- State Key Laboratory of Marine Resource Utilization in South China Sea, Hainan Aquaculture Breeding Engineering Research Center, Hainan Academician Team Innovation Center, Hainan University, Haikou, China
The fluctuations of dissolved oxygen (DO) often lead to hypoxia in aquaculture, which has a huge adverse impact on fish. This study mainly investigated the effects of long-term hypoxia on oxidative stress, immune response, and cell apoptosis in the liver of golden pompano (Trachinotus blochii), which is not tolerant to hypoxia. So we conducted a 14 day low oxygen stress experiment on the golden pompano with a DO of 3.0 ± 0.2 mg/L, then restore the DO to normal levels and continue the 14 day reoxygenation experiment. Results showed that hypoxia and reoxygenation led to significant changes in liver structure. During hypoxia and reoxygenation, the expression of oxidative stress related genes (SOD1, SOD2, GSH-Px, and CAT) and levels of antioxidant enzymes (CAT and MDA) in the liver were increased. Liver lysozyme activity and the relative expression of the pro-inflammatory factors interleukin (IL)-1β were significantly increased, but the expression of IL-34 was down-regulated during hypoxia. The expression of IL-12β was significantly increased during reoxygenation. The expression of anti-inflammatory factor IL-11 was decreased duringreoxygenation. The expression of toll like receptors (TLRs) -7, -8, and -9 increases after hypoxia and decreases after reoxygenation, indicating that both hypoxia and reoxygenation affect the immune response. In addition, during hypoxia and reoxygenation, TUNEL-positive signals increased, the bcl2/bax ratio decreased, the expression levels of caspases-3 and -8 were significantly up-regulated during hypoxia, and expression levels of caspases-9 was up-regulated during reoxygenation. In summary, hypoxia and reoxygenation can cause oxidative stress, induce inflammatory reactions, inhibit immune processes, activate apoptosis, and lead to liver damage of the golden pompano, which may be irreversible.
1 Introduction
The dissolved oxygen (DO) in water is a crucial ecological factor for aquatic aerobic species (Chen et al., 2017). Changes in DO are generally caused by environmental changes, as well as human activities (Tomanek, 2014; Levin and Breitburg, 2015; Pillet et al., 2016). Changes in DO concentrations affect fish growth, ingestion, and respiration as well as the physiological functions related to the immune response, fertility, and metabolism (Li et al., 2018; Magnoni et al., 2018; Xia et al., 2018; Ding et al., 2020). Different aquatic organisms face a wide range of oxygen thresholds from 0.28 to 4 mg/L in water bodies but the actual threshold is unknown because various aquatic species respond differently to low oxygen conditions, even a slight reduction in oxygen can adversely affect some organisms (Service, 2004; Paerl, 2006; Vaquer-Sunyer and Duarte, 2008). Furthermore, short-term and long-term hypoxia have irreversible harmful effects on fish (Leveelahti et al., 2014). Therefore, hypoxia is a problem in aquaculture with high stocking and intensive feeding (Farrell and Richards, 2009).
When fish are faced with fluctuating oxygen levels, they exhibit physiological and biochemical responses, such as activating antioxidant defenses and adjusting metabolism to adapt to stressful conditions, such as Cirrhinus mrigala (Weber et al., 2016; Varghese et al., 2017). However, when the duration of hypoxia is too long, the natural defense of fish is unable to cope with such adverse conditions. Previous studies have shown that hypoxia will interfere with the electron transport chain of Micropterus salmoides and produce excessive reactive oxygen species (ROS) (Yang et al., 2017). The accumulation of ROS stimulates the antioxidant defense system, which is composed of superoxide dismutase (SOD), catalase (CAT), and glutathione peroxidase (GSH-Px) as well as non-enzymatic antioxidants (Liu et al., 2011). Although fish, can defend against ROS, excessive levels of ROS cause oxidative damage, leading to the formation of malondialdehyde (MDA), which reflects the extent of oxidative damage, this has been confirmed in fish such as Micropterus salmoides and Paralichthysolivaceus (Cao et al., 2012; Yang et al., 2017).
Studies have shown a close correlation between oxidative stress and immunity and apoptosis and that oxidative stress triggers inflammation. Disease resistance in fish mainly relies on the innate immune system compared with mammals (Van Doan et al., 2017). The activity of the humoral immune factor lysozyme (LZM) reflects the strength of the immunity of fish to a certain extent (Zhou, 2006). Acid phosphatase (ACP) and alkaline phosphatase (AKP) are two important phosphatases that participate in degrading foreign proteins, carbohydrates, and lipids (Liu et al., 2004). Hypoxia significantly inhibits the immune system of Oreochromis niloticus compared with normoxia (Abdel-Tawwab et al., 2015). Long-term hypoxia decreases or delays the expression of immune-related genes in Salmo salar, thereby altering the immune response (Kvamme et al., 2013). A hypoxic environment produces excessive amounts of ROS and oxidative damage to tissues, which stimulates the PI3K/p38MAPK and NF-kB signaling pathways, resulting in the expression of downstream inflammation-related factors (Wu et al., 2019).
Oxidative stress promotes apoptosis (Zhang et al., 2018), such as lipid peroxidation and DNA damage, possibly leading to cell apoptosis, depending on the severity and duration of the exposure (Lushchak Volodymyr, 2014). In addition, the apoptosis regulator Bcl-2 acts as an inhibitor of apoptosis under hypoxic conditions, while Bax acts oppositely (Zheng et al., 2021). The caspase family is essential in extracellular and intracellular apoptotic pathways as well. The expression trends of the caspase-3 and caspase-9 genes in Acipenser sinensis and Tanichthys albonubes are consistent with environmental stress and increase with the stress duration (Kim et al., 2021). Prolonged exposure to low oxygen, for whatever reason, can lead to reduced feed intake, stunted growth and a high propensity for disease (Fitzgibbon et al., 2007; Portner, 2010).
The golden pompano, Trachinotus blochii, is mainly distributed in the temperate and subtropical waters of the Pacific and Indian Oceans. Bescuse of its high nutritional value makes it a popular commercial fish. The annual output of golden pompano has continued to increase in the past 10 years from 25,000 to 240,000 tons. With the continuous expansion of the scale of the golden pompano breeding industry, Improving aquaculture density on the basis of existing aquaculture conditions has become an effective means to increase profits. However, the oxygen consumption of the golden pompano is high (Sun et al., 2022), and the fluctuations in DO have a significant impact on its survival and growth. Increasing the density of aquaculture undoubtedly reduces the availability of dissolved oxygen in water, causing it to face low dissolved oxygen conditions for a long time. Moreover, it is mostly cultivated in nearshore waters, and the water quality conditions and environmental factors have a significant impact, leading to a significant increase in the incidence, duration, and severity of hypoxia stress. In this study, we simulated hypoxic conditions to assess the activities of oxidative stress biomarkers (MDA, SOD, CAT, and GSH-Px), innate immune-related enzymes (LZM, ACP, and AKP), as well as the expression of oxidative stress-related genes (SOD1, SOD2, SOD3, CAT, and GSH-Px) and immune-related genes (IL-1β, IL-12β, IL-11, IL-34, TLR7, TLR8, and TLR9). Hematoxylin and eosin (H&E) staining was used to observe the liver microstructure and terminal deoxynucleotidyl transferase dUTP nick end labeling (TUNEL) staining was performed to detect apoptosis. Then, we determined the expression of apoptosis-related factors (p53, caspase-8, caspase-3, caspase-9, Bcl-2, and Bax) to analyze the effect of hypoxia and reoxygenation on apoptosis of liver. The results will contribute to a better understanding of the regulatory mechanism of the response of fish to hypoxic stress and may help improve the production and breeding of golden pompano.
2 Materials and methods
2.1 Experimental fish and maintenance
A total of 150 healthy golden pompano were provided by Hainan Blue Grain Technology Co., Ltd (Sanya, China). The study fish were randomly selected from the same parent at the age of 6 months, with an average body weight of 190.0 ± 10.0 g. After transport to the laboratory, the fish were assigned to three plastic tanks (500 L) containing aerated seawater and held for 1 week. During this period, the water quality and water environment remained stable with DO (7.0 ± 0.5 mg/L), water temperature (26.0 ± 1.0°C), salinity (20 ± 10 ‰), total ammonia nitrogen (< 0.02 mg/L), and a natural photoperiod. The fish were fed a commercial pellet feed equal to 2% of the fish’s body weight (Tianma Co., Fujian, China) at 8:30 and 17:00. About 30% of the water was exchanged every day.
2.2 Hypoxic stress experiment
The one hundred and fifty experimental fish were randomly and equally distributed into the three fish tanks. During the experiment, except for the dissolved oxygen content, all other conditions were the same as the previous environmental conditions, including feeding and water exchange operations. The oxygen concentration in the tanks was reduced from the normal concentration (7.0 ± 0.2 mg/L) to the desired concentration for the hypoxic stress experiment (3.0 ± 0.2 mg/L) within 3 hours of stocking by filling the tanks with nitrogen and air. The DO level was monitored in real-time using an automatic DO controller that automatically adjusted the oxygen level in the culture tank. When the oxygen concentration in the water was higher than the set value, the controller automatically opened a valve to introduce nitrogen. At the same time, to ensure hypoxic conditions, we used the HQ30d portable dissolved oxygen meter (HACH, Loveland, CO, USA) to detect DO in the water every 2 hours (The experimental members rotate on duty every day). We sampled at the beginning of the experiment as a starting control (C). Samples were collected after 24 hours of hypoxia, which was recorded as day 1 (H1). Then, samples were collected on day 14 of hypoxia (H14) and day 14 of reoxygenation (R14), in which the DO was returned to the normal level for 14 days (Figure 1).
2.3 Sample collection
Three fish from each tank were randomly selected to collect samples on days 0 (as a control), 1 (H1), 14 (H14), and 28 (R14) and anesthetized by immersion in MS-222 (100 mg/L) (0.05% solution, Sigma, St. Louis, MO, USA). The spine of the fish was severed, and immediately draw blood from the caudal vein using heparin-rinsed syringes (1,250 IU/mL) and the fish liver was dissected. The liver was divided into three parts. One-third was fixed in 4% paraformaldehyde for H&E staining, the histological examination, and the TUNEL assay. The remaining two-thirds were placed in enzyme-free cryovials and stored at −80°C for enzyme activity assays and related mRNA expression assays.
2.4 Liver histological analysis
The one-third of the liver that was fixed in 4% paraformaldehyde solution was dehydrated through an ethanol series (70%, 90%, and 100%). The samples were cleared in xylene and embedded in paraffin. The wax blocks were sectioned at 5 µm using a microtome (Leica RM2125, Nussloch, Germany). The 5 µm slices were stained with H&E after dewaxing. The prepared sections were examined and photographed via microscopy (ECLIPSE 200, Nikon, Tokyo, Japan).
2.5 TUNEL assay
The paraffin-embedded liver tissue sections from one-third of the liver were dewaxed and rehydrated, then further processed using TDT according to the manual for the TUNEL apoptosis detection kit (Wuhan Saiweier Co., Wuhan, China). After reacting with the DAB chromogenic solution, the apoptotic cells appeared brownish-yellow. The apoptotic cells were imaged using a microscope.
2.6 Enzyme activity analyses
One-third of the liver was treated according to the instructions provided with the enzyme activity detection kit, homogenized in precooled saline to make a 10% mixture, and centrifuged at 2,500 rpm for 10 min at 4°C to obtain the supernatant. The whole blood was centrifuged to collect the plasma (2,500 rpm for 15 min at 4°C). The protein concentration was determined using a Coomassie Bright Blue staining kit (Product Code: A045-2). All indicators were measured by corresponding enzyme assay kits provided by the Nanjing Jiancheng Biological Research Institute (Nanjing, China, http://www.njjcbio.com/). Indicators included total antioxidant capacity T-(AOC) (A015-1), malondialdehyde (MDA) (A003-1), superoxide dismutase (T-SOD) (A001-1), catalase (CAT) (A007-1-1), glutathione peroxidase (GSH-Px) (A005-1), lysozyme (LZM) (A050-1-1), alkaline phosphatase (AKP) (A059-1-1), and acid phosphatase (ACP) (A060-1-1). Enzyme activities were measured using a microplate reader according to the wavelength, temperature, and time as described by the manufacturer’s protocols with the kits.
2.7 mRNA expression by quantitative polymerase chain reaction (PCR)
Total RNA was extracted from the remaining one-third of the liver tissue using TRIzol reagent (Invitrogen, Carlsbad, CA, USA). The RNA was detected as follows: (1) integrity was determined by gel electrophoresis, and (2) purity and concentration were examined using the NanoDrop Spectrophotometer 2000c (Thermo, Waltham, MA, USA). First-strand cDNA was synthesized using the HiScript®III All-in-one RT SuperMix Perfect for qPCR kit (Vazyme, Nanjing, China). The ChamQ Universal SYBR qPCR Master Mix kit (Vazyme) was used for qPCR. The total amplification reaction mixture was 10 μL, comprised of 5 μL of SYBR Master mix (2×), 0.2 μL of each primer, 4.1 μL of dd-H2O, and 0.5 μL of cDNA. The reaction program was performed at 95°C for 30 s, followed by 40 cycles at 95°C for 5 min, annealing at 60°C for 30 s, and 72°C for 30 s. The 2−ΔΔCT method was used to analyze relative gene expression (Livak and Schmittgen, 2001). β-actin was used as the internal reference gene to determine the relative expression levels of the genes, and amplification efficiency was > 90% (Sun et al., 2021). The core fragments of the genes were obtained from the NCBI database and our unpublished data. Primer Premier 5 software was used to design the primers. Table 1 lists the primer sequences.
2.8 Statistical analysis
The data were tabulated in Microsoft Excel (Microsoft Inc., Redmond, WA, USA). The statistical analysis was performed using SPSS 22.0 software (SPSS Inc. Chicago, IL, USA). The results are displayed as mean ± standard error (mean ± SE). Differences between controls and experimental groups were analyzed using F-test and independent sample t-tests. A P-value <0.05 was considered significant. We used GraphPad Prism 6.0 software (GraphPad Software Inc. La Jolla, CA, USA) to create the graphs.
3 Results
3.1 Liver histological observations
As shown in Figure 2A, the liver cytoplasm was homogeneous, and the cell nuclei were regular, round, and located in the centers of the cells at the control point (0 day). Hypoxia stress for one day did not cause changes in liver tissue structure (Figure 2B). However, the liver tissue structure changed significantly after 14 days of hypoxia, with an increase in the number of intracellular vacuoles, an increase in cell gaps, and liver cell atrophy (Figure 2C). Although the experimental fish experienced 14 days of reoxygenation, the morphology of the hepatocytes did not improve and the liver cells remained swollen with shifted nuclei and enlarged vacuoles (Figure 2D).
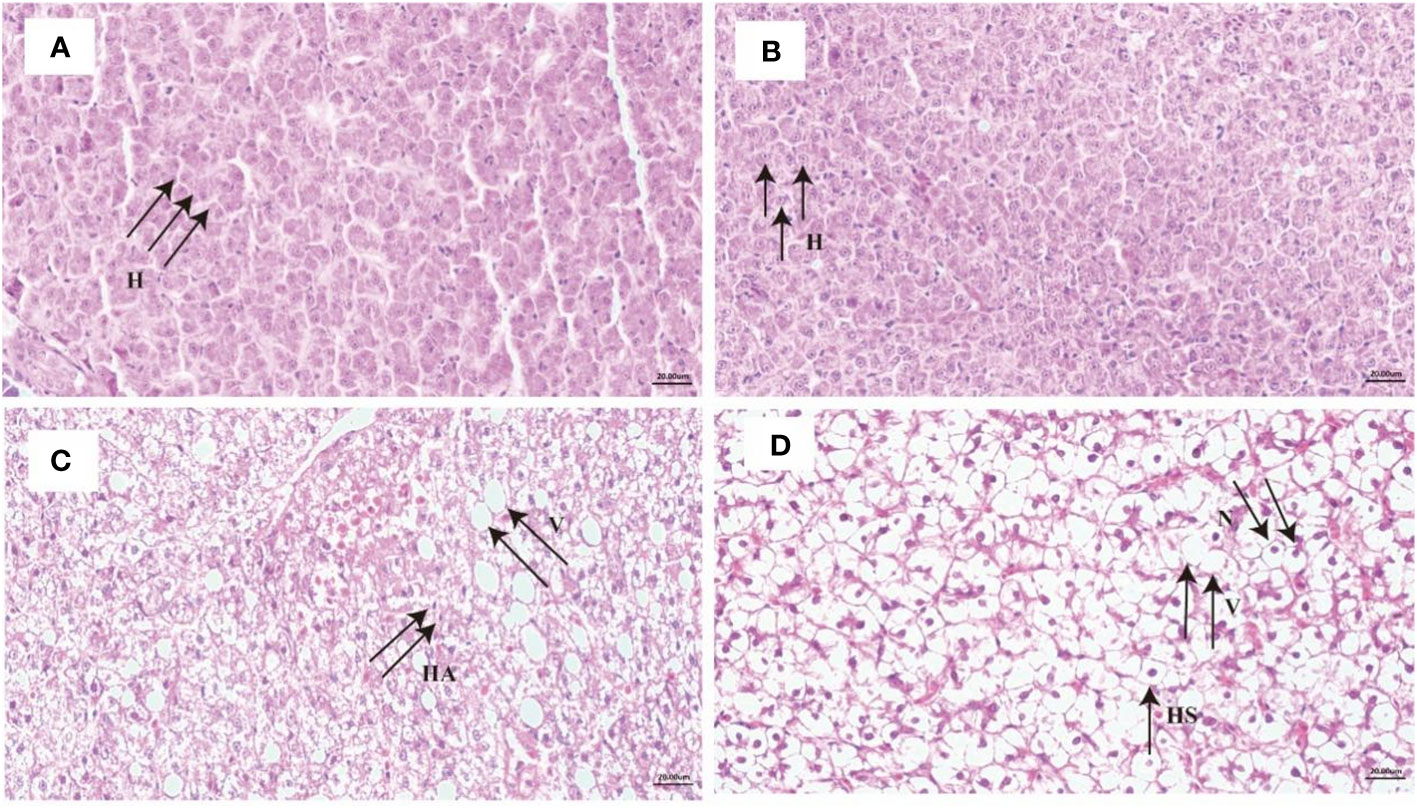
Figure 2 Liver histology in liver under long-term hypoxia and reoxygenation. (A) control, (B) 1 day of hypoxia, (C) 14 days of hypoxia and (D) 14 days of reoxygenation. Scale Bar is 20 μm; sections were 6-7 μm thick and stained with hematoxylin and eosin (H&E). H, hepatocytes; V, vacuole; HS, hepatocyte swelling; N, nuclear translocation; HA, hepatocyte atrophy.
3.2 Liver TUNEL assay
The TUNEL assay results of the liver were shown in Figure 3. Apoptosis signals (appeared yellow-brown) were almost not detected at the control point and H1 point (Figures 3A, B). In contrast, TUNEL-positive apoptotic cells (appeared yellow-brown) began to appear after 14 days of hypoxia (Figure 3C). The number of apoptotic cells were significant increased after 14 days of reoxygenation (p < 0.01) (Figures 3D, E).
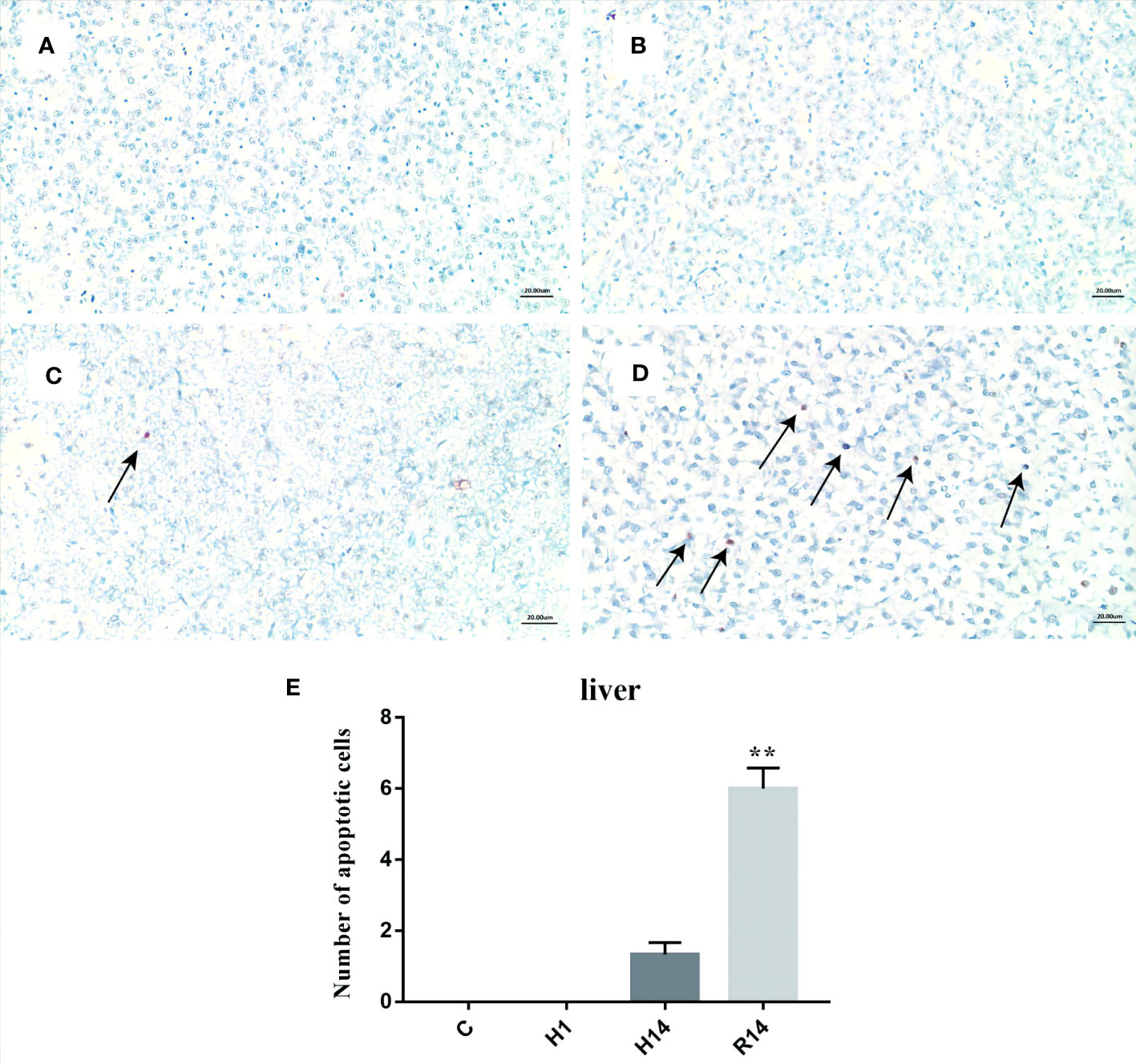
Figure 3 TUNEL staining analysis of liver tissue under long-term hypoxia and reoxygenation. (A) control, (B) 1 day of hypoxia, (C) 14 days of hypoxia, (D) 14 days of reoxygenation., and (E) number of apoptotic cells. Arrow: Tunel positive cells. Scale Bar is 20 μm. ** stand for extremely significant differences (p < 0.01) between the different point.
3.3 Plasma and liver antioxidant and immune enzyme activities
The activity of plasma CAT was significant increased after 14 days of hypoxia (p < 0.05), and restore to control level after reoxygenation (Figure 4A). The activity of plasma T-SOD was stable during entire experimental process (Figure 4B). The activity of plasma GSH-Px continues to increase after 1 day of hypoxic stress and remained at a high level after reoxygenation (p < 0.01) (Figure 4C). The content of plasma MDA was significant only increased after 14 days of hypoxia (p < 0.05), and restore to control level after reoxygenation (Figure 4D). The activity of plasma LZM was significant decreased after 1 days of hypoxia (p < 0.01), and significant decreased after reoxygenation (p < 0.01) (Figure 4E).
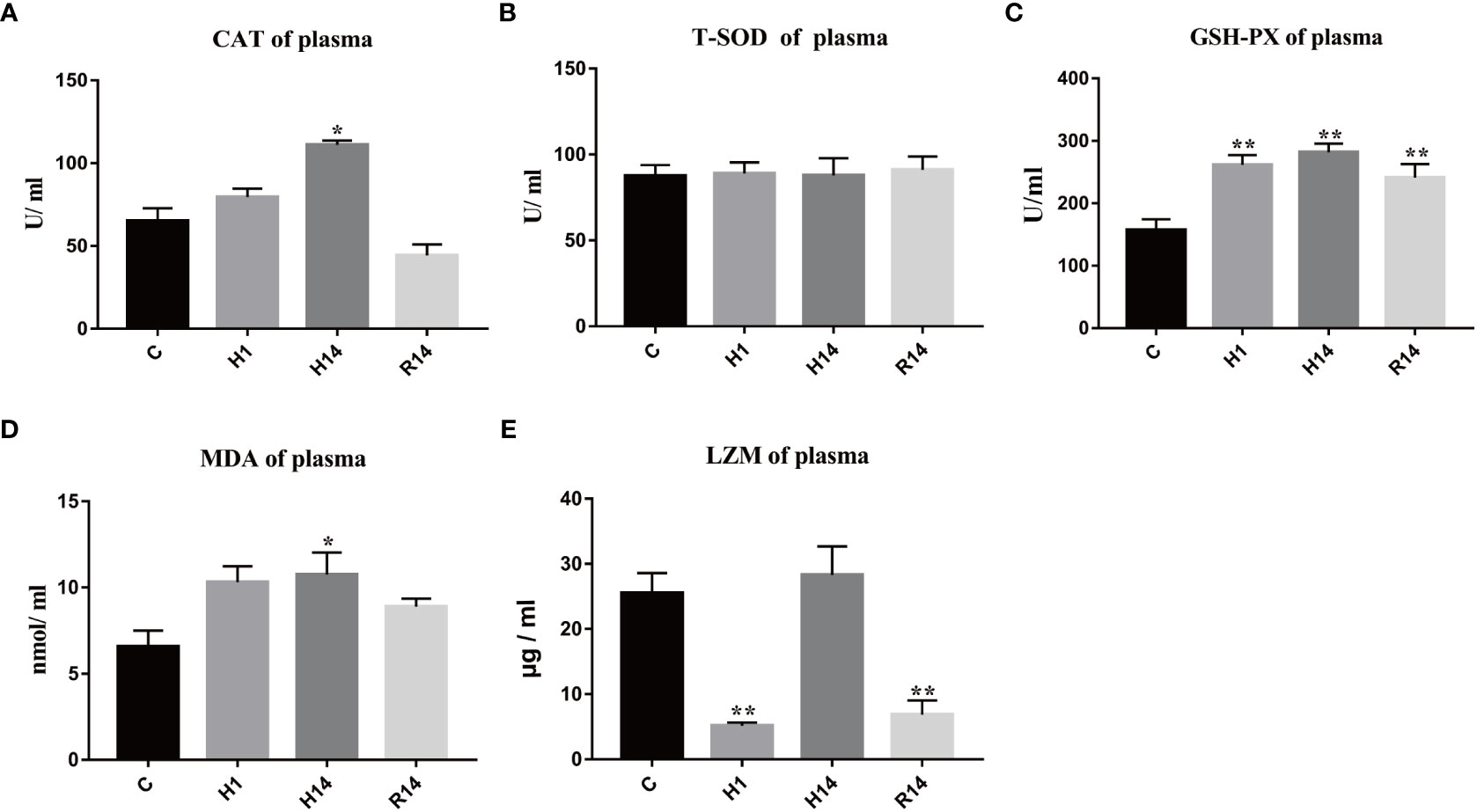
Figure 4 Changes in enzyme activity caused by long-term hypoxia and reoxygenation in plasma. Data are presented as the means ± SE (n = 3), * and ** stand for significant difference (p < 0.05) and extremely significant differences (p < 0.01) between the different point.
The activity of liver CAT was also significant increased after 14 days of hypoxia (p < 0.05), and restore to control level after reoxygenation (Figure 5A). The activity of liver T-SOD was only decreased after 14 days of hypoxia (p < 0.01) (Figure 5B). The activity of liver GSH-Px continues to decrease after 1 day of hypoxic stress and remained at a low level after reoxygenation (p < 0.01) (Figure 5C). The content of liver MDA was significant only increased after 14 days of hypoxia (p < 0.05), and restore to control level after reoxygenation (Figure 5D). The activity of liver T-AOC was decreased after 14 days of hypoxia and remained at a low level after reoxygenation (p < 0.05) (Figure 5E). The activity of liver LZM continues to increase after 1 day of hypoxic stress and remained at a high level after reoxygenation (p < 0.01) (Figure 5F). The activity of liver ACP was only decreased after 1 days of hypoxia (p < 0.05) (Figure 5G). But the activity of liver AKP was only decreased after 1 days of reoxygenation (p < 0.05) (Figure 5H).
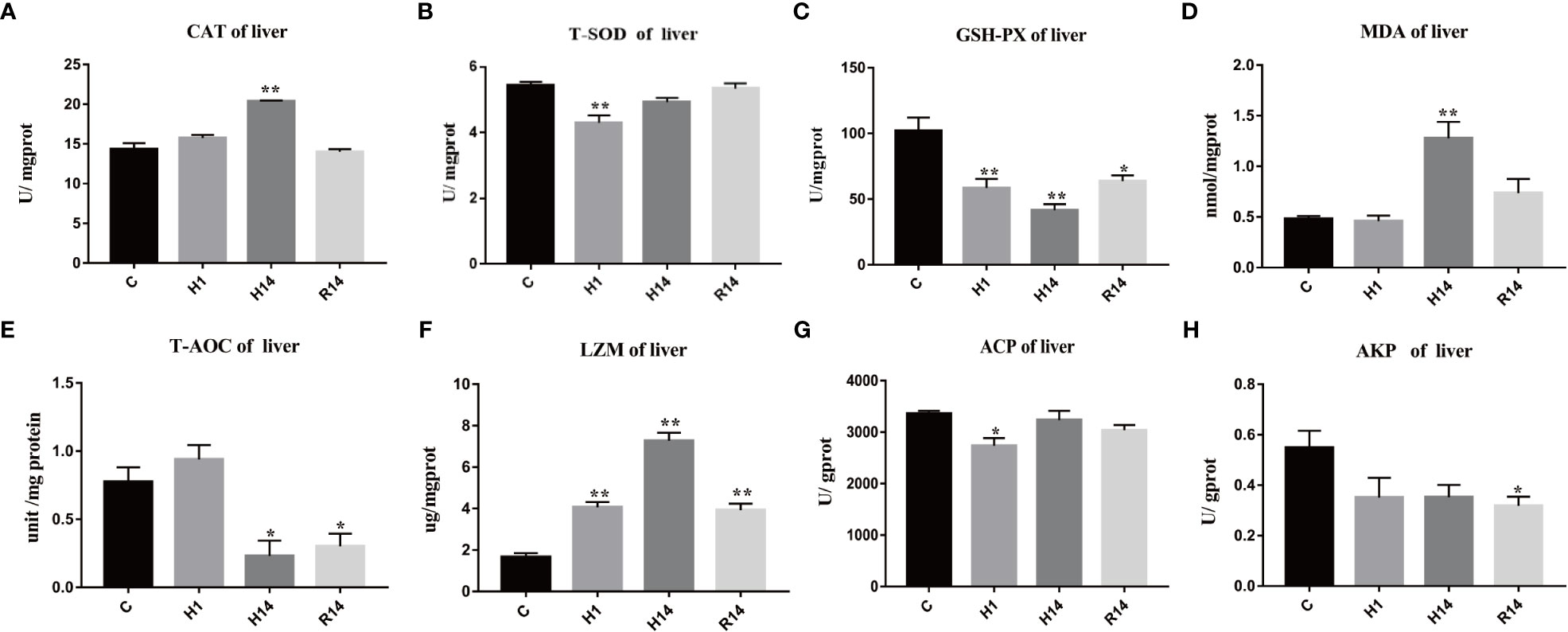
Figure 5 Changes in enzyme activity caused by long-term hypoxia and reoxygenation in liver. Data are presented as the means ± SE (n = 3), * and ** stand for significant difference (p < 0.05) and extremely significant differences (p < 0.01) between the different point.
3.4 Expression of oxidative stress genes in the liver
The expression level of CAT was significantly up-regulated after 1 day and 14 day of hypoxia (p < 0.01), and restore to control level after reoxygenation (Figure 6A). The expression level of GSH-Px was only up-regulated after reoxygenation (p < 0.05) (Figure 6B). The expression level of SOD1 continues to up-regulated after 1 day of hypoxic stress and remained at a high expression level after reoxygenation (p < 0.01) (Figure 6C). The expression level of SOD2 was significantly up-regulated after 1 day (H1) of hypoxia and restore to control level after reoxygenation (Figure 6D). The expression level of SOD3 continues to down-regulated after 1 day of hypoxic stress and remained at a low expression level after reoxygenation (p < 0.01) (Figure 6E).
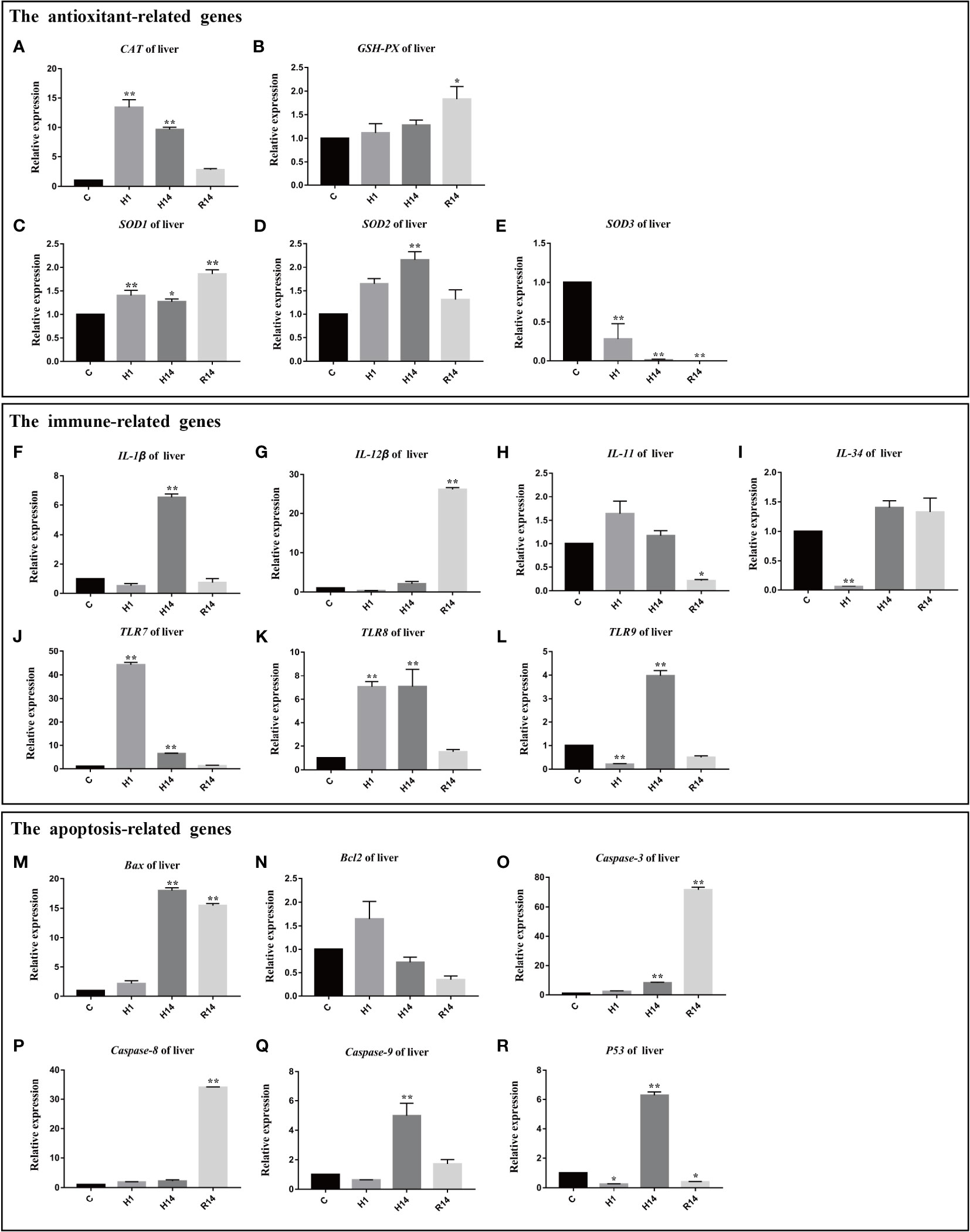
Figure 6 Expression of genes related to antioxidant, immune, regulated and apoptosis under long-term hypoxia and reoxygenation in liver. Data are presented as the means ± SE (n = 3), * and ** stand for significant difference (p < 0.05) and extremely significant differences (p < 0.01) between the different point.
3.5 Immune-related gene expression in the liver
The expression level of IL-1β was significantly up-regulated after 14 day of hypoxia (p <0.01) and restore to control level after reoxygenation (Figure 6F). The expression level of IL-12β was up-regulated after reoxygenation (p < 0.01) (Figure 6G). The expression level of IL-11 was down-regulated after reoxygenation (p < 0.01) (Figure 6H). The expression level of IL-34 was significantly down-regulated after 1 day of hypoxia (p < 0.01), and restore to control level after 14 day of hypoxia (Figure 6I). The expression levels of TLR7 and TLR8 were significantly increased after 1 day and 14 day of hypoxia (p < 0.01), and restore to control level after reoxygenation (Figures 6J, K). The expression level of TLR9 was significantly down-regulated after 1 day of hypoxia (p < 0.01), and significantly up-regulated after 14 days of hypoxia (p < 0.01), it returned to normal levels after reoxygenation (Figure 6L).
3.6 Expression of apoptosis-related genes in the liver
The expression level of Bax was significantly up-regulated after 14 day of hypoxia (p < 0.01) and remained at a high expression level after reoxygenation (p < 0.01) (Figure 6M). The expression level of Bcl2 was stable during entire experimental process (p > 0.05) (Figure 6N). The expression level of caspase-3 was significant up-regulated after 14 day of hypoxia (p < 0.01) and remained at a high expression level after reoxygenation (p < 0.01) (Figure 6O). The expression level of caspase-8 was only significantly up-regulated after reoxygenation (p < 0.01) (Figure 6P). The expression level of caspase-9 was significantly up-regulated after 14 day of hypoxia (p < 0.01), and restore to control level after reoxygenation (Figure 6Q). The expression level of P53 was significantly down-regulated after 1 day of hypoxia (p < 0.01), and significantly up-regulated after 14 days of hypoxia (p < 0.01), then it was significantly down-regulated after reoxygenation (p < 0.01) (Figure 6R).
4 Discussion
4.1 Sustained hypoxia and reoxygenation cause damage to the liver
Changes in tissue structure associated with the response to hypoxia in the teleost liver have been reported by several studies, such as cell hypertrophy, vacuolization, and cellular deformation (Wang et al., 2017; Silva et al., 2019). In this study, the number of vacuoles in hepatocytes increased after chronic hypoxic stress, and the intercellular spaces became larger. This finding is similar to the results of Chen et al. (2017) and was mainly due to the oxidative damage to liver tissue caused by prolonged hypoxic stress, which was confirmed by the oxidative stress state of the liver of golden pompano in our experiment.
After reoxygenation, the damage to the liver tissue did not recover and appeared to be more severe, with translocation of nuclei and a marked increase in the number of vacuoles. In mammals, ischemia-reperfusion (I/R) leads to oxidative damage to the liver (Kang et al., 2000), and hypoxia-reoxygenation in fish reproduces key features of the mammalian model. Similar results were reported by Yan et al. (2021) in which liver cell damage became worse during reoxygenation in Lateolabrax maculatus. However, there are also different results, such as the liver tissue damage was observed after 3 h of hypoxic stress in Rachycentron canadum, but the liver structure returned to normal, with neatly arranged cells and no vacuolization after a prolonged reoxygenation time (Huang et al., 2021). This different result may be related to the rate of increasing dissolved oxygen, as re-establishing the oxygen level leads to increased ROS production and the resulting oxidative stress causes tissue damage (Garbarino et al., 2015; Hermes-Lima et al., 2015). Based on our unpublished data, slowly increasing the DO minimized this kind of damage. Therefore, we suggest that the rate of increase in the DO during the early stage of reoxygenation should not be slow, but this requires more comprehensive and in-depth research.
4.2 Long-term hypoxia and reoxygenation induces oxidative stress
Hypoxia-induced oxidative stress is a pervasive response in vertebrates, and is strongly related to immune regulation (Gorr et al., 2010). Therefore, exploring oxidative parameters is important to understand the health status of fish. MDA is an epoxy compound produced by the reaction of free radicals and polyunsaturated fatty acids and has been used as an indicator of the degree of lipid peroxidation (Tsikas, 2017). Similarly, lipid peroxidation increased during hypoxic conditions (2, 4, and 6 weeks) in red seabream (Nam et al., 2020). After 14 days of hypoxic stress, the MDA content in plasma and liver significantly increased, indicating an enhanced cellular oxidative stress under hypoxic conditions. Moreover, the antioxidant enzyme, including GSH-Px, SOD, and CAT play roles in the antioxidant defense system (Livingstone, 2003). SOD is the only enzyme that acts on free radicals as a substrate and rapidly and effectively disproportionates superoxide anion (O2−) into H2O2 and O2, while CAT and GPx convert H2O2 to H2O and O2, thereby protecting cells from oxidative damage (Cao et al., 2012; Welker et al., 2012). The activity of plasma GSH-Px and CAT were increased after 1 day or 14 day of hypoxia. indicating that antioxidant enzymes CAT and GSH-Px, may play a greater role than SOD, although SOD forms the first line of defense against oxidative stress (Eyckmans et al., 2011; Dawood et al., 2021). The plasma MDA content decreased slightly after reoxygenation but remained higher than the control level, and there was a significant difference between GSH-Px activity and the control level, indicating that oxidative stress occurred during oxygen recovery, which may have been caused by excessive aerobic respiration after recovery of the DO concentration (Bickler and Buck, 2007; Walshe and D'Amore, 2008; Gorr et al., 2010).
Differences between the liver and the plasma may exist to cope with hypoxic stress. The decrease in T-AOC levels in this study suggests that hypoxia and reoxygenation processes reduce the antioxidant capacity of the liver, as T-AOC reflects the free radical scavenging activity throughout the body (Yang et al., 2021). Our results show that only subtle changes in SOD activity occurred or activity remained constant. This may be because the liver failed to respond to the earliest external environmental stress, leading to more oxidative damage (Wang et al., 2021). The other antioxidant enzymes varied widely, such as CAT and GSH-Px. Perhaps these two enzymes play a greater role in the antioxidant system (Sun et al., 2020). In contrast, the CAT and SOD1 genes were transcribed earlier and were up-regulated significantly after hypoxia, emphasizing the generation of hypoxia-induced oxidative stress. The CAT, SOD1, and SOD2 mRNA expression levels continued to increase after 14 days of hypoxia, indicating that long-term hypoxia continues to cause oxidative stress to the liver. The enhancement of antioxidant capacity occurs in preparation for oxidative stress caused by reoxidation (Hermes-Lima and Zenteno-Savın, 2002). GSH-Px and SOD1 expression levels remained high after oxygenation was restored. ROS are over-produced during reperfusion or reoxygenation, and the antioxidant defenses are impaired, leading to inflammation and apoptosis (Granger and Kvietys, 2015). There were temporal differences in the enzyme activities and gene expression of these antioxidant factors, possibly resulting from post-transcriptional initiation and termination as well as post-translational expression of their different forms (Pei et al., 2021).
4.3 Long-term hypoxia and reoxygenation suppresses immune
Continued exposure to a low-oxygen environment damage immune function and can cause death (Nam et al., 2020). The LZM level changes when an animal is subjected to external environmental stress, and mild hypoxic stimulation induces the synthesis of LZM to improve bactericidal ability. In our study, liver LZM activity increased after 14 days of hypoxic stress, indicating that hypoxia induces the production of LZM in the liver to enhance the ability to fight bacterial infections. The LZM levels decreased after reoxygenation but were still higher than in the control group, indicating that the fish were in a state of stress and nonspecific immunity enhanced the immune response to the hypoxic environment. AKP is an immune enzyme and a component of the nonspecific immune system of fish (Ma et al., 2022). In our results, AKP activity decreased. Another study showed that AKP activity decreases after 6 h of hypoxic stress, further indicating that hypoxic stress inhibits the activity of hydrolases involved in immunity. ACP and AKP are important lysosomal enzymes (Liu et al., 2019), and they decreased in our study. It may be that a large amount of ROS were produced, which caused an innate immune function disorder in golden pompano and inhibited ACP and AKP activities. Therefore, prolonged hypoxia not only caused tissue damage but also affected the immune system.
We analyzed the transcript levels of immune-related genes in golden pompano to assess changes in immunity. TLRs are a class of pattern-recognition receptors that induce immune responses by activating downstream signaling pathways through the production of inflammatory cytokines (Kawasaki and Kawai, 2014; Mokhtari et al., 2021). The accumulation of ROS activates TLRs, which triggers an immune response. In our study, the mRNA expression levels of all TLRs increased after hypoxic stress, which promoted the production of pro-inflammatory cytokines and chemokines. Thus, hypoxic stress enhanced susceptibility to subsequent infection and inflammatory signals by up-regulating the TLRs. Studies have shown that the inactivation of TLR9-mediated signal-dependent caspase plays an anti-apoptotic role (Hancz et al., 2012). Upregulating TLR7 inhibits activation of the NF-κB signaling pathway and promotes apoptosis in asthmatic mice (Song et al., 2021). However, after reoxygenation, TLRs expression levels all decreased, and the expression levels of caspases all increased, which promoted apoptosis. Inflammatory responses are controlled by anti- and pro-inflammatory mediators, including small peptides (e.g., cytokines) and transcription factors, such as NF-κB (Biddlestone et al., 2015). Mammalian studies have demonstrated that hypoxia and inflammation coexist and are inversely correlated (Watts and Walmsley, 2019). We determined that hypoxia provoked the expression of the pro-inflammatory factor IL-1β. At this time, reoxygenation up-regulated IL-12β levels. However, hypoxia and reoxygenation reduced the expression of the anti-inflammatory factor IL-11, which could not neutralize the pro-inflammatory factor, thereby failing to protect the liver and promoting apoptosis of hepatocytes (Joza et al., 2001). As chronic hypoxia modulates the transcriptional responses of important cytokines to immune challenges, it is speculated that the chronic hypoxic conditions impaired the innate immune defenses and disease resistance of golden pompano.
4.4 Long-term hypoxia and reoxygenation induces apoptosis in the liver
The antioxidant system of golden pompano activated to protect the cells during the hypoxic stress; however, when the level of oxidative stress exceeded tolerance, programmed cell death occurred. Our TUNEL assay results show that apoptotic signaling began to emerge after the chronic hypoxic stress because hypoxia inhibits the ability to produce ATP; the Na+-K+ ion pump cannot be maintained, and the cells eventually depolarize and undergo necrosis and apoptosis (Nilsson and Östlund-Nilsson, 2008). Hepatocyte apoptosis became more severe during reoxygenation. Studies have shown that hypoxia, reoxygenation, or both lead to increased levels of apoptosis, and that mammals and certain fish induce cell death shortly after reoxygenation (Lefevre et al., 2017). This phenomenon may be caused by H/R injury or the lag phase of the effect.
Apoptosis is a biochemical process of programmed death, triggered by three pathways, and mainly mediated by caspases and regulated by the Bcl2 family of protein molecules of which Bcl-2 and Bax are the most representative anti-apoptotic and pro-apoptotic genes (Yu et al., 2018; Zhang et al., 2022). The ratio of these two determines the direction of cell development (Li et al., 2017). In our study, Bax and Bcl-2 expression levels in the liver of golden pompano increased and decreased, respectively, under hypoxic stress, so the bcl2/bax ratio decreased gradually, which promoted apoptosis of liver cells. This is consistent with the expression trend of the bcl2/bax ratio in Hypophthalmichthys molitrix under hypoxic stress (Ding et al., 2018). After recovery of DO, the expression of Bax increased, that of Bcl2 decreased, and apoptosis was more frequent in hepatocytes. Yan et al. (2021) reported high expression levels of pro-apoptotic genes during reoxygenation, indicating that H/R injury occurred in the liver of Lateolabrax maculatus. Upregulation of p53 under hypoxic conditions promotes the apoptosis signal (Zheng et al., 2021). In the present study, p53 was significantly up-regulated after chronic hypoxic stress. In addition, apoptosis during hypoxia occurs mainly through the extrinsic death receptor pathway (caspase-8) and the endogenous mitochondrial pathway (caspase-9) (Cao et al., 2013; Luzio et al., 2013). These two pathways are triggered by different promoter caspases, but they both cause the downstream accumulation of caspase-3 leading to apoptosis (Ching et al., 2013). In our study, long-trem hypoxia increased the expression levels of the caspase-3 and caspase-9 genes, and reoxygenation recovery also up-regulated the expression of caspase-3 and caspase-8. These results suggest that hypoxic stress aggravated apoptosis through the extrinsic (death receptor) and intrinsic (mitochondrial) liver pathways.
5 Conclusions
T. blochii is an important commercial marine aquaculture fish in southern China. Therefore, intensive and high-density aquaculture has become the choice, despite issues associated with a low-oxygen environment that tends to occur in aquaculture. Hypoxia induced oxidative stress and liver tissue damage, as well as an inflammatory response, and promoted cell apoptosis in golden pompano. In addition, strong oxidative stress was detected during the reoxygenation period, which may have been caused by rapid reoxygenation. Sustained oxidative stress caused physiological disorder, resulting in an aggravated inflammatory response during reoxygenation. Therefore, we suggest that the rate of increase in the DO should not be slow during the early stage of reoxygenation, but this requires more comprehensive research.
Data availability statement
The original contributions presented in the study are included in the article/supplementary materials. Further inquiries can be directed to the corresponding author/s.
Ethics statement
The animal study was reviewed and approved by the Institutional Animal Care and Use Committee (IACUC) of the College of Ocean of Hainan University, Hainan, China, under permit no. HNUAUCC-2022-00045.
Author contributions
JS, JL, and YG conceived and designed the experiments; YG, FY, and TJ completed the rearing and stress experiments; LS, SS, and TJ collected the samples. YG, CJ, and FY completed the indicator measurements. YG performed the data analysis; YG wrote the paper; JS, JL, and FS guided the writing and proofread the final version of the manuscript. All authors contributed to the article and approved the submitted version.
Funding
This work was supported by the Hainan Province Natural Science Foundation of China (322QN261), the National Natural Science Foundation of China (NO.32160862), and the project of Hainan Yazhou Bay Seed Laboratory (B21HJ0105).
Acknowledgments
The authors are grateful to all of our laboratory members for their continuous technical advice and helpful discussions. Sincere thanks to Xian Zhang, Kai Xi Zhang, Jie Huang, Tian Xiu Li, De Cai Zheng, Yu Zhang, Da Zheng for collecting the samples.
Conflict of interest
The authors declare that the research was conducted in the absence of any commercial or financial relationships that could be construed as a potential conflict of interest.
Publisher’s note
All claims expressed in this article are solely those of the authors and do not necessarily represent those of their affiliated organizations, or those of the publisher, the editors and the reviewers. Any product that may be evaluated in this article, or claim that may be made by its manufacturer, is not guaranteed or endorsed by the publisher.
References
Abdel-Tawwab M., Hagras A. E., Elbaghdady H. A. M., Monier M. N. (2015). Effects of dissolved oxygen and fish size on Nile tilapia, Oreochromis niloticus (L.): growth performance, whole-body composition, and innate immunity. Aquaculture Int. 23 (5), 1261–1274. doi: 10.1007/s10499-015-9882-y
Bickler P. E., Buck L. T. (2007). Hypoxia tolerance in reptiles, amphibians, and fishes: life with variable oxygen availability. Annu. Rev. Physiol. 69 (1), 145–170. doi: 10.1146/annurev.physiol.69.031905.162529
Biddlestone J., Bandarra D., Rocha S. (2015). The role of hypoxia in inflammatory disease. Int. J. Mol. Med. 35 (4), 859–869. doi: 10.3892/ijmm.2015.2079
Cao J., Chen J., Wang J., Jia R., Xue W., Luo Y., et al. (2013). Effects of fluoride on liver apoptosis and Bcl-2, Bax protein expression in freshwater teleost, Cyprinus carpio. Chemosphere 91 (8), 1203–1212. doi: 10.1016/j.chemosphere.2013.01.037
Cao L., Huang W., Shan X. J., Ye Z. J., Dou S. Z. (2012). Tissue-specific accumulation of cadmium and its effects on antioxidative responses in Japanese flounder juveniles. Environ. Toxicol. Pharmacol. 33, 16–25. doi: 10.1016/j.etap.2011.10.003
Chen N., Wu M., Tang G. P., Wang H. J., Huang C. X., Wu X. J., et al. (2017). Effects of acute hypoxia and reoxygenation on physiological and immune responses and redox balance of Wuchang bream (Megalobrama amblycephala Yi ). Front. Physiol. 8. doi: 10.3389/fphys.2017.00375
Ching B., Chen X. L., Yong J. H., Wilson J. M., Hiong K. C., Sim E. W., et al. (2013). Increases in apoptosis, caspase activity and expression of p53 and bax, and the transition between two types of mitochondrion-rich cells, in the gills of the climbing perch, Anabas testudineus, during a progressive acclimation from freshwater to seawater. Front. Physiol. 4. doi: 10.3389/fphys.2013.00135
Dawood M. A., Noreldin A. E., Sewilam H. (2021). Long term salinity disrupts the hepatic function, intestinal health, and gills antioxidative status in Nile tilapia stressed with hypoxia. Ecotoxicol. Environ. Saf. 220, 112412. doi: 10.1016/j.ecoenv.2021.112412
Ding J., Liu C., Luo S., Zhang Y., Gao X., Wu X., et al. (2020). Transcriptome and physiology analysis identify key metabolic changes in the liver of the large yellow croaker (Larimichthys crocea) in response to acute hypoxia. Ecotoxicol. Environ. Saf. 189, 109957. doi: 10.1016/j.ecoenv.2019.109957
Ding C. Y., Shuang H. L., Yun L. I., Xue Y., Hong L. I., Hua W. R. (2018). Effects of hypoxia stress on cardiomyocyte apoptosis and the control for Bax, Bcl-2 expressions in Hypophthalmichthys molitrix. Freshw. Fisheries 48 (20), 10–15. doi: 10.13721/j.cnki.dsyy.2018.02.002
Eyckmans M., Celis N., Horemans N., Blust R., De Boeck G. (2011). Exposure to waterborne copper reveals differences in oxidative stress response in three freshwater fish species, Aquat. Toxicol. 103, 112–120. doi: 10.1016/j.aquatox.2011.02.010
Farrell A. P., Richards J. G. (2009). Defining hypoxia: an integrative synthesis of the responses of fish to hypoxia. Fish Physiol. 27, 487–503. doi: 10.1016/S1546-5098(08)00011-3
Fitzgibbon Q. P., Strawbridge A., Seymour R. S. (2007). Metabolic scope, swimming performance and the effects of hypoxia in the mulloway, Argyrosomus japonicus (Pisces: Scianeidae). Aquaculture 270, 358–368. doi: 10.1016/j.aquaculture.2007.04.038
Garbarino V. R., Orr M. E., Rodriguez K. A., Buffenstein R. (2015). Mechanisms of oxidative stress resistance in the brain: lessons learned from hypoxia tolerant extremophilic vertebrates. Arch. Biochem. Biophysics 576, 8–16. doi: 10.1016/j.abb.2015.01.029
Gorr T. A., Wichmann D., Hu J., Hermes-Lima M., Welker A. F., Terwilliger N., et al. (2010). Hypoxia tolerance in animals: biology and application. Physiol. Biochem. Zoology 83 (5), 733–752. doi: 10.1086/648581
Granger D. N., Kvietys P. R. (2015). Reperfusion injury and reactive oxygen species: The evolution of a concept. Redox Biol. 6, 524–551. doi: 10.1016/j.redox.2015.08.020
Hancz A., Koncz G., Szili D., Sármay G. (2012). TLR9-mediated signals rescue B-cells from Fas-induced apoptosis via inactivation of caspases. Immunol. Lett. 143 (1), 77–84. doi: 10.1016/j.imlet.2012.02.006
Hermes-Lima M., Moreira D. C., Rivera-Ingraham G. A., Giraud-Billoud M., Genaro-Mattos T. C., Campos É.G. (2015). Preparation for oxidative stress under hypoxia and metabolic depression: revisiting the proposal two decades later. Free Radical Biol. Med. 89, 1122–1143. doi: 10.1016/j.freeradbiomed.2015.07.156
Hermes-Lima M., Zenteno-Savın T. (2002). Animal response to drastic changes in oxygen availability and physiological oxidative stress. Comp. Biochem. Physiology Part C 133, 537–556. doi: 10.1016/s1532-0456(02)00080-7
Huang J. S., Guo Z. X., Zhang J. D., Wang W. Z., Wang Z. L., Amenyogbe E., et al. (2021). Effects of hypoxia-reoxygenation conditions on serum chemistry indicators and gill and liver tissues of cobia (Rachycentron canadum). Aquaculture Rep. 20, 100692. doi: 10.1016/j.aqrep.2021.100692
Joza N., Susin S. A., Daugas E., Stanford W. L., Cho S. K., Li C. Y., et al. (2001). Essential role of the mitochondrial apoptosis-inducing factor in programmed cell death. Nature 410 (6828), 549–554. doi: 10.1038/35069004
Kang P. M., Haunstetter A., Aoki H., Usheva A., Izumo S. (2000). Morphological and molecular characterization of adult cardiomyocyte apoptosis during hypoxia and reoxygenation. Circ. Res. 87 (2), 118–125. doi: 10.1161/01.RES.87.2.118
Kawasaki T., Kawai T. (2014). Toll-like receptor signaling pathways. Front. Immunol. 461. doi: 10.3389/fimmu.2014.00461
Kim J. E., Park H., Kim. T. H., Kang T. C. (2021). LONP1 Regulates Mitochondrial accumulations of HMGB1 and caspase-3 in CA1 and PV neurons following status epilepticus. Int. J. Mol. Sciencesm. 22 (5), 2275. doi: 10.3390/ijms22052275
Kvamme B. O., Gadan K., Finne-Fridell F., Niklasson L., Sundh H., Sundell K., et al. (2013). Modulation of innate immune responses in Atlantic salmon by chronic hypoxia-induced stress. Fish Shellfish Immunol. 34 (1), 55–65. doi: 10.1016/j.fsi.2012.10.006
Lefevre S., Stecyk J. A., Torp M. K., Løvold L. Y., Sørensen C., Johansen I. B., et al. (2017). Re-oxygenation after anoxia induces brain cell death and memory loss in the anoxia-tolerant crucian carp. J. Exp. Biol. 220 (21), 3883–3895. doi: 10.1242/jeb.165118
Leveelahti L., Rytkönen K. T., Renshaw G., Nikinmaa M. (2014). Revisiting redox-active antioxidant defenses in response to hypoxic challenge in both hypoxia-tolerant and hypoxia-sensitive fish species. Fish Physiol. Biochem. 40, 183–191. doi: 10.1007/s10695-013-9835-1
Levin L. A., Breitburg D. L. (2015). Linking coasts and seas to address ocean deoxygenation. Nat. Climate Change 5 (5), 401–403. doi: 10.1038/nclimate2595
Li D. D., Luo Z., Chen G. H., Song Y. F., Wei C. C., Pan Y. X. (2017). Identification of apoptosis-related genes bcl2 and bax from yellow catfish pelteobagrus fulvidraco and their transcriptional responses to waterborne and dietborne zinc exposure. Gene 633, 1–8. doi: 10.1016/j.gene.2017.08.029
Li M., Wang X., Qi C., Li E., Du Z., Qin J. G., et al. (2018). Metabolic response of Nile tilapia (Oreochromis niloticus) to acute and chronic hypoxia stress. Aquaculture 495, 187–195. doi: 10.1016/j.aquaculture.2018.05.031
Liu S. L., Jiang X. L., Hu X. K., Gong J., Hwang H., Mai K. (2004). Effects of temperature on non-specific immune parameters in two scallop species: Argopecten irradians (Lamarck 1819) and Chlamys farreri (Jones & Preston 1904). Aquaculture Res. 35, 678–682. doi: 10.1111/j.1365-2109.2004.01065.x
Liu X. L., Xi Q. Y., Yang L., Li H. Y., Jiang Q. Y., Shu G., et al. (2011). The effect of dietary Panax ginseng polysaccharide extract on the immune responses in white shrimp, Litopenaeus vannamei. Fish Shellfish Immunol. 30, 495–500. doi: 10.1016/j.fsi.2010.11.018
Liu Z., Yu P., Cai M., Wu D., Zhang M., Chen M., et al. (2019). Effects of microplastics on the innate immunity and intestinal microflora of juvenile Eriocheir sinensis. Sci. Total Environ. 685, 836–846. doi: 10.1016/j.scitotenv.2019.06.265
Livak J., Schmittgen T. D. (2001). Analysis of relative gene expression data using real-timequantitative PCR and the 2-ΔΔCt method. Methods 25 (4), 402–408. doi: 10.1006/meth.2001.1262
Livingstone D. R. (2003). Oxidative stress in aquatic organisms in relation to pollution and aquaculture. Rev. Médecine Vétérinaire 154 (6), 427–430.
Lushchak Volodymyr I. (2014). Free radicals, reactive oxygen species, oxidative stress and its classification. Chemico-Biological Interact 224, 164–175. doi: 10.1016/j.cbi.2014.10.016
Luzio A., Monteiro S. M., Fontaínhas-Fernandes A. A., Pinto-Carnide O., Matos M., Coimbra A. M. (2013). Copper induced up-regulation of apoptosis related genes in zebrafish (Danio rerio) gill. Aquat. Toxicol. 128, 183–189. doi: 10.1016/j.aquatox.2012.12.018
Ma S., Shu X., Wang W. X. (2022). Responses of two marine fish to organically complexed Zn: Insights from microbial community and liver transcriptomics. Sci. Total Environ. 835, 155457. doi: 10.1016/j.scitotenv.2022.155457
Magnoni L. J., Eding E., Leguen I., Prunet P., Geurden I., Ozório R. O., et al. (2018). Hypoxia, but not an electrolyte-imbalanced diet, reduces feed intake, growth and oxygen consumption in rainbow trout (Oncorhynchus mykiss). Sci. Rep. 8 (1), 1–14. doi: 10.1038/s41598-018-23352-z
Mokhtari Y., Pourbagheri-Sigaroodi A., Zafari P., Bagheri N., Ghaffari S. H., Bashash D. (2021). Toll-like receptors (TLRs): An old family of immune receptors with a new face in cancer pathogenesis. J. Cell. Mol. Med. 25 (2), 639–651. doi: 10.1111/jcmm.16214
Nam S. E., Haque M. N., Shin Y. K., Park H. S., Rhee J. S. (2020). Constant and intermittent hypoxia modulates immunity, oxidative status, and blood components of red seabream and increases its susceptibility to the acute toxicity of red tide dinoflagellate. Fish Shellfish Immunol. 105, 286–296. doi: 10.1016/j.fsi.2020.07.030
Nilsson G. E., Östlund-Nilsson S. (2008). Does size matter for hypoxia tolerance in fish? Biol. Rev. 83 (2), 173–189. doi: 10.1111/j.1469-185x.2008.00038.x
Paerl H. W. (2006). Assessing and managing nutrient-enhanced eutrophication in estuarine and coastal waters: Interactive effects of human and climatic perturbations. Ecol. Eng. 26 (1), 40–54. doi: 10.1016/j.ecoleng.2005.09.006
Pei X., Chu M., Tang P., Zhang H., Zhang X., Zheng X., et al. (2021). Effects of acute hypoxia and reoxygenation on oxygen sensors, respiratory metabolism, oxidative stress, and apoptosis in hybrid yellow catfish “Huangyou-1”. Fish Physiol. Biochem. 47 (5), 1429–1448. doi: 10.1007/S10695-021-00989-8
Pillet M., Dupont-Prinet A., Chabot D., Tremblay R., Audet C. (2016). Effects of exposure to hypoxia on metabolic pathways in northern shrimp (Pandalus borealis) and Greenland halibut (Reinhardtius hippoglossoides). J. Exp. Mar. Biol. Ecol. 483, 88–96. doi: 10.1016/j.jembe.2016.07.002
Portner H. O. (2010). Oxygen and capacity-limitation of thermal tolerance: a matrix for integrating climaterelated stressor effects in marine ecosystems. J. Of Exp. Biol. 213, 881–893. doi: 10.1242/jeb.037523
Service R. F. (2004). New dead zone off Oregon coast hints at sea change in currents. Science 305, 5687, 1099–1099. doi: 10.1126/science.305.5687.1099
Silva G., Matos L., Freitas J., Campos D., Vera Maria Fonseca de Almeida e Val (2019). Gene expression, genotoxicity, and physiological responses in an Amazonian fish, Colossoma macropomum (CUVIER 1818), exposed to roundup® and subsequent acute hypoxia. Comp. Biochem. Physiol. Part C: Toxicol. Pharmacol. 222, 49–58. doi: 10.1016/j.cbpc.2019.04.010
Song L., Luan B., Xu Q. R., Wang X. F. (2021). Effect of TLR7 gene expression mediating NF-κB signaling pathway on the pathogenesis of bronchial asthma in mice and the intervention role of IFN-γ. Eur. Rev. Med. Pharmacol. Sci. 25 (2), 866–879. doi: 10.26355/eurrev_202101_24655
Sun J. L., Jiang T., Gu Y., Song F. B., Wen X., Luo J. (2022). Differential immune and metabolic responses underlie differences in the resistance of Siganus oramin and Trachinotus blochii to Cryptocaryon irritans infection. Fish Shellfish Immunol. 120, 166–179. doi: 10.1016/j.fsi.2021.11.018
Sun J. L., Liu Y. F., Jiang T., Li Y. Q., Song F. B., Wen X., et al. (2021). Golden pompano (Trachinotus blochii) adapts to acute hypoxic stress by altering the preferred mode of energy metabolism. Aquaculture 542, 736842. doi: 10.1016/j.aquaculture.2021.736842
Sun J. L., Zhao L. L., Liao L., Tang X. H., Cui C., Liu Q., et al. (2020). Interactive effect of thermal and hypoxia on largemouth bass (Micropterus salmoides) gill and liver: Aggravation of oxidative stress, inhibition of immunity and promotion of cell apoptosis. Fish Shellfish Immunol. 98, 923–936. doi: 10.1016/j.fsi.2019.11.056
Tomanek L. (2014). Proteomics to study adaptations in marine organisms to environmental stress. Proteomics 105, 92–106. doi: 10.1016/j.jprot.2014.04.009
Tsikas D. (2017). Assessment of lipid peroxidation by measuring malondialdehyde (MDA) and relatives in biological samples: Analytical and biological challenges. Analytical Biochem. 524, 13–30. doi: 10.1016/j.ab.2016.10.021
Van Doan H., Hoseinifar S. H., Dawood M. A., Chitmanat C., Tayyamath K. (2017). Effects of Cordyceps militaris spent mushroom substrate and Lactobacillus plantarum on mucosal, serum immunology and growth performance of Nile tilapia (Oreochromis niloticus). Fish Shellfish Immunol. 70, 87–94. doi: 10.1016/j.fsi.2017.09.002
Vaquer-Sunyer R., Duarte C. M. (2008). Thresholds of hypoxia for marine biodiversity. Proc. Natl. Acad. Sci. U. S. A. 105 (40), 15452–15457. doi: 10.1073/pnas.0803833105
Varghese T., Pal A. K., Sahu N. P., Mishal P., Dasgupta S. (2017). Effects of hypoxia and dietary vitamin E on growth performance and oxidative status of Cirrhinus mrigala(Ham). Anim. Biol. 67 (2), 133–148. doi: 10.1163/15707563-00002526
Walshe T. E., D'Amore P. A. (2008). The role of hypoxia in vascular injury and repair. Annu. Rev. Pathology: Mech. Dis. 3, 615–643. doi: 10.1146/annurev.pathmechdis.3.121806.151501
Wang Q. F., Shen W. L., Hou C. C., Liu C., Wu X. F., Zhu J. Q. (2017). Physiological responses and changes in gene expression in the large yellow croaker Larimichthys crocea following exposure to hypoxia. Chemosphere 169, 418–427. doi: 10.1016/j.chemosphere.2016.11.099
Wang M., Wu F., Xie S., Zhang L. (2021). Acute hypoxia and reoxygenation: Effect on oxidative stress and hypoxia signal transduction in the juvenile yellow catfish (Pelteobagrus fulvidraco). Aquaculture 531, 735903. doi: 10.1016/j.aquaculture.2020.735903
Watts E. R., Walmsley S. R. (2019). Inflammation and hypoxia: HIF and PHD isoform selectivity. Trends Mol. Med. 25 (1), 33–46. doi: 10.1016/j.molmed.2018.10.006
Weber J. M., Choi K., Gonzalez A., Omlin T. (2016). Metabolic fuel kinetics in fish: swimming, hypoxia and muscle membranes. J. Exp. Biol. 219 (2), 250–258. doi: 10.1242/jeb.125294
Welker A. F., Campos É.G., Cardoso L. A., Hermes-Lima M. (2012). Role of catalase on the hypoxia/reoxygenation stress in the hypoxia-tolerant Nile tilapia. Am. J. Physiology-Regulatory Integr. Comp. Physiol. 302 (9), R1111–R1118. doi: 10.1152/.ajpregu.00243.2011
Wu Y., Zhou Y., Cao Z., Sun Y., Chen Y., Xiang Y., et al. (2019). Comparative analysis of the expression patterns of IL-1β, IL-11, and IL-34 in golden pompano (Trachinotus ovatus) following different pathogens challenge. Fish Shellfish Immunol. 93, 863–870. doi: 10.1016/j.fsi.2019.08.018
Xia J. H., Li H. L., Li B. J., Gu X. H., Lin H. R. (2018). Acute hypoxia stress induced abundant differential expression genes and alternative splicing events in heart of tilapia. Gene 639, 52–61. doi: 10.1016/j.gene.2017.10.002
Yan L., Wang P., Zhao C., Fan S., Lin H., Guo Y., et al. (2021). Toxic responses of liver in Lateolabrax maculatus during hypoxia and re-oxygenation. Aquat. Toxicol. 236, 105841. doi: 10.1016/j.aquatox.2021.105841
Yang X., Shi X., Wu M., Pang Y., Song X., Shi A., et al. (2021). Effects of melatonin feed on histology and antioxidant ability of the gills and oxygen consumption of Chinese mitten crab (Eriocheir sinensis), exposed to acute hypoxia stress. Aquaculture 544, 737015. doi: 10.1016/j.aquaculture.2021.737015
Yang S., Yan T., Wu H., Xiao Q., Fu H. M., Luo J., et al. (2017). Acute hypoxic stress: Effect on blood parameters, antioxidant enzymes, and expression of HIF-1alpha and GLUT-1 genes in largemouth bass (Micropterus salmoides). Fish Shellfish Immunol. 67, 449–458. doi: 10.1016/j.fsi.2017.06.035
Yu L. L., Yu H. H., Liang X. F., Li N., Wang X., Li F. H., et al. (2018). Dietary butylated hydroxytoluene improves lipid metabolism, antioxidant and anti-apoptotic response of largemouth bass (Micropterus salmoides). Fish Shellfish Immunol. 72, 220–229. doi: 10.1016/j.fsi.2017.10.054
Zhang M. Z., Li M., Wang R. X., Qian Y. (2018). Effects of acute ammonia toxicity on oxidative stress, immune response and apoptosis of juvenile yellow catfish Pelteobagrus fulvidraco and the mitigation of exogenous taurine. Fish Shellfish Immunol. 79, 313–320. doi: 10.1016/j.fsi.2018.05.036
Zhang X., Wang G., Wang T., Chen J., Feng C., Yun S., et al. (2022). Selenomethionine alleviated fluoride-induced toxicity in zebrafish (Danio rerio) embryos by restoring oxidative balance and rebuilding inflammation homeostasis. Aquat. Toxicol. 242, 106019. doi: 10.1016/j.aquatox.2021.106019
Zheng X., Fu D., Cheng J., Tang R., Chu M., Chu P., et al. (2021). Effects of hypoxic stress and recovery on oxidative stress, apoptosis, and intestinal microorganisms in Pelteobagrus vachelli. Aquaculture 543, 736945. doi: 10.1016/j.aquaculture.2021.736945
Keywords: Trachinotus blochii, hypoxia-reoxygenation, antioxidation, immunity, apoptosis
Citation: Gu Y, Sun JL, Yao FC, Jiang T, Jin CX, Shi LP, Sun SK, Song FB and Luo J (2023) Long-term hypoxia and reoxygenation induced oxidative stress lead to immunosuppression and apoptosis in golden pompano (Trachinotus blochii). Front. Mar. Sci. 10:1212571. doi: 10.3389/fmars.2023.1212571
Received: 26 April 2023; Accepted: 13 July 2023;
Published: 28 July 2023.
Edited by:
Xinxin Wang, Norwegian Institute of Food, Fisheries and Aquaculture Research (Nofima), NorwayReviewed by:
Song Yang, Sichuan Agricultural University, ChinaAmalia Pérez-Jiménez, University of Granada, Spain
Copyright © 2023 Gu, Sun, Yao, Jiang, Jin, Shi, Sun, Song and Luo. This is an open-access article distributed under the terms of the Creative Commons Attribution License (CC BY). The use, distribution or reproduction in other forums is permitted, provided the original author(s) and the copyright owner(s) are credited and that the original publication in this journal is cited, in accordance with accepted academic practice. No use, distribution or reproduction is permitted which does not comply with these terms.
*Correspondence: Jun Long Sun, MTk4OHN1bmp1bmxvbmdAc2luYS5jb20=; Jian Luo, bHVvamlhbkBoYWluYW51LmVkdS5jbg==
†These authors have contributed equally to this work