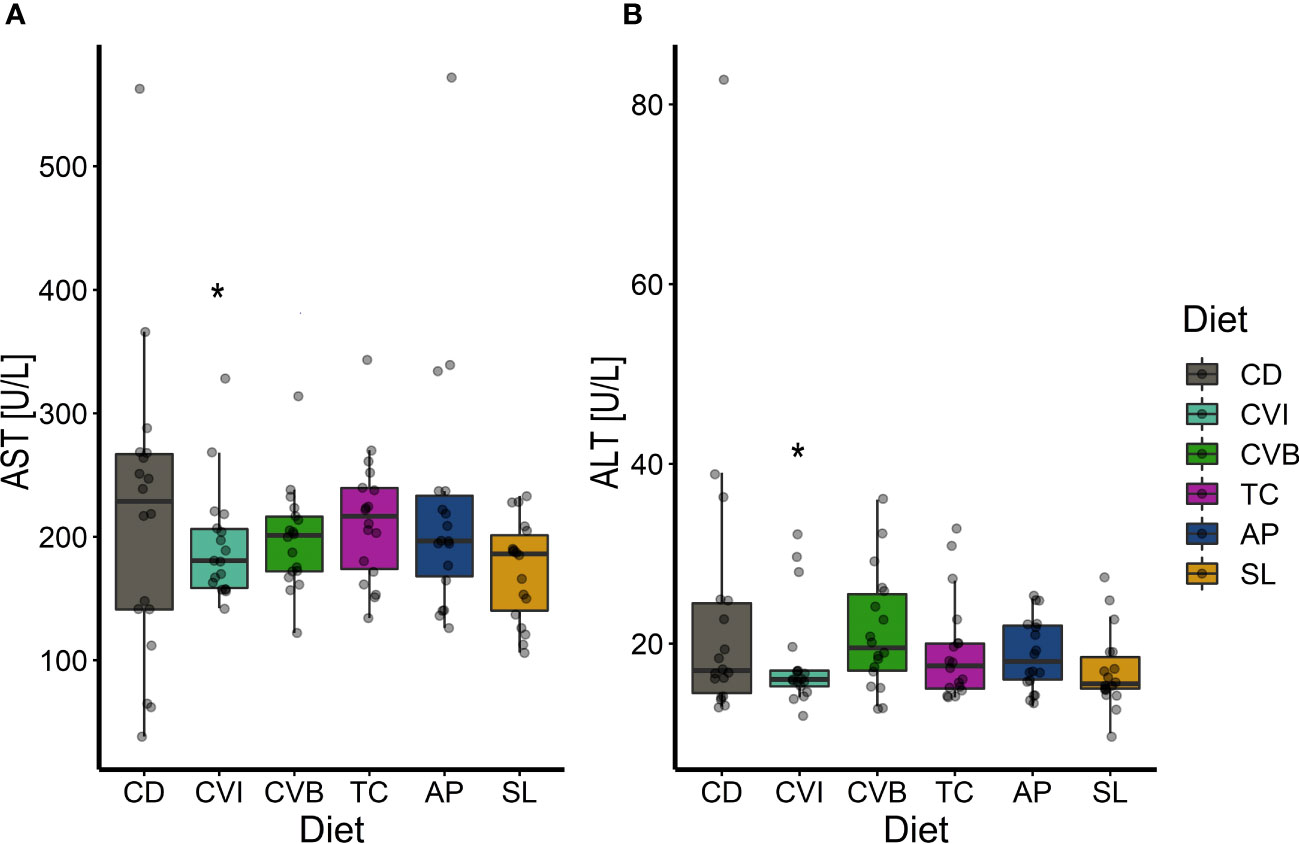
Figure 4 Boxplot of aspartate aminotransferase (AST) activity (A) and alanine aminotransferase (ALT) activity (B) in plasma of Atlantic salmon fed microalgae enriched diets for eight weeks (T2, n = 18). The fish received six different experimental diets: control (CD), Chlorella vulgaris intact (CVI), Chlorella vulgaris broken (CVB), Tetraselmis chuii (TC), Arthrospira platensis (AP) and Schizochytrium limacinum (SL) at an inclusion level of 8%. A significant difference (p < 0.05) compared to the control diet (CD) was assessed by Dunnett’s multiple comparisons and indicated with a *.
3.5 Liver proteins
Abundance of myeloperoxidase (Mpo) in the liver of Atlantic salmon fed a microalgae-enriched diet was lower in most cases at both timepoints compared to CD (Figure 5A). This effect was however only significant for fish fed AP at T1 (p = 0.049) with a 35% reduction compared to CD due to a large within group variation (Figure 5A). Though, AP at both timepoints showed the lowest variation. Sod1 protein level was induced by 3-fold in SL at T1 compared to CD (p = 0.10; Figure 5B).
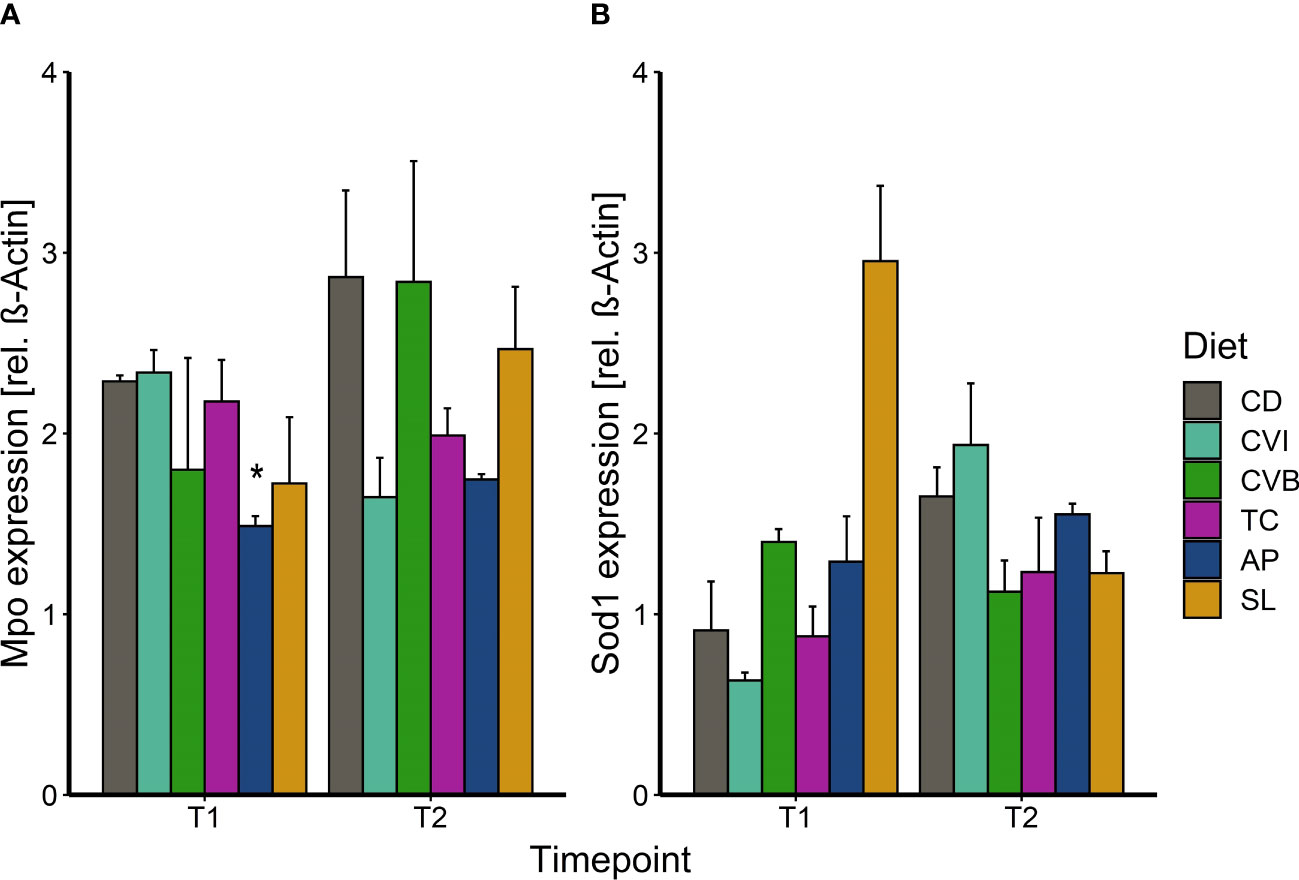
Figure 5 Protein expression of myeloperoxidase (A) and superoxide dismutase 1 (B) in liver tissue of Atlantic salmon following feeding the microalgae enriched diets for two weeks (T1) and eight weeks (T2) in brackish water. The fish received six different experimental diets: control (CD), Chlorella vulgaris intact (CVI), Chlorella vulgaris broken (CVB), Tetraselmis chuii (TC), Arthrospira platensis (AP) and Schizochytrium limacinum (SL) at an inclusion level of 8%. Data is presented as mean + SEM, n = 3 (pooled on tank level). A significant difference (p < 0.05) compared to the control diet (CD) was assessed by Dunnett’s multiple comparisons and indicated with a *.
3.6 Gene expression
Only few genes were significantly differentially expressed between microalgae fed groups compared to CD fish, due to a large overall variability in expression (Figures 6–8) although some large fold-changes were evident (Supplementary Figure 3). In the anterior intestine, increased drtp1-transcript levels were found in CVI- (2.8-fold, p = 0.065; Figure 6A) and CVB-fed salmon (7-fold; p = 0.023; Figure 6A). isg15 transcript levels were induced in all microalgae fed groups except for CVI (Figure 6B), although not statistically significant. il1r2 transcript levels were reduced by 2.7-fold in fish fed CVI (p = 0.039; Figure 6C) and il10rb levels were reduced in fish fed CVI (p = 0.028; Figure 6D) and TC (p = 0.039).
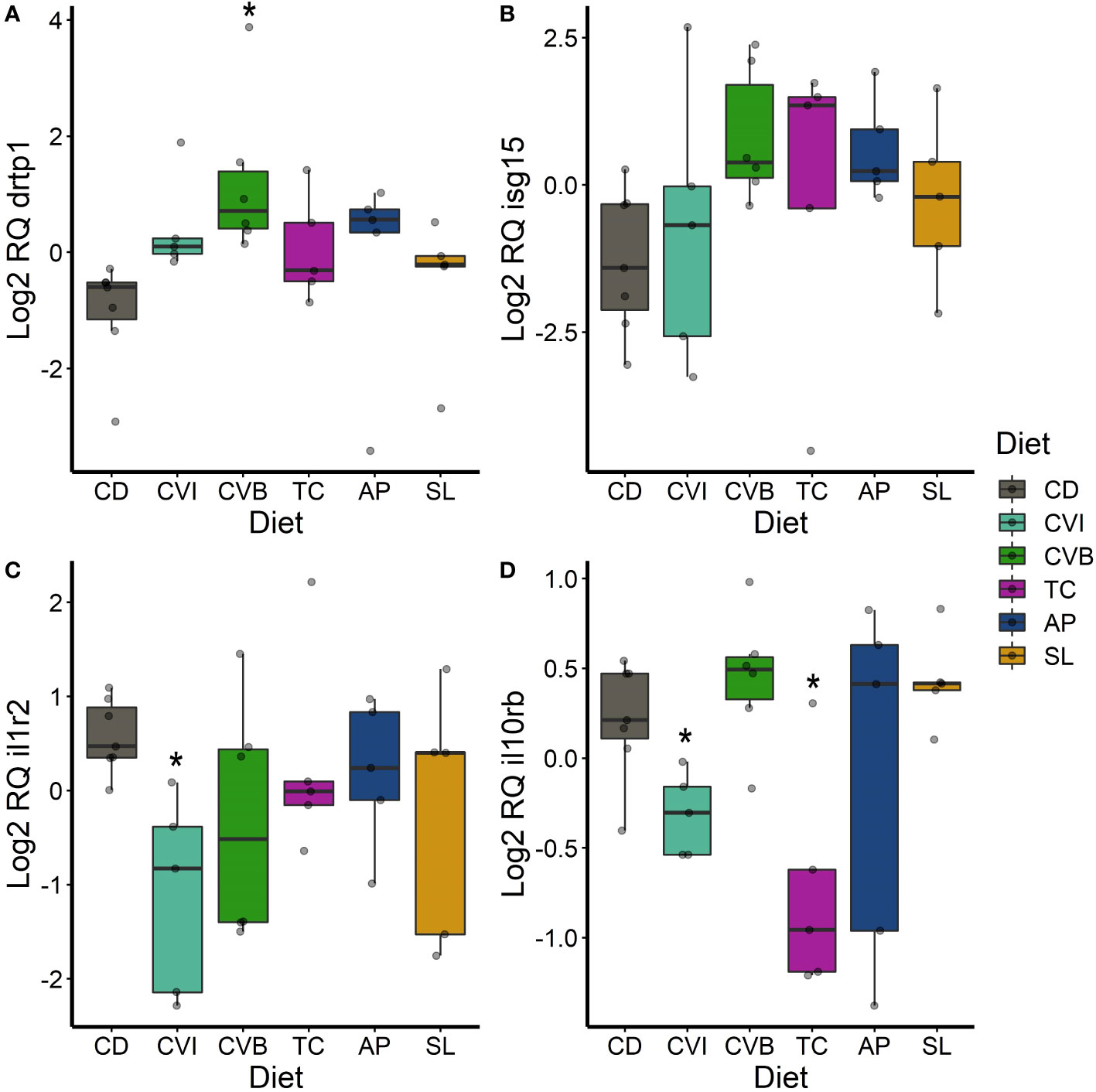
Figure 6 Boxplot of the gene expression in the anterior intestine of drtp1 (A), isg15 (B), il1r2 (C) and il10rb (D) of fish fed microalgae enriched diets for eight weeks. The fish received six different experimental diets: control (CD), Chlorella vulgaris intact (CVI), Chlorella vulgaris broken (CVB), Tetraselmis chuii (TC), Arthrospira platensis (AP) and Schizochytrium limacinum (SL) at an inclusion level of 8%. Expression values were normalized relative to the mean expression of all samples and log2 transformed (n = 5 – 7). A significant difference (p < 0.05) compared to the control diet (CD) was assessed by Dunnett’s multiple comparisons and indicated with a *.
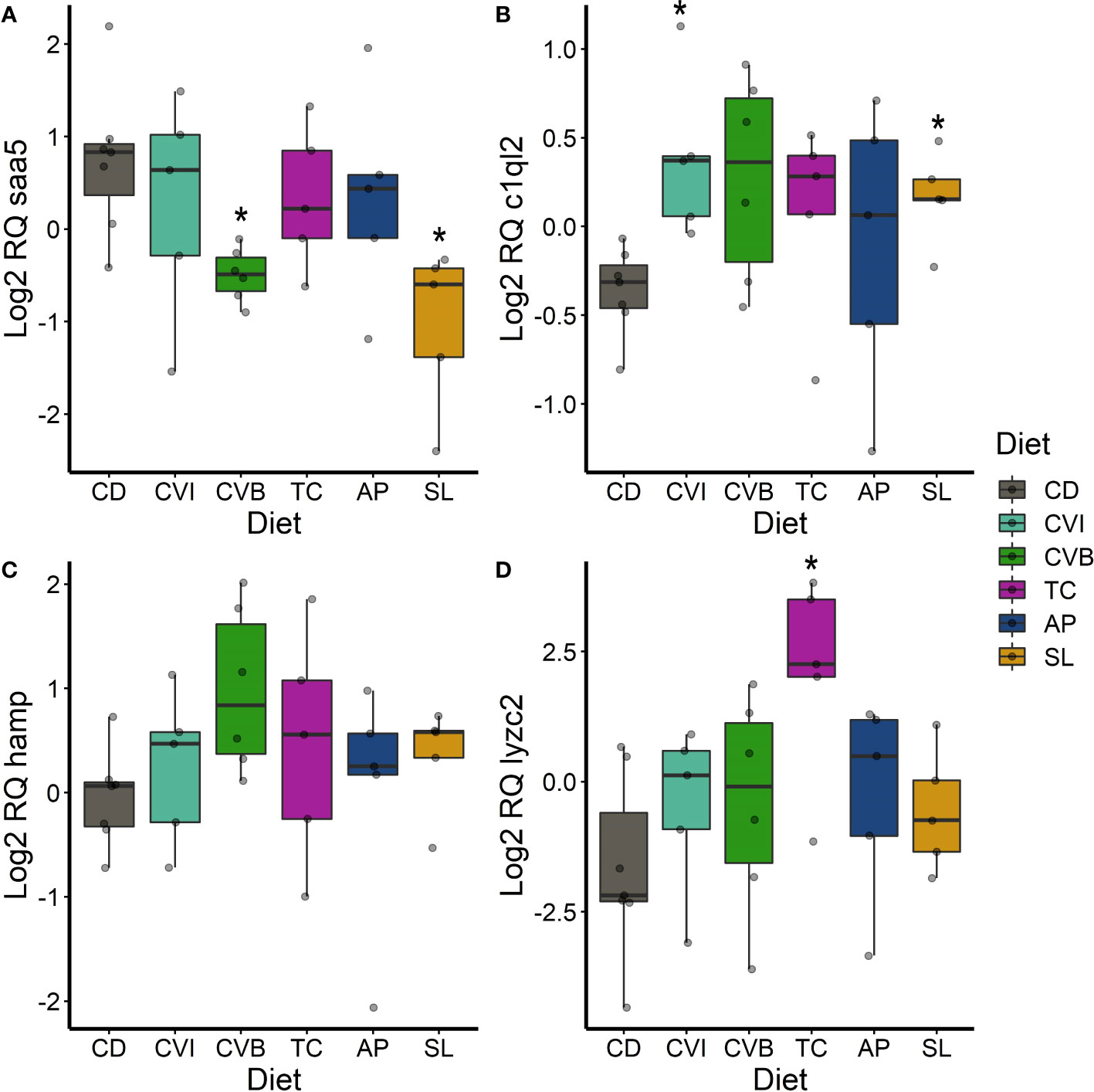
Figure 7 Boxplot of the gene expression in the liver of saa5 (A), c1ql2 (B), hamp (C) and lyzc2 (D) of fish fed microalgae enriched diets for eight weeks. The fish received six different experimental diets: control (CD), Chlorella vulgaris intact (CVI), Chlorella vulgaris broken (CVB), Tetraselmis chuii (TC), Arthrospira platensis (AP) and Schizochytrium limacinum (SL) at an inclusion level of 8%. Expression values were normalized relative to the mean expression of all samples and log2 transformed (n = 5 – 7). A significant difference (p < 0.05) compared to the control diet (CD) was assessed by Dunnett’s multiple comparisons and indicated with a *.
In the liver, the transcript levels of the acute-phase gene saa5 were significantly reduced in fish fed CVB (0.37-fold; p = 0.016; Figure 7A) and SL (0.29-fold; p = 0.012, Figure 7A). c1ql2 transcripts were significantly induced in fish fed CVI (p = 0.032) and SL (p = 0.03; Figure 7B). hamp transcripts were 2.2-fold higher concentrated in the liver of fish fed CVB (p = 0.066; Figure 7C) and lyzc2 transcripts were even 12-fold increased in fish fed TC (p = 0.04; Figure 7D) compared to the control group.
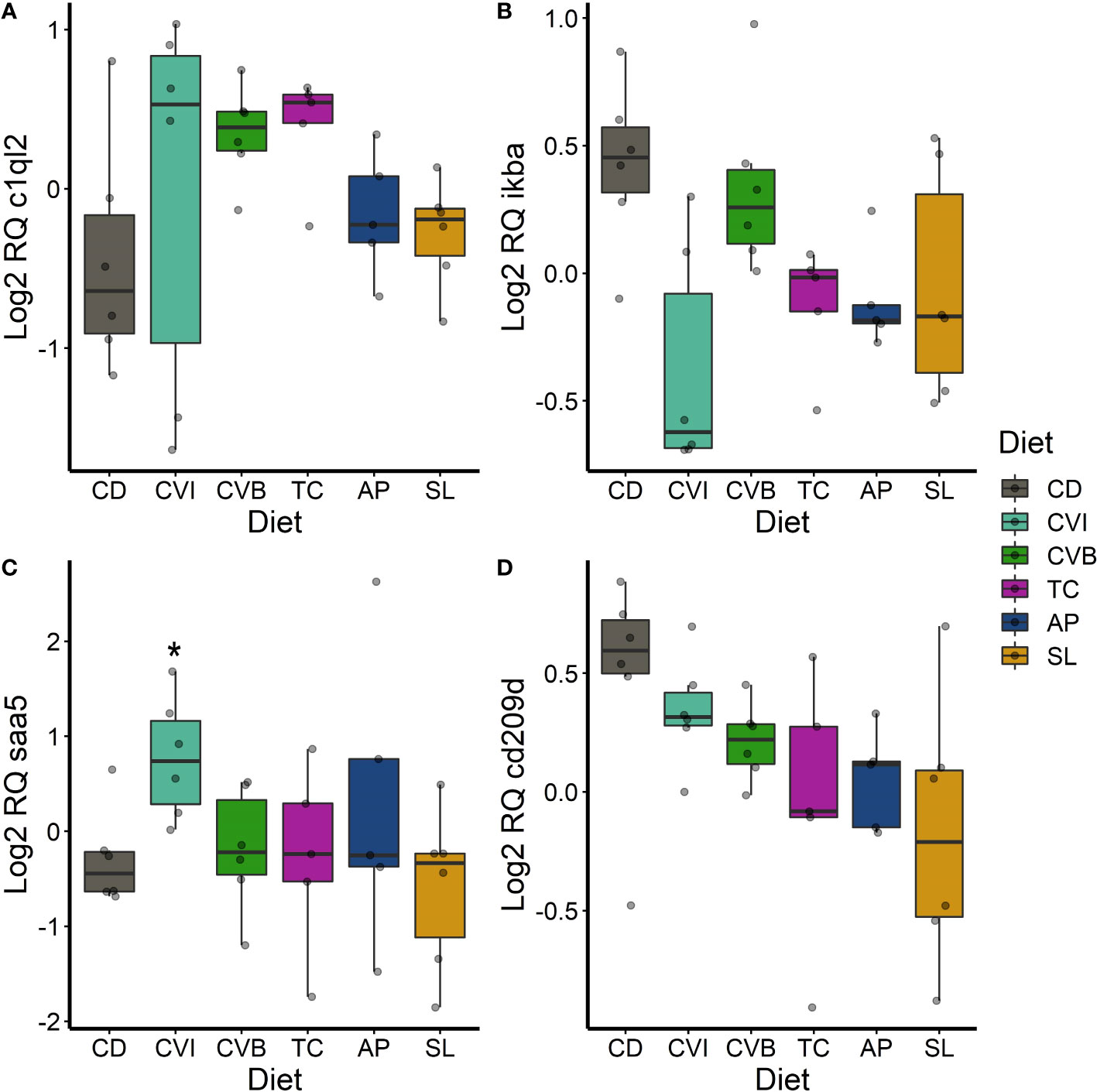
Figure 8 Boxplot of the gene expression in the spleen of c1ql2 (A), ikba (B), saa5 (C) and lyzc2 (D) of fish fed microalgae enriched diets for eight weeks. The fish received six different experimental diets: control (CD), Chlorella vulgaris intact (CVI), Chlorella vulgaris broken (CVB), Tetraselmis chuii (TC), Arthrospira platensis (AP) and Schizochytrium limacinum (SL) at an inclusion level of 8%. Expression values were normalized relative to the mean expression of all samples and log2 transformed (n = 5 – 7). A significant difference (p < 0.05) compared to the control diet (CD) was assessed by Dunnett’s multiple comparisons and indicated with a *.
In the spleen, c1ql2 transcripts were slightly 1.5-fold induced in fish fed CVB (p = 0.095) and TC (p = 0.097; Figure 8A). ikba transcripts were 0.59-fold reduced in the spleen of fish fed CVI (p = 0.06; Figure 8B). Furthermore, transcript abundance of saa5 was 2.1-fold increased in fish fed CVI (p = 0.034; Figure 8C). The transcript level of cd209d was reduced across all microalgae-fed fish, although not statistically significant (Figure 8D).
Since the levels of plasma markers and selected transcripts varied largely, we conducted a correlation analysis to identify connections and validate the overall utility of the used health parameters. However only alkaline phosphatase (ALP) activity in plasma significantly correlated with clra (R = 0.46, p = 0.008; Supplementary Figure 4A) and c4b transcript levels in the liver (R = 0.45, p = 0.008; Supplementary Figure 4B).
4 Discussion
Microalgae are gaining attention as a sustainable ingredient to replace fishmeal or oil in aquaculture diets (Shah et al., 2018; Kousoulaki et al., 2020; Sarker et al., 2020a; Sarker et al., 2020b) and further as a functional supplement, prebiotic and immunostimulant for farmed fish (Reyes-Becerril et al., 2013; Rahimnejad et al., 2017; Messina et al., 2019; Sun et al., 2019; Teimouri et al., 2019). In this study microalgae inclusion did not negatively affect performance of Atlantic salmon reared in recirculating aquaculture systems. However, we found that health, immunity as well as fatty acid and pigment deposition were influenced in an algae specific manner.
Inclusion of microalgae in fish feed has been shown to affect the growth performance via increasing feed intake or improving feed conversion in a variety of fish species (Table 6). Including 5% Chlorella sorokiniana in the diet increased feed intake and thus growth in rainbow trout (Chen et al., 2021) and including Chlorella vulgaris at levels of 10 to 15% improved feed intake and conversion in olive flounder (Rahimnejad et al., 2017). In this line including broken C. vulgaris in our study improved feed conversion efficiency but not feed intake in Atlantic salmon. Nevertheless at 8% inclusion level the palatability of the feeds in our study was not negatively affected, likely because these microalgae do not contain high amounts of anti-nutritional factors, as observed for other plant-based ingredients (Nagel et al., 2012; von Danwitz and Schulz, 2020). Voluntary feed intake decreases in many fish species in response to stress (Kulczykowska & Sánchez Vázquez, 2010). Transferring the salmon into seawater reduced appetite as previously described (Usher et al., 1991). Although microalgae were shown to increase feed intake in many species (Table 6) and mitigate acute stress (de Mattos et al., 2019), we found no indications that microalgae could increase feed intake during the critical time period of the first weeks in seawater. In contrast, a diet enriched with the feeding stimulant squid extract was able to improve feed intake of Atlantic salmon in this time period (Toften et al., 2003) and other ingredients which act as feed attractants might be explored in the future. The overall lower growth performance compared to other studies (Kousoulaki et al., 2020; Ytrestøyl et al., 2020) is likely attributed to the use of pelletized feeds (Kiron et al., 2012), as compared to high performance extruded feeds.
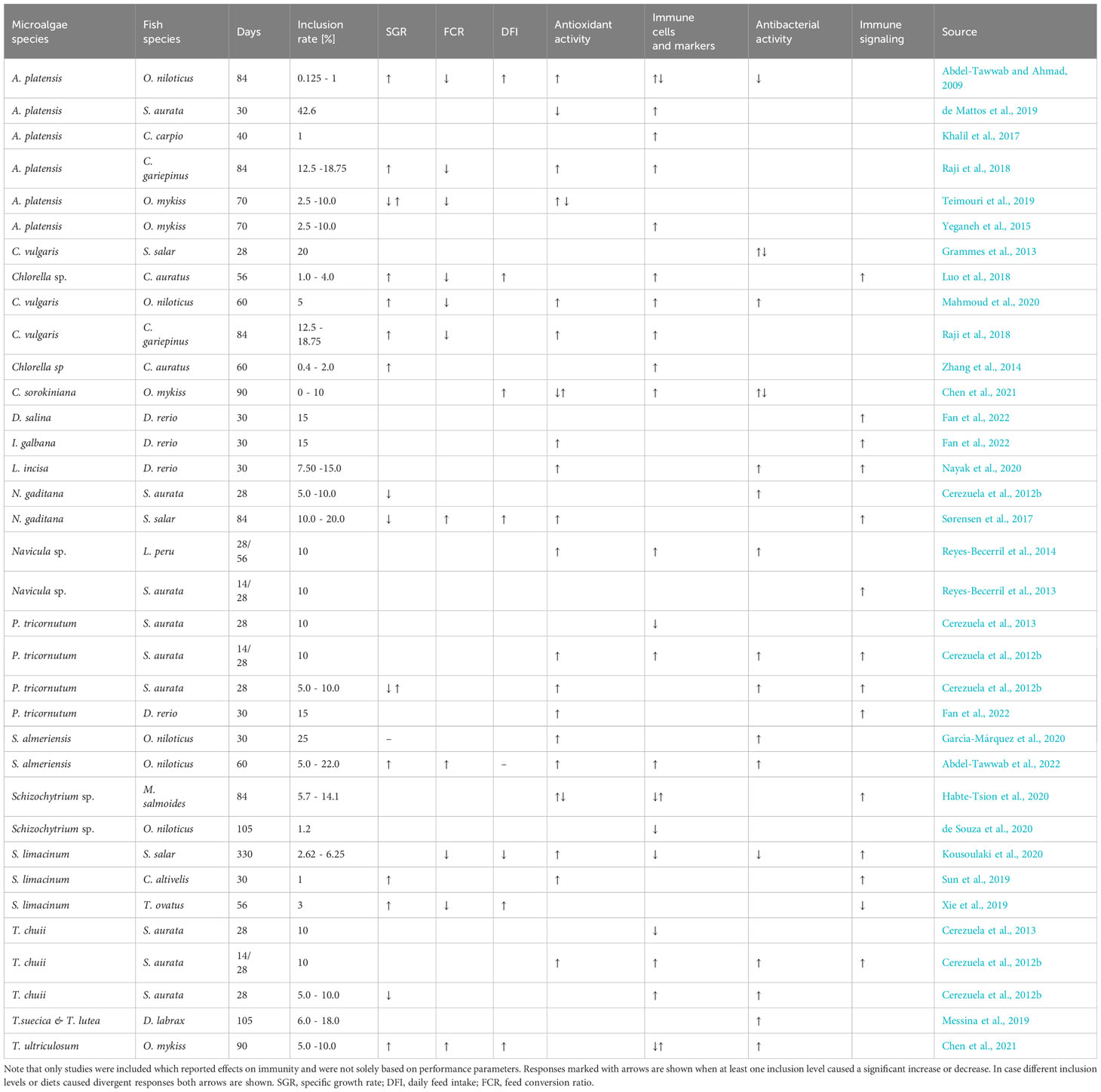
Table 6 Growth and immune effects of microalgae species incorporated in the diet and fed to different fish species.
Many microalgae species such as Chlorella and Tetraselmis contain a rigid cell wall, with a rigid cell wall fraction build of chitin- or chitosan-like polysaccharides (Domozych et al., 2012; Weber et al., 2022), which can reduce nutrient digestibility. Digestibility was reduced in Atlantic salmon fed with Chlorella vulgaris already at 6% inclusion (Tibbetts et al., 2017), but pre-extruded Nanochloropsis included at 10% improved dry matter digestibility and did not change protein digestibility in Atlantic salmon (Gong et al., 2020). The improved FCR found for fish fed broken C. vulgaris in our study, a species with a particularly rigid cell wall, is probably related to the destructed cell wall (Weber et al., 2022). The actual mechanism for improvements in feed conversion efficiency by microalgae is however not well understood, but seems to be related to promoting growth of beneficial intestinal bacteria (Ma et al., 2022), as well as improving intestinal health and nutrient uptake (Perera et al., 2020; Molina-Roque et al., 2022). Although inclusion levels of microalgae in diets vary greatly (Table 6) there seems to be a threshold at 10 – 15% inclusion for carnivorous fish, upon where growth performance is negatively affected likely because of reduced digestibility.
Besides its direct effects on growth, microalgae as a functional feed additive can influence body proximate composition and somatic indices by modulating energy metabolism. Low inclusion levels of microalgae derived nutraceuticals increased body proximate protein content, hepatosomatic index and glucose uptake capacity of the liver of gilthead sea bream, Sparus aurata (Perera et al., 2020). C. vulgaris in the diet was found to influence lipid metabolism in rats given a high fat diet, where it was able to lower triglycerides, total cholesterol and LDL cholesterol (Cherng and Shih, 2005). Similar results have been found in a study with humans (Ebrahimi-Mameghani et al., 2014). Reduced condition, hepatosomatic index and whole-body fat content in salmon fed diets containing broken C. vulgaris in our study also indicates interference with the lipid metabolism. It is however unclear whether increased metabolization or decreased deposition of lipid and glycogen took place as a response to the diet. However, in contrast to the above-mentioned studies, total cholesterol levels in plasma were unaffected.
Microalgae supplementation had a clear effect on the fatty acid profile of the fish muscle and levels found in the muscle of the salmon mirrored levels in the diets (Bell et al., 2001; Caballero et al., 2002). Increased levels of alpha-linolenic acid in the muscle of fish fed C. vulgaris (intact and broken) and T. chuii, as well as higher levels of DHA in fish fed S. limacinum resulted from higher contents of these specific fatty acids in the respective microalgae. This was evident although the experimental period of eight plus two weeks was rather short compared to other studies (Bell et al., 1993; Ruyter et al., 2000; Sissener et al., 2016). Atlantic salmon need to take up essential fatty acids via the diet and dietary requirements for EPA + DHA for Atlantic salmon post-smolts have been found to be ~ 0.5% of dry matter (Bou et al., 2017). All diets contained sufficient EPA and DHA, but high levels of DHA (2.5% of DM) in the diet containing S. limacinum were not reflected to the same degree in the muscle of the fish. The results must, however, be interpreted carefully, as preferential retention of specific fatty acids can influence the results in the muscle (Bell et al., 2003). Retention efficiency of DHA was indicated to be dose dependent (Glencross et al., 2014; Emery et al., 2016) and high dietary DHA in the S. limacinum diet could have reduced the need for an efficient retention of DHA in the muscle. Furthermore diets rich in saturated fatty acids (SFA) and monounsaturated fatty acids (MUFA) improve long-chain polyunsaturated fatty acid (LC-PUFA) metabolism efficiency (Xu et al., 2014; Emery et al., 2016). The diet enriched with S. limacinum had a lower MUFA content due to reduced inclusion of canola oil. Together with a lower digestibility of palmitic acid, which is present in high concentrations in Schizochytrium (Kousoulaki et al., 2020; Hart et al., 2021), this could have led to an overall lower fat content in the muscle of fish fed with this microalgae. Although palmitic acid was enriched in the diet containing S. limacinum, it was only slightly increased in the muscle at the end of the trial and its metabolic fate requires more attention in future studies. Muscle fat content was also reduced in salmon fed A. platensis for eight weeks in our study and Arthrospira platensis has been found to reduce hyperlipidaemia in the rat model (Hua et al., 2018; Li et al., 2019). However, no effect was detected in our study on cholesterol levels in plasma, which has been described by Hua et al. (2018).
Seawater transfer results in increased energy demands and coupled with reduced feed intake caused a significant reduction in the muscle fat content, primarily in the relative abundance of specific fatty acids of salmon smolts (Woo et al., 1978; Sheridan, 1989; Usher et al., 1991). Since monounsaturated fatty acids are preferentially used as metabolic fuel (Henderson, 1996) polyunsaturated fatty acids such as DHA were protected from being metabolized and not oxidized during the early time window in seawater. Hence, relative abundance of DHA in the muscle of all groups increased significantly after transfer to seawater and was linearly related to the decrease in total fat content of the muscle.
Besides polyunsaturated fatty acids also pigments are important in maintaining health and immune function in fish (de Carvalho & Caramujo, 2017). Lutein, the dominating pigment present in all muscle samples, was found to improve growth and antioxidant status of whiteleg shrimp Litopenaeus vannamei (Fang et al., 2021) and improve survival of goldfish Carassius auratus (Besen et al., 2019). Differences in the carotenoid profile among the diet groups were directly related to its feed origin, with lutein found in highest concentrations in fish fed broken C. vulgaris, followed by intact C. vulgaris and T. chuii. Sørensen et al. (2023) also found high concentrations of lutein in the muscle of salmon fed T. chuii biomass, but in contrast to their study, we did not detect any astaxanthin in the muscle samples. This could be explained by the lower inclusion of fishmeal in our diet, which is a natural source of astaxanthin (Lim et al., 2018) and further by a difference in the pigment profile of the used microalgae products. Lutein concentrations in the muscle of the salmon in our study were linearly related to total carotenoid concentration found in plasma samples, which could be caused by a dynamic equilibrium of carotenoids between the bloodstream and muscle. However, it could also imply that fish that accumulated more carotenoids over the entire trial also ingested more during the days before sampling. Aside from lutein, the xanthophylls zeaxanthin and violaxanthin were present in the muscle of fish fed A. platensis and T. chuii respectively. All detected carotenoids have important functions in maintaining eye-health in mammals (Giordano and Quadro, 2018) and could be investigated for the prevention of eye health disorders such as cataract in fish, a common problem in salmonid aquaculture (Waagbø et al., 2003; Bjerkås and Sveier, 2004).
While several studies have investigated health and immune effects of microalgae in fish, detected responses seem to depend on both microalgae species as well as fish species investigated (Table 6). We detected microalgae specific influences on health and immunity in Atlantic salmon at both local (intestine) and systemic (plasma, liver, spleen) scales. Adding broken C. vulgaris to the diet in this study lowered aspartate aminotransferase and alanine aminotransferase activity levels in the salmon plasma which may indicate improved liver health. Activity of these two enzymes is primarily considered an indicator of liver damage, as higher levels result from destructed or damaged liver cells (Huang et al., 2006). A meta-analysis on the effect of Chlorella supplementation on liver health in humans found an overall reduction of aspartate aminotransferase levels, while no effect was detected on alanine aminotransferase serum levels (Yarmohammadi et al., 2021).
Oxidative stress resulting from the increased formation of reactive oxygen species was shown to be reduced by the dietary intake of antioxidants in various fish species (reviewed by Hoseinifar et al., 2021). Superoxide dismutase 1, which catalyses the breakdown of superoxide radicals (Fukai and Ushio-Fukai, 2011), was induced in salmon fed S. limacinum after two weeks of feeding the diets. High amounts of DHA from S. limacinum are prone to peroxidation resulting in the formation of 4-hydroxyhexenal (4-HHE) which in turn can activate the Nrf2 antioxidant pathway inducing expression of sod (Yang et al., 2019). Several studies with mammalian cell lines have indicated that DHA is able to increase GSH content (Arab et al., 2006) as well as intracellular Sod and Gpx concentrations (Clementi et al., 2019). Our results indicate an early effect of the diet; however, protein levels after eight weeks were similar to the control diet. This suggests that beneficial effects of a functional diet may change over administration time and the potential temporal “habituation effect” requires more attention in future studies. Myeloperoxidase is a characteristic enzyme of neutrophil granulocytes which is involved in the oxidative burst response, where it catalyses the oxidation of chloride ions (Klebanoff, 1999; Frijhoff et al., 2015). It has been further associated with inflammatory processes and various diseases in humans (Davies & Hawkins, 2020). Reduced protein concentrations in the liver of salmon fed A. platensis could be caused by a lower abundance of neutrophil granulocytes in the liver, indicating no acute inflammatory response of the liver. Granulocytes in the blood of Nile tilapia Oreochromis niloticus fed diets containing different levels of A. platensis decreased strongly after 12 weeks of feeding (Abdel-Tawwab and Ahmad, 2009), but no inferences about the concentration or activity of Mpo has been made in this study.
The liver furthermore produces acute phase proteins and levels of transcripts encoding the acute phase protein serum amyloid A (saa5) were downregulated in fish fed broken C. vulgaris and S. limacinum. This highlights the potential anti-inflammatory role of these microalgae with described effects of their main chemical components in the literature; lutein in case of C. vulgaris (Chung et al., 2017; Demmig-Adams et al., 2020) and DHA in case of S. limacinum (Li et al., 2005; Mullen et al., 2010).
However, microalgae addition increased the expression of c1ql2 in the liver and spleen. C1ql2 is closely related to the first subcomponent of the complement system and might be therefore involved in the response to a variety of environmental conditions in Atlantic salmon (Krasnov et al., 2020; Beemelmanns et al., 2021). The precise function of c1ql2 is still unknown (Lao et al., 2008; Köbis et al., 2017). In addition to c1ql2, the upregulated levels of transcripts coding for the anti-microbial peptide hepcidin (hamp) and lysozyme (lyzc2) in the liver indicate a potentially enhanced anti-microbial defense by microalgae-enriched diets (Messina et al., 2019; García-Márquez et al., 2020).
Functional feed additives are thought to modulate the local immune response in the intestine and several of the investigated genes in the anterior intestine were modulated by the diet. The increased expression of drtp1 in all microalgae supplemented groups may be linked to a general response of the intestine towards novel antigens in the diet, but was also found to be induced after an acute phase response (Martin et al., 2006; Talbot et al., 2009). Interferon-stimulated gene 15 (isg15) was upregulated in the anterior intestine in response to the microalgae diets. This gene is induced by type 1 interferon and acts like a cytokine (Perng & Lenschow, 2018). Interestingly, other cytokine receptors, namely interleukin 1 receptor (il1r2) as well as interleukin 10 receptor (il10rb) were downregulated in most microalgae diets. This may indicate a reduced sensitivity of the intestine towards pro-inflammatory signals or a general reduction of pro-inflammatory signals present in the intestine. Grammes et al. (2013) showed that including Chlorella vulgaris in the diet had anti-inflammatory action and protected Atlantic salmon from developing a soybean meal-induced enteritis (SBMIE). In zebrafish Danio rerio feeding diets with PUFA-rich microalgae increased the expression of the anti-inflammatory cytokine il10 (Nayak et al., 2020). Interference of the diet with pro-inflammatory signaling was also detected in the spleen where expression of NFKB inhibitor alpha (ikba; Wang et al., 2009) was reduced in fish fed intact C. vulgaris.
The future of using microalgae as a functional feed ingredient largely depends on its production cost and economic benefits when incorporated into diets for Atlantic salmon. Although we investigated microalgae which are already cultivated at commercial scale, the current price (~20 – 30€ per kg) permits its use at higher inclusion levels only in restricted time periods. These might be during the production of juveniles, where feed costs are generally lower or in the final stage of production. Enhancing the product quality before slaughtering by increasing the fillet DHA and carotenoid content, with known benefits for human health can represent an economically viable strategy which should be further explored.
Conclusion
Our study revealed that microalgae addition of 8% to the diet could have positive effects on the health of Atlantic salmon reared in RAS without affecting its growth performance. We confirmed the transfer of important functional components of microalgae (polyunsaturated fatty acids and pigments) into the fish muscle, but the role and function of many of the functional compounds present in microalgae remains elusive and needs further investigation. Our results further indicate that microalgae enriched diets induce a local anti-inflammatory response in the intestine, improve oxidative stress response and stimulate complement and antibacterial responses in liver and spleen. Based on our comprehensive data, we encourage future studies to provide a holistic view on the health status of fish when evaluating functional feeds in aquaculture and investigate the use of microalgae enriched diets in other economically important production phases.
Data availability statement
The raw data supporting the conclusions of this article will be made available by the authors, without undue reservation.
Ethics statement
The experiment was approved by the animal welfare officer of the “Fraunhofer IMTE Büsum” and the local authority of Schleswig-Holstein, according to the German animal welfare law (NTP – ID 00043858-1-0). The study was conducted in accordance with the local legislation and institutional requirements.
Author contributions
JMu: Conceptualization, Data curation, Formal Analysis, Investigation, Visualization, Writing – original draft. MP: Data curation, Investigation, Writing – review & editing. JMo: Data curation, Methodology, Writing – review & editing. UO: Data curation, Methodology, Writing – review & editing. DRvM: Data curation, Writing – review & editing. AR: Data curation, Writing – review & editing. TG: Funding acquisition, Project administration, Writing – review & editing. JL: Writing – review & editing, Data curation, Methodology. TS: Data curation, Methodology, Writing – review & editing. HS: Conceptualization, Supervision, Writing – review & editing. CS: Conceptualization, Funding acquisition, Project administration, Supervision, Writing – review & editing.
Funding
The author(s) declare financial support was received for the research, authorship, and/or publication of this article. This work was funded by the BMBF within the project BioFiA (Project number 031B0915) and the project AQUATOR (Project number 031B0915H3). We furthermore acknowledge financial support by Land Schleswig-Holstein within the funding programme Open Access Publikationsfonds.
Acknowledgments
We are grateful to Michael Schlachter for his advice on experimental design and help during sampling and Petra Rettmann for assistance in the lab. Moreover, we acknowledge laboratory assistance at Max Rubner-Institut by Isabel Delgado, Iris Bagge, Frauke Grönwoldt, Annette Hollmann, Birte Fischer-Kassebart, Aaron Knappe and Martina Netzel, as well as by Julian Krinitskij at FBN. We thank Mario Hasler for statistical advice. We would further like to thank all colleagues and staff from Fraunhofer IMTE Büsum for their help during the experiment.
Conflict of interest
The authors declare that the research was conducted in the absence of any commercial or financial relationships that could be construed as a potential conflict of interest.
Publisher’s note
All claims expressed in this article are solely those of the authors and do not necessarily represent those of their affiliated organizations, or those of the publisher, the editors and the reviewers. Any product that may be evaluated in this article, or claim that may be made by its manufacturer, is not guaranteed or endorsed by the publisher.
Supplementary material
The Supplementary Material for this article can be found online at: https://www.frontiersin.org/articles/10.3389/fmars.2023.1273614/full#supplementary-material
References
Abdel-Tawwab M., Ahmad M. H. (2009). Live Spirulina (Arthrospira platensis) as a growth and immunity promoter for Nile tilapia, Oreochromis niloticus (L.), challenged with pathogenic Aeromonas hydrophila. Aquaculture Res. 40 (9), 1037–1046. doi: 10.1111/j.1365-2109.2009.02195.x
Abdel-Tawwab M., Mousa M. A. A., Mamoon A., Abdelghany M. F., Abdel-Hamid E. A. A., Abdel-Razek N., et al. (2022). Dietary Chlorella vulgaris modulates the performance, antioxidant capacity, innate immunity, and disease resistance capability of Nile tilapia fingerlings fed on plant-based diets. Anim. Feed Sci. Technol. 283, 115181. doi: 10.1016/j.anifeedsci.2021.115181
Ahmed N., Turchini G. M. (2021). Recirculating aquaculture systems (RAS): Environmental solution and climate change adaptation. J. Cleaner Production 297, 126604. doi: 10.1016/j.jclepro.2021.126604
Arab K., Rossary A., Flourié F., Tourneur Y., Steghens J.-P. (2006). Docosahexaenoic acid enhances the antioxidant response of human fibroblasts by upregulating γ-glutamyl-cysteinyl ligase and glutathione reductase. Br. J. Nutr. 95 (1), 18–26. doi: 10.1079/bjn20051626
Bae J., Hamidoghli A., Won S., Choi W., Lim S. G., Kim K. W., et al. (2020). Evaluation of seven different functional feed additives in a low fish meal diet for olive flounder. Paralichthys olivaceus. Aquaculture 525, 735333. doi: 10.1016/j.aquaculture.2020.735333
Bayne C. J., Gerwick L., Fujiki K., Nakao M., Yano T. (2001). Immune-relevant (including acute phase) genes identified in the livers of rainbow trout, Oncorhynchus mykiss, by means of suppression subtractive hybridization. Dev. Comp. Immunol. 25 (3), 205–217. doi: 10.1016/S0145-305X(00)00057-4
Beemelmanns A., Zanuzzo F. S., Xue X., Sandrelli R. M., Rise M. L., Gamperl A. K. (2021). The transcriptomic responses of Atlantic salmon (Salmo salar) to high temperature stress alone, and in combination with moderate hypoxia. BMC Genomics 22, 261. doi: 10.1186/s12864-021-07464-x
Bell J. G., Dick J. R., McVicar A. H., Sargent J. R., Thompson K. D. (1993). Dietary sunflower, linseed and fish oils affect phospholipid fatty acid composition, development of cardiac lesions, phospholipase activity and eicosanoid production in Atlantic salmon (Salmo salar). Prostaglandins Leukotrienes Essential Fatty Acids 49 (3), 665–673. doi: 10.1016/0952-3278(93)90075-8
Bell J., McEvoy J., Tocher D. R., McGhee F., Campbell P. J., Sargent J. R. (2001). Replacement of fish oil with rapeseed oil in diets of atlantic salmon (Salmo salar) affects tissue lipid compositions and hepatocyte fatty acid metabolism. J. Nutr. 131 (5), 1535–1543. doi: 10.1093/jn/131.5.1535
Bell J., Tocher D. R., Henderson R. J., Dick J. R., Crampton V. O. (2003). Altered fatty acid compositions in atlantic salmon (Salmo salar) fed diets containing linseed and rapeseed oils can be partially restored by a subsequent fish oil finishing diet. J. Nutr. 133 (9), 2793–2801. doi: 10.1093/jn/133.9.2793
Bergheim A., Drengstig A., Ulgenes Y., Fivelstad S. (2009). Production of Atlantic salmon smolts in Europe-Current characteristics and future trends. Aquacultural Eng. 41 (2), 46–52. doi: 10.1016/j.aquaeng.2009.04.004
Besen K. P., Melim E. W. H., da Cunha L., Favaretto E. D., Moreira M., Fabregat T. E. H. P. (2019). Lutein as a natural carotenoid source: Effect on growth, survival and skin pigmentation of goldfish juveniles (Carassius auratus). Aquaculture Res. 50 (8), 2200–2206. doi: 10.1111/are.14101
Bjerkås E., Sveier H. (2004). The influence of nutritional and environmental factors on osmoregulation and cataracts in Atlantic salmon (Salmo salar L). Aquaculture 235 (1–4), 101–122. doi: 10.1016/j.aquaculture.2003.10.005
Bou M., Berge G. M., Baeverfjord G., Sigholt T., Ostbye T. K., Romarheim O. H., et al. (2017). Requirements of n-3 very long-chain PUFA in Atlantic salmon (Salmo salar L): Effects of different dietary levels of EPA and DHA on fish performance and tissue composition and integrity. Br. J. Nutr. 117 (1), 30–47. doi: 10.1017/S0007114516004396
Bravo-Tello K., Ehrenfeld N., Solís C. J., Ulloa P. E., Hedrera M., Pizarro-Guajardo M., et al. (2017). Effect of microalgae on intestinal inflammation triggered by soybean meal and bacterial infection in zebrafish. PloS One 12 (11), e0187696. doi: 10.1371/journal.pone.0187696
Caballero M. J., Obach A., Rosenlund G., Montero D., Gisvold M., Izquierdo M. S. (2002). Impact of different dietary lipid sources on growth, lipid digestibility, tissue fatty acid composition and histology of rainbow trout, Oncorhynchus mykiss. Aquaculture 214 (1–4), 253–271. doi: 10.1016/S0044-8486(01)00852-3
Calabrese S., Nilsen T. O., Kolarevic J., Ebbesson L. O. E., Pedrosa C., Fivelstad S., et al. (2017). Stocking density limits for post-smolt Atlantic salmon (Salmo salar L.) emphasis on production performance and welfare. Aquaculture 468, 363–370. doi: 10.1016/j.aquaculture.2016.10.041
Carballo C., Chronopoulou E. G., Letsiou S., Maya C., Labrou N. E., Infante C., et al. (2018). Antioxidant capacity and immunomodulatory effects of a chrysolaminarin-enriched extract in Senegalese sole. Fish & Shellfish Immunology 82, 1–8. doi: 10.1016/J.FSI.2018.07.052
Carvalho M., Montero D., Rosenlund G., Fontanillas R., Ginés R., Izquierdo M. (2020). Effective complete replacement of fish oil by combining poultry and microalgae oils in practical diets for gilthead sea bream (Sparus aurata) fingerlings. Aquaculture 529, 735696. doi: 10.1016/j.aquaculture.2020.735696
Cerezuela R., Guardiola F. A., González P., Meseguer J., Esteban M.Á. (2012a). Effects of dietary Bacillus subtilis, Tetraselmis chuii, and Phaeodactylum tricornutum, singularly or in combination, on the immune response and disease resistance of sea bream (Sparus aurata L.). Fish Shellfish Immunol. 33 (2), 342–349. doi: 10.1016/j.fsi.2012.05.004
Cerezuela R., Guardiola F. A., Meseguer J., Esteban M. Á. (2012b). Enrichment of gilthead seabream (Sparus aurata L.) diet with microalgae: Effects on the immune system. Fish Physiol. Biochem. 38 (6), 1729–1739. doi: 10.1007/s10695-012-9670-9
Cerezuela R., Meseguer J., Esteban M.Á. (2013). Effects of dietary inulin, Bacillus subtilis and microalgae on intestinal gene expression in gilthead seabream (Sparus aurata L.). Fish Shellfish Immunol. 34 (3), 843–848. doi: 10.1016/j.fsi.2012.12.026
Chen W., Luo L., Han D., Long F., Chi Q., Hu Q. (2021). Effect of dietary supplementation with Chlorella sorokiniana meal on the growth performance, antioxidant status, and immune response of rainbow trout (Oncorhynchus mykiss). J. Appl. Phycology 33 (5), 3113–3122. doi: 10.1007/s10811-021-02541-w
Cherng J. Y., Shih M. F. (2005). Preventing dyslipidemia by Chlorella pyrenoidosa in rats and hamsters after chronic high fat diet treatment. Life Sci. 76 (26), 3001–3013. doi: 10.1016/j.lfs.2004.10.055
Chung R. W. S., Leanderson P., Lundberg A. K., Jonasson L. (2017). Lutein exerts anti-inflammatory effects in patients with coronary artery disease. Atherosclerosis 262, 87–93. doi: 10.1016/J.ATHEROSCLEROSIS.2017.05.008
Clementi M. E., Lazzarino G., Sampaolese B., Brancato A., Tringali G. (2019). DHA protects PC12 cells against oxidative stress and apoptotic signals through the activation of the NFE2L2/HO-1 axis. Int. J. Mol. Med. 43 (6), 2523–2531. doi: 10.3892/ijmm.2019.4170
Dalsgaard J., Lund I., Thorarinsdottir R., Drengstig A., Arvonen K., Pedersen P. B. (2013). Farming different species in RAS in Nordic countries: Current status and future perspectives. Aquacultural Eng. 53, 2–13. doi: 10.1016/j.aquaeng.2012.11.008
Davidson J., Summerfelt S., Espmark Å. M. O., Mota V. C., Marancik D., Earley R. L., et al. (2021). Effects of ozone on post-smolt Atlantic salmon (Salmo salar) performance, health, and maturation in freshwater recirculation aquaculture systems. Aquaculture 533, 736208. doi: 10.1016/j.aquaculture.2020.736208
Davies M. J., Hawkins C. L. (2020). The role of myeloperoxidase in biomolecule modification, chronic inflammation, and disease. Antioxidants Redox Signaling 32 (13), 957–981. doi: 10.1089/ars.2020.8030
de Carvalho C. C. C. R., Caramujo M. J. (2017). Carotenoids in aquatic ecosystems and aquaculture: A colorful business with implications for human health. Front. Mar. Sci. 4 (APR). doi: 10.3389/fmars.2017.00093
de Mattos B. O., López-Olmeda J. F., Guerra-Santos B., Ruiz C. E., García-Beltrán J. M., Ángeles-Esteban M., et al. (2019). Coping with exposure to hypoxia: modifications in stress parameters in gilthead seabream (Sparus aurata) fed spirulina (Arthrospira platensis) and brewer’s yeast (Saccharomyces cerevisiae). Fish Physiol. Biochem. 45 (6), 1801–1812. doi: 10.1007/s10695-019-00677-8
Demmig-Adams B., López-Pozo M., Stewart J. J., Adams W. W. (2020). Zeaxanthin and lutein: photoprotectors, anti-inflammatories, and brain food. Molecules 25 (16), 3607. doi: 10.3390/MOLECULES25163607
de Souza F. P., de Lima E. C. S., Urrea-Rojas A. M., Suphoronski S. A., Facimoto C. T., da Silva Bezerra J., et al. (2020). Effects of dietary supplementation with a microalga (Schizochytrium sp.) on the hemato-immunological, and intestinal histological parameters and gut microbiota of Nile tilapia in net cages. PloS One 15 (1), 1–19. doi: 10.1371/journal.pone.0226977
Domozych D. S., Ciancia M., Fangel J. U., Mikkelsen M. D., Ulvskov P., Willats W. G. T. (2012). The cell walls of green algae: A journey through evolution and diversity. Front. Plant Sci. 3 (MAY). doi: 10.3389/fpls.2012.00082
Donaldson M. (2012). Development of a rapid, simple assay of plasma total carotenoids. BMC Res. Notes 5 (1), 1–8. doi: 10.1186/1756-0500-5-521/FIGURES/5
Ebrahimi-Mameghani M., Aliashrafi S., Javadzadeh Y., AsghariJafarabadi M. (2014). The effect of chlorella vulgaris supplementation on liver en-zymes, serum glucose and lipid profile in patients with non-alcoholic fatty liver disease. Health Promotion Perspect. 4 (1), 107–115. doi: 10.5681/hpp.2014.014
Emery J. A., Norambuena F., Trushenski J., Turchini G. M. (2016). Uncoupling EPA and DHA in fish nutrition: dietary demand is limited in atlantic salmon and effectively met by DHA alone. Lipids 51 (4), 399–412. doi: 10.1007/s11745-016-4136-y
Fan J., Bao Q., Ma K., Li X., Jia J., Wu H. (2022). Antioxidant and innate immunity of Danio rerio against Edwardsiella tarda in response to diets including three kinds of marine microalgae. Algal Res. 64, 102689. doi: 10.1016/j.algal.2022.102689
Fang H. H., He X. S., Zeng H. L., Liu Y. J., Tian L. X., Niu J. (2021). Replacement of astaxanthin with lutein in diets of juvenile litopenaeus vannamei: effects on growth performance, antioxidant capacity, and immune response. Front. Mar. Sci. 8. doi: 10.3389/fmars.2021.803748
Frijhoff J., Winyard P. G., Zarkovic N., Davies S. S., Stocker R., Cheng D., et al. (2015). Clinical relevance of biomarkers of oxidative stress. Antioxidants Redox Signaling 23 (14), 1144–1170. doi: 10.1089/ars.2015.6317
Fujii R. (2000). The regulation of motile activity in fish chromatophores. Pigment Cell Res. / Sponsored by Eur. Soc. Pigment Cell Res. Int. Pigment Cell Soc. 13, 300–319. doi: 10.1034/j.1600-0749.2000.130502.x
Fukai T., Ushio-Fukai M. (2011). Superoxide dismutases: role in redox signaling, vascular function, and diseases. Antioxidants Redox Signaling 15 (6), 1583–1606. doi: 10.1089/ars.2011.3999
García-Márquez J., Rico R. M., Sánchez-Saavedra M., del P., Gómez-Pinchetti J. L., Acién F. G., et al. (2020). A short pulse of dietary algae boosts immune response and modulates fatty acid composition in juvenile Oreochromis niloticus. Aquaculture Res. 51 (11), 4397–4409. doi: 10.1111/are.14781
Giordano E., Quadro L. (2018). Lutein, zeaxanthin and mammalian development: Metabolism, functions and implications for health. Arch. Biochem. Biophysics 647, 33–40. doi: 10.1016/j.abb.2018.04.008
Glencross B. D., Tocher D. R., Matthew C., Gordon Bell J. (2014). Interactions between dietary docosahexaenoic acid and other long-chain polyunsaturated fatty acids on performance and fatty acid retention in post-smolt Atlantic salmon (Salmo salar). Fish Physiol. Biochem. 40 (4), 1213–1227. doi: 10.1007/s10695-014-9917-8
Gong Y., Sørensen S. L., Dahle D., Nadanasabesan N., Dias J., Valente L. M. P., et al. (2020). Approaches to improve utilization of Nannochloropsis oceanica in plant-based feeds for Atlantic salmon. Aquaculture 522 (February), 735122. doi: 10.1016/j.aquaculture.2020.735122
Grammes F., Reveco F. E., Romarheim O. H., Landsverk T., Mydland L. T., Øverland M. (2013). Candida utilis and Chlorella vulgaris counteract intestinal inflammation in Atlantic salmon (Salmo salar L.). PloS One 8 (12). doi: 10.1371/journal.pone.0083213
Griffiths M. J., Van Hille R. P., Harrison S. T. L. (2010). Selection of direct transesterification as the preferred method for assay of fatty acid content of microalgae. Lipids 45 (11), 1053–1060. doi: 10.1007/s11745-010-3468-2
Guzmán F, Wong G, Román T, Cárdenas C, Alvárez C, Schmitt P, et al. (2019). Identification of Antimicrobial Peptides from the Microalgae Tetraselmis suecica (Kylin) Butcher and Bactericidal Activity Improvement. Marine Drugs 17 (8), 453. doi: 10.3390/md17080453
Guzmán S., Gato A., Lamela M., Freire-Garabal M., Calleja J. M. (2003). Anti-inflammatory and immunomodulatory activities of polysaccharide from Chlorella stigmatophora and Phaeodactylum tricornutum. Phytotherapy Res. 17 (6), 665–670. doi: 10.1002/ptr.1227
Habte-Tsion H. M., Kolimadu G. D., Rossi W., Filer K., Kumar V. (2020). Effects of Schizochytrium and micro-minerals on immune, antioxidant, inflammatory and lipid-metabolism status of Micropterus salmoides fed high- and low-fishmeal diets. Sci. Rep. 10 (1), 1–13. doi: 10.1038/s41598-020-64286-9
Hart B., Schurr R., Narendranath N., Kuehnle A., Colombo S. M. (2021). Digestibility of Schizochytrium sp. whole cell biomass by Atlantic salmon (Salmo salar). Aquaculture 533, 736156. doi: 10.1016/j.aquaculture.2020.736156
Hasler M., Hothorn L. A. (2008). Multiple contrast tests in the presence of heteroscedasticity. Biometrical Journal: J. Math. Methods Biosci. 50 (5), 793–800. doi: 10.1002/bimj.200710466
Henderson R. J. (1996). Fatty acid metabolism in freshwater fish with particular reference to polyunsaturated fatty acids. Arch. Anim. Nutr. 49 (1), 5–22. doi: 10.1080/17450399609381859
Hoseinifar S. H., Yousefi S., Van Doan H., Ashouri G., Gioacchini G., Maradonna F., et al. (2021). Oxidative stress and antioxidant defense in fish: the implications of probiotic, prebiotic, and synbiotics. Rev. Fisheries Sci. Aquaculture 29 (2), 198–217. doi: 10.1080/23308249.2020.1795616
Hua P., Yu Z., Xiong Y., Liu B., Zhao L. (2018). Regulatory efficacy of spirulina platensis protease hydrolyzate on lipid metabolism and gut microbiota in high-fat diet-fed rats. Int. J. Mol. Sci. 19 (12), 4023. doi: 10.3390/ijms19124023
Huang X. J., Choi Y. K., Im H. S., Yarimaga O., Yoon E., Kim H. S. (2006). Aspartate aminotransferase (AST/GOT) and alanine aminotransferase (ALT/GPT) detection techniques. Sensors 6 (7), 756–782. doi: 10.3390/s6070756
ISO-IDF. (2002). “Milk fat-Preparation of fatty acid methyl esters,” in International standard ISO 15884-IDF 182:2002 (Brussels: International Dairy Federation).
Karl H., Oehlenschläger J., Bekaert K., Bergé J. P., Cadun A., Duflos G., et al. (2012). WEFTA interlaboratory comparison on total lipid determination in fishery products using the Smedes method. J. AOAC Int. 95 (2), 489–493. doi: 10.5740/jaoacint.11-041
Karlsen C., Ytteborg E., Timmerhaus G., Høst V., Handeland S., Jørgensen S. M., et al. (2018). Atlantic salmon skin barrier functions gradually enhance after seawater transfer. Sci. Rep. 8 (1), 1–12. doi: 10.1038/s41598-018-27818-y
Khalil S. R., Reda R. M., Awad A. (2017). Efficacy of Spirulina platensis diet supplements on disease resistance and immune-related gene expression in Cyprinus carpio L. exposed to herbicide atrazine. Fish Shellfish Immunol. 67, 119–128. doi: 10.1016/j.fsi.2017.05.065
Kiron V., Phromkunthong W., Huntley M., Archibald I., De Scheemaker G. (2012). Marine microalgae from biorefinery as a potential feed protein source for Atlantic salmon, common carp and whiteleg shrimp. Aquaculture Nutr. 18 (5), 521–531. doi: 10.1111/j.1365-2095.2011.00923.x
Klebanoff S. J. (1999). Myeloperoxidase. Proc. Assoc. Am. Physicians 111 (5), 383–389. doi: 10.1111/paa.1999.111.5.383
Köbis J. M., Rebl H., Goldammer T., Rebl A. (2017). Multiple gene and transcript variants encoding trout C-polysaccharide binding proteins are differentially but strongly induced after infection with Aeromonas salmonicida. Fish Shellfish Immunol. 60, 509–519. doi: 10.1016/j.fsi.2016.11.021
Kousoulaki K., Berge G. M., Mørkøre T., Krasnov A., Baeverfjord G., Ytrestøyl T., et al. (2020). Microalgal schizochytrium limacinum biomass improves growth and filet quality when used long-term as a replacement for fish oil, in modern salmon diets. Front. Mar. Sci. 7 (February). doi: 10.3389/fmars.2020.00057
Krasnov A., Afanasyev S., Nylund S., Rebl A. (2020). Multigene expression assay for assessment of the immune status of Atlantic Salmon. Genes 11 (11), 1–11. doi: 10.3390/genes11111236
Kulczykowska E., Sánchez Vázquez F. J. (2010). Neurohormonal regulation of feed intake and response to nutrients in fish: aspects of feeding rhythm and stress. Aquaculture Res. 41 (5), 654–667. doi: 10.1111/J.1365-2109.2009.02350.X
Laird N. M., Ware J. H. (1982). Random-effects models for longitudinal data. Biometrics 38 (4), 963–974. doi: 10.2307/2529876
Lao H.-H., Sun Y.-N., Yin Z.-X., Wang J., Chen C., Weng S.-P., et al. (2008). Molecular cloning of two C1q-like cDNAs in mandarin fish Siniperca chuatsi. Veterinary Immunol. Immunopathology 125 (1), 37–46. doi: 10.1016/j.vetimm.2008.05.004
Li H., Ruan X. Z., Powis S. H., Fernando R., Mon W. Y., Wheeler D. C., et al. (2005). EPA and DHA reduce LPS-induced inflammation responses in HK-2 cells: Evidence for a PPAR-γ–dependent mechanism. Kidney Int. 67 (3), 867–874. doi: 10.1111/J.1523-1755.2005.00151.X
Li T. T., Tong A. J., Liu Y. Y., Huang Z. R., Wan X. Z., Pan Y. Y., et al. (2019). Polyunsaturated fatty acids from microalgae Spirulina platensis modulates lipid metabolism disorders and gut microbiota in high-fat diet rats. Food and Chemical Toxicology. 131 110558. doi: 10.1016/J.FCT.2019.06.005
Lim K. C., Yusoff F. M., Shariff M., Kamarudin M. S. (2018). Astaxanthin as feed supplement in aquatic animals. Rev Aquacult 10, 738–773. doi: 10.1111/raq.12200
López Nadal A., Ikeda-Ohtsubo W., Sipkema D., Peggs D., McGurk C., Forlenza M., et al. (2020). Feed, microbiota, and gut immunity: using the zebrafish model to understand fish health. Front. Immunol. 11 (February). doi: 10.3389/fimmu.2020.00114
Lund H., Bakke A., Boysen P., Afanasyev S., Rebl A., Manji F., et al. (2022). Evaluation of immune status in two cohorts of atlantic salmon raised in different aquaculture systems (Case study). Genes 13 (5), 736. doi: 10.3390/genes13050736
Luo Z., Ye H. M., Gao Y., Ling S. C., Wei C. C., Zhu X. (2018). Chlorella additive increased growth performance, improved appetite and immune response of juvenile crucian carp Carassius auratus. Aquaculture Res. 49 (10), 3329–3337. doi: 10.1111/are.13797
Ma K., Chen S., Wu Y., Ma Y., Qiao H., Fan J., et al. (2022). Dietary supplementation with microalgae enhances the zebrafish growth performance by modulating immune status and gut microbiota. Appl. Microbiol. Biotechnol. 106 (2), 773–788. doi: 10.1007/s00253-021-11751-8
Mahmoud E. A., El-Sayed B. M., Mahsoub Y. H., El-Murr A., Neamat-Allah A. N. F. (2020). Effect of Chlorella vulgaris enriched diet on growth performance, hemato-immunological responses, antioxidant and transcriptomics profile disorders caused by deltamethrin toxicity in Nile tilapia (Oreochromis niloticus). Fish & Shellfish Immunology 102, 422–429. doi: 10.1016/j.fsi.2020.04.061
Martin S. A. M., Blaney S. C., Houlihan D. F., Secombes C. J. (2006). Transcriptome response following administration of a live bacterial vaccine in Atlantic salmon (Salmo salar). Mol. Immunol. 43 (11), 1900–1911. doi: 10.1016/j.molimm.2005.10.007
Messina M., Bulfon C., Beraldo P., Tibaldi E., Cardinaletti G. (2019). Intestinal morpho-physiology and innate immune status of European sea bass (Dicentrarchus labrax) in response to diets including a blend of two marine microalgae, Tisochrysis lutea and Tetraselmis suecica. Aquaculture 500(April 2018), 660–669. doi: 10.1016/j.aquaculture.2018.09.054
Molina-Roque L., Bárany A., Sáez M. I., Alarcón F. J., Tapia S. T., Fuentes J., et al. (2022). Biotechnological treatment of microalgae enhances growth performance, hepatic carbohydrate metabolism and intestinal physiology in gilthead seabream (Sparus aurata) juveniles close to commercial size. Aquaculture Rep. 25, 101248. doi: 10.1016/j.aqrep.2022.101248
Mullen A., Loscher C. E., Roche H. M. (2010). Anti-inflammatory effects of EPA and DHA are dependent upon time and dose-response elements associated with LPS stimulation in THP-1-derived macrophages. J. Nutr. Biochem. 21 (5), 444–450. doi: 10.1016/J.JNUTBIO.2009.02.008
Nagel F., von Danwitz A., Tusche K., Kroeckel S., van Bussel C. G. J., Schlachter M., et al. (2012). Nutritional evaluation of rapeseed protein isolate as fish meal substitute for juvenile turbot (Psetta maxima L.) — Impact on growth performance, body composition, nutrient digestibility and blood physiology. Aquaculture 356–357, 357–364. doi: 10.1016/J.AQUACULTURE.2012.04.045
Nakagawa S., Schielzeth H. (2013). A general and simple method for obtaining R2 from generalized linear mixed-effects models. Methods Ecol. Evol. 4 (2), 133–142. doi: 10.1111/j.2041-210x.2012.00261.x
Nakahara T., Yokochi T., Higashihara T., Tanaka S., Yaguchi T., Honda D. (1996). Production of docosahexaenoic and docosapentaenoic acids by Schizochytrium sp. isolated from yap islands. JAOCS J. Am. Oil Chemists’ Soc. 73 (11), 1421–1426. doi: 10.1007/BF02523506
Nayak S., Al Ashhab A., Zilberg D., Khozin-Goldberg I. (2020). Dietary supplementation with omega-6 LC-PUFA-rich microalgae regulates mucosal immune response and promotes microbial diversity in the zebrafish gut. Biology 9 (6), 119. doi: 10.3390/biology9060119
National Research Council (2011). Nutrient Requirements of Fish and Shrimp. (Washington, DC: National Academies Press). doi: 10.17226/13039
Ostermeyer U., Schmidt T. (2004). Differentiation of wild salmon, conventionally and organically farmed salmon. Deutsche Lebensmittel-Rundschau 100, 437–444.
Perera E., Sánchez-Ruiz D., Sáez M. I., Galafat A., Barany A., Fernández-Castro M., et al. (2020). Low dietary inclusion of nutraceuticals from microalgae improves feed efficiency and modifies intermediary metabolisms in gilthead sea bream (Sparus aurata). Sci. Rep. 10, 18676. doi: 10.1038/s41598-020-75693-3
Perng Y. C., Lenschow D. J. (2018). ISG15 in antiviral immunity and beyond. Nat. Rev. Microbiol. 16 (7), 423–439. doi: 10.1038/s41579-018-0020-5
Pinheiro J. C., Bates D. M. (2000). Linear mixed-effects models: basic concepts and examples. Mixed-Effects Models S S-Plus, 3–56. doi: 10.1007/978-1-4419-0318-1_1
Rahimnejad S., Lee S. M., Park H. G., Choi J. (2017). Effects of Dietary Inclusion of Chlorella vulgaris on Growth, Blood Biochemical Parameters, and Antioxidant Enzyme Activity in Olive Flounder, Paralichthys olivaceus. J. World Aquaculture Soc. 48 (1), 103–112. doi: 10.1111/jwas.12320
Raji A. A., Alaba P. A., Yusuf H., Abu Bakar N. H., Mohd Taufek N., Muin H., et al. (2018). Fishmeal replacement with Spirulina Platensis and Chlorella vulgaris in African catfish (Clarias gariepinus) diet: Effect on antioxidant enzyme activities and haematological parameters. Res. Veterinary Sci. 119 (April), 67–75. doi: 10.1016/j.rvsc.2018.05.013
Rasmussen H. M., Muzhingi T., Eggert E. M. R., Johnson E. J. (2012). Lutein, zeaxanthin, meso-zeaxanthin content in egg yolk and their absence in fish and seafood. J. Food Composition Anal. 27 (2), 139–144. doi: 10.1016/j.jfca.2012.04.009
Ren L. J., Ji X. J., Huang H., Qu L., Feng Y., Tong Q. Q., et al. (2010). Development of a stepwise aeration control strategy for efficient docosahexaenoic acid production by. Schizochytrium Appl. Microbiol. Biotechnol. 87 (5), 1649–1656. doi: 10.1007/s00253-010-2639-7
Reyes-Becerril M., Angulo C., Estrada N., Murillo Y., Ascencio-Valle F. (2014). Dietary administration of microalgae alone or supplemented with Lactobacillus sakei affects immune response and intestinal morphology of Pacific red snapper (Lutjanus Peru). Fish Shellfish Immunol. 40 (1), 208–216. doi: 10.1016/j.fsi.2014.06.032
Reyes-Becerril M., Guardiola F., Rojas M., Ascencio-Valle F., Esteban M.Á. (2013). Dietary administration of microalgae Navicula sp. affects immune status and gene expression of gilthead seabream (Sparus aurata). Fish Shellfish Immunol. 35 (3), 883–889. doi: 10.1016/j.fsi.2013.06.026
Riccio G., Lauritano C. (2020). Microalgae with immunomodulatory activities. Marine Drugs 18(1). doi: 10.3390/md18010002
Ruyet J. P., Labbé L., Le N., Sévère A., Le A., Le H., et al. (2008). Combined effects of water quality and stocking density on welfare and growth of rainbow trout (Oncorhynchus mykiss). Aquat. Living Resour. 21 (2), 185–195. doi: 10.1051/alr:2008024
Ruyter B., Røsjø C., Einen O., Thomassen M. S. (2000). Essential fatty acids in Atlantic salmon: Time course of changes in fatty acid composition of liver, blood and carcass induced by a diet deficient in n-3 and n-6 fatty acids. Aquaculture Nutr. 6 (2), 109–117. doi: 10.1046/j.1365-2095.2000.00136.x
Sarker P. K., Kapuscinski A. R., McKuin B., Fitzgerald D. S., Nash H. M., Greenwood C. (2020a). Microalgae-blend tilapia feed eliminates fishmeal and fish oil, improves growth, and is cost viable. Sci. Rep. 10, 19328. doi: 10.1038/s41598-020-75289-x
Sarker P. K., Kapuscinski A. R., Vandenberg G. W., Proulx E., Sitek A. J. (2020b). Towards sustainable and ocean-friendly aquafeeds: Evaluating a fish-free feed for rainbow trout (Oncorhynchus mykiss) using three marine microalgae species. Elementa: Science of the Anthropocene 8, 5. doi: 10.1525/elementa.404
Schüler L. M., Gangadhar K. N., Duarte P., Placines C., Molina-Márquez A. M., Léon-Bañares R., et al. (2020). Improvement of carotenoid extraction from a recently isolated, robust microalga, Tetraselmis sp. CTP4 (chlorophyta). Bioprocess Biosyst. Eng. 43, 785–796. doi: 10.1007/s00449-019-02273-9
Shah M. R., Lutzu G. A., Alam A., Sarker P., Kabir Chowdhury M. A., Parsaeimehr A., et al. (2018). Microalgae in aquafeeds for a sustainable aquaculture industry. J. Appl. Phycology 30 (1), 197–213. doi: 10.1007/s10811-017-1234-z
Sheridan M. A. (1989). Alterations in lipid metabolism accompanying smoltification and seawater adaptation of salmonid fish. Aquaculture 82 (1–4), 191–203. doi: 10.1016/0044-8486(89)90408-0
Sissener N. H., Torstensen B. E., Stubhaug I., Rosenlund G. (2016). Long-term feeding of Atlantic salmon in seawater with low dietary long-chain n-3 fatty acids affects tissue status of the brain, retina and erythrocytes. Br. J. Nutr. 115 (11), 1919–1929. doi: 10.1017/S0007114516000945
Smedes F. (1999). Determination of total lipid using non-chlorinated solvents. Analyst 124 (11), 1711–1718. doi: 10.1039/a905904k
Soleng M., Johansen L. H., Johnsen H., Johansson G. S., Breiland M. W., Rørmark L., et al. (2019). Atlantic salmon (Salmo salar) mounts systemic and mucosal stress responses to peracetic acid. Fish Shellfish Immunol. 93, 895–903. doi: 10.1016/j.fsi.2019.08.048
Sørensen M., Gong Y., Bjarnason F., Vasanth G. K., Dahle D., Huntley M., et al. (2017). Nannochloropsis oceania-derived defatted meal as an alternative to fishmeal in Atlantic salmon feeds. PloS One 12 (7), e0179907. doi: 10.1371/journal.pone.0179907
Sørensen M., Kousoulaki K., Hammerø R., Kokkali M., Kleingris D., Marti-Quijal F. J., et al. (2023). Mechanical processing of Phaeodactylum tricornutum and Tetraselmis chui biomass affects phenolic and antioxidant compound availability, nutrient digestibility and deposition of carotenoids in Atlantic salmon. Aquaculture 569, 739395. doi: 10.1016/j.aquaculture.2023.739395
Stiller K. T., Kolarevic J., Lazado C. C., Gerwins J., Good C., Summerfelt S. T., et al. (2020). The effects of ozone on Atlantic salmon post-smolt in brackish water—establishing welfare indicators and thresholds. Int. J. Mol. Sci. 21 (14), 1–17. doi: 10.3390/ijms21145109
Sun Y., Xiang Y., He M., Zhang X., Wang S., Guo W., et al. (2019). Evaluation of Lactococcus lactis HNL12 combined with Schizochytrium limacinum algal meal in diets for humpback grouper (Cromileptes altivelis). Fish Shellfish Immunol. 94 (June), 880–888. doi: 10.1016/j.fsi.2019.09.059
Talbot A. T., Smith T. J., Cairns M. T. (2009). Characterisation of the differentially regulated trout protein 1 (DRTP1) gene in rainbow trout (Oncorhynchus mykiss). Fish Shellfish Immunol. 26 (4), 589–598. doi: 10.1016/j.fsi.2008.09.013
Teimouri M., Yeganeh S., Mianji G. R., Najafi M., Mahjoub S. (2019). The effect of Spirulina platensis meal on antioxidant gene expression, total antioxidant capacity, and lipid peroxidation of rainbow trout (Oncorhynchus mykiss). Fish Physiol. Biochem. 45 (3), 977–986. doi: 10.1007/s10695-019-0608-3
Tibbetts S. M., Mann J., Dumas A. (2017). Apparent digestibility of nutrients, energy, essential amino acids and fatty acids of juvenile Atlantic salmon (Salmo salar L.) diets containing whole-cell or cell-ruptured Chlorella vulgaris meals at five dietary inclusion levels. Aquaculture 481, 25–39. doi: 10.1016/j.aquaculture.2017.08.018
Toften H., Arnesen A. M., Jobling M. (2003). Feed intake, growth and ionoregulation in Atlantic salmon (Salmo salar L.) smolts in relation to dietary addition of a feeding stimulant and time of seawater transfer. Aquaculture 217 (1–4), 647–662. doi: 10.1016/S0044-8486(02)00404-0
Usher M. L., Talbot C., Eddy F. B. (1991). Effects of transfer to seawater on growth and feeding in Atlantic salmon smolts (Salmo salar L.). Aquaculture 94 (4), 309–326. doi: 10.1016/0044-8486(91)90176-8
von Danwitz A., Schulz C. (2020). Effects of dietary rapeseed glucosinolates, sinapic acid and phytic acid on feed intake, growth performance and fish health in turbot (Psetta maxima L.). Aquaculture 516, 734624. doi: 10.1016/j.aquaculture.2019.734624
Waagbø R., Hamre K., Bjerkås E., Berge R., Wathne E., Torstensen B. (2003). Cataract formation in Atlantic salmon, Salmo salar L., smolt relative to dietary pro- and antioxidants and lipid level. J. Fish Dis. 26 (4), 213–229. doi: 10.1046/j.1365-2761.2003.00449.x
Wang L., Zhou Z.-C., Guo C.-J., Rao X.-Y., Xiao J., Weng S.-P., et al. (2009). The alpha inhibitor of NF-κB (IκBα) from the mandarin fish binds with p65 NF-κB. Fish Shellfish Immunol. 26 (3), 473–482. doi: 10.1016/j.fsi.2009.01.010
Weber S., Grande P. M., Blank L. M., Klose H. (2022). Insights into cell wall disintegration of Chlorella vulgaris. PloS One 17 (1 January 2022), e0262500. doi: 10.1371/journal.pone.0262500
Woo N. Y. S., Bern H. A., Nishioka R. S. (1978). Changes in body composition associated with smoltification and premature transfer to seawater in Coho salmon (Oncorhynchus kisutch) and King salmon (O. tschawytscha). J. Fish Biol. 13 (4), 421–428. doi: 10.1111/j.1095-8649.1978.tb03450.x
Wu N., Song Y. L., Wang B., Zhang X. Y., Zhang X. J., Wang Y. L., et al. (2016). Fish gut-liver immunity during homeostasis or inflammation revealed by integrative transcriptome and proteome studies. Sci. Rep. 6 (October), 1–17. doi: 10.1038/srep36048
Xie J., Fang H., Liao S., Guo T., Yin P., Liu Y., et al. (2019). Study on Schizochytrium sp. improving the growth performance and non-specific immunity of golden pompano (Trachinotus ovatus) while not affecting the antioxidant capacity. Fish Shellfish Immunol. 95, 617–623. doi: 10.1016/j.fsi.2019.10.028
Xu H., Dong X., Ai Q., Mai K., Xu W., Zhang Y., et al. (2014). Regulation of tissue LC-PUFA contents, Δ6 fatty acyl desaturase (FADS2) gene expression and the methylation of the putative FADS2 gene promoter by different dietary fatty acid profiles in Japanese seabass (Lateolabrax japonicus). PloS One 9 (1), e87726. doi: 10.1371/JOURNAL.PONE.0087726
Yarmohammadi S., Hosseini-Ghatar R., Foshati S., Moradi M., Hemati N., Moradi S., et al. (2021). Effect of Chlorella vulgaris on Liver Function Biomarkers: a Systematic Review and Meta-Analysis. Clin Nutr Res 10 (1), 83–94. doi: 10.7762/cnr.2021.10.1.83
Yeganeh S., Teimouri M., Amirkolaie A. K. (2015). Dietary effects of Spirulina platensis on hematological and serum biochemical parameters of rainbow trout (Oncorhynchus mykiss). Research in Veterinary Science 101, 84–88. doi: 10.1016/j.rvsc.2015.06.002
Ytrestøyl T., Takle H., Kolarevic J., Calabrese S., Timmerhaus G., Rosseland B. O., et al. (2020). Performance and welfare of Atlantic salmon, Salmo salar L. post-smolts in recirculating aquaculture systems: Importance of salinity and water velocity. Journal of the World Aquaculture Society 51 (2), 373–392. doi: 10.1111/jwas.12682
Ytrestøyl T., Hjelle E., Kolarevic J., Takle H., Rebl A., Afanasyev S., et al. (2023). Photoperiod in recirculation aquaculture systems and timing of seawater transfer affect seawater growth performance of Atlantic salmon (Salmo salar). Journal of the World Aquaculture Society 54 (1), 73–95. doi: 10.1111/jwas.12880
Keywords: microalgae, functional feed, bioactive compound, fatty acids, carotenoids, immunity, fish health, Atlantic salmon
Citation: Mueller J, Pauly M, Molkentin J, Ostermeyer U, van Muilekom DR, Rebl A, Goldammer T, Lindemeyer J, Schultheiß T, Seibel H and Schulz C (2023) Microalgae as functional feed for Atlantic salmon: effects on growth, health, immunity, muscle fatty acid and pigment deposition. Front. Mar. Sci. 10:1273614. doi: 10.3389/fmars.2023.1273614
Received: 06 August 2023; Accepted: 02 October 2023;
Published: 24 October 2023.
Edited by:
Ji Hyung Kim, Gachon University, Republic of KoreaReviewed by:
Adnan H. Gora, Central Marine Fisheries Research Institute (ICAR), IndiaCarlos Alfonso Alvarez-González, Universidad Juárez Autónoma de Tabasco, Mexico
Copyright © 2023 Mueller, Pauly, Molkentin, Ostermeyer, van Muilekom, Rebl, Goldammer, Lindemeyer, Schultheiß, Seibel and Schulz. This is an open-access article distributed under the terms of the Creative Commons Attribution License (CC BY). The use, distribution or reproduction in other forums is permitted, provided the original author(s) and the copyright owner(s) are credited and that the original publication in this journal is cited, in accordance with accepted academic practice. No use, distribution or reproduction is permitted which does not comply with these terms.
*Correspondence: Jonas Mueller, am11ZWxsZXJAdGllcnp1Y2h0LnVuaS1raWVsLmRl