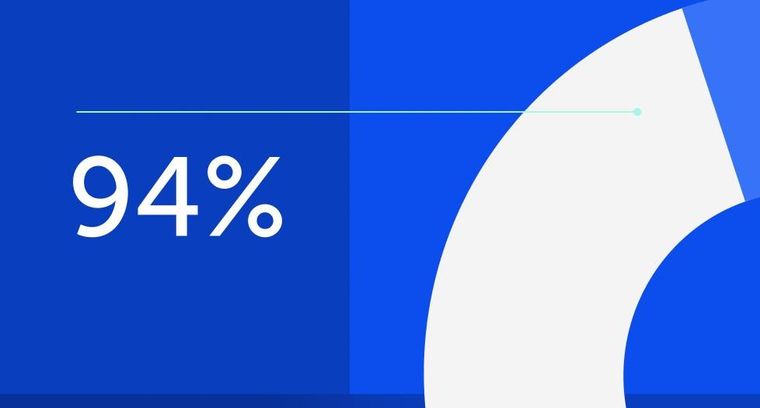
94% of researchers rate our articles as excellent or good
Learn more about the work of our research integrity team to safeguard the quality of each article we publish.
Find out more
REVIEW article
Front. Mar. Sci., 13 October 2023
Sec. Marine Biotechnology and Bioproducts
Volume 10 - 2023 | https://doi.org/10.3389/fmars.2023.1260709
This article is part of the Research TopicAdvances in Microalgal CO2 Fixation and BioenergyView all 4 articles
Microalgae are unicellular photosynthetic microorganisms that play a vital role in primary production and have diverse applications in various industries. They have high photosynthetic and metabolic capacities and can produce a variety of valuable metabolites, such as lipids, carbohydrates, pigments, and proteins. However, practical applications of microalgae are limited to high-value products due to the high production costs. Algal biotechnology faces challenges such as low energy utilization efficiency and product yield that are currently inadequate to fulfill commercial production. To overcome these challenges, emerging technologies have shown promise to achieve higher production efficiency, including molecular manipulation of photosynthetic efficiency and metabolic activities. Here, we provided an overview of the importance, diversity, and photosynthesis of microalgae, as well as strategies for enhancing their photosynthetic efficiency. We discussed various approaches for improving microalgal photosynthesis, including strain selection and optimization, rational genetic modification, and innovative technologies such as spectral recomposition of light, nanomaterials, advanced cultivation systems, and symbiotic systems. Additionally, we summarized metabolic engineering strategies that focus on optimizing the synthesis of value-added metabolites, such as pigments, long-chain polyunsaturated fatty acids, starch, proteins, and hydrogen in microalgae. By concentrating on improving photosynthetic efficiency and the synthesis of bioactive metabolites, this review provided valuable insights into enhancing microalgae production yields. Overcoming limitations in microalgae production costs can lead to broader applications in various industries. Furthermore, we highlight the potential of these strategies in increasing the efficiency of microalgae as a sustainable source for high-value products.
Photosynthesis is a vital biological process that utilizes light energy to convert inorganic carbon into organic matter. It consists of two stages: the light reactions and the dark reactions. The light reactions occur in the thylakoid membranes, where two photosystems (PS I and PS II) capture light energy, split water into oxygen and electrons, and generate NADPH and ATP through an electron transport chain. These products provide the necessary reducing power and energy for dark reactions. The light requirement varies depending on the biomass composition, with protein or lipid biomass requiring more photons (Wilhelm and Jakob, 2012). However, it’s imperative to acknowledge that both insufficient and excessive light levels can impede microalgal growth. Excessively high light, in particular, induces photoinhibition, primarily caused by the overproduction of reactive oxygen species (ROS) that harm vital cellular components, including photosynthetic pigments and proteins (Shi et al., 2020). Photoinhibition disrupts photosystem function, reducing the efficiency of ATP and NADPH production through the electron transport chain (Erickson et al., 2015). Consequently, the photosynthetic apparatus sustains damage, leading to diminished photosynthetic efficiency. In response to excess light and potential damage to the photosynthetic machinery, non-photochemical quenching (NPQ) becomes a pivotal photoprotective mechanism. NPQ dissipates surplus absorbed light energy as heat, thereby mitigating the risk of photodamage and oxidative stress, ultimately preserving photosynthetic efficiency (Finazzi and Minagawa, 2014). To effectively counter photoinhibition and optimize microalgal growth, it is imperative to maintain meticulous control over various light parameters, including intensity, duration, and spectral composition (Maltsev et al., 2021).
The dark reactions take place in the stroma, where NADPH and ATP produced by the light reactions are utilized to convert CO2 into carbohydrates. The primary pathway of carbon fixation is the Calvin-Benson cycle, also known as the C3 cycle or the reductive pentose phosphate cycle. This cycle involves four steps: carboxylation, reduction, regeneration, and product formation (Masojídek et al., 2013; Zhu et al., 2017; Prasad et al., 2021). Carboxylation is catalyzed by the enzyme RuBisCO, which combines carbon dioxide with a 5-carbon sugar called ribulose bisphosphate, resulting in the formation of two 3-carbon molecules of phosphoglycerate. Reduction involves the conversion of phosphoglycerate to a 3-carbon sugar known as phosphoglyceraldehyde (G3P) using NADPH and ATP. Regeneration comprises a series of reactions that transform G3P back into ribulose bisphosphate, providing the substrate for the next round of carboxylation. Finally, product formation involves the synthesis of carbohydrates and other organic compounds using some of the G3P molecules (Vuppaladadiyam et al., 2018; Masojídek et al., 2021; Wu et al., 2023).
Microalgae have evolved a CO2 concentrating mechanism (CCM) to overcome the low efficiency of RuBisCO and achieve high photosynthetic rates, even in environments with low CO2 levels (Reinfelder, 2011). CCM is an inducible, energy-dependent, light-regulated process that operates by transporting inorganic carbon (CO2 or HCO3-) from the external environment to the inside of the cell or converting inorganic carbon to organic carbon (C4 acids). This mechanism enables a high concentration of CO2 near the active centers of RuBisCO, thereby improving the efficiency of the C3 photosynthetic pathway and suppressing photorespiration (Singh et al., 2014). Cyanobacteria and other eukaryotic microalgae exhibit different structural features in their CCM, with cyanobacteria possessing a unique structural feature known as carboxysomes. Carboxysomes are microcompartments that house RuBisCO and carbonic anhydrase enzymes, crucial for the transportation of bicarbonate and the subsequent increase in localized CO2 concentration (Kaczmarski et al., 2019; Sun et al., 2020). Additionally, CCM plays a role in regulating the balance between the dark and light reactions of photosynthesis, as well as maintaining intracellular and extracellular pH and electrolyte balance (Kupriyanova et al., 2023).
Microalgae, comprising prokaryotic cyanobacteria and eukaryotic photosynthetic protists, are the most abundant primary producers that play a fundamental role in aquatic food webs. They contribute to nearly 50% of Earth’s oxygen production and primary productivity (Behrenfeld et al., 2001). With a long evolutionary history spanning billions of years, microalgae have undergone various endosymbiosis events, giving rise to different groups, such as green algae, red algae, heterokont algae, dinoflagellates, cryptophytes, and euglenids (Grama et al., 2022). Although an estimated 72,500 species of microalgae exist, only 44.50% of them have been isolated and described (Guiry, 2012). The phylogenetic diversity of microalgae allows for a wide range of applications as producers of various chemical substances.
Microalgae possess advantageous characteristics, including robust photosynthetic capacity, rapid growth rates, and efficient nutrient conversion, making them valuable for various industries. Through photosynthesis, microalgae excel in fixing CO2 at a significantly higher rate compared to terrestrial plants, converting carbon dioxide into organic matter and producing diverse bioactive compounds (Ambati et al., 2019; Purba et al., 2022). The potential applications of microalgae span fields such as biofuels, animal feed, food production, cosmetics, and pharmaceuticals (Maeda et al., 2018; Sathasivam et al., 2019). In the field of biofuel development, microalgae can produce biohydrogen through various metabolic pathways. Biohydrogen is a promising alternative source of renewable clean energy that only releases water vapor as a by-product, without emitting greenhouse gases or other pollutants into the air as fossil fuels do when they are burned (Wang Y. et al., 2020; Musa Ardo et al., 2022). Besides, microalgae biomass offers potential for biogas and biomethane production through anaerobic fermentation, representing a CO2-neutral energy source (Klassen et al., 2020). Microalgae also hold promise as a third-generation feedstock for bioethanol production, effectively addressing the need for renewable fuels in light of depleting fossil fuel reserves and the escalating emissions of greenhouse gases (Maity and Mallick, 2022). Moreover, microalgae biomass offers a rich and balanced nutritional profile for humans, including essential amino acids, fatty acids (especially long-chain polyunsaturated fatty acids, LC-PUFAs), vitamins, various polysaccharides and oligosaccharides, trace elements, and minerals (Tibbetts et al., 2015; Carrasco-Reinado et al., 2019; Wang A. et al., 2020; Yi et al., 2021). Additionally, microalgae contain bioactive compounds with anti-inflammatory, antioxidant, and immunomodulatory effects (Koyande et al., 2019; Manning et al., 2020; Fernandes and Cordeiro, 2021).
Furthermore, microalgae exhibit exceptional CO2-fixation efficiencies of up to 10%, surpassing that of other photosynthetic organisms (1 ~ 4%) (Williams and Laurens, 2010; Hepburn et al., 2019). The high growth rates in microalgae make them appealing cell factories, as demonstrated by the impressive biomass productivity of Phaeodactylum tricornutum UTEX 640, which can reach 70.83 mg/L/h (Butler et al., 2020). Likewise, Synechococcus sp. PCC 11901 exhibits swift growth, featuring a brief doubling time of approximately 2 hours and the capacity to amass around 33 grams of dry cell weight per liter under favorable conditions (Włodarczyk et al., 2020). Additionally, microalgae can be cultivated on a large scale without the need for arable land, making them environmentally friendly and minimizing competition with food production. Considering their mass production potential, minimal land requirements, and superior efficiency in converting CO2 into biomass, microalgae hold immense promise as sustainable cell factories for various industries. As shown in Table 1, the comparison with other species highlights both the advantages and challenges of harnessing microalgae’s potential for sustainable production. This not only opens up new avenues for high-value product synthesis but also offers a greener and more sustainable approach.
Although microalgae production technology is technically feasible, it currently faces challenges related to high production costs, limiting its widespread application to high-value products. To address this issue, it is essential to focus on reducing production costs and improving cost efficiency through various approaches. These approaches include cultivating novel microalgal strains with enhanced photosynthetic efficiency and high-yield production under different environmental conditions. Additionally, optimizing light-capturing and carbon-fixing components can contribute to higher productivity and cost-effectiveness. Researchers are actively exploring techniques such as multi-omics analyses and metabolic engineering strategies to overcome these challenges and achieve economic viability in microalgae production. By enhancing photosynthetic efficiency, we can potentially reduce production costs and expand the range of applications for microalgae as a sustainable and economically viable source.
The natural efficiency of energy conversion through photosynthesis is relatively low, with most plants only able to store 0.1 ~ 2% of solar energy (Kornienko et al., 2018). Microalgae can convert a higher percentage of the sun’s energy to biomass energy, estimated to be around 8 ~ 10%, and theoretically produce up to 77 g/m2/d of biomass (Melis, 2009; Formighieri et al., 2012). However, a realistic photosynthetic efficiency that microalgae can approach is 4.5% (Walker, 2009). Our research is centered on enhancing this inherent efficiency by fine-tuning the key processes of light energy utilization and carbon fixation. To achieve this goal, we aim to introduce targeted genetic modifications and innovative strategies in microalgae. Specifically, our objectives encompass increasing the efficiency of light energy utilization, accelerating carbon-to-biomass conversion rates, and promoting faster growth. These modifications will be achieved through various methodologies, including the optimization of photosynthetic enzyme activities, enhancement of light absorption efficiency such as through the modification of light-harvesting protein complexes, and augmentation of carbon fixation pathways. Various methods have been developed for this purpose, including random mutagenesis and rational genetic modification. Beyond these genetic approaches, innovative avenues like nanomaterial applications and ultrasound technology have emerged, unlocking novel means to bolster photosynthetic performance. Additionally, advanced cultivation systems have proven instrumental in optimizing microalgal growth and photosynthetic efficiency. Moreover, the exploration of symbiotic relationships between microalgae and bacteria presents a promising avenue. In such symbiotic systems, the bacterial community offers competitive advantages and crucial substances that further foster microalgal biomass growth.
In this section, we will discuss different approaches used to engineer photosynthesis, including mutagenesis and strain screening methods, genetic modification in microalgae, and technologies beyond gene editing and synthetic biology. These approaches hold great potential for enhancing energy conversion efficiency and biomass production in microalgae, contributing to the development of sustainable energy sources. Figure 1 provides an overview of the different approaches used to improve photosynthesis efficiency in microalgae.
Figure 1 Strategies for improving photosynthesis efficiency in microalgae. The left box represents random mutagenesis, which includes methods such as EMS, UV, and ARTP, along with adaptive laboratory evolution. The middle box depicts rational genetic modification, featuring a schematic representation of promoter engineering, CRISPR technology, gene insertion, chloroplast modification, and the integration of genome-scale modeling for metabolic pathways and modeling. The right box represents technologies beyond gene editing and synthetic biology, including ultrasound stimulation, nanomaterials, and the beneficial effects of bacterial communities. It is important to note that these strategies may vary in their application and effectiveness across different microalgal species. EMS, ethyl methanesulfonate; UV, ultraviolet; ARTP, atmospheric and room temperature plasma; CRISPR, clustered regularly interspaced short palindromic repeats; LHC, light-harvesting complexes; RuBP, ribulose-1,5-bisphosphate; CDs, carbon dots; GOQDs, graphene oxide quantum dots. Created with BioRender.com.
Traditional random mutagenesis, which is generally performed by physical and chemical mutagens, and ALE based on screening of advantageous mutations have demonstrated success in improving photosynthetic efficiency and biomass production. Physical mutagens include ultraviolet (UV) light, ionizing radiation, atmospheric and room temperature plasma (ARTP), and laser radiation (Bleisch et al., 2022). UV radiation induces DNA alterations, especially the formation of pyrimidine dimers while ionizing radiation causes more serious genetic damage, such as chromosomal aberrations. UV mutagenesis of Chlorella sp. enhanced biomass production by 7.6% higher than the wild type (Liu S. et al., 2015). ARTP mutagenesis uses charged particles, electromagnetic fields, neutral reactive species, and heat to generate mutants rapidly and with high diversity. A mutant of Crypthecodinium cohnii generated by ARTP had a 17.8% higher biomass concentration than the wild type (Liu B. et al., 2015). Laser radiation in the near-infrared and visible spectrum can also induce mutations, and many microalgae show tolerance to radiation in this spectrum. Laser radiation is rarely used to specifically enhance photosynthetic efficiency or biomass accumulation in microalgae and is mostly used to promote biodiesel production in microalgae (Politaeva et al., 2018; Faried et al., 2022). On the other hand, chemical mutagens such as ethyl methanesulfonate (EMS) induce alterations in base pairing and typical point mutations after DNA replication. For instance, EMS mutagenesis and selection media containing the herbicide quizalofop-P-ethyl were used to screen for mutagenic Chlorella sp. with maximum biomass yield and productivity 111% and 110% higher than the wild type, respectively (p < 0.01) (Tanadul et al., 2018).
High light and high carbon-containing gas induction promote microalgal biomass productivity, with a greater effect when combined. For instance, ALE of Chlorella vulgaris in a photobioreactor using optimized LED light yielded 2.11 g dry-cell-weight (gDCW) per liter (L) per day (Fu et al., 2012). Continuous passage of Chlamydomonas reinhardtii CC-124 strain under 120 µmol photons/m2/s for 5 years resulted in a new derivative strain, CC-124H, with 3-fold higher biomass productivity (Shin et al., 2017). Haematococcus pluvialis showed 1.3 times higher biomass production after 10 passages at 15% CO2, and astaxanthin yield (87.4 mg/L) increased 6 times with high light induction at 135 µmol photons/m2/s (Cheng et al., 2016). Moreover, ALE under 1% CO2 resulted in an evolved microalgal strain, Chlorella sp. AE1, with CO2 fixation rates of 0.60, 0.57, and 0.28 g·L−1·day−1 under 1%, 10%, and 30% CO2, respectively, indicating enhanced CO2 fixation capacity (Li and Zhao, 2023). High CO2 concentration ALE in microalgae enhances carbon metabolism and lipid accumulation through increased gene translation and protein expression. For instance, Chlorella PY-ZU1 cells exposed to 15% CO2 showed reduced intracellular carbonic anhydrase but higher transcriptional abundances of carbon fixation genes (Huang et al., 2017). Additionally, Haematococcus pluvialis exhibited upregulated genes related to photosynthesis under 15% CO2 (Li et al., 2017). However, excessively high CO2 concentrations can inhibit microalgal growth. Long-term high CO2 stress induces numerous gene mutations, peaking at 60% CO2 concentration. Excessively high CO2 levels hinder stable long-fragment gene mutations and inhibit transcription and translation, which makes it challenging for beneficial gene mutations to manifest in transcription. Furthermore, the inhibition of mismatch repair in transcription affects genetic variation, impacting microalgae’s response to high CO2 stress (Li, 2008). However, more research is needed to understand ALE’s effects on microalgal photosynthetic efficiency, particularly at CO2 levels exceeding 30% (Wang et al., 2022). Furthermore, the influx of excessive CO2 into the culture medium leads to a decrease in pH, which affects microalgal growth. ALE was performed under low pH stress on the Phaeodactylum tricornutum, and the evolved strains showed an average growth increase of 110.4% compared to the wild type at pH 5.5, where genes related to the core pathways, including photosynthesis, pH regulation/ion transport, as well as carbohydrate and fatty acid metabolism, were all up-regulated, demonstrating another feasibility of high CO2 to promote photosynthetic efficiency in microalgae (Su et al., 2023).
However, random mutagenesis is a lengthy process that cannot accurately identify the specific location of a desired mutation. Additionally, it could result in harmful mutations that may negatively impact the growth of microalgae. As a result, more targeted and precise molecular biology methods should be investigated to genetically alter microalgal metabolic processes to increase pigment production without hindering their growth and metabolism.
With the abundance of mutants generated by mutagenesis and ALE, accurate and efficient screening methods are needed to identify the most promising variants. Screening methods can be classified into quantitative and qualitative approaches.
The quantitative approaches involve conducting a high number of parallel experiments using traditional methods or microtiter plates, which require automation using laboratory robotic platforms to handle the process (Bleisch et al., 2022). However, improvements are needed, such as dispensing accuracy and cost reduction. A novel cultivation strategy, the high-density cultivation screening platform, has been developed to address these constraints. It utilizes a two-tier vessel, a hydrophobic membrane-mediated CO2 supply, high photon flux density, rapid turbulent mixing, and optimized layer thickness of the culture to enable high-density cultivation of phototrophic microorganisms (Lippi et al., 2018; Zeng et al., 2020).
The qualitative approaches involve mutant analysis at the single-cell level. Flow cytometry (FC) combined with fluorescence-activated cell sorting (FACS) is a preferred method for high-throughput screening, sorting cells based on their size, granularity, and fluorescence properties by passing them through a laser beam and using an electrical charge to separate them (Pereira et al., 2018; Işıl et al., 2021). Dual antibiotic selection transformation and FACS are useful techniques for identifying and isolating Chlamydomonas reinhardtii transformants with high expression of recombinant proteins (Sproles et al., 2022). However, extracellular target products cannot be easily analyzed. Droplet-based microfluidic chips are another high-throughput screening technique that utilizes microchannels to generate and manipulate droplets of picoliter to nanoliter volumes, allowing for high-throughput and precise reactions and analyses of biological samples under constant environmental conditions with high recovery rate after sorting (Kim et al., 2017; Mishra et al., 2021; Sung et al., 2022). However, they require unique process flows for every application and have a lower encapsulating speed compared to FC combined with FACS. Furthermore, microalgal screening techniques for vulnerable recombinant cells and other species remain to be developed. Besides, well-plate screening techniques have been used for rapid screening, which is based on pulse amplitude modulated (PAM) imaging of chlorophyll variable fluorescence to fast and conveniently measure the changes in the photosynthetic apparatus condition of the microalgae and enable the dynamic experiments without the need for exogenous contrast agents (Solovchenko et al., 2022). However, rapid evaporation of water and stirring problems still need to be addressed.
Using RNA-seq data, transcription factors (TFs) are identified and used to regulate many genes in a metabolic pathway. By binding specific DNA sequences with cis-elements of the gene target and by interacting with the RNA polymerase, TFs regulate the expression of specific target genes (Sproles et al., 2021). Study shows four TFs (CML246C, CMR124C, CMT597C, and CMT067C) enhance triacylglycerol (TAG) production in a red alga Cyanidioschyzon merolae by upregulating a key enzyme gene in the TAG biosynthesis pathway (Takahashi et al., 2021). In the case of Nannochloropsis gaditana, 18 out of 20 TFs as putative negative regulators of lipid production during nitrogen deprivation were knocked out, resulting in significantly enhanced lipid production, doubling the lipid yield to approximately 5.0 g/m2/d compared to the wild type, while maintaining similar growth rates (Ajjawi et al., 2017). A light-sensitive gene regulator NobZIP77 was revealed to control oil production in Nannochloropsis oceanica and can be manipulated to increase oil yield under different light and nutrient conditions. For example, the cell growth rate is maintained, and TAG productivity is almost tripled by NobZIP77 knockout under nitrogen sufficiency and white light (Zhang et al., 2022). Currently, TFs are used to enhance lipid or pigment production, and few studies have used them to modify photosynthesis-related reaction pathways.
When microalgae are genetically modified, specific exogenous genes are usually transferred into the genome of the microalgae. To enhance the expression of transgenes for recombinant proteins and metabolic products in microalgae, strong promoters, and reporter genes need to be designed. New synthetic expression elements have been designed within Chlamydomonas reinhardtii, resulting in an increased yield of the sesquiterpene (E)-alpha-bisabolene in the newly synthesized algal promoter (AβSAP(i)) by 18-fold compared to wild type and 4-fold compared to commonly used expression elements (Einhaus et al., 2021). An electroporation transformation system with strong endogenous promoter CRT (Pcrt) can be used to enhance heterologous expression with simple preparation and operation (Lee et al., 2020). Multiple gene expression was achieved in Nannochloropsis salina using glycine-serine-glycine spacer linked 2A self-cleaving peptides, which combined with a stronger promoter, resulted in a 9-fold increase in transformation efficiency (Koh et al., 2018). Transcript fusion using ble::E2A resulted in a 10-fold enhancement of the fluorescence signal of the green fluorescent protein gene expression in Scenedesmus acutus (Suttangkakul et al., 2019). Recently a new gene expression system has been identified in Nannochloropsis, where the nucleolus is a genomic safe harbor for Pol I transcription and can be used to construct transformed strains with consistent strong transgene expression (Südfeld et al., 2022). Furthermore, the development of a Modular Cloning toolkit (MoClo toolkit), a standardized synthetic biology tool based on Golden Gate cloning with 119 genetic components like promoters, UTRs, terminators, and more, has greatly facilitated genetic manipulation in microalga Chlamydomonas reinhardtii and revolutionized microalgal biotechnology (Crozet et al., 2018).
The revolutionary CRISPR technology has provided a powerful tool for enhancing photosynthetic efficiency in microalgae. Initially discovered as an adaptive immunity system in Escherichia coli, CRISPR functions by incorporating fragments of viral DNA as spacers, allowing cells to recognize and defend against future viral invasions (Barrangou et al., 2007). The CRISPR-Cas9 system, guided by RNA, accurately targets specific DNA sequences, enabling precise genetic modifications (Jinek et al., 2012). CRISPR technology has emerged as a potent tool for enhancing microalgae photosynthetic efficiency, enabling targeted genome editing to manipulate gene expression and develop industrial traits in various microalgae species, thus paving the way for scalable production of commodities and biofuels using photosynthetic cell factories (Patel et al., 2019).
Researchers have harnessed the power of CRISPR technology to enhance microalgae photosynthetic efficiency across various species. In Chlamydomonas reinhardtii, by down-regulating the PEPC1 gene responsible for carbon splitting, lipid production was enhanced to 893 mg/L at 35°C, alongside notable increases in biomass accumulation of 2.56 g/L (Lin and Ng, 2023). Similarly, in Chlorella sorokiniana, the utilization of dCas9 guided by guanine-rich sgRNA resulted in precise gene regulation, contributing to improved up to 65% protein content (Lin et al., 2022). Meanwhile, in Nannochloropsis oceanica, the activation of the g1248 gene, linked to DNA/mRNA methylation, via CRISPR activation, elevated growth rates and photosynthetic efficiency (Fv/Fm) by a remarkable 23% and 12%, respectively (Wei et al., 2022). In the cyanobacterium Synechocystis 6803, knockout of the CAO gene, pivotal in Chl a to Chl b conversion, led to a substantial 1.57-fold increase in biomass accumulation under strong light and high cell density conditions (Kirst et al., 2014). Similarly, the knockout of the ALB3b, linked to fucoxanthin-chlorophyll a/c synthesis in the diatom Phaeodactylum tricornutum, resulted in 30 ~ 40% higher rETRmax (the maximum relative electron transport rate) and Ek (light saturation index), underscoring the cells’ ability to adapt the photosynthetic apparatus to varying light intensities (Nymark et al., 2019).
However promising CRISPR technology is for boosting microalgae photosynthetic efficiency, certain limitations impede its seamless application. The intricate taxonomic diversity among microalgae poses a challenge in developing standardized transformation techniques and identifying suitable selection markers, which in turn hampers the efficiency of genome editing (Jeon et al., 2017). Moreover, the persistent issue of low transformation efficiency, particularly in less commercially exploited microalgae, coupled with concerns regarding off-target effects and the interference of microalgae’s inherent silencing systems, presents formidable obstacles that must be surmounted to effectively leverage CRISPR techniques for precisely enhancing microalgae’s photosynthetic potential (Lee et al., 2023).
RuBisCO is the key enzyme in the carbon fixation cycle, which catalyzes the combination of carbon dioxide and ribulose bisphosphate to form organic compounds (Beardall and Raven, 2016). However, RuBisCO also has a drawback, as it sometimes reacts with oxygen to produce phosphoglycolate, a process called photorespiration. Photorespiration consumes energy and already-fixed carbon and releases carbon dioxide and ammonia (Raven et al., 2020). Researchers have explored improving the efficiency of RuBisCO action by RuBisCO activation (Wei et al., 2017) and engineering RuBisCO mutants (Liang and Lindblad, 2017).
Moreover, researchers have explored alternative enzymes to RuBisCO, such as 6-phosphogluconate dehydrogenase (6PGDH), which is more efficient in catalyzing the CBB cycle. Its catalytic product can also be recycled into the CBB cycle, making it a promising alternative to RuBisCO (Bar-Even, 2018). Formate dehydrogenase (FDH) is another enzyme that converts carbon dioxide to formyl-tetrahydrofolate (THF) to produce serine with the aid of ATP, utilizing residual energy from central metabolism with high-efficiency (Bar-Even, 2016). However, there have been limited studies aimed at improving the photosynthetic efficiency of microalgae by designing new CO2 fixation pathways.
Optimizing the size of light-capturing antennae can significantly enhance photosynthetic performance (40% increase in biomass yield), which has not yet been validated within microalgae (Friedland et al., 2019). The binding of carbon dioxide to the α-subunit of the light-harvesting complexes isocyanidin enhances light energy transfer and fluorescence quantum yield in Synechocystis sp. PCC 6803 (Guillén-García et al., 2022). In addition, inactivating flavodiiron proteins (Flv1/Flv3) to remove natural competing electrons can accelerate the conversion of light energy to biochemicals, as demonstrated by simulation experiments (Assil-Companioni et al., 2020).
Depending on the metabolic pathway of the target product, researchers have used metabolic engineering to select key product-converting enzymes in the overexpression pathway or to shut down competing pathways to achieve an increase in the target product. This approach has led to many successes, such as a synthetic malonyl-CoA-glycerate (MCG) pathway was designed to enhance the CBB cycle, and implementation of this pathway in the cyanobacterium Synechococcus elongates PCC7942 was shown to enhance carbonate assimilation efficiency by approximately two folds (Yu et al., 2018). In the CBB cycle, the catalytic activity of RuBisCO produces the toxic compound 2-phosphoglycolate (2-PG), which leads to photorespiration, reducing the production of organic matter by microalgae. A malic acid cycle can lead to complete decarboxylation of 2-PG and increase carbon dioxide content in chloroplasts, which has been verified in tobacco cultivation to increase biomass and photosynthetic efficiency (South et al., 2019).
Nevertheless, the above approaches to modify the enzyme expression of metabolic pathways may not be optimal, and the manual selection of regulatory genes cannot accurately find the most critical enzymes in metabolic pathways. Therefore, genome-scale metabolic model reconstruction provides a more scientific and rapid way of thinking for predicting and optimizing metabolic engineering regulation. A method integrates chlorophyll fluorescence data into a metabolic model of Phaeodactylum tricornutum and improves the accuracy and understanding of the diatom’s growth and metabolism under different light conditions, which can help design bioengineering strategies to redirect excess light energy towards bioproducts (Broddrick et al., 2022).
With the help of information technology and computational software, several multi-omics databases and growth kinetic models of microalgae have been developed. One such example is the Nannochloropsis Design and Synthesis (NanDeSyn) database, which integrates gene annotation, transcriptomics, proteomics, and epigenomics of Nannochloropsis with various functions such as genome analysis, enrichment analysis, and metabolic pathway analysis (Gong et al., 2020).
Environmental factors like light intensity, temperature, and carbon and nitrogen concentrations play a vital role in microalgal cell light energy utilization and biomass production. To optimize these conditions, several microalgal kinetic models have been developed and validated. For instance, the quadruple-factor kinetic model, which considers carbon and nitrogen concentrations, light intensity, and temperature, was validated to calculate the optimal acetate and nitrogen concentrations, increasing lipid productivity by 50.9% (Bekirogullari et al., 2018). These models help determine the environmental conditions necessary for the growth and division of microalgal cells and estimate the time required for microalgae to complete their life cycle (Pahija and Hui, 2021). Additionally, the modified Cornet model integrates cell concentration and pigment composition to better predict light transmission during culture (Ma et al., 2022).
The use of various kinetic models of microalgae not only helps in simulating production processes and optimizing conditions but also aids in understanding the metabolic behavior of microalgae, thereby improving the efficiency of light energy and substrate utilization. For example, a mathematical model was used to describe the formation of biomass and the production of carbohydrates, proteins, and lipids under nutrient-sufficient and deficient conditions. The analysis of this model showed that the cell state significantly influences lipid production (Ryu et al., 2018).
In summary, various multi-omics databases and kinetic models of microalgae provide a better understanding of the metabolic behavior of microalgae, enabling the optimization of environmental conditions for the production of high-value compounds. Table 2 below summarizes the various types of kinetic models of microalgae and their applications.
Beyond gene editing and synthetic biology, various technologies and symbiotic systems have shown promise in enhancing the performance of microalgae.
To improve the efficiency of light utilization, the intracellular spectral recomposition (ISR) of light applied in the enhanced green fluorescent protein (eGFP) was conducted, with the expression of eGFP in Phaeodactylum tricornutum raising the efficiency of photosynthesis by 28% (Fu et al., 2017). In Chlorella, cells make the best use of red light (580 ~ 700 nm) and blue light (400 ~ 500 nm) while absorbing very little green light (500 ~ 600 nm). Carbon dots (CDs) that can absorb green light and emit red light were able to increase the cell density and chlorophyll productivity of Chlorella by 15% and 29%, respectively (Xue et al., 2020). Meanwhile, graphene oxide quantum dots (GOQDs) that emit blue light after excitation by UV light, when mixed in a Chlorella pyrenoidosa culture system, can effectively enhance the energy transfer of photosystem II (PSII) under high UV irradiation, thereby increasing the CO2 fixation rate by 20% (Yang et al., 2022).
Ultrasound stimulation has also been shown to enhance microalgae growth by increasing material passage through the membrane. In one study, the maximum biomass concentration of ultrasonically treated Scenedesmus sp. Z-4 was found to be 2.78 g/L, which was 26.9% higher than the control (Ren et al., 2019). Similarly, the lipid and β-carotene production of Tetradesmus obliquus SGM19 increased by 34.5% and 31.5%, respectively, due to ultrasound stimulation (Singh et al., 2019).
Nanomaterials, such as nanoparticles, have been found to increase the fixation of CO2 by microalgae. For instance, nanofibers containing nanoparticles, which can adsorb CO2 and expose the microalgae to more gas, were used to culture Chlorella fusca LEB 111, resulting in a 57.47% increase in CO2 fixation rate of 216.2 mg/L/d (Vaz et al., 2019).
Advancements in cultivation systems have emerged as pivotal factors in bolstering microalgal photosynthetic efficiency. Conventionally, open or closed cultivation systems have been employed (You et al., 2022). However, open systems, while cost-effective, often suffer from low photosynthetic efficiency and are susceptible to contamination, making it challenging to enhance CO2 utilization and biomass production effectively (Jerney and Spilling, 2018). In contrast, closed cultivation systems, such as photobioreactors (PBRs), offer greater control and enable more efficient utilization of light and CO2, consequently enhancing microalgal photosynthetic efficiency. Improved thin-layer flat plate PBRs with a titled angle have been developed to enhance light penetration, resulting in a 220% increase in biomass yield (Sun et al., 2016; Assunção and Malcata, 2020). Alternatively, by extending the residence time of carbon dioxide gas within the PBR, the areal productivity of Chlorella sorokiniana could reach 57 g/m2/d (Janoska et al., 2018).
In addition to these technological advancements, the symbiotic relationship between microalgae and bacteria has also been explored. One such example is the consortium of Tetraselmis indica and Pseudomonas aeruginosa, which was found to grow much better in dairy wastewater than pure microalgae culture, with 38.8% higher maximum biomass production (1454.88 mg/L) (Talapatra et al., 2021). Bacterial communities can also provide microalgae with essential substances such as sufficient vitamin B1 and B12 (Yao et al., 2019), as seen in the symbiotic relationship between Lingulodinium polyedrum and its bacterial community, which helps maintain their growth and reach their maximum growth rate (Cruz-López and Maske, 2016).
Microalgae possess the remarkable ability to secrete various metabolites during their growth, including pigments, LC-PUFAs, starch, proteins, biohydrogen, and other valuable molecules. To enhance the production efficiency of these high-value metabolites, metabolic engineering techniques are frequently employed. These strategies involve the overexpression of key enzyme genes in synthetic pathways or the knockout of essential enzyme genes in competing pathways, thereby directing the cellular resources toward the synthesis of targeted products. Figure 2 provides a simplified representation of the metabolic pathways involved in the production of pigments, PUFAs, and starch in microalgae. This figure offers a visual overview of the intricate biochemical processes and highlights the key steps that can be targeted for metabolic engineering interventions. By strategically manipulating these pathways, researchers can optimize the production of desired metabolites in microalgae.
Figure 2 Metabolic pathways of pigments, LC-PUFAs, and starch in a simplified version of the microalgal cell. The dashed lines represent an abridged representation of multiple reactions occurring within a pathway. The brown text indicates enzymes within the metabolic pathways. It is important to note that metabolic pathways may vary among different microalgal species. ACC, acetyl-CoA carboxylase; ADP, adenosine diphosphate; AGPase, ADP-glucose pyrophosphorylase; ARA, arachidonic acid; BKT, β-carotene ketolase; CIS, citrate synthase; CRTR-B, β-carotene hydroxylase; DES, desaturases; DGAT, diacylglycerol acyltransferase; DHA, docosahexaenoic acid; DMAPP, dimethylallyl pyrophosphate; DOF, DNA binding with one finger; DPA, docosapentaenoic acid; DXP, 1-deoxy-d-xylulose 5-phosphate; DXR, 1-deoxy-D-xylulose 5-phosphate reductase; DXS, 1-deoxy-D-xylulose 5-phosphate synthase; ELO, elongase; EPA, eicosapentaenoic acid; ER, endoplasmic reticulum; G1P, glucose-1-phosphate; GAP, glyceraldehyde-3-phosphate; GGPP, geranylgeranyl pyrophosphate; LACS, long-chain acyl-CoA synthetase; LCYE, lycopene epsilon cyclase; ME, malic enzyme; MEP, 2-c-methyl-d-erythritol 4-phosphate; OR, orange protein; TAG, triacylglycerol; VDE, violaxanthin de-epoxidase; ZEP, zeaxanthin epoxidase. Created with BioRender.com.
During photosynthesis in microalgae, a series of pigments, such as chlorophylls, carotenoids, and phycobiliproteins, are involved in the light capture and photoprotection processes (Srivastava et al., 2022). These pigments are environmentally friendly substitutes for synthetic dyes and offer numerous health advantages. However, the natural production of these pigments is insufficient to meet the growing demand in the market. The production of pigments can be influenced by environmental factors such as pH, temperature, light, salinity, and media nutrients (Saini et al., 2018). Additionally, techniques like gene editing and metabolic engineering can be employed to enhance pigment production in microalgae.
Chlorophyll is a common pigment in photoautotrophs such as cyanobacteria, algae, and plants. It is needed for the stability, folding, and membrane insertion of chlorophyll-binding proteins, and captures light at different wavelengths because of electron delocalization, making it a potent photoreceptor (Wang and Grimm, 2021). Visualizing the intricate process, the chlorophyll synthesis pathway can be represented as a complex cascade of enzymatic reactions. For a detailed illustration of the step-by-step progression of chlorophyll production, please refer to Figure 3. Chlorophyll a is the most prevalent isoform in microalgae. It is produced through a series of enzymatic reactions, with the transformation of protochlorophyllide to chlorophyllide mediated by light-independent or light-dependent protochlorophyllide oxidoreductase (Willows, 2020). To enhance chlorophyll production, modulation of TFs is a possible approach. Disruption of CrbZIP2, a bZIP TF in the green alga Chlamydomonas reinhardtii, reduces TAG levels but elevates chlorophyll content under conditions of nitrogen stress (Bai et al., 2021). Nevertheless, there are few metabolic engineering studies to enhance the intracellular chlorophyll content of microalgae.
Figure 3 Overview of biosynthesis of chlorophylls in the microalgal cell. The figure was adapted from previous publications (Willows, 2020; Srivastava et al., 2022). Arrows indicate biosynthetic pathways. The brown text indicates enzymes in metabolic pathways. Light green boxes indicate important pigments. AcsF, BchE or CTH1/Ycf54, MgPME oxidative cyclases; ALA, aminolevulinic acid; BchB/ChlB, BchN/ChlN, BchL/ChlL, light independent protochlorophyllide oxidoreductase; BchH/ChlH, BchD/ChlD, BchI/ChlI, magnesium chelatase subunits; Chl a, chlorophyll a; Chl b, chlorophyll b; ChlG, chlorophyll synthase; ChlM, S-adenosylmethionone Mg-protoporphyrin IX monomethylester transferase; ChlP, geranylgeranyl reductase/phytyl synthase; COA, chlorophyll a oxygenase; DV PChlide a, 3,8-divinyl protochlorophyllide a; DVR, divinyl reductase; GG Chl a, geranylgeranyl chlorophyll a; GluRS, glutamyl tRNA synthetase; GluTR or HemA, glutamyl-tRNA reductase; GSAT or HemL, glutamate-1-semialdehyde aminotransferase; Gun4, tetrapyrrole-binding protein; HemF or CPO, oxygen dependent coproporphyrinogen III oxidase; HemN, oxygen independent coproporphyrinogen III oxidase; MgP, Mg-protoporphyrin IX; MgPME, Mg-protoporphyrin IX monomethyl ester; MV Chlide a, 3,8-monovinyl chlorophyllide a; MV PChlide a, 3,8-monovinyl protochlorophyllide a; PBGD or HMBS or HemC, porphobilinogen deaminase/hydroxymethylbilane synthase; PBGS or ALAD or HemB, porphobilinogen synthase/ALA-dehydratase; Por, light dependent protochlorophyllide oxidoreductase; PPIX, protoporphyrin IX; PPO or HemG, protoporphyrinogen IX oxidase; UROD or HemE, uroporphyrinogen decarboxylase; UROS or HemD, uroporphyrinogen III synthase. Created with BioRender.com.
Carotenoids are a class of pigments abundant in microalgae, including lutein, canthaxanthin, astaxanthin, zeaxanthin, and fucoxanthin. For a comprehensive insight into the carotenoid synthesis pathway, Figure 4 showcases its step-by-step progression. One study found that DpAP2 is an AP2/ERF transcription factor that promotes carotenoid accumulation in Dunaliella parva. Specifically, DpAP2 can interact with three proteins, which may be associated with multiple biological processes. This lays a good foundation for a deep understanding of the regulatory mechanisms of DpAP2 and genetic engineering breeding (Shang et al., 2022). Recently, a comprehensive survey of genes and enzymes related to the carotenoid biosynthetic pathway has now been conducted, and structural modeling of three important enzymes, 1-deoxy-D-xylulose 5-phosphate reductase (DXR), 15-cis-phytoene synthase (PSY), and zeta-carotene desaturase (ZDS), has been achieved (Narang et al., 2021). Regarding Chlamydomonas reinhardtii, overexpression of 1-deoxy-D-xylulose 5-phosphate synthase gene (DXS) and reductase gene (DXR) boosted lutein and β-carotene synthesis by 1.9 and 1.7 times, respectively (Morikawa et al., 2018). Overexpression of the regulatory protein ORANGE (OR) increases carotenoid content in plant cells, and overexpression of mutant CrOR (CrORHis) in Chlamydomonas reinhardtii results in a 3-fold increase in carotenoid accumulation and upregulation of transcript abundance in almost all relevant synthetic genes (Yazdani et al., 2021). After high light induction, modified genes of β-carotene hydroxylase (CRTR-B) and β-carotenoid ketolase (BKT), two key enzymes for astaxanthin synthesis in Haematococcus pluvialis, were integrated into the genome of Dunaliella viridis chloroplasts. The content of astaxanthin and carotenoid ketolase in Dunaliella viridis increased to 77.5 ± 7.7 and 50.1 ± 0.8 μg per gram of cell dry weight, respectively, after high light induction (Lin et al., 2019). In Chlamydomonas reinhardtii, overexpressing BKT led to a significant conversion of native carotenoids, with approximately 50% transformed into astaxanthin and over 70% into other key ketocarotenoids. This metabolic enhancement had no adverse effects on growth or biomass production, even under intense light conditions. Remarkably, the leading BKT overexpression strain achieved productivities of ketocarotenoid up to 4.3 mg/L/day (Perozeni et al., 2020). Overexpression of three genes, Violaxanthin de-epoxidase (Vde), Vde-related (Vdr), and Zeaxanthin epoxidase 3 (Zep3), increased the fucoxanthin content of Phaeodactylum tricornutum by 4-fold (Manfellotto et al., 2020). Mutants knocking out the zeaxanthin epoxidase gene in Chlamydomonas reinhardtii showed a 56-fold increase in zeaxanthin content and a 47-fold increase in productivity compared to wild-type zeaxanthin (Baek et al., 2018). Simultaneous knockdown of lycopene epsilon cyclase (LCYE) and zeaxanthin epoxidase gene (ZEP) in Chlamydomonas reinhardtii resulted in 60% more lutein production and content than wild-type zeaxanthin after three days of culture (Song et al., 2020). ZEP and ADP-glucose pyrophosphorylase gene (AGP) of Chlamydomonas reinhardtii were knocked out with CRISPR-Cas9 to increase oil and macular pigment production by more than 80% under nitrogen-deficient conditions (Song et al., 2022).
Figure 4 Overview of biosynthesis of carotenoids in the microalgal cell. The figure was adapted from previous publications (Kang et al., 2022; Srivastava et al., 2022). Arrows indicate biosynthetic pathways and dotted lines represent unknown biosynthetic processes. The brown text indicates enzymes in metabolic pathways. Light pink boxes indicate important pigments. BCH, β-carotene hydrolase; BKT, β-carotene ketolase; CRTISO, carotene isomerase; CRTR-B, β-carotene hydroxylase; CYP97A, cytochrome P450 carotene β-hydroxylase; CYP97C, cytochrome P450 carotene ϵ-hydroxylase; DMAPP, dimethylallyl pyrophosphate; GGPP, geranylgeranyl pyrophosphate; GGPPS, geranylgeranyl diphosphate synthase; IDI, isopentenyl-diphosphate delta-isomerase; LCYB, lycopene β-cyclase; LCYE, lycopene ϵ-cyclase; NXS, neoxanthin synthase; PDS, phytoene desaturase; PSY, phytoene synthase; VDE, violaxanthin de-epoxidase; ZEP, zeaxanthin epoxidase; ZDS, ζ-carotene desaturase. Created with BioRender.com.
Phycobiliproteins are colored biliproteins with tetrapyrrole chromophores for extra light harvesting in cyanobacteria and red algae. They are classified as phycoerythrin (red biliprotein), phycocyanin (blue pigment-protein complex), and allophycocyanin based on their absorption maxima (Pagels et al., 2020). For a concise representation of the phycobiliprotein synthesis pathway, refer to Figure 5. Phycobiliprotein biosynthesis occurs through the enzymatic action of FeCh/HemH to form heme. Their biosynthesis is initiated when protoporphyrin IX is converted to heme. Afterward, heme oxygenase catalyzes the synthesis of biliverdin IX which is subsequently isomerized to yield PCBs (Willows, 2020). It has been shown that the expression level of the cpeB gene was upregulated approximately 5-fold in Rhodomonas sp. under optimal conditions for phycoerythrin production compared to the control group, implying that the cpeB gene may be a potential regulatory gene for promoting phycoerythrin production (Derbel et al., 2022). The gene encoding chlorophyll synthase (CHS1) silencing mutant of Porphyridium purpureum had a maximum increase in phycoerythrin content of 46.5% over the wild type in white light and could reach a maximum of 63.3% (37.03 mg/L) in red light (Jeon et al., 2021). Additionally, overexpression of cobA/hemD, hemG, and ho genes increased 29.3% phycocyanin concentration than the wild type in Arthrospira platensis (Manirafasha et al., 2018).
Figure 5 Overview of biosynthesis of phycobiliproteins in microalgae. The figure was adapted from previous publications (Willows, 2020; Srivastava et al., 2022). Arrows indicate biosynthetic pathways. The brown text indicates enzymes in metabolic pathways. Light red and blue boxes indicate important pigments. 3Z-PCB, 3Z-phycocyanobilin; 3Z-PEB, 3Z-phycoerythrobilin; BV, biliverdin IX a; DHBV, 15,16-dihydrobiliverdin; FeCh/HemH, ferrochelatase; Ho, heme oxygenase; PcyA, phycocyanobilin:ferredoxin oxidoreductase; PebA, 15,16-dihydrobiliverdin:ferredoxin oxidoreductase; PebB, phycoerythrobilin:ferredoxin oxidoreductase; PPIX, protoporphyrin IX. Created with BioRender.com.
Microalgae are capable of producing LC-PUFAs, including omega-3 (ω-3) and omega-6 (ω-6) fatty acids. These LC-PUFAs are distinguished based on the length of their carbon chains and the position of the first double bond, and they are widely acknowledged for their significant health benefits (Muñoz et al., 2021; Santin et al., 2022). Among these LC-PUFAs, eicosapentaenoic acid (EPA, C20:5) and docosahexaenoic acid (DHA, C22:6) belong to the ω-3 family, while arachidonic acid (ARA, C20:4) is part of the ω-6 family, making them particularly noteworthy targets in microalgae metabolic engineering endeavors.
The biosynthesis of LC-PUFAs in microalgae involves a series of desaturation and elongation reactions occurring within the endoplasmic reticulum (ER). Starting from stearic acid (18:0, SA), the pathway leads to the production of linoleic acid (18:2, LA), which is further transformed into arachidonic acid (ARA) and eicosapentaenoic acid (EPA) through the catalytic actions of Δ6 desaturases (Δ6-DES) and Δ5 desaturases (Δ5-DES), respectively. EPA can be further elongated and converted into docosahexaenoic acid (DHA) through additional enzymatic reactions involving Δ5 elongases (Δ5-ELO) and Δ4 desaturases (Δ4-DES) (Kang et al., 2022).
Metabolic engineering approaches have shown promising results in enhancing LC-PUFA production in microalgae. For instance, co-expression of desaturase 5b, which contributes to fatty acid desaturation, and malonyl CoA-acyl carrier protein transacylase, which catalyzes type II fatty acid synthesis, resulted in increased LC-PUFAs in Phaeodactylum tricornutum, with ARA, DHA, and EPA at 18.98 mg/g, 9.15 mg/g, and 85.35 mg/g dry weight, respectively (Wang et al., 2017). Regulation of three lipid-related genes in Chlamydomonas reinhartii: overexpression of DNA-binding with one finger (DOF) and knockdown of LACS2 and CIS increased intracellular lipid and unsaturated fatty acid content by 142% and 52%, respectively (Jia et al., 2022). Overexpression of fatty acid desaturases (FAD) increased LC-PUFA content in Nannochloropsis oceanica, where overexpression of Δ12 or Δ5 FAD coding sequences increased the molar ratio of EPA by 25% (Poliner et al., 2018). Overexpression of the key enzyme for modified fatty acids, Δ9-desaturase, in Phaeodactylum tricornutum, resulted in a 1.32 ~ 1.42-fold increase in EPA accumulation and a 31.88 ~ 42.03% increase in FA content (Smith et al., 2021). Overexpression of type 2 acyl-CoA: diacylglycerol acyltransferase (DGAT) in Phaeodactylum tricornutum resulted in a 3.8 ~ 8.5% increase in DHA content and 2.8 and 2-fold increases in EPA and DPA, respectively, compared to wild species in the presence of nitrogen deprivation (Haslam et al., 2020).
Microalgae accumulate starch as a storage metabolite when subjected to nutrient deprivation such as nitrogen, phosphorus, and sulfur starvation. Starch in microalgae can be harnessed for the production of biofuels, such as bioethanol and biohydrogen, as well as serve as a feedstock for bio-based chemicals (Ran et al., 2019; Maia et al., 2020). Starch synthesis in microalgae occurs in the chloroplast stroma, where glyceraldehyde 3-phosphate is converted into starch through a series of reactions. Starch is a high molecular weight d-glucose polymer linked by α-1,4 glycosidic bonds and is temporarily stored as insoluble granules within chloroplasts (Masojídek et al., 2013). The synthesis involves glucose activation, chain elongation, and chain branching steps (Ran et al., 2019). The critical step is the formation of nucleoside-diphosphate-glucose (ADP-glucose) from glucose 1-phosphate and ATP, catalyzed by the enzyme ADP-glucose pyrophosphorylase. ADP-glucose then serves as the glycosyl donor for starch chain elongation by starch synthase. Strategies to promote starch accumulation in microalgae often focus on regulating ADP-glucose pyrophosphorylase, as it directly influences starch formation (Choix et al., 2014; de Carvalho Silvello et al., 2022).
Omics tools and genetic validations have shed light on the pathways and enzymes involved in starch biosynthesis under nutrient depletion, facilitating strain development and engineering for enhanced starch production. Furthermore, modifications in lipid biosynthesis key enzymes have been found to enhance starch accumulation due to shared precursors between starch and lipids (de Carvalho Silvello et al., 2022; Pandey et al., 2022). In Chlamydomonas reinhardtii, co-expression of FAX1, FAX2, and ABCA2 genes resulted in a 1.8-fold increase in starch content under nitrogen-deficient conditions and a 53% increase under normal conditions. These genes are involved in lipid transport and their synergistic co-expression enhanced starch synthesis by upregulating STA1 gene expression and increasing AGPase enzymatic activity (Chen et al., 2023). Moreover, the pfl1 mutant of Chlamydomonas reinhardtii, which lacks pyruvate formate lyase activity, exhibited almost 2 times higher starch accumulation during the initial stages of sulfur deprivation compared to the wild type (Volgusheva et al., 2022). Interestingly, a study on Tetraselmis chui revealed that it can accumulate starch up to 56% of its relative biomass content under nitrogen deprivation, with starch-related gene regulation having a limited impact on starch accumulation. This finding suggests that starch content in Tetraselmis chui is primarily regulated through post-transcriptional mechanisms (Carnovale et al., 2022). In conclusion, the exploration of metabolic engineering technologies to improve carbohydrate biosynthesis in microalgae is still limited and requires further research.
Expanding beyond their role in starch production and metabolic engineering strategies, microalgae offer a versatile platform for protein production as well. Microalgae are abundant in proteins, constituting up to 70% of the biomass dry weight in certain species. Notable examples of protein-rich microalgae include Arthrospira, Chlorella, Scenedesmusi, Tetraselmis, and Phaeodactylum (Kumar R. et al., 2022; Lucakova et al., 2022). While there has been limited research on using metabolic engineering to enhance endogenous protein expression in microalgae, there have been significant studies focusing on the expression of exogenous proteins (Banerjee and Ward, 2022). To improve protein yield in microalgae through metabolic engineering, several strategies can be employed, including optimizing genetic elements for protein expression, such as using expression vectors or integrating heterologous genes into the nuclear or chloroplast genome (Wang K. et al., 2020; Wang et al., 2021). Manipulating the promoter and terminator regions of the gene of interest can also enhance protein expression (de Grahl et al., 2020). Other techniques involve codon optimization, gene stacking, and gene silencing (Liu et al., 2021).
Notably, recent research has demonstrated the successful production of bioactive proteins in microalgae. For instance, an inducible system based on the nuclear-encoded translation enhancer TDA1 (cTDA1) was successfully introduced in Chlamydomonas reinhardtii’s chloroplast, resulting in a ~1.9-fold increase in recombinant protein (GFP) production compared to the original expression system, showcasing the potential for enhanced protein yield through nuclear inducible chloroplast regulation (Carrera-Pacheco et al., 2020). In another study, a phosphate-induced plasmid expression system in Phaeodactylum tricornutum achieved a yield of 6.8 µg/L of the SARS-CoV-2 spike-protein receptor-binding domain (RBD), demonstrating the versatility and efficiency of microalgae as a platform for recombinant protein production (Slattery et al., 2022). Furthermore, novel, stable, and less light-dependent promoter and terminator pairs (Nub, SVP, 45582, and Pbt) were identified in Phaeodactylum tricornutum, offering the potential for fine-tuning gene expression and enhancing protein production under various growth conditions, including nitrogen limitation (Windhagauer et al., 2021).
In addition to their ability to produce valuable metabolites such as fatty acids, pigments, and proteins, microalgae also offer the potential for biohydrogen production through various pathways. Biohydrogen is a clean and renewable energy source with great promise for sustainable energy generation (Calijuri et al., 2022; Zhang et al., 2023). Microalgae possess two primary pathways for hydrogen production: photolysis and fermentation. In photolysis, particularly in green algae, light energy is harnessed to split water molecules into oxygen and hydrogen (Bechara et al., 2021). In contrast, fermentation involves anaerobic respiration by microalgae such as Lyngbya limnetica and Scenedesmus, resulting in the breakdown of organic matter into hydrogen gas and other byproducts (Srivastava et al., 2021).
Metabolic engineering plays a crucial role in maximizing hydrogen production in microalgae (Khan and Fu, 2020). Through targeted genetic modifications, researchers can manipulate pathways and key enzymes to optimize the metabolic flux toward hydrogen synthesis. One effective approach involves the deletion of the nitrate assimilation pathway in unicellular cyanobacteria (Synechocystis sp.) during dark fermentation, as it competes with hydrogenase for electrons. Eliminating this pathway significantly increased hydrogen production, with mutant strains showing up to 140 times higher hydrogen accumulation compared to the wild-type (Baebprasert et al., 2011).
Engineering hydrogenase and nitrogenase activity has been pivotal in promoting hydrogen production. Strategies include altering their structures, introducing foreign gene expressions, modifying oxygen absorption, and reducing hydrogen consumption (Li et al., 2022). Notably, Fe-Fe hydrogenase has exhibited 100 times higher activity than other hydrogenase types, making it a promising candidate for improving hydrogen production (Razu et al., 2019; Pandey et al., 2021).
A significant challenge in this field has been addressing the inhibition of hydrogenases by oxygen (Lu and Koo, 2019). To overcome this limitation, researchers have explored approaches such as limiting oxygen access to the enzyme’s active site through overexpression of hydrogenases with high oxygen tolerance. Site-directed mutagenesis has also been utilized to enhance oxygen tolerance, resulting in mutants with 30-fold increased hydrogen production compared to wild-type strains (Yang et al., 2019).
Under sulfur-deficient conditions, microalgae can sustain hydrogen production due to the limitation of PSII activity and reduced photosynthetic oxygen release (Sivaramakrishnan and Incharoensakdi, 2021). MicroRNAs (miRNAs) have been found to play a role in this process, with their up-regulation under sulfur-deficient conditions potentially influencing metabolic processes that lead to increased hydrogen production (Xu et al., 2019).
In addition to hydrogenases, nitrogenase is another critical enzyme involved in hydrogen production. Strategies have been developed to enhance biohydrogen production by selecting strains with high nitrogenase activity and suppressing the activity of Hup uptake hydrogenase (Tanvir et al., 2021). Eliminating the Hup activity proved highly effective in enhancing hydrogen accumulation in the strain. A mutant of cyanobacteria Nostoc sp. PCC 7422, developed with a hupL gene insertional disruption (ΔhupL), exhibited a 4 ~ 7 times increase in hydrogen production rate during the optimal hydrogen production stage compared to the wild-type strain (Yoshino et al., 2007). To maximize nitrogenase expression, cyanobacteria lacking the nif gene cluster have been used as host organisms. The integration of a minimal nif gene cluster from Bacteroides into the genome of non-nitrogen-fixing Synechococcus elongatus PCC 7942 has shown promise in creating a bioelectrochemical nitrogen fixation (e-BNF) system, which reached 21 times nitrogen fixation efficiency that of control groups (Dong et al., 2021).
Microalgae, with their exceptional photosynthetic efficiency, are increasingly recognized as a promising avenue for sustainable biomass production. To comprehensively evaluate their environmental sustainability, life cycle assessment (LCA) emerges as an invaluable tool. LCA encompasses various impact categories, including acidification potential (AP) and global warming potential (GWP) (Amini Toosi et al., 2020), providing a holistic view of microalgae-based processes, from cultivation to crucial processing stages like harvesting, dewatering, and extraction (Ketzer et al., 2018). This approach offers insights into resource utilization, emissions, and ecological footprint, providing a comprehensive environmental performance overview (de Souza et al., 2019).
Numerous studies have scrutinized the environmental sustainability of microalgal-based processes, particularly in bioenergy production. Exploring biodiesel production from Nannochloropsis in Denmark, multiple technology scenarios, including CO2 utilization, have demonstrated the potential to reduce greenhouse gas emissions and achieve lower GWP compared to fossil diesel (Monari et al., 2016). Simultaneously, the synergy of flare gas with a microalgae biorefinery reveals the possibility of a negative GWP, emphasizing the environmental advantages of these innovative methods (Beal et al., 2016). A techno-economic analysis and life cycle impact assessment evaluated the co-production of microalgae-based plastic feedstock with fuel, revealing substantial reductions of greenhouse gas emissions, ranging from 67 to 116%, compared to standard petroleum-based equivalents (Beckstrom et al., 2020).
While microalgae inherently sequester the greenhouse gas CO2 via photosynthesis during growth, emissions can still occur at production stages. Innovative strategies are being developed to reduce carbon footprints further. Notably, harnessing waste streams, such as digestate from anaerobic digestion processes, as alternative carbon sources and nutrients for microalgal cultivation is gaining attention (Collotta et al., 2018; Bussa et al., 2020). This approach highlights microalgae’s potential not only to reduce environmental pressures but also to contribute positively to waste management and resource utilization.
To further enhance environmental sustainability, it’s crucial to consider critical impact categories like GWP, which should be included in LCA analyses (Ubando et al., 2022). In line with this, strategies for improving the sustainability of microalgal biorefineries involve embracing circular bioeconomy concepts, optimizing waste biorefinery supply chains, and integrating innovative technologies such as thermochemical processes (Ioannidou et al., 2020). Additionally, the production of high-value products, such as antioxidants and PUFAs, within microalgal biorefineries can offset energy consumption and costs (Cortés et al., 2019; López et al., 2019). Integrating biochar production and wastewater treatment into microalgal biorefinery processes holds the potential for mitigating carbon emissions (De Bhowmick et al., 2019). Process systems engineering techniques and artificial neural networks are instrumental in systematically integrating technologies within biorefineries, aligning with circular economy principles (Gue et al., 2020).
Microalgae cultivation systems play a pivotal role in the sustainable production of microalgal biomass, which finds applications ranging from biofuels to high-value bioproducts. In addition to the commonly recognized open ponds (OPs) and photobioreactors (PBRs), there are two other noteworthy cultivation systems: two-stage hybrid cultivation systems (TSHCSs) and attached growth systems. These systems offer unique advantages and characteristics, making them significant contenders in the realm of microalgal biomass production. To provide a comprehensive overview, Table 3 outlines different cultivation systems along with their key features.
Techno-economic analysis (TEA) has been extensively employed to assess the economic viability of diverse microalgae cultivation systems, encompassing open raceway ponds (ORPs), photobioreactors (PBRs), and algal turf scrubbers. ORPs stand out as a cost-effective choice for large-scale microalgae cultivation, primarily due to their relatively modest capital expenditures (CapEx). Nevertheless, the challenges linked to high evaporative losses and contamination risks necessitate design enhancements and energy-efficient strategies to boost economic efficiency while sustaining high productivity (Bhatt et al., 2022). On the flip side, PBRs offer significantly superior biomass productivity, often surpassing open ponds (OPs) by a factor of 5 to 10 (Valdovinos-García et al., 2021). However, these systems come with substantial costs, including those associated with artificial lighting systems, gas cylinders, and cooling mechanisms (Kumar et al., 2021). TSHCSs offer higher biomass productivity and a lower carbon footprint compared to ORPs and PBRs (Aziz et al., 2020). Attached growth systems are considered economically favorable due to reduced biomass harvesting costs (Singh and Patidar, 2021). However, both these cultivation systems require further TEA to assess their economic viability, taking into account factors such as CO2 supply, CapEx, and labor costs.
The economic sustainability of microalgae cultivation systems is the result of a multifaceted interplay between various factors, encompassing CapEx, operational expenses (OpEx), energy consumption, cost-effectiveness, and biomass yields. To realize economic sustainability, concerted efforts should be directed toward minimizing diverse cost components. Currently, CapEx looms as a prominent economic focal point across most microalgae cultivation systems, with construction and raw material expenditures accounting for up to 88% of CapEx (Banerjee and Ramaswamy, 2019). Reducing the biomass production cost (BPC) is a pivotal endeavor, and scaling up microalgae cultivation facilities from 1 hectare to 10 hectares could yield a reduction of up to 67% in BPC (Schipper et al., 2021). Optimization of cultivation systems to enhance microalgae productivity is imperative. In this regard, a more profound comprehension of PBRs and a reduction in TEA prediction biases, guided by actual cultivation data, are critical. As technology advances and economic landscapes evolve, the economic sustainability of microalgae cultivation systems will continue to undergo refinement and improvement.
Microalgae have emerged as a promising source of biomass for producing food, pharmaceuticals, and other valuable compounds due to their high photosynthetic efficiency and rapid growth rate. Enhancing the efficiency of microalgal photosynthesis is fundamental to improving their potential as cell factories. Researchers have employed mutagenesis and ALE to obtain high-quality algal species and collect physiological and biochemical data to achieve optimal nutritional production and improved photosynthetic efficiency. However, while metabolic engineering has successfully increased target compound production, its impact on the growth and physiology of microalgal cells needs careful consideration. Modifications targeting essential components like RuBisCO and light-harvesting antenna complexes, which are prevalent in higher plants, have not been widely adopted in microalgae. Thus, a systematic metabolic engineering strategy, along with advanced genetic engineering tools specifically designed for microalgae, is necessary. Addressing challenges associated with these approaches, such as off-target effects and regulatory issues, requires further research.
Advancements in genetic engineering tools and metabolic engineering offer immense potential for the development of sustainable microalgal cell factories. To realize this potential, worldwide partnerships, and effective gene annotation tools are required to build a comprehensive collection of multi-omics data encompassing various microalgal species. These technological advancements hold significant implications for achieving global targets, such as reducing carbon emissions and meeting the United Nations Sustainable Development Goals (SDGs). Microalgae-based biorefineries can contribute to SDG targets related to climate action (SDG 13), affordable and clean energy (SDG 7), responsible consumption and production (SDG 12), and good health and well-being (SDG 3).
Continued efforts in improving microalgal productivity and photosynthetic efficiency through metabolic engineering, multi-omics technology, and synthetic biology are vital for the development of sustainable microalgal biorefineries. By leveraging these technologies and fostering international collaboration, microalgae-based biorefineries can significantly contribute to the global endeavor of achieving the SDGs and mitigating climate change.
JH: Writing – original draft, Writing – review & editing. WM: Writing – original draft, Writing – review & editing. YS: Writing – review & editing. CQ: Writing – review & editing. WF: Funding acquisition, Supervision, Writing – review & editing.
The author(s) declare financial support was received for the research, authorship, and/or publication of this article. This study was supported by the Hundred Talents Program of Zhejiang University, Science Foundation of Donghai Laboratory (Grant No. DH-2022KF0203), Key Scientific Research and Development Program of Hangzhou (Grant No. 202204T14), Icelandic Research Fund (grant number 207298-052), and Technology Development Fund (grant number 163922-0613).
The authors declare that the research was conducted in the absence of any commercial or financial relationships that could be construed as a potential conflict of interest.
The author(s) declared that they were an editorial board member of Frontiers, at the time of submission. This had no impact on the peer review process and the final decision.
All claims expressed in this article are solely those of the authors and do not necessarily represent those of their affiliated organizations, or those of the publisher, the editors and the reviewers. Any product that may be evaluated in this article, or claim that may be made by its manufacturer, is not guaranteed or endorsed by the publisher.
Ajjawi I., Verruto J., Aqui M., Soriaga L. B., Coppersmith J., Kwok K., et al. (2017). Lipid production in Nannochloropsis gaditana is doubled by decreasing expression of a single transcriptional regulator. Nat. Biotechnol. 35, 647–652. doi: 10.1038/nbt.3865
Al-Dailami A., Koji I., Ahmad I., Goto M. (2022). Potential of photobioreactors (PBRs) in cultivation of microalgae. J. Adv. Res. Appl. Sci. Eng. Technol. 27, 32–44. doi: 10.37934/araset.27.1.3244
Ambati R. R., Gogisetty D., Aswathanarayana R. G., Ravi S., Bikkina P. N., Bo L., et al. (2019). Industrial potential of carotenoid pigments from microalgae: Current trends and future prospects. Crit. Rev. Food Sci. Nutr. 59, 1880–1902. doi: 10.1080/10408398.2018.1432561
Amini Toosi H., Lavagna M., Leonforte F., Del Pero C., Aste N. (2020). Life cycle sustainability assessment in building energy retrofitting; A review. Sustain. Cities Soc 60, 102248. doi: 10.1016/j.scs.2020.102248
Assil-Companioni L., Büchsenschütz H. C., Solymosi D., Dyczmons-Nowaczyk N. G., Bauer K. K. F., Wallner S., et al. (2020). Engineering of NADPH supply boosts photosynthesis-driven biotransformations. ACS Catal. 10, 11864–11877. doi: 10.1021/acscatal.0c02601
Assunção J., Malcata F. X. (2020). Enclosed “non-conventional” photobioreactors for microalga production: A review. Algal Res. 52, 102107. doi: 10.1016/j.algal.2020.102107
Aziz M. M. A., Kassim K. A., Shokravi Z., Jakarni F. M., Liu H. Y., Zaini N., et al. (2020). Two-stage cultivation strategy for simultaneous increases in growth rate and lipid content of microalgae: A review. Renew. Sustain. Energy Rev. 119, 109621. doi: 10.1016/j.rser.2019.109621
Baebprasert W., Jantaro S., Khetkorn W., Lindblad P., Incharoensakdi A. (2011). Increased H2 production in the cyanobacterium Synechocystis sp. strain PCC 6803 by redirecting the electron supply via genetic engineering of the nitrate assimilation pathway. Metab. Eng. 13, 610–616. doi: 10.1016/j.ymben.2011.07.004
Baek K., Yu J., Jeong J., Sim S. J., Bae S., Jin E. (2018). Photoautotrophic production of macular pigment in a Chlamydomonas reinhardtii strain generated by using DNA-free CRISPR-Cas9 RNP-mediated mutagenesis. Biotechnol. Bioeng. 115, 719–728. doi: 10.1002/bit.26499
Bai F., Zhang Y., Liu J. (2021). A bZIP transcription factor is involved in regulating lipid and pigment metabolisms in the green alga Chlamydomonas reinhardtii. Algal Res. 59, 102450. doi: 10.1016/j.algal.2021.102450
Banerjee S., Ramaswamy S. (2019). Comparison of productivity and economic analysis of microalgae cultivation in open raceways and flat panel photobioreactor. Bioresour. Technol. Rep. 8, 100328. doi: 10.1016/j.biteb.2019.100328
Banerjee A., Ward V. (2022). Production of recombinant and therapeutic proteins in microalgae. Curr. Opin. Biotechnol. 78, 102784. doi: 10.1016/j.copbio.2022.102784
Bar-Even A. (2016). Formate assimilation: the metabolic architecture of natural and synthetic pathways. Biochemistry 55, 3851–3863. doi: 10.1021/acs.biochem.6b00495
Bar-Even A. (2018). Daring metabolic designs for enhanced plant carbon fixation. Plant Sci. 273, 71–83. doi: 10.1016/j.plantsci.2017.12.007
Barrangou R., Fremaux C., Deveau H., Richards M., Boyaval P., Moineau S., et al. (2007). CRISPR provides acquired resistance against viruses in prokaryotes. Science 315, 1709–1712. doi: 10.1126/science.1138140
Beal C. M., Davidson F. T., Webber M. E., Quinn J. C. (2016). Flare gas recovery for algal protein production. Algal Res. 20, 142–152. doi: 10.1016/j.algal.2016.09.022
Beardall J., Raven J. A. (2016). “Carbon acquisition by microalgae,” in The Physiology of Microalgae Developments in Applied Phycology. Eds. Borowitzka M. A., Beardall J., Raven J. A. (Cham: Springer International Publishing), 89–99. doi: 10.1007/978-3-319-24945-2_4
Bechara R., Azizi F., Boyadjian C. (2021). Process simulation and optimization for enhanced biophotolytic hydrogen production from green algae using the sulfur deprivation method. Int. J. Hydrog. Energy 46, 14096–14108. doi: 10.1016/j.ijhydene.2021.01.115
Beckstrom B. D., Wilson M. H., Crocker M., Quinn J. C. (2020). Bioplastic feedstock production from microalgae with fuel co-products: A techno-economic and life cycle impact assessment. Algal Res. 46, 101769. doi: 10.1016/j.algal.2019.101769
Behrenfeld M. J., Randerson J. T., McClain C. R., Feldman G. C., Los S. O., Tucker C. J., et al. (2001). Biospheric primary production during an ENSO transition. Science 291, 2594–2597. doi: 10.1126/science.1055071
Bekirogullari M., Pittman J. K., Theodoropoulos C. (2018). Multi-factor kinetic modelling of microalgal biomass cultivation for optimised lipid production. Bioresour. Technol. 269, 417–425. doi: 10.1016/j.biortech.2018.07.121
Bhatt A., Khanchandani M., Rana M. S., Prajapati S. K. (2022). Techno-economic analysis of microalgae cultivation for commercial sustainability: A state-of-the-art review. J. Clean. Prod. 370, 133456. doi: 10.1016/j.jclepro.2022.133456
Bleisch R., Freitag L., Ihadjadene Y., Sprenger U., Steingröwer J., Walther T., et al. (2022). Strain development in microalgal biotechnology—Random mutagenesis techniques. Life 12, 961–961. doi: 10.3390/life12070961
Broddrick J. T., Ware M. A., Jallet D., Palsson B. O., Peers G. (2022). Integration of physiologically relevant photosynthetic energy flows into whole genome models of light-driven metabolism. Plant J. 112, 603–621. doi: 10.1111/tpj.15965
Bussa M., Zollfrank C., Röder H. (2020). Life-cycle assessment and geospatial analysis of integrating microalgae cultivation into a regional economy. J. Clean. Prod. 243, 118630. doi: 10.1016/j.jclepro.2019.118630
Butler T., Kapoore R. V., Vaidyanathan S. (2020). Phaeodactylum tricornutum: A diatom cell factory. Trends Biotechnol. 38, 606–622. doi: 10.1016/j.tibtech.2019.12.023
Calijuri M. L., Silva T. A., Magalhães I. B., Pereira A. S. A., de P., Marangon B. B., et al. (2022). Bioproducts from microalgae biomass: Technology, sustainability, challenges and opportunities. Chemosphere 305, 135508. doi: 10.1016/j.chemosphere.2022.135508
Carnovale G., Lama C., Torres S., Rosa F., Mantecón L., Horn S. J., et al. 2022). Metabolic pathways for biosynthesis and degradation of starch in Tetraselmis chui during nitrogen deprivation and recovery. Bioresour. Technol. 354, 127222. doi: 10.1016/j.biortech.2022.127222
Carrasco-Reinado R., Escobar A., Carrera C., Guarnizo P., Vallejo R. A., Fernández-Acero F. J. (2019). Valorization of microalgae biomass as a potential source of high-value sugars and polyalcohols. LWT 114, 108385. doi: 10.1016/j.lwt.2019.108385
Carrera-Pacheco S. E., Hankamer B., Oey M. (2020). Light and heat-shock mediated TDA1 overexpression as a tool for controlled high-yield recombinant protein production in Chlamydomonas reinhardtii chloroplasts. Algal Res. 48, 101921. doi: 10.1016/j.algal.2020.101921
Chen R., Yamaoka Y., Feng Y., Chi Z., Xue S., Kong F. (2023). Co-Expression of Lipid Transporters Simultaneously Enhances Oil and Starch Accumulation in the Green Microalga Chlamydomonas reinhardtii under Nitrogen Starvation. Metabolites 13, 115. doi: 10.3390/metabo13010115
Cheng J., Li K., Yang Z., Lu H., Zhou J., Cen K. (2016). Gradient domestication of Haematococcus pluvialis mutant with 15% CO2 to promote biomass growth and astaxanthin yield. Bioresour. Technol. 216, 340–344. doi: 10.1016/j.biortech.2016.05.095
Chew K. W., Chia S. R., Show P. L., Yap Y. J., Ling T. C., Chang J.-S. (2018). Effects of water culture medium, cultivation systems and growth modes for microalgae cultivation: A review. J. Taiwan Inst. Chem. Eng. 91, 332–344. doi: 10.1016/j.jtice.2018.05.039
Choix F. J., Bashan Y., Mendoza A., de-Bashan L. E. (2014). Enhanced activity of ADP glucose pyrophosphorylase and formation of starch induced by Azospirillum brasilense in Chlorella vulgaris. J. Biotechnol. 177, 22–34. doi: 10.1016/j.jbiotec.2014.02.014
Collotta M., Champagne P., Mabee W., Tomasoni G. (2018). Wastewater and waste CO2 for sustainable biofuels from microalgae. Algal Res. 29, 12–21. doi: 10.1016/j.algal.2017.11.013
Cortés A., Moreira M. T., Feijoo G. (2019). Integrated evaluation of wine lees valorization to produce value-added products. Waste Manag. 95, 70–77. doi: 10.1016/j.wasman.2019.05.056
Crozet P., Navarro F. J., Willmund F., Mehrshahi P., Bakowski K., Lauersen K. J., et al. (2018). Birth of a photosynthetic chassis: A MoClo toolkit enabling synthetic biology in the microalga Chlamydomonas reinhardtii. ACS Synth. Biol. 7, 2074–2086. doi: 10.1021/acssynbio.8b00251
Cruz-López R., Maske H. (2016). The Vitamin B1 and B12 Required by the Marine Dinoflagellate Lingulodinium polyedrum Can be Provided by its Associated Bacterial Community in Culture. Front. Microbiol. 7. doi: 10.3389/fmicb.2016.00560
De Bhowmick G., Sarmah A. K., Sen R. (2019). Zero-waste algal biorefinery for bioenergy and biochar: A green leap towards achieving energy and environmental sustainability. Sci. Total Environ. 650, 2467–2482. doi: 10.1016/j.scitotenv.2018.10.002
de Carvalho Silvello M. A., Severo Gonçalves I., Patrícia Held Azambuja S., Silva Costa S., Garcia Pereira Silva P., Oliveira Santos L., et al. (2022). Microalgae-based carbohydrates: A green innovative source of bioenergy. Bioresour. Technol. 344, 126304. doi: 10.1016/j.biortech.2021.126304
de Grahl I., Rout S. S., Maple-Grødem J., Reumann S. (2020). Development of a constitutive and an auto-inducible high-yield expression system for recombinant protein production in the microalga Nannochloropsis oceanica. Appl. Microbiol. Biotechnol. 104, 8747–8760. doi: 10.1007/s00253-020-10789-4
Deniz I. (2020). Scaling-up of Haematococcus pluvialis production in stirred tank photobioreactor. Bioresour. Technol. 310, 123434. doi: 10.1016/j.biortech.2020.123434
Derbel H., Elleuch J., Tounsi L., Nicolo M. S., Rizzo M. G., Michaud P., et al. (2022). Improvement of biomass and phycoerythrin production by a strain of Rhodomonas sp. Isolated from the Tunisian coast of Sidi Mansour. Biomolecules 12, 885. doi: 10.3390/biom12070885
de Souza M. P., Hoeltz M., Brittes Benitez L., MaChado Ê. L., de Souza Schneider R., de C. (2019). Microalgae and clean technologies: A review. CLEAN – Soil Air Water 47, 1800380. doi: 10.1002/clen.201800380
Dong F., Lee Y. S., Gaffney E. M., Grattieri M., Haddadin H., Minteer S. D., et al. (2021). An engineered, non-diazotrophic cyanobacterium and its application in bioelectrochemical nitrogen fixation. Cell Rep. Phys. Sci. 2, 100444. doi: 10.1016/j.xcrp.2021.100444
Duman-Özdamar Z. E., Binay B. (2021). Production of Industrial Enzymes via Pichia pastoris as a Cell Factory in Bioreactor: Current Status and Future Aspects. Protein J. 40, 367–376. doi: 10.1007/s10930-021-09968-7
Einhaus A., Baier T., Rosenstengel M., Freudenberg R. A., Kruse O. (2021). Rational promoter engineering enables robust terpene production in microalgae. ACS Synth. Biol. 10, 847–856. doi: 10.1021/acssynbio.0c00632
Erickson E., Wakao S., Niyogi K. K. (2015). Light stress and photoprotection in Chlamydomonas reinhardtii. Plant J. 82, 449–465. doi: 10.1111/tpj.12825
Faried M., Samer M., Moselhy M. A., Yousef R. S., Ali A. S., Ahmed R. H., et al. (2022). Photobiostimulation of green microalga Chlorella sorokiniana using He–Ne red laser radiation for increasing biodiesel production. Biomass Convers. Biorefinery, 1–15. doi: 10.1007/s13399-021-02220-3
Fernandes T., Cordeiro N. (2021). Microalgae as sustainable biofactories to produce high-value lipids: biodiversity, exploitation, and biotechnological applications. Mar. Drugs 19, 573. doi: 10.3390/md19100573
Finazzi G., Minagawa J. (2014). “High light acclimation in green microalgae,” in Non-Photochemical Quenching and Energy Dissipation in Plants, Algae and Cyanobacteria Advances in Photosynthesis and Respiration. Eds. Demmig-Adams B., Garab G., Adams W. III, Govindjee (Dordrecht: Springer Netherlands), 445–469. doi: 10.1007/978-94-017-9032-1_21
Formighieri C., Franck F., Bassi R. (2012). Regulation of the pigment optical density of an algal cell: Filling the gap between photosynthetic productivity in the laboratory and in mass culture. J. Biotechnol. 162, 115–123. doi: 10.1016/j.jbiotec.2012.02.021
Friedland N., Negi S., Vinogradova-Shah T., Wu G., Ma L., Flynn S., et al. (2019). Fine-tuning the photosynthetic light harvesting apparatus for improved photosynthetic efficiency and biomass yield. Sci. Rep. 9, 1–12. doi: 10.1038/s41598-019-49545-8
Fu W., Chaiboonchoe A., Khraiwesh B., Sultana M., Jaiswal A., Jijakli K., et al. (2017). Intracellular spectral recompositioning of light enhances algal photosynthetic efficiency. Sci. Adv. 3, e1603096. doi: 10.1126/sciadv.1603096
Fu W., Gudmundsson O., Feist A. M., Herjolfsson G., Brynjolfsson S., Palsson B. Ø. (2012). Maximizing biomass productivity and cell density of Chlorella vulgaris by using light-emitting diode-based photobioreactor. J. Biotechnol. 161, 242–249. doi: 10.1016/j.jbiotec.2012.07.004
Gong Y., Kang N. K., Kim Y. U., Wang Z., Wei L., Xin Y., et al. (2020). The NanDeSyn database for Nannochloropsis systems and synthetic biology. Plant J. Cell Mol. Biol. 104, 1736–1745. doi: 10.1111/tpj.15025
Grama S. B., Liu Z., Li J. (2022). Emerging trends in genetic engineering of microalgae for commercial applications. Mar. Drugs 20, 285. doi: 10.3390/md20050285
Gue I. H. V., Ubando A. T., Tseng M.-L., Tan R. R. (2020). Artificial neural networks for sustainable development: a critical review. Clean Technol. Environ. Policy 22, 1449–1465. doi: 10.1007/s10098-020-01883-2
Guillén-García A., Gibson S. E. R., Jordan C. J. C., Ramaswamy V. K., Linthwaite V. L., Bromley E. H. C., et al. (2022). Allophycocyanin A is a carbon dioxide receptor in the cyanobacterial phycobilisome. Nat. Commun. 13, 5289. doi: 10.1038/s41467-022-32925-6
Guiry M. D. (2012). How many species of algae are there? J. Phycol. 48, 1057–1063. doi: 10.1111/j.1529-8817.2012.01222.x
Guo L., Diao W., Gao C., Hu G., Ding Q., Ye C., et al. (2020). Engineering Escherichia coli lifespan for enhancing chemical production. Nat. Catal. 3, 307–318. doi: 10.1038/s41929-019-0411-7
Haslam R. P., Hamilton M. L., Economou C. K., Smith R., Hassall K. L., Napier J. A., et al. (2020). Overexpression of an endogenous type 2 diacylglycerol acyltransferase in the marine diatom Phaeodactylum tricornutum enhances lipid production and omega-3 long-chain polyunsaturated fatty acid content. Biotechnol. Biofuels 13, 87. doi: 10.1186/s13068-020-01726-8
Hepburn C., Adlen E., Beddington J., Carter E. A., Fuss S., Mac Dowell N., et al. (2019). The technological and economic prospects for CO2 utilization and removal. Nature 575, 87–97. doi: 10.1038/s41586-019-1681-6
Huang Y., Cheng J., Lu H., He Y., Zhou J., Cen K. (2017). Transcriptome and key genes expression related to carbon fixation pathways in Chlorella PY-ZU1 cells and their growth under high concentrations of CO2. Biotechnol. Biofuels 10, 181. doi: 10.1186/s13068-017-0868-z
Huang J., Wan M., Jiang J., Zhang A., Zhang D. (2021). Evaluating the effects of geometry and arrangement parameter of flat panel photobioreactor on microalgae biomass production and economic performance in China. Algal Res. 57, 102343. doi: 10.1016/j.algal.2021.102343
Ioannidou S. M., Pateraki C., Ladakis D., Papapostolou H., Tsakona M., Vlysidis A., et al. (2020). Sustainable production of bio-based chemicals and polymers via integrated biomass refining and bioprocessing in a circular bioeconomy context. Bioresour. Technol. 307, 123093. doi: 10.1016/j.biortech.2020.123093
Işıl Ç., de Haan K., Göröcs Z., Koydemir H. C., Peterman S., Baum D., et al. (2021). Phenotypic analysis of microalgae populations using label-free imaging flow cytometry and deep learning. ACS Photonics 8, 1232–1242. doi: 10.1021/acsphotonics.1c00220
Janoska A., Barten R., de Nooy S., van Rijssel P., Wijffels R. H., Janssen M. (2018). Improved liquid foam-bed photobioreactor design for microalgae cultivation. Algal Res. 33, 55–70. doi: 10.1016/j.algal.2018.04.025
Jeon M. S., Han S.-I., Jeon M., Choi Y.-E. (2021). Enhancement of phycoerythrin productivity in Porphyridium purpureum using the clustered regularly interspaced short palindromic repeats/CRISPR-associated protein 9 ribonucleoprotein system. Bioresour. Technol. 330, 124974. doi: 10.1016/j.biortech.2021.124974
Jeon S., Lim J.-M., Lee H.-G., Shin S.-E., Kang N. K., Park Y.-I., et al. (2017). Current status and perspectives of genome editing technology for microalgae. Biotechnol. Biofuels 10, 267. doi: 10.1186/s13068-017-0957-z
Jerney J., Spilling K. (2018). “Large scale cultivation of microalgae: open and closed systems,” in Biofuels from Algae (Humana, New York, NY: Springer New York), 1–8. doi: 10.1007/7651_2018_130
Jia B., Yin J., Li X., Li Y., Yang X., Lan C., et al. (2022). Increased lipids in Chlamydomonas reinhardtii by multiple regulations of DOF, LACS2, and CIS1. Int. J. Mol. Sci. 23, 10176. doi: 10.3390/ijms231710176
Jinek M., Chylinski K., Fonfara I., Hauer M., Doudna J. A., Charpentier E. (2012). A programmable dual-RNA–guided DNA endonuclease in adaptive bacterial immunity. Science 337, 816–821. doi: 10.1126/science.1225829
Kaczmarski J. A., Hong N.-S., Mukherjee B., Wey L. T., Rourke L., Förster B., et al. (2019). Structural basis for the allosteric regulation of the sbtA bicarbonate transporter by the PII-like protein, SbtB, from Cyanobium sp. PCC7001. Biochemistry 58, 5030–5039. doi: 10.1021/acs.biochem.9b00880
Kang N. K., Baek K., Koh H. G., Atkinson C. A., Ort D. R., Jin Y.-S. (2022). Microalgal metabolic engineering strategies for the production of fuels and chemicals. Bioresour. Technol. 345, 126529. doi: 10.1016/j.biortech.2021.126529
Kaur R., Kumar A., Mandal D. (2023). “Insect cell factory for production of biomolecules,” in Biomanufacturing for Sustainable Production of Biomolecules. Eds. Singh V., Show P. L. (Singapore: Springer Nature), 283–296. doi: 10.1007/978-981-19-7911-8_14
Ketzer F., Skarka J., Rösch C. (2018). Critical review of microalgae LCA studies for bioenergy production. Bioenergy Res. 11, 95–105. doi: 10.1007/s12155-017-9880-1
Khan S., Fu P. (2020). Biotechnological perspectives on algae: a viable option for next generation biofuels. Curr. Opin. Biotechnol. 62, 146–152. doi: 10.1016/j.copbio.2019.09.020
Kim H. S., Hsu S.-C., Hsu S.-C., Han S.-I., Thapa H. R., Guzman A. R., et al. (2017). High-throughput droplet microfluidics screening platform for selecting fast-growing and high lipid-producing microalgae from a mutant library. Plant Direct 1, e00011. doi: 10.1002/pld3.11
Kirst H., Formighieri C., Melis A. (2014). Maximizing photosynthetic efficiency and culture productivity in cyanobacteria upon minimizing the phycobilisome light-harvesting antenna size. Biochim. Biophys. Acta 1837, 1653–1664. doi: 10.1016/j.bbabio.2014.07.009
Klassen V., Blifernez-Klassen O., Bax J., Kruse O. (2020). Wastewater-borne microalga Chlamydomonas sp.: A robust chassis for efficient biomass and biomethane production applying low-N cultivation strategy. Bioresour. Technol. 315, 123825. doi: 10.1016/j.biortech.2020.123825
Koh H. G., Kang N. K., Kim E. K., Jeon S., Shin S.-E., Lee B., et al. (2018). Advanced multigene expression system for Nannochloropsis salina using 2A self-cleaving peptides. J. Biotechnol. 278, 39–47. doi: 10.1016/j.jbiotec.2018.04.017
Kornienko N., Zhang J. Z., Sakimoto K. K., Yang P., Reisner E. (2018). Interfacing nature’s catalytic machinery with synthetic materials for semi-artificial photosynthesis. Nat. Nanotechnol. 13, 890–899. doi: 10.1038/s41565-018-0251-7
Koyande A. K., Chew K. W., Rambabu K., Tao Y., Chu D.-T., Show P.-L. (2019). Microalgae: A potential alternative to health supplementation for humans. Food Sci. Hum. Wellness 8, 16–24. doi: 10.1016/j.fshw.2019.03.001
Kumar R., Hegde A. S., Sharma K., Parmar P., Srivatsan V. (2022). Microalgae as a sustainable source of edible proteins and bioactive peptides – Current trends and future prospects. Food Res. Int. 157, 111338. doi: 10.1016/j.foodres.2022.111338
Kumar B. R., Mathimani T., Sudhakar M. P., Rajendran K., Nizami A.-S., Brindhadevi K., et al. (2021). A state of the art review on the cultivation of algae for energy and other valuable products: Application, challenges, and opportunities. Renew. Sustain. Energy Rev. 138, 110649. doi: 10.1016/j.rser.2020.110649
Kumar M., Zuniga C., Tibocha-Bonilla J. D., Smith S. R., Coker J., Allen A. E., et al. (2022). “Constraint-based modeling of diatoms metabolism and quantitative biology approaches,” in The Molecular Life of Diatoms. Eds. Falciatore A., Mock T. (Cham: Springer International Publishing), 775–808. doi: 10.1007/978-3-030-92499-7_26
Kupriyanova E. V., Pronina N. A., Los D. A. (2023). Adapting from low to high: an update to CO2-concentrating mechanisms of cyanobacteria and microalgae. Plants 12, 1569. doi: 10.3390/plants12071569
Lee J.-W., Lee M.-W., Ha J.-S., Kim D.-S., Jin E., Lee H.-G., et al. (2020). Development of a species-specific transformation system using the novel endogenous promoter calreticulin from oleaginous microalgae Ettlia sp. Sci. Rep. 10, 13947. doi: 10.1038/s41598-020-70503-2
Lee T.-M., Lin J.-Y., Tsai T.-H., Yang R.-Y., Ng I.-S. (2023). Clustered regularly interspaced short palindromic repeats (CRISPR) technology and genetic engineering strategies for microalgae towards carbon neutrality: A critical review. Bioresour. Technol. 368, 128350. doi: 10.1016/j.biortech.2022.128350
Li G.-M. (2008). Mechanisms and functions of DNA mismatch repair. Cell Res. 18, 85–98. doi: 10.1038/cr.2007.115
Li K., Cheng J., Lu H., Yang W., Zhou J., Cen K. (2017). Transcriptome-based analysis on carbon metabolism of Haematococcus pluvialis mutant under 15% CO2. Bioresour. Technol. 233, 313–321. doi: 10.1016/j.biortech.2017.02.121
Li S., Li F., Zhu X., Liao Q., Chang J.-S., Ho S.-H. (2022). Biohydrogen production from microalgae for environmental sustainability. Chemosphere 291, 132717. doi: 10.1016/j.chemosphere.2021.132717
Li D., Zhao Q. (2023). Study of carbon fixation and carbon partitioning of evolved Chlorella sp.’s strain under different carbon dioxide conditions. Biocatal. Agric. Biotechnol. 48, 102655. doi: 10.1016/j.bcab.2023.102655
Liang F., Lindblad P. (2017). Synechocystis PCC 6803 overexpressing RuBisCO grow faster with increased photosynthesis. Metab. Eng. Commun. 4, 29–36. doi: 10.1016/j.meteno.2017.02.002
Lin B., Cui Y., Yan M., Wang Y., Gao Z., Meng C., et al. (2019). Construction of astaxanthin metabolic pathway in the green microalga Dunaliella viridis. Algal Res. 44, 101697. doi: 10.1016/j.algal.2019.101697
Lin J.-Y., Lin W.-R., Ng I.-S. (2022). CRISPRa/i with Adaptive Single Guide Assisted Regulation DNA (ASGARD) mediated control of Chlorella sorokiniana to enhance lipid and protein production. Biotechnol. J. 17, 2100514. doi: 10.1002/biot.202100514
Lin J.-Y., Ng I.-S. (2023). Enhanced carbon capture, lipid and lutein production in Chlamydomonas reinhardtii under meso-thermophilic conditions using chaperone and CRISPRi system. Bioresour. Technol. 384, 129340. doi: 10.1016/j.biortech.2023.129340
Lippi L., Bähr L., Wüstenberg A., Wilde A., Steuer R. (2018). Exploring the potential of high-density cultivation of cyanobacteria for the production of cyanophycin. Algal Res. Biomass Biofuels Bioprod. 31, 363–366. doi: 10.1016/j.algal.2018.02.028
Liu B., Sun Z., Ma X., Yang B., Jiang Y., Wei D., et al. (2015). Mutation breeding of extracellular polysaccharide-producing microalga Crypthecodinium cohnii by a novel mutagenesis with atmospheric and room temperature plasma. Int. J. Mol. Sci. 16, 8201–8212. doi: 10.3390/ijms16048201
Liu X., Xie X., Du H., Sanganyado E., Wang W., Aslam M., et al. (2021). Bioinformatic analysis and genetic engineering approaches for recombinant biopharmaceutical glycoproteins production in microalgae. Algal Res. 55, 102276. doi: 10.1016/j.algal.2021.102276
Liu S., Zhao Y., Liu L., Ao X., Ma L., Wu M., et al. (2015). Improving Cell Growth and Lipid Accumulation in Green Microalgae Chlorella sp. via UV Irradiation. Appl. Biochem. Biotechnol. 175, 3507–3518. doi: 10.1007/s12010-015-1521-6
López G., Yate C., Ramos F. A., Cala M. P., Restrepo S., Baena S. (2019). Production of Polyunsaturated Fatty Acids and Lipids from Autotrophic, Mixotrophic and Heterotrophic cultivation of Galdieria sp. strain USBA-GBX-832. Sci. Rep. 9, 10791. doi: 10.1038/s41598-019-46645-3
Lu Y., Koo J. (2019). O2 sensitivity and H2 production activity of hydrogenases—A review. Biotechnol. Bioeng. 116, 3124–3135. doi: 10.1002/bit.27136
Lucakova S., Branyikova I., Hayes M. (2022). Microalgal proteins and bioactives for food, feed, and other applications. Appl. Sci. 12, 4402. doi: 10.3390/app12094402
Ma S., Zeng W., Huang Y., Zhu X., Xia A., Zhu X., et al. (2022). Revealing the synergistic effects of cells, pigments, and light spectra on light transfer during microalgae growth: A comprehensive light attenuation model. Bioresour. Technol. 348, 126777. doi: 10.1016/j.biortech.2022.126777
Maeda Y., Yoshino T., Matsunaga T., Matsumoto M., Tanaka T. (2018). Marine microalgae for production of biofuels and chemicals. Curr. Opin. Biotechnol. 50, 111–120. doi: 10.1016/j.copbio.2017.11.018
Maia J., Cardoso J. S., Mastrantonio D. J. d. S., Bierhals C. K., Moreira J. B., Costa J. A. V., et al. (2020). Microalgae starch: A promising raw material for the bioethanol production. Int. J. Biol. Macromol. 165, 2739–2749. doi: 10.1016/j.ijbiomac.2020.10.159
Maity S., Mallick N. (2022). Trends and advances in sustainable bioethanol production by marine microalgae: A critical review. J. Clean. Prod. 345, 131153. doi: 10.1016/j.jclepro.2022.131153
Maltsev Y., Maltseva K., Kulikovskiy M., Maltseva S. (2021). Influence of light conditions on microalgae growth and content of lipids, carotenoids, and fatty acid composition. Biology 10, 1060. doi: 10.3390/biology10101060
Manfellotto F., Stella G. R., Falciatore A., Brunet C., Ferrante M. I. (2020). Engineering the unicellular alga Phaeodactylum tricornutum for enhancing carotenoid production. Antioxid. Basel Switz. 9, E757. doi: 10.3390/antiox9080757
Manikandan N. A., Pakshirajan K., Pugazhenthi G. (2020). “Chapter 6 - Value addition of waste lignocellulosic biomass through polyhydroxybutyrate production,” in Waste Biorefinery. Eds. Bhaskar T., Pandey A., Rene E. R., Tsang D. C. W. (Amsterdam: Elsevier), 155–178. doi: 10.1016/B978-0-12-818228-4.00006-X
Manirafasha E., Murwanashyaka T., Ndikubwimana T., Rashid Ahmed N., Liu J., Lu Y., et al. (2018). Enhancement of cell growth and phycocyanin production in Arthrospira (Spirulina) platensis by metabolic stress and nitrate fed-batch. Bioresour. Technol. 255, 293–301. doi: 10.1016/j.biortech.2017.12.068
Manning S. R., Perri K. A., Blackwell K. (2020). “Bioactive polysaccharides from microalgae,” in Polysaccharides of Microbial Origin: Biomedical Applications. Eds. Oliveira J., Radhouani H., Reis R. L. (Cham: Springer International Publishing), 1–25. doi: 10.1007/978-3-030-35734-4_37-1
Marchev A. S., Yordanova Z. P., Georgiev M. I. (2020). Green (cell) factories for advanced production of plant secondary metabolites. Crit. Rev. Biotechnol. 40, 443–458. doi: 10.1080/07388551.2020.1731414
Masojídek J., Ranglová K., Lakatos G. E., Silva Benavides A. M., Torzillo G. (2021). Variables governing photosynthesis and growth in microalgae mass cultures. Processes 9, 820. doi: 10.3390/pr9050820
Masojídek J., Torzillo G., Koblížek M. (2013). “Photosynthesis in microalgae,” in Handbook of Microalgal Culture (Oxford: John Wiley & Sons, Ltd), 21–36. doi: 10.1002/9781118567166.ch2
Mehariya S., Goswami R. K., Verma P., Lavecchia R., Zuorro A. (2021). Integrated approach for wastewater treatment and biofuel production in microalgae biorefineries. Energies 14, 2282. doi: 10.3390/en14082282
Melis A. (2009). Solar energy conversion efficiencies in photosynthesis: Minimizing the chlorophyll antennae to maximize efficiency. Plant Sci. 177, 272–280. doi: 10.1016/j.plantsci.2009.06.005
Mishra S., Liu Y.-J., Liu Y.-J., Liu Y.-J., Chen C.-S., Yao D.-J., et al. (2021). An easily accessible microfluidic chip for high-throughput microalgae screening for biofuel production. Energies 14, 1817. doi: 10.3390/en14071817
Monari C., Righi S., Olsen S. I. (2016). Greenhouse gas emissions and energy balance of biodiesel production from microalgae cultivated in photobioreactors in Denmark: a life-cycle modeling. J. Clean. Prod. 112, 4084–4092. doi: 10.1016/j.jclepro.2015.08.112
Morikawa T., Uraguchi Y., Sanda S., Nakagawa S., Sawayama S. (2018). Overexpression of DnaJ-like chaperone enhances carotenoid synthesis in Chlamydomonas reinhardtii. Appl. Biochem. Biotechnol. 184, 80–91. doi: 10.1007/s12010-017-2521-5
Muñoz C. F., Südfeld C., Naduthodi M. I. S., Weusthuis R. A., Barbosa M. J., Wijffels R. H., et al. (2021). Genetic engineering of microalgae for enhanced lipid production. Biotechnol. Adv. 52, 107836. doi: 10.1016/j.bioteChadv.2021.107836
Musa Ardo F., Wei Lim J., Ramli A., Kee Lam M., Kiatkittipong W., Alaaeldin Abdelfattah E., et al. (2022). A review in redressing challenges to produce sustainable hydrogen from microalgae for aviation industry. Fuel 330, 125646. doi: 10.1016/j.fuel.2022.125646
Narang P. K., Dey J., Mahapatra S. R., Roy R., Kushwaha G. S., Misra N., et al. (2021). Genome-based identification and comparative analysis of enzymes for carotenoid biosynthesis in microalgae. World J. Microbiol. Biotechnol. 38, 8. doi: 10.1007/s11274-021-03188-y
Nymark M., Volpe C., Hafskjold M. C. G., Kirst H., Serif M., Vadstein O., et al. (2019). Loss of ALBINO3b insertase results in truncated light-harvesting antenna in diatoms. Plant Physiol. 181, 1257–1276. doi: 10.1104/pp.19.00868
Pagels F., Bonomi-Barufi J., Vega J., Abdala-Díaz R., Vasconcelos V., Guedes A. C., et al. (2020). Light quality triggers biochemical modulation of Cyanobium sp.—photobiology as tool for biotechnological optimization. J. Appl. Phycol. 32, 2851–2861. doi: 10.1007/s10811-020-02179-0
Pahija E., Hui C.-W. (2021). A practical approach for modelling the growth of microalgae with population balance equation. New Biotechnol. 62, 40–48. doi: 10.1016/j.nbt.2021.01.001
Pandey A., Kant G., Afzal S., Singh M. P., Singh N. K., Kumar S., et al. (2022). “Chapter 5 - Genetic manipulation of microalgae for enhanced biotechnological applications,” in Handbook of Algal Biofuels. Eds. El-Sheekh M., Abomohra A. E.-F. (Amsterdam: Elsevier), 97–122. doi: 10.1016/B978-0-12-823764-9.00019-4
Pandey A., Sinha P., Pandey A. (2021). Hydrogen production by sequential dark and photofermentation using wet biomass hydrolysate of Spirulina platensis: Response surface methodological approach. Int. J. Hydrog. Energy 46, 7137–7146. doi: 10.1016/j.ijhydene.2020.11.205
Parichehreh R., Gheshlaghi R., Mahdavi M. A., Elkamel A. (2019). Optimization of lipid production in Chlorella vulgaris for biodiesel production using flux balance analysis. Biochem. Eng. J. 141, 131–145. doi: 10.1016/j.bej.2018.10.011
Patel V. K., Soni N., Prasad V., Sapre A., Dasgupta S., Bhadra B. (2019). CRISPR–Cas9 system for genome engineering of photosynthetic microalgae. Mol. Biotechnol. 61, 541–561. doi: 10.1007/s12033-019-00185-3
Pereira H., Schulze P. S. C., Schüler L. M., Santos T., Barreira L., Varela J. (2018). Fluorescence activated cell-sorting principles and applications in microalgal biotechnology. Algal Res. 30, 113–120. doi: 10.1016/j.algal.2017.12.013
Perozeni F., Cazzaniga S., Baier T., Zanoni F., Zoccatelli G., Lauersen K. J., et al. (2020). Turning a green alga red: engineering astaxanthin biosynthesis by intragenic pseudogene revival in Chlamydomonas reinhardtii. Plant Biotechnol. J. 18, 2053–2067. doi: 10.1111/pbi.13364
Poliner E., Pulman J. A., Zienkiewicz K., Childs K., Benning C., Farré E. M. (2018). A toolkit for Nannochloropsis oceanica CCMP1779 enables gene stacking and genetic engineering of the eicosapentaenoic acid pathway for enhanced long-chain polyunsaturated fatty acid production. Plant Biotechnol. J. 16, 298–309. doi: 10.1111/pbi.12772
Politaeva N., Smyatskaya Y., Slugin V., Toumi A., Bouabdelli M. (2018). Effect of laser radiation on the cultivation rate of the microalga Chlorella sorokiniana as a source of biofuel. IOP Conf. Ser. Earth Environ. Sci. 115, 12001. doi: 10.1088/1755-1315/115/1/012001
Prasad R., Gupta S. K., Shabnam N., Oliveira C. Y. B., Nema A. K., Ansari F. A., et al. (2021). Role of microalgae in global CO2 sequestration: physiological mechanism, recent development, challenges, and future prospective. Sustainability 13, 13061. doi: 10.3390/su132313061
Purba L. D. A., Othman F. S., Yuzir A., Mohamad S. E., Iwamoto K., Abdullah N., et al. (2022). Enhanced cultivation and lipid production of isolated microalgae strains using municipal wastewater. Environ. Technol. Innov. 27, 102444. doi: 10.1016/j.eti.2022.102444
Ran W., Wang H., Liu Y., Qi M., Xiang Q., Yao C., et al. (2019). Storage of starch and lipids in microalgae: Biosynthesis and manipulation by nutrients. Bioresour. Technol. 291, 121894. doi: 10.1016/j.biortech.2019.121894
Raven J. A., Beardall J., Quigg A. (2020). “Light-driven oxygen consumption in the water-water cycles and photorespiration, and light stimulated mitochondrial respiration,” in Photosynthesis in Algae: Biochemical and Physiological Mechanisms Advances in Photosynthesis and Respiration. Eds. Larkum A. W. D., Grossman A. R., Raven J. A. (Cham: Springer International Publishing), 161–178. doi: 10.1007/978-3-030-33397-3_8
Razu M. H., Hossain F., Khan M. (2019). “Advancement of bio-hydrogen production from microalgae,” in Microalgae Biotechnology for Development of Biofuel and Wastewater Treatment. Eds. Alam A., Wang Z. (Singapore: Springer), 423–462. doi: 10.1007/978-981-13-2264-8_17
Rehmanji M., Suresh S., Nesamma A. A., Jutur P. P. (2021). “Chapter 26 - Microalgal cell factories, a platform for high-value-added biorenewables to improve the economics of the biorefinery,” in Microbial and Natural Macromolecules. Eds. Das S., Dash H. R. (Cambridge: Academic Press), 689–731. doi: 10.1016/B978-0-12-820084-1.00027-2
Reinfelder J. R. (2011). Carbon concentrating mechanisms in eukaryotic marine phytoplankton. Annu. Rev. Mar. Sci. 3, 291–315. doi: 10.1146/annurev-marine-120709-142720
Ren H.-Y., Xiao R.-N., Kong F., Zhao L., Xing D., Ma J., et al. (2019). Enhanced biomass and lipid accumulation of mixotrophic microalgae by using low-strength ultrasonic stimulation. Bioresour. Technol. 272, 606–610. doi: 10.1016/j.biortech.2018.10.058
Ryu K. H., Sung M.-G., Kim B., Heo S., Chang Y. K., Lee J. H. (2018). A mathematical model of intracellular behavior of microalgae for predicting growth and intracellular components syntheses under nutrient-replete and -deplete conditions. Biotechnol. Bioeng. 115, 2441–2455. doi: 10.1002/bit.26744
Saini D. K., Pabbi S., Shukla P. (2018). Cyanobacterial pigments: Perspectives and biotechnological approaches. Food Chem. Toxicol. 120, 616–624. doi: 10.1016/j.fct.2018.08.002
Santin A., Balzano S., Russo M. T., Palma Esposito F., Ferrante M. I., Blasio M., et al. (2022). Microalgae-based PUFAs for food and feed: current applications, future possibilities, and constraints. J. Mar. Sci. Eng. 10, 844. doi: 10.3390/jmse10070844
Sathasivam R., Radhakrishnan R., Hashem A., Abd_Allah E. F. (2019). Microalgae metabolites: A rich source for food and medicine. Saudi J. Biol. Sci. 26, 709–722. doi: 10.1016/j.sjbs.2017.11.003
Schipper K., Al-Jabri H. M. S. J., Wijffels R. H., Barbosa M. J. (2021). Techno-economics of algae production in the Arabian Peninsula. Bioresour. Technol. 331, 125043. doi: 10.1016/j.biortech.2021.125043
Shang C., Pang B., Zhang J., Yu L., Gan S., Li Y., et al. (2022). Identification of interacting proteins of transcription factor DpAP2 related to carotenoid biosynthesis from marine microalga Dunaliella parva. Front. Mar. Sci. 9. doi: 10.3389/fmars.2022.907065
Shi T.-Q., Wang L.-R., Zhang Z.-X., Sun X.-M., Huang H. (2020). Stresses as first-line tools for enhancing lipid and carotenoid production in microalgae. Front. Bioeng. Biotechnol. 8. doi: 10.3389/fbioe.2020.00610
Shin S.-E., Koh H. G., Kang N. K., Suh W. I., Jeong B.-R., Lee B., et al. (2017). Isolation, phenotypic characterization and genome wide analysis of a Chlamydomonas reinhardtii strain naturally modified under laboratory conditions: towards enhanced microalgal biomass and lipid production for biofuels. Biotechnol. Biofuels 10, 308. doi: 10.1186/s13068-017-1000-0
Singh G., Patidar S. K. (2021). Development and applications of attached growth system for microalgae biomass production. Bioenergy Res. 14, 709–722. doi: 10.1007/s12155-020-10195-8
Singh N., Roy K., Goyal A., Moholkar V. S. (2019). Investigations in ultrasonic enhancement of β-carotene production by isolated microalgal strain Tetradesmus obliquus SGM19. Ultrason. Sonochem. 58, 104697. doi: 10.1016/j.ultsonch.2019.104697
Singh S. K., Sundaram S., Kishor K. (2014). “Carbon-concentrating mechanism,” in Photosynthetic Microorganisms: Mechanism For Carbon Concentration SpringerBriefs in Materials. Eds. Singh S. K., Sundaram S., Kishor K. (Cham: Springer International Publishing), 5–38. doi: 10.1007/978-3-319-09123-5_2
Sivaramakrishnan R., Incharoensakdi A. (2021). “Cyanobacteria as renewable sources of bioenergy (Biohydrogen, bioethanol, and bio-oil production),” in Ecophysiology and Biochemistry of Cyanobacteria. Ed. Rastogi R. P. (Singapore: Springer Nature), 431–454. doi: 10.1007/978-981-16-4873-1_19
Slattery S. S., Giguere D. J., Stuckless E. E., Shrestha A., Briere L.-A. K., Galbraith A., et al. (2022). Phosphate-regulated expression of the SARS-CoV-2 receptor-binding domain in the diatom Phaeodactylum tricornutum for pandemic diagnostics. Sci. Rep. 12, 7010. doi: 10.1038/s41598-022-11053-7
Smith R., Jouhet J., Gandini C., Nekrasov V., Marechal E., Napier J. A., et al. (2021). Plastidial acyl carrier protein Δ9-desaturase modulates eicosapentaenoic acid biosynthesis and triacylglycerol accumulation in Phaeodactylum tricornutum. Plant J. 106, 1247–1259. doi: 10.1111/tpj.15231
Solovchenko A., Vasilieva S. G., Zaitsev P., Lukyanov A. A., Skripnikova E. V., Antal T. K. (2022). Approaches to rapid screening of pharmaceutical xenobiotic effects on microalgae via monitoring of photosynthetic apparatus condition. J. Appl. Phycol. 34, 353–361. doi: 10.1007/s10811-021-02660-4
Song I., Kim J., Baek K., Choi Y., Shin B., Jin E. (2020). The generation of metabolic changes for the production of high-purity zeaxanthin mediated by CRISPR-Cas9 in Chlamydomonas reinhardtii. Microb. Cell Factories 19, 220. doi: 10.1186/s12934-020-01480-4
Song I., Kim S., Kim J., Oh H., Jang J., Jeong S. J., et al. (2022). Macular pigment-enriched oil production from genome-edited microalgae. Microb. Cell Factories 21, 27. doi: 10.1186/s12934-021-01736-7
Sousa C. A., Sousa H., Vale F., Simões M. (2021). Microalgae-based bioremediation of wastewaters - Influencing parameters and mathematical growth modelling. Chem. Eng. J. 425, 131412. doi: 10.1016/j.cej.2021.131412
South P. F., Cavanagh A. P., Liu H. W., Ort D. R. (2019). Synthetic glycolate metabolism pathways stimulate crop growth and productivity in the field. Science 363, eaat9077. doi: 10.1126/science.aat9077
Sproles A. E., Berndt A., Fields F. J., Mayfield S. P. (2022). Improved high-throughput screening technique to rapidly isolate Chlamydomonas transformants expressing recombinant proteins. Appl. Microbiol. Biotechnol. 106, 1677–1689. doi: 10.1007/s00253-022-11790-9
Sproles A. E., Fields F. J., Smalley T. N., Le C. H., Badary A., Mayfield S. P. (2021). Recent advancements in the genetic engineering of microalgae. Algal Res. 53, 102158. doi: 10.1016/j.algal.2020.102158
Srivastava N., Hussain A., Kushwaha D., Haque S., Mishra P. K., Gupta V. K., et al. (2021). Nickel ferrite nanoparticles induced improved fungal cellulase production using residual algal biomass and subsequent hydrogen production following dark fermentation. Fuel 304, 121391. doi: 10.1016/j.fuel.2021.121391
Srivastava A., Kalwani M., Chakdar H., Pabbi S., Shukla P. (2022). Biosynthesis and biotechnological interventions for commercial production of microalgal pigments: A review. Bioresour. Technol. 352, 127071. doi: 10.1016/j.biortech.2022.127071
Su Y., Xu M., Brynjólfsson S., Fu W. (2023). Physiological and molecular insights into adaptive evolution of the marine model diatom Phaeodactylum tricornutum under low-pH stress. J. Clean. Prod. 412, 137297. doi: 10.1016/j.jclepro.2023.137297
Südfeld C., Pozo-Rodríguez A., Manjavacas Díez S. A., Wijffels R. H., Barbosa M. J., D’Adamo S. (2022). The nucleolus as a genomic safe harbor for strong gene expression in Nannochloropsis oceanica. Mol. Plant 15, 340–353. doi: 10.1016/j.molp.2021.11.003
Sun Y., Huang F., Dykes G. F., Liu L.-N. (2020). Diurnal regulation of in vivo localization and CO2-fixing activity of carboxysomes in Synechococcus elongatus PCC 7942. Life 10, 169. doi: 10.3390/life10090169
Sun Y., Liao Q., Huang Y., Xia A., Fu Q., Zhu X., et al. (2016). Integrating planar waveguides doped with light scattering nanoparticles into a flat-plate photobioreactor to improve light distribution and microalgae growth. Bioresour. Technol. 220, 215–224. doi: 10.1016/j.biortech.2016.08.063
Sung Y. J., Lee J. S., Sim S. J. (2022). Accelerated sunlight-driven conversion of industrial flue gas into biofuels by microfluidic high-throughput screening towards improving photosynthesis in microalgae under fluctuating light. Chem. Eng. J. 443, 136487. doi: 10.1016/j.cej.2022.136487
Suttangkakul A., Sirikhachornkit A., Juntawong P., Puangtame W., Chomtong T., Srifa S., et al. (2019). Evaluation of strategies for improving the transgene expression in an oleaginous microalga Scenedesmus acutus. BMC Biotechnol. 19, 4. doi: 10.1186/s12896-018-0497-z
Takahashi S., Okubo R., Kanesaki Y., Zhou B., Takaya K., Watanabe S., et al. (2021). Identification of transcription factors and the regulatory genes involved in triacylglycerol accumulation in the unicellular red alga Cyanidioschyzon merolae. Plants 10, 971. doi: 10.3390/plants10050971
Talapatra N., Gautam R., Mittal V., Ghosh U. K. (2021). A comparative study of the growth of microalgae-bacteria symbiotic consortium with the axenic culture of microalgae in dairy wastewater through extraction and quantification of chlorophyll. Mater. Today Proc. 80, 2268–2273. doi: 10.1016/j.matpr.2021.06.227
Tanadul O.-U.-M., Noochanong W., Jirakranwong P., Chanprame S. (2018). EMS-induced mutation followed by quizalofop-screening increased lipid productivity in Chlorella sp. Bioprocess Biosyst. Eng. 41, 613–619. doi: 10.1007/s00449-018-1896-1
Tanvir R. U., Zhang J., Canter T., Chen D., Lu J., Hu Z. (2021). Harnessing solar energy using phototrophic microorganisms: A sustainable pathway to bioenergy, biomaterials, and environmental solutions. Renew. Sustain. Energy Rev. 146, 111181. doi: 10.1016/j.rser.2021.111181
Tibbetts S. M., Milley J. E., Lall S. P. (2015). Chemical composition and nutritional properties of freshwater and marine microalgal biomass cultured in photobioreactors. J. Appl. Phycol. 27, 1109–1119. doi: 10.1007/s10811-014-0428-x
Tibocha-Bonilla J. D., Zuñiga C., Godoy-Silva R. D., Zengler K. (2018). Advances in metabolic modeling of oleaginous microalgae. Biotechnol. Biofuels 11, 241. doi: 10.1186/s13068-018-1244-3
Ting H., Haifeng L., Shanshan M., Zhang Y., Zhidan L., Na D. (2017). Progress in microalgae cultivation photobioreactors and applications in wastewater treatment: A review. Int. J. Agric. Biol. Eng. 10, 1–29. doi: 10.25165/ijabe.v10i1.2705
Ubando A. T., Anderson S. Ng E., Chen W.-H., Culaba A. B., Kwon E. E. (2022). Life cycle assessment of microalgal biorefinery: A state-of-the-art review. Bioresour. Technol. 360, 127615. doi: 10.1016/j.biortech.2022.127615
Valdovinos-García E. M., Petriz-Prieto M. A., Olán-Acosta M. d. l. Á., Barajas-Fernández J., Guzmán-López A., Bravo-Sánchez M. G. (2021). Production of microalgal biomass in photobioreactors as feedstock for bioenergy and other uses: A techno-economic study of harvesting stage. Appl. Sci. 11, 4386. doi: 10.3390/app11104386
Vaz B. da S., Costa J. A. V., Morais M. (2019). Innovative nanofiber technology to improve carbon dioxide biofixation in microalgae cultivation. Bioresour. Technol. 273, 592–598. doi: 10.1016/j.biortech.2018.11.054
Volgusheva A. A., Petrova E. V., Kukarskikh G. P., Dubini A., Antal T. K. (2022). Influence of Fermentation Reactions on Continuous Hydrogen Photoproduction by Microalga Chlamydomonas reinhardtii under Sulfur Deficiency. Mosc. Univ. Biol. Sci. Bull. 77, 25–31. doi: 10.3103/S0096392522010060
Vuppaladadiyam A. K., Yao J. G., Florin N., George A., Wang X., Labeeuw L., et al. (2018). Impact of flue gas compounds on microalgae and mechanisms for carbon assimilation and utilization. ChemSusChem 11, 334–355. doi: 10.1002/cssc.201701611
Walker D. A. (2009). Biofuels, facts, fantasy, and feasibility. J. Appl. Phycol. 21, 509–517. doi: 10.1007/s10811-009-9446-5
Wang Z., Cheng J., Sun Y., You X., Chu F., Yang W. (2022). Mutation adaptation and genotoxicity of microalgae induced by Long-Term high CO2 stress. Chem. Eng. J. 445, 136745. doi: 10.1016/j.cej.2022.136745
Wang K., Cui Y., Wang Y., Gao Z., Liu T., Meng C., et al. (2020). Chloroplast genetic engineering of a unicellular green alga Haematococcus pluvialis with expression of an antimicrobial peptide. Mar. Biotechnol. 22, 572–580. doi: 10.1007/s10126-020-09978-z
Wang K., Gao Z., Wang Y., Meng C., Li J., Qin S., et al. (2021). The chloroplast genetic engineering of a unicellular green alga Chlorella vulgaris with two foreign peptides co-expression. Algal Res. 54, 102214. doi: 10.1016/j.algal.2021.102214
Wang P., Grimm B. (2021). Connecting chlorophyll metabolism with accumulation of the photosynthetic apparatus. Trends Plant Sci. 26, 484–495. doi: 10.1016/j.tplants.2020.12.005
Wang X., Liu Y.-H., Wei W., Zhou X., Yuan W., Balamurugan S., et al. (2017). Enrichment of long-chain polyunsaturated fatty acids by coordinated expression of multiple metabolic nodes in the oleaginous microalga Phaeodactylum tricornutum. J. Agric. Food Chem. 65, 7713–7720. doi: 10.1021/acs.jafc.7b02397
Wang A., Yan K., Chu D., Nazer M., Lin N. T., Samaranayake E., et al. (2020). “Microalgae as a mainstream food ingredient: demand and supply perspective,” in Microalgae Biotechnology for Food, Health and High Value Products. Eds. Alam A., Xu J.-L., Wang Z. (Singapore: Springer), 29–79. doi: 10.1007/978-981-15-0169-2_2
Wang Y., Yang H., Zhang X., Han F., Tu W., Yang W. (2020). Microalgal hydrogen production. Small Methods 4, 1900514. doi: 10.1002/smtd.201900514
Wei L., Jiang Z., Liu B. (2022). A CRISPR/dCas9-based transcription activated system developed in marine microalga Nannochloropsis oceanica. Aquaculture 546, 737064. doi: 10.1016/j.aquaculture.2021.737064
Wei L., Wang Q., Xin Y., Lu Y., Xu J. (2017). Enhancing photosynthetic biomass productivity of industrial oleaginous microalgae by overexpression of RuBisCO activase. Algal Res. 27, 366–375. doi: 10.1016/j.algal.2017.07.023
Wilhelm C., Jakob T. (2012). “3 Balancing the conversion efficiency from photon to biomass,” in Microalgal Biotechnology: Potential and Production (Berlin, Boston: De Gruyter), 39–54. doi: 10.1515/9783110225020.39
Williams P. J. le B., Laurens L. M. L. (2010). Microalgae as biodiesel & biomass feedstocks: Review & analysis of the biochemistry, energetics & economics. Energy Environ. Sci. 3, 554–590. doi: 10.1039/B924978H
Willows R. D. (2020). “Biosynthesis of chlorophyll and bilins in algae,” in Photosynthesis in Algae: Biochemical and Physiological Mechanisms Advances in Photosynthesis and Respiration. Eds. Larkum A. W. D., Grossman A. R., Raven J. A. (Cham: Springer International Publishing), 83–103. doi: 10.1007/978-3-030-33397-3_5
Windhagauer M., Abbriano R. M., Ashworth J., Barolo L., Jaramillo-Madrid A. C., Pernice M., et al. (2021). Characterisation of novel regulatory sequences compatible with modular assembly in the diatom Phaeodactylum tricornutum. Algal Res. 53, 102159. doi: 10.1016/j.algal.2020.102159
Włodarczyk A., Selão T. T., Norling B., Nixon P. J. (2020). Newly discovered Synechococcus sp. PCC 11901 is a robust cyanobacterial strain for high biomass production. Commun. Biol. 3, 1–14. doi: 10.1038/s42003-020-0910-8
Wu W., Tan L., Chang H., Zhang C., Tan X., Liao Q., et al. (2023). Advancements on process regulation for microalgae-based carbon neutrality and biodiesel production. Renew. Sustain. Energy Rev. 171, 112969. doi: 10.1016/j.rser.2022.112969
Xu L., Fan J., Wang Q. (2019). Omics application of bio-hydrogen production through green alga Chlamydomonas reinhardtii. Front. Bioeng. Biotechnol. 7. doi: 10.3389/fbioe.2019.00201
Xue R., Fu L., Dong S., Yang H., Zhou D. (2020). Promoting Chlorella photosynthesis and bioresource production using directionally prepared carbon dots with tunable emission. J. Colloid Interface Sci. 569, 195–203. doi: 10.1016/j.jcis.2020.02.080
Yang L., Su Q., Si B., Zhang Y., Zhang Y., Yang H., et al. (2022). Enhancing bioenergy production with carbon capture of microalgae by ultraviolet spectrum conversion via graphene oxide quantum dots. Chem. Eng. J. 429, 132230. doi: 10.1016/j.cej.2021.132230
Yang D.-W., Syn J.-W., Hsieh C.-H., Huang C.-C., Chien L.-F. (2019). Genetically engineered hydrogenases promote biophotocatalysis-mediated H2 production in the green alga Chlorella sp. DT. Int. J. Hydrog. Energy 44, 2533–2545. doi: 10.1016/j.ijhydene.2018.11.088
Yao S., Lyu S., An Y., Lu J., Gjermansen C., Schramm A. (2019). Microalgae–bacteria symbiosis in microalgal growth and biofuel production: a review. J. Appl. Microbiol. 126, 359–368. doi: 10.1111/jam.14095
Yazdani M., Croen M. G., Fish T. L., Thannhauser T. W., Ahner B. A. (2021). Overexpression of native ORANGE (OR) and OR mutant protein in Chlamydomonas reinhardtii enhances carotenoid and ABA accumulation and increases resistance to abiotic stress. Metab. Eng. 68, 94–105. doi: 10.1016/j.ymben.2021.09.006
Yi Z., Su Y., Brynjolfsson S., Olafsdóttir K., Fu W. (2021). “Chapter 3 - Bioactive polysaccharides and their derivatives from microalgae: biosynthesis, applications, and challenges,” in Studies in Natural Products Chemistry. Ed. Atta-ur-Rahman (Amsterdam: Elsevier), 67–85. doi: 10.1016/B978-0-323-91095-8.00007-6
Yoshino F., Ikeda H., Masukawa H., Sakurai H. (2007). High photobiological hydrogen production activity of a Nostoc sp. PCC 7422 uptake hydrogenase-deficient mutant with high nitrogenase activity. Mar. Biotechnol. 9, 101–112. doi: 10.1007/s10126-006-6035-3
You X., Yang L., Zhou X., Zhang Y. (2022). Sustainability and carbon neutrality trends for microalgae-based wastewater treatment: A review. Environ. Res. 209, 112860. doi: 10.1016/j.envres.2022.112860
Yu H., Li X., Duchoud F., Chuang D. S., Liao J. C. (2018). Augmenting the Calvin–Benson–Bassham cycle by a synthetic malyl-CoA-glycerate carbon fixation pathway. Nat. Commun. 9, 1–10. doi: 10.1038/s41467-018-04417-z
Zeng W., Guo L., Xu S., Chen J., Chen J. B., Zhou J. (2020). High-throughput screening technology in industrial biotechnology. Trends Biotechnol. 38, 888–906. doi: 10.1016/j.tibtech.2020.01.001
Zeng J., Wang Z., Chen G. (2021). Biological characteristics of energy conversion in carbon fixation by microalgae. Renew. Sustain. Energy Rev. 152, 111661. doi: 10.1016/j.rser.2021.111661
Zhang P., Xin Y., He Y., Tang X., Shen C., Wang Q., et al. (2022). Exploring a blue-light-sensing transcription factor to double the peak productivity of oil in Nannochloropsis oceanica. Nat. Commun. 13, 1664. doi: 10.1038/s41467-022-29337-x
Zhang J., Xue D., Wang C., Fang D., Cao L., Gong C. (2023). Genetic engineering for biohydrogen production from microalgae. iScience 26, 107255. doi: 10.1016/j.isci.2023.107255
Keywords: microalgae, photosynthesis, metabolism, biomass, sustainable production
Citation: Hu J, Meng W, Su Y, Qian C and Fu W (2023) Emerging technologies for advancing microalgal photosynthesis and metabolism toward sustainable production. Front. Mar. Sci. 10:1260709. doi: 10.3389/fmars.2023.1260709
Received: 18 July 2023; Accepted: 19 September 2023;
Published: 13 October 2023.
Edited by:
Christophe Brunet, Anton Dohrn Zoological Station Naples, ItalyReviewed by:
Xupeng Cao, Chinese Academy of Sciences (CAS), ChinaCopyright © 2023 Hu, Meng, Su, Qian and Fu. This is an open-access article distributed under the terms of the Creative Commons Attribution License (CC BY). The use, distribution or reproduction in other forums is permitted, provided the original author(s) and the copyright owner(s) are credited and that the original publication in this journal is cited, in accordance with accepted academic practice. No use, distribution or reproduction is permitted which does not comply with these terms.
*Correspondence: Weiqi Fu, d2VpcWlmdUB6anUuZWR1LmNu
†Present address: Cheng Qian, School of Biological Sciences, The University of Hong Kong, Hong Kong, Hong Kong SAR, China
Disclaimer: All claims expressed in this article are solely those of the authors and do not necessarily represent those of their affiliated organizations, or those of the publisher, the editors and the reviewers. Any product that may be evaluated in this article or claim that may be made by its manufacturer is not guaranteed or endorsed by the publisher.
Research integrity at Frontiers
Learn more about the work of our research integrity team to safeguard the quality of each article we publish.