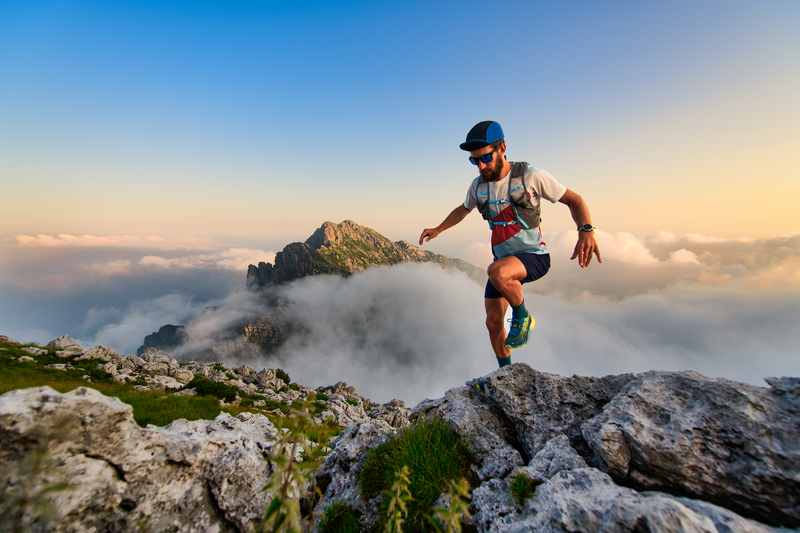
95% of researchers rate our articles as excellent or good
Learn more about the work of our research integrity team to safeguard the quality of each article we publish.
Find out more
ORIGINAL RESEARCH article
Front. Mar. Sci. , 29 June 2023
Sec. Marine Ecosystem Ecology
Volume 10 - 2023 | https://doi.org/10.3389/fmars.2023.1203809
Climate change associated sea surface temperature rise could significantly affect the physiology, and consequently geographic distribution of seagrasses. Photosynthetic and respiratory responses of warm affinity Halophila nipponica and cold affinity Zostera marina to a change in water temperature (7–26°C) were investigated to estimate whole-plant carbon balance for prediction of the future geographic distributions of these seagrasses in the northwestern Pacific region. It was hypothesized that H. nipponica and Z. marina showed distinctly different patterns of carbon balance with changes in water temperature. Photosynthetic and respiratory rates of H. nipponica and Z. marina generally increased with increasing water temperature, except for Ic and Ik of H. nipponica and α of Z. marina. H. nipponica showed high Pmax and α values, which could support a greater respiratory demand related to the higher proportion of non-photosynthetic tissues (~67%), compared with Z. marina (~34%). The whole-plant carbon balance of Z. marina remained positive throughout the experiment, although it decreased to nearly zero during the high water-temperature period. H. nipponica exhibited a negative carbon balance during winter and early spring, which corresponded with severely limited growth at water temperatures< 15°C. These results suggest that increases in water temperature, particularly during winter, in relation to continuous climate change, could induce substantial changes in the seagrass ecosystem structure and corresponding changes in coastal ecosystem services in the temperate coastal waters of the northwestern Pacific region. The results of this study will provide valuable information on the effective management and conservation of coastal and estuarine ecosystems under ongoing climate change.
Coastal and estuarine seagrass ecosystems are significantly affected by global climate change (Thomson et al., 2015; Chefaoui et al., 2018; Duarte et al., 2018). The annual sea surface temperature in the Northern Hemisphere is predicted to increase by approximately 0.05°C to 0.5°C decade–1 by the end of the 21st century (Alexander et al., 2018); this warming is amplified in shallow coastal waters (Oczkowski et al., 2015). Rising seawater temperatures induced by global climate change could—directly and indirectly—affect the physiology, behavior, and life cycles of marine organisms (Poloczanska et al., 2013; Birchenough et al., 2015). The tropicalization of temperate marine ecosystems has been pronounced worldwide; these climate-regime shifts are causing a geographical redistribution of marine organisms, such as the poleward expansion of tropical/subtropical marine biota (Vergés et al., 2014; Hyndes et al., 2016; Wernberg et al., 2016; Cavanaugh et al., 2019; Osland et al., 2021). Seagrasses are a specialized group of flowering plants adapted to the marine environment that form highly productive ecosystems, thereby offering numerous ecological, economical, and social services to shallow coastal areas (Dewsbury et al., 2016; Nordlund et al., 2018; Unsworth et al., 2019). Increased water temperature has contributed to northward range extension, phenological changes, and shifts in the species composition of seagrasses in many geographical regions (Kim et al., 2009; Virnstein and Hall, 2009; Shields et al., 2019; Gerakaris et al., 2020).
Seagrass species in the genera Zostera and Phyllospadix are mainly distributed on soft sediments and rocky substrata, respectively, from the intertidal zone to a water depth of ~25 m in the northern parts of the western Pacific Region (Lee et al., 2018; Yoshida et al., 2019; Park et al., 2020; Xu et al., 2021). Most seagrasses inhabiting the coastal waters of the northwestern Pacific are low-temperature-adapted species, with optimal growth during spring and a significant reduction in growth during the summer high water-temperature period (Kikuchi et al., 2001; Lee et al., 2005; Park and Lee, 2009). Halophila nipponica was first reported in temperate regions of the Japanese archipelago in 2006, and it was observed on the southern coast of the Korean peninsula in 2007 (Kuo et al., 2006a; Kuo et al., 2006b; Uchimura et al., 2008; Kim et al., 2009). Several H. nipponica meadows are currently present on the southern coast of Korea and in the Japanese archipelago (Kuo et al., 2006a; Kuo et al., 2006b; Lee et al., 2018). H. nipponica exhibits a distinctly different growth pattern from the pattern of temperate seagrasses, such as Zostera spp. and Phyllospadix spp., in this region (Lee et al., 2005; Park and Lee, 2009: Kim et al., 2012). The optimal growth temperature for H. nipponica is approximately 25°C, and there is no growth inhibition during the high water-temperature conditions in summer. Productivity is severely restricted at water temperatures< 15°C (Kim et al., 2012). The growth pattern of H. nipponica indicates that this species possesses the tropical/subtropical growth characteristics of the genus Halophila (Kim et al., 2012). The persistence of warm affinity H. nipponica in the northern part of the western Pacific Region is presumably permitted by the warm Kuroshio Current in this region (Uchimura et al., 2008; Kim et al., 2009); it may also be related to the continuous climate change-induced increase in water temperature.
Carbon balance, which is determined on the basis of carbon assimilation by photosynthesis and carbon consumption via respiration, is a potential indicator of a plant’s response to environmental change (Collier et al., 2011; Marín-Guirao et al., 2013; Moreno-Marín et al., 2018). The photosynthesis–irradiance (P–I) relationship is required to assess the carbon balance. When photosynthesis exceeds the carbon demands from respiration and growth, seagrass maintains a positive carbon balance, whereas plants will exhibit a negative carbon balance when the carbon demands cannot be met through photosynthesis (Lee et al., 2007). Because of structural characteristics, the carbon balance of seagrasses is more complex than the carbon balance of phytoplankton or algae. A large proportion of seagrass biomass is allocated to below-ground tissues, which are supported by leaf photosynthesis, indicating that seagrasses have a high light requirement (Lee et al., 2007; Tanaka and Nakaoka, 2007). Because below-ground tissues can significantly affect the carbon balance of the entire seagrass plant, the contribution of below-ground tissues should be considered when estimating the carbon balance of seagrasses.
Photosynthetic processes of seagrasses, which are affected by various environmental factors, exhibit considerable seasonal variations (Beca-Carretero et al., 2018; Moreno-Marín et al., 2018). The metabolic rates of seagrasses are particularly sensitive to variations in water temperature; they typically increase with increasing water temperature (Lee et al., 2007). Seagrass usually requires more light under high water-temperature conditions to maintain a positive carbon balance, suggesting that reduced light availability is more harmful to seagrass growth during the high water-temperature period in summer (Staehr and Borum, 2011; Said et al., 2021). The parameters of the P–I relationship also vary among and within species (Tanaka and Nakaoka, 2007; Collier et al., 2011; Burkholz et al., 2019). Thus, seasonal photosynthetic and respiratory responses to various irradiance and temperature conditions should be examined to assess the whole-plant carbon balance of each seagrass species; such information eventually could be used to predict the fate of each seagrass species in the context of global climate change.
In this study, we examined the photosynthetic and respiratory responses of two seagrasses, cold affinity Z. marina and warm affinity H. nipponica, to various water temperature conditions; we aimed to predict the fates of these species in the context of continuous increases in seawater temperature conditions in the northwestern Pacific Ocean. We hypothesized that, compared with the cold affinity Z. marina, the warm affinity H. nipponica would exhibit a more positive carbon balance in higher water-temperature conditions. The whole-plant carbon balance on areal basis can be estimated by considering seasonal photosynthetic production in relation to irradiance and temperature, respiration, and tissue biomass. Therefore, we measured seasonal P–I curves, dark respiration rates of above- and below-ground tissues, biomass, as well as in situ water temperature and underwater irradiance. This study will provide valuable information for prediction of the future geographic distributions of these seagrass species, as well as effective management and conservation of coastal ecosystems in the northwestern Pacific region in the context of continuous global climate change.
Continuous measurements of in situ underwater photon flux density (PFD) and water temperature were conducted from July 2010 to August 2011 at the study site on Namhae Island, near the southern coast of Korea. Underwater PFD was monitored at 15-min intervals in a mixed seagrass meadow using the Odyssey photosynthetic irradiance recording system (Dataflow Systems, Christchurch, New Zealand). The light sensor was regularly cleaned at 4-week intervals to minimize fouling by epiphytes and sediment. Before deployment at the study site, the Odyssey sensor was calibrated by the collection of concurrent quantum measurements for 1 week using the LI-1400 data logger and LI-193SA spherical quantum sensor (Li-Cor, Lincoln, NE, USA). Daily PFD (mol photons m−2 day−1) was calculated as the sum of quantum flux across a 24-h period. Water temperature was monitored every 15 min using a HOBO StowAway Tidbit temperature data logger (Onset Computer Corp., Bourne, MA, USA), and measured values were averaged daily. The PFD and water temperature loggers were deployed at the canopy level of Z. marina, approximately 20 cm above the sediment.
H. nipponica and Z. marina shoots were collected seasonally at the study site on Namhae Island (34°43′40″N, 128°02′07″E). H. nipponica was distributed in slightly deeper water depths of ~2–7 m relative to the mean lower low water, whereas Z. marina occurred at water depths of ~1–4 m relative to the mean lower low water (Kim et al., 2020b). Shoots of both seagrass species were collected at water depths of ~2.5–3 m in the mixed seagrass meadow. Whole seagrass materials including leaves, rhizomes, and roots were carefully collected by hand in early April, June, and late August (in situ water temperatures of ~9, 16, and 24°C, respectively), then transported to the laboratory in a cool box filled with seawater and ice to maintain the ambient seawater temperature during the sampling times. The shoots were thoroughly cleaned of dead tissues, epiphytes, and sediment using filtered seawater. Seawater for incubation experiments was collected from the study site in early April, June, and late August and filtered through a glass microfiber filter (47 mm GF/C™, Whatman,Piscataway, NJ, USA). The collected seawater conditions, including nutrient concentrations and salinity, were presented in Supplementary Table 1. Before incubation experiments, the seagrasses were progressively acclimated to the target temperature by ~1°C per day during sampling times (7 and 10°C in April, 14 and 18°C in June, and 22 and 26°C in August) under saturation irradiance (~450 μmol photons m−2 s−1; Lee et al., 2007) from a 14:10 light:dark cycle for 1 or 2 days. The target temperatures for the experiment fell within the range of seasonal water temperature variations at the study site, which ranged from 7.5 to 25.9°C (Kim et al., 2020b). We attempted to conduct all incubation experiments at each target temperature when the field temperature conditions closely matched the respective target temperatures. All incubation experiments were performed within 3 days of seagrass specimen collection.
Incubation experiments (n = 4) were conducted in a temperature-controlled growth room at the following temperatures: 7 and 10°C in April, 14 and 18°C in June, and 22 and 26°C in August. Photosynthetic O2 evolution was measured using a 2-mm oxygen dipping probe with coated sensor foil connected to a four-channel fiber optic oxygen transmitter (OXY-4 mini; PreSens Precision Sensing GmbH, Regensburg, Germany). Before taking measurements, each O2 probe was calibrated using a conventional two-point calibration method, following the instruction manual of OXY-4 mini (www.presens.de). The calibration was performed using oxygen-free water (0% air saturation) and air-saturated water (100% air saturation). The seawater in the incubation chambers was purged with N2 gas to reduce the initial O2 concentration to approximately 20% of saturation, and maintained O2 concentrations within the range of approximately 20% to 80% saturation throughout the experiment. For each species, one or two intact H. nipponica or Z. marina plants were placed inside a transparent cylindrical chamber made of Plexiglass. The chambers had a diameter of 6.5 cm and a height of 10 cm for H. nipponica, and a height of 40 cm for Z. marina (n = 4 for each species). To prevent diffusion limitation, the seawater in all incubation chambers was continuously stirred using a magnetic stirrer. A photosynthesis vs. irradiance curve (P–I curve) was obtained under nine light level steps (0 to 875 μmol photons m−2 s−1) with a light-emitting diode lamp and reflector; each step lasted 10–20 min after the slope of the dissolved oxygen exhibited linear stabilization. The net chamber photosynthetic rate was determined from the slope by linear regression analysis of the oxygen value recorded at each light level. The gross photosynthetic rate (PG) at each light level was calculated using the net chamber photosynthetic rate (PN) and the chamber respiration rate (R; 0 μmol photons m−2 s−1 in light level), then normalized to leaf biomass (μmol O2 g−1 DW min−1):
P–I curves were fitted to the data using the hyperbolic tangent function of Jassby and Platt (1976):
where Pmax is the maximum gross photosynthetic rate, α is the light-limited slope of the P–I curve, and I is irradiance. Saturation and compensation irradiances were determined as Pmax/α and –R/α, respectively (Herzka and Dunton, 1997). The daily photosynthetic production (P) was calculated by using the daily average irradiance for each day and the parameters obtained from the P–I curve corresponding to each temperature treatment (7, 10, 14, 18, 22, and 26°C). These parameters were applied to the respective day based on the range of in situ water temperature, which was categorized as follows: ≤ 8°C, 8–12°C, 12–16°C, 16–20°C, 20–24°C, ≥ 24°C, respectively (Supplementary Table 2). Then the daily photosynthetic production was integrated (PG(integrated)).
The whole-plant carbon balance was defined as the net photosynthetic production on an areal basis (PN-areal). Whole-plant carbon balance was estimated using seagrass biomass, dark respiration, and daily integrated photosynthetic production. All seagrass materials, including above- and below-ground tissues, inside a haphazardly thrown quadrat (0.2 × 0.2 m; n = 4) were collected to measure biomass from July 2010 to June 2011. The seagrass samples were rinsed in tap water to remove epiphytes and sediment, separated into above- and below-ground tissues (leaves + sheath and rhizome + roots, respectively), and dried at 60°C to constant weight. The samples were weighed and converted to per unit area estimates (g dry weight m−2).
To measure the dark respiration rate of each seagrass tissue (n = 4), above- and below-ground tissues were incubated in cylindrical chambers for 1 h under dark conditions at the projected temperatures (7, 10, 14, 18, 22, and 26°C). After incubation, the seagrass tissues were dried at 60°C to constant weight. Dark respiration rates were normalized to the dry weight of each seagrass tissue and are expressed as μmol O2 g−1 DW min−1.
To estimate gross and net photosynthetic production on an areal basis, daily integrated photosynthetic production (PG(integrated)) and dark respiration (RD) of the above- and below-ground tissues (Ra and Rb, respectively) were multiplied by areal seagrass biomass (Herzka and Dunton, 1998). The RD values obtained for each temperature treatment (7, 10, 14, 18, 22, and 26°C) were applied to the corresponding day, depending on the range of in situ water temperature, which were categorized as follows: ≤ 8°C, 8–12°C, 12–16°C, 16–20°C, 20–24°C, ≥ 24°C, respectively (Supplementary Table 2):
where PG-areal and PN-areal are gross and net photosynthetic production (mg C m−2 day−1) on an areal basis, respectively; RD-area and B are dark respiration (Ra and Rb; mg C m−2 min−1) and areal biomass (Ba and Bb; g DW m−2) of the above- and below-ground tissues, respectively. Photosynthetic and respiratory quotients of unity were assumed to be 1 mol O2 = 1 mol C (Herzka and Dunton, 1998).
All values are presented as means ± standard errors, and statistical analyses were conducted using SPSS 23.0 software (SPSS Inc., Chicago, IL, USA). Significant differences among water temperatures in terms of photosynthetic parameters (e.g., Pmax, α, Ic, and Ik) and the dark respiration rates of above- and below-ground tissues were determined by one-way analysis of variance. One-way analysis of variance was also performed to detect differences in PFD and water temperature among the sampling months. The data were subjected to evaluations of normality and homogeneity of variance to determine whether they met the assumptions of parametric statistics. If necessary, the data were log- or square-root-transformed. p-values< 0.05 were considered statistically significant. Means were analyzed by the Student-Newman-Keuls test to determine where differences were present.
Daily average PFD exhibited considerable fluctuation, and the monthly average PFD exhibited a significant (p< 0.001) difference according to sampling time (Figure 1A; Table 1). The monthly average PFD tended to increase during spring and summer, then decrease during fall and winter; it ranged from 3.9 mol photons m−2 day−1 in February 2011 to 19.3 mol photons m−2 day−1 in August 2010. Water temperature varied in a seasonal manner, with the highest temperature (25.9°C) in September 2010 and lowest temperature (7.5°C) in February 2011 (Figure 1B). The annual mean water temperature at the study site during the experimental period was 15.8°C.
Figure 1 Underwater photon flux density (A) and water temperature (B) at the study site on the southern coast of Korea from July 2010 to June 2011.
Table 1 Results of one-way ANOVA testing the significant difference in underwater photon flux density (PFD) and water temperature among sampling months (M) and the effects of water temperatures (T) on maximum gross photosynthetic rate (Pmax), photosynthetic efficiency (α), compensation irradiance (Ic), and saturation irradiance (Ik), and dark respiration (RD) of Halophila nipponica and Zostera marina.
The P–I curves for whole plants of H. nipponica and Z. marina varied according to water temperature (Figure 2). P–I curves for both seagrass species showed a general pattern of rapid increases in gross photosynthesis with increasing light intensity at low irradiance; maximum gross photosynthesis was reached at high irradiance. There was no evidence of photoinhibition at high irradiance (Figure 2).
Figure 2 Gross photosynthesis–irradiance curves for Halophila nipponica (A) and Zostera marina (B) at the various water temperature treatments. The temperature treatments of 7 and 10°C, 14 and 18°C, and 22 and 26°C were implemented in early April, June, and late August, respectively. Values are means ± SE (n = 4).
The maximum gross photosynthetic rates (Pmax) of both seagrass species significantly (p< 0.001) differed among water temperature treatments and tended to increase with increasing water temperature (Figure 3A; Table 1). The Pmax value of H. nipponica ranged from 6.5 μmol O2 g−1 DW min−1 at 7°C to 18.5 μmol O2 g−1 DW min−1 at 26°C; the Pmax value of Z. marina ranged from 2.2 μmol O2 g−1 DW min−1 at 10°C to 6.6 μmol O2 g−1 DW min−1 at 26°C. The photosynthetic efficiency (α; μmol O2 g−1 DW min−1/μmol photons m−2 s−1) of H. nipponica significantly (p< 0.001) differed among temperatures, ranging from 0.0233 at 10°C to 0.0610 at 26°C; however, the photosynthetic efficiency of Z. marina did not significantly (p = 0.405) differ among water temperature treatments (Figure 3B; Table 1). The compensation and saturation irradiance (Ic and Ik, respectively) for H. nipponica did not significantly (p = 0.372 and p = 0.305, respectively) differ according to water temperature, whereas Ic and Ik for Z. marina exhibited significant (p< 0.001 and p< 0.01, respectively) differences among water temperature treatments (Figures 3C, D; Table 1).
Figure 3 Maximum gross photosynthetic rate (Pmax; (A)), photosynthetic efficiency (α; (B)), compensation irradiance (Ic; (C)), and saturation irradiance (Ik: (D)) for Halophila nipponica and Zostera marina at the various water temperature treatments. Different letters on the bars indicate significant (p< 0.05) differences. The temperature treatments of 7 and 10°C, 14 and 18°C, and 22 and 26°C were implemented in early April, June, and late August, respectively. Values are means ± SE (n = 4).
Significant (all p< 0.001) differences among water temperature treatments were observed for the dark respiration rates of the above- and below-ground tissues of H. nipponica and Z. marina (Figure 4; Table 1). Dark respiration (μmol O2 g−1 DW min−1) of the H. nipponica above-ground tissues ranged from −0.84 at 7°C to −3.13 at 26°C, whereas dark respiration of Z. marina ranged from −0.32 at 10°C to −1.11 at 26°C (Figure 4). The dark respiration rates of below-ground tissues of H. nipponica and Z. marina were also highest (−2.36 and −0.9 μmol O2 g−1 DW min−1, respectively) at 26°C, whereas they were lowest at 10°C and 7°C (−0.55 and −0.16 μmol O2 g−1 DW min−1, respectively). The dark respiration rates of above- and below-ground tissues for both species tended to increase more rapidly at higher temperatures (18–26°C) than at lower temperatures (7–14°C). Compared with Z. marina, the above- and below-ground tissues of H. nipponica required more respiratory carbon demands during water temperature treatments (Figure 4).
Figure 4 Dark respiration rates of above- and below-ground tissues for Halophila nipponica (A) and Zoster marina (B) at the various water temperature treatments. Different letters on the bars indicate significant (p< 0.05) differences. The temperature treatments of 7 and 10°C, 14 and 18°C, and 22 and 26°C were implemented in early April, June, and late August, respectively. Values are means ± SE (n = 4).
Although total, above-, and below-ground biomasses of H. nipponica and Z. marina exhibited distinct seasonal variations, both species had slightly different seasonal patterns (Figures 5A–C). Total and above-ground biomasses of H. nipponica were highest during summer (July–August 2010) and lowest during early spring (April); below-ground biomass increased during fall and decreased during winter and spring (Figures 5A–C). Total, above-, and below-ground biomasses of Z. marina were highest during early summer (June 2011) and lowest during fall and winter (Figures 5A–C). The proportion of H. nipponica below-ground to total biomass ranged from 47.3% on July 2010 to 86.5% on March 2011, whereas the corresponding proportion for Z. marina ranged from 23.1% on June 2011 to 53.1% on February 2011 (Figure 5D). Below-ground H. nipponica and Z. marina biomasses constituted 67.0% and 34.0% of the total corresponding biomasses, on average, during the experimental period.
Figure 5 Total (A), above- (B) and below-ground (C) biomass and proportion of below-ground to total biomass (D) for Halophila nipponica and Zostera marina at the study site on the southern coast of Korea from July 2010 to June 2011. Values are means ± SE (n = 4).
The daily H. nipponica and Z. marina carbon balance at the study site fluctuated more in spring and summer than in winter (Figure 6). The carbon balance of both species tended to increase when the PFD increased during summer. The monthly average carbon balance in H. nipponica was positive in July, August, and October 2010, as well as June 2011; it was negative during the other months (Figure 6A; Table 2). The monthly Z. marina carbon balance was positive throughout the experimental period; it was highest (100.0 mg C m−2 day−1) during July 2010 and lowest (0.5 mg C m−2 day−1) during February 2011 (Figure 6B; Table 2).
Figure 6 Daily and monthly average carbon balance for Halophila nipponica (A) and Zostera marina (B) at the study site on the southern coast of Korea from Jul 2010 to June 2011.
Table 2 The carbon (C) balance of Zostera marina and species in genus Halophila reported under various conditions.
Contrary to expectations, the two seagrass species, warm affinity H. nipponica and cold affinity Z. marina, did not show distinctly contrasting photosynthetic and respiratory responses to increasing water temperature in the present study. Photosynthesis generally increases with increasing temperature up to a specific temperature, then decreases at higher temperatures (Pedersen et al., 2016; Collier et al., 2017; Beca-Carretero et al., 2018). The high water temperatures that impact seagrass photosynthesis are distinct in temperate and tropical seagrass species. Halodule uninervis and Zostera muelleri, which are generally regarded as tropical and temperate species on the Great Barrier Reef, respectively, have contrasting responses to increased water temperature (Collier et al., 2011). The photosynthetic rates and net carbon production of H. uninervis increase with water temperature up to 33°C; in Z. muelleri, those parameters rapidly decrease at 33°C. This result suggests that, compared with the tropical species H. uninervis, the temperate seagrass species Z. muelleri is more susceptible to increasing water temperature (Collier et al., 2011). In the shallow brackish water of Denmark, the maximum photosynthetic rates of Z. marina consistently increase from 5°C to 25°C and then decrease at 30°C and 35°C (Staehr and Borum, 2011). The photosynthetic rate of Z. marina on the west coast of Sweden consistently increases with increasing water temperature up to ~29°C; the Q10 values for the photosynthetic rate are ~4-fold higher at 10–20°C and 20–30°C than at 30–35°C, suggesting a decline in photosynthetic activity above 30°C (Rasmusson et al., 2019). The maximum net photosynthetic rate of Z. marina in Denmark also continuously increases up to 27°C (Hansen et al., 2022). Thus, optimal temperatures for photosynthesis in these Z. marina populations, which were distributed in higher latitudinal regions (55–58°N) than our study site, range from 25°C to 29°C. The threshold temperature for high-temperature stress in Z. marina occurred near its southern distribution limit (~37°N), located at similar latitude to our study site, was suggested to range from 26°C to 30°C (Hammer et al., 2018). Considering these results, the optimal temperature for Z. marina photosynthesis would be 26–30°C, regardless of the latitudinal location of the population. The highest water temperature treatment of 26°C in the present study may be within the optimal temperature range for Z. marina photosynthesis; thus, we did not observe any decline in photosynthetic activity at the highest temperature treatment used in the present study. However, Z. marina biomass significantly decreased during the highest water-temperature (25–26°C) period in September at the present study site, although photosynthetic activity did not decrease under the highest water-temperature condition.
The optimal temperature for Z. marina growth in Korean coastal waters is between 15°C and 20°C; large decreases in growth are observed at higher water temperatures (Lee et al., 2005). However, our results indicate that the optimal temperature for Z. marina photosynthesis differs from the optimal temperature for the growth of this species. The optimal temperatures for photosynthesis in seagrasses are generally higher than optimal temperatures for the growth (Lee et al., 2007; Georgiou et al., 2016; Collier et al., 2017). This discrepancy may be related to the greater number of factors that affect plant growth, compared with photosynthesis (Lee et al., 2007; Said et al., 2021). Importantly, plant growth is the result of a combination of multiple factors, including nutrient availability and uptake, respiration, leaf senescence, and photosynthesis (Lee et al., 2007; George et al., 2018; Hammer et al., 2018; Moreno-Marín et al., 2018). Additionally, an alternative explanation could be the impact of temperature on biogeochemical processes occurring in the sediment (Sanz-Lázaro et al., 2011). Elevated temperatures can lead to an increased oxygen demand from seagrasses and microorganisms present in the sediment surrounding the below-ground tissues. This increased oxygen demand makes the plants more susceptible to in situ conditions characterized by anoxia and the presence of toxic sulfide (Holmer and Hasler-Sheetal, 2014; Hammer et al., 2018). Thus, Z. marina may have different optimal temperatures for growth and photosynthesis.
Underwater irradiance usually decreases during summer when Z. marina undergoes thermal stress in the Z. marina meadows on the southern coast of Korea (Park et al., 2009; Kim et al., 2014; Suonan et al., 2022). The decline in underwater irradiance at the level of the seagrass canopy is attributed to an augmentation in particulate organic matter, primarily originating from phytoplankton. This increase in particulate organic matter is linked to high temperatures, surface irradiance, and precipitation during the summer season (Ahn et al., 2006; Lee et al., 2017; Kim et al., 2019). Thus, Z. marina populations in this region experience high mortality because of high water temperatures and severe light restrictions during summer (Lee et al., 2005; Kim et al., 2014; Suonan et al., 2022). In the present study, the water temperature reached its highest point in September 2010, while underwater irradiance significantly decreased during the same period. This coincided with a dramatic decline in Z. marina biomass. Thus, the decline in biomass was likely caused by the combined effects of high water temperature and reduced light conditions at that time, rather than solely due to high water-temperature stress, because seagrasses require higher levels of irradiance under elevated water-temperature conditions (Lee et al., 2007; Ralph et al., 2007). This decline in Z. marina growth during summer may have contributed to the difference in optimal temperatures between photosynthesis and growth observed in this study.
Intra- and interspecies differences in photosynthetic parameters have been reported (Lee et al., 2007; Collier et al., 2017; Beca-Carretero et al., 2018). Higher α and lower Ik, which can result in comparatively greater net photosynthesis, were identified in two Amphibolis species in Western Australia, compared with two Posidonia species in that region, thereby contributing to higher maximum in situ specific growth rates in the Amphibolis species (Masini and Manning, 1997). In the present study, the Pmax and α values for H. nipponica were much higher than those values for Z. marina. Seagrass species in the genus Halophila are distributed over a wide range from tropical to temperate waters and have the greatest depth limit of > 85 m; these characteristics indicate that such species are extremely eurybiotic (den Hartog and Kuo, 2006; Short et al., 2007). Furthermore, Halophila species are opportunistic because of their rapid growth rate among seagrass species, as well as their ability to inhabit a wide array of environmental conditions. The proportion of below-ground to total biomass was much higher in H. nipponica (~67%) than in Z. marina (~34%) throughout the experimental period. Because of the higher biomass allocation to below-ground biomass relative to above-ground biomass, H. nipponica may have a greater respiratory demand, compared with Z. marina. The simultaneously higher Pmax and α in H. nipponica than in Z. marina may constitute an acclimation mechanism to the higher respiratory demand in H. nipponica. Additionally, Halophila species are petiolate plants with elliptical or ovate blades along rhizomes or on distal nodes of an erect stem, which are more efficient at harvesting light compared with the strap-shaped or lanceolate leaves of seagrasses, such as Z. marina. These metabolic and morphological adaptations of H. nipponica could lead to similar Ic and Ik between the two seagrass species, although the respiration rate was higher in H. nipponica than in Z. marina.
In the present study, the H. nipponica and Z. marina photosynthetic parameters generally increased with increasing water temperature. These results are consistent with previous findings (Herzka and Dunton, 1997; Staehr and Borum, 2011; Olivé et al., 2013; Georgiou et al., 2016). Low Pmax, α, Ic, and Ik in T. testudinum were observed under low water-temperature conditions during winter; those photosynthetic parameters increased with increasing water temperature during the early spring and summer (Herzka and Dunton, 1997). Higher Pmax and R for Z. marina and Cymodocea nodosa during spring and summer, along with higher Pmax, Ic, and Ik for Halophila stipulacea at higher water temperatures, have also been reported (Staehr and Borum, 2011; Olivé et al., 2013; Georgiou et al., 2016). However, Ic and Ik of H. nipponica and α of Z. marina did not significantly differ among temperature treatments. The increases in Ic and Ik and a generally constant α of Heterozostera tasmanica with increasing water temperature are mainly affected by changes in Pmax and dark respiration in response to increasing water temperature (Bulthuis, 1987). The α value for H. johnsonii in Florida is higher at higher temperatures (Torquemada et al., 2005), but the α value for H. stipulacea is higher at lower temperatures; this difference implies photo-acclimation to maximize carbon fixation under various environmental conditions (Georgiou et al., 2016). Overall, the results indicated that photosynthetic parameters were mainly controlled by changes in water temperature but did not always show a clear seasonal trend, suggesting acclimation to local environmental conditions.
Although many studies have been conducted regarding P–I relationships and respiration in seagrasses, most studies have simply estimated photosynthesis and respiration under various environmental conditions (Tanaka and Nakaoka, 2007; Staehr and Borum, 2011; Georgiou et al., 2016; Beca-Carretero et al., 2018; Said et al., 2021). Few studies have calculated the seasonal carbon balance of seagrass using the photosynthetic and respiration rates, biomass, and in situ underwater PFD (Table 2; Alcoverro et al., 2001). In the present study, the seasonal carbon balance, which reflects a plant’s responses to environmental fluctuations throughout the year, was estimated via seasonal variations in photosynthesis, respiration, biomass, and underwater PFD.
Importantly, we found that the Z. marina carbon balance varied in a seasonal manner. It increased during the spring and early summer, then decreased during the fall and winter; these trends were related to seasonal variations in the P–I relationship. Although Z. marina did not have a monthly negative carbon balance throughout the experiment, the monthly average daily carbon balance decreased to nearly zero during the highest water-temperature period in September 2010. The growth of Z. marina in Korean coastal waters is usually greatest at water temperatures of 15–20°C during spring, then dramatically decreases during the high water-temperature period in summer (Lee et al., 2005; Park et al., 2009; Qin et al., 2020b; Suonan et al., 2022). Z. marina photosynthesis continuously increases up to 26°C, but biomass significantly decreases when the water temperature is higher than 15–20°C in this region (Lee et al., 2005); these observations indicate a stronger effect of heat stress on biomass than on photosynthesis (George et al., 2018). Compared with changes in the physiological characteristics of Z. marina, reductions in shoot density and biomass because of high water-temperature conditions (25–30°C) are more evident in culture experiments (Nejrup and Pedersen, 2008; Kim et al., 2020a). Under high water-temperature conditions, seagrass requires more light to maintain a positive carbon balance; under reduced light conditions, seagrass is more sensitive to high water-temperature stress (Lee et al., 2007; Ralph et al., 2007). Thus, the significant light reduction during September 2010 may have caused a rapid decline in the carbon balance and, consequently, biomass of Z. marina at the study site, indicating that the effects of reduced light are more harmful in summer than in winter.
However, H. nipponica had a negative carbon balance for several months in the present study. The seasonal carbon balance pattern for H. nipponica was consistent with the in situ growth pattern of this species at the study site on the southern coast of Korea. The maximum growth of H. nipponica was observed during the highest water-temperature period in this region; no reduction in the growth of this species has been observed under high summer water-temperature conditions (Kim et al., 2012). The productivity of H. nipponica is severely restricted at water temperatures< 15°C, and the winter minimum productivity lasts until May when water temperature increases to ~15°C (Kim et al., 2012). In the present study, the proportion of H. nipponica below-ground to total biomass rapidly increased by 86.5% during the low water-temperature periods in winter and early spring, leading to high respiratory demands in non-photosynthetic tissues. The high respiratory demands and lower photosynthetic performance during the low water-temperature periods may have contributed to the negative carbon balance in H. nipponica during these times. Although H. nipponica is widely distributed in temperate waters of Korea and Japan, this species is presumably less tolerant of low water temperatures, indicating that it is warm-adapted (Kim et al., 2012; Kim et al., 2020b). H. nipponica also had a negative carbon balance in September 2010, which may have been related to the low underwater PFD during this period. Because seagrass requires more light for a positive carbon balance under high water-temperature conditions, in situ underwater irradiance of< 10 mol photons m–2 day–1 during this time may have been insufficient to maintain a positive carbon balance. These results suggest that Z. marina is adapted/acclimated to the local temperature regime in Korean coastal waters, whereas H. nipponica growth is limited during low water-temperature periods in winter and early spring.
Our results indicate that the carbon balances of H. nipponica and Z. marina were not entirely consistent with their growth patterns in this study or previous studies (Lee et al., 2005; Kim et al., 2012). We could not find clear evidence regarding potential changes in seagrass ecosystem structure related to a continuous increase in water temperature in our study region. Cymodocea rotundata is distributed in a shallower zone than Cymodocea serrulata in the Indo-Pacific tropical region of southwestern Japan, although the Ic, Ik, and respiration rate are lower in C. rotundata than in C. serrulata (Tanaka and Nakaoka, 2007). The photosynthesis and respiration trends of C. rotundata and C. serrulata do not fully explain their depth distributions, suggesting that other factors influence the depth ranges of these two species (Tanaka and Nakaoka, 2007). The effects of temperature on photosynthesis in Halophila ovalis, a widely distributed species across both tropical and temperate regions, were inconsistent at similar geographical sites, suggesting that seagrass was acclimated to local environmental factors controlling photosynthesis (e.g., light, nutrients, depth, and fine spatial-scale differences in temperature) (Said et al., 2021). Thus, in situ growth patterns of seagrasses can be explained by a combination of numerous metabolic and environmental factors, rather than by photosynthesis and respiration rates alone.
Reductions in Z. marina growth and reproduction related to long-term increases in sea surface temperature, as well as seasonal temperature anomalies (e.g., marine heatwaves), have been reported in the study region in the northwestern Pacific Ocean (Kim et al., 2020a; Qin et al., 2020a; Qin et al., 2020b). These decreases in Z. marina growth during high water-temperature periods under in situ conditions were not fully explained by increased water temperature-induced changes in the patterns of photosynthesis and respiration. Our results showed that Z. marina photosynthetic and respiration rates significantly increased under the highest water-temperature treatments; consequently, Z. marina maintained a positive carbon balance at the highest water temperature. Because H. nipponica exhibited a negative carbon balance during low water-temperature periods in winter and spring, the severely restricted growth of H. nipponica during low water-temperature periods was explained by seasonal changes in photosynthesis and respiration. Thus, increases in water temperature, particularly during winter, related to continuous climate change will be advantageous for H. nipponica growth in this region. In a previous study, small fast-growing H. nipponica rapidly recolonized, in contrast to the large slower-growing Z. marina in disturbed areas (Kim et al., 2020b). The results of the present and previous studies suggest that the expansion of H. nipponica meadows is more rapid than the expansion of Z. marina under climate change-related increases in water temperature in the temperate coastal waters of the northwestern Pacific; such differences may lead to changes in coastal seagrass ecosystem structure such as primary production and blue carbon sequestration and corresponding changes in coastal ecosystem services in this region.
The raw data supporting the conclusions of this article will be made available by the authors, without undue reservation.
SK and K-SL conceptualized the experiments. SK and HK performed the experiments. SK, ZS, FZ, and K-SL analyzed the results. All authors contributed to the article and approved the submitted version.
This research was supported by the National Research Foundation of Korea (NRF) grant funded by the Ministry of Education (NRF-2019R1A2C1090641 and NRF-2021R1I1A1A01049507) and “Responses of species-populations to climate change scenario” program of Korea institute of Marine Science & Technology Promotion (KIMST) funded by the Ministry of Oceans and Fisheries (KIMST-20220559).
We thank YK Kim, JW Ku, KY Kim for their many hours of field and laboratory assistance.
The authors declare that the research was conducted in the absence of any commercial or financial relationships that could be construed as a potential conflict of interest.
All claims expressed in this article are solely those of the authors and do not necessarily represent those of their affiliated organizations, or those of the publisher, the editors and the reviewers. Any product that may be evaluated in this article, or claim that may be made by its manufacturer, is not guaranteed or endorsed by the publisher.
The Supplementary Material for this article can be found online at: https://www.frontiersin.org/articles/10.3389/fmars.2023.1203809/full#supplementary-material
Ahn Y.-H., Shanmugam P., Ryu J.-H., Jeong J.-C. (2006). Satellite detection of harmful algal bloom occurrences in Korean waters. Harmful Algae 5 (2), 213–231. doi: 10.1016/j.hal.2005.07.007
Alcoverro T., Manzanera M., Romero J. (2001). Annual metabolic carbon balance of the seagrass Posidonia oceanica: the importance of carbohydrate reserves. Mar. Ecol. Prog. Ser. 211, 105–116. doi: 10.3354/meps211105
Alcoverro T., Zimmerman R. C., Kohrs D. G., Alberte R. S. (1999). Resource allocation and sucrose mobilization in light-limited eelgrass Zostera marina. Mar. Ecol. Prog. Ser. 187, 121–131. doi: 10.3354/meps187121
Alexander M. A., Scott J. D., Friedland K. D., Mills K. E., Nye J. A., Pershing A. J., et al. (2018). Projected sea surface temperatures over the 21st century: changes in the mean, variability and extremes for large marine ecosystem regions of northern oceans. Elementa-Sci. Anthrop. 6, 9. doi: 10.1525/elementa.191
Beca-Carretero P., Olesen B., Marbà N., Krause-Jensen D. (2018). Response to experimental warming in northern eelgrass populations: comparison across a range of temperature adaptations. Mar. Ecol. Prog. Ser. 589, 59–72. doi: 10.3354/meps12439
Birchenough S. N. R., Reiss H., Degraer S., Mieszkowska N., Borja Á., Buhl-Mortensen L., et al. (2015). Climate change and marine benthos: a review of existing research and future directions in the north Atlantic. Wiley Interdiscip. Rev.-Clim. Change 6 (2), 203–223. doi: 10.1002/wcc.330
Bulthuis D. A. (1987). Effects of temperature on photosynthesis and growth of seagrasses. Aquat. Bot. 27 (1), 27–40. doi: 10.1016/0304-3770(87)90084-2
Burkholz C., Duarte C. M., Garcias-Bonet N. (2019). Thermal dependence of seagrass ecosystem metabolism in the red Sea. Mar. Ecol. Prog. Ser. 614, 79–90. doi: 10.3354/meps12912
Cavanaugh K. C., Dangremond E. M., Doughty C. L., Williams A. P., Parker J. D., Hayes M. A., et al. (2019). Climate-driven regime shifts in a mangrove–salt marsh ecotone over the past 250 years. Proc. Natl. Acad. Sci. U.S.A. 116 (43), 21602–21608. doi: 10.1073/pnas.1902181116
Chefaoui R. M., Duarte C. M., Serrão E. A. (2018). Dramatic loss of seagrass habitat under projected climate change in the Mediterranean Sea. Global Change Biol. 24 (10), 4919–4928. doi: 10.1111/gcb.14401
Collier C. J., Ow Y. X., Langlois L., Uthicke S., Johansson C. L., O'Brien K. R., et al. (2017). Optimum temperatures for net primary productivity of three tropical seagrass species. Front. Plant Sci. 8. doi: 10.3389/fpls.2017.01446
Collier C. J., Uthicke S., Waycott M. (2011). Thermal tolerance of two seagrass species at contrasting light levels: implications for future distribution in the great barrier reef. Limnol. Oceanogr. 56 (6), 2200–2210. doi: 10.4319/lo.2011.56.6.2200
den Hartog C., Kuo J. (2006). “Taxonomy and biogeography of seagrasses,” in Seagrasses: biology, ecology and conservation. Eds. Larkum A. W. D., Orth R. J., Duarte C. M. (Dordrecht: Springer Netherlands), 1–23.
Dennison W. C., Alberte R. S. (1985). Role of daily light period in the depth distribution of Zostera marina (eelgrass). Mar. Ecol. Prog. Ser. 25, 51–65.
Dennison W. C., Alberte R. S.. (1986). Photoadaptation and growth of Zostera marina L. (eelgrass) transplants along a depth gradient. J. Exp. Mar. Biol. Ecol. 93 (3), 265–282. doi: 10.1016/0022-0981(86)90217-0
Dewsbury B. M., Bhat M., Fourqurean J. W. (2016). A review of seagrass economic valuations: gaps and progress in valuation approaches. Ecosyst. Serv. 18, 68–77. doi: 10.1016/j.ecoser.2016.02.010
Duarte B., Martins I., Rosa R., Matos A. R., Roleda M. Y., Reusch T. B. H., et al. (2018). Climate change impacts on seagrass meadows and macroalgal forests: an integrative perspective on acclimation and adaptation potential. Front. Mar. Sci. 5. doi: 10.3389/fmars.2018.00190
Erftemeijer P. L. A., Stapel J. (1999). Primary production of deep-water Halophila ovalis meadows. Aquat. Bot. 65 (1), 71–82. doi: 10.1016/S0304-3770(99)00032-7
George R., Gullström M., Mangora M. M., Mtolera M. S. P., Björk M. (2018). High midday temperature stress has stronger effects on biomass than on photosynthesis: a mesocosm experiment on four tropical seagrass species. Ecol. Evol. 8 (9), 4508–4517. doi: 10.1002/ece3.3952
Georgiou D., Alexandre A., Luis J., Santos R. (2016). Temperature is not a limiting factor for the expansion of Halophila stipulacea throughout the Mediterranean Sea. Mar. Ecol. Prog. Ser. 544, 159–167. doi: 10.3354/meps11582
Gerakaris V., Lardi P.-l., Issaris Y. (2020). First record of the tropical seagrass species halophila decipiens ostenfeld in the Mediterranean Sea. Aquat. Bot. 160, 103151. doi: 10.1016/j.aquabot.2019.103151
Hammer K. J., Borum J., Hasler-Sheetal H., Shields E. C., Sand-Jensen K., Moore K. A. (2018). High temperatures cause reduced growth, plant death and metabolic changes in eelgrass Zostera marina. Mar. Ecol. Prog. Ser. 604, 121–132. doi: 10.3354/meps12740
Hansen A. B., Pedersen A. S., Kühl M., Brodersen K. E. (2022). Temperature effects on leaf and epiphyte photosynthesis, bicarbonate use and diel O2 budgets of the seagrass Zostera marina l. Front. Mar. Sci. 9. doi: 10.3389/fmars.2022.822485
Herzka S. Z., Dunton K. H. (1997). Seasonal photosynthetic patterns of the seagrass Thalassia testudinum in the western gulf of Mexico. Mar. Ecol. Prog. Ser. 152, 103–117. doi: 10.3354/meps152103
Herzka S. Z., Dunton K. H. (1998). Light and carbon balance in the seagrass Thalassia testudinum : evaluation of current production models. Mar. Biol. 132 (4), 711–721. doi: 10.1007/s002270050435
Holmer M., Hasler-Sheetal H. (2014). Sulfide intrusion in seagrasses assessed by stable sulfur isotopes–a synthesis of current results. Front. Mar. Sci. 1. doi: 10.3389/fmars.2014.00064
Hyndes G. A., Heck J. K. L., Vergés A., Harvey E. S., Kendrick G. A., Lavery P. S., et al. (2016). Accelerating tropicalization and the transformation of temperate seagrass meadows. BioScience 66 (11), 938–948. doi: 10.1093/biosci/biw111
Jassby A. D., Platt T.. (1976). Mathematical formulation of the relationship between photosynthesis and light for phytoplankton. Limnol. Oceanogr. 21 (4), 540–547. doi: 10.4319/lo.1976.21.4.0540
Josselyn M., Fonseca M., Niesen T., Larson R. (1986). Biomass, production and decomposition of a deep water seagrass, Halophila decipiens ostenf. Aquat. Bot. 25, 47–61. doi: 10.1016/0304-3770(86)90039-2
Kikuchi K., Kawasaki Y., Sato S. (2001). Effect of seasonal changes on the carbohydrate levels of eelgrass Zostera marina at odawa bay. Fish. Sci. 67 (4), 755–757. doi: 10.1046/j.1444-2906.2001.00317.x
Kim S. H., Kim Y. K., Kim H., Lee K.-S. (2020b). Recolonization dynamics of warm affinity Halophila nipponica in a temperate seagrass meadow with Zostera marina. Front. Mar. Sci. 7. doi: 10.3389/fmars.2020.00500
Kim S. H., Kim J.-H., Park S. R., Lee K.-S. (2014). Annual and perennial life history strategies of Zostera marina populations under different light regimes. Mar. Ecol. Prog. Ser. 509, 1–13. doi: 10.3354/meps10899
Kim S. H., Kim Y. K., Park S. R., Li W.-T., Lee K.-S. (2012). Growth dynamics of the seagrass Halophila nipponica, recently discovered in temperate coastal waters of the Korean peninsula. Mar. Biol. 159 (2), 255–267. doi: 10.1007/s00227-011-1804-6
Kim Y., Lee J. H., Kang J. J., Lee J. H., Lee H. W., Kang C. K., et al. (2019). River discharge effects on the contribution of small-sized phytoplankton to the total biochemical composition of POM in the gwangyang bay, Korea. Estuar. Coast. Shelf. S. 226, 106293. doi: 10.1016/j.ecss.2019.106293
Kim J. B., Park J.-I., Jung C.-S., Lee P.-Y., Lee K.-S. (2009). Distributional range extension of the seagrass Halophila nipponica into coastal waters off the Korean peninsula. Aquat. Bot. 90 (3), 269–272. doi: 10.1016/j.aquabot.2008.10.007
Kim M., Qin L.-Z., Kim S. H., Song H.-J., Kim Y. K., Lee K.-S. (2020a). Influence of water temperature anomalies on the growth of Zostera marina plants held under high and low irradiance levels. Estuar. Coast. 43 (3), 463–476. doi: 10.1007/s12237-019-00578-2
Kuo J., Kanamoto Z., Iizumi H., Aioi K., Mukai H. (2006a). Seagrasses from the nansei islands, southern Japanese archipelago: species composition, distribution and biogeography. Mar. Ecol. 27 (4), 290–298. doi: 10.1111/j.1439-0485.2006.00098.x
Kuo J., Kanamoto Z., Iizumi H., Mukai H. (2006b). Seagrasses of the genus Halophila thouars (Hydrocharitaceae) from Japan. Acta Phytotax. Geobot. 57 (2), 129–154. doi: 10.18942/apg.KJ00004622858
Lee K.-S., Kim S. H., Kim Y. K. (2018). “Current status of seagrass habitat in Korea,” in The wetland book: II: distribution, description, and conservation. Eds. Finlayson C. M., Milton G. R., Prentice R. C., Davidson N. C. (Dordrecht: Springer Netherlands), 1589–1596.
Lee J. H., Lee D., Kang J. J., Joo H. T., Lee J. H., Lee H. W., et al. (2017). The effects of different environmental factors on the biochemical composition of particulate organic matter in gwangyang bay, south Korea. Biogeosciences 14 (7), 1903–1917. doi: 10.5194/bg-14-1903-2017
Lee K.-S., Park S. R., Kim J.-B. (2005). Production dynamics of the eelgrass, Zostera marina in two bay systems on the south coast of the Korean peninsula. Mar. Biol. 147 (5), 1091–1108. doi: 10.1007/s00227-005-0011-8
Lee K.-S., Park S. R., Kim Y. K. (2007). Effects of irradiance, temperature, and nutrients on growth dynamics of seagrasses: a review. J. Exp. Mar. Biol. Ecol. 350 (1–2), 144–175. doi: 10.1016/j.jembe.2007.06.016
Marín-Guirao L., Sandoval-Gil J. M., Bernardeau-Esteller J., Ruíz J. M., Sánchez-Lizaso J. L. (2013). Responses of the Mediterranean seagrass Posidonia oceanica to hypersaline stress duration and recovery. Mar. Environ. Res. 84, 60–75. doi: 10.1016/j.marenvres.2012.12.001
Masini R. J., Manning C. R. (1997). The photosynthetic responses to irradiance and temperature of four meadow-forming seagrasses. Aquat. Bot. 58 (1), 21–36. doi: 10.1016/S0304-3770(97)00008-9
Moreno-Marín F., Brun F. G., Pedersen M. F. (2018). Additive response to multiple environmental stressors in the seagrass Zostera marina l. Limnol. Oceanogr. 63 (4), 1528–1544. doi: 10.1002/lno.10789
Nejrup L. B., Pedersen M. F. (2008). Effects of salinity and water temperature on the ecological performance of Zostera marina. Aquat. Bot. 88 (3), 239–246. doi: 10.1016/j.aquabot.2007.10.006
Nordlund L. M., Jackson E. L., Nakaoka M., Samper-Villarreal J., Beca-Carretero P., Creed J. C. (2018). Seagrass ecosystem services–what's next? Mar. pollut. Bull. 134, 145–151. doi: 10.1016/j.marpolbul.2017.09.014
Oczkowski A., McKinney R., Ayvazian S., Hanson A., Wigand C., Markham E. (2015). Preliminary evidence for the amplification of global warming in shallow, intertidal estuarine waters. PLoS One 10 (10), e0141529. doi: 10.1371/journal.pone.0141529
Olivé I., Vergara J. J., Pérez-Lloréns J. L. (2013). Photosynthetic and morphological photoacclimation of the seagrass Cymodocea nodosa to season, depth and leaf position. Mar. Biol. 160 (2), 285–297. doi: 10.1007/s00227-012-2087-2
Osland M. J., Stevens P. W., Lamont M. M., Brusca R. C., Hart K. M., Waddle J. H., et al. (2021). Tropicalization of temperate ecosystems in north America: the northward range expansion of tropical organisms in response to warming winter temperatures. Global Change Biol. 27 (13), 3009–3034. doi: 10.1111/gcb.15563
Park S. R., Kim J.-H., Kang C.-K., An S., Chung I. K., Kim J. H., et al. (2009). Current status and ecological roles of Zostera marina after recovery from large-scale reclamation in the nakdong river estuary, Korea. Estuar. Coast. Shelf. S. 81 (1), 38–48. doi: 10.1016/j.ecss.2008.10.003
Park J.-I., Kim J. H., Song H.-J., KIM G. Y. (2020). Distribution of the seagrass, Zostera spp. in ulleungdo. Sea: J. Korean Soc. Oceanography 25 (4), 106–116. doi: 10.7850/jkso.2020.25.4.106
Park J.-I., Lee K.-S. (2009). Peculiar growth dynamics of the surfgrass Phyllospadix japonicus on the southeastern coast of Korea. Mar. Biol. 156 (11), 2221–2233. doi: 10.1007/s00227-009-1250-x
Pedersen O., Colmer T. D., Borum J., Zavala-Perez A., Kendrick G. A. (2016). Heat stress of two tropical seagrass species during low tides – impact on underwater net photosynthesis, dark respiration and diel in situ internal aeration. New Phytol. 210 (4), 1207–1218. doi: 10.1111/nph.13900
Poloczanska E. S., Brown C. J., Sydeman W. J., Kiessling W., Schoeman D. S., Moore P. J., et al. (2013). Global imprint of climate change on marine life. Nat. Clim. Change 3, 919–925. doi: 10.1038/nclimate1958
Qin L.-Z., Kim S. H., Song H.-J., Kim H. G., Suonan Z., Kwon O., et al. (2020a). Long-term variability in the flowering phenology and intensity of the temperate seagrass Zostera marina in response to regional sea warming. Ecol. Indic. 119, 106821. doi: 10.1016/j.ecolind.2020.106821
Qin L.-Z., Kim S. H., Song H.-J., Suonan Z., Kim H., Kwon O., et al. (2020b). Influence of regional water temperature variability on the flowering phenology and sexual reproduction of the seagrass Zostera marina in Korean coastal waters. Estuar. Coast. 43 (3), 449–462. doi: 10.1007/s12237-019-00569-3
Ralph P. J., Durako M. J., Enríquez S., Collier C. J., Doblin M. A. (2007). Impact of light limitation on seagrasses. J. Exp. Mar. Biol. Ecol. 350 (1–2), 176–193. doi: 10.1016/j.jembe.2007.06.017
Rasmusson L. M., Gullström M., Gunnarsson P. C. B., George R., Björk M. (2019). Estimation of a whole plant Q10 to assess seagrass productivity during temperature shifts. Sci. Rep. 9 (1), 12667. doi: 10.1038/s41598-019-49184-z
Said N. E., McMahon K., Lavery P. S. (2021). Accounting for the influence of temperature and location when predicting seagrass (Halophila ovalis) photosynthetic performance. Estuar. Coast. Shelf. S. 257, 107414. doi: 10.1016/j.ecss.2021.107414
Sanz-Lázaro C., Valdemarsen T., Marín A., Holmer M. (2011). Effect of temperature on biogeochemistry of marine organic-enriched systems: implications in a global warming scenario. Ecol. Appl. 21 (7), 2664–2677. doi: 10.1890/10-2219.1
Shields E. C., Parrish D., Moore K. (2019). Short-term temperature stress results in seagrass community shift in a temperate estuary. Estuar. Coast. 42 (3), 755–764. doi: 10.1007/s12237-019-00517-1
Short F., Carruthers T., Dennison W., Waycott M. (2007). Global seagrass distribution and diversity: a bioregional model. J. Exp. Mar. Biol. Ecol. 350 (1–2), 3–20. doi: 10.1016/j.jembe.2007.06.012
Staehr P. A., Borum J. (2011). Seasonal acclimation in metabolism reduces light requirements of eelgrass (Zostera marina). J. Exp. Mar. Biol. Ecol. 407 (2), 139–146. doi: 10.1016/j.jembe.2011.05.031
Suonan Z., Kim S. H., Qin L.-Z., Kim H., Zhang F., Lee K.-S. (2022). Increased coastal nutrient loading enhances reproductive intensity of Zostera marina: implications for seagrass meadow resilience. Front. Mar. Sci. 9. doi: 10.3389/fmars.2022.832035
Tanaka Y., Nakaoka M. (2007). Interspecific variation in photosynthesis and respiration balance of three seagrasses in relation to light availability. Mar. Ecol. Prog. Ser. 350, 63–70. doi: 10.3354/meps07103
Thomson J. A., Burkholder D. A., Heithaus M. R., Fourqurean J. W., Fraser M. W., Statton J., et al. (2015). Extreme temperatures, foundation species, and abrupt ecosystem change: an example from an iconic seagrass ecosystem. Global Change Biol. 21 (4), 1463–1474. doi: 10.1111/gcb.12694
Torquemada Y. F., Durako M. J., Lizaso J. L. S. (2005). Effects of salinity and possible interactions with temperature and pH on growth and photosynthesis of Halophila johnsonii eiseman. Mar. Biol. 148 (2), 251–260. doi: 10.1007/s00227-005-0075-5
Uchimura M., Faye E. J., Shimada S., Inoue T., Nakamura Y. (2008). A reassessment of Halophila species (Hydrocharitaceae) diversity with special reference to Japanese representatives. Bot. Mar. 51 (4), 258–268. doi: 10.1515/BOT.2008.036
Unsworth R. K., Nordlund L. M., Cullen-Unsworth L. C. (2019). Seagrass meadows support global fisheries production. Conserv. Lett. 12 (1), e12566. doi: 10.1111/conl.12566
Vergés A., Steinberg P. D., Hay M. E., Poore A. G. B., Campbell A. H., Ballesteros E., et al. (2014). The tropicalization of temperate marine ecosystems: climate-mediated changes in herbivory and community phase shifts. Proc. R. Soc B: Biol. Sci. 281 (1789), 20140846. doi: 10.1098/rspb.2014.0846
Virnstein R. W., Hall L. M. (2009). Northern range extension of the seagrasses Halophila johnsonii and Halophila decipiens along the east coast of Florida, USA. Aquat. Bot. 90 (1), 89–92. doi: 10.1016/j.aquabot.2008.05.007
Wernberg T., Bennett S., Babcock R. C., de Bettignies T., Cure K., Depczynski M., et al. (2016). Climate-driven regime shift of a temperate marine ecosystem. Science 353 (6295), 169–172. doi: 10.1126/science.aad8745
Xu S., Qiao Y., Xu S., Yue S., Zhang Y., Liu M., et al. (2021). Diversity, distribution and conservation of seagrass in coastal waters of the liaodong peninsula, north yellow Sea, northern China: implications for seagrass conservation. Mar. pollut. Bull. 167, 112261. doi: 10.1016/j.marpolbul.2021.112261
Yoshida G., Hori M., Shimabukuro H., Hamaoka H., Onitsuka T., Hasegawa N., et al. (2019). “Carbon sequestration by seagrass and macroalgae in Japan: estimates and future needs,” in Blue carbon in shallow coastal ecosystems: carbon dynamics, policy, and implementation. Eds. Kuwae T., Hori M. (Singapore: Springer Singapore), 101–127.
Keywords: carbon budget, climate change, Halophila nipponcia, photosynthesis, Zostera marina
Citation: Kim SH, Kim H, Suonan Z, Zhang F and Lee K-S (2023) Photosynthesis and whole-plant carbon balances of warm affinity Halophila nipponica and cold affinity Zostera marina in relation to water temperature rise: implication for future geographic distribution. Front. Mar. Sci. 10:1203809. doi: 10.3389/fmars.2023.1203809
Received: 11 April 2023; Accepted: 19 June 2023;
Published: 29 June 2023.
Edited by:
Kasper Elgetti Brodersen, University of Copenhagen, DenmarkReviewed by:
Sutinee Sinutok, Prince of Songkla University, ThailandCopyright © 2023 Kim, Kim, Suonan, Zhang and Lee. This is an open-access article distributed under the terms of the Creative Commons Attribution License (CC BY). The use, distribution or reproduction in other forums is permitted, provided the original author(s) and the copyright owner(s) are credited and that the original publication in this journal is cited, in accordance with accepted academic practice. No use, distribution or reproduction is permitted which does not comply with these terms.
*Correspondence: Kun-Seop Lee, a2xlZUBwdXNhbi5hYy5rcg==
Disclaimer: All claims expressed in this article are solely those of the authors and do not necessarily represent those of their affiliated organizations, or those of the publisher, the editors and the reviewers. Any product that may be evaluated in this article or claim that may be made by its manufacturer is not guaranteed or endorsed by the publisher.
Research integrity at Frontiers
Learn more about the work of our research integrity team to safeguard the quality of each article we publish.