- Department of Ecology, Evolution, and Marine Biology, University of California Santa Barbara, Santa Barbara, CA, United States
Kelp forests of the California Current System have experienced prolonged marine heatwave (MHW) events that overlap in time with the phenology of life history events (e.g., gametogenesis and spawning) of many benthic marine invertebrates. To study the effect of thermal stress from MHWs during gametogenesis in the purple sea urchin (Strongylocentrotus purpuratus) and further, whether MHWs might induce transgenerational plasticity (TGP) in thermal tolerance of progeny, adult urchins were acclimated to two conditions in the laboratory – a MHW temperature of 18°C and a non-MHW temperature of 13°C. Following a four-month long acclimation period (October–January), adults were spawned and offspring from each parental condition were reared at MHW (18°C) and non-MHW temperatures (13°C), creating a total of four embryo treatment groups. To assess transgenerational effects for each of the four groups, we measured thermal tolerance of hatched blastula embryos in acute thermal tolerance trials. Embryos from MHW-acclimated females were more thermally tolerant with higher LT50 values as compared to progeny from non-MHW-acclimated females. Additionally, there was an effect of female acclimation state on offspring body size at two stages of embryonic development - early gastrulae and prism, an early stage echinopluteus larvae. To assess maternal provisioning as means to also alter embryo performance, we assessed gamete traits from the differentially acclimated females, by measuring size and biochemical composition of eggs. MHW-acclimated females had eggs with higher protein concentrations, while egg size and lipid content showed no differences. Our results indicate that TGP plays a role in altering the performance of progeny as a function of the thermal history of the female, especially when thermal stress coincides with gametogenesis. In addition, the data on egg provisioning show that maternal experience can influence embryo traits via egg protein content. Although this is a laboratory-based study, the results suggest that TGP may play a role in the resistance and tolerance of S. purpuratus early stages in the natural kelp forest setting.
1 Introduction
Marine heatwave (MHW) events—defined as discrete periods of anomalously high sea surface temperatures (Hobday et al., 2016)—have emerged over the last decade as extreme climatic disturbance events which can occur at large spatial and temporal scales (Hobday et al., 2018; Oliver et al., 2021). Globally, MHWs have had significant effects on coastal marine ecosystems, ranging from mass mortality events (Cavole et al., 2016; Hughes et al., 2017; Wernberg, 2021; Garrabou et al., 2022), species range shifts (Lonhart et al., 2019; Sanford et al., 2019), and changes in biodiversity (Rogers-Bennett and Catton, 2019; Hart et al., 2020; Weitzman et al., 2021; Michaud et al., 2022) to effects on organismal processes such as reproduction (Leach et al., 2021; Minuti et al., 2021) and development (Leung et al., 2017; Tanner et al., 2020; Clare et al., 2022; Minuti et al., 2022). Because recent research indicates that MHWs are expected to increase in frequency and duration in the future (Oliver et al., 2018; Guo et al., 2022; Jacox et al., 2022), understanding how these events will impact species, especially at their most temperature-sensitive stages, will provide critical insight into how MHWs might alter population and community structure. Specifically, research on the timing of MHWs relative to the timing of critical life history events is an important consideration when exploring the physiological mechanisms by which environmental thermal stress might impact organismal performance.
Notably, for organisms with complex life histories, such as marine invertebrates, early developmental stages are often the most vulnerable to environmental stress, with early embryos and juveniles showing the most sensitivity across taxa (Pandori and Sorte, 2018). For many benthic marine invertebrates, there are significant knowledge gaps in three areas – how early stages will respond to the acute thermal stress from MHWs, the physiological mechanisms that confer resilience, and lastly, how the timing of MHWs in nature align with the timing of life history events. Mechanistically, the thermal tolerance of embryos and larvae can be influenced via inherent plasticity expressed during development, or alternatively via parentally-sourced mechanisms. Here, maternal provisioning of eggs and/or transgenerational plasticity (TGP) could transduce tolerance to the embryo as a function of adult environmental history (Donelson et al., 2016). As part of this special topic, we present the results from a laboratory experiment that mimicked MHW patterns observed at our kelp forest study site, and acclimated adult purple sea urchins (Strongylocentrotus purpuratus) in the laboratory at a time that coincided with gametogenesis in local natural populations. We then tested the hypothesis that adult thermal experience would influence the tolerance of progeny via TGP. To investigate maternal investment as a specific mechanism of TGP, we examined whether changes in egg provisioning would correlate to the thermal acclimation treatment of the adults.
Our laboratory conditions mimicked a major MHW event that occurred in our region, the extreme event also known as ‘the Blob’. This notable MHW event occurred from January 2014 – August 2016 (Gentemann et al., 2017), and, during this time, sea surface temperature (SST) increased an average of 1- 4°C (Cavole et al., 2016; Di Lorenzo and Mantua, 2016). This event had drastic impacts in the Northeast Pacific from the Gulf of Alaska to Baja California that ranged across levels of biological organization (Cavole et al., 2016; Arafeh-Dalmau et al., 2019). Regionally, in the Santa Barbara Channel (SBC) and the local kelp forests, this event has had lasting effects on the kelp forest community, altering local biodiversity (Michaud et al., 2022), interacting with disease (Reed et al., 2016), and changing the quality of kelp as food (Lowman et al., 2022). Since the Blob, recurrence of shorter term MHW events are continuing to be documented (Leach et al., 2021) raising concern for the health of these productive coastal ecosystems going into the future.
Determining how kelp forest ecosystems will be impacted by MHWs will be dependent on our understanding of organismal responses of ecologically important species. In kelp forests, S. purpuratus is a dominant herbivorous grazer capable of transforming ecosystems (Pearse, 2006; Smith and Tinker, 2022). Vulnerability to elevated temperatures can vary throughout the sea urchin life cycle, where early developmental stages are often the most sensitive to environmental stress (Byrne, 2011). While increases in environmental temperatures can have a positive effect on early stages by increasing growth and rate of development, thus reducing planktonic larval duration, if temperatures exceed upper thermal thresholds, early forms can experience an increase in abnormal development and/or mortality (Byrne, 2011). Adults are generally less susceptible than embryos to warming in sea urchin species, however heat stress associated with MHWs has shown to have lasting effects on urchin survival, immune response, and reproduction (Shanks et al., 2020; Minuti et al., 2021). From an ecological development perspective (Sultan, 2007), large parts of the purple urchin’s reproductive cycle take place when MHWs occur in the SBC (Figure 1). MHWs have tended to occur in the late summer to early winter, a time period that coincides with the period of gametogenesis in purple sea urchins (Cochran and Engelmann, 1975; Strathmann, 1987) (Figure 1). In addition, S. purpuratus spawns in this region from December – February (G. Hofmann, pers. observ.). Thus, the overlap between timing of MHWs and gametogenesis in purple urchins makes this species an ideal candidate for examining mechanisms of parental effects in response to local MHWs. From an experimental physiology perspective, this temporal alignment in nature renders parental acclimation in the laboratory during gametogenesis a way to explore whether the thermal history of adults influences the tolerance of their early-stage progeny.
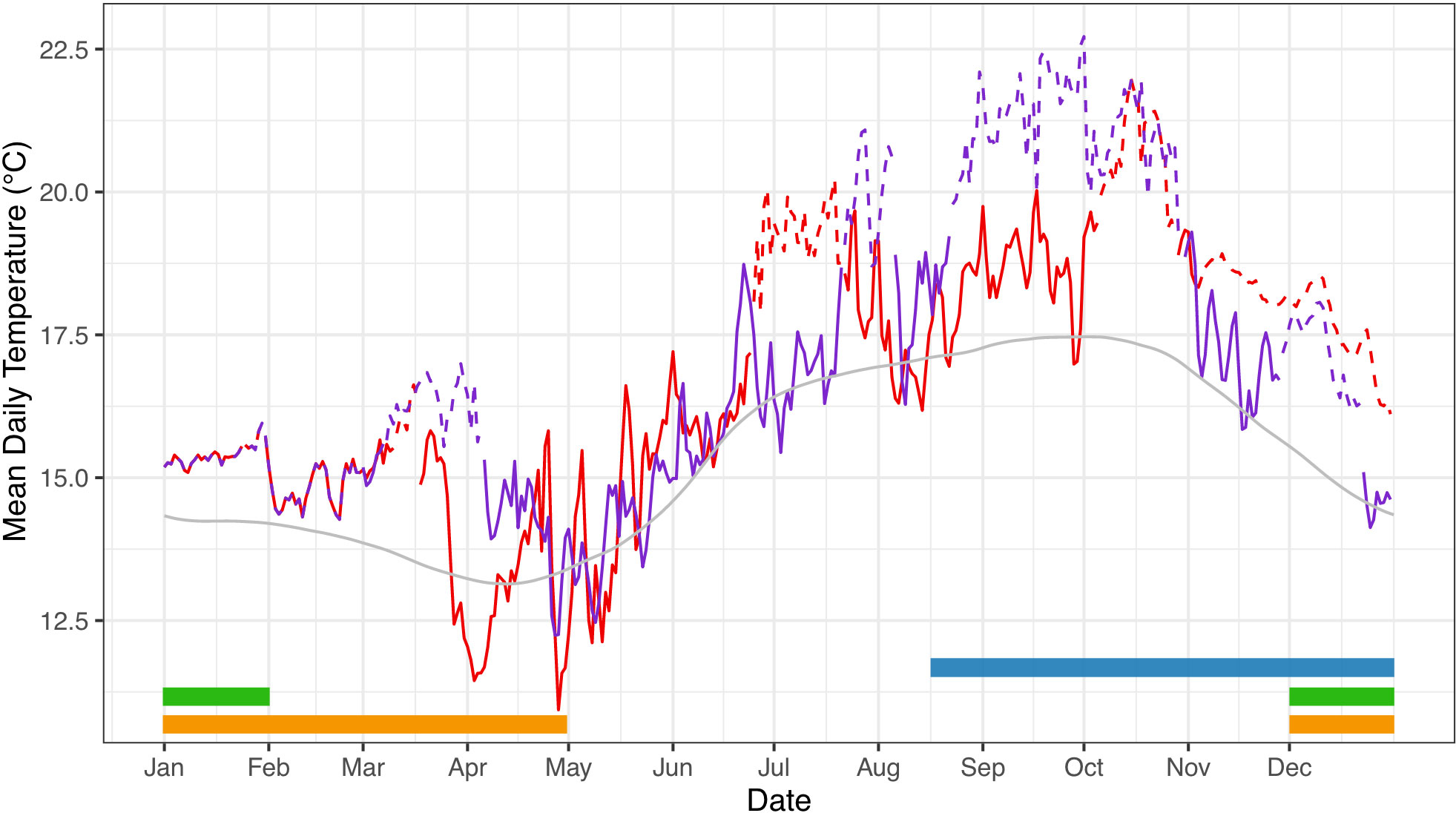
Figure 1 Mean daily temperature experienced by adult purple sea urchins during the 2014-2015 MHW. Temperature data were collected on the benthos using ONSET HOBO temperature loggers by the Santa Barbara Coastal LTER. The red and purple lines represent 2014 and 2015, respectively; the grey line represents the climatological mean (2001-2021). The occurrence of a MHW event, identified using metrics established by Hobday et al. (2016), is indicated by a dashed line for both 2014 (red) and 2015 (purple). Timing of life history events for S. purpuratus are represented by colored bars: gametogenesis (blue), spawning (green), and planktonic larval duration (yellow) (Rogers-Bennett, 2013; Okamoto et al., 2020).
Transgenerational plasticity, which refers to modification of offspring phenotype induced by signals experienced by the parents, is well documented in marine taxa (Donelson et al., 2016; Zhao et al., 2020; Truong et al., 2022). In general, TGP has been studied extensively in marine invertebrates with mixed results depending on taxa and the context of the environmental factor in question (Byrne et al., 2020). At present, TGP or “carry-over” effect studies in a MHW context (i.e., where the temperature regime in an experiment matches that of actual temperature patterns during local MHWs) are few, but emerging data are providing important insight into how thermal stress from MHWs interacts with the phenology of life history events (Gall et al., 2021; Leach et al., 2021; Minuti et al., 2022).
In this study, we examined TGP as a mechanism to increase physiological performance during critical developmental stages of S. purpuratus when exposed to prolonged MHW conditions. Specifically, our aim was to investigate maternal effects in a benthic marine invertebrate that routinely experiences MHWs in situ and ask whether exposure to MHW temperatures during oogenesis in female urchins induced a transgenerational response, or carry-over effect, when exposed to a simulated prolonged MHW in the laboratory. For this study, adult purple sea urchins were acclimated under two conditions – temperatures that mimicked average MHW conditions typical of the 2014-2016 MHW in the SBC (18°C), and a non-MHW average habitat temperature (13°C) during the period of gametogenesis. Following a four-month acclimation period, adults were spawned and offspring from each maternal acclimation treatment (MHW vs. non-MHW temperature) were reared reciprocally at MHW temperatures (18°C), and non-MHW temperatures (13°C). To test for evidence of TGP in this reciprocal rearing design, we assessed multiple traits throughout early development including thermal tolerance and measurements of body size. To assess the potential for maternal investment as a mechanism or TGP we measured egg traits such as egg size and biochemical composition. The results contribute to our understanding of how ecologically important species of kelp forest ecosystems respond to acute thermal stress from MHWs.
2 Materials and methods
2.1 Animal collection and adult conditioning
Adult purple sea urchins, Strongylocentrotus purpuratus (Stimpson, 1857), were collected on SCUBA subtidally near Goleta Bay, CA, in September 2020. Urchins were collected under the California Department of Fish and Wildlife Scientific Collecting permit #SC-9228 to the Santa Barbara Coastal LTER, and all protocols followed the guidelines of animal care and use at University of California, Santa Barbara. The mean temperature at the site was 16.8 ± 0.9°C on the day of collection. Following collection, urchins were held in a flow-through seawater table at ambient temperature (~16.4°C) for 24 hours before being placed in the adult acclimation treatments. During this period urchins were monitored for any disease or premature spawning and removed if needed.
To determine how maternal conditioning influenced provisioning and subsequent offspring performance, adult urchins were acclimated in either a warm (W: ~18.3°C) or cool (C: ~13.1°C) temperature condition representing MHW and non-MHW conditions, respectively (see Supplementary Material Figure 1). Urchins were held in the acclimation treatments from October 2020 to January 2021, which coincides with the window of gametogenesis for S. purpuratus populations in the SBC (Strathmann, 1987). Adult acclimation treatments consisted of three 37L replicate tanks held in a seawater table, acting as a water bath, to maintain temperature. Seawater was supplied to the treatment tanks via a dripper irrigation system with a flow rate of 12L/hr. The temperature of the seawater table was controlled using a Delta Star® heat pump with a Nema 4x digital temperature controller (AquaLogic, San Diego, CA, USA). The temperature of each tank was monitored daily using a wire thermocouple (Omega HH81a). Aquarium pumps (Aqua-Supreme) were installed in each tank to maintain proper water flow. Ten adult urchins were randomly selected and added to each treatment tank at the beginning of the acclimation period (N=60 total, 10 urchins per tank replicate for each temperature condition) (Figure 2). Each week seven fronds of kelp (Macrocystis pyrifera) were added to each tank and urchins fed ab libitum. Urchins were inspected daily, and any individuals that showed signs of disease or death were removed.
2.2 Urchin spawning and larval culturing
Following the four-month conditioning period, urchins were induced to spawn by injecting 0.53M KCl into the coelom (Strathmann, 1987). Sperm was collected “dry” via pipette, and then stored on ice in 1.5mL microcentrifuge tubes until activated. Eggs were collected by inverting females over a beaker containing UV sterilized 0.35micron filtered seawater (FSW). Sperm and egg quality were checked under a compound microscope. Females with the highest quality eggs (determined via visual inspection of size, shape, and transparency) from each adult acclimation treatment were selected for test fertilizations with potential sires. To test for gamete compatibility, a sample of eggs from each female were combined with diluted sperm from four different non-MHW males. The male with highest sperm quality and compatibility (e.g., good motility and >95% fertilization) was chosen as the sire. Eggs from five females from each acclimation treatment, with the highest quality eggs and without fertilization incompatibilities, were then pooled in two separate beakers, creating two egg pools (i.e., a pool of eggs from MHW acclimated females, and a pool from non-MHW acclimated females). Each female contributed approximately 72,000 eggs to each pool. Beakers containing pooled eggs were gently mixed while diluted, activated sperm (at approx. 105 cells per mL) from the chosen sire was added in small increments to avoid polyspermy. Since this study focused on examining maternal effects, only one sire from the non-MHW group was chosen to reduce any variation in offspring phenotype attributed to paternal genetic variation. Once pooled eggs reached 95% fertilization, embryos were deposited in larval culturing containers for the rearing phase of the experiment.
For the reciprocal culturing experiment embryos were raised at two different developmental temperatures: a MHW temperature (~18.5°C) hereafter denoted as ‘W’ and a non-MHW temperature (~12.7°C) hereafter denoted as ‘C’. This experiment created four offspring combination treatments that are denoted as: WW, WC, CW, CC, where the first letter represents the condition for the adult, and the second letter indicates the temperature at which embryos underwent development. Each of the four groups were raised in three replicate culture containers (Figure 2). Culturing containers consisted of two nested 19L buckets. The inner bucket, which held the embryos, had cutouts that had been covered and sealed with 35μm mesh. The mesh covered cutouts allowed water to leave the bucket without losing embryos. Filtered seawater was fed into a reservoir tank, allowing the water to come to the treatment temperature prior to being fed into culture container using irrigation drippers at a rate of 6L/hr. To maintain treatment temperatures, reservoir tanks and larval culturing containers were held in seawater tables, acting as water baths, in the same set up described for adult treatment tanks. A paddle attached to a motor on each lid of the containers gently stirred the seawater to suspend embryos. Each container contained 12L of FSW and was stocked with 122,000 embryos. Seawater temperature in each culturing container was closely monitored using a wire thermocouple.
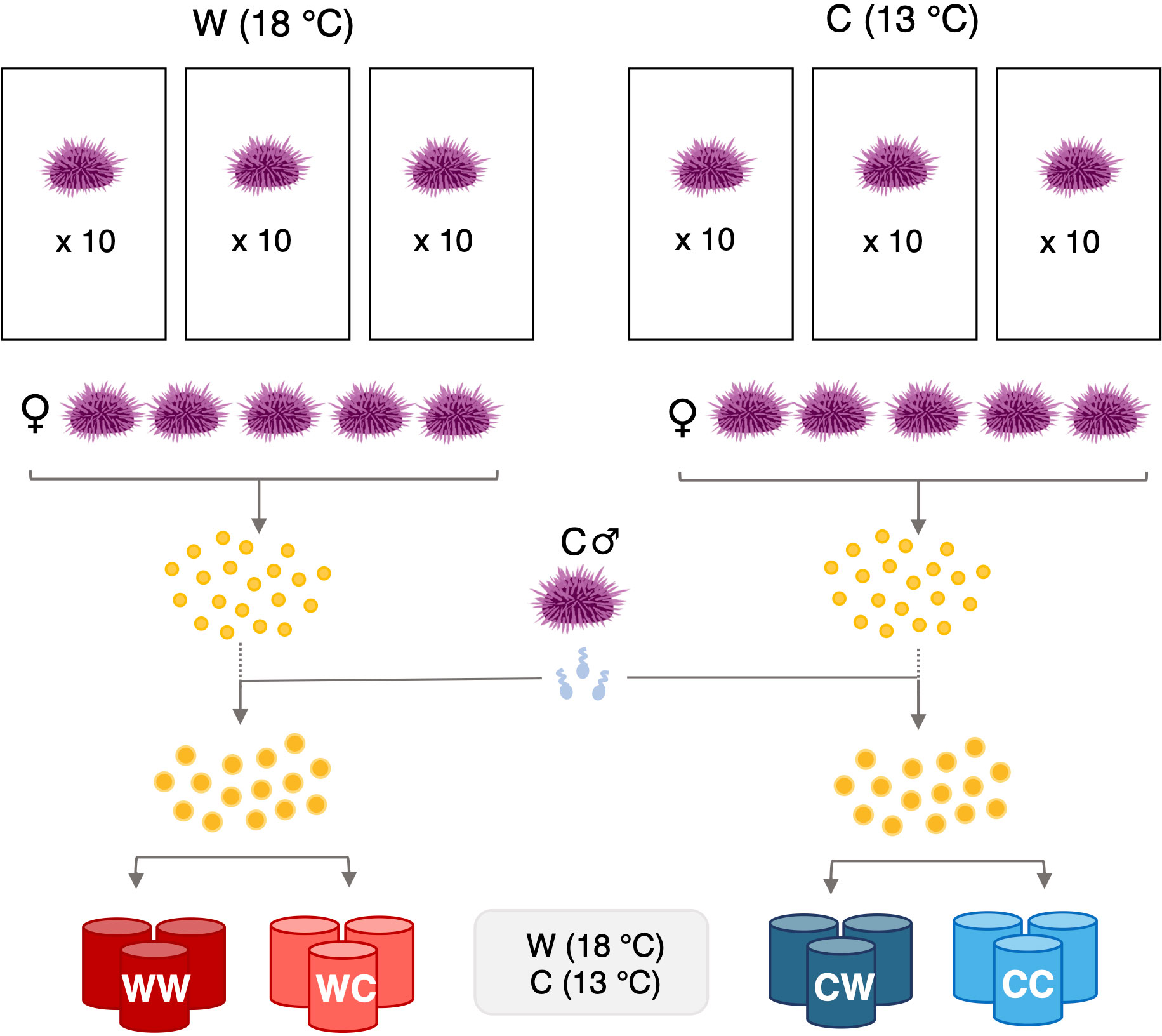
Figure 2 Experimental design. Adult S. purpuratus (N=60) were acclimated in either a MHW (W: 18°C) or a non-MHW (C: 13°C) condition, for a four-month period, during gametogenesis. Eggs from each maternal treatment were pooled (N=5 females per treatment) and fertilized using sperm from a single non-MHW- acclimated male. Embryos from each cross were reared at either a MHW or non-MHW temperature, resulting in four groups: WW, WC, CW, and CC. Each group was raised in three replicate culture vessels.
2.3 Sampling through development
Embryo sampling was conducted at three stages during development: hatched blastula (HB), early gastrula (EG), and an early form of echinopluteus larvae, the prism stage (PR). Each stage was sampled when embryos displayed distinguishing developmental characteristics described in Strathmann (1987). Specifically, hatched blastula stage were characterized by hatching, where the embryo is no longer enclosed in the embryonic envelope; HB were sampled at 16 hours post-fertilization (hpf) for WW and CW treatments and 23hpf for WC and CC treatments. The early gastrula stage was characterized as the embryo displaying invagination at the vegetal plate and was sampled at 21hpf for WW and CW treatments and 34hpf for WC and CC treatments. The prism stage was characterized as the full formation of the tripartite archenteron, in addition to growth of the body rods, and was sampled at 44hpf for WW and CW treatments, 58hpf for WC, and 55hpf for CC treatments.
2.4 Thermal tolerance
To determine the effects and interaction of maternal acclimation and developmental temperature on the performance of the four treatments (WW, WC, CC, CW), subsets of hatched blastula embryos from each treatment were tested in an acute thermal tolerance trial. Methods for this trial were as described by Wong and Hofmann (2020) with some modification. Briefly, using a temperature gradient heat block, scintillation vials containing 3.5 mL of FSW were brought to seven temperatures (17.5, 20.5, 26.4, 24.7, 28.0, 29.8, 31.1°C). Embryos from replicate culturing containers in each treatment were pooled so that each container contributed equally to the thermal tolerance trial. For the trials, 1,000 embryos in 500μL of FSW were added to the preheated scintillation vials, and were capped to prevent evaporation. Vials containing the embryos were maintained in the heat block for 1hr, at which point additional cool FSW was added to each vial and the vials were transferred to a 14°C environmental chamber for a recovery phase. During the recovery phase, embryos were allowed to proceed through development to the prism stage, an early pre-feeding larval stage. The timing of the appearance of embryos progressing to the prism stage was assessed visually using microscopy; approximately 43 hours elapsed from the start of the recovery period and to the beginning of the scoring process. Periodic water changes were conducted to make sure water in vials did not become hypoxic. Using approximately 100 individuals from each vial, we screened for advancement to the prism. Larvae were scored as alive if swimming or cilia movement was observed. LT50 (the temperature at which the population experiences 50% mortality) was calculated for each treatment. While previous studies have measured mortality immediately following exposure to an acute thermal stress, for this experiment mortality was measured once the embryos reached the prism stage. This allowed for a more accurate measure of survival by adding a recovery phase to the acute temperature trial.
2.5 Embryo and larval morphometric analysis
For HB and EG, 500 embryos from each culturing container were preserved in 2% formalin in FSW. In addition, 500 PR larvae from each culturing container were preserved in 2% formalin in FSW buffered with 100mM sodium perborate to a pH of 8.7, a formulation that reduces degradation of the skeletal rods. For each stage, preservation in 2% formalin was achieved by adding an equal volume of 4% formalin (in FSW or buffered FSW) to the sample volume.
Preserved embryos were promptly photographed for morphometric analysis using a compound microscope (Olympus BX50) with a 10x objective and attached digital camera (Motic 10 MP and Motic Image Plus software). Images were measured using ImageJ (National Institutes of Health, USA) and calibrated using a stage micrometer. For HB and EG images, individual embryos (N=35) from each treatment replicate were oriented so the full lateral view was visible, 2D area was measured and calculated by tracing around the perimeter of the embryo. For PR images, individuals (N=35) from each treatment replicate were oriented in a lateral view, so that the side profile of the archenteron was visible, 2D area was similarly measured by tracing around the perimeter of the embryo. At the prism stage, skeletal rod length was also determined by measuring from the tip of the body rod to the tip of the postoral rod.
2.6 Analysis of egg traits
To assess maternal investment in females from the different acclimation treatments, we measured egg traits including egg size and biochemical composition. Eggs were preserved and measured using the same methods as described above for embryos. For imaging, 500 eggs from each contributing female (N=10) were collected promptly after spawning, and preserved in 2% formalin in FSW for morphometric analysis. Individual eggs (N=35) from each female were measured by calculating the average of three diameters and two-dimensional (2D) area.
For lipid quantification, 2,000 eggs were deposited in a 1.5mL cryovial. Each sample was centrifugated to concentrate eggs (approximately 30s at 16,000 RCF) (Eppendorf 5415C Centrifuge), the water layer was carefully removed, and the sample was flash frozen in liquid nitrogen and stored at -80°C until analysis. Two tubes from each contributing female were used to quantify total lipid concentration. Total lipid was extracted using methods described in Wong et al. (2019), a method based on Sewell (2005) with some modification. The concentrated eggs from each sample were suspended in 250μL of Milli-Q water and sonicated at 2amps for a total of 45s at 15s intervals (Fisher Scientific Sonic Dismembrator 500). Each homogenized sample was then transferred to a 5mL glass V-vial (Wheaton) and combined with 125μL of chloroform (HPLC grade) and 250μL of methanol (HPLC grade). Samples were shaken vigorously and then centrifuged at 2,680 RCF for 5min at 4°C (Beckman Coulter Allegra™ 21R Centrifuge). Using a pulled Pasteur pipette the aqueous and chloroform layers were transferred to a clean V-vial; chloroform and water were then added to the final volume ratio of 4:3:2 of water:chloroform:methanol. Samples were then hand shaken and centrifuged again at 2,680 RCF for 5min at 4°C. After centrifuging, the chloroform layer was carefully transferred to a clean glass vial. All samples were flushed with N2 and stored at -20°C prior to analysis.
Total lipid was quantified spectrophotometrically using modified methods based on Marsh and Weinstein (1966). Briefly, samples were placed under a flow of N2 to allow chloroform to evaporate (Reacti-Vap™ Evaporators TS-18826, Thermo Scientific™). Once dried, 500μL of sulfuric acid (ACS grade) was added to each sample, covered with aluminum foil, and dried in a furnace at 200°C for 15min. Samples were allowed to cool for 10min prior to the addition of 2.5mL of deionized water to each vial. Samples were allowed to cool for an additional 10min and then absorbance of each sample was measured on a spectrophotometer (Shimadzu UV-1800) at 375nm. A standard curve of a known mass of lipid within a range of 30-300μg was also prepared and run in parallel with each batch of samples (R2 = 0.98-0.99). The lipid profile used to generate the standard curve consisted of major lipid classes found in S. purpuratus eggs which were previously reported in Wong et al. (2019); specifically, the standard was comprised of 51% triacylglycerol (Glyceryl tripalmitate, Sigma-Aldrich, Catalog No. T5888), 38% phospholipid (L-α-Phosphatidylcholine, Sigma-Aldrich, Catalog No. P3556), and 11% sterol (Cholesterol, Sigma-Aldrich, Catalog No. C8667).
Eggs used for protein quantification were prepared using the same process as described for lipid quantification. Three tubes from each contributing female were used to quantify total protein concentration. Protein was extracted from 2,000 eggs from each females using methods described in Wong et al. (2019). Briefly, 100μL of homogenization buffer (20mM Tris-HCl, (pH 7.6); 130mM NaCl; 5mM EDTA) with 1% Triton-X and 1% Protease Inhibitor Cocktail (Catalog number P8340, Sigma-Aldrich) was added to each sample of concentrated eggs and sonicated on ice for 20s. Samples were then shaken (Thermolyne M71735 Slow Speed Roto Mix) on ice for 15min, followed by centrifugation at 16,000 RCF for 20min (Eppendorf 5415C Centrifuge). The supernatant was transferred to a 1.5mL microcentrifuge tube and stored at -20°C until analysis. Total protein content was quantified using the Pierce™ BCA Protein Assay Kit following manufacturer’s instructions (Catalog No. 23225, Pierce Biotechnology, Rockford, IL, USA). The standard curve was generated using bovine serum albumin (R2 = 0.99).
2.7 Statistical analysis
All statistical analyses were conducted in R Studio (2022.12.0 + 353). Adult survival data was generated using the Kaplan-Meier method (Rich et al., 2010) and analyzed using a log-rank test. For the thermal tolerance trial, the influence of maternal acclimation temperature and offspring developmental temperature on survivorship in response to temperature was analyzed using a generalized linear mixed effect model (lme4 package) (Bates et al., 2015). The binary data (alive vs. dead) were fitted using a logit link. Models were compared, and the best fit was determined using standard model reduction approach for fixed effects, their interactions, and random effects; where the Akaike information criterion (AIC) was used to determine the best fit model. Maternal and offspring temperature were set as fixed effects and vial number was set as a random effect. A Wald chi-squared test was conducted to determine the significance of each factor on survivorship. The LT50 value was calculated using a logistic regression for each treatment. The influence of maternal condition and the developmental temperature on body size at each stage (i.e., HB, EG, and PR), was similarly analyzed using a linear mixed-effect model. Maternal and offspring temperature were set as fixed effects and individual culturing containers were set as a random effect. For egg traits, the influence of maternal acclimation on egg size (i.e., average diameter and 2D area), total lipid, and total protein content was analyzed using a linear mixed-effect model. For egg size, total lipid, and total protein content, maternal temperature condition was set as a fixed effect while each individual mother was set as a random effect.
3 Results
3.1 Observations following acclimation of adult urchins
Adult S. purpuratus were successfully acclimated in the laboratory to two experimental temperature conditions, MHW (18°C) and non-MHW (13°C) conditions, with some mortality. Throughout the four-month acclimation period, mortality was observed in both treatments with a trend of higher survival in the non-MHW treatment (90%), as compared to the MHW treatment (73%). However, the difference in survival probability between treatments was non-significant (Figure 3) (log-rank test: χ2 = 2.8, df=1, p=0.10). Following the acclimation, surviving urchins from each treatment were spawned, providing ample material to create the embryo families and raise cultures. However, we observed decreased spawning success in MHW-acclimated adults as compared to non-MHW-acclimated adults where 50% of surviving urchins in the MHW treatment spawned as compared to the non-MHW treatment where all adults were induced to spawn.
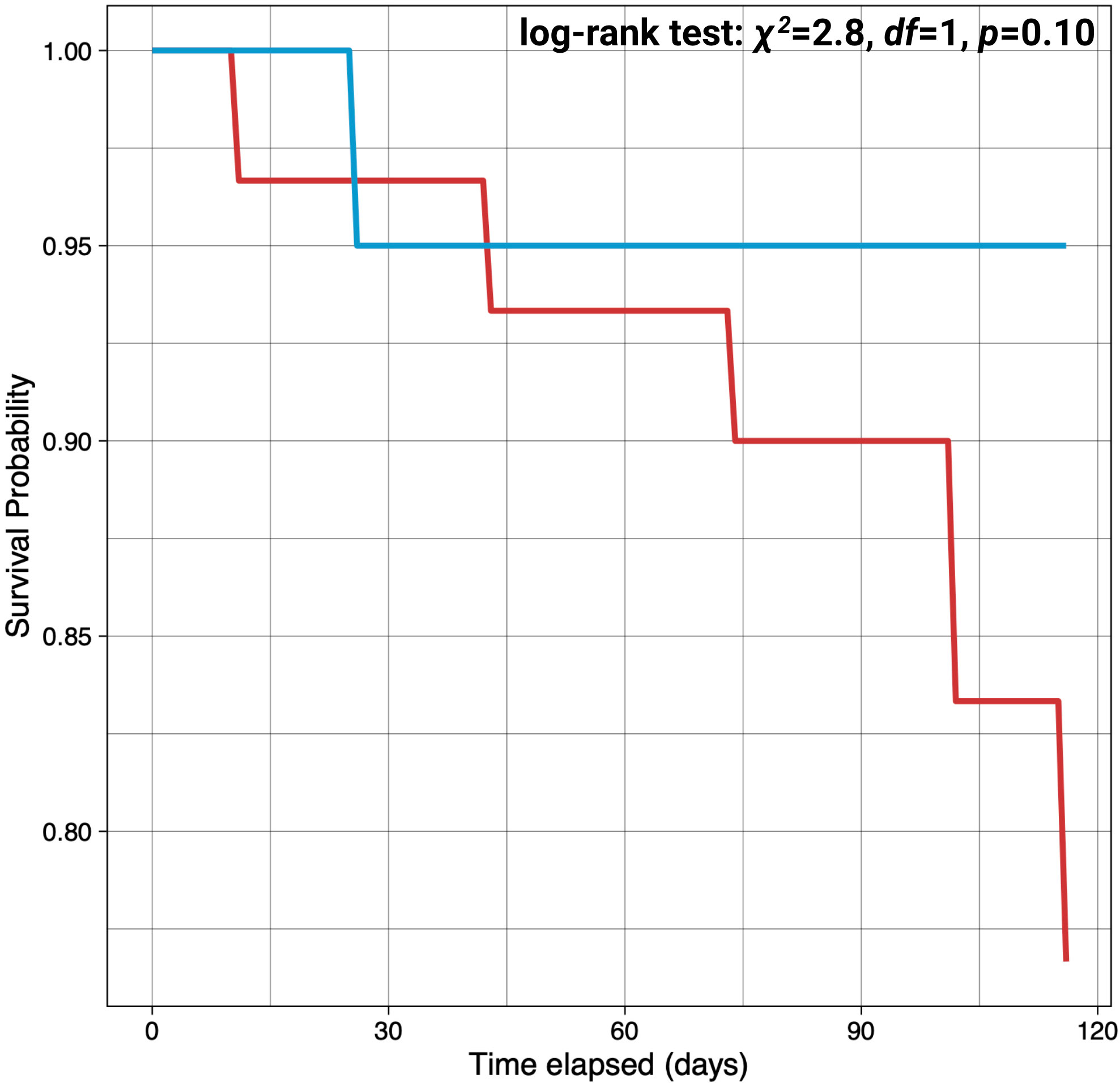
Figure 3 Survivorship in S. purpuratus adults over 4 months of acclimation at marine heatwave (18°C) and non-marine heatwave (13°C) temperatures. Adult urchins were acclimated to either a MHW temperature of 18°C or non-MHW temperature of 13°C for a four-month period from October to January. The red and blue lines represent survival probability for MHW or non-MHW condition, respectively.
3.2 Thermal tolerance
Our study did detect a carry-over effect of maternal thermal history to the progeny. In the thermal tolerance trial, progeny from females acclimated to MHW temperatures (denoted as ‘W’) had a higher thermal tolerance to acute thermal stress than progeny from non-MHW-acclimated females (denoted as ‘C’), with higher percentages of larvae alive following the recovery period. Embryos from females acclimated to 18°C, the MHW condition, had higher LT50 values than those acclimated to 13°C, the non-MHW condition (Figure 4). Across the experiment, calculated LT50 ranged from 27.2 - 28.4°C. For each treatment, LT50 values for WW, WC, CW, and CC were 28.2, 28.4, 27.9, 27.2°C, respectively. When comparing the influence of maternal condition, a comparison of WW vs. CW treatments suggested that embryos, when reared at higher temperatures, gained a modest increase in tolerance (+0.3°C) if mothers experienced MHW temperatures during the period of oogenesis. We also found evidence that embryo environmental conditions influenced thermal tolerance. Here, elevated developmental temperature increased thermal tolerance in progeny from females naïve to MHW exposure, where LT50 of the CW family was 0.7°C higher than the CC family.
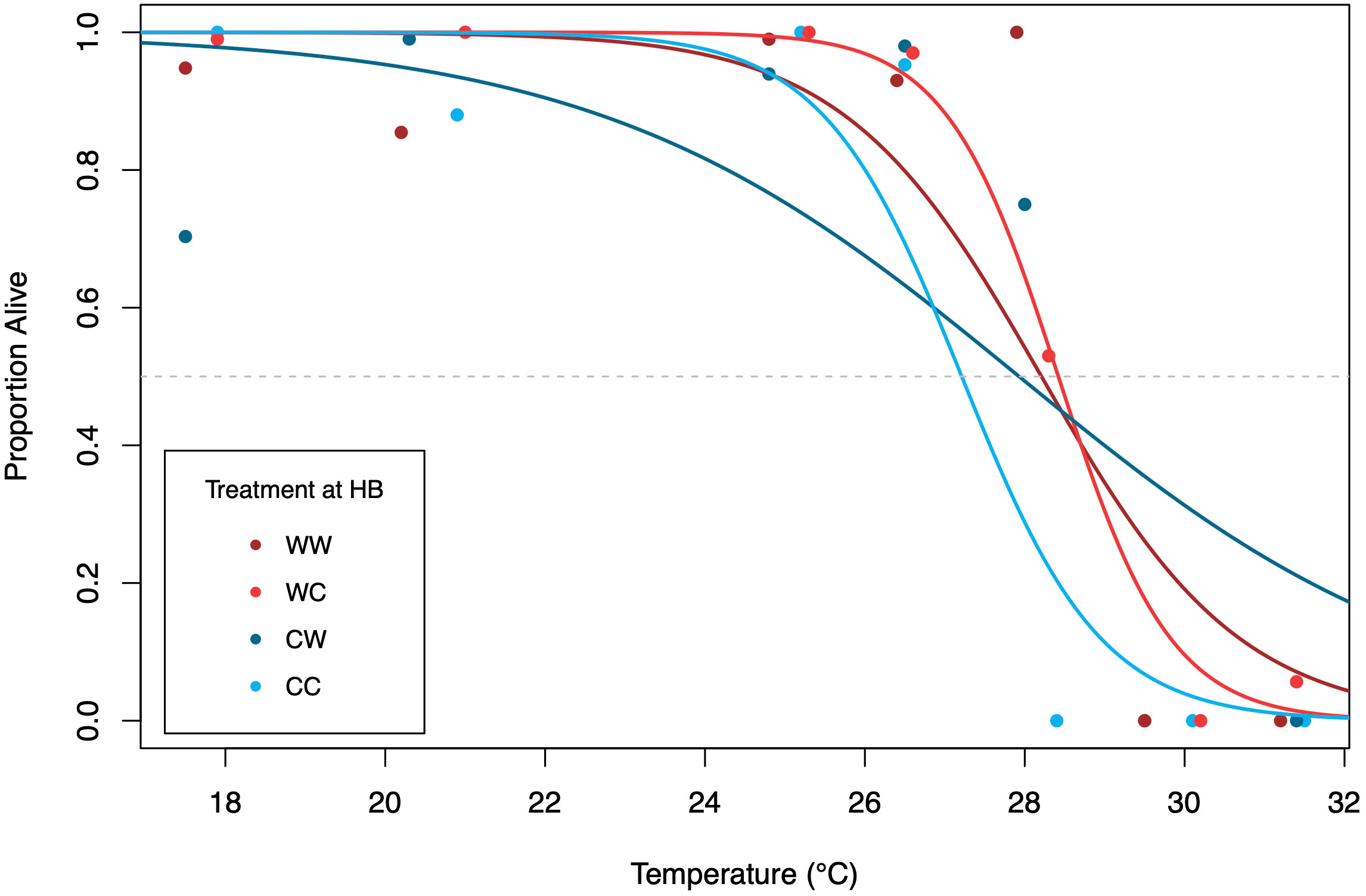
Figure 4 Survivorship of early-stage S. purpuratus from the four treatments. Hatched blastula from each of the four treatments were exposed to a 1-h acute temperature trial and then allowed to recover at 14°C. Following recovery and development to prism the proportion of live prism larvae were scored. Colored lines represent a logistic regression for each treatment. LT50 is shown at the intersection of the dashed grey line with the color-coded line for each group with different parentage and different developmental temperature.
A generalized linear mixed-effect model found that maternal acclimation, temperature at which offspring were raised, and their interaction all had a significant effect on embryo thermal tolerance (Table 1). These results indicated that the thermal history of the females and the developmental temperature of the embryo both influenced thermal tolerance of early-stage purple sea urchins in the thermal tolerance trial.
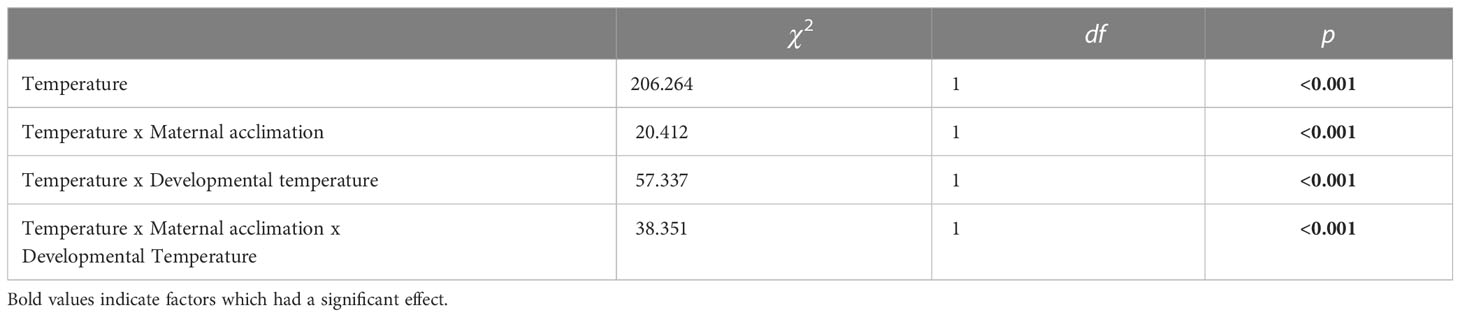
Table 1 A Wald chi-squared test was used to determine the significance of each factor: temperature of acute exposure, maternal acclimation temperature, and offspring developmental temperature; and their interaction on survivorship.
3.3 Embryo morphometrics
The influence of maternal acclimation and offspring developmental temperature on body size differed as a function of stage, where maternal acclimation significantly influenced embryo body size at the early gastrula and prism stage, while offspring developmental temperature had a significant influence only at the prism stage (Table 2). Difference in body size amongst treatments were most evident at the prism stage, where on average, offspring from MHW-acclimated females were 7.1% larger than those from non-MHW-acclimated females. Additionally, offspring raised at the MHW temperature were on average 14.3% larger (2D area) than those raised at the non-MHW temperature and had skeletal rod lengths that was on average 53.5% longer than those raised in the non-MHW condition (Figure 5). Results from the best fit linear mixed effect models are summarized in Table 2.
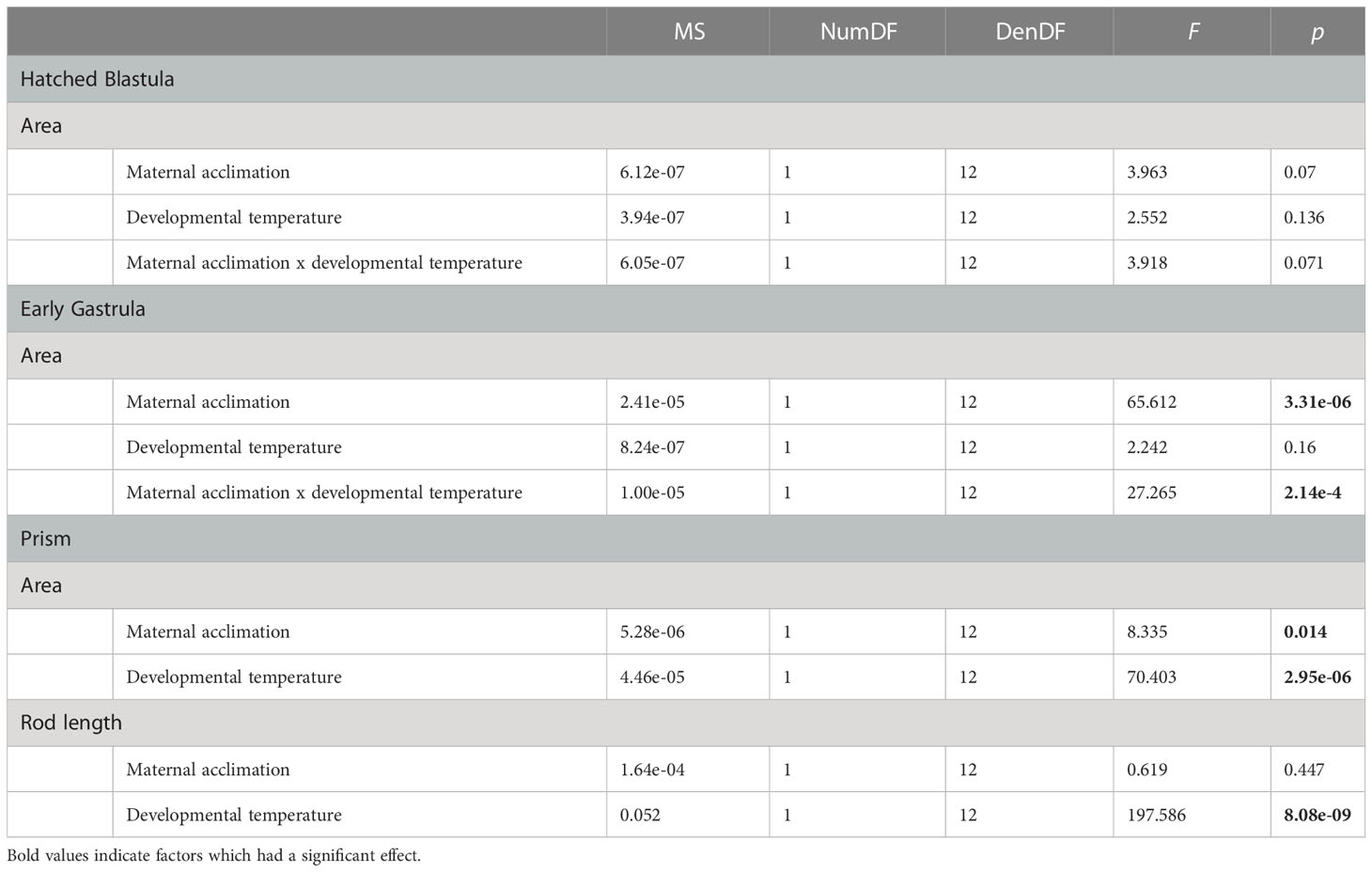
Table 2 Type III analysis of variance table with Satterthwaite’s method for the linear mixed effect models showing the effect of maternal acclimation and offspring developmental temperature on body size (i.e., area and skeletal rod length) of early developmental stages of S. purpuratus.
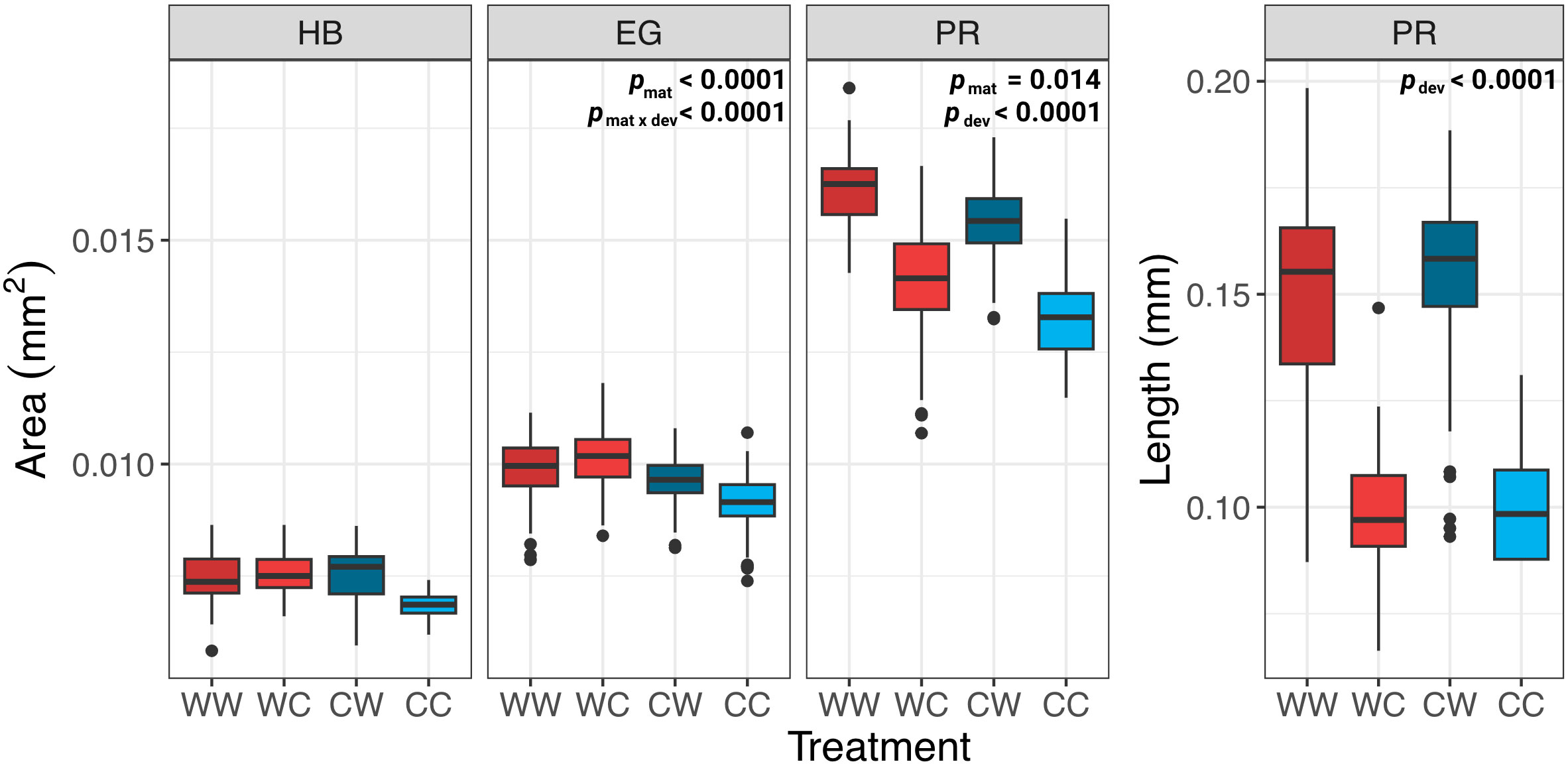
Figure 5 Morphometric analysis of embryo and prism stage S. purpuratus. Body size of embryos and larvae at three developmental stages: hatched blastula (HB), early gastrula (EG), and prism (PR), for each family combination (WW, WC, CW, & CC). Only results with significant p-values are displayed on each graph.
3.4 Egg morphometrics and biochemistry
In general, maternal temperature acclimation had no effect on egg size. Although eggs from non-MHW-acclimated females trended larger in size, maternal environmental history during oogenesis did not significantly influence average egg diameter (F1,8 = 0.1272, p= 0.7286) or 2D area (F1,8 = 0.5882, p= 0.4608). Average egg diameter for MHW- and non-MHW-acclimated mothers was 0.0798 ± 0.023 and 0.0801 ± 0.023mm, respectively. The average 2D area of eggs from MHW- and non-MHW-acclimated females was 0.0051 ± 0.003 and 0.0052 ± 0.003mm2, respectively (Figures 6A, B).
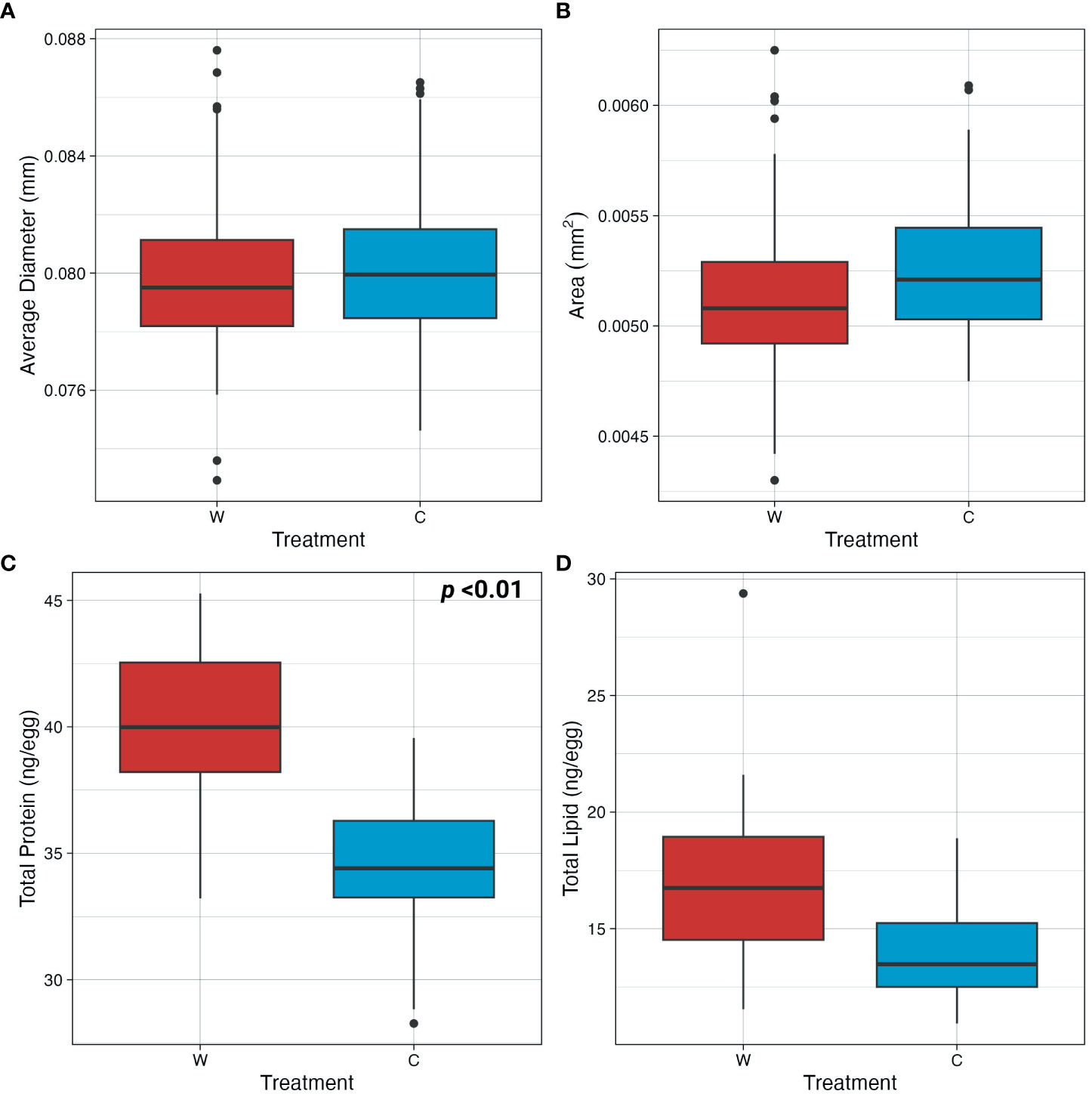
Figure 6 Egg morphometrics and assessment of biochemical storage biomolecules. (A) Diameter and (B) 2D area of eggs from females acclimated to MHW (W) and non-MHW (C) conditions. (C) Total protein (ng/egg) and (D) total lipid content (ng/egg) of eggs from females acclimated to MHW (W) and non-MHW (C) conditions. Only results with significant p-values are displayed on each graph.
In contrast to morphometric traits, the biochemical composition of the eggs did vary in a comparison of females acclimated to MHW vs. non-MHW temperatures. Specifically, protein concentrations were higher in eggs from MHW-acclimated females as compared to eggs from non-MHW-acclimated females (F1,8 = 11.184, p<0.01). Total protein content from MHW and non-MHW acclimated mothers was 40.4 ± 3.38 and 34.6 ± 3.23 ng/egg, respectively (Figure 6C). In contrast, maternal thermal history did not have a significant effect on total lipid content of eggs (F1,8 = 2.3268, p =0.1575), although trending differences in lipid content were observed. Total lipid content in eggs from MHW- acclimated females was on average 17.6 ± 5.19 as compared to 14.0 ± 2.62 ng/egg in MHW- acclimated females (Figure 6D).
4 Discussion
The goal of this study was to investigate TGP as a mechanism of resilience to MHW-relevant thermal stress in early developmental stages of the purple sea urchin, S. purpuratus. Designing experiments parameterized by local thermal extremes, we found that parental thermal history influenced thermal tolerance of progeny, where females acclimated to MHW temperatures (18°C) during gametogenesis produced more thermally tolerant progeny as compared to their non-MHW acclimated counterparts (13°C). Our findings further showed that maternal acclimation significantly influenced offspring body size at various stages in development (i.e., EG and PR), where embryos from MHW-acclimated females were larger. In exploring specific mechanisms of TGP, we hypothesized that differences in maternal provisioning could contribute to the observed differences in offspring phenotype. Here, in an assessment of egg size and the biochemical composition of eggs, we found that eggs from differentially acclimated females differed significantly in total protein content, with eggs from females maintained at higher temperatures having greater total protein. In combination, these results indicated that prolonged exposure to anomalously high environmental temperatures, as would be experienced during MHW events in nature, could influence reproductive success in situ.
In general, there are relatively few studies that have examined the effects of MHWs on gamete and offspring traits with an eye to three important elements of an ocean-climate study – here, parental effects that could drive plasticity, ecologically relevant temperatures for that species/populations under study, and species-specific phenology. In this regard, important studies on echinoderms have shown that a New Zealand species, Evechinus chloroticus, when acclimated to high summer temperatures decreased reproductive output (Delorme and Sewell, 2016). In our study, operating in an ecologically matched time window for oogenesis, we observed a similar trend were S. purpuratus adults acclimated to MHW conditions had lower spawning success when compared to those held at control conditions. In a study of carry-over effects in Heliocidaris erythrogramma, Minuti and colleagues found that MHW- acclimated females produced larger, more thermally tolerant progeny at early developmental stages (Minuti et al., 2021). Similar observations were made in this study on the temperate, kelp forest species, S. purpuratus. Further, in a study of sperm traits in S. purpuratus, male urchins acclimated to local MHW conditions (20°C) had decreased fertilization success when compared to males exposed to non-MHW conditions (15°C) (Leach et al., 2021). Our understanding of MHW effects on reproductive and offspring traits is growing with accumulating evidence noting that early stage and developmental steps are likely influenced by high temperatures typical of MHWs, especially if the thermal stress aligns with critical life history events such as gametogenesis.
4.1 Transgenerational effects on offspring thermal tolerance
The main objective of the study was to examine whether adult environmental experience in MHW conditions would influence the performance of their progeny. Ecologically, this is significant because MHW events can occur throughout the period in which purple urchins are broadcast spawning and thus, early development could occur at anomalously high temperatures in situ (Figure 1). Using laboratory experiments, we queried whether adults could “prime” their progeny during periods of anomalously elevated temperatures, or whether MHW stress during gametogenesis would have deleterious effects on offspring performance. Using acute temperature trial assays used previously on early stage echinoderms (Wong and Hofmann, 2020), we observed that maternal exposure to MHW temperatures increased thermal tolerance in progeny (Figure 4), as demonstrated by higher LT50 values.
These results join other studies that examined TGP in marine invertebrates in response to thermal stress, where the offspring response varied with species, timing, and nature of parental conditioning (Putnam and Gates, 2015; Shama, 2015; Morley et al., 2017; Rivera et al., 2021; Bernal et al., 2022; Waite and Sorte, 2022). For example, in a study on the marine polychaetae, Ophryotrocha labronica, researchers found that the thermal tolerance of the progeny tracked the timing of the temperature exposure of the female, where tolerance of offspring differed depending on whether maternal acclimation occurred during early or late oogenesis (Massamba-N'Siala et al., 2014). These results match the outcome of our experiment in S. purpuratus. Together, these findings underscore the significance of the timing of the warming events associated with MHWs and their subsequent effects on offspring resilience. Since the nature of MHWs can vary from prolonged to short-term events (Oliver et al., 2021), transgenerational responses may differ depending on duration of maternal exposure.
4.2 Transgenerational effects on embryo and larval body size
In addition to measuring upper thermal tolerance as a metric of offspring performance, we examined body size, an important functional trait of echinoderms in larval ecology and physiology (Strathmann, 1971; Allen and Marshall, 2014) that has shown to correlate to performance in studies of local adaptation and plasticity in S. purpuratus (Kelly et al., 2013; Strader et al., 2022). In this study using MHW-relevant temperatures, we found that maternal thermal history significantly influenced body size (measured as 2D area) for early gastrula embryos and prism larvae (Figure 5), where offspring from MHW-acclimated females were larger in size, potentially indicating a positive transgenerational effect, since larger size in larvae is often associated with higher fitness (Strathmann, 1971; Allen and McAlister, 2007). Offspring developmental temperature also influenced body size for prism-stage larvae, which was evident in the observed differences in skeletal rod length. An interaction between maternal thermal history and developmental temperature was seen only at the early gastrula stage, where differences in body size within each developmental treatment were dependent on the maternal experience.
Other studies examining transgenerational effects in response to elevated temperature conditions in echinoderms have reported mixed effects on larval size. For example, studies on Strongylocentrotus intermedius and Tripneustes gratilla, show that adults exposed to long-term elevated temperatures, 15 months and 6 months respectively, produced progeny that were smaller in size compared to progeny from adults conditioned to ambient temperatures (Zhao et al., 2018; Karelitz et al., 2019). In addition, adult long-term acclimation to +2°C temperature conditions did not affect body size of the progeny in Heliocidaris erythrogamma (Harianto et al., 2021). Overall, measurement of body size at different stages in development may contribute to the differences seen amongst studies. Furthermore, length of adult exposure, differences in species plasticity, and population-level responses likely contribute to reported differences in transgenerational responses.
4.3 Maternal provisioning as a general mechanism of TGP
Mechanistically, maternal contributions to shifts in tolerance of the progeny could manifest in two ways - 1) as maternal energetic investment in the eggs, and/or 2) as maternally-derived egg transcripts and proteins working to reduce cellular damage due to heat stress during early development. Historically maternal energetic investment has been assessed through measurements of egg size and biochemical composition (i.e., lipids and proteins) where studies have shown that egg size and nutritional macromolecules are significant in determining conditions and performance of developing progeny in echinoderms (Jaeckle, 1995; Prowse et al., 2008; Moran et al., 2013; Peters-Didier and Sewell, 2017). Given these data on echinoderm eggs, we hypothesized that observed differences in offspring phenotype would be due to differences in maternal energetic provisioning (i.e., egg size and biochemical composition). In testing this hypothesis, we found that acclimation of females to MHW temperatures during oogenesis resulted in significantly altered egg characteristics. Specifically, although egg size was unaffected by maternal acclimation, eggs from MHW- acclimated females had higher protein content and had total lipid content that trended upward (Figure 6).
For marine invertebrates, egg size is often used a standard measurement of maternal investment as it has been linked to numerous adaptive traits in developing embryos and larvae such as fertilization success, larval development, and post-metamorphosis survival (Moran and McAlister, 2009; Allen and Marshall, 2014). While differences in egg size were not observed in our study, past research examining the effects of maternal acclimation temperature on egg size report varying outcomes (Foo and Byrne, 2017). In combination, data in the literature suggest that species and the duration of thermal exposure are likely significant determinants of egg size (Skadsheim, 1989; Suckling et al., 2015; Guillaume et al., 2016; Karelitz et al., 2019). However, when examining provisioning of eggs, focusing solely on size may not be the best predictor of energetic content. In echinoid species specifically, while egg size generally scales with energy content, at the intraspecific level this relationship is weakened (McEdward and Morgan, 2001; Moran and McAlister, 2009).
In this light, to further assess differences in egg quality, we measured total protein and lipid concentrations. Protein concentrations were significantly higher in eggs from females with the history of high temperature conditioning (Figure 6C). In addition, although there was a trend indicating higher lipid levels as well, there was not a significant difference between eggs of MHW- and non-MHW-acclimated females. In general, the levels of protein and lipid measured here are consistent with other measurements in S. purpuratus (Matson et al., 2012; Wong et al., 2019). Studies examining how these macromolecules are used throughout development show that lipids (specifically triglycerides) are major energy fuels, while structural lipids (e.g., phospholipids) and proteins are used to construct the larval body (Byrne et al., 2008). These patterns of biochemical utilization support our results where larger larvae developed from more protein rich eggs (i.e., eggs from MHW-acclimated females).
While not evaluated in our study, differences in egg protein characteristics may potentially influence thermal tolerance of early-stage embryos via maternal transmission of heat shock proteins. In support of this hypothesis, a study examining gonad tissue of acclimated adult urchins (20 vs 26°C) found higher levels of Hsp70 protein in the warm-acclimated urchins (Harianto et al., 2021). While measurements of gonad tissue do not identify standing stocks of defensome proteins in spawned eggs, this study does highlight the potential for maternal acclimation via changes in egg protein characteristics to alter tolerance of developing embryos. In addition to proteins, maternally sourced transcripts may also contribute to differences in thermal tolerance at early embryonic stages. Together these inherited molecules are necessary for driving early developmental processes, and it is not until the maternal-to-zygotic transition that embryos are able to activate their own genome (Hamdoun and Epel, 2007; Schier, 2007). With this in mind, we chose to study hatched blastula embryos, specifically, as studies have shown that these early-stage embryos still rely on maternal transcripts and are unable to completely activate elements of the embryonic defensome, e.g., genes for molecular chaperones and heat-shock proteins (Sconzo et al., 1995).
To further highlight physiological mechanisms that contribute to TGP, transcriptomic studies on urchins have shown that parental acclimation to different temperatures influence gene expression in offspring during early development (Shi et al., 2020). Similar results have been observed in S. purpuratus under variable conditions of temperature and pCO2 (Wong et al., 2018). While transcriptomic techniques have aided our ability to determine the molecular underpinning of TGP, to shed light on maternal provisioning mechanisms, future studies should incorporate long-term acclimation during gametogenic windows followed by examination of maternally sourced RNA and proteins in eggs and early embryos. Assessment of the temperature-related egg changes in a climate-change context could be further investigated by examining the translatome, an approach that has been used in other echinoderms (Chassé et al., 2018).
4.4 Effects of developmental temperature on offspring phenotype
In addition to addressing transgenerational processes, the factorial design of the experiment allowed us to examine how the temperature at which embryos developed influenced their phenotypic and functional traits (i.e., body size and thermal tolerance). We found that progeny from non-MHW acclimated females reared in non-MHW conditions had the lowest LT50 value. However, thermal tolerance of these progeny increased when reared at the higher MHW temperature (Figure 4). This outcome has been observed in other echinoderm species. In a study on red urchins (Mesocentrotus franciscanus), embryos reared at 17°C had an increased thermal tolerance as compared to embryos reared at 13°C (Wong and Hofmann, 2020), indicating that if rearing temperatures do not exceed upper thermal limits there is a potential for a resistance response in early-stage embryos. In our study, developmental temperature also had a significant effect on body size at the latest stage evaluated (i.e., PR), where development in MHW conditions was positively associated with body size and body rod length (Figure 5).
5 Conclusion
This study provides evidence that TGP may contribute to the capacity to resist the thermal stress associated with MHWs in a benthic marine invertebrate. Findings from our thermal tolerance trials showed that offspring with a history of MHW exposure, whether it be via maternal history or during development, had higher LT50 values than those that were naïve to MHW conditions, suggesting that both trans- and intragenerational acclimation were acting to increase tolerance to heat stress. These results suggest that prior exposure to elevated temperature can increase tolerance of early-stage S. purpuratus. Since early life history stages are often considered to be the most sensitive to abiotic stressors (Pandori and Sorte, 2018), and are currently being affected by MHWs (Rogers et al., 2021), maternal effects such as those observed in our study may act to ameliorate negative impacts of MHWs.
It is important to note that there are limitations to the interpretation of the results of this laboratory experiment. Specifically, it is unclear how long this resistance will last, either in the lifetime of individuals, or across subsequent generations. While carry-over effects may increase tolerance to thermal stress in a single generation, past TGP studies have shown that acquired tolerances may not persist within or across generations (Byrne et al., 2020). Additionally, due to the variable nature of MHW events it is important to consider the possibility of environmental mismatches between parents and offspring and how this may influence inherited traits (Baker et al., 2019). Future research that incorporates the spatio-temporal patterns of MHWs at the local level and further, matches these events to the phenology of critical life history events, will improve our ability to forecast impacts of MHWs on coastal marine ecosystems. Variation in thermal patterns are likely to have significantly different impacts on important life history events depending on the species and life history strategies in question, a perspective that has been noted in the ocean global change biology literature (Bautista and Crespel, 2021). Lastly, food quality and availability during MHW conditions will also influence adult condition in situ. Lowman and colleagues have reported lower nutritional quality of kelp exposed to high temperatures (Lowman et al., 2022), which may subsequently impact urchin growth and reproduction (McBride et al., 2004).
From a global change perspective, MHWs are predicted to become a dominant force in many of the world’s oceans (Oliver et al., 2021; Smith et al., 2023) and a greater understanding of how ecologically and economically important species respond to the thermal stress associated with MHWs will be critical in predicting the vulnerability of key fisheries (Smith et al., 2021) and on how biodiversity and ecosystem health might be altered in warming coastal oceans (Smale et al., 2019; Michaud et al., 2022). Overall, TGP studies will provide critical insight into mechanisms of acclimation in response to these thermally stressful events that function at ecological timescales, providing time for adaptation to catch up.
Data availability statement
The datasets presented in this study can be found in online repositories. The names of the repository/repositories and accession number(s) can be found below: https://doi.org/10.6073/pasta/37b2d8d777d8827169acc2392af05b27.
Ethics statement
Ethical review and approval were not required for the study on animals in accordance with the local legislation and institutional requirements.
Author contributions
GH led the conception and design of the larger project and acquired funding. JC and GH designed the experiment. JC, AM, and GH conducted the laboratory experiment. JC and AM collected the data. JC conducted the statistical analysis. JC and GH wrote the manuscript. All authors have read and approved the final manuscript.
Funding
This research was supported by an award from the U.S. National Science Foundation (NSF) (NSF award 2131283 to GH). JC and AM were each supported by NSF Graduate Research Fellowships under Grant No. 1650114. Collection of temperature data was supported by an NSF award to the SBC LTER (OCE-1831937) for which GH is a co-PI (Director: Dr. Robert Miller).
Acknowledgments
We thank Christoph Pierre for assistance with urchin and kelp collection at UC Santa Barbara. We also thank members of the Hofmann lab, Erin De Leon Sanchez and Samuel Bogan, for their assistance in urchin spawning, larval culturing, and sample collection.
Conflict of interest
The authors declare that the research was conducted in the absence of any commercial or financial relationships that could be construed as a potential conflict of interest.
Publisher’s note
All claims expressed in this article are solely those of the authors and do not necessarily represent those of their affiliated organizations, or those of the publisher, the editors and the reviewers. Any product that may be evaluated in this article, or claim that may be made by its manufacturer, is not guaranteed or endorsed by the publisher.
Supplementary material
The Supplementary Material for this article can be found online at: https://www.frontiersin.org/articles/10.3389/fmars.2023.1212781/full#supplementary-material
References
Allen R. M., Marshall D. J. (2014). Egg size effects across multiple life-history stages in the marine annelid Hydroides diramphus. PloS One 9 (7), e102253. doi: 10.1371/journal.pone.0102253
Allen J. D., McAlister J. S. (2007). Testing rates of planktonic versus benthic predation in the field. J. Exp. Mar. Biol. Ecol. 347 (1), 77–87. doi: 10.1016/j.jembe.2007.03.010
Arafeh-Dalmau N., Montaño-Moctezuma G., Martínez J. A., Beas-Luna R., Schoeman D. S., Torres-Moye G. (2019). Extreme marine heatwaves alter kelp forest community near its equatorward distribution limit. Front. Mar. Sci. 6. doi: 10.3389/fmars.2019.00499
Baker B. H., Sultan S. E., Lopez-Ichikawa M., Waterman R. (2019). Transgenerational effects of parental light environment on progeny competitive performance and lifetime fitness. Philos. Trans. R. Soc. B: Biol. Sci. 374 (1768), 20180182. doi: 10.1098/rstb.2018.0182
Bautista N. M., Crespel A. (2021). Within- and trans-generational environmental adaptation to climate change: perspectives and new challenges. Front. Mar. Sci. 8. doi: 10.3389/fmars.2021.729194
Bates D., Mächler M., Bolker B., Walker S. (2015). Fitting linear mixed-effects models using lme4. J. Stat. Softw. 67 (1), 1–48. doi: 10.18637/jss.v067.i01
Bernal M. A., Ravasi T., Rodgers G. G., Munday P. L., Donelson J. M. (2022). Plasticity to ocean warming is influenced by transgenerational, reproductive, and developmental exposure in a coral reef fish. Evolutionary Appl. 15 (2), 249–261. doi: 10.1111/eva.13337
Byrne M. (2011). Impact of ocean warming and ocean acidification on marine invertebrate life history stages: vulnerabilities and potential for persistence in a changing ocean. Oceanogr. Mar. Biol. 49, 1–42.
Byrne M., Foo S. A., Ross P. M., Putnam H. M. (2020). Limitations of cross- and multigenerational plasticity for marine invertebrates faced with global climate change. Global Change Biol. 26 (1), 80–102. doi: 10.1111/gcb.14882
Byrne M., Prowse T. A. A., Sewell M. A., Dworjanyn S., Williamson J. E., Vaïtilingon D. (2008). Maternal provisioning for larvae and larval provisioning for juveniles in the toxopneustid sea urchin Tripneustes gratilla. Mar. Biol. 155 (5), 473–482. doi: 10.1007/s00227-008-1045-5
Cavole L. M., Demko A. M., Diner R. E., Giddings A., Koester I., Pagniello C. M. L. S., et al. (2016). Biological impacts of the 2013–2015 warm-water anomaly in the northeast Pacific winners, losers, and the future. Oceanography 29 (2), 273–285. doi: 0.5670/oceanog.2016.32
Chassé H., Aubert J., Boulben S., Le Corguillé G., Corre E., Cormier P., et al. (2018). Translatome analysis at the egg-to-embryo transition in sea urchin. Nucleic Acids Res. 46 (9), 4607–4621. doi: 10.1093/nar/gky258
Clare X. S., Kui L., Hofmann G. E. (2022). Larval thermal tolerance of kellet's whelk (Kelletia kelletii) as a window into the resilience of a wild shellfishery to marine heatwaves. J. Shellfish Res. 41 (2), 283–290. doi: 10.2983/035.041.0214
Cochran R. C., Engelmann F. (1975). Environmental regulation of the annual reproductive season of Strongylocentrotus purpuratus (Stimpson). Biol. Bull. 148 (3), 393–401. doi: 10.2307/1540516
Delorme N. J., Sewell M. A. (2016). Effects of warm acclimation on physiology and gonad development in the sea urchin Evechinus chloroticus. Comp. Biochem. Physiol. A Mol. Integr. Physiol. 198, 33–40. doi: 10.1016/j.cbpa.2016.03.020
Di Lorenzo E., Mantua N. (2016). Multi-year persistence of the 2014/15 north Pacific marine heatwave. Nat. Climate Change 6 (11), 1042–1047. doi: 10.1038/nclimate3082
Donelson J. M., Wong M., Booth D. J., Munday P. L. (2016). Transgenerational plasticity of reproduction depends on rate of warming across generations. Evolutionary Appl. 9 (9), 1072–1081. doi: 10.1111/eva.12386
Foo S. A., Byrne M. (2017). Marine gametes in a changing ocean: impacts of climate change stressors on fecundity and the egg. Mar. Environ. Res. 128, 12–24. doi: 10.1016/j.marenvres.2017.02.004
Gall M. L., Holmes S. P., Campbell H., Byrne M. (2021). Effects of marine heatwave conditions across the metamorphic transition to the juvenile sea urchin (Heliocidaris erythrogramma). Mar. Pollut. Bull. 163, 111914. doi: 10.1016/j.marpolbul.2020.111914
Garrabou J., Gómez-Gras D., Medrano A., Cerrano C., Ponti M., Schlegel R., et al. (2022). Marine heatwaves drive recurrent mass mortalities in the Mediterranean Sea. Global Change Biol. 28 (19), 5708–5725. doi: 10.1111/gcb.16301
Gentemann C. L., Fewings M. R., García-Reyes M. (2017). Satellite sea surface temperatures along the West Coast of the United States during the 2014–2016 northeast Pacific marine heat wave. Geophysical Res. Lett. 44 (1), 312–319. doi: 10.1002/2016GL071039
Guillaume A. S., Monro K., Marshall D. J. (2016). Transgenerational plasticity and environmental stress: do paternal effects act as a conduit or a buffer? Funct. Ecol. 30 (7), 1175–1184. doi: 10.1111/1365-2435.12604
Guo X., Gao Y., Zhang S., Wu L., Chang P., Cai W., et al. (2022). Threat by marine heatwaves to adaptive large marine ecosystems in an eddy-resolving model. Nat. Climate Change 12 (2), 179–186. doi: 10.1038/s41558-021-01266-5
Hamdoun A., Epel D. (2007). Embryo stability and vulnerability in an always changing world. Proc. Natl. Acad. Sci. 104 (6), 1745–1750. doi: 10.1073/pnas.0610108104
Harianto J., Aldridge J., Torres Gabarda S. A., Grainger R. J., Byrne M. (2021). Impacts of acclimation in warm-low pH conditions on the physiology of the Sea urchin Heliocidaris erythrogramma and carryover effects for juvenile offspring. Front. Mar. Sci. 7. doi: 10.3389/fmars.2020.588938
Hart L. C., Goodman M. C., Walter R. K., Rogers-Bennett L., Shum P., Garrett A. D., et al. (2020). Abalone recruitment in low-density and aggregated populations facing climatic stress. J. Shellfish Res. 39, 359–373. doi: 10.2983/035.039.0218
Hobday A. J., Alexander L. V., Perkins S. E., Smale D. A., Straub S. C., Oliver E. C. J., et al. (2016). A hierarchical approach to defining marine heatwaves. Prog. Oceanogr. 141, 227–238. doi: 10.1016/j.pocean.2015.12.014
Hobday A. J., Oliver E. C. J., Sen Gupta A., Benthuysen J. A., Burrows M. T., Donat M. G., et al. (2018). Categorizing and naming marine heatwaves. Oceanography 31 (2), 162–173. doi: 10.5670/oceanog.2018.205
Hughes T. P., Kerry J. T., Álvarez-Noriega M., Álvarez-Romero J. G., Anderson K. D., Baird A. H., et al. (2017). Global warming and recurrent mass bleaching of corals. Nature 543 (7645), 373–377. doi: 10.1038/nature21707
Jacox M. G., Alexander M. A., Amaya D., Becker E., Bograd S. J., Brodie S., et al. (2022). Global seasonal forecasts of marine heatwaves. Nature 604 (7906), 486–490. doi: 10.1038/s41586-022-04573-9
Jaeckle W. B. (1995). Variation in size, energy content, and biochemical composition of invertebrate eggs: Correlates with mode of development, In McEdward L. (Ed) Ecology of marine invertebrate larvae (CRC Press), 49–77.
Karelitz S., Lamare M. D., Mos B., De Bari H., Dworjanyn S. A., Byrne M. (2019). Impact of growing up in a warmer, lower pH future on offspring performance: transgenerational plasticity in a pan-tropical sea urchin. Coral Reefs 38 (6), 1085–1095. doi: 10.1007/s00338-019-01855-z
Kelly M. W., Padilla-Gamiño J. L., Hofmann G. E. (2013). Natural variation and the capacity to adapt to ocean acidification in the keystone sea urchin Strongylocentrotus purpuratus. Global Change Biol. 19 (8), 2536–2546. doi: 10.1111/gcb.12251
Leach T. S., BuyanUrt B., Hofmann G. E. (2021). Exploring impacts of marine heatwaves: paternal heat exposure diminishes fertilization success in the purple sea urchin (Strongylocentrotus purpuratus). Mar. Biol. 168 (7), 103. doi: 10.1007/s00227-021-03915-x
Leung J. Y. S., Connell S. D., Russell B. D. (2017). Heatwaves diminish the survival of a subtidal gastropod through reduction in energy budget and depletion of energy reserves. Sci. Rep. 7 (1), 17688. doi: 10.1038/s41598-017-16341-1
Lonhart S. I., Jeppesen R., Beas-Luna R., Crooks J. A., Lorda J. (2019). Shifts in the distribution and abundance of coastal marine species along the eastern Pacific ocean during marine heatwaves from 2013 to 2018. Mar. Biodiver. Records 12 (1), 13. doi: 10.1186/s41200-019-0171-8
Lowman H. E., Emery K. A., Dugan J. E., Miller R. J. (2022). Nutritional quality of giant kelp declines due to warming ocean temperatures. Oikos 2022 (7). doi: 10.1111/oik.08619
Marsh J. B., Weinstein D. B. (1966). Simple charring method for determination of lipids. J. Lipid Res. 7 (4), 574–576.
Massamba-N'Siala G., Prevedelli D., Simonini R. (2014). Trans-generational plasticity in physiological thermal tolerance is modulated by maternal pre-reproductive environment in the polychaete Ophryotrocha labronica. J. Exp. Biol. 217 (Pt 11), 2004–2012. doi: 10.1242/jeb.094474
Matson P. G., Yu P. C., Sewell M. A., Hofmann G. E. (2012). Development under elevated pCO2 conditions does not affect lipid utilization and protein content in early life-history stages of the purple sea urchin, Strongylocentrotus purpuratus. Biol. Bull. 223 (3), 312–327. doi: 10.1086/BBLv223n3p312
McBride S. C., Price R. J., Tom P. D., Lawrence J. M., Lawrence A. L. (2004). Comparison of gonad quality factors: color, hardness and resilience, of Strongylocentrotus franciscanus between sea urchins fed prepared feed or algal diets and sea urchins harvested from the northern California fishery. Aquaculture 233 (1), 405–422. doi: 10.1016/j.aquaculture.2003.10.014
McEdward L. R., Morgan K. H. (2001). Interspecific relationships between egg size and the level of parental investment per offspring in echinoderms. Biol. Bull. 200 (1), 33–50. doi: 10.2307/1543083
Michaud K. M., Reed D. C., Miller R. J. (2022). The Blob marine heatwave transforms California kelp forest ecosystems. Commun. Biol. 5 (1) 1143. doi: 10.1038/s42003-022-04107-z
Minuti J. J., Byrne M., Campbell H., Hemraj D. A., Russell B. D. (2022). Live-fast-die-young: carryover effects of heatwave-exposed adult urchins on the development of the next generation. Global Change Biol. 28 (19), 5781–5792. doi: 10.1111/gcb.16339
Minuti J. J., Byrne M., Hemraj D. A., Russell B. D. (2021). Capacity of an ecologically key urchin to recover from extreme events: physiological impacts of heatwaves and the road to recovery. Sci. Total Environ. 785, 147281. doi: 10.1016/j.scitotenv.2021.147281
Moran A. L., McAlister J. S. (2009). Egg size as a life history character of marine invertebrates: is it all it's cracked up to be? Biol. Bull. 216 (3), 226–242. doi: 10.1086/BBLv216n3p226
Moran A. L., McAlister J. S., Whitehill E. A. G. (2013). Eggs as energy: revisiting the scaling of egg size and energetic content among echinoderms. Biol. Bull. 224 (3), 184–191. doi: 10.1086/BBLv224n3p184
Morley S. A., Nguyen K. D., Peck L. S., Lai C. H., Tan K. S. (2017). Can acclimation of thermal tolerance, in adults and across generations, act as a buffer against climate change in tropical marine ectotherms? J. Thermal Biol. 68, 195–199. doi: 10.1016/j.jtherbio.2016.09.007
Okamoto D. K., Schroeter S. C., Reed D. C. (2020). Effects of ocean climate on spatiotemporal variation in sea urchin settlement and recruitment. Limnol. Oceanogr. 65 (9), 2076–2091. doi: 10.1002/lno.11440
Oliver E. C. J., Benthuysen J. A., Darmaraki S., Donat M. G., Hobday A. J., Holbrook N. J., et al. (2021). Marine heatwaves. Annu. Rev. Mar. Sci. 13 (1), 313–342. doi: 10.1146/annurev-marine-032720-095144
Oliver E. C. J., Donat M. G., Burrows M. T., Moore P. J., Smale D. A., Alexander L. V., et al. (2018). Longer and more frequent marine heatwaves over the past century. Nat. Commun. 9:1324. doi: 10.1038/s41467-018-03732-9
Pandori L. L. M., Sorte C. J. B. (2018). The weakest link: sensitivity to climate extremes across life stages of marine invertebrates. Oikos 128 (5), 621–629. doi: 10.1111/oik.05886
Pearse J. S. (2006). Ecological role of purple sea urchins. Science 314 (5801), 940–941. doi: 10.1126/science.1131888
Peters-Didier J., Sewell M. A. (2017). Maternal investment and nutrient utilization during early larval development of the sea cucumber Australostichopus mollis. Mar. Biol. 164 (9), 178. doi: 10.1007/s00227-017-3209-7
Prowse T. A. A., Sewell M. A., Byrne M. (2008). Fuels for development: evolution of maternal provisioning in asterinid sea stars. Mar. Biol. 153 (3), 337–349. doi: 10.1007/s00227-007-0809-7
Putnam H. M., Gates R. D. (2015). Preconditioning in the reef-building coral Pocillopora damicornis and the potential for trans-generational acclimatization in coral larvae under future climate change conditions. J. Exp. Biol. 218 (15), 2365–2372. doi: 10.1242/jeb.123018
Reed D., Washburn L., Rassweiler A., Miller R., Bell T., Harrer S. (2016). Extreme warming challenges sentinel status of kelp forests as indicators of climate change. Nat. Commun. 7 (1), 13757. doi: 10.1038/ncomms13757
Rich J. T., Neely J. G., Paniello R. C., Voelker C. C., Nussenbaum B., Wang E. W. (2010). A practical guide to understanding Kaplan-Meier curves. Otolaryngol Head Neck Surg. 143 (3), 331–336. doi: 10.1016/j.otohns.2010.05.007
Rivera H. E., Chen C., Gibson M. C., Tarrant A. M. (2021). Plasticity in parental effects confers rapid larval thermal tolerance in the estuarine anemone Nematostella vectensis. J. Exp. Biol. 224 (5):jeb236745. doi: 10.1242/jeb.236745
Rogers L. A., Wilson M. T., Duffy-Anderson J. T., Kimmel D. G., Lamb J. F. (2021). Pollock And “the Blob”: impacts of a marine heatwave on walleye pollock early life stages. Fisheries Oceanogr. 30 (2), 142–158. doi: 10.1111/fog.12508
Rogers-Bennett L. (2013). Chapter 27. Strongylocentrotus franciscanus and Strongylocentrotus purpuratus. Dev. Aquac. Fish. Sci. 38, 413–435.
Rogers-Bennett L., Catton C. A. (2019). Marine heat wave and multiple stressors tip bull kelp forest to sea urchin barrens. Sci. Rep. 9 (1), 15050. doi: 10.1038/s41598-019-51114-y
Sanford E., Sones J. L., García-Reyes M., Goddard J. H. R., Largier J. L. (2019). Widespread shifts in the coastal biota of northern California during the 2014–2016 marine heatwaves. Sci. Rep. 9 (1), 4216. doi: 10.1038/s41598-019-40784-3
Schier A. F. (2007). The maternal-zygotic transition: death and birth of RNAs. Science 316 (5823), 406–407. doi: 10.1126/science.1140693
Sconzo G., Ferraro M. G., Amore G., Giudice G., Cascino D., Scardina G. (1995). Activation by heat shock of hsp70 gene transcription in Sea urchin embryos. Biochem. Biophys. Res. Commun. 217 (3), 1032–1038. doi: 10.1006/bbrc.1995.2873
Sewell M. (2005). Utilization of lipids during early development of the sea urchin Evechinus chloroticus. Mar. Ecol. Prog. Ser. 304, 133–142. doi: 10.3354/meps304133
Shama L. N. S. (2015). Bet hedging in a warming ocean: predictability of maternal environment shapes offspring size variation in marine sticklebacks. Global Change Biol. 21 (12), 4387–4400. doi: 10.1111/gcb.13041
Shanks A. L., Rasmuson L. K., Valley J. R., Jarvis M. A., Salant C., Sutherland D. A., et al. (2020). Marine heat waves, climate change, and failed spawning by coastal invertebrates. Limnol. Oceanogr. 65 (3), 627–636. doi: 10.1002/lno.11331
Shi D., Zhao C., Chen Y., Ding J., Zhang L., Chang Y. (2020). Transcriptomes shed light on transgenerational and developmental effects of ocean warming on embryos of the sea urchin Strongylocentrotus intermedius. Sci. Rep. 10 (1), 7931. doi: 10.1038/s41598-020-64872-x
Skadsheim A. (1989). Regional variation in amphipod life history: effects of temperature and salinity on breeding. J. Exp. Mar. Biol. Ecol. 127 (1), 25–42. doi: 10.1016/0022-0981(89)90207-4
Smale D. A., Wernberg T., Oliver E. C. J., Thomsen M., Harvey B. P., Straub S. C., et al. (2019). Marine heatwaves threaten global biodiversity and the provision of ecosystem services. Nat. Climate Change 9 (4), 306–30+. doi: 10.1038/s41558-019-0412-1
Smith K. E., Burrows M. T., Hobday A. J., King N. G., Moore P. J., Gupta A. S., et al. (2023). Biological impacts of marine heatwaves. Annu. Rev. Mar. Sci. 15 (1), null. doi: 10.1146/annurev-marine-032122-121437
Smith K. E., Burrows M. T., Hobday A. J., Sen Gupta A., Moore P. J., Thomsen M., et al. (2021). Socioeconomic impacts of marine heatwaves: global issues and opportunities. Science 374 (6566), eabj3593. doi: 10.1126/science.abj3593
Smith J. G., Tinker M. T. (2022). Alternations in the foraging behaviour of a primary consumer drive patch transition dynamics in a temperate rocky reef ecosystem. Ecol. Lett. 25 (8), 1827–1838. doi: 10.1111/ele.14064
Stimpson W. (1857). On the crustacea and echinodermata of the Pacific shores of north America (Boston: Boston Society of Natural History).
Strader M. E., Wolak M. E., Simon O. M., Hofmann G. E. (2022). Genetic variation underlies plastic responses to global change drivers in the purple sea urchin, Strongylocentrotus purpuratus. Proc. R. Soc. B: Biol. Sci. 289 (1981), 20221249. doi: 10.1098/rspb.2022.1249
Strathmann R. R. (1971). The feeding behavior of planktotrophic echinoderm larvae: mechanisms, regulation, and rates of suspension feeding. J. Exp. Mar. Biol. Ecol. 6, 109–160. doi: 10.1016/0022-0981(71)90054-2
Strathmann M. F. (1987). Reproduction and development of marine invertebrates of the northern Pacific coast data and methods for the study of eggs, embryos, and larvae (Seattle WA USA: University of Washington Press).
Suckling C. C., Clark M. S., Richard J., Morley S. A., Thorne M. A. S., Harper E. M., et al. (2015). Adult acclimation to combined temperature and pH stressors significantly enhances reproductive outcomes compared to short-term exposures. J. Anim. Ecol. 84 (3), 773–784. doi: 10.1111/1365-2656.12316
Sultan S. E. (2007). Development in context: the timely emergence of eco-devo. Trends Ecol. Evol. 22 (11), 575–582. doi: 10.1016/j.tree.2007.06.014
Tanner R., Bowie R., Stillman J. H. (2020). Thermal exposure and transgenerational plasticity influence embryonic success in a bivoltine estuarine sea hare. Mar. Ecol. Prog. Series. doi: 10.3354/meps13207
Truong K. N., Vu N. A., Doan N. X., Bui C. V., Le M. H., Vu M. T. T., et al. (2022). Transgenerational exposure to marine heatwaves ameliorates the lethal effect on tropical copepods regardless of predation stress. Ecol. Evol. 12 (8):e9149. doi: 10.1002/ece3.9149
Waite H. R., Sorte C. J. B. (2022). Negative carry-over effects on larval thermal tolerances across a natural thermal gradient. Ecology 103 (1), e03565. doi: 10.1002/ecy.3565
Weitzman B., Konar B., Iken K., Coletti H., Monson D., Suryan R., et al. (2021). Changes in rocky intertidal community structure during a marine heatwave in the northern Gulf of Alaska. Front. Mar. Sci. 8. doi: 10.3389/fmars.2021.556820
Wernberg T. (2021). “Marine heatwave drives collapse of kelp forests in Western Australia,” in Canadell J. G., Jackson R. B.. (eds) Ecosystem collapse and climate change. Ecological Studies, vol 241. Springer, Cham.
Wong J. M., Hofmann G. E. (2020). The effects of temperature and pCO2 on the size, thermal tolerance and metabolic rate of the red sea urchin (Mesocentrotus franciscanus) during early development. Mar. Biol. 167 (3), 33. doi: 10.1007/s00227-019-3633-y
Wong J. M., Johnson K. M., Kelly M. W., Hofmann G. E. (2018). Transcriptomics reveal transgenerational effects in purple sea urchin embryos: adult acclimation to upwelling conditions alters the response of their progeny to differential pCO2 levels. Mol. Ecol. 27 (5), 1120–1137. doi: 10.1111/mec.14503
Wong J. M., Kozal L. C., Leach T. S., Hoshijima U., Hofmann G. E. (2019). Transgenerational effects in an ecological context: conditioning of adult sea urchins to upwelling conditions alters maternal provisioning and progeny phenotype. J. Exp. Mar. Biol. Ecol. 517, 65–77. doi: 10.1016/j.jembe.2019.04.006
Zhao L., Shirai K., Tanaka K., Milano S., Higuchi T., Murakami-Sugihara N., et al. (2020). A review of transgenerational effects of ocean acidification on marine bivalves and their implications for sclerochronology. Estuar. Coast. Shelf Sci. 235:106620. doi: 10.1016/j.ecss.2020.106620
Keywords: marine heatwave, transgenerational effects, life history, thermal tolerance, marine invertebrate, sea urchin, Strongylocentrotus purpuratus
Citation: Chamorro JD, McDonald AM and Hofmann GE (2023) Transgenerational plasticity as a mechanism of response to marine heatwaves in the purple sea urchin, Strongylocentrotus purpuratus. Front. Mar. Sci. 10:1212781. doi: 10.3389/fmars.2023.1212781
Received: 26 April 2023; Accepted: 26 June 2023;
Published: 21 July 2023.
Edited by:
Anthony (Tony) John Hickey, The University of Auckland, New ZealandReviewed by:
Natali Delorme, Cawthron Institute, New ZealandBrendon Dunphy, The University of Auckland, New Zealand
Copyright © 2023 Chamorro, McDonald and Hofmann. This is an open-access article distributed under the terms of the Creative Commons Attribution License (CC BY). The use, distribution or reproduction in other forums is permitted, provided the original author(s) and the copyright owner(s) are credited and that the original publication in this journal is cited, in accordance with accepted academic practice. No use, distribution or reproduction is permitted which does not comply with these terms.
*Correspondence: Jannine D. Chamorro, amRjaGFtb3Jyb0B1Y3NiLmVkdQ==