- 1Smithsonian Marine Station, Fort Pierce, FL, United States
- 2Department of Environmental Engineering Sciences, Engineering School of Sustainable Infrastructure and Environment, University of Florida, Gainesville, FL, United States
- 3Red Sea Research Center, Biological and Environmental Science and Engineering, King Abdullah University of Science and Technology (KAUST), Thuwal, Saudi Arabia
Deoxygenation is emerging as a major threat to coral reefs where it can have catastrophic effects, including mass coral mortality. Some coral species cannot survive more than a few days of exposure to low oxygen conditions, while others can tolerate deoxygenation for weeks, suggesting that coral tolerance to lowered dissolved oxygen (DO) concentrations is species-specific. However, hypoxia thresholds for corals have not yet been fully defined, and more information is needed to understand if tolerance to deoxygenation is consistent across all life stages. In this study, we tested the influence of severe (1.5 mg L-1 DO) and intermediate (3.5 mg L-1 DO) deoxygenation on larval settlement and survival during the early recruitment life phase of Colpophyllia natans, Orbicella faveolata, and Pseudodiploria strigosa. Exposure to deoxygenation over a 3-day settlement period did not significantly impact larval survival nor settlement rates compared to ambient DO concentrations (6 mg L-1 DO) for all three species. However, recruit survivorship in C. natans and O. faveolata after further exposure to severe deoxygenation was reduced compared to intermediate deoxygenation and control DO conditions. After 45 days of exposure to severe deoxygenation only 2.5 ± 2.5% of the initial O. faveolata had survived the larval and recruit stages compared to 22.5 ± 4.5% in control oxygen conditions. Similarly, C. natans survival was 13.5 ± 6.0% under severe deoxygenation, compared to 41.0 ± 4.4% in the control treatment. In contrast, survival of P. strigosa larvae and recruits was not different under deoxygenation treatments compared to the control, and higher overall, relative to the other species, indicating that P. strigosa is more resilient to severe deoxygenation conditions during its earliest life stages. This study provides unique insights into species-specific variation in the tolerance of coral recruits to deoxygenation with implications for whether this life history stage may be a demographic bottleneck for three ecologically important Caribbean coral species. Given the increasing frequency and severity of deoxygenation events in Caribbean coastal waters, these results are an important contribution to the growing body of research on deoxygenation as a threat to coral reef persistence in the Anthropocene, with implications for conservation and restoration efforts integrating coral recruitment into reef recovery efforts.
Introduction
Coral reefs are among the most diverse and productive ecosystems on the planet, providing habitat for a vast array of marine organisms and supporting healthy coastlines and communities (Woodhead et al., 2019). However, coral reef ecological function and persistence is threatened due to dramatic changes in marine climatic conditions of the Anthropocene (He and Silliman, 2019). Ocean warming and acidification (OA) have been broadly explored for decades as the two dominant global-scale drivers of coral reef ecosystem decline, yet only recently has deoxygenation been identified as a third parallel, immediate, and severe threat facing coral reef ecosystems (Altieri et al., 2017; Hughes et al., 2020; Johnson et al., 2021a). Ocean deoxygenation describes the warming-induced decreases in seawater dissolved oxygen (DO) observed since the 1950’s, as rising temperatures reduce the capacity for seawater to maintain DO concentrations (Keeling et al., 2010). Deoxygenation occurs over a range of durations and spatial scales (Breitburg et al., 2018), including episodic oxygen depletion that can occur locally as organic loading and eutrophication of coastal waters lead to algal blooms and enhanced microbial activity which can deplete seawater DO (Rabalais et al., 2014; Adelson et al., 2022).
Oxygen plays a pivotal role in the trophic interactions and ecological function of coral reefs (Nelson and Altieri, 2019); therefore, they are particularly vulnerable to deoxygenation. Since tropical coral reefs exist in shallow coastal waters, including enclosed bays or lagoons that often have high seawater residence times, there can be limited capacity for reoxygenation and a heightened sensitivity to drivers of changing DO. Degradation of excessive quantities of seaweed, such as pelagic Sargassum spp., can result in episodic deoxygenation of shallow coastal waters where decaying algae accumulates, and has been associated with mortality in corals and seagrasses (van Tussenbroek et al., 2017; Rodríguez-Martínez et al., 2019). Wastewater runoff is also a driver of algal blooms, eutrophication, and heightened microbial respiration, which can lead to oxygen depletion (Tuholske et al., 2021). Additionally, coral spawn slicks are associated with hypoxic stress in some circumstances, as excess organic matter and decomposition in the water column can cause oxygen depletion (Hobbs and Macrae, 2012). Reports of mass mortality in response to deoxygenation events on coral reefs have been made across many sites (e.g., Hobbs and Macrae, 2012; Altieri et al., 2017; Johnson et al., 2018; Johnson et al., 2021a; Castrillón-Cifuentes et al., 2023) and deoxygenation is now considered a threat to survival of at least 10% of all coral reefs (Altieri et al., 2017). A meta-analysis of global coral reef dissolved oxygen data revealed that 50% of coral reefs currently experience mild (≤ 4 mg L-1 DO) and 13% experience severe (≤ 2 mg L-1) deoxygenation (Pezner et al., 2023). Even so, it is likely that deoxygenation events on coral reefs remain underreported due to a lack of long-term data and inconsistent monitoring programs which do not always capture rapid deoxygenation events. Incidences of deoxygenation on coral reefs are predicted to increase as climatic conditions continue to change at an unprecedented rate (Altieri and Gedan, 2015; Altieri et al., 2021). Up to 94% of coral reefs may be exposed to some degree of deoxygenation by 2100 under ‘business as usual’ climate scenarios (Pezner et al., 2023).
Hypoxic responses in corals can range from reduced physiological function to complete mortality, and it is assumed that hypoxia is activated in coral reef organisms exposed to concentrations of < 2 mg L-1 DO (63 µmol L-1) (Nelson and Altieri, 2019). However, it is likely that thresholds vary between functional groups and species (Vaquer-Sunyer and Duarte, 2008). In corals, inter-specific hypoxic response limits have been noted: for example, resilience to deoxygenation was observed in Orbicella faveolata which survived over 11 days of exposure to critically low levels of DO (<1 mg L-1), yet Acropora cervicornis suffered complete mortality within 5 days of exposure to the same conditions (Johnson et al., 2021b). Since coral reef ecosystems experience a range of DO concentrations over diel cycles due to high rates of oxygen-consuming respiration at night, balanced with oxygen-producing photosynthesis during daylight hours, corals have some adaptive capabilities to fluctuations in DO (Deleja et al., 2022). Oxygen bioavailability is mediated in the diffusive boundary layer (DBL), the thin sheet of seawater directly in contact with the coral surface (Patterson, 1992; Shashar et al., 1996). Within the DBL, oxygen molecules and other solutes are passively transferred between seawater and the coral surface. Oxygen supersaturation can occur in the DBL during periods of intense photosynthetic activity, and oxygen depletion due to respiration at night, providing evidence that corals are regularly exposed to a range of oxygen concentrations at the micro-scale (Altieri et al., 2021). At the macro-scale, some coral species have been found to survive near-anoxic conditions in extreme environments such as shallow lagoons and mangrove roots (Camp et al., 2017; Camp et al., 2019; Altieri et al., 2021), further creating selective pressure that may drive variation in response to deoxygenation.
While adult scleractinian coral colonies are sessile and therefore unable to escape low oxygen conditions, coral sexual reproduction involves a larval, pelagic phase during which time coral planulae are mobile. Swimming larvae actively seek appropriate habitat for settlement and metamorphosis into juvenile coral recruits (Ritson-Williams et al., 2009). This planktonic larval phase usually lasts several days, although larval competency can range from weeks to months (Richmond and Hunter, 1990; Wilson and Harrison, 1998; Heyward and Negri, 2010). Larvae are sensitive to environmental conditions such as temperature (Nozawa and Harrison, 2007) and OA (Albright, 2011), which can affect larval survival, swimming, and settlement behaviors, with the potential to shorten the competency time of larvae and limit dispersal distance (Heyward and Negri, 2010). The mechanisms driving larval settlement selection are not fully understood; however, the influence of environmental change such as ocean warming and acidification can negatively affect recruitment rates (Albright and Langdon, 2011; Doropoulos et al., 2012; Webster et al., 2013; Alessi et al., 2019). While warming and OA occur in parallel with deoxygenation, the role of DO concentration as a physiological cue or threat to survival in coral larvae and recruits requires further research.
Knowledge about the impact of deoxygenation on coral recruitment is scarce, but the few studies undertaken in this area point to important effects of deoxygenation on the early life phases of coral. Alderdice et al. (2022) found significant changes to gene expression in Acropora selago larvae after exposure to 12 hours of low (2 mg L-1) oxygen concentrations, despite no changes to observable swimming behaviors. In a settlement choice experiment by Jorissen and Nugues (2021) A. cytherea and A. pulchra larvae preferentially settled in areas with higher DO concentrations when placed in a settlement chamber with a gradient of low- or high- DO seawater. They also found that larvae reduced downward swimming behavior (indicative of settlement) by over 96% in low DO conditions, which correlated with lower settlement rates, demonstrating that lowered oxygen concentration negatively influenced settlement rates in these species (Jorissen and Nugues, 2021).
Understanding the potential for reduced settlement success in response to depleted DO concentrations is critical for estimating the current and future impact that deoxygenation will have on coral populations. Juvenile recruits of broadcast spawning corals have become rare on Caribbean coral reefs and low rates of successful recruitment are likely to be a bottleneck to ecosystem recovery (Hughes and Tanner, 2000; Randall et al., 2020). The impact of deoxygenation on coral recruitment success could have serious implications for the ability of coral populations to regenerate. At the most practical level, understanding the oxygen needs of coral larvae and the role of oxygen in settlement success will provide valuable insight for reef restoration efforts focused on upscaling and streamlining larval rearing programs and outplanting (e.g., Banaszak et al., 2023). However, for many important coral species no published data are available on their response to deoxygenation during early life stages. Few coral hypoxia studies compare physiological response between species, and most of the existing studies involve only a short duration of exposure to low oxygen. To address these research gaps, we directly compare the response of three coral species exposed to deoxygenation over an extended exposure time. This study aims to (a) quantify the impact of deoxygenation on coral larval settlement and early survival, and (b) to identify tolerance of 3 Caribbean reef-building species during exposure to oxygen depletion representing intermediate (3.5 mg L-1) and severe (1.5 mg L-1) deoxygenation conditions already evident on contemporary corals reefs.
Methods
Mesocosm system
Experiments were conducted at the Smithsonian Marine Station (SMS) mesocosm facility between August-October 2022. The mesocosm system is described in detail in Johnson et al. (2021b). It consists of 12 independent, closed-circulation 40-liter tanks (Aqualogic) which were filled with filtered seawater (0.5 followed by 0.2 µm and CoralLife UV light). In each tank, seawater was circulated through a temperature control unit with an aquarium pump (Aqualogic) to maintain water temperature at 27 ± 0.5°C. Partial seawater changes (50%) were carried out twice weekly and salinity was maintained with the daily addition of approx. 500 ml reverse osmosis (RO) fresh water to each tank to counteract the effect of evaporation. Ambient lighting was maintained for 12 hours per day at PAR 173 ± 12 µmol photons m−2 s−1. Dissolved oxygen (DO, mg L-1) concentration was measured continuously with an Oxyguard DO sensor in each tank, linked to a Neptune Apex control system. Oxyguard DO probe membranes were renewed at the start and calibrated each week throughout the experiment. Experimental DO concentrations were manipulated to create 2 deoxygenation treatments, 3.5 mg L-1 and 1.5 mg L-1, and a control treatment of 6 mg L-1. Concentration of DO was controlled by bubbling nitrogen through the water causing oxygen to be expelled. Nitrogen was directly injected into a small circulation pump, so that it was mixed with water and dispersed throughout the tank. Care was taken to direct bubbles away from study organisms. An automated system of solenoid valves controlled the release of compressed nitrogen into each tank to maintain consistent DO concentrations at the target value. Control tanks were set up with an identical system but bubbled ambient air. In 6 of the tanks (2 per treatment), pH was logged each minute with Neptune pH sensors, which were calibrated 2 – 3 times per week following manufacturer protocol. Daily measurements of pH (NBS scale), DO, and temperature were collected using a YSI Pro 4 (pH was calibrated every 48 hours using YSI calibration buffers at pH 4, pH 7 and pH 10; DO was calibrated using 100% saturated air every 3 – 4 days) and temperature was cross-referenced daily with a handheld traceable thermometer (Fisherbrand) (Table 1).
Study organisms
Larvae of three species of critically endangered, broadcast spawning corals were obtained from the Florida Aquarium Center for Conservation and Biscayne National Park in August 2022. The larvae were produced from gametes collected from spawning coral colonies at field sites (Orbicella faveolata) and from aquarium-reared corals (Colpophyllia natans, Pseudodiploria strigosa) (Table 2). Larvae were produced via ex-situ assisted fertilization conducted following standard protocols (O’Neil et al., 2021; Banaszak et al., 2023) and swimming larvae were transported in plastic containers filled with filtered, oxygenated seawater to the SMS mesocosm facility where they were stored in plastic containers prior to settlement assays. Water changes using filtered (0.2 µm) seawater (27 ± 1°C) were carried out every 48 hours using 80 - 100 µm mesh to filter larvae. Larvae were placed in the experiment when they were competent to settle and metamorphose, between 3 to 9 days post-fertilization (Table 2 for full timeline). The timeline of larval competency (the time during which the larvae are capable of settlement and metamorphosis) is generally capped at 30 days (Wilson and Harrison, 1998), and all the larvae used in this study were within that timeframe.
Survival and settlement of coral larvae
Experimental coral larvae were exposed to one of three DO concentrations (1.5, 3.5, or 6 mg L-1) using settlement chambers (Figure 1) placed in the mesocosm, with 4 replicate tanks per DO treatment (n=4). Fifty larvae from a given species were placed in each settlement chamber, and one settlement chamber for each species of coral larvae was placed in a given mesocosm tank, although different species started incubating at different times. Species-specific differences in spawning date and larval development resulted in a staggered start dates for each species. Since P. strigosa larvae were abundant they were divided into two batches (referred to as P. strigosa 1 and P. strigosa 2 herein), which were placed into the settlement chambers 6 days apart (Table 2).
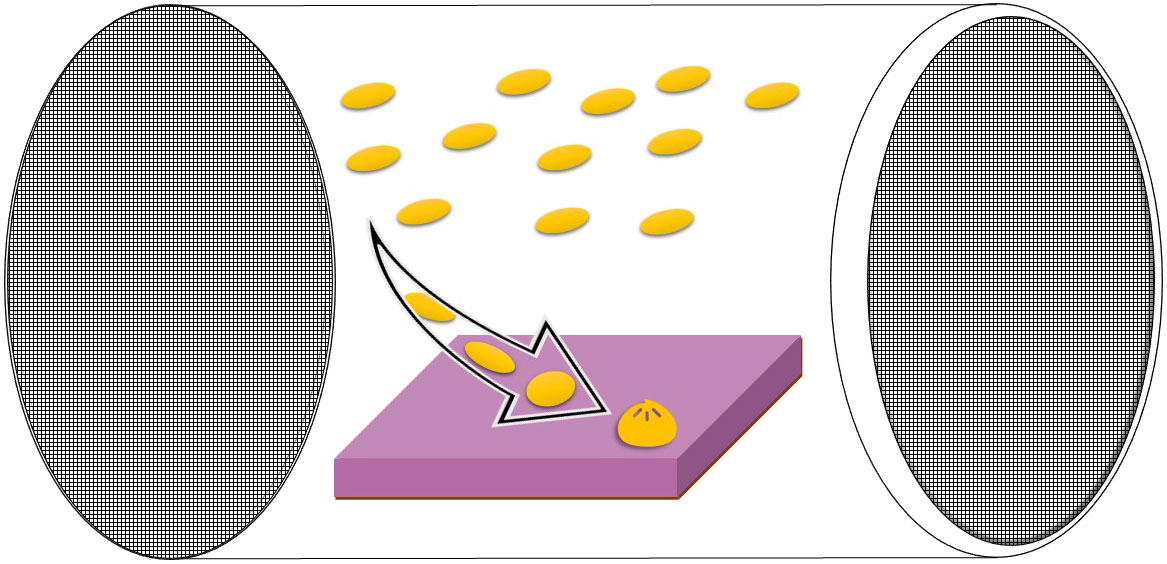
Figure 1 Diagram of the settlement chamber design used in this study (see also Kuffner et al., 2006 and Ritson-Williams et al., 2020) with settlement tile and larvae placed inside. The arrow demonstrates progression of swimming larvae through to settlement and metamorphosis on the substrate tile. Chamber made from PVC plastic tubing capped with mesh at either end (100 or 180 µm). One mesh cap can be removed to insert larvae and settlement tile into the chamber.
Settlement chambers consisted of a PVC tube (Length 10 cm, inner diameter 5.6 cm) fitted with mesh on both ends (100 µm pore size for O. faveolata and P. strigosa 2, or 180 µm pore size for C. natans and P. strigosa 1). Mesh was glued directly to the tube on one end of the chamber and to a removable lid on the other end (Figure 1). For each settlement chamber, swimming larvae were selected and transferred using a pipette into transparent larval settlement chambers (described in Ritson-Williams et al., 2020) each holding a settlement tile (5 x 5 x 1 cm surface area) which had been pre-conditioned for approx. 7 weeks at a Florida Keys reef site (Wonderland Reef) and then maintained in flow through seawater at the SMS facility for approx. 3 months prior to the study. Settlement chambers were placed in mesocosms parallel to the flow of the seawater. Each mesocosm held up to four chambers, one containing each of the 3 species of coral larvae (and two for the P. strigosa larvae) (Table 2).
Three days after the chambers were placed into the mesocosms, they were removed, and the tops, bottoms, and sides of the tiles were inspected for settled larvae under dissecting microscopes. The following categories of settlement were reported: (a) settled on the tile, (b) settled on the chamber or mesh, (c) swimmers, and (d) floating metamorphosed. Mortality was assumed for missing larvae, and sometimes decomposing larvae were observed during these counts indicating mortality was occurring. For purposes of analysis, categories a-d were considered surviving larvae, and categories a-b were considered settled larvae.
Survival of juvenile coral recruits
After the initial 3-day count, settlement tiles were removed from the chambers and returned to mesocosm tanks and maintained under the same experimental (or control) oxygen treatments for 42 more days (total of 45 days). Tiles were placed onto rigid plastic mesh with 1.5 cm openings in the tanks to minimize damage to corals settled on the underside of the tile. Corals were not fed during the experiment. Tiles were removed from the tanks for counts of surviving recruits after a further 42 days of exposure to the different oxygen treatments. Surviving coral recruits were counted on tops, bottoms, and sides of the tiles under dissecting microscopes.
Data analysis
All analyses were conducted in R Programming Language with RStudio version 4.2.2 (R Core Team, 2021) using the packages Tidyverse (Wickham, 2019) and Reshape2 (Wickham, 2007) for data wrangling, and ggplot2 (Wickham, 2016) and ggpubr for data visualization (Kassambara, 2023). The survival of larvae and settlement rate (on day 3), and recruit survival (on day 45) were measured as a proportion of the original number of swimming larvae placed into each chamber (n=50). Rates of larval survival, larval settlement, and recruit survival were all arcsine transformed prior to the analysis to support the use of parametric tests. Assumptions of normality were assessed using histograms, QQ plots and Shapiro-Wilkes tests, and variance was found to be equal between variables based on the results of Bartlett tests. Two-way ANOVAs were used to identify significant influence of species, deoxygenation treatment, and/or the interaction of these two factors on dependent variables. The data were subset and a priori one-way ANOVAs were conducted on individual species to test the effect of dissolved oxygen treatment for each species separately. Post-hoc tests were carried out with the emmeans (Lenth, 2016) and agricolae packages (Mendiburu and Yaseen, 2020) using Tukey HSD to determine pairwise significance when ANOVA p-values were ≤ 0.05.
Results
After 3 days of exposure to one of three DO treatments: control (6 mg L-1 DO), intermediate deoxygenation (3.5 mg L-1 DO), or severe deoxygenation (1.5 mg L-1 DO), overall survival (the total proportion of larvae swimming, settled, and floating metamorphosed) per species ranged between 40.2 to 75.3% of the original swimming larvae placed into the settlement chambers (Figure 2). Hereafter, data are presented as mean percentages with standard error (mean % ± SE) of the original 50 larvae placed into each chamber, unless otherwise indicated. Deoxygenation did not have a significant effect on larval survivorship (ANOVA F1.87, p = 0.17). Species-specific differences in survival rates were significant (Figure 2; Table 3; ANOVA F5.23, p <0.005), with the greatest survival overall for a species average across DO treatments in P. strigosa 2 (75.3 ± 5.7%) and the lowest in C. natans (40.2 ± 6.63%) across treatments.
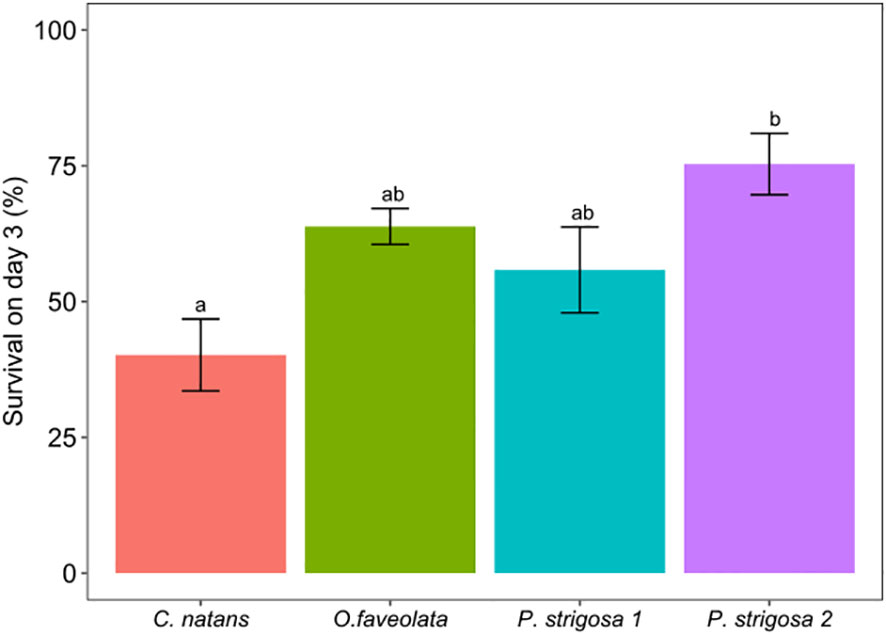
Figure 2 Percentage (%) of coral larvae surviving on day 3 for all species under all treatments. Survival counts include swimming larvae, settled coral recruits (both on tiles and inside of chamber), and larvae which had metamorphosed while floating in the water column. Data presented as mean percentage of the original 50 larvae placed inside each chamber. Error bars show ±1 standard error and letters show Tukey posthoc comparsions. Letters displayed over bar plots show significant differences between treatments.
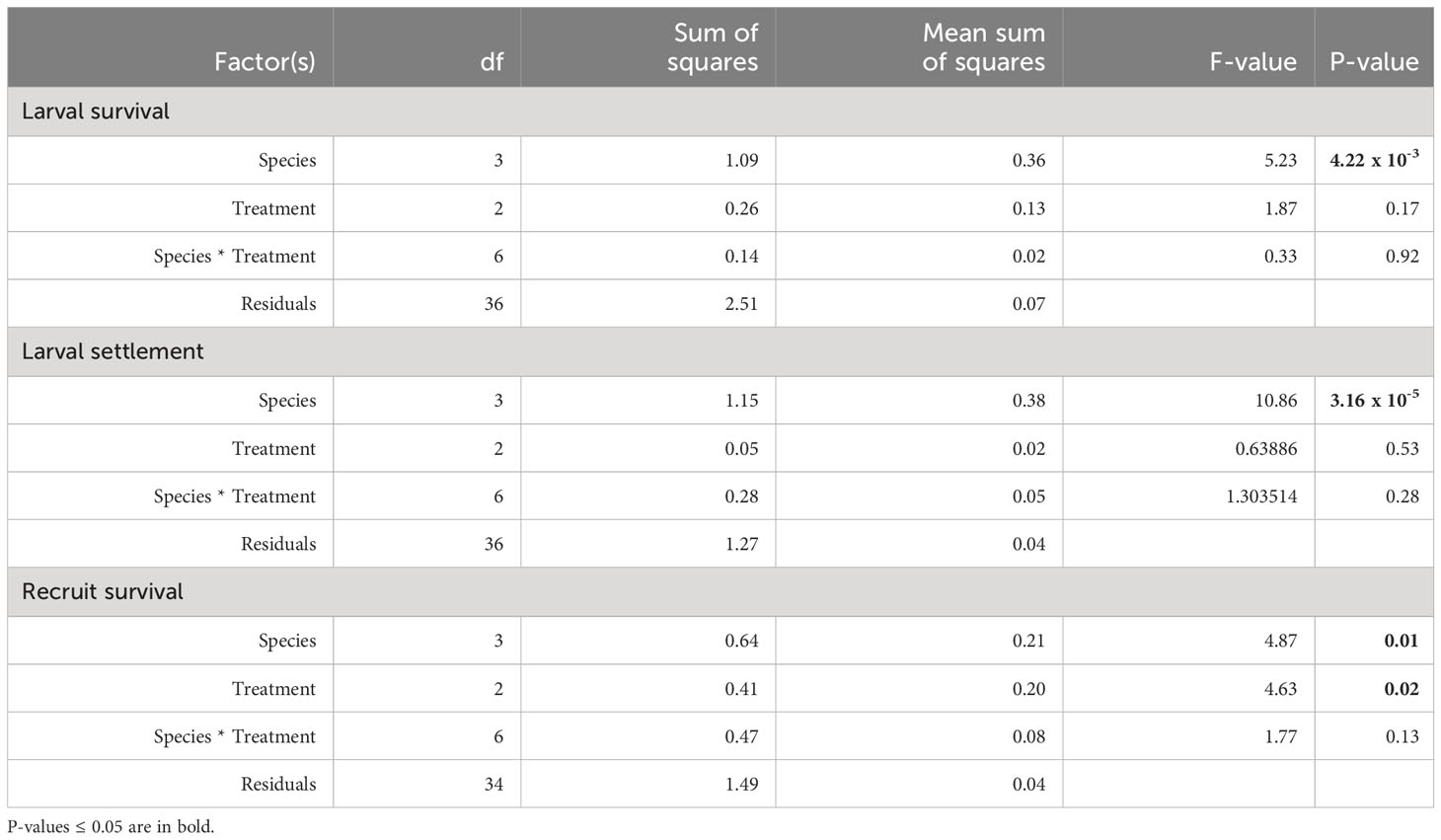
Table 3 Results of 2-way ANOVA models testing treatment and species as factors affecting larval survival and settlement after 3 days of exposure, and surviving recruits after an additional 42 days of exposure to DO treatments.
Settlement occurred in all chambers. Rates of settlement were species-specific (ANOVA F10.86, p <0.0001), with no significant interaction between species and DO treatment (Table 3). DO treatment did not have a statistically significant effect on settlement (Figure 3; Table 3). Settlement rates were highest in the second batch of P. strigosa in all treatments (57.3 ± 4.2%) and lowest in O. faveolata (21.3 ± 4.4%).
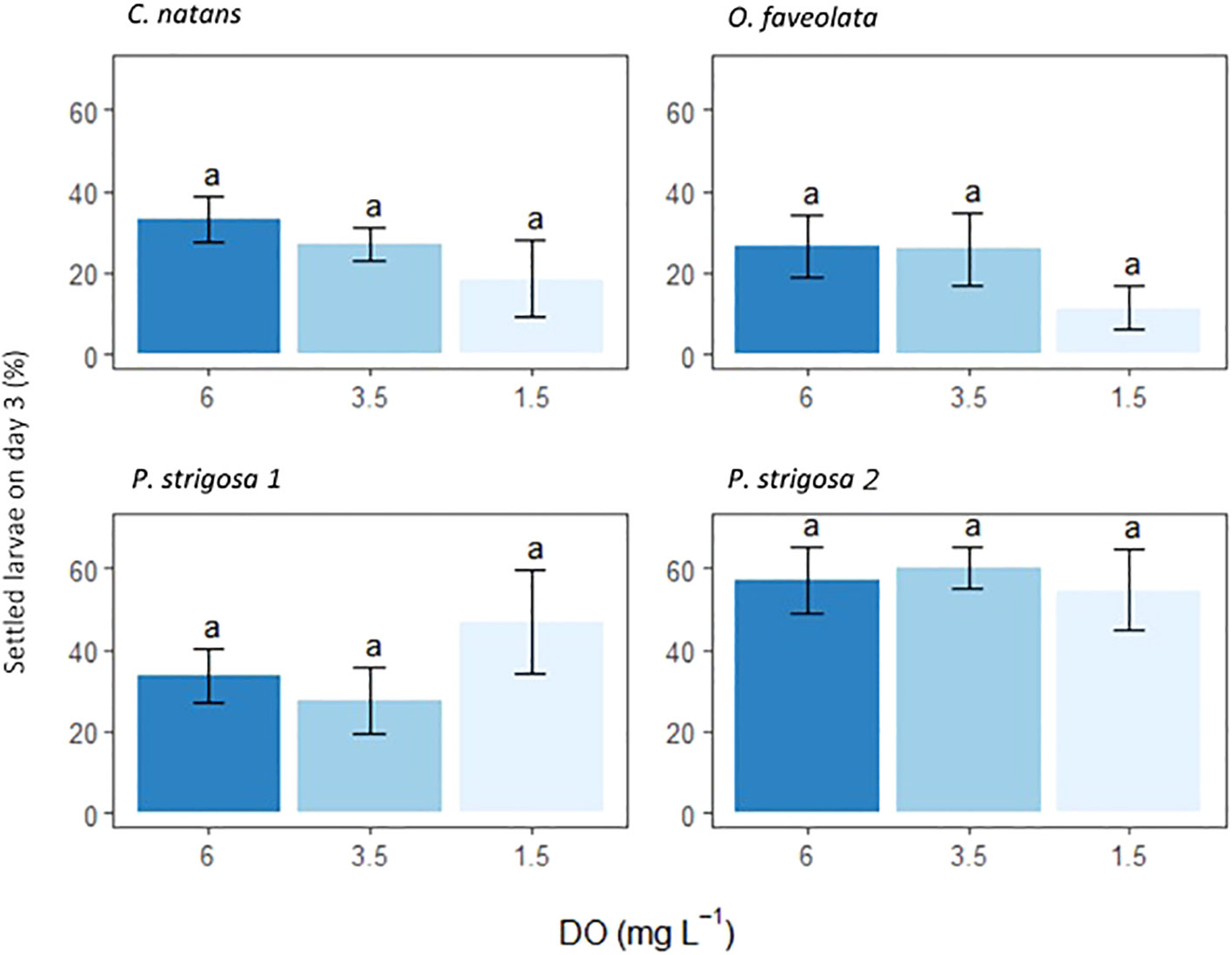
Figure 3 Settlement of coral larvae 3 days after being placed in the settlement chambers. Settled counts are presented as percentage (%) of initial 50 larvae. Settled counts include coral recruits counted both on settlement tiles and chamber interiors. A two-way ANOVA found that only species had p-value <0.05, full output in Table 3. Letters above bars are Tukey post-hoc tests conducted on each species. Error bars show ± 1 standard error.
At the 45-day timepoint, both DO treatment and species had significant effects on the number of surviving recruits, with no significant interaction between these factors (Table 3). Recruit counts were lowest in C. natans and O. faveolata exposed to severe deoxygenation (1.5 mg L-1 DO), with 13.5 ± 6.0% and 2.5 ± 2.5% of the original larvae surviving as recruits (Figure 4). DO concentration did not have a significant effect on P. strigosa recruit survival in either batch. The highest number of recruits counted in any treatment were P. strigosa 2 exposed to intermediate (3.5 mg L-1 DO) deoxygenation, with 45.5 ± 5.3% recruit survival. Differences between species in the control treatment (6 mg L-1 DO) were not significant. In the intermediate deoxygenation treatment (3.5 mg L-1 DO), recruit survival was similar between C. natans, O. faveolata, and P. strigosa (batch 1 and 2). The difference in recruit survival at 45 days between intermediate deoxygenation and control treatments was not significant in any species. In some cases, the number of recruits counted on a tile on day 45 was higher than settlers counted on day 3. This occurred primarily in control and intermediate treatments only, except for 1 severe deoxygenation tile with C. natans, in which 12 settled corals were counted on day 3 and 14 recruits on day 45.
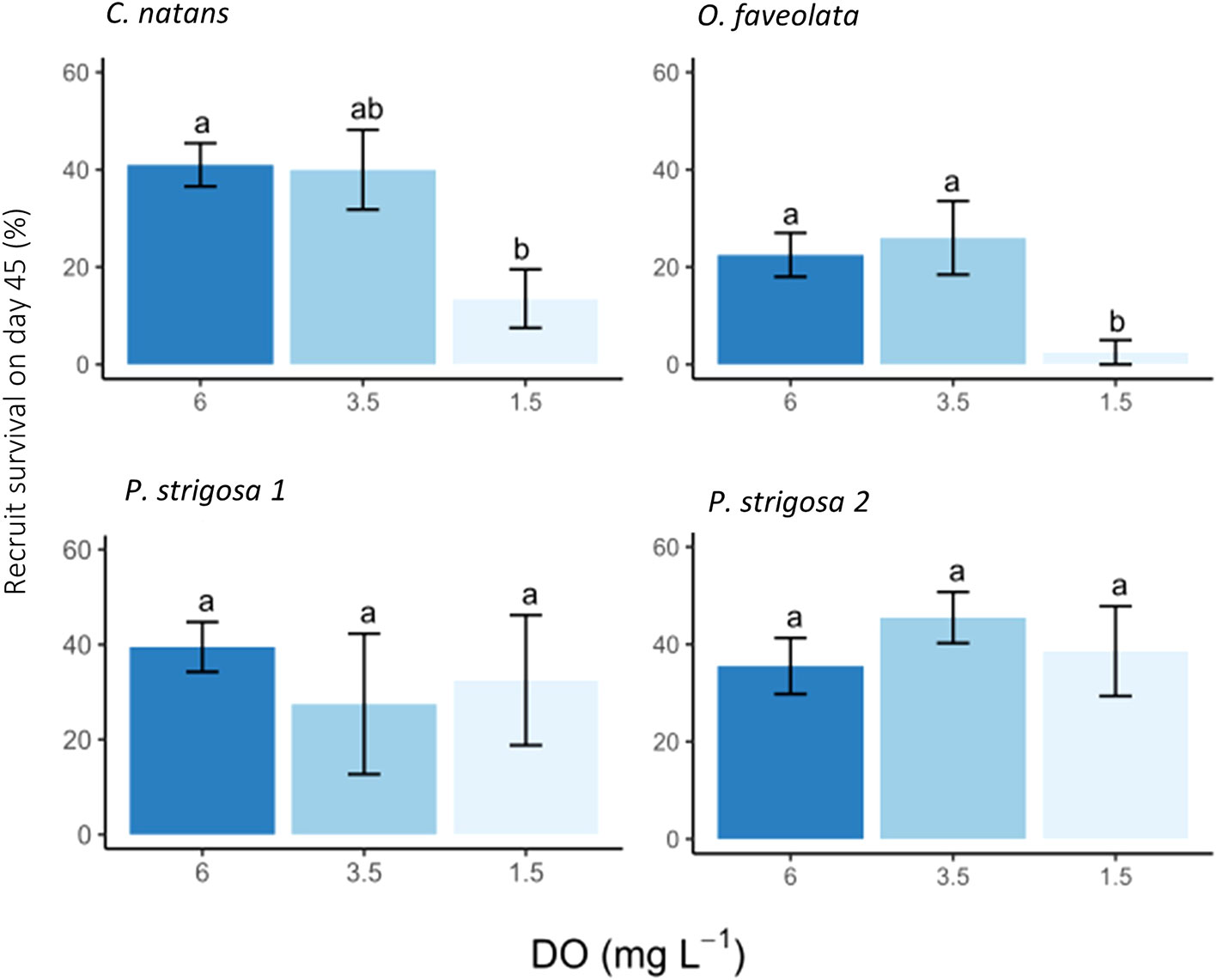
Figure 4 Coral recruit survival after 42 days of post-settlement deoxygenation exposure (45 days total including 3 days of exposure during larval phase) presented as % of initial 50 larvae placed in each chamber. A two-way ANOVA found that both species and treatment had p-value <0.05. C. natans and O. faveolata showed lower recruit survival in the 1.5 mg L-1 DO treatment than P. strigosa, which was not affected by deoxygenation treatment. Error bars show ± 1 standard error and letters above bars show Tukey post-hoc tests conducted on each species. ANOVA results are in Table 3.
Discussion
In this study, recruit survival was lowest in C. natans and O. faveolata exposed to severe deoxygenation. However, deoxygenation did not reduce P. strigosa survival. For all species, larval survival and settlement rates were not impacted by exposure to 3 days of deoxygenation. Despite its importance as an emerging threat for coral reefs, information about the impact of deoxygenation on different coral species and early life stages is limited. Indeed, to the best of our knowledge, this is the first study to directly measure survival and settlement of coral larvae and recruits in C. natans, O. faveolata, and P. strigosa exposed to persistent deoxygenation.
Survival and settlement of coral larvae under deoxygenation
Neither intermediate (3.5 mg L-1) nor severe (1.5 mg L-1) deoxygenation had a significant effect on larval survival (Figure 2). Since coral larvae contain high lipid content and endogenous reserves to support many days or weeks of pre-settlement swimming, our findings imply that larvae are able to maintain sufficient metabolic rates such that oxygen availability at the concentrations we tested are not a strong factor for their survival. There were no prior studies quantifying the impact of deoxygenation on these species at the larval phase for comparison, but our results support previous findings with Acropora spp. that larval survival was not reduced in response to deoxygenation (Alderdice et al., 2022). Thermal stress has been shown to increase larval respiration in O. faveolata (Serrano et al., 2018), and there are no studies on respiration in C. natans larvae for comparison.
Similar to larval survival rates, we found that larval settlement rates were not significantly driven by deoxygenation conditions. This appears to run contrary to previous findings that coral larvae preferred to settle in water with a higher concentration of DO and actively avoided low-DO conditions for settlement (Jorissen and Nugues, 2021). It may be that when there is a choice, coral larvae prefer to settle in ambient DO concentrations but given only one option they will nevertheless settle under low DO conditions within a similar time frame.
Rates of larval survival and settlement were different between species, with the highest settlement and survival consistently observed in P. strigosa across all DO treatments. P. strigosa recruits have survived over longer timeframes than other spawning Caribbean coral species in recruitment experiments (Ritson-Williams et al., 2016), and it is one of the species predicted to increase in abundance as environmental conditions change (Edmunds, 2010). Our study identifies yet another stress (deoxygenation) to which they are more resilient, suggesting they are likely to play an increasingly important role on reefs of the future and should be a target of restoration activities. The second batch of P. strigosa had the highest rates of larval survival (>70% of larvae) and settlement (>50%) across treatments. The chambers used for P. strigosa 2 had a smaller gauge mesh than chambers used for P. strigosa 1 (100 µm compared to 180 µm, respectively), which could have impacted the results, however there was no evidence (no swimming or settled larval found outside of the mesh barrier) that larvae had escaped the larger mesh size used for P. strigosa 1. The differences between the two batches of P. strigosa were likely due to the difference in age of P. strigosa 2 compared to P. strigosa 1; the larvae of the two batches were produced at the same time and maintained under the same conditions, however there were 6 days between placing each batch into the settlement chambers, which may have influenced settlement behavior and sensitivity to environmental cues. Prior work has revealed increased mortality at early and late stages of the larval phase (Graham et al., 2008), and our results suggest likewise that coral larvae are more sensitive to hypoxic stress earlier in their development. This is also further evidence that depletion of energy reserves is not the primary factor limiting tolerance to deoxygenation as older corals with lower remaining reserves would be expected to be more sensitive to deoxygenation if that were the case.
Recruit survival
Survival of C. natans and O. faveolata recruits exposed to 45 days of severe deoxygenation treatment (1.5 mg L-1) was reduced in comparison to control and intermediate deoxygenation, indicating higher sensitivity to deoxygenation in these species than P. strigosa, which exhibited no difference in survivorship between treatments. Since this sensitivity was not observable at the larval stage, it is possible that the acquisition of symbionts impacted tolerance to deoxygenation. The larvae used in this study were asymbiotic, therefore their energetic needs were met entirely by maternal reserves and through respiration until symbionts were acquired after recruitment had taken place. Recruits with symbionts likely acquired their symbionts from conditioned tiles since no adult corals were placed in the mesocosms. Since respiration relies upon the availability of O2, it is possible that C. natans and O. faveolata recruits have higher respiratory needs than P. strigosa, which did not exhibit any reduction in settlement nor recruit survival in response to deoxygenation. In the current study, almost all O. faveolata recruits (97.5 ± 2.5% of original larvae) had died after 45 days of exposure to deoxygenation. This aligns with previous findings that respiration rates in O. faveolata colonies were reduced in response to severe deoxygenation (Gravinese et al., 2022). One potential mechanism driving variable hypoxia response could be the micro-scale dynamics of oxygen exchange, which take place inside the DBL. The speed of molecular oxygen transfer is controlled by coral surface area, DBL thickness, and water flow rate (Shashar et al., 1993; Shashar et al., 1996; Mass et al., 2011). Metabolism increases with flow rate (Patterson et al., 1991; Mass et al., 2010), and it is possible that reduced water velocity inside settlement chambers may have slowed oxygen transfer for respiratory processes of asymbiotic larvae in this study. However, measuring water flow inside the settlement chambers was not possible within the constraints of our study and instrument capacity. Future research should target such knowledge gaps to better understand the micro-scale fluctuations in DBL oxygen dynamics and their role in hypoxia response.
In some cases, apparent increases in the number of recruits between initial settlement and 42-days post-settlement were observed, likely due to difficulty with finding the smallest recruits on tiles immediately after settlement. After 42 days of development, the coral recruits were slightly larger and had acquired symbionts based on observations of the color of the recruits, which made them more visible. Additionally, crevices are one of the preferred settlement micro-habitats for coral larvae, and recruits settled within a crevice on the tile may have been missed on the first count on day 3 but became visible as tentacles were extended by day 45. For this reason, we did not analyze change over time for given replicates, instead we compared differences among species and treatments in larval survival, settlement, and recruit survivorship for a given time point. We assume that the rate of error was the same within each species, although we interpret the data with the caveat that our findings may indicate an underestimation of initial settlement rates, and therefore the real rate of reduction in recruits over time.
Tolerance thresholds are species-specific and life stage dependent
Our study complements prior research on coral tolerance to deoxygenation by providing additional life history stages to species that were previously examined. The reduced survival of 45-day-old O. faveolata recruits exposed to 1.5 mg L-1 DO (2.5 ± 2.5%) in comparison with control and intermediate deoxygenation treatments (22.5 ± 4.5 and 26 ± 7.55%, respectively) contributes to previous results investigating the impact of low-DO on adult O. faveolata corals. In Johnson et al. (2021b), adult colonies of O. faveolata were tolerant to severe deoxygenation over 11 days with no signs of bleaching or mortality, indicating that exposure time may play a critical role in hypoxia response, and that this may also vary at different life history stages within coral species. Since intermediate deoxygenation (3.5 mg L-1 DO) did not significantly impact recruit survival in our study, we infer that the threshold for C. natans and O. faveolata at the recruit stage lies somewhere between intermediate and severe deoxygenation categories (1.5 and 3.5 mg L-1 DO). More research is needed to explore the interaction between coral life history and hypoxia response.
As mass coral bleaching and mortality events have increased over recent decades, the importance of recruitment is critical to coral population recovery and resilience (Hughes et al., 2019). The current study is one of the first to look at this critical demographic bottleneck with both larval survival and post-settlement early recruit survival under low-DO conditions. Our results indicate that studies looking at larval survival and settlement over limited timescales of hours to days may underestimate the effects of longer-term exposure deoxygenation events (for example, 45 days in the current study) on the survival of coral recruits, and so longer-term studies are needed to better understand the potential for deoxygenation to impede ecosystem recovery (Adelson et al., 2022). With the current focus on enhancing and upscaling reef restoration efforts and increasing the yield of assisted fertilization efforts for endangered coral species, there is a critical need to improve understanding of the oxygen requirements for different species of coral larvae and early juvenile recruits. Rearing of larvae and recruits for reef restoration currently lacks information on the optimum DO conditions for settlement and survivorship, and the findings of such studies on deoxygenation will be important to guide restoration protocols. For example, our study would suggest that juvenile coral rearing tanks should be designed to meet species-specific oxygen requirements to reduce the risk of recruit mortality.
Conclusions
While settlement and survivorship of larvae were not significantly affected by intermediate nor severe deoxygenation, recruit survival over 45 days was reduced in two of the species tested. C. natans and O. faveolata are both endangered, yet ecologically important species in the Caribbean region, and this study found that longer-term exposure to deoxygenation reduced juvenile recruit survival. This could have significant implications for the ability of coral larvae to settle and form new colonies and suggests that deoxygenation may be excluding such coral species from some habitats, by acting as demographic bottleneck at the recruit stage, even in the absence of dead adult colonies, which are typically the most recognized evidence for hypoxic stress. Conversely, no difference in recruit survival was observed between deoxygenation treatments in P. strigosa which appears to be a hardy species that is resilient to low oxygen stress. More research incorporating different coral species, life stages, and deoxygenation exposure durations is needed to better understand the mechanisms by which ocean deoxygenation affects corals and to develop strategies to mitigate its impacts.
Data availability statement
Data is available at Figshare DOI: 10.6084/m9.figshare.24161937.
Ethics statement
The manuscript presents research on animals that do not require ethical approval for their study.
Author contributions
JM: Investigation, Methodology, Software, Supervision, Writing – original draft, Writing – review & editing, Conceptualization, Data curation, Formal Analysis, Funding acquisition. AD: Data curation, Investigation, Methodology, Writing – review & editing. JS: Investigation, Methodology, Writing – review & editing. LN: Data curation, Investigation, Methodology, Writing – review & editing. CD: Writing – review & editing, Investigation, Methodology. AA: Funding acquisition, Project administration, Resources, Writing – review & editing. VP: Conceptualization, Funding acquisition, Investigation, Methodology, Project administration, Resources, Supervision, Writing – review & editing. MJ: Conceptualization, Funding acquisition, Investigation, Methodology, Project administration, Resources, Supervision, Writing – review & editing.
Funding
This project was funded by the Link Foundation to JM and Smithsonian Marine Station postdoctoral fellowship awards to JM and AD; funds from the University of Glasgow-Smithsonian mobility award, the US-UK Fulbright Commission, and the Coral Conservation Society to JM; and an award from the National Oceanic and Atmospheric Administration’s National Centers for Coastal Ocean Science Competitive Research Program to AA, MJ, and VP (NA18NOS4780170) through the University of Florida.
Acknowledgments
The authors thank Samantha Scheibler, Sara Swaminathan, Woody Lee, Carolina Melendez Declet, Natalie Danek, and Cassady Dougan for their technical expertise and support setting up and maintaining the larval experiments and mesocosms. Keri O’Neil (Florida Aquarium Center for Conservation) and Amanda Bourque (Biscayne National Park) provided larvae used for this study. Ilsa Kuffner, Andrew Bruckner, Thomas Moore, John Hunt, Kimberly Puglise, and Elizabeth Shaver provided feedback and advice on experimental design. Thank you to Leona Kustra for proofreading the article. Research was conducted under permit FKNMS-2018-129 to AA from the Office of National Marine Sanctuaries, Biscayne National Park permit #BISC-2021-SCI-0015 to VP for O. faveolata larvae, and conditioning of tiles in the field was conducted under permit FKNMS-2019-24 to JS. This is contribution #264 from the Coastal Hypoxia Research Program and #1200 from the Smithsonian Marine Station at Ft. Pierce.
Conflict of interest
The authors declare that the research was conducted in the absence of any commercial or financial relationships that could be construed as a potential conflict of interest.
Publisher's note
All claims expressed in this article are solely those of the authors and do not necessarily represent those of their affiliated organizations, or those of the publisher, the editors and the reviewers. Any product that may be evaluated in this article, or claim that may be made by its manufacturer, is not guaranteed or endorsed by the publisher.
Supplementary material
The Supplementary Material for this article can be found online at: https://www.frontiersin.org/articles/10.3389/fmars.2023.1254965/full#supplementary-material
References
Adelson A. E., Altieri A. H., Boza X., Collin R., Davis K. A., Gaul A., et al. (2022). Seasonal hypoxia and temperature inversions in a tropical bay. Limnol Oceanogr 67, 2174–2189. doi: 10.1002/LNO.12196
Albright R. (2011). Reviewing the effects of ocean acidification on sexual reproduction and early life history stages of reef-Building corals. J. Mar. Biol., 2011, 473615. doi: 10.1155/2011/473615
Albright R., Langdon C. (2011). Ocean acidification impacts multiple early life history processes of the Caribbean coral Porites astreoides. Glob Chang Biol. 17, 2478–2487. doi: 10.1111/j.1365-2486.2011.02404.x
Alderdice R., Pernice M., Cárdenas A., Hughes D. J., Harrison P. L., Boulotte N., et al. (2022). Hypoxia as a physiological cue and pathological stress for coral larvae. Mol. Ecol. 31, 571–587. doi: 10.1111/MEC.16259
Alessi C., Giomi F., Furnari F., Sarà G., Chemello R., Milazzo M. (2019). Ocean acidification and elevated temperature negatively affect recruitment, oxygen consumption and calcification of the reef-building Dendropoma cristatum early life stages: Evidence from a manipulative field study. Sci. Total Environ. 693, 133476. doi: 10.1016/J.SCITOTENV.2019.07.282
Altieri A. H., Harrison S. B., Seemann J., Collin R., Diaz R. J., Knowlton N. (2017). Tropical dead zones and mass mortalities on coral reefs. Proc. Natl. Acad. Sci. 114 (14), 3660–3665. doi: 10.1073/pnas.1621517114
Altieri A. H., Gedan K. B. (2015). Climate change and dead zones. Glob Chang Biol. 21, 1395–1406. doi: 10.1111/GCB.12754
Altieri A. H., Johnson M. D., Swaminathan S. D., Nelson H. R., Gedan K. B. (2021). Resilience of tropical ecosystems to ocean deoxygenation. Trends Ecol. Evol. 36, 227–238. doi: 10.1016/J.TREE.2020.11.003
Banaszak A. T., Marhaver K. L., Miller M. W., Hartmann A. C., Albright R., Hagedorn M., et al. (2023). Applying coral breeding to reef restoration: best practices, knowledge gaps, and priority actions in a rapidly-evolving field. Restor. Ecol. 31 (7), e13913. doi: 10.1111/REC.13913
Breitburg D., Levin L. A., Oschlies A., Grégoire M., Chavez F. P., Conley D. J., et al. (2018). Declining oxygen in the global ocean and coastal waters. Sci. 359 (6371), eaam7240. doi: 10.1126/SCIENCE.AAM7240
Camp E. F., Edmondson J., Doheny A., Rumney J., Grima A. J., Huete A., et al. (2019). Mangrove lagoons of the Great Barrier Reef support coral populations persisting under extreme environmental conditions. Mar. Ecol. Prog. Ser. 625, 1–14. doi: 10.3354/MEPS13073
Camp E. F., Nitschke M. R., Rodolfo-Metalpa R., Houlbreque F., Gardner S. G., Smith D. J., et al. (2017). Reef-building corals thrive within hot-acidified and deoxygenated waters. Sci. Rep. 7, 1–9. doi: 10.1038/s41598-017-02383-y
Castrillón-Cifuentes A. L., Zapata F. A., Giraldo A., Wild C. (2023). Spatiotemporal variability of oxygen concentration in coral reefs of Gorgona Island (Eastern Tropical Pacific) and its effect on the coral Pocillopora capitata. PeerJ 11, e14586. doi: 10.7717/PEERJ.14586/SUPP-3
Deleja M., Paula J. R., Repolho T., Franzitta M., Baptista M., Lopes V., et al. (2022). Effects of hypoxia on coral photobiology and oxidative stress. Biol. (Basel) 11, 1068. doi: 10.3390/BIOLOGY11071068/S1
Doropoulos C., Ward S., Diaz-Pulido G., Hoegh-Guldberg O., Mumby P. J. (2012). Ocean acidification reduces coral recruitment by disrupting intimate larval-algal settlement interactions. Ecol. Lett. 15, 338–346. doi: 10.1111/j.1461-0248.2012.01743.x
Edmunds P. J. (2010). Population biology of Porites astreoides and Diploria strigosa on a shallow Caribbean reef. Mar. Ecol. Prog. Ser. 418, 87–104. doi: 10.3354/MEPS08823
Graham E. M., Baird A. H., Connolly S. R. (2008). Survival dynamics of scleractinian coral larvae and implications for dispersal. Coral Reefs 27, 529–539. doi: 10.1007/s00338-008-0361-z
Gravinese P. M., Douwes A., Eaton K. R., Muller E. M. (2022). Ephemeral hypoxia reduces oxygen consumption in the Caribbean coral Orbicella faveolata. Coral Reefs 41, 13–18. doi: 10.1007/S00338-021-02197-5
He Q., Silliman B. R. (2019). Climate change, human impacts, and coastal ecosystems in the anthropocene. Curr. Biol. 29, R1021–R1035. doi: 10.1016/J.CUB.2019.08.042
Heyward A. J., Negri A. P. (2010). Plasticity of larval pre-competency in response to temperature: Observations on multiple broadcast spawning coral species. Coral Reefs 29, 631–636. doi: 10.1007/S00338-009-0578-5
Hobbs J. P. A., Macrae H. (2012). Unusual weather and trapped coral spawn lead to fish kill at a remote coral atoll. Coral Reefs 31, 961. doi: 10.1007/S00338-012-0918-8
Hughes D. J., Alderdice R., Cooney C., Kühl M., Pernice M., Voolstra C. R., et al. (2020). Coral reef survival under accelerating ocean deoxygenation. Nat. Clim. Change 10 (4), 296–307. doi: 10.1038/s41558-020-0737-9
Hughes T. P., Kerry J. T., Baird A. H., Connolly S. R., Chase T. J., Dietzel A., et al. (2019). Global warming impairs stock–recruitment dynamics of corals. Nature 568, 387–390. doi: 10.1038/s41586-019-1081-y
Hughes T. P., Tanner J. E. (2000). Recruitment failure, life histories, and long-term decline of Caribbean corals. Ecology 81, 2250–2263. doi: 10.1890/0012-9658
Johnson M. D., Rodriguez L. M., Altier A. H. (2018). Shallow-water hypoxia and mass mortality on a Caribbean coral reef. Bull. Mar. Sci. 94 (1), 143–144. doi: 10.5343/bms.2017.1163
Johnson M. D., Scott J. J., Leray M., Lucey N., Bravo L. M. R., Wied W. L., et al. (2021a). Rapid ecosystem-scale consequences of acute deoxygenation on a Caribbean coral reef. Nat. Commun. 12, 1–12. doi: 10.1038/s41467-021-24777-3
Johnson M. D., Swaminathan S. D., Nixon E. N., Paul V. J., Altieri A. H. (2021b). Differential susceptibility of reef-building corals to deoxygenation reveals remarkable hypoxia tolerance. Sci. Rep. 11, 1–12. doi: 10.1038/s41598-021-01078-9
Jorissen H., Nugues M. M. (2021). Coral larvae avoid substratum exploration and settlement in low-oxygen environments. Coral Reefs 40, 31–39. doi: 10.1007/S00338-020-02013-6/FIGURES/2
Kassambara A. (2023) “ggplot2” Based Publication Ready Plots [R package ggpubr version 0.6.0]. Available at: https://CRAN.R-project.org/package=ggpubr (Accessed March 5, 2023).
Keeling R. F., Körtzinger A., Gruber N. (2010). Ocean deoxygenation in a warming world. Ann. Rev. Mar. Sci. 2, 199–229. doi: 10.1146/ANNUREV.MARINE.010908.163855
Kuffner I. B., Walters L. J., Becerro M. A., Paul V. J., Ritson-Williams R., Beach K.S. (2006). Inhibition of coral recruitment by macroalgae and cyanobacteria. Mar. Ecol. Prog. Ser. 323, 107–117. doi: 10.3354/meps323107
Lenth R. V. (2016). Least-squares means: The R package lsmeans. J. Stat. Softw. 69(1), 1–33. doi: 10.18637/jss.v069.i01
Mass T., Brickner I., Hendy E., Genin A. (2011). Enduring physiological and reproductive benefits of enhanced flow for a stony coral. Limnol Oceanogr 56, 2176–2188. doi: 10.4319/LO.2011.56.6.2176
Mass T., Genin A., Shavit U., Grinstein M., Tchernov D. (2010). Flow enhances photosynthesis in marine benthic autotrophs by increasing the efflux of oxygen from the organism to the water. Proc. Natl. Acad. Sci. U.S.A. 107, 2527–2531. doi: 10.1073/PNAS.0912348107
Mendiburu F., Yaseen M. (2020) agricolae: Statistical procedures for agricultural research. R package version 1.4.0. Available at: https://myaseen208.github.io/agricolae/https://cran.r-project.org/package=agricolae.
Nelson H. R., Altieri A. H. (2019). Oxygen: the universal currency on coral reefs. Coral Reefs 38, 177–198. doi: 10.1007/s00338-019-01765-0
Nozawa Y., Harrison P. L. (2007). Effects of elevated temperature on larval settlement and post-settlement survival in scleractinian corals, Acropora solitaryensis and Favites chinensis. Mar. Biol. 152, 1181–1185. doi: 10.1007/S00227-007-0765-2
O’Neil K. L., Serafin R. M., Patterson J. T., Craggs J. R. K. (2021). Repeated ex situ Spawning in Two Highly Disease Susceptible Corals in the Family Meandrinidae. Front. Mar. Sci. 8. doi: 10.3389/FMARS.2021.669976
Patterson M. R. (1992). A mass transfer explanation of metabolic scaling relations in some aquatic invertebrates and algae. Sci. (1979) 255, 1421–1423. doi: 10.1126/SCIENCE.255.5050.1421
Patterson M. R., Sebens K. P., Olson R. R. (1991). In situ measurements of flow effects on primary production and dark respiration in reef corals. Limnol Oceanogr 36, 936–948. doi: 10.4319/lo.1991.36.5.0936
Pezner A. K., Courtney T. A., Barkley H. C., Chou W.-C., Chu H.-C., Clements S. M., et al. (2023). Increasing hypoxia on global coral reefs under ocean warming. Nat. Climate Change 2023, 1–7. doi: 10.1038/s41558-023-01619-2
Rabalais N. N., Cai W. J., Carstens J., Conley D. J., Fry B., Hu X., et al. (2014). Eutrophication-driven deoxygenation in the coastal ocean. Oceanography 27, 172–183. doi: 10.5670/OCEANOG.2014.21
Randall C. J., Negri A. P., Quigley K. M., Foster T., Ricardo G. F., Webster N. S., et al. (2020). Sexual production of corals for reef restoration in the Anthropocene. Mar. Ecol. Prog. Ser. 635, 203–232. doi: 10.3354/MEPS13206
R Core Team (2021). R: A Language and Environment for Statistical Computing. Vienna, Austria: R Foundation for Statistical Computing. Available at: https://www.R-project.org/.
Richmond R. H., Hunter C. L. (1990). Reproduction and recruitment of corals: comparisons among the Caribbean, the Tropical Pacific, and the Red Sea. Mar. Ecol. Prog. Ser. 60, 185–203. doi: 10.3354/meps060185
Ritson-Williams R., Arnold S. N., Fogarty N. D., Steneck R. S., Vermeij M. J. A., Paul V. J. (2009). New perspectives on ecological mechanisms affecting coral recruitment on reefs. Smithson Contrib Mar. Sci. 38, 437–457. doi: 10.5479/si.01960768.38.437
Ritson-Williams R., Arnold S. N., Paul V. J. (2016). Patterns of larval settlement preferences and post-settlement survival for seven Caribbean corals. Mar. Ecol. Prog. Ser. 548, 127–138. doi: 10.3354/MEPS11688
Ritson-Williams R., Arnold S. N., Paul V. J. (2020). The impact of macroalgae and cyanobacteria on larval survival and settlement of the scleractinian corals Acropora palmata, A. cervicornis and Pseudodiploria strigosa. Mar. Biol. 167, 1–12. doi: 10.1007/S00227-019-3639-5
Rodríguez-Martínez R. E., Medina-Valmaseda A. E., Blanchon P., Monroy-Velázquez L. V., Almazán-Becerril A., Delgado-Pech B., et al. (2019). Faunal mortality associated with massive beaching and decomposition of pelagic Sargassum. Mar. pollut. Bull. 146, 201–205. doi: 10.1016/J.MARPOLBUL.2019.06.015
Serrano X. M., Miller M. W., Hendee J. C., Jensen B. A., Gapayao J. Z., Pasparakis C., et al. (2018). Effects of thermal stress and nitrate enrichment on the larval performance of two Caribbean reef corals. Coral Reefs 37, 173–182. doi: 10.1007/S00338-017-1645-Y/TABLES/1
Shashar N., Cohen Y., Loya Y. (1993). Extreme diel fluctuations of oxygen in diffusive boundary layers surrounding stony corals. Biol. Bull. 185 (3) 455–461. doi: 10.2307/1542485
Shashar N., Kinane S., Jokiel P. L., Patterson M. R. (1996). Hydromechanical boundary layers over a coral reef. J. Exp. Mar. Biol. Ecol. 199, 17–28. doi: 10.1016/0022-0981(95)00156-5
Tuholske C., Halpern B. S., Blasco G., Villasenor J. C., Frazier M., Caylor K. (2021). Mapping global inputs and impacts from of human sewage in coastal ecosystems. PloS One 16, e0258898. doi: 10.1371/JOURNAL.PONE.0258898
van Tussenbroek B. I., Hernández Arana H. A., Rodríguez-Martínez R. E., Espinoza-Avalos J., Canizales-Flores H. M., González-Godoy C. E., et al. (2017). Severe impacts of brown tides caused by Sargassum spp. on near-shore Caribbean seagrass communities. Mar. pollut. Bull. 122, 272–281. doi: 10.1016/J.MARPOLBUL.2017.06.057
Vaquer-Sunyer R., Duarte C. M. (2008). Thresholds of hypoxia for marine biodiversity. Proc. Natl. Acad. Sci. U.S.A. 105, 15452–15457. doi: 10.1073/PNAS.0803833105
Webster N. S., Uthicke S., Botté E. S., Flores F., Negri A. P. (2013). Ocean acidification reduces induction of coral settlement by crustose coralline algae. Glob Chang Biol. 19, 303–315. doi: 10.1111/gcb.12008
Wickham H. (2007). Reshaping data with the reshape package. J. Stat. Softw 21, 1–20. doi: 10.18637/JSS.V021.I12
Wickham H. (2016) ggplot2: Elegant Graphics for Data Analysis. Available at: https://ggplot2.tidyverse.org.
Wickham H. (2019). Welcome to the tidyverse. J. Open Source Software 4 (43), 1686. doi: 10.21105/joss.01686
Wilson J. R., Harrison P. L. (1998). Settlement-competency periods of larvae of three species of scleractinian corals. Mar. Biol. 131, 339–345. doi: 10.1007/S002270050327
Keywords: climate change, deoxygenation, coral reefs, larvae, recruitment, settlement, mesocosm
Citation: Mallon JE, Demko AM, Sneed JM, Newman L, Dugan C, Altieri AH, Paul VJ and Johnson MD (2023) The influence of deoxygenation on Caribbean coral larval settlement and early survival. Front. Mar. Sci. 10:1254965. doi: 10.3389/fmars.2023.1254965
Received: 07 July 2023; Accepted: 13 September 2023;
Published: 02 October 2023.
Edited by:
Michael Yu Roleda, University of the Philippines Diliman, PhilippinesReviewed by:
Francisco O. Borges, Center for Marine and Environmental Sciences (MARE), PortugalDan Tchernov, University of Haifa, Israel
Copyright © 2023 Mallon, Demko, Sneed, Newman, Dugan, Altieri, Paul and Johnson. This is an open-access article distributed under the terms of the Creative Commons Attribution License (CC BY). The use, distribution or reproduction in other forums is permitted, provided the original author(s) and the copyright owner(s) are credited and that the original publication in this journal is cited, in accordance with accepted academic practice. No use, distribution or reproduction is permitted which does not comply with these terms.
*Correspondence: Jennifer E. Mallon, SmVubmlmZXIubWFsbG9uQGZ1bGJyaWdodG1haWwub3Jn