- Scripps Institution of Oceanography, University of California San Diego, La Jolla, CA, United States
Introduction: Growing fishing pressures and ocean warming are potential concerns for populations of brown box crabs, Lopholithodes foraminatus, at the southern end of their geographic distribution. In Southern California, brown box crabs are thought to occupy a broad depth gradient (intertidal zone - 547 m), which exposes them to temperatures ranging from 8°C - 24°C. This large temperature span presents challenges for these ectothermic animals because the rates of their physiological processes, and ultimately their dietary needs, behavior, and health, vary with temperature. Here we examined how temperature affects the feeding behavior of brown box crabs to better understand their ecology in warmer regions of their distribution.
Methods: Adult crabs were exposed to one of three temperature treatments (8°C, 15°C, and 20°C, N=10) for 2 months. Weekly throughout the exposure period, crabs were given two similar-sized prey items of different crushing strength (clam and mussel). Claw pinch force, prey preference, time to consume prey, and number of prey consumed were recorded prior to the start of the experiment and weekly for the duration of the study, with the exception of pinch force, which was recorded bi-weekly. We hypothesized that crabs will have a stronger pinch force at warmer temperatures, prefer prey items that require less breaking force at the coldest temperature, consume prey faster at warmer temperatures, and consume more prey at warmer temperatures.
Results: Our results confirm that pinch force is consistently greater at 15°C compared to 8°C, however, crabs at 8°C consumed more clams (higher strength) while those at 15°C consumed more mussels. Crabs at 8°C also consumed prey items faster than crabs at 15°C but ate a similar number of prey. Furthermore, there was 100% mortality at 20°C within 8 days of exposure, indicating their proximity to lethal limits within Southern California.
Discussion: These results show that brown box crab feeding behavior exhibits temperature thresholds, which may alter their nutritional state, community interactions, and distribution under both short-term and long-term changes in ocean temperature.
1 Introduction
Marine organisms cope with a suite of environmental parameters that change over different time scales, with temperature being one of the most dynamic factors in coastal environments (White et al., 1997; Cutler et al., 2003; Archer et al., 2004). Species that occupy shallow waters contend with temperature shifts during tides, storms, upwelling, seasonal changes, episodic El Niño events, and through long-term ocean warming due to climate change, but the most rapid changes may occur as species move across depths. Both short and long-term changes in temperature can have major influence on species mortality (Wernberg, 2021), development (Sastry, 1966; Peck, 2005), physiology (Freitas et al., 2010; Nguyen et al., 2011; Gunderson et al., 2016), behavior (Nguyen et al., 2011; Pimentel et al., 2016), and distribution (Perry et al., 2005; Schofield et al., 2009). With species that are of critical ecological and commercial importance, including many marine fishes and crustaceans, determining the effects of temperature on different aspects of their physiology and behavior is crucial for understanding how they deal with a dynamic environment under current conditions and future climate change scenarios.
Marine ectotherms, such as crustaceans, are especially sensitive to changes in temperature within their environment, as the rates of their physiological processes, metabolism in particular, are directly affected by environmental temperature (Newell and Branch, 1980; Schulte, 2015; Abram et al., 2017; Shields, 2019). Crustaceans generally exhibit an increased metabolic rate with increasing temperature in both field (Childress et al., 1990; Cowles et al., 1991; Vianna et al., 2020) and laboratory studies (Leffler, 1972; Siikavuopio and James, 2015), but there are exceptions to this trend. For example, shrimp in the family Oplophoridae off the Hawaiian Islands were found to have higher rates of oxygen consumption at lower temperatures, with non-migrating species having minimal effects compared to those that migrate between shallower and deeper depths (Cowles et al., 1991). In the intertidal zone, a fiddler crab species living in unvegetated areas exhibited increased oxygen consumption and metabolic rate with increasing temperature, whereas a congener inhabiting vegetated areas experienced the opposite trend (Vianna et al., 2020). The authors postulate that the species living in vegetated areas are exposed to cooler average temperatures and, thus, have a lower thermal tolerance compared to species living in unvegetated areas where they cannot rely on habitat to regulate temperature exposure. Though the relationship varies, the reactiveness of metabolism to temperature persists across species and habitats.
The relationship between metabolism and temperature has direct impacts on energy acquisition, activity, and feeding, as species modify their behaviors to cope with metabolic demands (Hill, 1980; Stoner et al., 2010; Stoner et al., 2013; Siikavuopio and James, 2015; Yuan et al., 2017). Consequently, feeding behavior is a useful measure of how an organisms’ performance and survivorship may change with environmental temperature. For species that occupy higher trophic levels, temperature-driven changes in predation behavior, including prey selection and feeding efficiency, can strongly influence the activity of individuals and overall community dynamics (Lima and Dill, 1990). Thus, determining the relationship between temperature and predation behavior is necessary for predicting the survivorship of a species in a dynamic environment, as well as the potential consequences to the food chains they comprise (Elner and Hughes, 1978). While there is a significant body of work demonstrating that fish metabolism and feeding behavior is temperature sensitive (Volkoff and Rønnestad, 2020), similar research on invertebrates, particularly crustaceans, is lacking. This is surprising given that crabs play crucial predatory roles in benthic and intertidal communities, where they typically consume a broad diversity of prey (Britayev et al., 2010). Most laboratory studies demonstrate that across a variety of crab species, increasing temperature results in increased metabolic rate and growth, and consequently an increased consumption of prey (Hill, 1980; Stoner et al., 2010; Stoner et al., 2013; Siikavuopio and James, 2015; Yuan et al., 2017). For example, Chinese mitten crabs had higher daily food intake at warmer temperatures when fed prey pellets ad libitum (Yuan et al., 2017). Complementary to greater prey consumption at higher temperatures are changes in foraging behavior. Giant mud crabs, for instance, became more active and spent more time outside of their burrow-like structures at warmer temperatures (Hill, 1980). Beyond spending more time foraging to satisfy a greater appetite at warmer temperatures, other aspects of feeding behavior, such as prey preference and handling captured prey, particularly hard-shelled species, are just as important but far less studied. Durophagous crabs may spend significant time and energy attempting to break the strong shells of various mollusks, and the effort afforded to a particular prey may depend on environmental temperature. An experiment on Japanese stone crabs revealed that they not only found prey more quickly at warmer temperatures, but they also broke through hard-shelled prey faster (Wu et al., 2017). In contrast, prey preference, mostly measured as preferred prey size, appears to be less sensitive to temperature (Elner and Hughes, 1978; Sanchez-Salazar et al., 1987; Sungail et al., 2013; Wu et al., 2017). The effects of temperature on the preferred prey species of crabs is unknown, but has been documented in other animals, such as reef fish (Ferrari et al., 2015). A deeper analysis of crab feeding behavior that includes prey handling and prey preference could yield overlooked responses to temperature that are valuable to our understanding of the ecosystem-level impacts of changing temperatures to benthic communities.
Above certain temperatures, ectotherm performance of feeding behaviors (e.g., foraging rates, activity, amount of prey consumed) generally decreases. As observed in several fish species, appetite increases with temperature, but then ceases before they reach their critical temperature (Volkoff and Rønnestad, 2020). This pattern can be explained by the oxygen and capacity limited thermal tolerance (OCLTT) framework (Pörtner et al., 2017). Under this framework, an animal’s oxygen demand increases as temperature rises, but aerobic metabolism can suffice only to a certain point. Once temperatures exceed an animal’s thermal optimum, they enter the pejus (Tp) range, wherein circulation and ventilation can no longer supply sufficient oxygen to meet the animal’s rising oxygen demand. In the pejus range, animals switch to anaerobic metabolism and must reallocate their limited energy resources to survival, leaving little or none for other activities, including energy acquisition and storage (Pörtner et al., 2006). The OCLTT framework highlights changes to performance that occur at temperatures well below a species’ critical thermal maxima (Pörtner et al., 2017) that may otherwise be overlooked if only examining thermal ecology through more traditional methods of determining critical thermal maxima. Therefore, ecophysiology and behavioral studies that assess the effects of temperature on aspects of performance, such as feeding behavior, can be useful tools for understanding the thermal range of organisms and their responses to climate warming, as well as ecosystem-level implications.
While temperature-induced changes in feeding behavior and general activity are largely driven by metabolic demands, mechanical power output of muscles, and the sensitivity of neuromuscular physiology to temperature, are also contributing mechanisms (Bennett, 1985; Langfeld et al., 1989; Josephson and Stokes, 1994; Altringham and Block, 1997). Prior studies on the skeletal muscle of ectothermic species revealed that rates of force development and muscle contraction and relaxation increase with elevated temperature (Bennett, 1985; Langfeld et al., 1989), so that, generally, mechanical power output also increases with temperature (Josephson, 1993). Like other physiological processes, these increases in neuromuscular activity may only occur until an animal reaches the pejus range. In fish, swimming performance increases with temperature, but decreases once the optimal temperature is reached (Volkoff and Rønnestad, 2020). Maintaining muscle performance is critical for many decapod crustacean species that use their claws to capture, manipulate, and break hard-shell prey items (Vermeij, 1977). As species encounter temperature gradients, their ability to generate sufficient force to break hard-shell prey may vary, leading to shifts in the size or type of prey consumed. Such dietary shifts can affect a species’ health as well as their distribution, both of which are consequential for marine ecosystems and fisheries.
The brown box crab, Lopholithodes foraminatus, is an ecologically and economically important species that spans a broad depth and geographic range, yet virtually nothing is known about their thermal ecology or feeding behavior. With few known biological studies on the brown box crab (Duguid and Page, 2009; Duguid and Page, 2011), and only one occurring in California (Stroud, 2022), gathering essential ecological information about the species is especially urgent considering that an Experimental Fishery Permit (EFP) was created for brown box crab in Southern California in 2019 to evaluate its potential as a commercial fishery (California Department of Fish and Wildlife Update on the Box Crab Experimental Fishing Permit Program). Brown box crabs are found in the Eastern Pacific from the Aleutian Islands, Alaska to San Diego, California, and reported as inhabiting depths between the low intertidal zone to over 500 m, though they typically reside below 18 m (Donaldson and Byersdorfer, 2005).This depth range has not been confirmed through other studies, as research on brown box crab is very limited and does not address their biogeography. However, prior experimental fisheries data of brown box crab in Alaska, Washington, and Oregon show that the species has been caught across much of its reported depth range (1 - 500 m) (Zhang et al., 2023). There are no data on the full depth range of brown box crab in Southern California, because there was no experimental fishery in the region prior to 2019. Nonetheless, this broad geographic and reported depth range would naturally expose brown box crabs to a wide range of environmental temperatures. In deeper waters off the Southern California coast, brown box crabs are exposed to temperatures averaging 8°C (Southwest Fisheries Science Center, 2023). While brown box crabs are unlikely to experience temperatures above 15°C in the shallower part of their depth range (Donaldson and Byersdorfer, 2005; Southwest Fisheries Science Center, 2023), intertidal and subtidal waters in Southern California can reach temperatures of up to 24°C in the summer or during extreme marine heatwave events that can last for weeks to months (Kekuewa et al., 2022). Little is known about the migration behavior of brown box crabs, but their close relatives, several species of king crabs (Donaldson and Byersdorfer, 2005; Hall and Thatje, 2011), typically reside at depths below 100 m and have been observed migrating to mate and hatch in warmer, shallower waters (0 - 100 m) (Stone et al., 1992; Donaldson and Byersdorfer, 2005). A year-long ultrasonic biotelemetry study on female red king crabs in Auke Bay, Alaska tracked crab movements across their depth range and revealed that females moved to deeper, cooler waters in the spring after mating, staying at those depths through early November. Afterwards, they moved back to warmer, shallower waters to molt and mate. In addition, this study uncovered that king crabs moved at average rates of over 80 m per day, exposing them to a range of depths and associated temperatures over a short timescale (Stone et al., 1992). While it is unknown if brown box crabs exhibit these same movement patterns, their zoeal stages were able to survive in the laboratory at 16°C (Duguid and Page, 2009), which is a higher threshold than other lithodid species from colder regions. This may indicate that brown box crabs occupy a wider thermal niche than related species and that they may tolerate warmer temperatures associated with shallower depths (Hall and Thatje, 2011). It would be beneficial to study how brown box crab metabolism and feeding behavior are affected by temperature variability to better understand their thermal ecology and the implications of this for their ecological and economic role in the Southern California benthos.
The primary goal of this study was to determine if the feeding behavior of brown box crabs is sensitive to environmental temperature. Considering that metabolic rate and muscle power output are both affected by temperature in other marine ectotherms, we hypothesized that box crab feeding behavior would be temperature-dependent. Feeding behavior was assessed as a series of metrics that included claw pinch force, prey preference, and appetite. Specifically, we predicted that crabs would exhibit higher pinch force at warmer temperatures, prefer prey items that require less breaking force at cooler temperatures but have no preference at warmer temperatures, consume prey items more quickly at warmer temperatures, and consume more prey items at warmer temperatures. We tested our hypothesis and predictions by carrying out a controlled laboratory experiment exposing wild-caught brown box crabs to a series of temperatures spanning those within their presumed depth range in Southern California.
2 Materials and methods
2.1 Animal collection and holding methods
Intermolt adult brown box crabs Lopholithodes foraminatus (Figure 1A) were captured by local fishers using baited traps offshore of Cortes Bank, California, USA (32.4667° N, 119.1667° W). All individuals were collected between MayA-August 2022 from a depth range of 100 - 250 m at bottom temperatures of 9 - 12°C (Southwest Fisheries Science Center, 2023). Crabs were immediately transported to Scripps Institution of Oceanography (SIO), University of California San Diego where they were maintained in large holding tanks receiving flow-through seawater pumped from the Scripps pier (3 - 4 m depth, 300 m offshore) and chilled to 10 - 12°C. Crabs were fed a mixed diet of mussels (Mytilus californianus) and bean clams (Donax gouldii) weekly until the start of the experiment. Individuals were held in these conditions for 10 - 14 weeks prior to the start of the experiment. Thirty individuals (20 M, 10 F) ranging from 199.26 - 710.39 g (530.00 ± 130.49 g M, 287.88 ± 35.05 g F) and 90.24 -140.05 mm carapace width (126.28 ± 10.14 mm M, 103.72 ± 5.98 mm F) were used for the experiment.
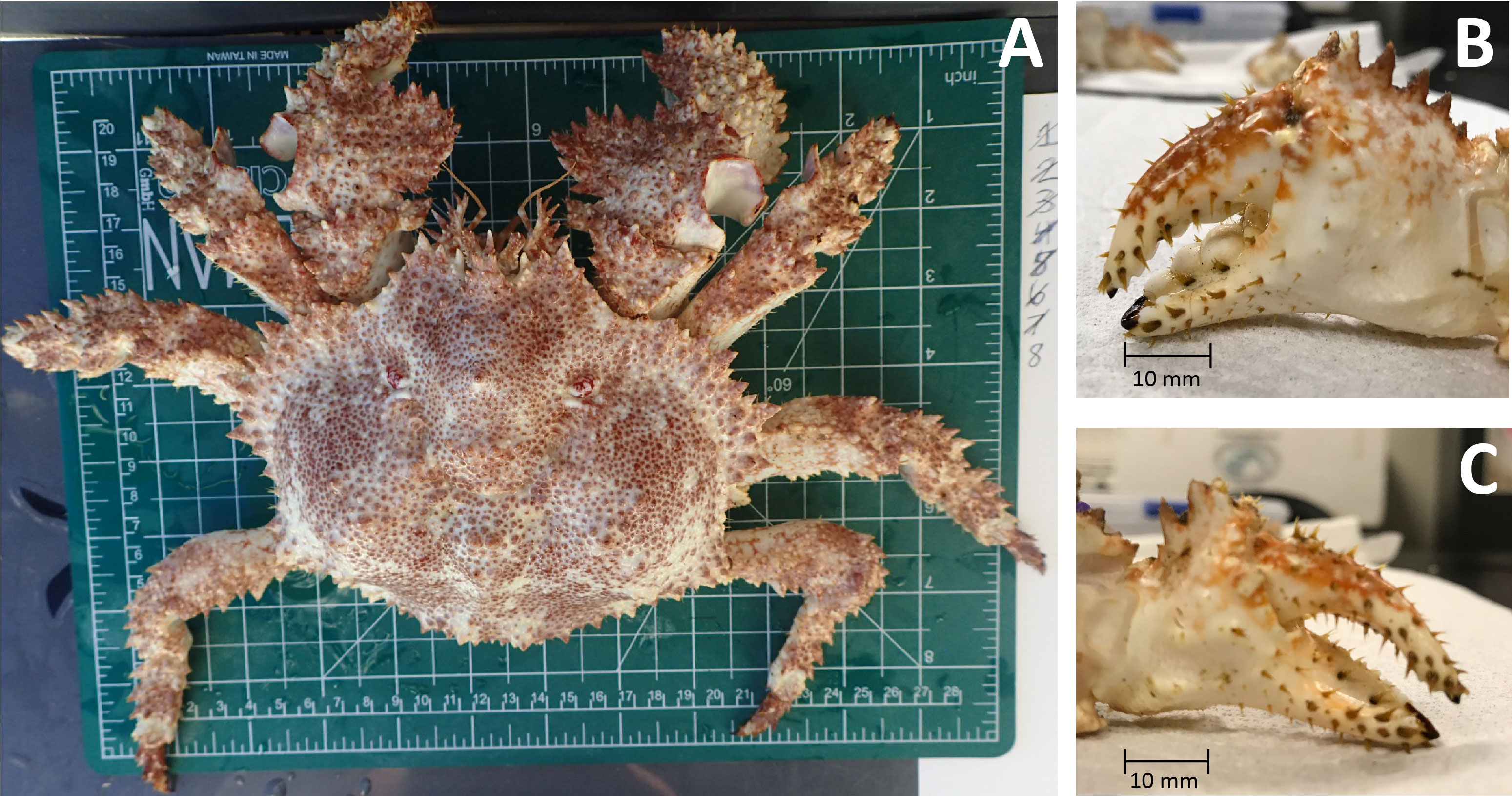
Figure 1 (A) Male brown box crab Lopholithodes foraminatus. Close up views of (B) right crusher claw and (C) left pincer claw.
2.2 Experimental set-up
One week prior to the start of the experiment, crabs were moved into individual 7.5 L plastic tanks that each received flow-through seawater from one of three header tanks (30 gal). Each of the header tanks received filtered seawater pumped from the SIO pier at either ambient (12°C) or chilled (9-10°C) temperatures. Three treatment temperatures (8°C, 15°C, and 20°C) were chosen to reflect the range that crabs may encounter in their local habitat. A low temperature of 8°C was selected because this represents the mean minimum temperature at deepest depth where brown box crabs have been reported at in Southern California (Southwest Fisheries Science Center, 2023). The intermediate temperature of 15°C represents the mean of Southern California waters at the reported typical upper depth range (18 m) of brown box crab (Southwest Fisheries Science Center, 2023).). The 20°C treatment was selected to represent the upper threshold of temperatures that brown box crabs would experience if occupying the shallowest (0 - 10 m) part of their reported depth range in Southern California. Data from the Scripps Ocean Acidification Real-time (SOAR) Monitoring Program was used to inform this upper treatment temperature. In summer 2019, water temperatures exceeded 19.9°C for 81/88 days, with an average summer temperature of 21.50 ± 1.30°C. In summer 2020, water temperatures exceeded 19.9°C for 84/108 days, with an average summer temperature of 21.58 ± 1.29°C (Clements et al., 2023; SOAR Dataset). The low temperature treatment (8°C) was achieved by cooling the chilled seawater with an aquarium chiller, whereas the higher temperature treatments (15°C and 20°C) were achieved by warming the ambient seawater with aquarium heaters. Box crabs were semi-randomly assigned to the three temperature treatments so that sex and size were evenly distributed. All individuals started at an ambient temperature of 12°C and header tanks were subsequently heated or cooled at a rate of 1°C per day until the desired temperature was reached. This rate of temperature change matched that of several other studies working on similar species (Stoner et al., 2010; Yuan et al., 2017).
The temperature in each of the header tanks was continuously monitored (data logged every 10 minutes) for the duration of the experiment using an Apex Lite aquarium controller with Apex Neptune temperature probes (accuracy 0.1°C, Neptune Systems, Morgan Hill, CA, USA) and maintained within ± 0.5°C of the target temperature. In addition, a hand-held thermometer (accuracy 0.5°C, HANNA Instruments, Woonsocket, RI, USA) was used to record the temperatures in each of the individual tanks containing crabs daily. The experiment was run for 10 weeks.
2.3 Feeding behavior
Three different metrics were used to assess brown box crab feeding behavior in response to temperature: claw pinch force, prey preference, and appetite level. Each of these metrics were assessed prior to the start of the experiment (week 0) as a baseline, after which claw pinch force measurements were made every two weeks while prey preference and appetite were recorded every week for the duration of the experiment.
Maximum pinch force of both the crusher and pincer claws was measured in air following the methods of Singh et al. (2000). Crabs were positioned on a custom platform with the pollex of the claw held in place by a curved metal bracket secured to the platform. The dactyl of the claw was then opened to maximum gape and positioned on a hook connected to a force sensor (CI-6537, ± 50 N range, PASCO, Roseville, CA, USA). Crabs readily pinched multiple times, with forces being recorded for 1 minute. Maximum pinch force was identified as the highest peak value on the force output curve produced using the PASCO Capstone Software (see Figure 2).
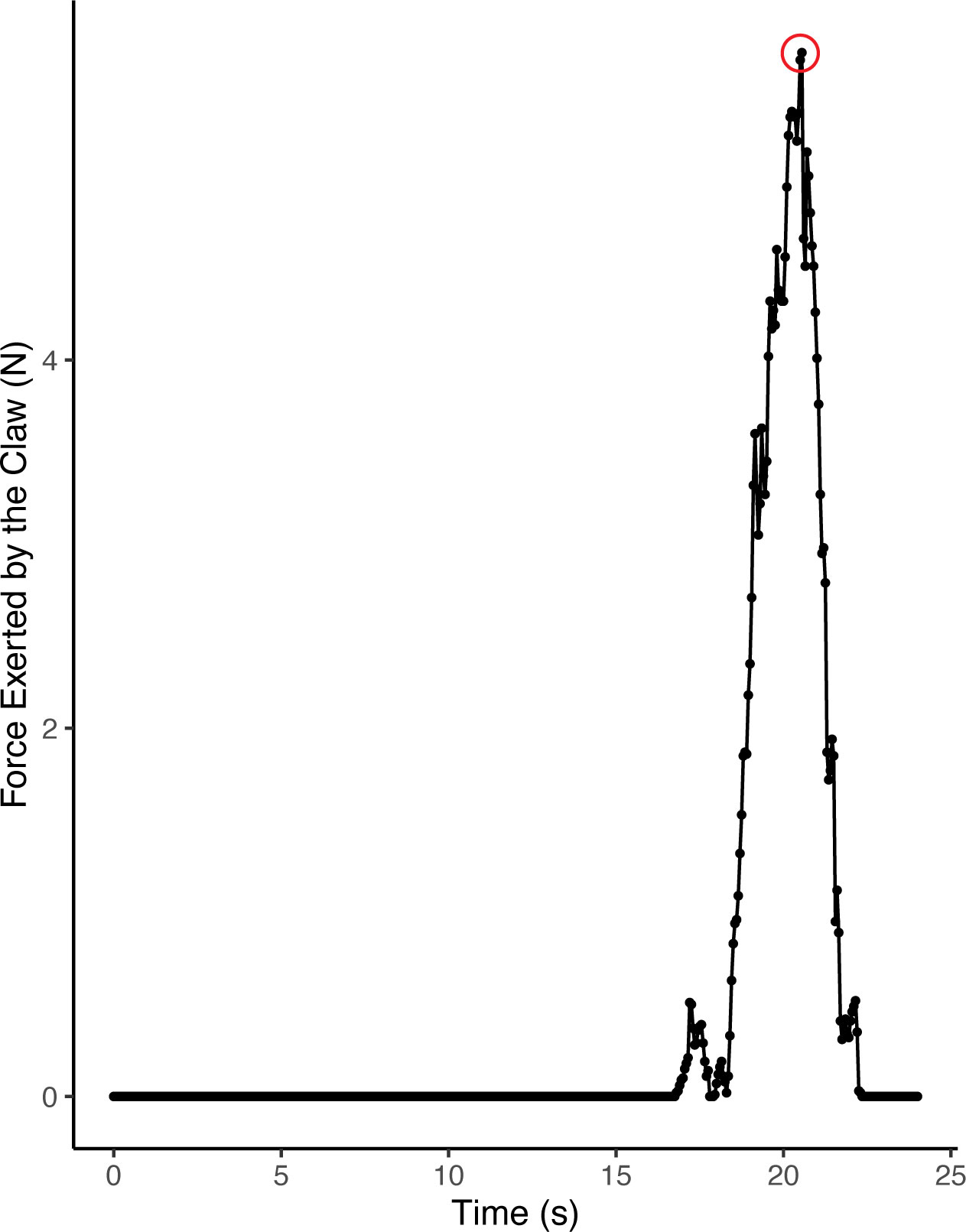
Figure 2 Example of claw pinch force measurement. The peak, or maximum force (red circle), was used for analysis.
To assess prey preference, crabs were offered two hard-shell prey items: a bean clam Donax gouldii and a mussel Mytilus californianus. Little is known about the diet of brown box crabs (Donaldson and Byersdorfer, 2005), but they are durophagous when held in the aquarium and readily consume these molluscs species. Both bivalve species occur in the shallow waters off of San Diego, CA and have overlapping size ranges. Mussels and clams with shell length of ~20 mm (19.73 ± 2.57 mm) were used for the experiment, and preliminary compression tests revealed that they had different crushing strengths. Mechanical tests were performed on live D. gouldii (n=9, 16.66 - 19.69 mm) and M. californianus (n=10, 16.01 - 21.59 mm) using a universal materials testing machine (E1000, Instron, Norwood, MA, USA) equipped with a 50 N static load cell (model 2530-437, 0.5 kN maximum, 0.125 N resolution, Instron, Norwood, MA, USA). Both species were positioned horizontally between two steel compression plates such that the compressive force was exerted on the widest part of the shell, the umbo. Compressive forces were applied at a rate of 20 N/min until fracture occurred, with the force at fracture considered to be the breaking strength. On average, it took twice as much force to break D. gouldii (101.74 ± 30.12 N) than to break M. californianus (49.69 ± 21.23 N). Thus, D. gouldii was considered to be a stronger prey item. Every week throughout the study, a clam and mussel of equal size were placed in the experimental tanks equidistant from the crab. We determined prey preference by noting which prey item each crab consumed first.
To assess appetite, we recorded the time it took for each crab to consume its first prey item. Prior studies on other marine ectotherms have also used feeding time as a metric to measure appetite (Hill, 1980; Diluzio et al., 2017; Moretto et al., 2022). Brown box crabs feed only about once per week when held in the laboratory (pers. observ.), so it is not feasible to observe and record the specific time that a crab consumes its prey. When provided with prey, some crabs begin to consume the item within the first day, whereas others take up to several days. We therefore binned the time to consume prey into three categories: within 24 hours, within 1 week, or no consumption. These categories were then assigned a score for analysis: a crab received a 0 for the week if they did not consume prey within that week, a 1 if they consumed at least one prey item within the week, and a 2 if they consumed at least one prey item within 24 hours. Crabs with higher scores consumed prey faster and were therefore considered to have a greater appetite. Generally, crabs consumed all soft tissue of the prey item regardless of the species. We also assessed appetite by recording how many total prey items (maximum of 2) were consumed by each crab every week over the course of the experiment.
2.4 Statistical analysis
All data were analyzed in R Studio v4.2.2 and tested for normality using a Shapiro-Wilk test and for homogeneity of variances using a Barlett test. Maximum pinch force was analyzed separately for crusher (Figure 1B) and pincer (Figure 1C) claws and compared across temperature treatments over the duration of the experiment using a repeated measures one-way analysis of covariance (ANCOVA) with carapace width as the covariate (rstatix package). The difference in maximum pinch force between the start and end of the experiment (tend - t0) was compared between treatment groups for the crusher claw using a Wilcoxon Rank test and for the pincer claw using a Pairwise t-test.
To examine the effect of temperature on prey preference, we determined the frequency that each individual consumed a clam first over the duration of the experiment (10 total feeding trials). We calculated each frequency value by dividing the number of times an individual consumed a clam first by the total number of feeding trials. The frequency of consuming a clam first was compared between the treatment groups using a Pairwise t-test.
Crab appetite was assessed via two metrics: time to feed and amount of prey items consumed. Time to feed was calculated by adding up the scores each individual received over the course of the experiment. Total scores for individual crabs were then compared between treatments using a Pairwise t-test. The number of prey items consumed each week per crab were totaled and then compared between treatments using a Pairwise t-test.
3 Results
3.1 Survivorship
All crabs in the 8°C and 15°C treatments survived the experiment, but there was 100% mortality in the 20°C treatment within 8 days of exposure (Figure 3). Therefore, no data were collected from the 20°C treatment.
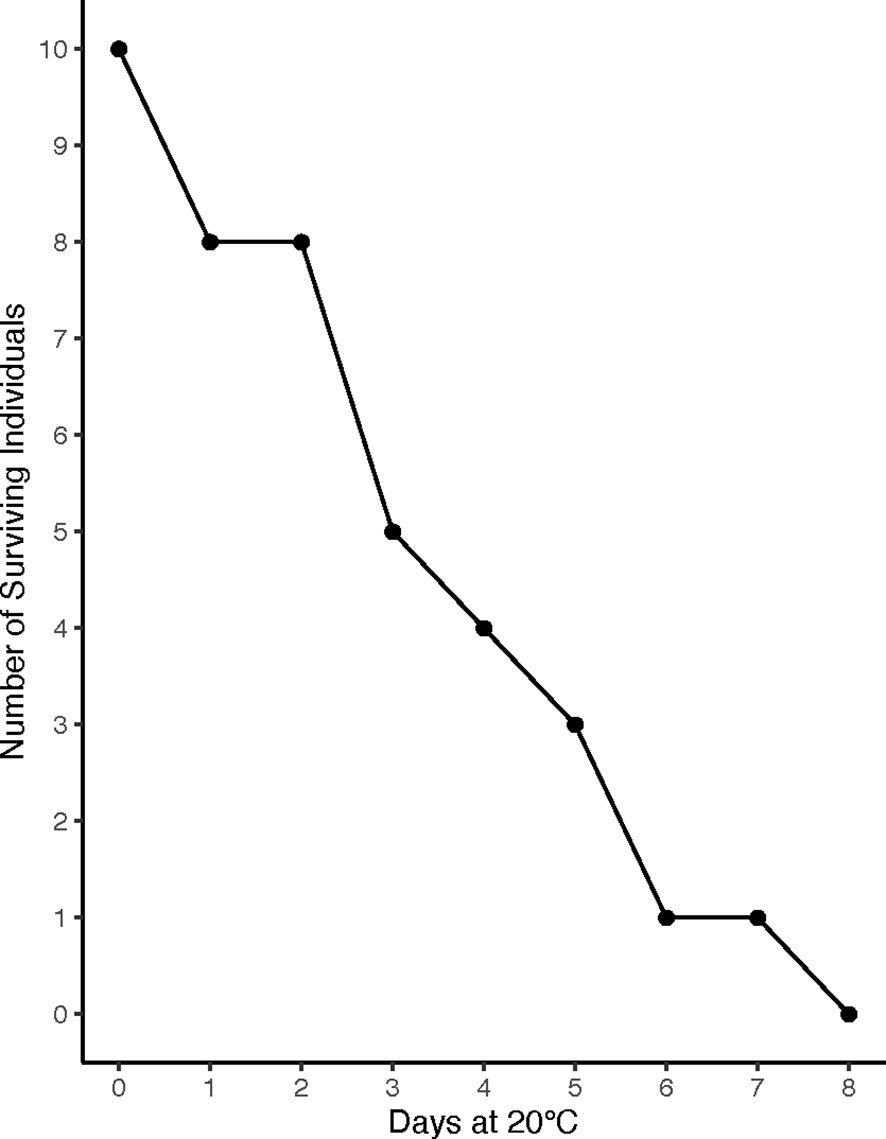
Figure 3 Survivorship at 20°C. Crabs in the 20°C treatment experienced consistent mortality during the first week of exposure, reaching 100% mortality by day 8 of the experiment (n=10).
3.2 Claw pinch force
There was no significant difference between temperature treatments in crusher claw maximum pinch force over time (F1,108 = 2.359, p = 0.128, Table 1, Figure 4A), but there was for the pincer claw (F1,108 = 6.784, p = 0.002, Table 1). Pinch force of the pincer claw increased steadily for crabs in the 15°C and decreased steadily for those in the 8°C (Figure 4B). At the start of the experiment (week 0), crabs in both the 8°C and 15°C treatments had similar crusher claw pinch forces (8°C: 3.46 ± 2.58 N, 15°C: 2.96 ± 1.81 N; pairwise t-test, N = 10, 10, p = 0.607) and pincer claw pinch forces (8°C: 2.44 ± 1.72 N, 15°C: 2.38 ± 1.83 N; pairwise t-test, p = 0.941), but these changed by the end of the experiment. The overall change in maximum pinch force that occurred between the start and the end of the experiment was significantly different between the treatment groups for the both crusher (Wilcoxon Rank Sum Test, W = 7, p < 0.0001) and the pincer claws (pairwise t-test, p = 0.0001). Crabs in the 8°C treatment experienced an average decrease in pinch force of -0.476 N for the crusher (Figure 4C) and -0.417 N for the pincer (Figure 4D). On the other hand, crabs in the 15°C treatment experienced an average increase in pinch force of +1.00 N for the crusher (Figure 4C) and +1.06 N for the pincer (Figure 4D). Carapace width had a significant effect on claw pinch force throughout the experiment in both treatments and for both claws (crusher: F1,108 = 95.609, p < 0.0001; pincer: F1,108 = 115.569, p < 0.0001, Table 1) with pinch force increasing with size of the crab.
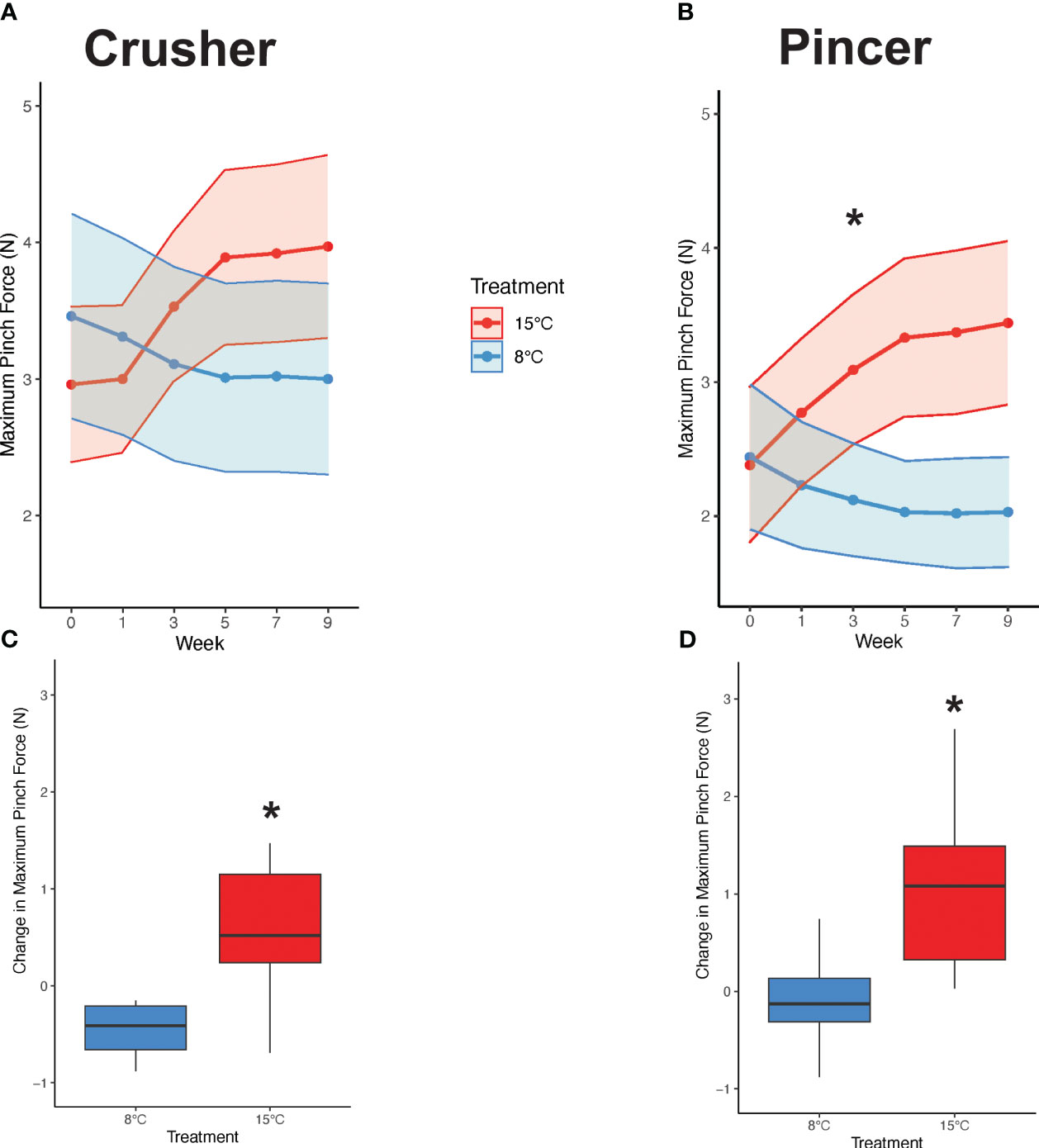
Figure 4 Claw pinch force over time. The average maximum pinch force was not significantly different between the warm and cool treatments over time for the crusher claw (A). The average maximum pinch force decreased steadily over time in the cold treatment and increased steadily over time in the warm treatment for the pincer claw (B). A repeated measures one-way ANCOVA was used to analyze the data in (A, B) (n=10 per treatment). The overall mean change in maximum claw pinch force was significantly greater for crabs in the warm treatment than the cold treatment for both crusher (C) and pincer (D) claws. The data in (C, D) were analyzed using a pairwise t-test (n=10 per treatment). Box boundaries are the first and third quartiles, the black line is the mean, whiskers are 1.5 times the interquartile range, and dots are outliers. Asterisks (*) indicate statistical significance (p <0.05). Error bars represent standard error.
3.3 Feeding behavior
Crabs in the 8°C treatment had a significantly higher preference for clams compared to crabs in the 15°C treatment (pairwise t-test, p < 0.0001), and this was consistent throughout the duration of the experiment. On average, crabs in the 8°C treatment consumed clams first for 74% of the feeding trials, whereas crabs in the 15°C treatment only consumed clams first for 21% of the feeding trials (Figure 5). Crabs in the 8°C treatment consumed prey significantly faster than crabs in the 15°C treatment over the duration of the experiment (pairwise t-test, p = 0.003) (Figure 6A). Crabs in the 8°C treatment typically consumed prey within 24 hours (63% of feeding trials), whereas they only consumed prey within 1 week for 22% of feeding trials and did not eat for 15% of feeding trials. On the other hand, the time for crabs in the 15°C treatment to consume prey was more variable (37% within 24 hours, 31% within 1 week, and 32% did not eat). Crabs in the 8°C treatment most frequently consumed both prey items (62% of feeding trials), whereas crabs in the 15°C treatment most frequently consumed 1 prey item (39% of feeding trials). Thus, crabs in the 8°C treatment appear to have consumed a greater amount of prey items than crabs in the 15°C treatment over the course of the experiment (8°C: 15.1 ± 4.09 prey items, 15°C: 10.5 ± 6.38 prey items), but the difference was not statistically significant (p = 0.071) (Figure 6B).
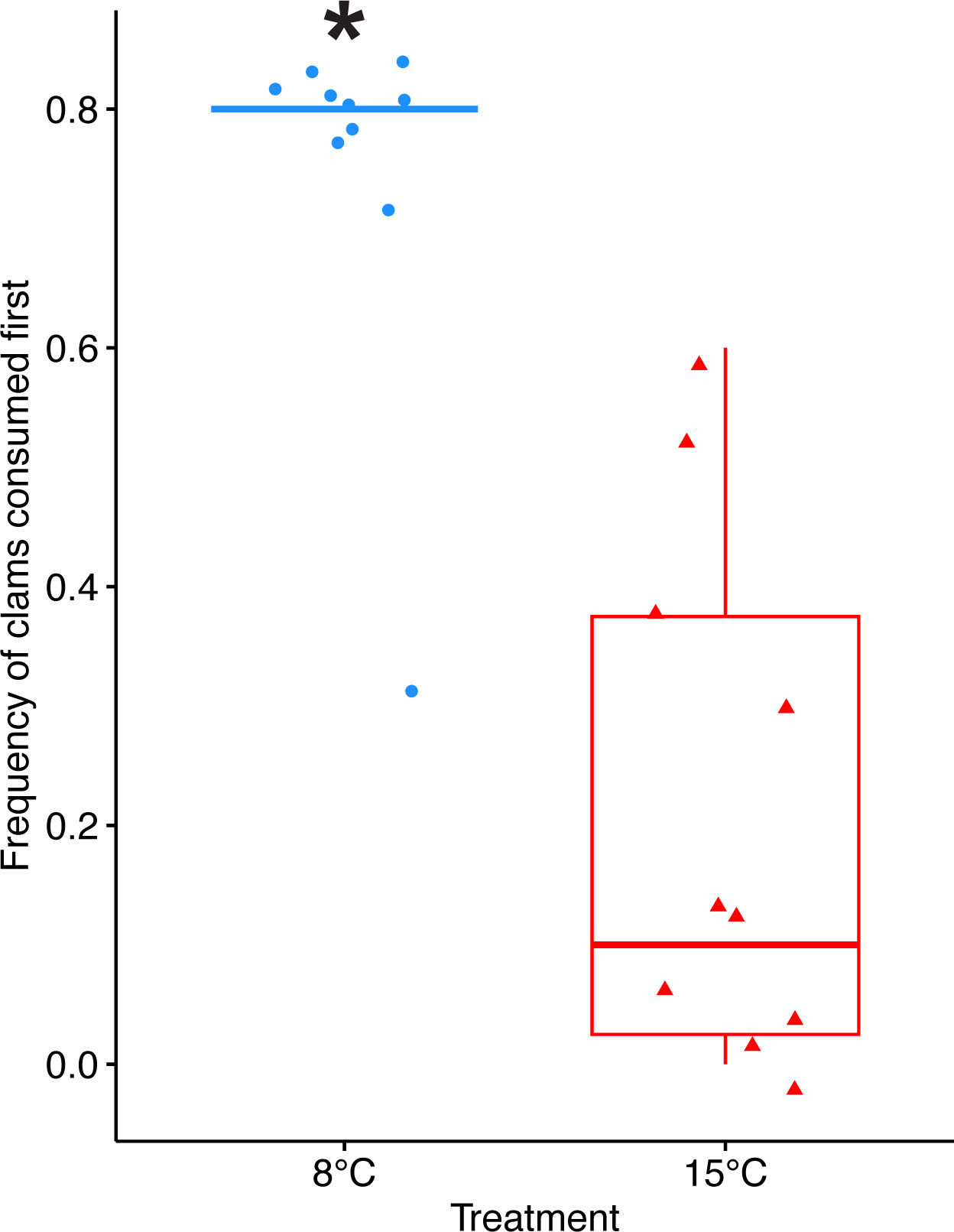
Figure 5 Prey preference for clams. Crabs in the cold treatment consumed clams first at a significantly higher frequency than crabs in the warm treatment. A pairwise t-test was used to compare across treatments (n=10 per treatment). Box boundaries are the first and third quartiles, black line is the mean, whiskers are 1.5 times the interquartile range, and shapes (circles and triangles) represent individual data points. Asterisks (*) indicate statistical significance (p < 0.05).
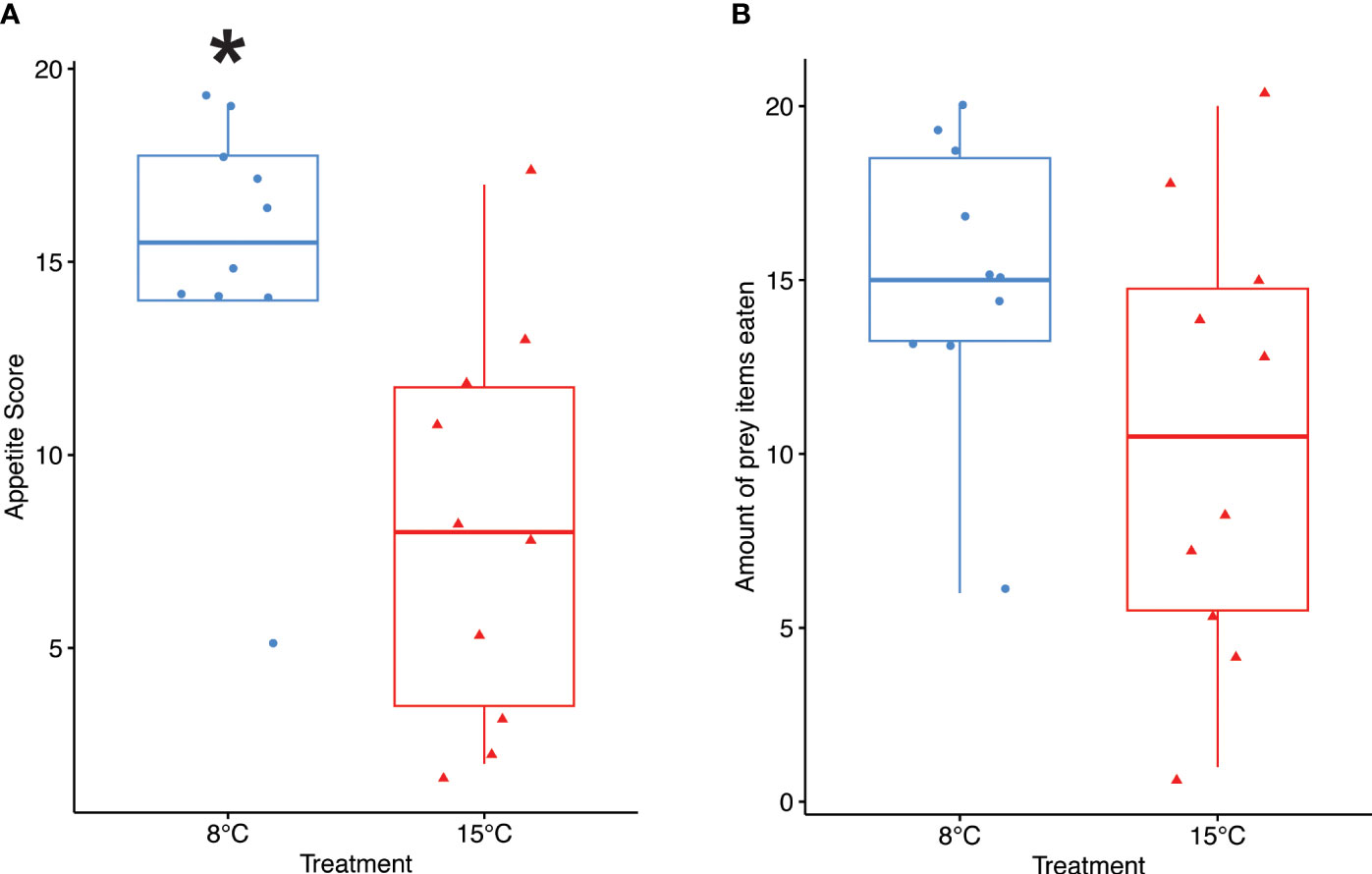
Figure 6 Appetite over time. Appetite level was measured as the time to consume prey (A) and the number of prey items eaten (B). A pairwise t-test was used to compare treatments (n=10 per treatment). Crabs in the cold treatment had a significantly higher appetite score than crabs in the warm treatment, indicating that they consumed prey more quickly, but the number of prey items eaten was not different between the two temperature treatments. Box boundaries are the first and third quartiles, black line is the mean, whiskers are 1.5 times the interquartile range, and shapes (circles and triangles) represent individual data points. Asterisks (*) indicate statistical significance (p < 0.05).
4 Discussion
Our study examined the influence of environmental temperature on the feeding behavior of brown box crabs in Southern California, which is the southernmost part of their geographic distribution and is characterized by comparatively large variations in temperature. Crabs acclimated to different temperatures representative of those throughout their depth range (8, 15, 20°C) showed immediate and persistent changes in feeding behavior over a two-month period, but not all aspects aligned with our hypotheses. As predicted, crabs in the warmer (15°C) treatment pinched with greater force than those in the cooler (8°C) treatment, but contrary to our predictions, the former had a strong preference for the weaker mussels while the latter preferred the stronger clams. Also contrary to our predictions, crabs at 8°C consumed prey items faster than those at 15°C, yet there were no significant differences in the number of prey items consumed. Furthermore, 100% mortality occurred quickly in the warmest (20°C) treatment, suggesting that brown box crabs are living close to their thermal limits in Southern California.
4.1 Thermal ecology
In Southern California, surface waters, down to about 10 m, exceed 20°C in summer months (Southwest Fisheries Science Center, 2023, Clements et al., 2023; SOAR Dataset) or during episodic warm water events (Kekuewa et al., 2022). Yet, we found that brown box crabs are unable to survive long at 20°C in the lab but can subsist at 8°C and 15°C for extended periods of at least two months. The 100% mortality that occurred within one week of exposure to 20°C indicates that this temperature is an upper thermal limit. This is consistent with two closely related species of king crab Paralithodes camtschaticus and P. platypus, which cannot survive at temperatures above 24.3°C and 21.3°C, respectively (Long and Daly, 2017). Thus, adult brown box crabs in Southern California could be subjected to lethal temperatures if they occupy shallow water during the summer. Even though these mortality data are extraneous to the feeding behavior focus of this study, they provide the first insights into brown box crab thermal ecology in Southern California and are pertinent to the emerging fishery.
The lethality of 20°C seawater for brown box crabs offers potential insight into their depth and geographic limits as well as their movement patterns. In Southern California, it is likely that adult crabs do not inhabit shallow waters throughout the year, as they do in the northern part of their range, where temperatures remain cool (Ladd and Stabeno, 2012). Related species of king crabs are known to migrate to shallow depths to mate and spawn (Stone et al., 1992; Donaldson and Byersdorfer, 2005), and if these same migrations occur in brown box crabs, their reproductive timing and/or depth may differ in Southern California waters. Tracking brown box crab movement patterns in the benthos across their geographic range is needed to uncover their temperature and depth limits. It would also be beneficial to repeat this study with brown box crabs captured from across their geographic range to determine if trends in feeding behavior hold across populations in both cold and warm regions. As ocean warming progresses, current brown box crab distributions and movement patterns could change, affecting local ecosystems and the sustainability of the growing fishery.
4.2 Pinch force
Like other decapod species with dimorphic claws, the crusher and pincer claws of brown box crabs function differently and generate different pinch forces. The crusher generated greater pinch forces (3.20 ± 2.04 N) than the pincer (2.41 ± 1.73 N) when first measured at week 0. After 10 weeks of exposure to either cool (8°C) or warm (15°C) seawater, the maximum pinch force of the pincer claw decreased by 16.8% and increased by 44.5%, respectively. The crusher claw followed the same trend over time, with cold acclimated crabs decreasing pinch force by 13.2% whereas warm acclimated crabs increased pinch force by 33.9%. These results demonstrate that brown box crab claw pinch force is responsive to environmental temperature. Similar conclusions were made in stone crabs, where in vivo claw closer muscle stress decreased in crabs acclimated to cold temperatures in the lab (Blundon, 1989). This was true for individuals from northern and southern populations, so regardless of their adaptation to different environmental temperature regimes. On the other hand, blue crabs from northern and southern populations showed no effect of laboratory temperature acclimation on in vivo claw closer muscle stress (Blundon, 1989), further demonstrating the variability in crustacean sensitivity to temperature. If brown box crabs pinch with less force at colder temperatures in their natural environment, then prey acquisition may be diminished at deeper depths and negatively impact survivorship in deeper, prey-limited environments (Childress et al., 1987). Alternatively, brown box crabs migrating to warmer, shallower waters or experiencing increases in environmental temperature, may increase their claw pinch force and consequently expand their potential prey options.
In this experiment, brown box crab crusher claws only exerted forces averaging 3.2 N, which wasn’t much greater than the 2.4 N average force generated by the pincer claws. These low forces are surprising given that crabs effectively crushed prey with breaking strengths ranging from 50 N to more than 100 N. Granted these breaking strengths were measured at the umbo, the strongest part of the shell of clams (Blundon, 1989) and not necessarily the point where crabs crush bivalves, there is a significant mismatch between the force exerted by the crusher claw and the force needed to crush prey. It is also surprising that there was not a greater difference in force production between the two claws given their difference in morphology and mechanical advantage. In other species, such as the blue crab Callinectes sapidus, crusher claws pinched with forces greater than 100 N while the pincers produced forces closer to 60 N (Blundon and Kennedy, 1982). The low forces produced by the crusher claws of brown box crabs could be due to their consistent reluctance to pinch with their crusher claws. Crabs generally held their claws close to their bodies and were disinclined to open them. This behavior sometimes required us to manually open their claws to position in the testing apparatus, and this artificial override of animal motivation may have contributed to low estimates of crusher claw pinch forces. Nonetheless, this approach produced clear results and similar pinch force devices have been used successfully with other species (Manríquez et al., 2021; Longmire et al., 2022), though they may have been more aggressive and willing to pinch than brown box crabs. Still, it is also possible that brown box crabs do not exert strong pinch forces in general. Preliminary pinch force data collected for the California king crab, a close relative that also occupies colder, deep water, show that this species has a low average pinch force of 1-2 N (Culver and Moretto, unpublished data).
Despite crusher claw pinch forces being potentially underestimated in this experiment, they still changed significantly after exposure to cool (8°C) and warm (15°C) temperature treatments, although the magnitudes of these changes were less than for the pincer claw. This could be due to the issues with motivation, as discussed above, but could also be due to differences in the neuromuscular physiology of the two claw types. Crusher claws are substantially larger than pincer claws and are primarily composed of slow muscle fibers, whereas pincers are composed of fast muscle fibers (Bennett, 1985; Taylor, 2000). Slow muscle fibers use energy over longer time periods and are less prone to fatigue, whereas fast muscle fibers produce greater force, but fatigue more quickly (Bennett, 1985). In addition to these key differences in muscle fibers, there is also disparity in neural innervation between the two claw types (Atwood, 1967; Govind, 1984). It is not unheard of that different muscle types respond divergently to changes in temperature. In the scup fish, Stenotomus chrysops, red muscle force production did not change with increased temperature, but pink muscle force increased (Coughlin and Rome, 1996). These observations, along with evidence that the neuromuscular physiology of crustaceans is temperature sensitive (Stephens and Atwood, 1982; Hamilton et al., 2007), presents the possibility that dimorphic claws have different relationships with temperature.
4.3 Prey preference
Environmentally-induced changes in either a crab’s metabolic needs or the mechanical efficiency of their claws could lead to dietary shifts (Reichmuth et al., 2009). In this study, brown box crabs acclimated to two temperatures displayed a consistent difference in prey preference, with crabs preferring clams at 8°C and mussels at 15°C. These results confirmed that there is a shift in prey preference with temperature, but not as predicted based on our hypothesis about claw mechanical output. We expected that crabs acclimated to the cooler temperature would prefer mussels, because they require less breaking force and ectotherms generally exhibit decreased activity at colder temperatures (Hall and Thatje, 2011). Yet, cold acclimated crabs preferred the stronger clams. In contrast, we expected that crabs acclimated to warmer temperatures would exhibit no prey preference, yet they preferred mussels. There are several possible explanations for these unexpected results. First, brown box crabs in the 15°C treatment could have been closer to a thermal limit (particularly since 20°C was a lethal temperature). As described by the OCLTT framework, if an organism is at a temperature above its thermal optima and enters the pejus range, we see declines in performance behaviors because of a switch to anaerobic metabolism, where an animal reallocates all of its limited energy resources to survival. This could explain why we observed crabs at 15°C opting for prey with a lower breaking force (mussels) as this might limit excess energy expenditure. Brown box crabs in the 8°C treatment were further from this potential thermal limit, and therefore would not need to reduce energy expenditures. Thus, crabs could preferentially consume the harder-shelled prey (clams) at this temperature because more energy could be expended on prey handling. While the thermal optima for brown box crabs has yet to be determined, their increased performance at the colder treatment aligns with closely related species of king crab that have thermal optima ranging from 4 - 10°C (Christiansen et al., 2015; Swiney et al., 2017).
There are other important aspects of prey selection that were not assessed in this study but could also drive the observed preferences of brown box crabs. First, crabs likely do not break shells at the umbo, where breaking strength was measured, but rather at weaker regions of the valves. Our estimates of shell strength may, therefore, not accurately reflect the forces that crabs need to exert when crushing mussels and clams, nor do they account for ease of handling of these two shell types. We were never able to observe brown box crab feeding during our experiment, however, other crab species that consume bivalves have been known to hold the prey item with one claw while using the other claw to pinch the prey item multiple times until it breaks (Bertness and Cunningham, 1981; Wójcik et al., 2015). Brown box crab preferences may factor in prey handling rather than simply breaking strength. Second, the nutritional value of the clams and mussels used in this study may differ and be assessable by crabs. Nutrition-based prey preference has been described in other marine invertebrates, including a species of octopus that selected crabs over clams in a series of controlled laboratory experiments due to the higher lipid content of the crabs (Onthank and Cowles, 2011), and certain species of copepods that selected for ciliates over phytoplankton to increase carbon intake (Broglio et al., 2004). Mussels and clams, albeit different species than those used in this study, have different values of macronutrients (Wright et al., 2018), the needs of which by crabs may change at different temperatures. Lastly, changes in prey preference could stem from temperature effects on sensory systems. Both olfaction and vision are known to be sensitive to temperature in fish (Reilly and Thompson, 2007) and could potentially alter how animals detect and perceive prey.
4.4 Appetite
Metabolic activity is generally higher at warmer temperatures and crabs may need to consume more food to sustain these increased demands (Childress et al., 1990; Siikavuopio and James, 2015), as seen a variety of ectothermic fish species (Raderman-Little, 1979; Volkoff and Rønnestad, 2020). We assessed crab appetite as both the number of prey items consumed and the time it took to consume prey. Crabs in the 8°C treatment consumed prey items significantly faster than crabs in the 15°C treatment, but did not consume a statistically greater number of prey. Consuming prey faster at the colder temperature may be indicative of greater appetite, or efficiency, but this did not translate into more prey consumed. Colder temperatures can reduce digestion rate, as observed in fish (Raderman-Little, 1979), which would presumably delay satiation. Offering ad libitum amounts of prey may have altered our outcome on the total prey consumed, but brown box crabs generally consume small amounts of food when held in the lab at 12°C. While we originally predicted that crabs would have an increased appetite at warmer temperatures due to the increase in metabolism that occurs with temperature in ectotherms (Leffler, 1972; Newell and Branch, 1980; Vianna et al., 2020), crabs at the cooler temperature had a greater appetite (i.e., shorter time to consume prey) than those at the warmer temperature. These results further suggest that brown box crabs in the 15°C treatment could have been closer to a thermal limit, as we again saw declines in performance behaviors (appetite) in the warmer treatment. The greater appetite at 8°C also suggests that brown box crabs can allocate more energy to consuming prey at this colder temperature and that they may require more prey to maintain homeostasis.
Brown box crab appetite changes with temperature in the lab, and if this extends to their natural environment, then it might affect their movement patterns and depth gradient. Satisfying a greater appetite in colder, deeper water could be challenging since prey availability tends to be more limited at deeper depths (Childress et al., 1987), requiring more foraging and energy expenditure. To cope with these energetic constraints, brown box crabs may have to migrate shallower, where prey is more abundant, but their appetite is reduced. Regardless of temperature, brown box crabs have a relatively small appetite compared to other species of crabs (Taylor, pers. observ.), so it is unlikely that they have much of an impact on local mollusk populations. In the lab, brown box crabs only consume mollusks, suggesting that they may have a limited diet and consumer role in Southern California. Determining the diet of brown box crabs and whether it varies with depth is essential in understanding their prey acquisition in the wild and how it will be impacted by short- and long-term changes in ocean temperature. Using stable isotope and gut content analysis to study brown box crab diet across their depth range needs to be explored.
4.5 Conclusions and implications for conservation and fishery management
Our study provides the first insights into the thermal ecology and feeding behavior of brown box crabs at the southern end of their distribution. We show that brown box crab feeding behavior is temperature-dependent, which has critical implications for species survival, distribution, and their development as a fishery in Southern California. Claw pinch force was responsive to temperature, which means that their ability to acquire prey may be significantly affected as temperatures warm in a changing climate. Despite increases in force production capacity with temperature, box crabs preferred prey with stronger shells and ate prey faster when acclimated to the cold temperature treatment, suggesting that metabolic and energy requirements win-out over claw strength. While our survivorship, prey preference, and appetite findings suggest that brown box crabs are restricted to temperatures below 20°C and exhibit increased feeding and energy expenditure at colder temperatures (8°C), further study to determine their thermal range and thermal optima is needed to better understand the thermal ecology of this species. Future studies that measure changes to oxygen consumption and heart rate across their temperature differential would be especially enlightening. Furthermore, our study encompassed a size range spanning adults of both sexes, with males being larger than females, but found no effect of body size, and by proxy sex, on any aspect of feeding behavior measured. Our sampling only included large crabs of regulation size and was dominated by males, and thus it was inadequate to decipher potential differences between sexes, which may have different energetic requirements. For a more comprehensive assessment of the thermal ecology of brown box crabs, it would be beneficial to extend physiological and behavioral studies to include males and females across life stages and reproductive cycles.
Despite uncertainties of the brown box crab’s depth and thermal range, it is particularly noteworthy that 20°C is a lethal temperature for brown box crabs in the lab, yet it is typical of surface and subtidal waters in Southern California during summer months and marine heatwave events. During these warm water periods, crabs may be restricted to greater depths, which can affect their breeding and spawning behavior. Thus, tracking brown box crabs across their geographic range is essential for understanding how they adapt to the warm waters at the southern part of their range. Doing so would uncover whether brown box crabs need to inhabit deeper depths in Southern California compared to their northern counterparts. The inability of brown box crabs to survive at temperatures exceeding 20°C also has important implications for their emergent fishery. While fishers are typically setting traps for box crabs deeper than 100 m, where temperatures remain cool, when they pull up their traps crabs are rapidly exposed to warm surface waters that exceed 20°C in summer months. Indeed, local fishers in San Diego, California reported increased mortality of brown box crabs caught between July-August 2022, coinciding with average surface water temperatures of around 21°C (Southwest Fisheries Science Center, 2023, Clements et al., 2023; SOAR Dataset). This increased mortality of caught crabs during summer months and their inability to survive above 20°C in our study behoove fishery regulators to close the current year-round fishing season during summer months or anomalous warm water events.
Ultimately, this study has demonstrated that brown box crab feeding behavior is temperature-dependent, similar to what has been observed across many fish species, and it uncovered several knowledge gaps that need to be filled to understand brown box crab survival in a changing climate and their viability as a commercial fishery. Further studies should explore what prey brown box crabs are eating in their natural environments, the true depth range of the species, and their critical thermal maxima across life history stages.
Data availability statement
The datasets presented in this study can be found in online repositories. The names of the repository/repositories and accession number(s) can be found below: https://doi.org/10.5061/dryad.jwstqjqft.
Ethics statement
The requirement of ethical approval was waived by the Institutional Animal Care and Use Committee (IACUC) Office at University of California San Diego for the studies involving animals because ethical review and approval was not required for the study on animals in accordance with the local legislation and institutional requirements. The studies were conducted in accordance with the local legislation and institutional requirements.
Author contributions
WM and JT contributed to the conception and design of the study. WM collected the data, performed statistical analyses, and organized the database. WM wrote the first draft of the manuscript. WM and JT contributed to the revision, read, and approved the submitted version.
Funding
The authors would like to thank the Scripps Institution of Oceanography Master’s Student Research Award for providing funding to make this project possible.
Acknowledgments
The authors would like to thank Dr. Carolynn Culver at California Sea Grant for sharing her expertise on brown box crabs and for coordinating with local fishers to collect animals, undergraduate intern Annemarie Hancock for her help running the experiment, Phil Zerofski and Christine Steinke for animal husbandry and aquarium maintenance support, and the Taylor Lab for general project support and feedback. Long term temperature data sets were provided by the Scripps Ocean Acidification Real-time (SOAR) Monitoring Program, a long-term coastal ocean acidification monitoring set supported by Dr. Jennifer Smith, Dr. Todd Martz and Dr. Andrew Dickson and managed by Samantha Clements at Scripps Institution of Oceanography in La Jolla, CA. Significant funding for collection of these data was provided by the Ellen Browning Foundation.
Conflict of interest
The authors declare that the research was conducted in the absence of any commercial or financial relationships that could be construed as a potential conflict of interest.
Publisher’s note
All claims expressed in this article are solely those of the authors and do not necessarily represent those of their affiliated organizations, or those of the publisher, the editors and the reviewers. Any product that may be evaluated in this article, or claim that may be made by its manufacturer, is not guaranteed or endorsed by the publisher.
References
Abram P. K., Boivin G., Moiroux J., Brodeur J. (2017). Behavioural effects of temperature on ectothermic animals: unifying thermal physiology and behavioural plasticity. Biol. Rev. 92, 1859–1876. doi: 10.1111/brv.12312
Altringham J. D., Block B. A. (1997). Why do tuna maintain elevated slow muscle temperatures? Power output of muscle isolated from endothermic and ectothermic fish. J. Exp. Biol. 200, 2617–2627. doi: 10.1242/jeb.200.20.2617
Archer D., Martin P., Buffett B., Brovkin V., Rahmstorf S., Ganopolski A. (2004). The importance of ocean temperature to global biogeochemistry. Earth Planet. Sci. Lett. 222, 333–348. doi: 10.1016/j.epsl.2004.03.011
Atwood H. L. (1967). Crustacean neuromuscular mechanisms. Am. Zool. 7, 527–552. doi: 10.1093/icb/7.3.527
Bennett A. F. (1985). Temperature and muscle. J. Exp. Biol. 115, 333–344. doi: 10.1242/jeb.115.1.333
Bertness M. D., Cunningham C. (1981). Crab shell-crushing predation and gastropod architectural defense. J. Exp. Mar. Biol. Ecol. 50, 213–230. doi: 10.1016/0022-0981(81)90051-4
Blundon J. A. (1989). Effects of temperature and thermal history on neuromuscular properties of two crustacean species. J. Comp. Physiol. B 158, 689–696. doi: 10.1007/BF00693006
Blundon J. A., Kennedy V. S. (1982). Mechanical and behavioral aspects of blue crab, Callinectes sapidus (Rathbun), predation on Chesapeake Bay bivalves. J. Exp. Mar. Biol. Ecol. 65, 47–65. doi: 10.1016/0022-0981(82)90175-7
Britayev T. A., Rzhavsky A. V., Pavlova L. V., Dvoretskij A. G. (2010). Studies on impact of the alien Red King Crab (Paralithodes camtschaticus) on the shallow water benthic communities of the Barents Sea. J. Appl. Ichthyol. 26, 66–73. doi: 10.1111/j.1439-0426.2010.01494.x
Broglio E., Saiz E., Calbet A., Trepat I., Alcaraz M. (2004). Trophic impact and prey selection by crustacean zooplankton on the microbial communities of an oligotrophic coastal area (NW Mediterranean Sea). Aquat. Microb. Ecol. 35, 65–78. doi: 10.3354/ame035065
Childress J. J., Cowles D. L., Favuzzi J. A., Mickel T. J. (1990). Metabolic rates of benthic deep-sea decapod crustaceans decline with increasing depth primarily due to the decline in temperature. Deep Sea Res. Part Oceanogr. Res. Pap. 37, 929–949. doi: 10.1016/0198-0149(90)90104-4
Childress J. J., Felbeck H., Somero G. N. (1987). Symbiosis in the deep sea. Sci. Am. 256, 114–121. doi: 10.1038/scientificamerican0587-114
Christiansen J. S., Sparboe M., Sæther B.-S., Siikavuopio S. I. (2015). Thermal behaviour and the prospect spread of an invasive benthic top predator onto the Euro-Arctic shelves. Divers. Distrib. 21, 1004–1013. doi: 10.1111/ddi.12321
Clements S. C., Kram S. L., Takeshita Y., Dickson A., Martz T., Smith J. E. (2023). Scripps Ocean Acidification Real-time (SOAR) Dataset (La Jolla, CA: Scripps Institution of Oceanography).
Coughlin D. J., Rome L. C. (1996). The roles of pink and red muscle in powering steady swimming in scup, stenotomus chrysops1. Am. Zoologist 36, 666–677. doi: 10.1093/icb/36.6.666
Cowles D. L., Childress J. J., Wells M. E. (1991). Metabolic rates of midwater crustaceans as a function of depth of occurrence off the Hawaiian Islands: Food availability as a selective factor? Mar. Biol. 110, 75–83. doi: 10.1007/BF01313094
Cutler K. B., Edwards R. L., Taylor F. W., Cheng H., Adkins J., Gallup C. D., et al. (2003). Rapid sea-level fall and deep-ocean temperature change since the last interglacial period. Earth Planet. Sci. Lett. 206, 253–271. doi: 10.1016/S0012-821X(02)01107-X
Diluzio A. R., Baliga V. B., Higgins B. A., Mehta R. S. (2017). Effects of prey characteristics on the feeding behaviors of an apex marine predator, the California moray (Gymnothorax mordax). Zool. Jena Ger. 122, 80–89. doi: 10.1016/j.zool.2017.03.002
Donaldson W., Byersdorfer S. (2005). Biological Field Techniques for Lithodid Crabs (Alaska Sea Grant: University of Alaska Fairbanks).
Duguid W. D. P., Page L. R. (2009). Larval and early post-larval morphology, growth, and behaviour of laboratory reared Lopholithodes foraminatus (brown box crab). J. Mar. Biol. Assoc. U. K. 89, 1607–1626. doi: 10.1017/S002531540900068X
Duguid W. D. P., Page L. R. (2011). Biennial reproduction with embryonic diapause in Lopholithodes foraminatus (Anomura: Lithodidae) from British Columbia waters. Invertebr. Biol. 130, 68–82. doi: 10.1111/j.1744-7410.2011.00221.x
Elner R. W., Hughes R. N. (1978). Energy maximization in the diet of the shore crab, carcinus maenas. J. Anim. Ecol. 47, 103–116. doi: 10.2307/3925
Ferrari M. C. O., Munday P. L., Rummer J. L., McCormick M. I., Corkill K., Watson S.-A., et al. (2015). Interactive effects of ocean acidification and rising sea temperatures alter predation rate and predator selectivity in reef fish communities. Glob. Chang. Biol. 21, 1848–1855. doi: 10.1111/gcb.12818
Freitas V., Cardoso J. F. M. F., Lika K., Peck M. A., Campos J., Kooijman S. A. L. M., et al. (2010). Temperature tolerance and energetics: a dynamic energy budget-based comparison of North Atlantic marine species. Philos. Trans. R. Soc B Biol. Sci. 365, 3553–3565. doi: 10.1098/rstb.2010.0049
Govind C. K. (1984). Development of asymmetry in the neuromuscular system of lobster claws. Neurobiology 10, 1423-1445. doi: 10.2307/1541340
Gunderson A. R., Armstrong E. J., Stillman J. H. (2016). Multiple stressors in a changing world: the need for an improved perspective on physiological responses to the dynamic marine environment. Annu. Rev. Mar. Sci. 8, 357–378. doi: 10.1146/annurev-marine-122414-033953
Hall S., Thatje S. (2011). Temperature-driven biogeography of the deep-sea family Lithodidae (Crustacea: Decapoda: Anomura) in the Southern Ocean. Polar Biol. 34, 363–370. doi: 10.1007/s00300-010-0890-0
Hamilton J. L., Edwards C. R., Holt S. R., Worden M. K. (2007). Temperature dependent modulation of lobster neuromuscular properties by serotonin. J. Exp. Biol. 210, 1025–1035. doi: 10.1242/jeb.02717
Hill B. J. (1980). Effects of temperature on feeding and activity in the crab Scylla serrata. Mar. Biol. 59, 189–192. doi: 10.1007/BF00396867
Josephson R. K. (1993). Contraction dynamics and power output of skeletal muscle. Annu. Rev. Physiol. 55, 527–546. doi: 10.1146/annurev.ph.55.030193.002523
Josephson R., Stokes D. (1994). Contractile properties of a high-frequency muscle from a crustacean - mechanical power output. J. Exp. Biol. 187, 295–303. doi: 10.1242/jeb.187.1.295
Kekuewa S. A., Courtney T. A., Cyronak T., Andersson A. J. (2022). Seasonal nearshore ocean acidification and deoxygenation in the Southern California Bight. Sci. Rep. 12 (1), 17969. doi: 10.1038/s41598-022-21831-y
Ladd C., Stabeno P. J. (2012). Stratification on the Eastern Bering Sea shelf revisited. Deep Sea Res. Part II Top. Stud. Oceanogr. 65, 72–83. doi: 10.1016/j.dsr2.2012.02.009
Langfeld K. S., Altringham J. D., Johnston I. A. (1989). Temperature and the force-velocity relationship of live muscle fibres from the teleost myoxocephalus scorpius. J. Exp. Biol. 144, 437–448. doi: 10.1242/jeb.144.1.437
Leffler C. W. (1972). Some effects of temperature on the growth and metabolic rate of juvenile blue crabs, Callinectes sapidus, in the laboratory. Mar. Biol. 14, 104–110. doi: 10.1007/BF00373209
Lima S. L., Dill L. M. (1990). Behavioral decisions made under the risk of predation: a review and prospectus. Can. J. Zool. 68, 619–640. doi: 10.1139/z90-092
Long W. C., Daly B. (2017). Upper thermal tolerance in red and blue king crab: sublethal and lethal effects. Mar. Biol. 164. doi: 10.1007/s00227-017-3190-1
Longmire K. S., Seitz R. D., Seebo M. S., Brill R. W., Lipcius R. N. (2022). Biological responses of the predatory blue crab and its hard clam prey to ocean acidification and low salinity. Mar. Ecol. Prog. Ser. 701, 67–81. doi: 10.3354/meps14198
Manríquez P. H., González C. P., Seguel M., Garcia-Huidobro M. R., Lohrmann K. B., Domenici P., et al. (2021). The combined effects of ocean acidification and warming on a habitat-forming shell-crushing predatory crab. Sci. Total Environ. 758, 143587. doi: 10.1016/j.scitotenv.2020.143587
Moretto W. I., Stahl A. K., Mehta R. S. (2022). Effects of acute temperature change on California moray prey manipulation and transport behavior. Zoology 154, 126030. doi: 10.1016/j.zool.2022.126030
Newell R. C., Branch G. M. (1980). “The influence of Temperature on the Maintenance of Metabolic Energy Balance in Marine Invertebrates,” in Advances in Marine Biology. Eds. Blaxter J.H. S., Russell F.S., Yonge M. (Academic Press) 17, 329–396.
Nguyen K. D. T., Morley S. A., Lai C.-H., Clark M. S., Tan K. S., Bates A. E., et al. (2011). Upper temperature limits of tropical marine ectotherms: global warming implications. PloS One 6, e29340. doi: 10.1371/journal.pone.0029340
Onthank K. L., Cowles D. L. (2011). Prey selection in Octopus rubescens: possible roles of energy budgeting and prey nutritional composition. Mar. Biol. 158, 2795–2804. doi: 10.1007/s00227-011-1778-4
Peck L. S. (2005). Prospects for survival in the Southern Ocean: vulnerability of benthic species to temperature change. Antarct. Sci. 17, 497–507. doi: 10.1017/S0954102005002920
Perry A. L., Low P. J., Ellis J. R., Reynolds J. D. (2005). Climate change and distribution shifts in marine fishes. Science 308, 1912–1915. doi: 10.1126/science.1111322
Pimentel M. S., Faleiro F., Marques T., Bispo R., Dionísio G., Faria A. M., et al. (2016). Foraging behaviour, swimming performance and malformations of early stages of commercially important fishes under ocean acidification and warming. Clim. Change 137, 495–509. doi: 10.1007/s10584-016-1682-5
Pörtner H. O., Bennett A. F., Bozinovic F., Clarke A., Lardies M. A., Lucassen M., et al. (2006). Trade-offs in thermal adaptation: the need for a molecular to ecological integration. Physiol. Biochem. Zool. PBZ 79, 295–313. doi: 10.1086/499986
Pörtner H.-O., Bock C., Mark F. C. (2017). Oxygen- and capacity-limited thermal tolerance: bridging ecology and physiology. J. Exp. Biol. 220, 2685–2696. doi: 10.1242/jeb.134585
Raderman-Little R. (1979). The effect of temperature on the turnover of taste bud cells in catfish. Cell Proliferation 12, 269–280. doi: 10.1111/j.1365-2184.1979.tb00149.x
Reichmuth J. M., Roudez R., Glover T., Weis J. S. (2009). Differences in prey capture behavior in populations of blue crab (Callinectes sapidus rathbun) from contaminated and clean estuaries in new Jersey. Estuaries Coasts 32, 298–308. doi: 10.1007/s12237-008-9130-z
Reilly C. R. L., Thompson S. H. (2007). Temperature effects on low-light vision in juvenile rockfish (genus Sebastes) and consequences for habitat utilization. J. Comp. Physiol. A Neuroethol. Sens Neural Behav. Physiol. 193, 943–953. doi: 10.1007/s00359-007-0247-5
Sanchez-Salazar M. E., Griffiths C. L., Seed R. (1987). The effect of size and temperature on the predation of cockles Cerastoderma edule (L.) by the shore crab Carcinus maenas (L.). J. Exp. Mar. Biol. Ecol. 111, 181–193. doi: 10.1016/0022-0981(87)90054-2
Sastry A. N. (1966). Temperature effects in reproduction of the bay scallop, aequipecten irradians lamarck. Biol. Bull. 130, 118–134. doi: 10.2307/1539958
Schofield G., Bishop C. M., Katselidis K. A., Dimopoulos P., Pantis J. D., Hays G. C. (2009). Microhabitat selection by sea turtles in a dynamic thermal marine environment. J. Anim. Ecol. 78, 14–21. doi: 10.1111/j.1365-2656.2008.01454.x
Schulte P. M. (2015). The effects of temperature on aerobic metabolism: towards a mechanistic understanding of the responses of ectotherms to a changing environment. J. Exp. Biol. 218, 1856–1866. doi: 10.1242/jeb.118851
Shields J. D. (2019). Climate change enhances disease processes in crustaceans: case studies in lobsters, crabs, and shrimps. J. Crustac. Biol. 39, 673–683. doi: 10.1093/jcbiol/ruz072
Siikavuopio S. I., James P. (2015). Effects of temperature on feed intake, growth and oxygen consumption in adult male king crab Paralithodes camtschaticus held in captivity and fed manufactured diets. Aquac. Res. 46, 602–608. doi: 10.1111/are.12207
Singh G., Block J., Rebach S. (2000). Measurement of the crushing force of the crab claw. Crustaceana 73, 633–637. doi: 10.1163/156854000504606
Southwest Fisheries Science Center (2023) California cooperative oceanic fisheries investigations (CalCOFI) database. Available at: https://www.fisheries.noaa.gov/inport/item/20691.
Stephens P. J., Atwood H. L. (1982). Thermal acclimation in A crustacean neuromuscular system. J. Exp. Biol. 98, 39–47. doi: 10.1242/jeb.98.1.39
Stone R. P., O’Clair C. E., Shirley T. C. (1992). Seasonal migration and distribution of female red king crabs in a Southeast Alaskan Estuary. J. Crustac. Biol. 12, 546–560. doi: 10.2307/1548836
Stoner A. W., Copeman L. A., Ottmar M. L. (2013). Molting, growth, and energetics of newly-settled blue king crab: Effects of temperature and comparisons with red king crab. J. Exp. Mar. Biol. Ecol. 442, 10–21. doi: 10.1016/j.jembe.2013.02.002
Stoner A. W., Ottmar M. L., Copeman L. A. (2010). Temperature effects on the molting, growth, and lipid composition of newly-settled red king crab. J. Exp. Mar. Biol. Ecol. 393, 138–147. doi: 10.1016/j.jembe.2010.07.011
Stroud A. M. (2022). Size at maturity, reproductive cycle and fecundity of the southern California brown box crab, Lopholithodes foraminatus, and implications for developing a new targeted fishery. UC Santa Barbara. ProQuest ID: Stroud_ucsb_0035N_15412. Merritt ID: ark:/13030/m5kq5464. Available at: https://escholarship.org/uc/item/4w95d0r0.
Sungail J., Brown A. C., Alpert K., Maurukas J. (2013). Prey selection by Gulf of Maine green crabs (Carcinus maenas), rock crabs (Cancer irroratus) and American lobsters (Homarus americanus): A laboratory study. J. Exp. Mar. Biol. Ecol. 449, 294–303. doi: 10.1016/j.jembe.2013.09.021
Swiney K. M., Long W. C., Foy R. J. (2017). Decreased pH and increased temperatures affect young-of-the-year red king crab (Paralithodes camtschaticus). ICES J. Mar. Sci. 74, 1191–1200. doi: 10.1093/icesjms/fsw251
Taylor G. M. (2000). Maximum force production: why are crabs so strong? Proc. R. Soc B Biol. Sci. 267, 1475–1480. doi: 10.1098/rspb.2000.1167
Vermeij G. J. (1977). Patterns in crab claw size: the geography of crushing. Syst. Biol. 26, 138–151. doi: 10.1093/sysbio/26.2.138
Vianna B., da S., Miyai C. A., Augusto A., Costa T. M. (2020). Effects of temperature increase on the physiology and behavior of fiddler crabs. Physiol. Behav. 215, 112765. doi: 10.1016/j.physbeh.2019.112765
Volkoff H., Rønnestad I. (2020). Effects of temperature on feeding and digestive processes in fish. Temperature 7, 307–320. doi: 10.1080/23328940.2020.1765950
Wernberg T. (2021). “Marine Heatwave Drives Collapse of Kelp Forests in Western Australia,” in Ecosystem Collapse and Climate Change. Eds. Canadell J. G., Jackson R. B. (Cham: Springer International Publishing), 325–343.
White W. B., Lean J., Cayan D. R., Dettinger M. D. (1997). Response of global upper ocean temperature to changing solar irradiance. J. Geophys. Res. Oceans 102, 3255–3266. doi: 10.1029/96JC03549
Wójcik D., Normant M., Dmochowska B., Fowler A. (2015). Impact of Chinese mitten crab Eriocheir sinensis on blue mussel Mytilus edulis trossulus – laboratory studies of claw strength, handling behavior, consumption rate, and size selective predation. Oceanologia 57, 263–270. doi: 10.1016/j.oceano.2015.03.003
Wright A., Fan Y., Baker G. (2018). Nutritional value and food safety of bivalve molluscan shellfish. J. Shellfish Res. 37, 695–708. doi: 10.2983/035.037.0403
Wu F., Wang T., Cui S., Xie Z., Dupont S., Zeng J., et al. (2017). Effects of seawater pH and temperature on foraging behavior of the Japanese stone crab Charybdis japonica. Mar. pollut. Bull. 120, 99–108. doi: 10.1016/j.marpolbul.2017.04.053
Yuan Q., Wang Q., Zhang T., Li Z., Liu J. (2017). Effects of water temperature on growth, feeding and molting of juvenile Chinese mitten crab Eriocheir sinensis. Aquaculture 468, 169–174. doi: 10.1016/j.aquaculture.2016.10.007
Keywords: crustacea, ecophysiology, feeding ecology, fishery, ocean warming
Citation: Moretto WI and Taylor JRA (2023) Temperature-dependent feeding behavior in the brown box crab, Lopholithodes foraminatus. Front. Mar. Sci. 10:1241950. doi: 10.3389/fmars.2023.1241950
Received: 17 June 2023; Accepted: 16 August 2023;
Published: 08 September 2023.
Edited by:
Mohamad Nor Azra, Universiti Malaysia Terengganu, MalaysiaReviewed by:
Folco Giomi, Independent Researcher, Padova, ItalyMarkus Frederich, University of New England, United States
Copyright © 2023 Moretto and Taylor. This is an open-access article distributed under the terms of the Creative Commons Attribution License (CC BY). The use, distribution or reproduction in other forums is permitted, provided the original author(s) and the copyright owner(s) are credited and that the original publication in this journal is cited, in accordance with accepted academic practice. No use, distribution or reproduction is permitted which does not comply with these terms.
*Correspondence: Wave I. Moretto, d21vcmV0dG9AdWNzZC5lZHU=