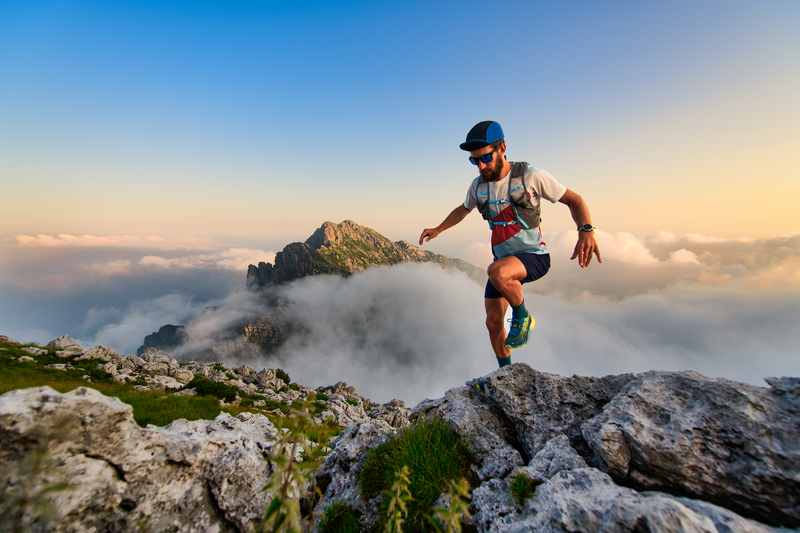
95% of researchers rate our articles as excellent or good
Learn more about the work of our research integrity team to safeguard the quality of each article we publish.
Find out more
ORIGINAL RESEARCH article
Front. Mar. Sci. , 13 November 2023
Sec. Marine Megafauna
Volume 10 - 2023 | https://doi.org/10.3389/fmars.2023.1238837
This article is part of the Research Topic Conservation Implications of Pathogens, Parasites, and Pollutants for Sea Turtles View all 6 articles
Sea turtle nesting beaches are experiencing increased sand temperatures as climate change progresses. In one major green turtle (Chelonia mydas) nesting beach in the northern Great Barrier Reef, over 99 percent of hatchlings are female. The effects of contaminants on sea turtle hatchling sex determination are not often explored. Liver samples were collected from green turtle hatchlings that were sacrificed for histological sex determination in a parallel study on the effects of sand cooling on sex ratios, and analysed for trace elements via acid digestion and organic contaminants via in vitro cytotoxicity bioassays. Chromium, antimony, barium, and cadmium have previously been demonstrated to be estrogenic, and concentrations of these elements were used to calculate three estrogenic indexes for each clutch: predicted relative estrogenic potency (PEEQA), the sum of percent trace elements above the median of all samples (TEOM), and the sum of percent estrogenic elements above the median of all samples (EstroEOM). Excluding an outlier clutch, cadmium, antimony, and EstroEOM had significant positive relationships with sex ratio deviation. Mean clutch cobalt, lead, antimony and barium, also had a significant positive relationship with clutch sex ratio. There was no relationship between in vitro cytotoxicity of liver extracts and sex ratio, however, 9% of hatchlings had organic contaminants high enough to suggest potential cellular damage. Contaminant effects on sex determination are likely to be caused by a mixture of contaminant interactions as well as temperature. Many trace elements detected in this study have also been linked to negative health effects on hatchlings in previous studies. Considering the risks of feminization due to climate change and potential contaminant effects on hatchling health and sex determination, future studies exploring contaminant effects on sea turtle hatchling sex determination are recommended.
The world’s ocean and surface temperatures are increasing due to climate change (Loarie et al., 2009; Abraham et al., 2013), adding challenges to the recovery of threatened species (Hawkes et al., 2009; Hamann et al., 2010). Sea turtles travel, sometimes thousands of kilometres, from their foraging grounds to sandy beaches in the regions where they hatched to lay their eggs (Meylan et al., 1990; Bowen et al., 1992; Limpus et al., 1992). Sea turtles have temperature-dependent sex determination (TSD), in which nest temperature causes a series of epigenetic and hormonal changes that determine hatchling sex development (Valenzuela and Lance, 2004; Warner, 2011). As a result, there are many studies investigating whether climate change could negatively affect sea turtle population recovery. For example, increased sand temperature on nesting beaches on the northern Great Barrier Reef (GBR) is already causing 99% female bias in hatchling production of green turtle (Chelonia mydas) (Jensen et al., 2018).
As a reproductive strategy, TSD can offer resilience to increased temperatures by producing more females, and thereby, increasing reproductive capacity (Santidrián Tomillo et al., 2015; Santidrián Tomillo and Spotila, 2020). However, more recent studies have found that moderate- to high-temperature scenarios still lead to declining northern GBR green turtle populations (Blechschmidt et al., 2020), and that female-skewed sex ratios take time to manifest within populations (Hays et al., 2023). Additional concerns that can compound nest feminization include sea turtle nests reaching upper thermal thresholds, reducing hatchling health and survival (Fuentes et al., 2011; Fleming et al., 2020; Page-Karjian et al., 2022). For example, loggerhead sea turtles nesting within Cabo Verde islands are predicted to reach over 99% female hatchling production, with 90% of nests reaching upper thermal limits under current climate change predictions (Tanner et al., 2019). To combat these issues, recent studies have explored using artificial means, such as cool water irrigation, to decrease sand and nest temperatures, and thus increase the proportion of male hatchlings produced on warmer nesting beaches (Lolavar and Wyneken, 2021; Porter et al., 2021; Smith et al., 2021). Both freshwater and sea water irrigation have been found to be effective at reducing nest temperatures and producing males, without decreasing hatching success in situ (Lolavar and Wyneken, 2021; Porter et al., 2021; Smith et al., 2021).
Due to the invasive nature of assessing the sex of sea turtle hatchlings (removal and histological investigation of the gonads), the sex ratio of incubating clutches is often predicted or modelled. Depending on the methods used, models estimating sex ratio from sand temperature can vary significantly, and may not be as accurate as previously believed (Georges et al., 1994; Wyneken and Lolavar, 2015). There are many other factors, such as nest depth, shading, and metabolic heating that can affect nest temperatures, and hence sex ratios (van de Merwe et al., 2006). The current study proposes that anthropogenic contaminants may be an additional factor that can influence hatchling sex determination.
Anthropogenic contaminants have been found to disrupt a variety of endocrine and reproductive systems in reptiles (Gardner and Oberdorster, 2005; Boggs et al., 2011; Barraza et al., 2021). In sea turtles, only dichlorodiphenyldichloroethylene (DDE) has been investigated directly, and was found not to influence sea turtle hatchling sex determination at the concentrations tested (Podreka et al., 1998). However, when examining the literature on other reptile species, many studies have found that contaminants can skew sex ratios of TSD species such as alligators, freshwater turtles, and caimans (Bergeron et al., 1994; Crain et al., 1997; Beldomenico et al., 2007; Canesini et al., 2018). For example, pond slider (Trachemys scripta) eggs dosed with a mixture of polychlorinated biphenyls (PCBs) produced more female-biased clutches than dosing with single PCB congener (Bergeron et al., 1994).
It is known that contaminants accumulate in green turtles (Keller, 2003; Finlayson et al., 2016; Cortes-Gomez et al., 2017), mainly from foraging near contaminated areas (manuscript in review), and that these contaminants can be maternally offloaded to their eggs, resulting in in ovo hatchling exposure (van de Merwe et al., 2009; Perrault et al., 2011; Perrault et al., 2016). We hypothesised that this maternal transfer of contaminants may influence sex determination in green turtle hatchlings. Considering the logistical and ethical difficulties in conducting a direct dosing experiment on green turtle embryos, the influence of contaminants in altering sex determination was investigated in conjunction with a concurrent study that assessed the impact of fresh and sea water irrigation on hatchling sex ratios in situ. Hatchlings that emerged from these manipulated nests were analysed for inorganic and organic contaminants, and concentrations were interpreted in the context of expected and actual sex ratios to assess possible evidence of contaminant-induced sex ratio skewing.
In the concurrent nest cooling study, research procedures and methods were approved by animal ethics committee at the University of Queensland (SBS/237/20), and egg and hatchling collection was completed under a Queensland Parks and Wildlife Services (QPWS) scientific purposes permit (PTU19-002377-1). All efforts were made to minimise suffering and maximise the amount of data produced from each animal.
The concurrent nest cooling experiment was a part of WWF-Australia’s Turtle Cooling Project, which aimed to reduce the risk of excessive feminization of green turtles across Asia-Pacific (Young et al. in press). Green turtle clutches were collected from Heron Island (latitude -23.8416, longitude 151.2498), a small coral sand cay on the southern GBR, roughly 80 km off the coast of Gladstone, Queensland, Australia. Heron Island is a medium density green turtle nesting site (200 – 1800 nesting females per season) and was selected because it is a long-term monitoring site for the southern GBR nesting population of green turtles.
Clutches (n = 17) were relocated within two hours of oviposition with HOBO MX2303 wireless temperature probes placed amongst eggs, with the nest and temperature logged every hour throughout incubation. The relocated clutches were arranged in a Latin square design pattern to avoid column or row effects between clutches. Five different irrigation regiments were used: a control with no nest irrigation (Control), freshwater irrigation of the nest (Freshwater), sea water irrigation of the nest (Seawater), chilled seawater irrigation of the nest (Cold Seawater), and twice the amount of sea water irrigation (2x Seawater; Table 1). Data on the relationships between incubation temperature, incubation period, and hatchling sex-ratio were gathered from published literature (Bustard and Greenman, 1967; Miller and Limpus, 1981; Booth and Astill, 2001; Booth et al., 2004; Burgess et al., 2006). Using these data, a predictive non-linear algorithm for the relationship between incubation temperature and embryonic development rate and hatchling sex-ratio was formulated (Smith et al., 2021). Nest temperatures were downloaded periodically from HOBO temperature probes throughout incubation, without disturbing the sand, and used to calculate when the developing embryos were midway through development so that the irrigation treatments could be applied at the time when a decrease in temperature is effective in altering hatchling sex ratios (Porter et al., 2021).
Table 1 Summary data for each green turtle clutch including mean sex determining period (SDP) temperature, the predicted % females (based on constant temperature equivalents), the measured % females (based on histological examination of gonads), and the percent sex ratio deviation of the clutch from predicted % females, with positive percentages indicating more females than expected.
A constant temperature equivalent (CTE; Georges et al., 1994) was then calculated using hourly nest temperature data during the sex determining period (SDP) for each nest, and used to calculate the predicted hatchling sex ratio for each nest from the relationship between incubation temperature and the proportion of female hatchlings produced at different temperatures. Briefly, a quadratic model was used to predict the hourly embryonic development based on temperature readings (Booth et al., 2022). The product of the incubation temperature and the amount of development at the temperature is summed during the sex determining period to calculate a constant temperature equivalent. The actual sex ratio of each nest was determined from histological examination of the gonads of 10 hatchlings from each nest (Young et al., 2023).
Once hatchlings had emerged from their nests, they were euthanised by placing them inside a sealed container for 1 h with 2-4 mL of isoflurane (gaseous anesthetic). Once deceased, each hatchling was dissected, and both left and right kidney‐gonad complexes were removed. Gonad histology was carried out as described in Young et al. (in press) for sex identification. Hatching success was also calculated for each clutch post-emergence.
Hatchling liver samples (0.5 - 1 g wet weight) were dissected from 16 of the clutches (no hatchlings from clutch 7 were collected) that were sacrificed for histological examination of gonads. Liver samples were then partitioned into two halves, one stored in an Eppendorf tube for trace element analysis, and one wrapped in aluminium foil and stored in an Eppendorf tube for organic contaminant analysis. Liver samples were freeze dried (Christ Alpha 1-4 LSCbasic, Germany) for 12 h, and kept frozen at -20°C until analysis.
Inductively coupled plasma mass spectrometry (ICP-MS) was used to analyse 22 trace elements (see Supplementary Material; Table 6.6) in 78 hatchling liver samples, or roughly five samples per clutch. Briefly, liver samples (~0.25 g dry weight, accurately weighed) were microwave-digested (CEM Mars 6; model 910905; EPA method 3052) with 4.5 mL nitric acid, 1 mL hydrochloric acid, and 1 mL hydrogen peroxide within pressurised Teflon vessels. Sample batches included laboratory blanks (MilliQ water) and dogfish liver standard reference material DOLT-5 (National Research Council Canada). Digests were diluted 1:50 in MilliQ water, and 100 µL was injected into the ICP-MS (Agilent Technologies; 7900). Metal concentrations were analysed using Agilent Chemstation software (ICP-MS MassHunter version 4.3). Trace elements concentrations were blank adjusted within batches with the mean laboratory blank concentrations. All trace elements were within 85 – 115% of DOLT-5 certified values with less than 5% standard error.
Organic contaminants were extracted via a QuEChERS (quick, easy, cheap, effective, rugged, and safe) method used in previous research with sea turtles (Dogruer et al., 2018; Finlayson et al., 2020). The QuEChERS method extracts a wide range of organic contaminants, such as polycyclic aromatic hydrocarbons (PAHs), polychlorinated biphenyls (PCBs), and polybrominated diphenyl ethers (PBDEs) (Anastassiades et al., 2003; Escher et al., 2021). This method has previously been used to assess organic contaminant loads in foraging green turtles along the eastern Australia coast (Finlayson et al., 2022).
Briefly, freeze dried liver samples (n = 78) were thawed and homogenised in 50 mL Falcon tubes with 10 mL of MilliQ water, using a laboratory hand blender. The hand blender was solvent-cleaned with acetone and water between each sample. Two ceramic homogenisers were added to centrifuge tubes and vortexed for 1 min, followed by addition of 15 mL of acetonitrile and further vortexing for 1 min. Five grams of magnesium sulfate and 1 g of sodium chloride were added to the Falcon tube and shaken for 1 min. Mixtures were then centrifuged at 1990×g for 8 min at 10°C. The supernatant was aliquoted into glass vials and evaporated under a gentle stream of nitrogen gas. Dried extracts were reconstituted in 1 mL methanol and transferred into glass amber vials with Teflon lids. Extracts were stored at -20°C until use in the cytotoxicity bioassay.
The toxicity of the liver extracts (containing a mixture of organic chemicals) to green turtle primary skin fibroblasts was measured using a resazurin cell viability assay, following previously established methods (Finlayson et al., 2019a; Finlayson et al., 2021). Briefly, green turtle primary skin fibroblasts (GT10s-p), established in Finlayson et al. (2019b) were grown in RPMI-1640 medium with 10% foetal bovine serum (FBS) and incubated at 30°C with 5% CO2. A subsample of each methanol extract was evaporated using nitrogen gas and reconstituted in RPMI-1640 medium with 5% FBS, resulting in a relative enrichment factor of 2, corresponding to twice the contaminant concentration in liver samples (Finlayson et al., 2020).
A flat clear bottom 96-well microtiter plate was seeded with 3×104 cells per well and incubated for 24 h at 30°C with 5% CO2. After 24 h, a separate dosing plate was used to prepare extracts in a serial dilution (2-fold, 4 point) along with: Triton X-100 (0.1%) as a positive control, chromium in a 6-point, 2-fold dilution as a reference compound, and a negative control of RPMI medium (supplemented with 5% FBS). Medium from the seeded plate was then replaced with medium from the dosing plate (containing samples and controls) for a 24-h exposure. All controls and extracts were added to the seeded plate in duplicate. After 24 h, 20 µL of 0.15 mg/mL resazurin in phosphate buffered saline and 80 µL of RPMI medium (5% FBS) were added to each well for a further 24-h incubation. Following resazurin incubation, each well’s fluorescence was measured at λex = 544 nm and λem = 590 nm using a Spark plate reader (Tecan, Switzerland). Each plate analysed 10 extracts/controls, and extracts were tested in at least two independent assay runs on separate days.
The percent viability of cells for each sample/reference dilution with an assay was calculated by Eq (1). F signifies mean fluorescence of sample/reference duplicates, NC signifies mean fluorescence of the negative control duplicates, and PC signifies mean fluorescence of the positive control duplicates.
Mean percent viability (from multiple assay runs) of the sample/reference dilutions were plotted against the log concentration of sample/reference (expressed as relative enrichment factor) in GraphPad Prism 5 (GraphPad Software Inc), and the Hill Slope equation was used to calculate the relative enrichment factor (REF) required to produce 20% decrease in cell viability (IC20) of each sample, and the reference compound. The results were then expressed as a Toxic Unit (TU), the reciprocal of the IC20. A high TU therefore indicates greater toxicity, and TU = 1 indicates that contaminant concentrations present in the sample decreased cell viability by exactly 20% (at a relative enrichment factor of 1).
An α of 0.05 was used for statistical analyses in R (v. 4.2.0) with RStudio (v. 2022.06.0) and GraphPad Prism 5 (GraphPad Software Inc). Trace elements below limits of detection were replaced by detection frequency times the limit of detection, which was calculated as three times the standard deviation of laboratory blanks. Kaplan-Meier adjustments were not possible with these data as most elements measured were either all above detection limits or had >70% data below LOD (Helsel, 2012). Elements with over 70% below LOD include caesium and mercury. Sex ratio deviation (%) was calculated as: measured % females – predicted % females, with a positive sex ratio deviation indicating more females than predicted.
To assess the potential impact of pollutants on skewing hatchling clutch sex ratio, several parameters were calculated to represent estrogenic potential or overall contamination levels: 1) predicted 17β-estradiol equivalent activity (PEEQA), 2) percent trace elements over median (TEOM), and 3) percent estrogenic elements over median (EstroEOM). Choe et al. (2003) tested 28 metals in a reporter gene assay for estrogenic activity (MCF7-ERE) and reported the relative estrogenic potencies for four elements: antimony (1.62×10-3), chromium (7.68×10-4), cadmium (2.45×10-4), and barium (3.57×10-5). Estrogenic potencies were determined as concentrations needed to achieve the same effect as 17β-estradiol to MCF 7-ERE human breast cancer cells (Choe et al., 2003). These relative potencies were multiplied by the concentration of the elements measured in hatchling livers and summed to calculate a PEEQA (in ng 17β-estradiol per g) for each hatchling. For the second measure (TEOM), the number of trace elements per hatchling over the median of all samples was summed for each clutch then divided by the number of elements and multiplied by 100%. This value was calculated to highlight unusually high contaminant loads in a clutch. For example, if all five hatchlings in a clutch had 10 out of 20 elements over the median of those elements across the corresponding clutch samples, that would correspond to a TEOM value of (5 [hatchlings] × 10 [out of 20 elements])/100 [representing a maximum of five hatchlings having all 20 elements] *100% = 50%. A similar method was used to calculate the last measure (EstroEOM), except that only four estrogenic elements (chromium, barium, antimony, and cadmium) over the median of all hatchlings was calculated for each clutch. For example, if all five hatchlings in a clutch had three of the four estrogenic elements over the median of those elements across all samples, that would result in a EstroEOM value of (5 [hatchlings] × 3 [out of four elements])/20 [representing a maximum of five hatchlings with all 4 elements] * 100% = 75%.
To compare the relationship between non-parametric means of individual trace elements, a generalised additive model was used to calculate the relationship between these calculated parameters and sex ratio or sex ratio deviation. To offset the influence of temperature, clutch 17, identified as an outlier by Grubbs test, was excluded from generalised additive models between trace elements and sex ratio. Spearman’s correlations were used to assess the relationship between mean clutch contaminants and clutch hatching success.
This study found that contaminant loads in hatchling livers had significant effects on sex ratio and sex ratio deviation. Of the 16 clutches sampled, eleven clutches had more females than predicted (8 – 90% more females), three had more males (9 – 33% more males), and two matched the predicted sex ratio (Table 1). As cobalt, lead, antimony, barium, PEEQA, and EstroEOM increased, so did the female ratio of the clutch (Figure 1). Lead, chromium, antinomy, barium, and cadmium have been previously studied for their endocrine disrupting effects on animal reproductive health, especially in mammals and fish (Adeel et al., 2017; Lecomte et al., 2017). A previous study found these elements to be estrogenic in a human estrogen reporter gene assay, particularly barium, antimony, chromium, and cadmium (Choe et al., 2003). Antimony is commonly found in waterways due to plastic bottle degradation (Mihucz and Záray, 2016), and has been found in higher concentration in blood of turtles foraging near agriculture centers in Eastern Australia (Villa et al., 2016). Barium exposure in zebrafish caused increased production of the female-specific hormone estradiol in male fish (Kwon et al., 2016). Cadmium and chromium have been extensively studied for their negative reproductive effects on fish (Chakraborty, 2021), including reducing sperm quality in pejerrey (Odontesthes bonariensis) fish (Gárriz and Miranda, 2020). Cadmium exposure in pond sliders (Trachemys scripta) has been shown to alter the transcription of dmrt1 and aromatase (Mizoguchi et al., 2022), both of which are important for sex determination. Other trace elements, such as lead, have been directly linked to endocrine disruption in sea turtles, with a previous study finding that increasing concentrations of lead inhibited estrogen and testosterone binding in plasma of nesting green turtles (Ikonomopoulou et al., 2009).
Figure 1 The relationship between mean clutch cobalt (p = 0.02; A), lead (p = 0.04; B), antimony (p = 0.03; C), barium (p = 0.02; D), sex determining period (SDP) temperature (p = 0.06; E), predicted 17b-estradiol equivalent activity (PEEQA, p = 0.01; F), estrogenic elements over median (EstroEOM, p = 0.001; G), and trace elements over median (TEOM, p = 0.006; H) and green turtle clutch sex ratio (female:male), excluding clutch 17. Element concentrations are μg/g dry weight.
The relationship between sex ratio deviation and contaminants was less evident in the current study. Cadmium had a significant positive relationship with sex ratio deviation, while PEEQA and EstroEOM trended with sex ratio deviation but did not show a significant relationship (Figure 2). The current study used previous research to calculate relative estrogen potency, but the same study demonstrated that different metal species can have different relative estrogenic potencies (Choe et al., 2003). The methods used in the current study cannot account for these differences and the potential for mixture effects with organic compounds. In addition, previous research has found that the ability for organic compounds such as PCBs to inhibit estrogen receptor affinity can vary across mammalian and reptilian models (Matthews and Zacharewski, 2000). However, cadmium, a known endocrine disrupting compound, did have a significant relationship with sex ratio deviation, highlighting the need for future research investigating how contaminants could cause disruptions to sex determination in sea turtle hatchlings, as they could have population-level effects.
Figure 2 The relationship between mean clutch cadmium (p = 0.03; A), antimony (p = 0.09; C), and estrogenic elements over median (EstroEOM, p = 0.09; E) and clutch sex deviation (left panels). The relationship between mean clutch cadmium (p = 0.002; B), antimony (p = 0.02; D), and estrogenic elements over median (EstroEOM, p = 0.03; F) and green turtle clutch sex deviation excluding clutch 13 (right panels). Element concentrations are μg/g dry weight.
Multiple reptilian studies have shown that contaminants can override temperature and potentially impact reproductive output of TSD animals (Barraza et al., 2021). In previous research, freshwater turtle and crocodilian studies found contaminant-driven sex determination disruption, but direct evidence of contaminant effects on the sex determination of sea turtles has been elusive (Barraza et al., 2021), with a single study showing no effect on sex ratios when dosing with DDE (Podreka et al., 1998). However, synergistic effects have been found in pond sliders as eggs dosed with PCB mixtures produced more female-bias clutches than with single PCB congener dosing (Bergeron et al., 1994). PCB dosing in another pond slider study found that some PCB congeners had no effect on TSD, while other congeners acted synergistically to disrupt sex determination (Matsumoto et al., 2014). In a model using snapping turtle (Chelydra serpentina) data, endocrine disruption via PCBs caused small variations in predicted pivotal temperature and sex determination towards feminization (Salice et al., 2014). Additional modeling from the same study suggested that even small increases in feminization could lead to population declines in long-lived species (Salice et al., 2014). Conversely, if contaminants masculise hatchlings, they may provide some resistance to feminization as climate change increases temperature, but at the potential cost of individual health. There has been some research demonstrating that exogenous estrogens and higher temperatures produce more females pond sliders than estrogen dosing alone (Wibbels et al., 1991). Therefore, as nesting beach temperatures increase across the globe, maternally transferred estrogenic contaminants could further exacerbate the feminizing effect of increased temperature. Exogenous estrogen dosing has also been found to have no effect on sex determination during extreme temperature events, but a significant effect at milder temperatures in lizards (Warner et al., 2017). For sea turtles, these studies suggest that nesting females with high contaminant loads nesting on beaches with mild temperatures may have the highest risk of altered sex ratios. This becomes especially important as beaches with mild temperatures are expected to offset the further increasing female bias already seen in northern GBR nesting beaches (Jensen et al., 2018).
In this study, cell-based methods were used to measure the overall effect of the complex mixture of organic contaminants on green turtle cell viability, instead of attempting to analyse individual organic contaminants. We did not find a significant relationship between the organic contaminant load (as measured by cell viability) and sex ratio (p = 0.5) or sex ratio deviation (p = 0.4) (data not shown). However, organic contaminant load differences were found between males and females from the same clutch in some comparisons. Including all clutches, significantly higher toxicity (TUIC20) was measured in extracts from male compared to female hatchlings (p = 0.02). Of the eight hatchlings with a TU > 1, seven (88%) were male. These data indicate that among the clutches examined, male hatchlings had higher organic contaminant concentrations in the liver than female hatchlings, and in some cases, high enough to cause cellular damage (as measured by our cytotoxicity assay).
It is important to note that not all contaminant comparisons with hatchling sex ratio or sex ratio deviation matched expectations. In addition, many of the clutches had individuals that were outliers in one or two elements compared to other individuals within their clutch. Typically, contaminant loads tend to be similar among hatchlings from the same clutch (Guirlet et al., 2010; Páez-Osuna et al., 2010; van de Merwe et al., 2010; Perrault et al., 2011; Perrault et al., 2016). There were no significant relationships between any contaminant and size (mass or carapace length), temperature, or nest irrigation treatment group. Outlier concentrations were not consistently from the same clutch and only confined to one or two elements, reducing the chance that these data are due to sampling or laboratory errors. These data may demonstrate that hatchlings contaminant loads differ within a clutch, potentially due to differences in egg contamination. One particularly unusual clutch, clutch 13, had high sex deviation but low estrogenic and overall trace element concentrations (Table 1; Figure 2). Removing clutch 13 from sex ratio deviation comparisons resulted in cadmium (p = 0.002), antimony (p = 0.02), and EstroEOM (p = 0.03) having a significant positive relationship with sex ratio deviation (Figure 2). Many organic contaminants or endocrine disruptors can be far more estrogenic than trace elements (Choe et al., 2003; Leusch et al., 2009), and estrogen receptor binding of contaminants can vary by species (Matthews and Zacharewski, 2000; Matthews et al., 2000). However, our measure of organic contaminants did not correlate to sex ratio deviation. One possibility for this pattern is that the estrogenic effects of organic contaminants is not necessarily associated with cytotoxicity, but rather driven by a few specific and highly potent estrogenic mimics. Estrogenic chemicals induce an estrogenic response at concentrations well below cytotoxic concentrations, and it is therefore not possible to estimate the estrogenic activity associated with organic contaminants in hatchling livers from the cytotoxicity results. As make-up of the organic contaminants in hatchling samples can vary based on maternal contaminant loads, assessing the estrogenic potential from organic contaminants could be beneficial for future research. Regardless, cadmium, antimony, cobalt, lead, barium, TEOM, EstroEOM, and PEEQA had significant relationships with clutch sex ratio, while mean sex determining period temperature did not (Figure 1E). There have been studies that have found synergistic relationships between temperature and contaminant accumulation, such as in amphipods (Jacobson et al., 2007). It is possible that sea turtles may have inherent differences in male and female trace element accumulation due to incubation temperature. However, our data suggest that, at least in a temperature range where a mixed sex ratio can develop, some contaminants have a stronger correlation to sex ratio than temperature. Studies find contaminants in eggshell and albumen. There may be preferential maternal transfer in certain tissues such as liver, which is often used to assess organic contaminants due to its high lipid content. Considering the relationships between other contaminants and sex ratio deviation, these data indicate that there may be a potential for contaminants to influence hatchling sex determination.
Finally, it is important to place these hatchling liver contaminant concentrations in the context of sea turtle health, more generally. Many of the trace elements and toxicity measures have been studied for their negative health effects in sea turtles. Few studies have examined hatchling livers for trace elements with two studies examining green turtle hatchlings in Oman (Al-Rawahy et al., 2007) and Saudi Arabia (Tanabe et al., 2022), and one study examining loggerhead hatchlings in Turkiye (Kaska and Furness, 2001). Most trace elements, except selenium, were similar or less than the concentrations found in these other studies (Supplementary Material; Table 6.7 and 6.8). In green turtles, barium was correlated with reduced hatching success (Souza et al., 2018), and vanadium has been negatively correlated with hatching success in leatherback turtles (Dennis et al., 2020). In this study, only iron negatively correlated (p = 0.03, rho = -0.54) with hatching success. To date, there have not been any studies finding a negative correlation between iron and hatchling success in green turtles. However, while iron is an essential element, there are studies that have found excess iron to cause reduced hatching success in freshwater insects and lampreys (Myllynen et al., 1997; Rousch et al., 1997). Organic contaminants such as persistent organic pollutants have been correlated with reduced hatchling size in green turtles (van de Merwe et al., 2010). Liver extracts from male hatchlings exhibited significantly higher cytotoxicity than female, often above the threshold of 1 TU (suggestive of cellular toxicity at the concentrations detected), indicating that either they might be more vulnerable to contaminants or that male livers contain more cytotoxic endogenous compounds. The current study is the first to assess hatchling organic contaminants with an effects-based method, using primary turtle cell bioassays. Out of 78 hatchling liver samples tested, only one sample did not induce a cytotoxic response in green turtle primary skin fibroblasts. Compared to previous research in foraging immature green turtles throughout Queensland (Finlayson et al., 2021), hatchling liver samples had toxic unit values comparable to blood extracts from turtles foraging within foraging grounds throughout Queensland. Previous research has shown that maternal organic contaminants are transferred to offspring (van de Merwe et al., 2010), with less lipophilic PCB congeners being transferred more readily. Potentially, less lipophilic contaminants are more cytotoxic within green turtle fibroblasts than more lipophilic contaminants. However, the in vitro cytotoxicity of two similarly lipophilic compounds, 4,4′-dichlorodiphenyldichloroethylene (4,4’-DDE) and perfluorononanoic acid (PFNA), were compared within green turtle, loggerhead turtle, dugong, Burrunan dolphin, and common bottlenose dolphin cell lines (Finlayson and van de Merwe, 2021). There were species differences in cytotoxicity in PFNA, but not 4,4’-DDE, and PFNA was less cytotoxic in green turtle cells than 4,4’DDE. These data suggest that there is likely wide variation in in vitro cytotoxicity between compounds, and that variation is likely to increase when accounting for mixture effects and differences in compounds that are maternally transferred.
While the current study cannot point to a specific contaminant that causes feminizing in sea turtle hatchlings, this study demonstrates that chemical mixtures can possibly affect sex ratio and sex ratio deviation, trending towards feminization. Considering the relatively low sample size of the current study (16 nests), finding significant positive relationships to sex ratio and sex ratio deviation with contaminant mixtures within hatchling sea turtles suggests that contaminants may affect sex determination. Cadmium was the only element measured with a moderate relationship with sex ratio deviation, and cadmium has been shown to alter the transcription of two genes important for sex determination in freshwater turtles (Mizoguchi et al., 2022). However, it is likely that other (unmeasured) contaminants may also contribute to the effects reported here. As contaminant mixtures and temperature can have interactive estrogenic effects, the risk for hatchling feminization from contaminants can be a combination of maternal contaminant exposure from foraging and nesting site temperatures. In vitro bioassays were a useful tool for assessing organic contaminant exposure in hatchling organ tissue. Many of the contaminants found in the current study have demonstrated negative health effects in previous research among multiple sea turtle species. Considering the risks of feminization due to climate change and the potential negative health effects of anthropogenic contaminants, the possibility that hatchling development is disrupted by anthropogenic contaminants should be investigated further.
The original contributions presented in the study are included in the article/Supplementary Material. Further inquiries can be directed to the corresponding author.
The animal study was approved by Griffith University Animal Ethics Committee. The study was conducted in accordance with the local legislation and institutional requirements.
AB, KF, FL, and JM contributed to the writing, and conception of this submission. AB contributed to the data analysis. LY, CS, CH, and DB contributed to the data collection of this submission. All authors contributed to the article and approved the submitted version.
AB was supported by the Griffith University Postgraduate Research Scholarship, the Griffith University International Postgraduate Research Scholarship, and the Griffith University Completion Assistance Postgraduate Research Scholarship. We thank the World Wildlife Fund for Nature – Australia (WWF-AU) and its donor, Koala.com, for funding this study as part of the WWF-led ‘Turtle Cooling Project”.
The authors would like to thank Melissa Staines for their assistance in the field as well as Natali Marinovski, and the various Griffith University volunteers for their assistance in the laboratory.
The authors declare that the research was conducted in the absence of any commercial or financial relationships that could be construed as a potential conflict of interest.
All claims expressed in this article are solely those of the authors and do not necessarily represent those of their affiliated organizations, or those of the publisher, the editors and the reviewers. Any product that may be evaluated in this article, or claim that may be made by its manufacturer, is not guaranteed or endorsed by the publisher.
The Supplementary Material for this article can be found online at: https://www.frontiersin.org/articles/10.3389/fmars.2023.1238837/full#supplementary-material
Abraham J. P., Baringer M., Bindoff N. L., Boyer T., Cheng L. J., Church J. A., et al. (2013). A review of global ocean temperature observations: Implications for ocean heat content estimates and climate change. Rev. Geophys. 51, 450–483. doi: 10.1002/rog.20022
Adeel M., Song X., Wang Y., Francis D., Yang Y. (2017). Environmental impact of estrogens on human, animal and plant life: A critical review. Environ. Int. 99, 107–119. doi: 10.1016/j.envint.2016.12.010
Al-Rawahy S. H., AlKindi A. Y., Elshafie A., Ibrahim M., Al Bahry S. N., Al Siyabi S. S., et al. (2007). Accumulation of metals in the egg yolk and liver of hatchling of green turtles Chelonia mydas at Ras Al Hadd, Sultanate of Oman. J. Biol. Sci. 7, 925–930. doi: 10.3923/jbs.2007.925.930
Anastassiades M., Lehotay S. J., Štajnbaher D., Schenck F. J. (2003). Fast and easy multiresidue method employing acetonitrile extraction/partitioning and “dispersive solid-phase extraction” for the determination of pesticide residues in produce. J. AOAC. Int. 86, 412–431. doi: 10.1093/jaoac/86.2.412
Barraza A. D., Finlayson K. A., Leusch F. D. L., van de Merwe J. P. (2021). Systematic review of reptile reproductive toxicology to inform future research directions on endangered or threatened species, such as sea turtles. Environ. Pollut. 286, 117470. doi: 10.1016/j.envpol.2021.117470
Beldomenico P. M., Rey F., Prado W. S., Villarreal J. C., Muñoz-de-Toro M., Luque E. H. (2007). In ovum exposure to pesticides increases the egg weight loss and decreases hatchlings weight of Caiman latirostris (Crocodylia: Alligatoridae). Ecotoxicol. Environ. Saf. 68, 246–251. doi: 10.1016/j.ecoenv.2006.12.018
Bergeron J. M., Crews D., McLachlan J. A. (1994). PCBs as environmental estrogens: turtle sex determination as a biomarker of environmental contamination. Environ. Health Perspect. 102, 780–781. doi: 10.1289/ehp.94102780
Blechschmidt J., Wittmann M. J., Blüml C. (2020). Climate change and green sea turtle sex ratio—preventing possible extinction. Genes (Basel). 11. doi: 10.3390/genes11050588
Boggs A. S. P., Botteri N. L., Hamlin H. J., Guillette L. J. (2011). “Endocrine disruption of reproduction in reptiles,” in Hormones and Reproduction of Vertebrates. 3, 373–396. doi: 10.1016/B978-0-12-374930-7.10014-7
Booth D. T., Astill K. (2001). Incubation temperature, energy expenditure and hatchling size in the green turtle (Chelonia mydas), a species with temperature-sensitive sex determination. Aust. J. Zool. 49, 389–396. doi: 10.1071/ZO01006
Booth D. T., Burgess E., McCosker J., Lanyon J. M. (2004). The influence of incubation temperature on post-hatching fitness characteristics of turtles. Int. Congr. Ser. 1275, 226–233. doi: 10.1016/j.ics.2004.08.057
Booth D. T., Turner A. G., Laloë J., Limpus C. J. (2022). How well do embryo development rate models derived from laboratory data predict embryo development in sea turtle nests? J. Exp. Zool. Part A. Ecol. Integr. Physiol. 337, 516–526. doi: 10.1002/jez.2585
Bowen B. W., Meylan A. B., Ross J. P., Limpus C. J., Balazs G. H., Avise J. C. (1992). Global population structure and natural history of the green turtle (Chelonia mydas) in terms of matriarchal phylogeny. Evol. (N. Y). 46, 865–881. doi: 10.2307/2409742
Burgess E. A., Booth D. T., Lanyon J. M. (2006). Swimming performance of hatchling green turtles is affected by incubation temperature. Coral Reefs 25, 341–349. doi: 10.1007/s00338-006-0116-7
Bustard R., Greenman P. (1967). Physical and chemical factors affecting hatching in the green sea turtle, Chelonia mydas (L.). Ecol. Soc Am. 49, 269–276. doi: 10.2307/1934455
Canesini G., Stoker C., Galoppo G. H., Durando M. L., Tschopp M. V., Luque E. H., et al. (2018). Temperature- vs. estrogen-induced sex determination in Caiman latirostris embryos: Both females, but with different expression patterns of key molecules involved in ovarian development. Gen. Comp. Endocrinol. 259, 176–188. doi: 10.1016/j.ygcen.2017.11.024
Chakraborty S. B. (2021). Non-essential heavy metals as endocrine disruptors: evaluating impact on reproduction in teleosts. Proc. Zool. Soc 74, 417–431. doi: 10.1007/s12595-021-00399-x
Choe S. Y., Kim S. J., Kim H. G., Lee J. H., Choi Y., Lee H., et al. (2003). Evaluation of estrogenicity of major heavy metals. Sci. Total. Environ. 312, 15–21. doi: 10.1016/S0048-9697(03)00190-6
Cortes-Gomez A. A., Romero D., Girondot M. (2017). The current situation of inorganic elements in marine turtles: A general review and meta-analysis. Environ. Pollut. 229, 567–585. doi: 10.1016/j.envpol.2017.06.077
Crain D. A., Guillette L. J., Rooney A. A., Pickford D. B. (1997). Alterations in steroidogenesis in alligators (Alligator mississippiensis) exposed naturally and experimentally to environmental contaminants. Environ. Health Perspect. 105, 528–533. doi: 10.1289/ehp.97105528
Dennis M. M., Poppenga R., Conan A., Hill K., Hargrave S., Maroun V., et al. (2020). Leatherback sea turtle (Dermochelys coriacea) hatch success and essential and nonessential metals in eggs and embryos from nests in St. Kitts, (2015). Mar. Pollut. Bull. 161, 111726. doi: 10.1016/j.marpolbul.2020.111726
Dogruer G., Weijs L., Tang J. Y., Hollert H., Kock M., Bell I., et al. (2018). Effect-based approach for screening of chemical mixtures in whole blood of green turtles from the Great Barrier Reef. Sci. Total. Environ. 612, 321–329. doi: 10.1016/j.scitotenv.2017.08.124
Escher B., Neale P. A., Leusch F. D. L. (2021). Bioanalytical Tools in Water Quality Assessment, Water Intelligence Online. (New York and London: IWA publishing). doi: 10.2166/9781843393689
Finlayson K. A., Leusch F. D. L., van de Merwe J. P. (2016). The current state and future directions of marine turtle toxicology research. Environ. Int. 94, 113–123. doi: 10.1016/j.envint.2016.05.013
Finlayson K. A., Leusch F. D. L., Limpus C. J., van de Merwe J. P. (2019a). Towards the development of standardised sea turtle primary cell cultures for toxicity testing. Ecotoxicol. Environ. Saf. 173, 63–70. doi: 10.1016/j.ecoenv.2019.01.117
Finlayson K. A., Leusch F. D. L., van de Merwe J. P. (2019b). Cytotoxicity of organic and inorganic compounds to primary cell cultures established from internal tissues of Chelonia mydas. Sci. Total. Environ. 664, 958–967. doi: 10.1016/j.scitotenv.2019.02.052
Finlayson K. A., Leusch F. D. L., Villa C. A., Limpus C. J., van de Merwe J. P. (2021). Combining analytical and in vitro techniques for comprehensive assessments of chemical exposure and effect in green sea turtles (Chelonia mydas). Chemosphere 274, 129752. doi: 10.1016/j.chemosphere.2021.129752
Finlayson K. A., Limpus C. J., van de Merwe J. P. (2022). Temporal changes in chemical contamination of green turtles (Chelonia mydas) foraging in a heavily industrialised seaport. Sci. Total. Environ. 817, 152848. doi: 10.1016/j.scitotenv.2021.152848
Finlayson K. A., Madden Hof C. A., van de Merwe J. P. (2020). Development and application of species-specific cell-based bioassays to assess toxicity in green sea turtles. Sci. Total. Environ. 747, 142095. doi: 10.1016/j.scitotenv.2020.142095
Finlayson K. A., van de Merwe J. P. (2021). Differences in marine megafauna in vitro sensitivity highlights the need for species-specific chemical risk assessments. Aquat. Toxicol. 239, 105939. doi: 10.1016/j.aquatox.2021.105939
Fleming K. A., Perrault J. R., Stacy N. I., Coppenrath C. M., Gainsbury A. M. (2020). Heat, health and hatchlings: Associations of in situ nest temperatures with morphological and physiological characteristics of loggerhead sea turtle hatchlings from Florida. Conserv. Physiol. 8, 1–17. doi: 10.1093/conphys/coaa046
Fuentes M. M. P. B., Limpus C. J., Hamann M. (2011). Vulnerability of sea turtle nesting grounds to climate change. Glob. Change Biol. 17, 140–153. doi: 10.1111/j.1365-2486.2010.02192.x
Gárriz Á., Miranda L. A. (2020). Effects of metals on sperm quality, fertilization and hatching rates, and embryo and larval survival of pejerrey fish (Odontesthes bonariensis). Ecotoxicology 29, 1072–1082. doi: 10.1007/s10646-020-02245-w
Georges A., Limpus C., Stoutjesdijk R. (1994). Hatchling sex in the marine turtle Caretta caretta is determined by proportion of development at a temperature, not daily duration of exposure. J. Exp. Zool. 270, 432–444. doi: 10.1002/jez.1402700504
Guirlet E., Das K., Thomé J. P., Girondot M. (2010). Maternal transfer of chlorinated contaminants in the leatherback turtles, Dermochelys coriacea, nesting in French Guiana. Chemosphere 79, 720–726. doi: 10.1016/j.chemosphere.2010.02.047
Hamann M., Godfrey M. H., Seminoff J. A., Arthur K., Barata P. C. R., Bjorndal K. A., et al. (2010). Global research priorities for sea turtles: Informing management and conservation in the 21st century. Endanger. Species. Res. 11, 245–269. doi: 10.3354/esr00279
Hawkes L. A., Broderick A. C., Godfrey M. H., Godley B. J. (2009). Climate change and marine turtles. Endanger. Species. Res. 7, 137–154. doi: 10.3354/esr00198
Hays G. C., Laloë J. O., Lee P. L. M., Schofield G. (2023). Evidence of adult male scarcity associated with female-skewed offspring sex ratios in sea turtles. Curr. Biol. 33, R14–R15. doi: 10.1016/j.cub.2022.11.035
Helsel D. R. (2012). Statistics for Censored Environmental Data. (Hoboken New Jersey: John Wiley & Sons, Inc.).
Ikonomopoulou M. P., Olszowy H., Hodge M., Bradley A. J. (2009). The effect of organochlorines and heavy metals on sex steroid-binding proteins in vitro in the plasma of nesting green turtles, Chelonia mydas. J. Comp. Physiol. B. 179, 653–662. doi: 10.1007/s00360-009-0347-3
Jacobson T., Prevodnik A., Sundelin B. (2007). Combined effects of temperature and a pesticide on the baltic amphipod Monoporeia affinis. Aquat. Biol. 1, 269–276. doi: 10.3354/ab00028
Jensen M. P., Allen C. D., Eguchi T., Bell I. P., LaCasella E. L., Hilton W. A., et al. (2018). Environmental warming and feminization of one of the largest sea turtle populations in the world. Curr. Biol. 28, 154–159 e4. doi: 10.1016/j.cub.2017.11.057
Kaska Y., Furness R. W. (2001). Heavy metals in marine turtle eggs and hatchlings in the mediterranean. Zool. Middle. East. 24, 127–132. doi: 10.1080/09397140.2001.10637891
Keller J. M. (2003). Occurrence and effects of organochlorine contaminants in sea turtles (University Microfilms).
Kwon B., Ha N., Jung J., Kim P. G., Kho Y., Choi K., et al. (2016). Effects of barium chloride exposure on hormones and genes of the hypothalamic-pituitary-gonad axis, and reproduction of zebrafish (Danio rerio). Bull. Environ. Contam. Toxicol. 96, 341–346. doi: 10.1007/s00128-016-1731-9
Lecomte S., Habauzit D., Charlier T. D., Pakdel F. (2017). Emerging estrogenic pollutants in the aquatic environment and breast cancer. Genes (Basel) 8. doi: 10.3390/genes8090229
Leusch F. D. L., Moore M. R., Chapman H. F. (2009). Balancing the budget of environmental estrogen exposure: the contribution of recycled water. Water Sci. Technol. 60, 1003–1012. doi: 10.2166/wst.2009.398
Limpus C. J., Milier J. D., Parmenter C. J., Reimer D., McLachlan N., Webb R. (1992). Migration of green (Chelonia mydas) and loggerhead (Caretta caretta) turtles to and from eastern Australian rookeries. Wildl. Res. 19, 347–357. doi: 10.1071/WR9920347
Loarie S. R., Duffy P. B., Hamilton H., Asner G. P., Field C. B., Ackerly D. D. (2009). The velocity of climate change. Nature 462, 1052–1055. doi: 10.1038/nature08649
Lolavar A., Wyneken J. (2021). Effects of supplemental watering on loggerhead (Caretta caretta) nests and hatchlings. J. Exp. Mar. Bio. Ecol. 534, 151476. doi: 10.1016/j.jembe.2020.151476
Matsumoto Y., Hannigan B., Crews D. (2014). Embryonic PCB exposure alters phenotypic, genetic, and epigenetic profiles in turtle sex determination, A biomarker of environmental contamination. Endocrinology 155, 4168–4177. doi: 10.1210/en.2014-1404
Matthews J., Celius T., Halgren R., Zacharewski T. (2000). Differential estrogen receptor binding of estrogenic substances: a species comparison. J. Steroid Biochem. Mol. Biol. 74, 223–234. doi: 10.1016/S0960-0760(00)00126-6
Matthews J., Zacharewski T. (2000). Differential binding affinities of PCBs, HO-PCBs, and aroclors with recombinant human, rainbow trout (Onchorhynkiss mykiss), and green anole (Anolis carolinensis) estrogen receptors, using a semi-high throughput competitive binding assay. Toxicol. Sci. 53, 326–339. doi: 10.1093/toxsci/53.2.326
Meylan A. B., Bowsen B. W., Avise J. C. (1990). A genetic test of the natal homing versus social facilitation models for green turtle migration. Mol. Ecol. Evol. Org. Side. Sel. Writings Avis. Lab. 248, 71–74. doi: 10.1142/9789814317764_0001
Mihucz V. G., Záray G. (2016). Occurrence of antimony and phthalate esters in polyethylene terephthalate bottled drinking water. Appl. Spectrosc. Rev. 51, 163–189. doi: 10.1080/05704928.2015.1105243
Miller J. D., Limpus C. J. (1981). Incubation period and sexual differentiation in the green turtle Chelonia mydas (L.), in: Proceedings of the Melbourne Herpetological Symposium (Australia: The Zoological Board of Victoria Melbourne), 66–73.
Mizoguchi B., Topping N. E., Lavin A. M., Valenzuela N. (2022). Cadmium ecotoxic effects on embryonic Dmrt1 and aromatase expression in chrysemys picta turtles may implicate changes in DNA methylation. Genes (Basel). 13. doi: 10.3390/genes13081318
Myllynen K., Ojutkangas E., Nikinmaa M. (1997). River water with high iron concentration and low pH causes mortality of lamprey roe and newly hatched larvae. Ecotoxicol. Environ. Saf. 36, 43–48. doi: 10.1006/eesa.1996.1484
Páez-Osuna F., Calderón-Campuzano M. F., Soto-Jiménez M. F., Ruelas-Inzunza J. R. (2010). Lead in blood and eggs of the sea turtle, Lepidochelys olivacea, from the Eastern Pacific: Concentration, isotopic composition and maternal transfer. Mar. Pollut. Bull. 60, 433–439. doi: 10.1016/j.marpolbul.2009.10.004
Page-Karjian A., Stacy N. I., Morgan A. N., Coppenrath C. M., Manire C. A., Herbst L. H., et al. (2022). Morphologic and physiologic characteristics of green sea turtle (Chelonia mydas) hatchlings in southeastern Florida, USA. J. Comp. Physiol. B. Biochem. Syst. Environ. Physiol. 192, 751–764. doi: 10.1007/s00360-022-01450-9
Perrault J. R., Bauman K. D., Greenan T. M., Blum P. C., Henry M. S., Walsh C. J. (2016). Maternal transfer and sublethal immune system effects of brevetoxin exposure in nesting loggerhead sea turtles (Caretta caretta) from western Florida. Aquat. Toxicol. 180, 131–140. doi: 10.1016/j.aquatox.2016.09.020
Perrault J., Wyneken J., Thompson L. J., Johnson C., Miller D. L. (2011). Why are hatching and emergence success low? Mercury and selenium concentrations in nesting leatherback sea turtles (Dermochelys coriacea) and their young in Florida. Mar. Pollut. Bull. 62, 1671–1682. doi: 10.1016/j.marpolbul.2011.06.009
Podreka S., Georges A., Maher B., Limpus C. J. (1998). The environmental contaminant DDE fails to influence the outcome of sexual differentiation in the marine turtle Chelonia mydas. Environ. Health Perspect. 106, 185–188. doi: 10.1289/ehp.98106185
Porter E., Booth D. T., Limpus C. J., Staines M. N., Smith C. E. (2021). Influence of short-term temperature drops on sex-determination in sea turtles. J. Exp. Zool. Part A. Ecol. Integr. Physiol. 335, 649–658. doi: 10.1002/jez.2509
Rousch J. M., Simmons T. W., Kerans B. L., Smith B. P. (1997). Relative acute effects of low pH and high iron on the hatching and survival of the water mite (Arrenurus manubriator) and the aquatic insect (Chironomus riparius). Environ. Toxicol. Chem. 16, 2144–2150. doi: 10.1897/1551-5028(1997)016<2144:RAEOLP>2.3.CO;2
Salice C. J., Rowe C. L., Eisenreich K. M. (2014). Integrative demographic modeling reveals population level impacts of PCB toxicity to juvenile snapping turtles. Environ. pollut. 184, 154–160. doi: 10.1016/j.envpol.2013.08.031
Santidrián Tomillo P., Genovart M., Paladino F. V., Spotila J. R., Oro D. (2015). Climate change overruns resilience conferred by temperature-dependent sex determination in sea turtles and threatens their survival. Glob. Change Biol. 21, 2980–2988. doi: 10.1111/gcb.12918
Santidrián Tomillo P., Spotila J. R. (2020). Temperature-dependent sex determination in sea turtles in the context of climate change: uncovering the adaptive significance. BioEssays 42, 1–6. doi: 10.1002/bies.202000146
Smith C., Booth D., Crosby A., Miller J., Staines M., Versace H., et al. (2021). Trialling seawater irrigation to combat the high nest temperature feminisation of green turtle Chelonia mydas hatchlings. Mar. Ecol. Prog. Ser. 667, 177–190. doi: 10.3354/meps13721
Souza N. L. N., Carneiro M. T. W. D., Pimentel E. F., Frossard A., Freire J. B., Endringer D. C., et al. (2018). Trace elements influence the hatching success and emergence of Caretta caretta and Chelonia mydas. J. Trace Elem. Med. Biol. 50, 117–122. doi: 10.1016/j.jtemb.2018.06.007
Tanabe L. K., Scott K., Dasari V., Berumen M. L. (2022). An assessment of heavy metals in green sea turtle (Chelonia mydas) hatchlings from Saudi Arabia’s largest rookery, Ras Baridi. PeerJ 10. doi: 10.7717/peerj.13928
Tanner C. E., Marco A., Martins S., Abella-Perez E., Hawkes L. A. (2019). Highly feminised sex-ratio estimations for the world’s third-largest nesting aggregation of loggerhead sea turtles. Mar. Ecol. Prog. Ser. 621, 209–219. doi: 10.3354/meps12963
Valenzuela N., Lance V. (2004). “Temperature-Dependent sex determination,” in Reptilian Incubation: Environment, Evolution, and Behavior. (Smithsonian Books), 211–227.
van de Merwe J. P., Hodge M., Olszowy H. A., Whittier J. M., Ibrahim K., Lee S. Y. (2009). Chemical contamination of green turtle (Chelonia mydas) eggs in Peninsular Malaysia: Implications for conservation and public health. Environ. Health Perspect. 117, 1397–1401. doi: 10.1289/ehp.0900813
van de Merwe J. P., Hodge M., Whittier J. M., Ibrahim K., Lee S. Y. (2010). Persistent organic pollutants in the green sea turtle Chelonia mydas: Nesting population variation, maternal transfer, and effects on development. Mar. Ecol. Prog. Ser. 403, 269–278. doi: 10.3354/meps08462
van de Merwe J., Ibrahim K., Whittier J. (2006). Effects of nest depth, shading, and metabolic heating on nest temperatures in sea turtle hatcheries. Chelonian. Conserv. Biol. 5, 210–215. doi: 10.2744/1071-8443(2006)5[210:EONDSA]2.0.CO;2
Villa C. A., Flint M., Bell I., Hof C., Limpus C. J., Gaus C. (2016). Trace element reference intervals in the blood of healthy green sea turtles to evaluate exposure of coastal populations. Environ. Pollut. 220, 1465–1476. doi: 10.1016/j.envpol.2016.10.085
Warner D. A. (2011). Sex determination in reptiles, in: Hormones and Reproduction of Vertebrates. Vol. 3 (Elsevier), 1–38. doi: 10.1016/B978-0-12-374930-7.10001-9
Warner D. A., Mitchell T. S., Bodensteiner B. L., Janzen F. J. (2017). The effect of hormone manipulations on sex ratios varies with environmental conditions in a turtle with temperature-dependent sex determination. J. Exp. Zool. Part A. Ecol. Integr. Physiol. 327, 172–181. doi: 10.1002/jez.2085
Wibbels T., Bull J. J., Crews D. (1991). Synergism between temperature and estradiol: A common pathway in turtle sex determination? J. Exp. Zool. 260, 130–134. doi: 10.1002/jez.1402600117
Wyneken J., Lolavar A. (2015). Loggerhead sea turtle environmental sex determination: Implications of moisture and temperature for climate change based predictions for species survival. J. Exp. Zool. Part B. Mol. Dev. Evol. 324, 295–314. doi: 10.1002/jez.b.22620
Keywords: organic contaminant, sea turtle, sex ratio, trace elements, sex determination
Citation: Barraza AD, Young L, Smith CE, Booth DT, Hof CAM, Finlayson KA, Leusch FDL and van de Merwe JP (2023) Exploring contaminants as a disruptor of temperature-dependent sex determination in sea turtle hatchlings. Front. Mar. Sci. 10:1238837. doi: 10.3389/fmars.2023.1238837
Received: 12 June 2023; Accepted: 17 October 2023;
Published: 13 November 2023.
Edited by:
Andrea D. Phillott, Flame University, IndiaReviewed by:
Adriana A. Cortés-Gómez, Systématique et Évolution (ESE), FranceCopyright © 2023 Barraza, Young, Smith, Booth, Hof, Finlayson, Leusch and van de Merwe. This is an open-access article distributed under the terms of the Creative Commons Attribution License (CC BY). The use, distribution or reproduction in other forums is permitted, provided the original author(s) and the copyright owner(s) are credited and that the original publication in this journal is cited, in accordance with accepted academic practice. No use, distribution or reproduction is permitted which does not comply with these terms.
*Correspondence: Arthur D. Barraza, QXJ0aHVyLkJhcnJhemFAZ3JpZmZpdGh1bmkuZWR1LmF1
Disclaimer: All claims expressed in this article are solely those of the authors and do not necessarily represent those of their affiliated organizations, or those of the publisher, the editors and the reviewers. Any product that may be evaluated in this article or claim that may be made by its manufacturer is not guaranteed or endorsed by the publisher.
Research integrity at Frontiers
Learn more about the work of our research integrity team to safeguard the quality of each article we publish.