- 1Hampton University, Department of Marine and Environmental Science, Hampton, VA, United States
- 2Habitat Conservation, Habitat Protection, National Oceanic and Atmospheric Administration (NOAA) National Marine Fisheries Service (NMFS), Silver Spring, MD, United States
- 3Fisheries Behavioral Ecology Program, Resource Assessment and Conservation Engineering Division, Alaska Fisheries Science Center, National Oceanic and Atmospheric Administration (NOAA), National Marine Fisheries Service (NMFS), Hatfield Marine Science Center, Newport, OR, United States
- 4James J. Howard Laboratory, Northeast Fisheries Science Center, National Oceanic and Atmospheric Administration (NOAA) National Marine Fisheries Service (NMFS), Highlands, NJ, United States
MicroRNAs (miRNAs) are epigenetic markers with a key role in post-transcriptional gene regulation. Several studies have described the dysregulation of miRNAs in temperature and hypoxic stress responses of marine organisms, but their role in the response to acidification conditions has remained relatively underexplored. We investigated the differential expression of miRNAs in whole brain tissue of Arctic cod (Boregogadus saida) exposed to elevated aqueous CO2 levels representative of future climate change predictions. We detected the expression of 17 miRNAs of interest that are either directly or indirectly associated with reduced auditory performance; 12 of the 17 miRNAs showed significant differential expression in high treatment vs. low (control) aqueous CO2 conditions. Target gene predictions indicated that these miRNAs are likely involved with inner ear maintenance, hair cell degradation, age-related hearing loss, neural inflammation, and injury. The highest differential expression was observed in mir-135b, which is linked with increased neural inflammation and injury that may be associated with neurosensory dysfunction. Collectively, these results elucidate the contributions of miRNA mechanisms underlying CO2-induced sensory deficits in fishes facing abiotic environmental change and suggest strong potential for this approach to yield novel insights into the mechanistic effects of climate change on marine organisms.
Introduction
The multifocal effects of climate change on marine organisms have received considerable concern and attention from the scientific community over the past few decades (Brierley and Kingsford, 2009; Heuer and Grosell, 2014; Sumby et al., 2021). Marine organisms are affected both by the increasing temperatures of the ocean and also by CO2-induced ocean acidification. The oceans have been acting as a CO2 sink, absorbing nearly 30% of atmospheric CO2 that has been rising for the past few centuries (Doney et al., 2009). Elevated aqueous CO2 thus results in a set of chemical reactions in the seawater that reduce pH, carbonate ion (CO32-) concentrations, and saturation states of calcium carbonate minerals (CaCO3). Since the Industrial Revolution, ocean pH has declined by approximately 0.1, and is projected to decline an additional 0.1 – 0.4 units by the end of the century (Garcia-Soto et al., 2021).
Fishes exposed to elevated environmental CO2 can experience acute hypercapnia and acidosis followed by an influx in plasma HCO3- to either compensate for or neutralize the acidosis (Heuer and Grosell, 2014). The consequences of fish being in a chronic state of balancing has yet to be elucidated. However, studies collectively show that CO2 exposure affects sensory performance in fishes, e.g. altering taste preferences (Rong et al., 2020), impairing olfaction (Williams et al., 2019; Porteus et al., 2021), slowing retinal function (Chung et al., 2014), and reducing auditory sensitivity (Radford et al., 2021).
Previous studies suggest that neurosensory cues strongly influence fish behavior, interspecific interactions, and even habitat selection (Nagelkerken et al., 2019). This is especially true of olfactory and auditory cues that are used by larval fishes for orientation as well as locating and settling on quality nondegraded habitat (Kaplan and Mooney, 2016; Gordon et al., 2018; Bilodeau and Hay, 2022; Hu et al., 2022). Furthermore, sound can play a critical role in predator-prey interactions, territorial defense, and reproductive behavior (Looby et al., 2022). Auditory impairment could be especially detrimental for soniferous fishes, such as croakers and drums (Sciaenidae) or Arctic cod (Gadidae; Boreogadus saida) that vocalize to locate and aggregate with conspecifics as an intrinsic part of their reproductive behavior (Ramcharitar et al., 2006; Horodysky et al., 2008; Lindseth and Lobel, 2018; Riera et al., 2018). Therefore, the disruption of sensory functions by abiotic environmental and/or anthropogenic change could alter life histories both in single species contexts and across species interactions, resulting in potentially large-scale ecological and ecosystem consequences (Horodysky et al., 2022).
The specific mechanisms through which CO2-induced ocean acidification hinders auditory performance remain inferred but as yet undemonstrated. Currently, a leading mechanistic hypothesis for observed CO2-induced auditory deficits centers on the hypertrophy and/or asymmetric growth of otolithic auditory end organs (Bignami et al., 2013; Holmberg et al., 2019) in fish reared under high levels of CO2 exposure that are consistent with projected future levels of ocean acidification (Radford et al., 2021). However, significant reduction in auditory performance has also been observed in fish exposed to elevated CO2 conditions in the absence of changes in otolith morphology (Horodysky, in preparation). This suggests strongly that morphology alone may not be the sole or proximate mechanism hindering auditory performance, and that underlying neurological mechanisms are likely (sensu Nilsson et al., 2012). One feasible way to elucidate the molecular underpinnings of this process is to investigate environmentally-induced epigenetic disruption of gene expression associated with neurosensory performance (Gemenetzi and Lotery, 2014; Zhao et al., 2019).
MicroRNAs (miRNA) are epigenetic regulators (Yao et al., 2019), which alter gene expression by promoting messenger RNA (mRNA) degradation or translational inhibition. They consist of short non-coding RNA sequences and their expression levels may be influenced by environmental surroundings, especially environmental stressors (Bonin et al., 2019). The interaction of environment – miRNA – gene expression makes miRNAs integral components of an organism’s ability to adapt to environmental changes. While the effects of some abiotic environmental stressors (e.g. temperature and salinity) on miRNA expression in fishes have been documented (Bizuayehu et al., 2015), the effect of CO2 –induced ocean acidification on miRNA expression remains relatively unexplored.
The purpose of this preliminary study was to determine if exposure to elevated aqueous CO2 affects miRNA expression in whole brain tissue of Arctic cod (Boreogadus saida) juveniles. This study focused on miRNAs with potential mRNA targets associated with the auditory system either directly or indirectly.
Methods
Fish husbandry
Wild arctic cod broodstock were collected from the Beaufort Sea and transferred to the NOAA Alaska Fisheries Science Center, Hatfield Marine Science Center, Newport, Oregon, USA. Broodstock were spawned in captivity and fry were raised on a diet of rotifers, enriched artemia, Otohime dry food, and chopped capelin and gel feed to the early juvenile stage in a 3025 L tank of filtered, temperature-controlled (5°C ± 1) seawater.
Treatment exposure
Juvenile Arctic cod were then transferred to 110 L circular experimental tanks at a stocking density of 4 individuals/tank and exposed to either acidified or control (i.e. ambient conditions) for four months to determine the effects of CO2 exposure on auditory electrophysiological performance (Horodysky, in preparation). Honeywell Durafet III probes continuously monitored pH in one conditioning tank for each treatment with automated injection of CO2 into the seawater to maintain treatment pH of 7.26 ± 0.04 (low pH treatment) and 7.85 ± 0.06 (control pH treatment that emulated the ambient pH at which fish were reared). Both treatments were maintained at 5.5°C ± 0.78. All husbandry and experiments were approved by the Hampton University Institutional Animal Care and Use Committee (protocol# 2019-0201-001C2) and followed all relevant laws of the United States. Weekly water samples were drawn and fixed with mercuric chloride, then later analyzed for dissolved inorganic carbon (DIC) and total alkalinity (TA) at the Ocean Acidification Research Center at University of Alaska at Fairbanks (Table 1). For further details regarding the experimental system and water sampling protocols see Andrade et al., 2018 and Hurst et al., 2019.

Table 1 Carbonate system parameters measured weekly during experimental exposures of Arctic cod (Boreogadus saida) to projected levels of ocean acidification.
Sample collection and analysis
Compared to the pH 7.9 ambient controls, fish held at pH 7.2 exhibited a significant reduction in auditory performance (Horodysky, in preparation). Following auditory testing, fish were euthanized with an overdose of benzocaine (>300 mg/L), whole brain tissue was extracted, placed in RNAlater®, then held in -20°C until processed. MiRNAs were extracted and purified from the brain tissue following the mirVana purification kit protocol (Invitrogen), including enrichment for small RNAs. A total of six samples, three from pH 7.2 and three from pH 7.9, were sent to Genewiz (South Plainfield, NJ) for small RNA sequencing. Illumina TrueSeq Small RNA library Prep Kits (Illumina, San Diego, CA) were used to prepare small RNA sequencing libraries according to the manufacturer’s protocol. The sequencing library was validated on the Agilent TapeStation 4200 (Agilent Technologies) and quantified by using a Qubit 2.0 Fluorometer (Invitrogen, Carlsbad, CA). The libraries were multiplexed, clustered on a single lane of a flowcell and loaded on the Illumina HiSeq 4000. The samples were sequenced as 2x150bp Paired End (PE). Raw sequence data (.bcl files) generated from Illumina HiSeq were converted into fastq files and demultiplexed using Illumina’s bcl2fastq 2.17 software. One mismatch was allowed for index sequence identification. The raw sequence reads had adapters removed and were trimmed using CLC Genomics Server 10 (Qiagen). Only reads with lengths 15 to 31 bp were retained. These reads were then compared and annotated using miRbase v. 22 (Griffiths-Jones, 2004). Due to the lack of an annotated genome for Arctic cod, miRNAs were identified in miRbase and literature via blast searches to known miRNAs from model species (e.g. Atlantic cod, Gadus morhua (gmo), Japanese rice fish, Oryzias latipes (ola), zebrafish Danio rerio (dre), and mouse Mus musculus (mmu). Mature sequence hit counts against each miRNA (expression values) were quantile normalized and Baggerly’s exact tests were performed (accounts for the proportion of read counts across treatments; Baggerly et al., 2003) in CLC Genomics Server 10. Significant differential miRNA expression (DE) was considered at FDR- p < 0.05.
Importantly, we conservatively opted to only analyze miRNAs that are highly conserved across several metazoan taxa, which have had an associated phenotype with hearing loss, neurosensory dysfunction, or neurological impairment according to miRBase v.22 and additional literature searches.
The conservation of the identified miRNA sequences against the associated phenotype model organisms was estimated by obtaining the E-value from Mirbase.org, percent query sequence coverage. The percent target coverage is the percent of the query sequence length included in the alignment. Sequence alignments were conducted in Jalview 2.11.2.4 (Waterhouse et al., 2009) and rfam (http://rfam.xfam.org).
Results
We obtained an average of ~ 45 million raw reads per sample (Supplementary Table S1). From these, 35,668 mature miRNA sequences were obtained from Arctic cod brain tissues. The vast majority (34,818 sequences) were unannotated, and 870 sequences were identified from known sources in miRbase (annotated; Table 2).

Table 2 Summary of mature miRNA reads of known and unknown sequences for each sample of whole brain tissue of Arctic cod (B. saida).
This is not surprising due to the lack of an annotated genome for the Arctic cod, which also does not have mature miRNA sequences in miRbase. However, the majority (76%) of the identified mature miRNA of interest showed substantial homogeneity (containing an E-value of ≤ 0.006) when aligned to the mature sequence of the reference organism. Considering all of the data, approximately 70% of annotated mature miRNAs identified overlapped between control and treatment samples (normalized expression values for each mature sequence are available in Supplementary Table S2).
Our dataset was further reduced by removing miRNAs that exhibited a normalized expression value level below 175 in either treatment. Among the total annotated mature miRNA sequences, 17 miRNAs of interest were identified by our phenotypic association analysis conducted in miRBase and additional literature. These miRNAs were either directly or indirectly associated with hearing loss in a model organism (Table 3). However, among the total miRNAs of interest, 12 miRNAs were significantly differentially expressed (DE) between the control and low pH treatments (Figure 1).
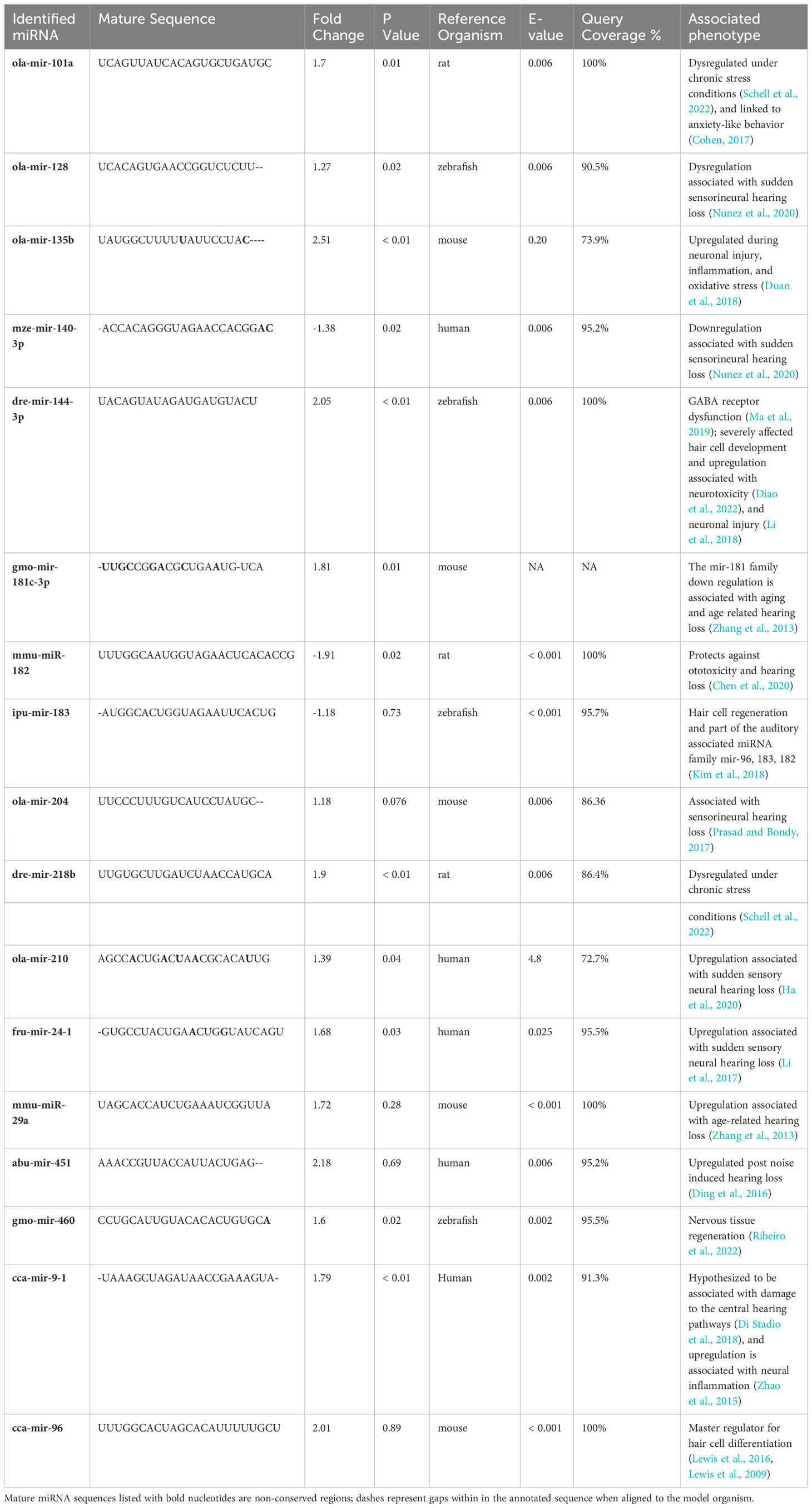
Table 3 Overview of identified mature miRNA sequences of whole brain tissue of Arctic cod (B. saida) that are hypothesized to be associated with auditory deficit.
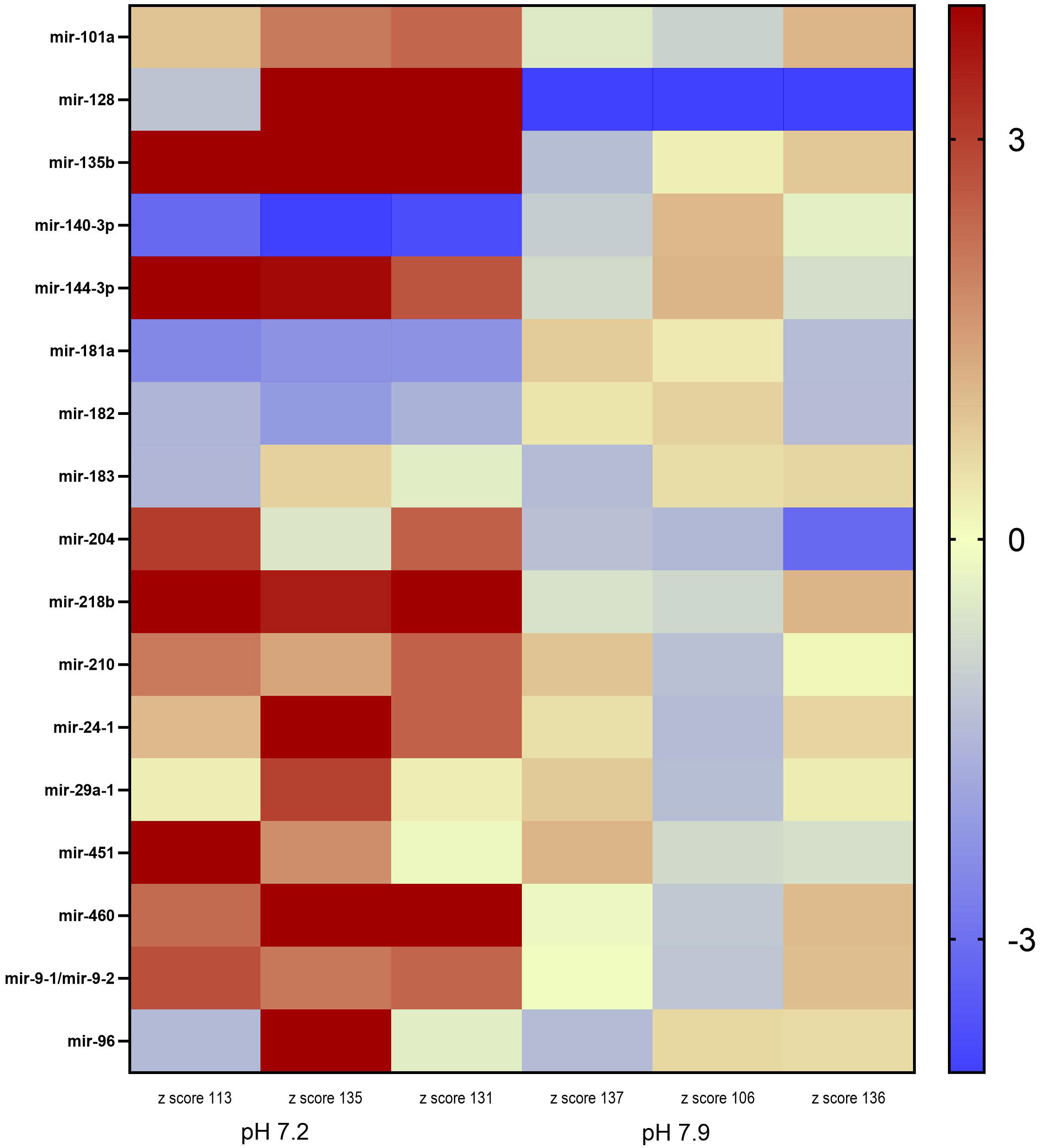
Figure 1 Heatmap of the differential miRNA expression in juvenile Arctic cod (B. saida) brain tissue following a 4 month exposure to pH 7.2 and pH 7.9. Color indicates z-score from low/downregulated (blue) to high/upregulated (red).
Inter-individual variance was observed in the expression levels of the 17 miRNA in the two pH treatments (Figure 1). However, mir-135b, mir-144-3p, mir-140-3p, mir-218b, mir-460, and mir-9-1 were significantly DE across all three individuals within the pH 7.2 treatment. Mir-135b had the highest fold change between treatments (2.51; Table 3).
Discussion
MiRNAs are integral components of organismal stress responses, but little is known of their role in maintaining neurosensory function under elevated CO2 conditions. Using Arctic cod whole brain tissues, our study uncovered the significant differential expression of 12 miRNAs following exposure to acidified conditions. Our analytical focus on highly conserved miRNAs with known neurosensory roles in model organisms revealed several miRNAs of interest for future functional studies and charts a path forward for miRNA investigations of organisms without an annotated genome.
The select group of 17 miRNAs identified in this study have been directly or indirectly associated with hair cell damage and hearing loss in model organisms, and 12 of those were significantly DE. Cod exposed to ocean acidification conditions demonstrated significant upregulation of mir-101a and mir-218b, which can be demonstrative of chronic stress and indirectly associated with sudden sensory neural hearing loss (Masuda and Kanzaki, 2013). Significant DEs of mir-140-3p, mir-210, mir-24-1, mir-181c-3p, and mir-9-1 are directly associated with sudden sensory neural hearing loss in model organisms (Table 3). We caution that mir-181c-3p is not a highly conserved miRNA, so we were unable to acquire the E-value or the percentage of query coverage; this miRNA needs validation in future experiments. Perhaps most interesting was the significant upregulation of mir-144-3p under low pH conditions. The upregulation of this miRNA has been correlated with GABAergic dysfunction (following Nilsson et al., 2012), which is hypothesized to play a role in CO2-induced sensory impairment and is further associated with neurotoxicity and neural injury.
Strikingly, mir-135b showed the highest fold increase (2.51) in fish exposed to low pH. When upregulated, this miRNA helps protect against neuroinflammation and oxidative stress (Schell et al., 2022); the latter stressors have been correlated with hair cell degradation and hearing loss in zebrafish, mice, and humans (Huang et al., 2000; Canlon et al., 2013; Chen et al., 2022). Interestingly, mir-135b is hypothesized to play a role in inner ear development, maintenance, and protection, suggesting its importance in early life history. Yet this miRNA has also been highlighted in studies of elderly human subjects with and without hearing loss, suggesting the importance of its role as a protectant (Sekine et al., 2017). It is therefore possible that environmental exposure to high CO2/low pH induces both neural tissue and hair cell damage in fishes, and that the upregulation of mir-135b evidences a strong compensatory physiological protective response.
There is ample evidence of auditory recovery in fishes following exposure to stressors (Scholik and Yan, 2001; Amoser and Ladich, 2003; Breitzler et al., 2020). Smith et al. (2004) demonstrated partial recovery of hair cells 8 days after acoustic trauma in goldfish (Carrasius auratus), with full recovery of auditory function in 14 days. Collectively, this raises an interesting question of whether environmentally-induced dysregulation of miRNAs associated with auditory performance would impede the potential for hair cell regeneration and auditory recovery in fishes. There remain interesting open questions about the deleterious effects of intensity and duration of CO2 exposure on neurosensory system change and resulting impacts on recovery times from environmental stress. Concerns about auditory performance and recovery in marine organisms are magnified given the rapid increases in anthrophony and other marine noise over the past century as global oceans become louder and auditory cuescapes more complex (Duarte et al., 2021; Horodysky et al., 2022).
While several promising miRNAs associated with audition were detected in our study, our inferences were admittedly limited by a high degree of individual variation across small sample sizes. The individual variation in miRNA expression may be due to considerable intraspecific variation in auditory performance and/or differential resilience to environmental change (Ladich and Fay, 2013; Schunter et al., 2016; Horodysky, in preparation); individual trait diversity must be understood to predict ecological resilience to climate change (Ward et al., 2016). In fact, we suggest such individual variance in miRNA expression could actually be harnessed in future studies to provide insight into differential adaptation to environmental change. Some individuals may be more resilient to certain environmental stressors, with adaptive potential for positive transgenerational outcomes; conversely, transgenerational effects of ocean acidification may have adverse epigenetic consequences for poorly adapted lineages, reducing fitness of offspring (Schunter et al., 2019).
Other caveats of our approach bear consideration, yet we collectively believe that this technique provides an exciting new tool for studies of physiological performance of fisheries resources in the Anthropocene. The use of whole brain in our miRNA extractions may have contributed to within-treatment variance, and a finer dissection of brain structures could reduce potential for cross-contamination of tissue surrounding or encapsulated in the brain and adjacent otoliths (e.g., blood and endolymph). Whole brain samples may dilute region-specific concentrations of specialized miRNAs that are highly DE in very defined locations of the brain. Finer-scale brain-tissue expression profiles can also reveal more specific miRNA roles in the auditory process, while simultaneously identifying other neurosensory processes and potential impairments. To that end, we observed DE of miRNAs associated with olfactory (mi-140 and mir-214), reproductive (mir-29), and visual (retinal degeneration; mir-183, mir-182, mir-96, mir-7, mir-124) impairment. However, DE in the whole brain alone may not be sufficiently indicative to characterize visual or reproductive impairment; we strongly recommend also assaying additional tissues from relevant organ systems (i.e. retinas and/or gonads, in this example) in the future. Although beyond the scope of the present Brief Research Report, transcriptomics would allow researchers to connect the regulome with functional consequences, allowing far more explicit prediction/identification of novel miRNAs and their target genes that may be affected by CO2-induced ocean acidification. Such an approach requires a genome assembly for the study species, which does not exist at present for B. saida; we suggest that this is a worthwhile undertaking to advance mechanistic understanding. Finally, and perhaps most excitingly, this miRNA approach can efficiently survey a study system or exposure protocol to generate mechanistic hypotheses across a myriad of physiological systems. Such an approach may be ideal to guide future experiments and extends well to studies of stressors resulting from climate change, ocean acidification, pollutants, and other human activities.
In this study, we identified 12 significantly DE miRNAs between low and high CO2 exposures, which are either directly or indirectly associated with reduced auditory performance in Arctic cod. We suggest that CO2 exposure induces miRNA dysregulation that can lead to or exacerbate hair cell damage, hinder recovery, and promote neuroinflammation; conjointly these may mechanistically drive or magnify the observed reductions in auditory performance in fish hearing studies. In species with observed otolith hypertrophy or asymmetry following exposure to ocean acidification, CO2-induced miRNA dysregulation may be synergistic in causing observed auditory deficits. But more importantly, this neurological mechanism may be the proximal cause of auditory deficits in species with unaltered otolith morphology following CO2 exposure. We contend that many neurological processes in fishes may be generally affected by elevated CO2, however, the exact mechanistic underpinnings, as well as the extent and duration of this impairment may be species and/or life stage specific, warranting careful investigation. Collectively, we conclude that miRNA sequencing provides a promising approach to guide future physiological and behavioral studies of the effects of abiotic and anthropogenic change on organismal sensory structure and function, particularly when paired with physiological performance assays and miRNA functional studies.
Data availability statement
The data presented in the study are deposited in the NIH SRA repository, BioProject accession PRJNA1007783; sample accession #s: SRX21439117-SRX21439122 and are accessible at: https://www.ncbi.nlm.nih.gov/sra/PRJNA1007783.
Ethics statement
The animal study was approved by Hampton University Institutional Animal Care and Use Committee (IACUC). The study was conducted in accordance with the local legislation and institutional requirements.
Author contributions
CS analyzed the data, created figures, and wrote the manuscript. CB funded microRNA extraction and analysis, assisted with data analysis, and edited the manuscript. CM conducted fish husbandry, collected seawater carbonate chemistry samples, and edited the manuscript. TH funded and oversaw all fish husbandry components and CO2 exposures, and edited the manuscript. AH planned experiments and logistics, selected and dissected fish, funded microRNA analysis, and assisted in manuscript writing and editing. All authors contributed to the article and approved the submitted version.
Funding
AH and CS were supported by NSF-CAREER #1846004 while at Hampton University. CB was supported by NSF HRD# 2000211. Arctic cod exposures, dissections, and molecular procedures were also supported by NSF HRD #1600691 (to AH at Hampton University) and by a grant to TH from NOAA’s Ocean Acidification Program.
Acknowledgments
The authors thank two reviewers for comments that strengthened this manuscript, and J. Andrade, S. Haines, P. Iseri, and M. Ottmar for assistance with laboratory culture and fish sampling. N. Monacci performed seawater chemical analyses. L. Spencer provided valuable comments on an earlier version of this manuscript.
Conflict of interest
The authors declare that the research was conducted in the absence of any commercial or financial relationships that could be construed as a potential conflict of interest.
The author AH declared that they were an editorial board member of Frontiers, at the time of submission. This had no impact on the peer review process and the final decision.
Publisher’s note
All claims expressed in this article are solely those of the authors and do not necessarily represent those of their affiliated organizations, or those of the publisher, the editors and the reviewers. Any product that may be evaluated in this article, or claim that may be made by its manufacturer, is not guaranteed or endorsed by the publisher.
Supplementary material
The Supplementary Material for this article can be found online at: https://www.frontiersin.org/articles/10.3389/fmars.2023.1247344/full#supplementary-material
References
Amoser S., Ladich F. (2003). Diversity in noise-induced temporary hearing loss in otophysine fishes. J. Acoust Soc. Am. 113 (4), pp.2170–2179. doi: 10.1121/1.1557212
Andrade J. F., Hurst T. P., Miller J. A. (2018). Behavioral responses of a coastal flatfish to predation-associated cues and elevated CO2. J. Sea Res. 140, 11–21. doi: 10.1016/j.seares.2018.06.013
Baggerly K., Deng L., Morris J., Aldaz C. (2003). Differential expression in SAGE: accounting for normal between-library variation. Bioinformatics 19 (12), 1477–1483. doi: 10.1093/bioinformatics/btg173
Bignami S., Enochs I. C., Manzello D. P., Sponaugle S., Cowen R. K. (2013). Ocean acidification alters the otoliths of a pantropical fish species with implications for sensory function. Proc. Natl. Acad. Sci. 110 (18), 7366–7370. doi: 10.1073/pnas.1301365110
Bilodeau S. M., Hay M. E. (2022). Chemical cues affecting recruitment and juvenile habitat selection in marine versus freshwater systems. Aquat. Ecol. 56 (2), 339–360. doi: 10.1007/s10452-021-09905-x
Bizuayehu T. T., Johansen S. D., Puvanendran V., Toften H., Babiak I. (2015). Temperature during early development has long-term effects on microRNA expression in Atlantic cod. BMC Genomics 16 (1), 1–12. doi: 10.1186/s12864-015-1503-7
Bonin C. A., van Wijnen A. J., Lewallen E. A. (2019). MicroRNA applications in marine biology. Curr. Mol. Biol. Rep. 5 (4), 167–175. doi: 10.1007/s40610-019-00124-w
Breitzler L., Lau I. H., Fonseca P. J., Vasconcelos R. O. (2020). Noise-induced hearing loss in zebrafish: investigating structural and functional inner ear damage and recovery. Hearing Res. 391, 107952.
Brierley A. S., Kingsford M. J. (2009). Impacts of climate change on marine organisms and ecosystems. Curr. Biol. 19 (14), R602–R614. doi: 10.1016/j.cub.2009.05.046
Canlon B., Theorell T., Hasson D. (2013). Associations between stress and hearing problems in humans. Hearing Res. 295, 9–15. doi: 10.1016/j.heares.2012.08.015
Chen J., Liu Z., Yan H., Xing W., Mi W., Wang R., et al. (2020). miR-182 prevented ototoxic deafness induced by co-administration of kanamycin and furosemide in rats. Neurosci. Lett. 723, 134861.
Chen D., Jia G., Zhang Y., Mao H., Zhao L., Li W., et al. (2022). Sox2 overexpression alleviates noise-induced hearing loss by inhibiting inflammation-related hair cell apoptosis. J. Neuroinflamm. 19 (1), 1–16. doi: 10.1186/s12974-022-02414-0
Chung W. S., Marshall N. J., Watson S. A., Munday P. L., Nilsson G. E. (2014). Ocean acidification slows retinal function in a damselfish through interference with GABAA receptors. J. Exp. Biol. 217 (3), 323–326. doi: 10.1242/jeb.092478
Cohen J. L. (2017). Identification of the microRNA miR-101a and its target ezh2 as contributors to rodent anxiety-like behavior (Doctoral dissertation, The University of Alabama at Birmingham).
Doney S. C., Fabry V. J., Feely R. A., Kleypas J. A. (2009). Ocean acidification: the other CO2 problem. Annu. Rev. Mar. Sci. 1, 169–192. doi: 10.1146/annurev.marine.010908.163834
Diao W., Qian Q., Sheng G., He A., Yan J., Dahlgren, et al. (2022). Triclosan targets miR-144 abnormal expression to induce neurodevelopmental toxicity mediated by activating PKC/MAPK signaling pathway. J. Hazardous Materials 431, 128560.
Ding L., Liu J., Shen H. X., Pan L. P., Liu Q. D., Zhang H. D., et al. (2016). Analysis of plasma microRNA expression profiles in male textile workers with noise-induced hearing loss. Hearing Res. 333, 275–282.
Di Stadio A., Pegoraro V., Giaretta L., Dipietro L., Marozzo R., Angelini C. (2018). Hearing impairment in MELAS: new prospective in clinical use of microRNA, a systematic review. Orphanet J. Rare Dis. 13, 1–9.
Duan Q., Sun W., Yuan H., Mu X. (2018). MicroRNA-135b-5p prevents oxygen-glucose deprivation and reoxygenation-induced neuronal injury through regulation of the GSK-3β/Nrf2/ARE signaling pathway. Arch. Med. Sci. 14 (4), 735–744.
Duarte C. M., Chapuis L., Collin S. P., Costa D. P., Devassy R. P., Eguiluz V. M., et al. (2021). The soundscape of the Anthropocene ocean. Science 371 (6529), eaba4658. doi: 10.1126/science.aba4658
Garcia-Soto C., Cheng L., Caesar L., Schmidtko S., Jewett E. B., Cheripka A., et al. (2021). An overview of ocean climate change indicators: Sea surface temperature, ocean heat content, ocean pH, dissolved oxygen concentration, arctic sea ice extent, thickness and volume, sea level and strength of the AMOC (Atlantic Meridional Overturning Circulation). Front. Mar. Sci 8. doi: 10.3389/fmars.2021.642372
Gemenetzi M., Lotery A. J. (2014). The role of epigenetics in age-related macular degeneration. Eye 28 (12), 1407–1417. doi: 10.1038/eye.2014.225
Gordon T. A., Harding H. R., Wong K. E., Merchant N. D., Meekan M. G., McCormick M. I., et al. (2018). Habitat degradation negatively affects auditory settlement behavior of coral reef fishes. Proc. Natl. Acad. Sci. 115 (20), 5193–5198. doi: 10.1073/pnas.1719291115
Griffiths-Jones S. (2004). The microRNA registry. Nucleic Acids Res. 32 (suppl_1), D109–D111. doi: 10.1093/nar/gkh023
Ha S. M., Hwang K. R., Park I. H., Park S., Choi J. S., Park D. J., et al. (2020). Circulating microRNAs as potentially new diagnostic biomarkers of idiopathic sudden sensorineural hearing loss. Acta Oto-Laryngologica 140 (12), 1013–1020.
Heuer R. M., Grosell M. (2014). Physiological impacts of elevated carbon dioxide and ocean acidification on fish. Am. J. Physiology-Regulat Integr. Comp. Physiol. 307 (9), R1061–R1084. doi: 10.1152/ajpregu.00064.2014
Holmberg R. J., Wilcox-Freeburg E., Rhyne A. L., Tlusty M. F., Stebbins A., Nye S. W. Jr., et al. (2019). Ocean acidification alters morphology of all otolith types in Clark’s anemonefish (Amphiprion clarkii). PeerJ 7, e6152. doi: 10.7717/peerj.6152
Horodysky A. Z., Brill R. W., Fine M. L., Musick J. A., Latour R. J. (2008). Acoustic pressure and acceleration thresholds in six sciaenid fishes. J. Exp. Biol. 211 (9), 1504–1511. doi: 10.1242/jeb.016196
Horodysky A. Z., Schweitzer C. C., Brill R. W. (2022). “Applied sensory physiology and behavior, In: Conservation Physiology for the Anthropocene – A Systems Approach,” in Fish physiology, vol. 39. Eds. Cooke S. J., Fangue N. A., Farrell A. P., Brauner C. J., Eliason E. J., 33–90. doi: 10.1016/bs.fp.2022.04.002
Hu Y., Majoris J. E., Buston P. M., Webb J. F. (2022). Ear development in select coral reef fishes: clues for the role of hearing in larval orientation behavior? Ichthyol Herpetol 110 (4), 759–775. doi: 10.1643/i2022029
Huang T., Cheng A. G., Stupak H., Liu W., Kim A., Staecker H., et al. (2000). Oxidative stress-induced apoptosis of cochlear sensory cells: otoprotective strategies. Int. J. Dev. Neurosci. 18 (2-3), 259–270. doi: 10.1016/S0736-5748(99)00094-5.
Hurst T. P., Copeman L. A., Haines S. A., Meredith S. A., Daniels K., Hubbard K. M. (2019). Elevated CO2 alters behavior, growth, and lipid composition of Pacific cod larvae. Mar. Environ. Res. 145, 52–65. doi: 10.1016/j.marenvres.2019.02.004
Kaplan M. B., Mooney T. A. (2016). Coral reef soundscapes may not be detectable far from the reef. Sci. Rep. 6 (1), 1–10. doi: 10.1038/srep31862
Kim C. W., Han J. H., Wu L., Choi J. Y. (2018). microRNA-183 is essential for hair cell regeneration after neomycin injury in zebrafish. Yonsei Med. J. 59 (1), 141–147.
Ladich F., Fay R. R. (2013). Auditory evoked potential audiometry in fish. Rev. Fish Biol. Fish 23 (3), 317–364. doi: 10.1007/s11160-012-9297-z
Lewis M. A., Buniello A., Hilton J. M., Zhu F., Zhang W. I., Evans S., et al. (2016). Exploring regulatory networks of miR-96 in the developing inner ear. Sci. Rep. 6, 23363.
Lewis M. A., Quint E., Glazier A. M., Fuchs H., De Angelis M. H., Langford C., et al. (2009). An ENU-induced mutation of miR-96 associated with progressive hearing loss in mice. Nat. Genet. 41 (5), 614–618.
Li Q., Peng X., Huang H., Li J., Wang F., Wang J. (2017). RNA sequencing uncovers the key microRNAs potentially contributing to sudden sensorineural hearing loss. Medicine 96 (47).
Li Y., Zhao Y., Cheng M., Qiao Y., Wang Y., Xiong W., et al. (2018). Suppression of microRNA-144-3p attenuates oxygen–glucose deprivation/reoxygenation-induced neuronal injury by promoting Brg1/Nrf2/ARE signaling. J. Biochem. Mol. Toxicol. 32 (4), e22044.
Lindseth A. V., Lobel P. S. (2018). Underwater soundscape monitoring and fish bioacoustics: a review. Fishes 3 (3), 36. doi: 10.3390/fishes3030036
Looby A., Cox K., Bravo S., Rountree R., Juanes F., Reynolds L. K., et al. (2022). A quantitative inventory of global soniferous fish diversity. Rev. Fish Biol. Fish, 1–15. doi: 10.1007/s11160-022-09702-1
Ma K., Zhang H., Wang S., Wang H., Wang Y., Liu J., et al. (2019). The molecular mechanism underlying GABAergic dysfunction in nucleus accumbens of depression-like behaviours in mice. J. Cell. Mol. Med. 23 (10), 7021–7028.
Masuda M., Kanzaki J. (2013). Cause of idiopathic sudden sensorineural hearing loss: The stress response theory. World J. Otorhinolaryngol 3 (3), 42–57. doi: 10.5319/wjo.v3.i3.42
Nagelkerken I., Doney S. C., Munday P. L. (2019). Consequences of anthropogenic changes in the sensory landscape of marine animals. Oceanog Mar. Biol 57:229–64. doi: 10.1201/9780429026379-5
Nilsson G. E., Dixson D. L., Domenici P., McCormick M. I., Sørensen C., Watson S. A., et al. (2012). Near-future carbon dioxide levels alter fish behaviour by interfering with neurotransmitter function. Nat. Clim. Change 2 (3), 201–204. doi: 10.1038/nclimate1352
Nunez D. A., Wijesinghe P., Nabi S., Yeh D., Garnis C. (2020). microRNAs in sudden hearing loss. Laryngoscope 130 (6), E416–E422.
Porteus C. S., Roggatz C. C., Velez Z., Hardege J. D., Hubbard P. C. (2021). Acidification can directly affect olfaction in marine organisms. J. Exp. Biol. 224 (14), jeb237941. doi: 10.1242/jeb.237941
Prasad K. N., Bondy S. C. (2017). MicroRNAs in hearing disorders: their regulation by oxidative stress, inflammation and antioxidants. Front. Cell. Neurosci. 11, 276.
Radford C. A., Collins S. P., Munday P. L., Parsons D. (2021). Ocean acidification effects on fish hearing. Proc. R. Soc. B 288 (1946), 20202754. doi: 10.1098/rspb.2020.2754
Ramcharitar J., Gannon D. P., Popper A. N. (2006). Bioacoustics of fishes of the family Sciaenidae (croakers and drums). Trans. Am. Fish Soc. 135 (5), 1409–1431. doi: 10.1577/T05-207.1
Ribeiro A. O., de Oliveira A. C., Costa J. M., Nachtigall P. G., Herkenhoff M. E., Campos V. F., et al. (2022). MicroRNA roles in regeneration: Multiple lessons from zebrafish. Dev. Dynamics 251 (4), 556–576.
Riera A., Rountree R. A., Pine M. K., Juanes F. (2018). Sounds of Arctic cod (Boreogadus saida) in captivity: A preliminary description. J. Acoust Soc. America 143 (5), EL317–EL321. doi: 10.1121/1.5035162
Rong J., Tang Y., Zha S., Han Y., Shi W., Liu G. (2020). Ocean acidification impedes gustation-mediated feeding behavior by disrupting gustatory signal transduction in the black sea bream, Acanthopagrus schlegelii. Mar. Environ. Res. 162, 105182. doi: 10.1016/j.marenvres.2020.105182
Schell G., Roy B., Prall K., Dwivedi Y. (2022). miR-218: A stress-responsive epigenetic modifier. Non-coding RNA 8 (4), 55. doi: 10.3390/ncrna8040055
Scholik A. R., Yan H. Y. (2001). Effects of underwater noise on auditory sensitivity of a cyprinid fish. Hearing Res. 152 (1-2), 17–24. doi: 10.1016/S0378-5955(00)00213-6
Schunter C., Ravasi T., Munday P. L., Nilsson G. E. (2019). Neural effects of elevated CO2 in fish may be amplified by a vicious cycle. Conserv. Physiol. 7 (1), coz100. doi: 10.1093/conphys/coz100
Schunter C., Welch M. J., Ryu T., Zhang H., Berumen M. L., Nilsson G. E., et al. (2016). Molecular signatures of transgenerational response to ocean acidification in a species of reef fish. Nat. Climate Change 6 (11), 1014–1018. doi: 10.1038/nclimate3087
Sekine K., Matsumura T., Takizawa T., Kimura Y., Saito S., Shiiba K., et al. (2017). Expression profiling of microRNAs in the inner ear of elderly people by real-time PCR quantification. Audiol Neurotol 22 (3), 135–145. doi: 10.1159/000479724
Smith M. E., Kane A. S., Popper A. N. (2004). Noise-induced stress response and hearing loss in goldfish (Carassius auratus). J. Exp. Biol. 207 (3), 427–435. doi: 10.1242/jeb.00755
Sumby J., Haward M., Fulton E. A., Pecl G. T. (2021). Hot fish: The response to climate change by regional fisheries bodies. Mar. Policy 123, 104284. doi: 10.1016/j.marpol.2020.104284
Ward R. D., Friess D. A., Day R. H., Mackenzie R. A. (2016). Impacts of climate change on mangrove ecosystems: a region by region overview. Ecosyst Health sustainability 2 (4), e01211. doi: 10.1002/ehs2.1211
Waterhouse A. M., Procter J. B., Martin D. M. A., Clamp M., Barton G. J. (2009). "Jalview Version 2 - a multiple sequence alignment editor and analysis workbench". Bioinformatics 25 (9), 1189–1191. doi: 10.1093/bioinformatics/btp033
Williams C. R., Dittman A. H., McElhany P., Busch D. S., Maher M. T., Bammler T. K., et al. (2019). Elevated CO2 impairs olfactory-mediated neural and behavioral responses and gene expression in ocean-phase coho salmon (Oncorhynchus kisutch). Global Change Biol. 25 (3), 963–977. doi: 10.1111/gcb.14532
Yao Q., Chen Y., Zhou X. (2019). The roles of microRNAs in epigenetic regulation. Curr. Opin. Chem. Biol. 51, 11–17.
Zhang Q., Liu H., McGee J., Walsh E. J., Soukup G. A., He D. Z. (2013). Identifying microRNAs involved in degeneration of the organ of corti during age-related hearing loss. PloS One 8 (4), e62786.
Zhao Y., Pogue A. I., Lukiw W. J. (2015). MicroRNA (miRNA) signaling in the human CNS in sporadic Alzheimer’s disease (AD)-novel and unique pathological features. Int. J. Mol. Sci. 16 (12), 30105–30116.
Keywords: microRNA, ocean acidification, environmental stressors, climate change, hearing loss, fish, neurosensory
Citation: Schweitzer CC, Bonin CA, Magel C, Hurst TP and Horodysky AZ (2023) Dysregulation of microRNAs may contribute to neurosensory impairment in Arctic cod (Boreogadus saida) following CO2 exposure. Front. Mar. Sci. 10:1247344. doi: 10.3389/fmars.2023.1247344
Received: 25 June 2023; Accepted: 28 August 2023;
Published: 05 October 2023.
Edited by:
Asaduzzaman Md, Chattogram Veterinary and Animal Sciences University, BangladeshReviewed by:
Cristian Gallardo-Escárate, University of Concepcion, ChileValeria Di Dato, Anton Dohrn Zoological Station Naples, Italy
Copyright © 2023 Schweitzer, Bonin, Magel, Hurst and Horodysky. This is an open-access article distributed under the terms of the Creative Commons Attribution License (CC BY). The use, distribution or reproduction in other forums is permitted, provided the original author(s) and the copyright owner(s) are credited and that the original publication in this journal is cited, in accordance with accepted academic practice. No use, distribution or reproduction is permitted which does not comply with these terms.
*Correspondence: Andrij Z. Horodysky, YW5kcmlqLmhvcm9keXNreUBub2FhLmdvdg==