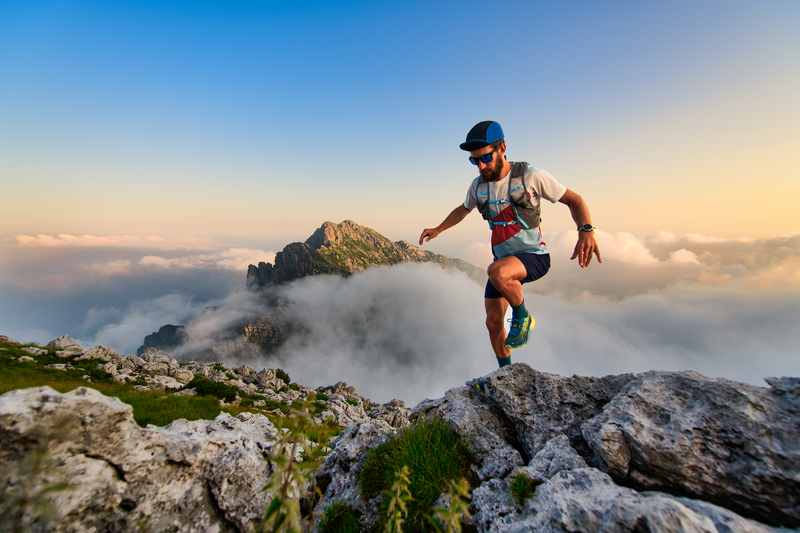
95% of researchers rate our articles as excellent or good
Learn more about the work of our research integrity team to safeguard the quality of each article we publish.
Find out more
ORIGINAL RESEARCH article
Front. Mar. Sci. , 17 August 2023
Sec. Marine Biology
Volume 10 - 2023 | https://doi.org/10.3389/fmars.2023.1240599
Introduction: The common periwinkle Littorina littorea is a delicacy in southern Europe. Enabling aquaculture production would be measure to avoid future over-harvesting and ensure a constant market supply.
Methods: However, knowledge gaps in reproductive biology needs to be filled to allow setting up rearing protocols.
Results: We investigated effects of temperature and salinity on embryonic development by incubating eggs at ten different temperatures in steps every 2°C, form 5 to 23°C) and four salinities (20 – 40). Embryonic development was clearly temperature dependent; eggs hatched after three days at the highest temperature while it took 16 days to hatch at the lowest temperature. Calculating effective day-degrees (DDeff) allowed expressing the thermal history of the embryogenesis and concluding that this species hatches on average at 61 (SD 3.7) DDeff. Larval growth- and developmental rates showed a different response to the temperature extremes as snails with the smallest shell lengths at hatch were found for the highest and lowest temperatures (204.5, SD = 11.6, and 202.9µm, SD = 13.7 respectively). Viable larvae only hatched at temperatures above 9°C. Larvae hatched at lower temperatures did not develop a shell or swimming lobes. Salinity and temperature significantly influenced time-to-hatch and hatching success; the lowest salinity (20PSU) lead to later hatching (80-100DDeff) and lower hatching success compared to higher salinities (25, 30, and 40, hatch around 40-50 DDeff). Neither hatch rate nor time-to-hatch showed differences among the higher salinities, suggesting a salinity threshold below 25. There was no significant interaction between salinity and temperature on time-to-hatch or hatching success. Larval diet had a significant effect on growth rates during the planktonic stage. Veliger larvae fed on two different live microalgae mono-diets (Dunaliella tertiolecta and Rhodomonas baltica) successfully passed their pelagic phase at the same time with no differences in growth rates, while veliger larvae fed on either of two preserved microalgae products (Nannochloropis sp. and Tetrasemis sp. frozen pastes) were not growing.
Discussion: We conclude that embryonal development can be successfully completed at temperatures between 7 and 20°C, while lower or higher temperatures should be avoided. Successfully hatched larvae can be fed on mono-microalgae diets until settlement.
The common periwinkle Littorina littorea (Linnaeus 1758) is a littoral snail species commonly found on rocky shores in temperate marine systems of the northern hemisphere. Due to the dynamic nature of the littoral habitat, this species is considered being well adapted to fluctuations in salinity and temperatures (Petraitis, 2002), and is said to actively search for favourable temperatures (Miller and Denny, 2011).
The common periwinkle Littorina littorea (Linnaeus 1758) is considered a delicacy in southern Europe and it is harvested in huge numbers (Cummins et al., 2002). Catches fluctuate widely between years within countries, but landings were stable around 40.000 to 60.000 tonnes per year from 1960 to the early 2000’s. Since then, only about 15% of these periwinkle catches were harvested (1.000 to 5.000 t) (FAO, 2022). The decrease was mainly because of lower reported landings in the U.K and Ireland.
Periwinkles play an important role in littoral habitats, and are often the major grazer consuming microphytobenthos (Watson and Norton, 1985). As herbivores, periwinkles graze on newly settled macrophytes. A preference for green and red algae for instance can facilitate brown algae recruitment (Johnson and Mcdermott, 2018) and can control seaweed and sea grass recruitment (Eschweiler et al., 2009). This grazing behaviour reduces algal cover and frees up space for colonialization by other species, hence periwinkles also play a role in altering species succession in hard substratum ecosystems (Lubchenco, 1983). They further break down larger pieces of seaweeds, hence make it available as food for smaller organisms, increase nutrient release, and surface area for microbial colonialization, contributing to carbon turnover (Jaschinski and Sommer, 2010).
Despite the ecological importance of this species, little focus have been put on its conservation and stock monitoring (Johnson and Mcdermott, 2018). As Littorina species have a long lifespan, and increased fertility with increased age (Hohenlohe, 2002), the current harvesting with focus on larger individuals makes the species more prone to over-exploitation and possibly a fishery-induced population imbalance towards comprising smaller individuals over time (Doyle et al., 2022). The periwinkle lives in the marine tidal zones along the coast, which is a very accessible habitat for collection, making harvesting of the species relatively easy. To relieve pressure on the wild stocks and create a long-term sustainable industry, it is important to investigate the aquaculture potential of the species. While some information is available regarding the diet preference, habitat and growth in juvenile and adult L. littorea, there is still lack of knowledge surrounding the reproduction and early life stages. To develop large-scale aquaculture production of L. littorea, it is necessary to gain knowledge on how environmental parameters affect egg incubation, the effects of temperature and salinity on embryonic development, and to study larval nutrition and cues that induces settlement and metamorphosis to produce production protocols, all of which are common bottlenecks in marine aquaculture.
Littoral species need a higher tolerance for changes in environmental conditions than more deep water species (Zhang et al., 2014). L. littorea is a hyper-hypo osmoregulator (Podbielski et al., 2022), with the lower tipping point being around a salinity of 14. The tipping point in that publication is defined as the salinity at which growth equals zero, as the costs for osmoregulation are so high that no growth is possible anymore. While adult animals can protect against salinity stress through behavioural responses like shell closure or emersion (Taylor and Andrews, 1988) egg and larval stages are bound to the aquatic environment. Early life stages are often more prone to stressors, such as salinity or temperature (Anger, 1996), hence we can expect an increased sensitivity to e.g. salinity in L. littorea embryos compared to older snails with a higher osmotic capacity and better developed avoindance mechanisms. Global change induced increased runoffs from freshwater could impact the recruiting of the species (Génio et al., 2008).
Development is temperature dependent in poikilothermic animals, as biochemical processes are temperature sensitive. All enzymatic processes, such as protein biosynthesis are temperature dependent (Clarke, 2006). Hence, time as such is a poor predictor for development in poikilothermic organisms, as temperature plays such a major role. The integration of temperature can be done using a degree-day approach, which multiplies the animals body temperature by the time the animal experienced this temperature. Day-degrees are a better indication for development in poikilotherm organisms than time, as it integrates the temperature into the development, leading to a measure of temperature life history (Malzahn et al., 2003; Neuheimer and Taggart, 2007). Plotting day-degrees versus incubation temperature should lead to a straight line with no significant slope if this approach would completely integrate the temperature influence on development (Kamler, 2002). However, within a biologically sound temperature range, especially at lower temperatures, the deviation from such an ideal line can be considerable. The reason is that below a biological temperature threshold no development takes place (Atkinson, 1996). This temperature is called t0 and is species specific, hence t0 needs to be established for each species or population (Honsey et al., 2023). This biological t0 can then be subtracted from the incubation temperature leading to the effective temperature the organisms experienced for growth or development to calculate the effective day-degrees, which drastically reduces the residual variation in a model (Kamler, 2002).
Temperature influences growth and development in various ways. As a general rule, an organism’s size tends to decrease with increasing temperature (Atkinson, 1994). This phenomenon has been linked to fundamental metabolic rates (Brown et al., 2004). However, growth and development might not necessarily be coupled processes, and in fact they have been shown to respond different to temperature (Forster et al., 2011). An explanation for de-coupling of growth and developmental rates is the difference in activation energy required for the processes driving growth (protein biosynthesis) and development (cell division), which means that organisms reared at a range of temperatures reach the same developmental stage at a smaller size at higher temperatures (Van Der Have and De Jong, 1996).
Due to the impact salinity as well as temperature have on biological processes, and the development and wellbeing of early life stages in particular, we can expect these two stressors to interact. We further predict these interactions to be most severe at the extremes, for example the lower salinity tolerance at a salinity of 14 (Podbielski et al., 2022) being shifted upwards when extremely low or high temperature adds stress. Such interactions have been described in fish many times (Åsheim et al., 2022), however, it has to be noted that the nature of salinity-temperature interactions on osmotic stress is often owed to comparisons between species of populations.
Once the larvae hatches, they soon rely on external nourishment once the yolk reserves are depleted. L. littorea produces pelagic, planktivorous veliger larvae, which in nature probably feeds on a large variety of microalgae, mixotrophs and small heterotrophs. From an aquaculture perspective, supplying a large variety of different microalgae species at this stage is not feasible, as it is cost- and labour intense, and prone to failures (Robert and Trintignac, 1997). Typically, mollusc aquaculture avoids producing live algae and strives to use substitutes such as algal pastes, concentrates or frozen products, despite that such products are usually outperformed by live microalgae (Sales et al., 2022). The reasons for this are manifold and can be related to e.g. loss of nutrients or the loss of cell integrity caused by preservation (Muller-Feuga, 2000). Microalgae differ vastly in size, shape, nutrient content, biochemical composition, and digestibility. Only a few species fulfil the requirements of allowing for an easy and stable production whilst having a suitable size and adequate nutritional profiles (Brown, 2002), and not much has changed in the produced species portfolio during the last 40 years. Not all of the microalgae species used in aquaculture are suitable for all farmed species, e.g. Dunaliella tertiolecta is infamous for its fatty acid profiles, lacking the essential long chained polyunsaturated fatty acid docosahexaenoic acid (22:6n-3; DHA), but is favourable because of its carotenoid concentrations. Despite the shortage of DHA, D. tertiolecta was successfully used as a diet for rearing veliger larvae of the queen conch (Strombus gigas). Rhodomonas species, however, are known for their well-balanced fatty acid profiles and have been used in mollusc aquaculture numerous times (Aldana-Aranda and Patino Suarez, 1998; Gagné et al., 2010). Consequently, it is simply not enough to look at the biochemical composition of algae to assess its suitability as food for veliger larvae, as other factors such as digestibility, size and macro- and micronutrients might also play a vital role in this developmental stage. Additionally, successful aquaculture production of L. littorea will depend on identification of the environmental cues necessary to induce settlement and metamorphosis of the larvae. These cues are currently completely unknown for L. littorea, but a universal mechanism depending on the presence of a favourable biofilm has been suggested for marine larvae (Hadfield, 2011; Freckelton et al., 2017; Wichard and Beemelmanns, 2018).
To investigate the effect of temperature and salinity on L. littorea embryonic growth and development until settlement, we incubated eggs at a range of temperatures and salinities and monitored the timing of hatch and hatching success. To evaluate the effect of different diets we chose two live microalgae species, differing in their nutritional value; Dunaliella tertiolecta as the less favourable species and Rhodomonas baltica as a nutritious microalga, as well as two preserved commercial algae products; concentrated Nannochloropis sp. and a mix of Nannochloropis sp. and Tetrasemis sp., both representing an easy-to-use, off-the-shelf solution.
To investigate the effects of temperature, salinity and feed type on egg and larval stages of L. littorea we conducted four experiments. A graphical summary of the four experiments is given in Figure 1.
Figure 1 Graphical summary of the four experiments conducted on the different life stages of L. littorea.
Adult specimens of L. littorea were collected from the intertidal zone around Oksvoll (Ørland Municipality, Trøndelag county, Norway) during early May 2020 and transferred to flow-through holding tanks (52 × 36 × 18 cm L x W x H) at SINTEF SeaLab (Trondheim, Norway) kept at a 16L:8D light regime and 10°C. The seawater used for broodstock husbandry and the experiments in our study was collected from the Trondheim fjord at 70 meters depth, and was sand filtered (20 µm), protein skimmed and bag-filtered (1 µm) before use. The salinity of the water was between 33 and 34 PSU. The size of the collected snails ranged between 25 - 34 mm shell height, however, a narrower size range (26 – 31 mm shell height) was selected and separated from the rest to be used in all subsequent experiments to avoid the influence of female size (Chaparro et al., 2019). The snails were fed a mix of sugar kelp (Saccharina latissima), Ascophyllum nodosum and sea lettuce (Ulva sp.) for the full duration of the experimental period which lasted from May to August 2020.
Newly spawned eggs were retrieved from a broodstock of adult L. littorea (n=30 – 50) kept in a 10-L bucket filled with filtered seawater (33 PSU) at 10°C. The eggs were distributed into 10 glass beakers (750 mL) at a density of 10-15 eggs mL-1 and exposed to ten different temperatures in a temperature gradient apparatus (modified after Thomas et al., 1963). The initial temperatures spanned from 7.7 to 15.4°C, which were then gradually adjusted to the target temperatures of the experiment over 24 hours to allow the eggs to acclimatize. The final temperatures were 5, 7, 9, 11, 14, 16, 17, 19, 21 and 23°C, kept constant throughout the experiment which lasted 17 days for the coldest temperature. The eggs were sampled every 15 day-degree (hereafter referred to as “DD”) by gently stirring the water in the beakers with a spoon to resuspend the sedimented eggs before removing 2 mL of water from the centre of the beaker.
We calculated DD by multiplying the duration until 50% hatch by the incubation temperature. We then calculated effective degree days using linear regression analysis of developmental rate (the reciprocate of time-to-hatch) versus temperature. We used the slope and the y-intercept of the linear regression to calculate t0 (temperature at which no development occurs) (Dettlaff, 1986; Dettlaff, 2003). Subtracting t0 from t gave the effective temperature of the incubation period. Multiplying the effective temperature by the duration to 50% hatch then leads to “effective day-degrees” (hereafter referred to as “DDeff”).
Adult snails were incubated in the temperature gradient apparatus in parallel to the experiment described above. Six egg laying snails were incubated for 24 hours in triplicate beakers at each of the aforementioned temperatures. After 24 hours, adult snails were removed, and the presence of eggs was visually confirmed in each beaker before proceeding. The larvae were sampled after 110 DD and fixed in 4% paraformaldehyde (PFA) in phosphate buffer.
To measure shell length of the larvae 110 DD post-fertilisation, the larvae were rinsed in phosphate buffer using a sieve (64 μm, Sefar NITEX®, Sefar AG, Switzerland) to remove the fixative. Rinsed larvae were transferred into a petri dish filled with phosphate buffer and photographed using a camera equipped stereo microscope (Leica M205C). Finally, 30 images of different larvae per replicate were measured using the software ImageJ (Rasband, 1997–2012.).
A salinity and temperature gradient was established to investigate the effect of salinity and temperature on development- and hatching rates. We created a five-step salinity gradient (20, 25, 30, 35 and 40 PSU) using sea salt from evaporated seawater, and distilled water. The water mixtures were aerated to ensure sufficient oxygen levels (>100% dissolved oxygen; DO) before being added to transparent cell culture flasks (50 mL, Greiner Bio-One, CELLSTAR® TC).
Five cell culture flasks were filled for each salinity. Newly spawned eggs were retrieved from the broodstock, and approximately 50 eggs were added into each culture flask to yield a concentration of 1 egg mL-1. One flask from each salinity was then incubated at each of the five different temperatures (7.5, 11.0, 15.1, 18.3 and 22.0°C) in the temperature gradient table. The flasks were monitored daily, and hatched larvae were removed and counted. The experiment was terminated at 110 DD when most of the eggs were expected to have hatched. The remaining, un-hatched eggs were counted and fixated in 4% PFA.
We chose the gradient in both, temperature and salinity rather wide to ensure we also establish boundaries in which the organisms develops and functions normal, even though this species will experience these extremes rarely.
To investigate the effect of different microalgae diets on larval growth and survival, we incubated eggs at 16°C and a salinity of 34 PSU for 96 DD in 24-h light. A small sample was removed to visually confirm hatch, and the larvae were distributed amongst transparent cell culture flasks (n=24, V=50 mL, Greiner bio-one, CellStar® TC, model 690160) at a concentration of approximately 10 larvae mL-1. The flasks were thereafter randomly assigned to the five treatments (four diets plus one starvation control) with five replicates for each treatment. The seawater used for the larval feeding experiment was collected from the Trondheim fjord at 70 meters depth, and was sand filtered (20 µm), protein skimmed and bag-filtered (1 µm) before use. The salinity of the water was between 33 and 34 PSU.
Larvae were fed with fresh and live Rhodomonas baltica or Dunaliella tertiolecta, or with either of two processed diets; Nannochloropsis Frozen Paste (BlueBioTech Gmbh, Germany) or Rotifer Diet (Instant Algae®, Reed Mariculture, USA, a paste consisting of Nannochloropsis sp. and Tetraselmis sp.). Live, semi-continuously cultured and exponentially growing microalgae were acquired from glass bottles (20 L, 34 PSU, 20 – 22°C) that were continuously illuminated (250 µmol m-2 s-1) and aerated with atmospheric air added with 1–2% carbon dioxide (CO2). The seawater was chlorinated and dechlorinated according to Hoff and Snell (2008), and Conwy growth medium (Walne, 1970) was added (1 mL L−1 seawater), before use. Daily feeding was initially set to yield a final concentration of 100 000 cells mL-1 in the culture flasks for the two diets consisting of live microalgae. For the pastes, which consisted of smaller-celled species, the feed concentrations were doubled (200 000 cells mL-1). To ensure stable feed densities and water quality on a day-to-day basis, the whole water volume was exchanged daily by sieving the larvae over a 64 µm sieve and then flush them into clean flasks. Feeding density was gradually decreased, as we recognised that the algal concentrations were too high, and aggregates formed which might have captured the larvae. Final feeding densities were 30 000 cells mL-1.
The flasks were distributed into two ICES incubators (HYDROBIOS, Kiel, Germany) set at 18 ± 0.5°C and 24-h light. The devices allowed the flasks to continuously rotate at approximately 5-6 rounds per minute (rpm) to keep both the larvae and the microalgae in suspension. Five larvae from each flask were removed, photographed, and fixated every fourth day. The experiment lasted until the larvae were ready to settle, which in our trial was defined as the loss of the velum. Shell length was measured on photographed larvae using a camera equipped stereo microscope (Leica M205C). Five larvae per flask for each sampling were measured using the software ImageJ (Rasband, 1997–2012.). The remaining larvae at the end of the experiment were counted for survival estimation.
The decrease in time until 50% hatch followed an exponential model, hence data were linearized by ln-transformation and tested for linearity by linear regression analysis. To test a potential residual relationship between DD and DDeff until 50% hatch and the incubation temperature we used linear regression analysis.
The effect of temperature on size at hatch was tested using a one-way-ANOVA, followed by the Holm-Sidak post-hoc test.
The effect of temperature and salinity on time to first hatch and cumulative hatching success was investigated by two-way-Anova, salinity and temperature being the factors and time to hatch, or cumulative hatch at 110 DD, being the dependent variable. In case of significant results, a Holm-Sidak post-hoc test was used to further explore differences.
To compare larval growth in relation to diet from the feeding trial, we first compared larval length at day 9 post-hatch (last day where we had larvae in all treatments) by means of ANOVA. The data failed the homogeneity of variance assumption (Brown-Forsythe test), hence a Kruskal-Wallace one way Anova on ranks was initiated. Differences between treatments were assessed the by Dunns Multiple Comparison method.
To analyse potential differences in growth trajectory until settlement of larvae receiving live algae, length was linearized by calculating the natural logarithm. Linearized data was then used as the dependent variable in a one-way ANCOVA, with diet type as the factor and age as the co-variate.
Statistical differences were accepted as significant with p < 0.05 for all statistical tests.
Temperature had a significant effect on the development of early life stages of L. littorea. The time to 50% hatch of L. littorea significantly decreased with increasing temperature (Figure 2). The decrease in time till 50% hatch followed an exponential model, hence data linearized by ln-transformation and tested for linearity by linear regression analysis (r=0.97, temperature= -10.35 days to hatch + 31.18, slope significant from zero, p<0.001). While it took 16 days from fertilisation to hatch at 4.8°C, the incubation period decreased to about three days at 23°C. Incorporating thermal history into time to hatch should theoretically incorporate all temperature effects on development and should leave no differences in the thermal integral until hatch between temperatures. Calculating DD (incubation temperature * days to hatch) revealed a less pronounced temperature dependence (slope= -0.47), with higher DD to hatch at lower temperatures (linear regression analysis, p=0.06, r=0.60). Incorporating t0 into the calculations of DD, the negative relationship between temperature and DD to 50% hatch diminishes even more, as the influence of t0 is strongest at low temperatures, reducing the slope to 0.03. There was no significant relationship between temperature and DDeff. This allowed for calculating a common thermal integral to hatch across temperatures. The average 50% hatch was reached at DDeff= 61 ± 3.7 SD).
Figure 2 Relationship between incubation temperature and developmental time expressed as: effective day-degrees (red circles), day-degrees (black circles) and days until 50% hatch (dark red triangles) of Littorina littorea larvae.
The incubation temperature had a pronounced effect on the size of the snail larvae at 110 DD (one-way-ANOVA, F 7, 681 = 35.15, p<0.01). The two lowest incubation temperatures led to no viable hatch, with less developed, abnormal larvae (Figure 3A). The larvae incubated at 5 °C had an abnormal body shape, being very round like a trochophore larvae, without clearly differentiated swimming lobes. They had cilia, but lacked a shell, and internal organs appeared to be relatively unprotected. The larvae hatching in 7 °C were somewhat more developed, with developed swimming lobes and a more oblong body, covered by a fragmentary larval shell they were not able to retract into (Figure 3B). Eggs incubated at temperatures between 9.1 and 23°C developed into normal, viable veliger larvae at hatching. They had a fully developed shell, which they were able to retract completely into (Figure 3C). The shells were transparent and the eyespots and cilia were present and clearly visible through the shells. Shell lengths showed a bell-shaped distribution with smaller larvae being produced at the highest (mean size 204 µm at 22.8°C) and lowest (mean size 202 µm at 9°C) temperatures, whilst the largest larvae were found in the temperature range 14 – 17°C (mean sizes 218 - 224 µm) (Figure 4).
Figure 3 Exemplary images of Littorina littorea larvae hatched from eggs incubated at (A) 4.8°C, (B) 6.9°C, and (C) 11.3°C highlighting typical abnormal development, such as the lack (A), or an incomplete developed shell (B). C, Cilia; E, Eye; S, Shell; SO, Shell opening.
Figure 4 Littorina littorea veliger larvae shell length at 110 day-degrees after fertilization. Letters denote statistically significant different groups. Box: 25th percentile, median, 75th percentile. Whiskers= 90th and 10th percentiles, circles are outliers. *, no viable hatch.
Embryos of L. littorea successfully developed into viable larvae and hatched at all temperature-salinity combinations. The incubation time to hatching of L. littorea larvae was clearly temperature influenced; higher temperatures lead to an earlier hatch (Figure 5). Calculating DDeff using t0 established in the previous experiment removed the significant influence of temperature on duration to hatch and there was no significant interaction between salinity and temperature on time until hatch. (two-way ANOVA, summary statistics in Table 1). Salinity significantly affected the thermal integral of incubation time to hatch. A salinity of 20 PSU resulted in a significantly later hatching (89.9 DDeff SD = 13.5) than at the other salinities (59.5 DDeff, SD = 8.9), which did not differ from each other (two-way ANOVA, p<0.05, Holm-Sidak post-hoc test) (Figure 6). In summary, all temperature/salinity combinations from a salinity of 25 and a temperature of 9°C result in a similar timing of hatching.
Figure 5 Relationship between temperature, salinity and duration to first hatch of larval Littorina littorea. The colours represent the time in days to first hatch, blue: early hatch, red: delayed hatch.
Table 1 Summary of 2 way-ANOVA testing the effect of temperature (T) and salinity (S) on the effective thermal integral (DDeff) until hatch.
Figure 6 Relationship between temperature, salinity and duration to first hatch of larval Littorina littorea. The colours represent the effective day-degrees (DDeff) at first hatch, blue: early hatch, red: delayed hatch.
The cumulative hatching success at 110 DD was not affected by temperature, while salinity had a significant effect on hatching success. However, no significant interactions between temperature and salinity were found (two-way ANOVA, summary statistics in Table 2). The lowest salinity tested (20 PSU) resulted in significantly lower hatching success than higher salinities (30 and 40 PSU), whereas the other combinations were not different from each other (Holm-Sidak post-hoc test p<0.05). However, combining the effect of salinity and temperature on hatching success suggests a promising combination of a salinity of 25 and an incubation temperature of 15°C, while the effect of temperature dwindles at a salinity of 30 or more (Figure 7).
Table 2 Summary of 2 way-ANOVA testing the effect of temperature (T) and salinity (T) on the cumulative hatching success at 110 DD as a common thermal integral.
Figure 7 Relationship between temperature, salinity and the percentage of hatched larval Littorina littorea at 110 day-degrees. The colours represent hatch at 110 day-degrees, blue: low hatching success, red: high hatching success.
Survival: There was a clear separation in survival of larvae receiving live algae (R. baltica or D. tertiolecta) from those receiving processed microalgae pastes (Nannochloropsis sp. or Rotifer Diet) and the starvation control. No larvae in the starvation control survived beyond day 9 post hatch, and the same was also found for the larvae receiving processed microalgae, suggesting the larvae could not utilize processed microalgae as feed (Figure 8). Larvae fed either of the two live microalgae survived until settlement at day 22 post-hatch, however, showed low survival rates of 3.4 and 1.8% for larvae fed D. tertiolecta and R. baltica, respectively.
Figure 8 Growth of shell length of larval Littorina littorea fed different diets until larvae were ready for settlement. * denotes significant differences between larvae fed Dunaliella spec or Rhodomonas baltica and the other three treatments.
Growth: The pattern of a clear separation between larvae fed live or processed microalgae was also mirrored in larval growth. At day 9 post-hatch, which was the last sampling with larvae in all treatments, length of larvae fed the two processed algae pastes did not differ from the non-fed larvae (Dunns Multiple Comparison p< 0.05). Larvae fed live algae were significantly larger than the ones receiving processed algae, and there was no difference between larval size among those larvae receiving live algae (R. baltica and D. tertiolecta) (Dunns Multiple Comparison, p>0.05). On day 9, starved larvae or larvae fed processed algae were on average 192.4µm (SD = 11.7), while larvae fed live micro algae were on average 279.1µm (SD = 30.5)
Comparing the size of the two groups surviving until settlement at day 22 post-hatch revealed no significant difference (t-test, t(85)= -3.02, p= 0.002), with larvae receiving R. baltica being 350.2 µm on average, while those receiving D. tertiolecta reached 346.1µm on average (SD = 25.3 and 28.1).
While the size at settlement was affected by diet type, the growth trajectory until settlement for the two treatments receiving fresh microalgae was not affected by diet type (ANCOVA, p>0.05), and larvae receiving either of the live algae resorbed their vela at the same time (22 dph, Figures 8, 9).
Figure 9 Photographs of Littorina littorea veliger larvae at different ages fed different diets. Larvae fed preserved microalgae (Nannochloropsis sp. and Rotifer Diet) and the control group (starvation control) died after 9 days.d.
Temperature had a significant effect on egg incubation duration and embryonic development. Increasing temperatures shortened the time to hatch drastically, which is typical for poikilothermic animals (Wolpert et al., 2015). Similar relationships have previously been reported for gastropod eggs (Zotin and Ef, 2014), and for several fish species (Kupren et al., 2008). In poikilothermic organisms, early life chronological age is irrelevant as a measure to which developmental processes can be associated. Because of the influence on developmental rates temperature has, it must be integrated to allow for meaningful predictions and comparisons within and between studies. Several approaches have been put forward to incorporate thermal history into development, which all lead nearer to the core, but also have disadvantages, such as non-linear models using constants with no biological meaning. Calculating t0 and using this measure to calculate DDeff allowed us to incorporate the effect of temperature into time-to-hatch, which enabled us to calculate a thermal integral from fertilisation to hatch for a specific population of L. littorea. This value can now be used for comparisons with other L. littorea populations from different areas to investigate adaptations across the vast geographical areas this species occurs. Mccoy et al. (2020) investigated L. littorea egg development under hypoxia but reported that the control group (normoxia at 14°C) reached 50% hatch after 8.8 days. Using the t0 established it his study, this would relate to 65 DDeff, thus reasonably matching the average calculated for the current experiment (60.7 DDeff).
The observation that growth and development might be biologically de-coupled processes dates back more than half a century, when Von Bertalanffy (1960) stated that the reason might be that anabolic processes scale linearly with temperature, while catabolic processes scale in the form of a power function to temperature. This would lead to a hump-shaped relationship between growth rate and temperature. Several other hypotheses have been formulated to explain relationships such as size dependency on temperature. Amongst them is the ‘temperature-size-rule’ (Atkinson, 1994), which is based on observations such as published by Van Der Have and De Jong (1996), who reported that Drosophila melanogaster mass and wing size decreases with increasing temperature. The authors supply a mechanistic explanation for the general decrease in size with temperature, arguing that the activation energy for DNA replication (as a measure for development) is higher than the one for protein biosynthesis (representative for growth) resulting in smaller cells at higher temperatures. An increase in in size with decreasing temperatures has also been shown for the marine calanoid copepod Acartia tonsa by Leandro et al. (2006). The authors found that weight-specific growth rates increased exponentially with temperature, an observation which has also been shown for other calanoid copepod species (Mauchline, 1998). Temperature also influences reproductive output in A. tonsa which lay eggs of decreasing size with increasing temperatures, whereof low temperatures negatively affect hatching success and -rates (Hansen et al., 2010). The theoretical models put forward by Von Bertalanffy (1960), or Atkinson (1994) or Forster and Hirst (2012) have in common that they predict organisms’ size being smaller towards the upper tolerable temperatures, but also, often neglected, smaller sizes at the lower margin of tolerable temperatures. This matches our observation that the length of the snail larvae showed a hump-shaped relationship to temperature indicating that we covered the full thermal window of L. littorea, including the not so often reported lower margin. Here we have a clear indication that the lowest temperatures for embryonic development in our experiment were below the thermal window of L. littorea, as no viable hatch occurred, and the few larvae that hatched did not develop a shell (at the lowest temperature), or an irregular shaped shell (at the second lowest temperature).
The littoral habitat of L. littorea is characterized by large changes in salinity. While average sea surface salinity rarely drops below 30 PSU during early spring, winds, rain, sun, tidal changes and river runoff can greatly impact temperature and water conditions, and creating large fluctuations in salinity (Richmond and Woodin, 1996). Species living in the littoral zone therefore need a higher tolerance for changes in environmental conditions than more deep water species (Zhang et al., 2014), including the egg and larval stages (Anger, 1996). The sensitivity we found to low salinity in L. littorea embryos indicate that increased freshwater runoff could impact the recruitment of this species (Génio et al., 2008). While adult L. littorea can protect themselves against salinity-induced stress through behavioural responses like shell closure or emersion (Taylor and Andrews, 1988), egg and larval stages are bound to the aquatic environment. While little is known about the buoyancy of L. littorea egg capsules, we observed that the eggs sedimented in stagnant, full-strength seawater, suggesting that egg density might allow them to stay further down in the water column keeping them safe from low-salinity surface waters. The embryos successfully developed and hatched at all salinities, only the lowest salinity resulted in significantly delayed hatch, and significantly lower hatching success, suggesting L. littorea is well adapted to normal environmental fluctuations in their natural habitat.
One could expect interactions between the two tested factors, temperature and salinity. In fish it has been shown that sea trout (Salmo trutta) reduces osmotic stress at low temperatures by migrating from the sea into brackish or freshwater (Thomsen et al., 2007). Contrary, cutthroat trout (Oncorynhus clarki) have less stress at higher salinities when water temperatures are higher than at lower water temperatures. However, in our experiment we did not find significant interactions between temperature and salinity on L. littorea development. This is in line with results presented by Deschaseaux et al. (2010), who found no interaction between salinity and temperature on egg survival and oxidative stress markers in three marine snail species. The authors extended their study beyond hatch and reported significant effects of both, temperature and salinity on growth and biomarkers in larval gastropods, but again, found no significant interactive effects of both factors (Deschaseaux et al., 2011). Bashevkin and Pechenik (2015) investigated salinity and temperature interactions on larval and juvenile growth in the gastropod Crepidula fornicate, and found mixed results in a series of experiments, with around 50% of their analysis showed significant interactions between salinity and temperature, while the other half of their analysis showed no interactions. An important result of this study are the differences in inorganic content, which were lower at low temperatures and low salinities compared to higher temperatures at the same salinity, suggesting a shift in osmolites from inorganic to organic osmolites, as described by Podbielski et al. (2022).
Many aquatic molluscs have planktivorous veliger larvae, which are depending on microalgae as their diet during this crucial phase of their development. Especially farmed molluscs such as several bivalve species are well researched in relation to their nutritional requirements (Laing, 1987; Cheng et al., 2020; Da Costa et al., 2020). Gastropods are less studied, perhaps as they play a minor role in aquaculture. The global gastropod aquaculture production was 580 000 tons in 2020 compared to 1.6 million tons bivalves (FAO, 2022). L. littorea is to date not cultivated and little is known on the nutritional requirements of the veliger larvae. In our feeding trial, we used two live microalgae, and two different preserved products. Veliger larvae fed with both species of live algae (R. baltica and D. tertiolecta) seemingly grew well and reduced their vela towards the end of the trial, suggesting that these two algae species allow for a successful larval development as mono-diets with no differences in growth rates. This is interesting, as D. tertiolecta produces no, or only minute traces, of the two essential fatty acids eicosapentaenoic acid (20:5n-3; EPA) and DHA. Nevejana et al. (2003) for example, demonstrated that veliger larvae of the bivalve Argopecten purpuratus were able to grow when fed D. teriolecta exclusively, but that an addition of DHA and EPA drastically increased growth rates. A successful development of eyes was not present in Argopecten purpuratus veligers reared only on D. tertiolecta (Nevejana et al., 2003), which points towards the immense role DHA plays in eye development of marine larval species, e.g. fish (Bell et al., 1995; Tocher, 2003). Rhodomonas species on the contrary are known to have a very balanced LC-PUFA profile and are of high nutritional quality for planktonic consumers such as copepods (Malzahn et al., 2015) or bivalve veliger larvae (Tremblay et al., 2007). In our experiment, there was no apparent difference between larval growth and development fed the two different diets, suggesting that L. littorea might be able to de novo-synthesise DHA. There is a growing body of literature reporting the ability of invertebrates to elongate and desaturate mono-unsaturated 18C fatty acids (Kabeya et al., 2018; Holm Nielsen et al., 2019; Kabeya et al., 2020), and the larval stage of L. littorea might be added to this list, however further research is needed as assess this mechanism. Moreover, we cannot conclude whether these diets can support larval development throughout settlement and metamorphosis.
The two preserved microalgae diets did not enable growth and development at all. Both pastes are used to feed other aquatic invertebrates such as rotifers, copepods, or bivalves routinely. Potential explanations for this result could be that L. littorea veliger do not ingest or digest these species. The preservation process might eliminate certain ques necessary for the larvae to identify the cells as food. This would have drastic consequences for non-filter feeding organisms. Phytoplankton cells not being ingested or digested by planktivores is a common pattern reported for a whole suite of animals in freshwater and in saltwater environments, and can be related to size and shape (Paul et al., 2021), surface structure and chemistry (Martel, 2009; Meunier et al., 2016) and digestibility (Van Donk et al., 1997; Boersma, 2000).
Lora-Vilchis and Maeda-Martinez (1997) fed scallop (Argopecten ventricosus-circularis) larvae on ten different fresh and alive microalgae species, and only seven were eaten by the larvae. These larvae ingested Tetraselmis suecica and Nannochloropsis oculata but were not able to digest the latter. The pearl oyster Pternia sterna have been shown to feed even more selectively. Martínez-Fernández et al. (2004) offered ten different live species of microalgae to pearl oyster veliger, which ingested only Nannochloris sp., Pavlova lutheri and Isochrysis aff. galbana (T-ISO), of which only P. lutheri and I. galbana were digested. The other seven species were not ingested. To sum up, a correct choice of microalgae species is key to successful larval rearing.
The use of a substitute for live microalgae in larval rearing is tempting, as it reduces work load and eliminates risks related to algae culture crashes (Robert and Trintignac, 1997). Muller-Feuga (2000) stated that no preserved microalgae, neither pastes, dried algae nor frozen products could beat live algae for mollusc larval rearing. This might be a bold statement, but is backed by a more recent review by Sales et al. (2022). The authors evaluated a large body of literature to assess to which degree and for which species algae pastes or concentrates can substituted live microalgae. They reported that amongst the organisms fed on substitutes, bivalves, copepods and shrimps were those with the lowest acceptance rates for concentrates. Contrasting, rotifers and daphnids were more readily accepting and thriving on concentrates as diets. Southgate et al. (2017) for example fed four different algae concentrates to veliger of the bivalve Tridacna noae at different developmental stages. None of the four microalgae species used was ingested by one-day post hatch veliger, whereas at 2- and 3-days post hatch, all four species were ingested to various degrees; however the cells from two of these species were not digested. This shows that ingestion and digestion can differ with both developmental stage and the quality of the food source, hence, timing for introduction and food type should consequently be carefully considered when developing hatchery protocols for gastropod veliger.
While the successful rearing of L. littorea larvae until settlement is a huge step forward, research is still needed focussing on settlement, metamorphosis and early juvenile phases, which are regarded to be the real bottlenecks (Jablonski and Lutz, 1983; Hohenlohe, 2002; Castelo Branco et al., 2014). While the mechanism behind the settlement process is largely unknown, larvae have been described as reluctant to settle until presented with subtidal rocks and associated biota (Strathmann and Strathmann, 2007). There is an increasing amount of research pointing towards favourable biofilms as being the main cue necessary for inducing settlement and successfully complete metamorphosis in a large range of marine larvae, suggesting that there might be a universal mechanism behind this process (Hadfield, 2011; Freckelton et al., 2017; Wichard and Beemelmanns, 2018). Settlement-signals in biofilms are normally produced by bacteria, but diatoms have also been reported to induce settlement in marine invertebrate larvae (Le Tourneux and Bourget, 1988; Harder et al., 2002). Investigating the coherence of early nutrition and substrate types for inducing settlement and metamorphosis should be a focus of future research following this study. This is the final step towards closing the life cycle of this species which is a prerequisite for enabling aquaculture of L. littorea.
The development of L. littorea embryos is temperature related, and by calculating the effective thermal life history, time to hatch can be predicted for incubation temperatures between 5 and 23°C. The time to first hatch and hatching success was most favourable at salinities at or above 25 PSU, and at temperatures between 12 and 18°C. Veliger larvae can successfully be reared until settlement on mono-algae diets of Rhodomonas baltica and Dunaliella tertiolecta, but did not grow on commercial diets of frozen Nannochloropsis sp. and Tetraselmis sp. Whether this result was caused by microalgal species or because of the preservation process is unknown.
The raw data supporting the conclusions of this article will be made available by the authors, without undue reservation.
The manuscript presents research on animals that do not require ethical approval for their study.
TL: formal analysis, investigation, writing – original draft. AM: conceptualization, Investigation, writing – original draft, writing – review & editing, data analyses, visualization. EK: writing – original draft, writing – review & editing, AH: conceptualization, writing – original draft, writing – review & editing, funding acquisition, project administration. TL and AM contributed equally to this manuscript. All authors contributed to the article and approved the submitted version.
This work was conducted in the project “STRANDSNEGL” funded by Regionalt Forskningsfond Trøndelag (grant no. #299075), Statsnail AS and Norwegian Fishfarming Technologies AS.
The laboratory work was carried out within the framework of the national research infrastructure “Norwegian Center for Plankton Technology (#245937/F50)”. The authors thank Marianne Nymark at SINTEF Ocean for proofreading an early version of the manuscript. This work is based on the MSc thesis of Tora Lillebjerka, Faculty of Natural Sciences, Norwegian University of Science and Technology.
The authors declare that the research was conducted in the absence of any commercial or financial relationships that could be construed as a potential conflict of interest.
All claims expressed in this article are solely those of the authors and do not necessarily represent those of their affiliated organizations, or those of the publisher, the editors and the reviewers. Any product that may be evaluated in this article, or claim that may be made by its manufacturer, is not guaranteed or endorsed by the publisher.
Aldana-Aranda D., Patino Suarez V. (1998). Overview of diets used in larviculture of three Caribbean conchs: Queen conch Strombus gigas, milk conch Strombus costatus and fighting conch Strombus pugilis. Aquaculture 167, 163–178. doi: 10.1016/s0044-8486(98)00304-4
Anger K. (1996). Salinity tolerance of the larvae and first juveniles of a semiterrestrial grapsid crab, Armases miersii (Rathbun). J. Exp. Mar. Biol. Ecol. 202, 205–223. doi: 10.1016/0022-0981(96)00022-6
Åsheim E. R., Andreassen A. H., Morgan R., Silvestre M., Jutfelt F. (2022). Water salinity does not affect acute thermal tolerance (CTmax) in zebrafish Danio rerio. bioRxiv. 12. doi: 10.1101/2022.08.02.502531
Atkinson D. (1994). Temperature and organism size - A biological law for ectotherms? Adv. Ecol. Res. 25, 1–54. doi: 10.1016/S0065-2504(08)60212-3
Atkinson D. (1996). “Ectotherm life-history responses to developmental temperature,” in Animals and temperature: phenotypic and evolutionary adaptation. Eds. Bennett A. F., Johnston I. A. (Cambridge: Cambridge University Press), 183–204.
Bashevkin S. M., Pechenik J. A. (2015). The interactive influence of temperature and salinity on larval and juvenile growth in the gastropod Crepidula fornicata (L.). J. Exp. Mar. Biol. Ecol. 470, 78–91. doi: 10.1016/j.jembe.2015.05.004
Bell M., Batty R., Dick J., Fretwell K., Navarro J., Sargent J. (1995). Dietary deficiency of docosahexaenoic acid impairs vision at low light intensities in juvenile herring (Clupea harengus L.). Lipids 30, 443–449. doi: 10.1007/BF02536303
Boersma M. (2000). The nutritional quality of P-limited algae for Daphnia. Limnology Oceanog. 45, 1157–1161. doi: 10.4319/lo.2000.45.5.1157
Brown M. R. (2002). “Nutritional value and use of microalgae in aquaculture,” in Avances en Nutrición Acuícola VI. Memorias del VI Simposium Internacional de Nutrición Acuícola. 3 al 6 de Septiembre del 2002. Eds. Cruz-Suárez L. E., Ricque-Marie D., Tapia-Salazar M., & Gaxiola-Cortés M. G., Simoes N. (Cancún, Quintana Roo, México), 281–292.
Brown J. H., Gillooly J. F., Allen A. P., Savage V. M., West G. B. (2004). Toward a metabolic theory of ecology. Ecology 85, 1771–1789. doi: 10.1890/03-9000
Castelo Branco R., Antas P., Cunha I. (2014). Preliminary data on Littorina littorea development under rearing conditions. Front. Mar. Sci. Conference Abstract: IMMR| International Meeting on Marine Research 2014. doi: 10.3389/conf.fmars.2014.02.00029
Chaparro O. R., Cubillos V. M., Montory J. A., Navarro J. M., Andrade-Villagrán P. V. (2019). Reproductive biology of the encapsulating, brooding gastropod Crepipatella dilatata Lamarck (Gastropoda, Calyptraeidae). PloS One 14, e0220051. doi: 10.1371/journal.pone.0220051
Cheng P., Zhou C., Chu R., Chang T., Xu J., Ruan R., et al. (2020). Effect of microalgae diet and culture system on the rearing of bivalve mollusks: Nutritional properties and potential cost improvements. Algal Res. 51, 102076. doi: 10.1016/j.algal.2020.102076
Clarke A. (2006). Temperature and the metabolic theory of ecology. Funct. Ecol. 20, 405–412. doi: 10.1111/j.1365-2435.2006.01109.x
Cummins V., Coughlan S., Mcclean O., Connolly N., Mercer J., Burnell G. (2002). An assessment of the potential for the sustainable development of the edible periwinkle, Littorina littorea, industry in Ireland. Mar. Resource Series Dublin. 62.
Da Costa F., Cerviño-Otero A., Iglesias Ó., Cruz A., Guévélou E. (2020). Hatchery culture of European clam species (family Veneridae). Aquaculture Int. 28, 1675–1708. doi: 10.1007/s10499-020-00552-x
Deschaseaux E., Taylor A., Maher W. (2011). Measure of stress response induced by temperature and salinity changes on hatched larvae of three marine gastropod species. J. Exp. Mar. Biol. Ecol. 397, 121–128. doi: 10.1016/j.jembe.2010.11.023
Deschaseaux E. S. M., Taylor A. M., Maher W. A., Davis A. R. (2010). Cellular responses of encapsulated gastropod embryos to multiple stressors associated with climate change. J. Exp. Mar. Biol. Ecol. 383, 130–136. doi: 10.1016/j.jembe.2009.12.013
Dettlaff T. A. (1986). The rate of development in poikilothermic animals calculated in astronomical and relative time units. J. Thermal Biol. 11, 1–7. doi: 10.1016/0306-4565(86)90010-0
Dettlaff T. A. (2003). Time as a Parameter of Development of Poikilothermic Animals. Comments to the Book by L. Wolpert et al.“Principles of Development,” Oxford Univ. Press, 1998. Russian Journal of Developmental Biology, 34, 69–74. doi: 10.1023/A:1023340128147
Doyle D., Frias J., Gammell M. P., Lynch M., Nash R. (2022). Assessing the morphological impacts of long-term harvesting in intertidal gastropods using historical data and morphometric tools. J. Molluscan Stud. 88(3), eyac019. doi: 10.1093/mollus/eyac019
Eschweiler N., Molis M., Buschbaum C. (2009). Habitat-specific size structure variations in periwinkle populations (Littorina littorea) caused by biotic factors. Helgoland Mar. Res. 63, 119–127. doi: 10.1007/s10152-008-0131-x
Forster J., Hirst A. G. (2012). The temperature-size rule emerges from ontogenetic differences between growth and development rates. Funct. Ecol. 26, 483–492. doi: 10.1111/j.1365-2435.2011.01958.x
Forster J., Hirst A. G., Atkinson D. (2011). How do organisms change size with changing temperature? The importance of reproductive method and ontogenetic timing. Funct. Ecol. 25, 1024–1031. doi: 10.1111/j.1365-2435.2011.01852.x
Freckelton M. L., Nedved B. T., Hadfield M. G. (2017). Induction of invertebrate larval settlement; different bacteria, different mechanisms? Sci. Rep. 7, 42557.
Gagné R., Tremblay R., Pernet F., Miner P., Samain J.-F., Olivier F. (2010). Lipid requirements of the scallop Pecten maximus (L.) during larval and post-larval development in relation to addition of Rhodomonas salina in diet. Aquaculture 309, 212–221. doi: 10.1016/j.aquaculture.2010.09.040
Génio L., Sousa A., Vaz N., Dias J. M., Barroso C. (2008). Effect of low salinity on the survival of recently hatched veliger of Nassarius reticulatus (L.) in estuarine habitats: A case study of Ria de Aveiro. J. Sea Res. 59, 133–143. doi: 10.1016/j.seares.2007.09.001
Hadfield M. G. (2011). Biofilms and marine invertebrate larvae: what bacteria produce that larvae use to choose settlement sites. Annu. Rev. Mar. Sci. 3, 453–470. doi: 10.1146/annurev-marine-120709-142753
Hansen B. W., Drillet G., Kozmer A., Madsen K. V., Pedersen M. F., Sørensen T. F. (2010). Temperature effects on copepod egg hatching: does acclimatization matter? J. Plankton Res. 32, 305–315. doi: 10.1093/plankt/fbp122
Harder T., Lam C., Qian P.-Y. (2002). Induction of larval settlement in the polychaete Hydroides elegans by marine biofilms: an investigation of monospecific diatom films as settlement cues. Mar. Ecol. Prog. Ser. 229, 105–112. doi: 10.3354/meps229105
Hoff F. H., Snell T. W. (2008). Plankton Culture Manual. (Dade City, Florida: Florida Aquafarms, Inc.), 186.
Hohenlohe P. A. (2002). Life history of Littorina scutulata and L. plena, sibling gastropod species with planktotrophic larvae. Invertebrate Biol. 121, 25–37. doi: 10.1111/j.1744-7410.2002.tb00126.x
Holm Nielsen B. L., Gøtterup L., Jørgensen T. S., Hansen B. W., Hansen L. H., Mortensen J., et al. (2019). n-3 PUFA biosynthesis by the copepod Apocyclops royi documented using fatty acid profile analysis and gene expression analysis. Biol. Open 8(2), bio038331. doi: 10.1242/bio.038331
Honsey A. E., Rypel A. L., Venturelli P. A. (2023). Guidance for selecting base temperatures when using degree-days in fish growth analyses. Can. J. Fisheries Aquat. Sci. 80, 459–562. doi: 10.1139/cjfas-2022-01971
Jablonski D., Lutz R. A. (1983). Larval ecology of marine benthic invertebrates: paleobiological implications. Biol. Rev. 58, 21–89. doi: 10.1111/j.1469-185X.1983.tb00380.x
Jaschinski S., Sommer U. (2010). Positive effects of mesograzers on epiphytes in an eelgrass system. Mar. Ecol. Prog. Ser. 401, 77–85. doi: 10.3354/meps08412
Johnson M. P., Mcdermott T. (2018). Picking a way forward: valuing and managing traditional shellfish gathering for Littorina littorea. Aquat. Living Resour. 31, 35. doi: 10.1051/alr/2018024
Kabeya N., Fonseca M. M., Ferrier D. E. K., Navarro J. C., Bay L. K., Francis D. S., et al. (2018). Genes for de novo biosynthesis of omega-3 polyunsaturated fatty acids are widespread in animals. Sci. Adv. 4, eaar6849. doi: 10.1126/sciadv.aar6849
Kabeya N., Gür I., Oboh A., Evjemo J. O., Malzahn A. M., Hontoria F., et al. (2020). Unique fatty acid desaturase capacities uncovered in Hediste diversicolor illustrate the roles of aquatic invertebrates in trophic upgrading. Philos. Trans. R. Soc. B. 375, 20190654. doi: 10.1098/rstb.2019.0654
Kamler E. (2002). Ontogeny of yolk-feeding fish: an ecological perspective. Rev. Fish Biol. Fisheries 12, 79–103. doi: 10.1023/A:1022603204337
Kupren K., Mamcarz A., Kucharczyk D., Prusińska M., Krejszeff S. (2008). Influence of water temperature on eggs inCubation time and embryonic development of fish from genus Leuciscus. Polish J. Natural Sci. 23, 461–481. doi: 10.2478/v10020-008-0036-9
Laing I. (1987). The use of artificial diets in rearing bivalve spat. Aquaculture 65, 243–249. doi: 10.1016/0044-8486(87)90237-7
Leandro S. M., Tiselius P., Queiroga H. (2006). Growth and development of nauplii and copepodites of the estuarine copepod Acartia tonsa from southern Europe (Ria de Aveiro, Portugal) under saturating food conditions. Mar. Biol. 150, 121–129. doi: 10.1007/s00227-006-0336-y
Le Tourneux F., Bourget E. (1988). Importance of physical and biological settlement cues used at different spatial scales by the larvae of Semibalanus balanoides. Mar. Biol. 97, 57–66. doi: 10.1007/BF00391245
Lora-Vilchis M. C., Maeda-Martinez A. N. (1997). Ingestion and digestion index of catarina scallop Argopecten ventricosus-circularis, Sowerby II 1842, veliger larvae with ten microalgae species. Aquaculture Res. 28, 905–910. doi: 10.1046/j.1365-2109.1997.00917.x
Lubchenco J. (1983). Littornia and Fucus: effects of herbivores, substratum heterogeneity, and plant escapes during succession. Ecology 64, 1116–1123. doi: 10.2307/1937822
Malzahn A. M., Aberle N., Schoo K. L., Boersma M. (2015). Culture conditions affect the nutritional value of the copepod Acartia tonsa. J. Agric. Mar. Sci. 19, 40–46. doi: 10.24200/jams.vol20iss0pp40-46
Malzahn A. M., Clemmesen C., Rosenthal H. (2003). Temperature effects on growth and nucleic acids in laboratory-reared larval coregonid fish. Mar. Ecol. Prog. Ser. 259, 285–293. doi: 10.3354/meps259285
Martel C. (2009). Conceptual bases for prey biorecognition and feeding selectivity in the microplanktonic marine phagotroph Oxyrrhis marina. Microbial Ecol. 57, 589–597. doi: 10.1007/s00248-008-9421-8
Martínez-Fernández E., Acosta-Salmón H., Rangel-Dávalos C. (2004). Ingestion and digestion of 10 species of microalgae by winged pearl oyster Pteria sterna (Gould 1851) larvae. Aquaculture 230, 417–423. doi: 10.1016/S0044-8486(03)00416-2
Mccoy J. C. S., Spicer J. I., Tills O., Rundle S. D. (2020). Both maternal and embryonic exposure to mild hypoxia influence embryonic development of the intertidal gastropod Littorina littorea. J. Exp. Biol. 223, 12. doi: 10.1242/jeb.221895
Meunier C. L., Boersma M., Wiltshire K., Malzahn A. M. (2016). Even zooplankton eats what it needs: copepod selective feeding and its consequences for marine systems. Oikos 125, 50–58. doi: 10.1111/oik.02072
Miller L. P., Denny M. W. (2011). Importance of behavior and morphological traits for controlling body temperature in littorinid snails. Biol. Bull. 220, 209–223. doi: 10.1086/BBLv220n3p209
Muller-Feuga A. (2000). The role of microalgae in aquaculture: situation and trends. J. Appl. Phycol. 12, 527–534. doi: 10.1023/A:1008106304417
Neuheimer A. B., Taggart C. T. (2007). The growing degree-day and fish size-at-age: the overlooked metric. Can. J. Fisheries Aquat. Sci. 64, 375–385. doi: 10.1139/f07-003
Nevejana N., Saeza I., Gajardoa G., Sorgeloos P. (2003). Supplementation of EPA and DHA emulsions to a Dunaliella tertiolecta diet: effect on growth and lipid composition of scallop larvae, Argopecten purpuratus (Lamarck 1819). Aquaculture 217, 613–632. doi: 10.1016/S0044-8486(02)00585-9
Paul C., Sommer U., Matthiessen B. (2021). Composition and dominance of edible and inedible phytoplankton predict responses of Baltic sea summer communities to elevated temperature and CO2. Microorganisms 9(11), 2294. doi: 10.3390/microorganisms9112294
Petraitis P. S. (2002). Effects of intraspecific competition and scavenging on growth of the periwinkle Littorina littorea. Mar. Ecol. Prog. Ser. 236, 179–187. doi: 10.3354/meps236179
Podbielski I., Hiebenthal C., Hajati M.-C., Bock C., Bleich M., Melzner F. (2022). Capacity for cellular osmoregulation defines critical salinity of marine invertebrates at low salinity. Front. Mar. Sci. 9. doi: 10.3389/fmars.2022.898364
Rasband W. (1997–2012). ImageJ (Bethesda, Maryland, USA: U.S. National Institutes of Health). imagej.nih.gov/ij/.
Richmond C. E., Woodin S. A. (1996). Short-term fluctuations in salinity: effects on planktonic invertebrate larvae. Mar. Ecol. Prog. Ser. 133, 167–177. doi: 10.3354/meps133167
Robert R., Trintignac P. (1997). Substitutes for live microalgae in mariculture: a review. Aquat. Living Resour. 10, 315–327. doi: 10.1051/alr:1997035
Sales R., Lopes R. G., Derner R. B., Tsuzuki M. Y. (2022). Concentrated microalgal biomass as a substitute for fresh microalgae produced on site at hatcheries. Aquaculture Res. 53, 5771–5786. doi: 10.1111/are.16072
Southgate P. C., Braley R. D., Militz T. A. (2017). Ingestion and digestion of micro-algae concentrates by veliger larvae of the giant clam, Tridacna noae. Aquaculture 473, 443–448. doi: 10.1016/j.aquaculture.2017.02.032
Strathmann M. F., Strathmann R. R. (2007). An extraordinarily long larval duration of 4.5 years from hatching to metamorphosis for teleplanic veligers of Fusitriton oregonensis. Biol. Bull. 213, 152–159. doi: 10.2307/25066631
Taylor P. M., Andrews E. B. (1988). Osmoregulation in the intertidal gastropod Littorina littorea. J. Exp. Mar. Biol. Ecol. 122, 35–46. doi: 10.1016/0022-0981(88)90210-9
Thomas W. H., Scotten H. L., Bradshaw J. S. (1963). Thermal gradient inCubators for small aquatic organisms. Limnology Oceanog. 8, 357–360. doi: 10.4319/lo.1963.8.3.0357
Thomsen D. S., Koed A., Nielsen C., Madsen S. S. (2007). Overwintering of sea trout (Salmo trutta) in freshwater: escaping salt and low temperature or an alternate life strategy? Can. J. Fisheries Aquat. Sci. 64, 793–802.10.1139/f07-059. doi: 10.1139/f07-059
Tocher D. R. (2003). Metabolism and functions of lipids and fatty acids in teleost fish. Rev. Fisheries Sci. 11, 107–184. doi: 10.1080/713610925
Tremblay R., Cartier S., Miner P., Pernet F., Quéré C., Moal J., et al. (2007). Effect of Rhodomonas salina addition to a standard hatchery diet during the early ontogeny of the scallop Pecten maximus. Aquaculture 262, 410. doi: 10.1016/j.aquaculture.2006.10.009
Van Der Have T. M., De Jong G. (1996). Adult size in ectotherms: temperature effects on growth and differentiation. J. Theor. Biol. 183, 329–340. doi: 10.1006/jtbi.1996.0224
Van Donk E., Luerling M., Hessen D. O., Lokhorst G. M. (1997). Altered cell wall morphology in nutrient-deficient phytoplankton and its impact on grazers. Limnology Oceanog. 42, 357–364. doi: 10.4319/lo.1997.42.2.0357
Von Bertalanffy L. (1960). “Principles and theory of growth,” in Fundamental Aspects of Normal and MALIgnant Growth. Ed. Nowinski W. W. (Amsterdam: Elsevier).
Walne P. R. (1970). Studies on the food value of nineteen genera of algae to juvenile bivalves of the genera Ostrea, Crassostrea, Mercenaria, and Mytilus. Fish Investig. 26, 162.
Watson D. C., Norton T. A. (1985). Dietary preferences of the common periwinkle, Littorina littorea (L.). J. Exp. Mar. Biol. Ecol. 88, 193–211. doi: 10.1016/0022-0981(85)90230-8
Wichard T., Beemelmanns C. (2018). Role of chemical mediators in aquatic interactions across the prokaryote–eukaryote boundary. J. Chem. Ecol. 44, 1008–1021. doi: 10.1007/s10886-018-1004-7
Wolpert L., Tickle C., Arias A. M. (2015). Principles of development (USA: Oxford University Press).
Zhang H., Cheung S. G., Shin P. K. S. (2014). The larvae of congeneric gastropods showed differential responses to the combined effects of ocean acidification, temperature and salinity. Mar. pollut. Bull. 79, 39–46. doi: 10.1016/j.marpolbul.2014.01.008
Keywords: snail aquaculture, larval development, embryonic development, temperature life history, Littorina littorea
Citation: Lillebjerka T, Malzahn AM, Kjørsvik E and Hagemann A (2023) Effects of temperature, salinity and diet on embryonic and early larval development in Littorina littorea (Gastropoda: Littorinimorpha). Front. Mar. Sci. 10:1240599. doi: 10.3389/fmars.2023.1240599
Received: 15 June 2023; Accepted: 27 July 2023;
Published: 17 August 2023.
Edited by:
Alexander Ereskovsky, Institut Mediterranéen de Biodiversité et d’Ecologie marine et continentale (IMBE), FranceReviewed by:
Omar Hernando Avila-Poveda, Universidad Autonoma de Sinaloa, MexicoCopyright © 2023 Lillebjerka, Malzahn, Kjørsvik and Hagemann. This is an open-access article distributed under the terms of the Creative Commons Attribution License (CC BY). The use, distribution or reproduction in other forums is permitted, provided the original author(s) and the copyright owner(s) are credited and that the original publication in this journal is cited, in accordance with accepted academic practice. No use, distribution or reproduction is permitted which does not comply with these terms.
*Correspondence: Andreas Hagemann, YW5kcmVhcy5oYWdlbWFubkBzaW50ZWYubm8=
†These authors have contributed equally to this work and share first authorship
Disclaimer: All claims expressed in this article are solely those of the authors and do not necessarily represent those of their affiliated organizations, or those of the publisher, the editors and the reviewers. Any product that may be evaluated in this article or claim that may be made by its manufacturer is not guaranteed or endorsed by the publisher.
Research integrity at Frontiers
Learn more about the work of our research integrity team to safeguard the quality of each article we publish.