- 1MARBEC (Marine Biodiversity, Exploitation and Conservation), Univ Montpellier, CNRS, Ifremer, IRD, Montpellier, France
- 2MEDIMEER (Mediterranean platform for Marine Ecosystems Experimental Research), Observatoire de Recherche Méditerranéen de l’Environnement, Univ Montpellier, CNRS, IRD, INRAE, Sète, France
- 3Sorbonne Université, CNRS, Laboratoire d’Océanographie Microbienne (LOMIC), Observatoire Océanologique de Banyuls, Banyuls/mer, France
The Mediterranean region is undergoing an increase in the frequency and intensity of extreme rainfall events, resulting in terrestrial runoffs that can affect aquatic environments in coastal regions. The goal of this study was to investigate the effects of terrestrial runoff on natural coastal planktonic assemblages. For this purpose, an in situ mesocosm experiment was conducted in May 2021 in the Mediterranean Thau Lagoon. A terrestrial runoff event was simulated in duplicate mesocosms by adding natural forest soil that was left to maturate naturally for two weeks in river water. After the addition of maturated soil, the abundance and diversity within the planktonic food web, from viruses to metazooplankton, were monitored for 18 days. The addition of maturated soil to the terrestrial runoff treatment greatly depressed the light availability in the mesocosms and potentially enhanced flocculation and sedimentation in the mesocosms, resulting in an immediate negative effect on phytoplankton, decreasing the chlorophyll-a (Chl-a) concentration by 70% for 12 days. Afterward, remineralized nutrient in the terrestrial runoff treatment induced a subsequent positive effect on phytoplankton, which resulted in a diatom bloom and an increase in picophytoplankton and cyanobacteria abundance toward the end of the experiment. Overall, the Chl-a concentration was 30% lower in the terrestrial runoff treatment over the 18 days of experiment, whereas bacteria were 15% more abundant than in the control. This suggests that over the course of the experiment, the addition of maturated soil favoured bacteria instead of phytoplankton at the base of the planktonic food web. The addition of the maturated soil was detrimental for all protozooplankton groups and mixotrophic dinoflagellates, but seemed to favour metazooplankton, notably mollusk larvae, copepod nauplii, and rotifers. This implies that in the terrestrial runoff treatment, the preferential pathway for biomass transfer was through the direct consumption of bacteria and/or phytoplankton by metazooplankton. Therefore, in Thau Lagoon, after a terrestrial runoff, the transfer of biomass within the planktonic food web would potentially be more efficient by promoting direct transfer from the base to the top of the food web, subsiding intermediate trophic levels such as protozooplankton.
1 Introduction
Extreme rainfall is a natural event that causes terrestrial runoff, which can export large quantities of terrestrial organic and mineral substances into coastal aquatic environments (Fouilland et al., 2012; Meunier et al., 2017). Aquatic environments characterized by a strong land interface, such as semi-closed coastal lagoons, are particularly affected by this phenomenon (Alpert et al., 2002; Nunes et al., 2009; Liess et al., 2016). According to future climate change scenarios, extreme rainfall events in the Mediterranean region are expected to increase in intensity and frequency (Alpert et al., 2002; Sánchez et al., 2004).
Even though all inorganic nutrients are originally from terrestrial ecosystems, terrestrial runoff can carry even more particulate and dissolved organic matter, and mineral nutrients into marine environments which composition depends on the location and type of soil (Nunes et al., 2009; Deininger et al., 2016), and can benefit microorganisms in the microbial food web. Bacteria could benefit from higher allochthonous organic matter resources (Robinson, 2008; Meunier et al., 2017), whereas both bacteria and phytoplankton may benefit from mineral nutrient enrichment (Nixon, 1981; Guadayol et al., 2009).
Alternatively, the discharge of humic matter into coastal ecosystems can also reduce light penetration and darken water colour (the so-called brownification process), which may affect phytoplankton physiology, production, and biomass (Ask et al., 2009; Soulié et al., 2022). Therefore, the balance between light attenuation and nutrient enrichment caused by runoff may drive phytoplankton and bacterial responses to these events. Studies investigating terrestrial runoff in northern lakes often indicate that the potential negative effects of light attenuation on phytoplankton due to the increased terrestrial matter can be partially counterbalanced by the input of organic and inorganic nutrients (Ask et al., 2009; Meunier et al., 2017). In accordance with this, a study investigating a Mediterranean coastal lagoon reported higher phytoplankton abundance upon soil addition as a response to higher nutrient concentrations, indicating that phytoplankton adapted very quickly to light attenuation, which was not sufficient to induce a clear negative effect (Deininger et al., 2016).
Phytoplankton and bacteria are two essential food sources on which the entire microbial food web functions relies (Azam et al., 1983; Mostajir et al., 2015). Through bottom-up effects, terrestrial runoff could also impact grazers and ultimately the functioning of the whole plankton food web (Deininger et al., 2016; Brett et al., 2017; Meunier et al., 2017). In addition, higher particulate matter concentrations that reduce light penetration in the water column would also be detrimental for visual predators, having a direct negative effect on their grazing efficiency and, ultimately, their abundance (Soulié et al., 2022). However, Liess et al. (2015) reported no effects of soil addition on protozooplankton and metazooplankton, whereas they reported an increase in phytoplankton abundance in an in situ mesocosm study conducted in the Mediterranean Thau Lagoon, indicating that terrestrial runoff may induce a rupture in the prey-predator link responses.
Studies assessing the effect of terrestrial runoff or humic substance addition on aquatic ecosystems functioning have been increasing in the past few years, particularly in the northern freshwater ecosystems. However, little is known regarding terrestrial runoff in coastal environments and how marine plankton respond to it.
Furthermore, a large gap remains concerning broad-range investigations of plankton communities, encompassing multiple trophic levels, from viruses to zooplankton. In line with these issues, the goal of the present study was to assess the responses of coastal plankton communities to simulated terrestrial runoff in Mediterranean coastal waters. The novelty of the present investigation is to study the impact of terrestrial runoff simultaneously on key components of the plankton food web including viruses, bacteria, different groups of phytoplankton, protozooplankton and metazooplankton, to determine how their dynamics would be affected by an experimental terrestrial runoff, and whether this could affect the biomass transfer within the plankton food web.
An in situ mesocosm experiment was conducted in a Mediterranean coastal lagoon in spring 2021, during which the responses of physical, chemical, and biological variables, including viruses, bacteria, phytoplankton, and zooplankton communities, toward an experimental terrestrial runoff were investigated during the 20 days of the experiment.
2 Materials and methods
Thau lagoon is located in South of France (Figure 1) and is a mesotrophic Mediterranean lagoon of 75 km2, characterized by a great amplitude in water temperature from 4°C to 30°C due to the shallowness of the water column (4 m depth in average) and with a gradient of salinity ranging from 34 to 38 (Souchu et al., 2010, Trombetta et al., 2019). Thau lagoon is subject every year to the so called “Cevenol” events that are characterized by heavy rainfall that can last for few days usually in early fall. These extreme meteorological events lead to flooding and runoff in the watershed of the Vène River, which is the main inlet of the Thau Lagoon. When a flood occurs in Thau lagoon, it usually brings high concentrations of nitrate, silicate, and suspended matter (up to 42.7 µM N and 47.4 µM Si), while decreasing the salinity along a gradient from 37 to 19.5 (Pecqueur et al., 2011).
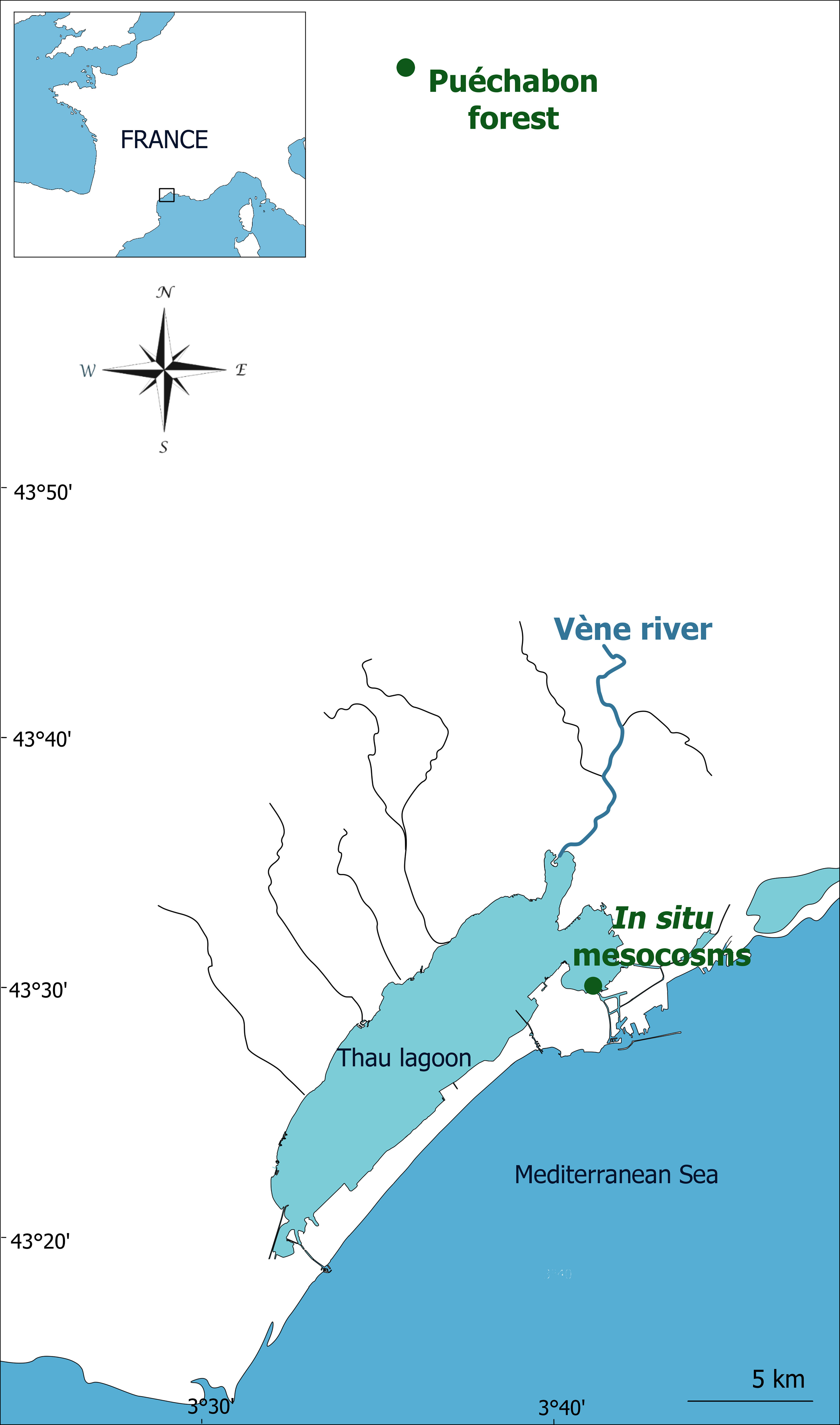
Figure 1 Location of the in situ mesocosms in the coastal Tau lagoon, and of the Puéchabon forest and Vène River from which were sampled the soil and river water respectively, for the preparation of the natural terrestrial runoff.
To simulate terrestrial runoff into the Lagoon, a Mediterranean oak forest soil typical of the watershed of the lagoon was collected from the Puéchabon state forest, located approximately 30 km North from Thau lagoon (3°35′45′′E, 43°44′29′′N). The Puéchabon forest was last cut in 1942 and is now fully preserved (Rambal et al., 2004), portraying a typical regional Mediterranean forest. After removing the upper humus layer, the surface clay-rich soil was collected and screened roughly over a 1 cm mesh. On the same day that the soil was shovelled, river water was collected from the Vène River, which is known for its episodic river flash floods (Pecqueur et al., 2011) reaching the Thau Lagoon. The river water was then sieved through a 200 µm mesh to remove any large particles.
To simulate the residential time of the soil in the river water, we chose to mature the soil naturally as the artificial maturation would have required chemical extraction (Deininger et al., 2016). Thus, the soil and river water were mixed to a proportion of 416 g of fresh soil per 1 L of river water, based on the protocol described by Müller et al. (2018) and the description of a real river flash flood in the Thau Lagoon (Fouilland et al., 2012). This stock solution was then left to maturate for two weeks into several transparent polycarbonate carboys placed into a pool supplied continuously with Thau Lagoon water. During maturation, carboys were homogenized manually and aerated daily.
2.1 Mesocosm experiment
A mesocosm experiment was conducted from May 3rd to 21st, 2021 in the Mediterranean coastal Thau Lagoon at the Mediterranean platform for Marine Ecosystems Experimental Research (MEDIMEER 43240 0000 N, 3360 0000 E, Sète, France).
On day 0 of the experiment (May 3rd), six mesocosms (hermetic closed bags) were immersed in the lagoon and filled simultaneously using six pipelines with 2200 L of water from a 1000 µm screened pool of lagoon water sampled at 1 m depth and approximately 70 m from the shore using a pump (SXM2/A SG, Flygt). Mesocosms were 1.2 m wide, 3 m long, and were made of 200-µm-thick vinyl acetate mixed polyethylene transparent material strengthened by nylon mesh (Insinööritoimisto Haikonen Ky). Once the mesocosms were filled, the water column inside each mesocosm was 2 m deep. All mesocosms were covered by domes made from transparent polyvinyl chloride, allowing a transmission of 73% of the photosynthetically available radiation (PAR) to prevent rain or any air-driven contamination. Each mesocosm was equipped with a pump (Rule 360), connected to a tube in the middle of the mesocosms that pumped the water at 50 cm depth and released it 1 m below. Pumps were set at a 3.5 d-1 turnover time, to gently mix the water column inside the mesocosms. Three mesocosms consisting only of Thau lagoon water served as “Control” treatment, whereas in three other mesocosms, 7 L of maturated soil was added after the initial sampling of all mesocosms on day 1 of the experiment. It should be noted that due to the defective function of the mixing pump in one of the control and terrestrial runoff treatments, data from these two mesocosms were excluded from the analysis. Therefore, the data presented hereafter represent the mean of duplicate mesocosms for each treatment within the range of observations.
2.2 Physical, chemical, and biological variables
Salinity was measured every morning using a multiparameter probe (ProSolo, YSI) during the sampling of the mesocosms. The PAR was measured using an underwater quantum sensor (Li-Cor Li-193), whereas the temperature was measured using a temperature probe (Campbell Scientific Thermistore Probe 107), both immersed at 1 m depth.
The daily quantity of photosynthetically active protons received by a surface of 1 m2, hereafter referred as daily light integral at 1 m depth (DLI), was calculated using high-frequency PAR measurements (µmol m−2 s−1) following Equation 1 (Soulié et al., 2022).
All mesocosms were sampled using 5 L Niskin bottles every morning at 08:00 for 20 days. Multiples sub-samples were taken from the Niskin bottles for different chemical analyses. For each analysis, one sample was taken by mesocosm.
Suspended matter (SM), particulate organic carbon and nitrogen (POC and PON, respectively) concentrations were determined from the same water sample (0.5–1 L) which was filtered over burned GF/F filters (25 mm in diameter). For the SM, filters were weighed with a high precision scale (Mettler Toledo) before use and after the filtration and being placed in a stove at 60°C for 12h at least. POC and PON concentrations were determined from the same filter using a CHN analyser (Unicube, Elementar).
Dissolved organic carbon (DOC) samples (20 mL) were filtered through two overlaid combusted (450°C, 6 h) 25 mm GF/F filters (Whatman). The filtrate was transferred to glass vials and poisoned with 85% H3PO4 and kept at 4°C until analysis. DOC concentrations were measured using the high catalytic oxidation technique with a Shimadzu TOC-V analyser (TOC-L-CSH) (Benner and Strom, 1993). DOC and POC concentrations were summed to calculate the total organic carbon (TOC) concentration.
For nutrient analyses, two acid-washed polycarbonate bottles were filled with 50 mL of mesocosm water. Samples were then filtered through 0.45 µm Gelman filter and stored at −20°C until analysis. Nitrate (NO3−), nitrite (NO2−), orthophosphate (PO43−), and silicate (SiO2) concentrations were then determined using an automated colorimeter (Skalar Analytical, with detection limits of 0.125 µM of NO3−, 0.008 µM of NO2−, 0.021 µM of PO43−, and 0.123 µM of SiO2), and ammonium (NH4+) concentrations were determined using the fluorometric method (Holmes et al., 1999).
To investigate the plankton community samples for pigment concentration analysis, flow cytometry, and microscopy, were collected daily. For pigment analysis 0.5 to 1 L samples were filtered over glass-fibre filters (Whatman GF/F, 0.7 μm pore size and 25 mm diameter) in a light-attenuated room using a low vacuum pump. The filters were then quickly frozen in liquid nitrogen and stored at −80°C until analysis. Pigments concentrations and specifically chlorophyll-a (Chl-a), were determined by high-performance liquid chromatography (HPLC, Shimadzu) using the method of Zapata et al. (2000) following the protocol of Vidussi et al. (2011).
For the flow cytometry analysis, samples of 1.5 mL were taken and fixed with glutaraldehyde Grade I at 4% final concentration and kept at −80°C. The small phytoplankton community (< 10 μm) was analyzed using a CytoFLEX flow cytometer (Beckman Coulter, set at a high speed for 3 min). Different phytoplankton groups were then identified and enumerated based on their relative forward or side scatter (FSC and SSC) and their natural pigment fluorescence emissions (Chl-a, phycoerythrin). Two groups of nanophytoplankton (hereafter referred to as Nano1 and Nano2), one group of picophytoplankton (abbreviated as Pico), and one group of Synechococcus (called Cyano) were identified. Larger phytoplankton (> 10 μm) samples (100 mL) were fixed with formaldehyde (4% final dilution), stored in the dark at 4°C, and were analyzed by microscopy. Samples from days 1, 2, 6, 9, 12, 16, and 18 were sedimented in an Utermöhl chamber for 24 h and organisms > 10 μm were counted using an inverted microscope (Olympus IX70). Organisms were identified when possible to the genus levels (Kraberg et al., 2010) and gathered into taxonomic classes.
Samples were also taken every morning to investigate the viruses (1.5 mL), bacteria (1.5 mL), and heterotrophic nanoflagellates (HNF, 4 mL) communities using flow cytometry analysis. Samples of bacteria and HNF were fixed with glutaraldehyde Grade I, at 4% final concentration, and virus samples were fixed with 0.02 µm filtered Grade1 glutaraldehyde, at 2% final concentration. Samples were then frozen into liquid nitrogen and stored at −80°C until analysis. Virus samples were stained with SYBR Green I (0.25% final concentration), and then analyzed using a FACSCanto2 flow cytometer (Becton–Dickinson). Virus-like particles were counted in terms of SSC and induced green fluorescence (530/30 nm) (Brussaard, 2004). Bacterial samples were stained using SYBR Green I (0.25% final concentration) (Marie et al., 1997), analyzed using a FACSCanto2 flow cytometer (Becton–Dickinson), and identified and counted regarding their SSC and induced green fluorescence (530/30 nm). HNF samples were stained with SYBRGreen I (0.25% final concentration) and analyzed using a FACSCanto2 flow cytometer (Becton–Dickinson), according to Christaki et al. (2011). The HNF population was then determined based on SSC and green fluorescence (530/30 nm) and enumerated.
Protozooplankton samples (220 mL) for ciliates and dinoflagellates identification and quantification, were collected daily from the second Niskin, fixed with acidic Lugol (1.8% final dilution), and kept in the dark at 4°C. Samples (days 1, 2, 6, 9, 12, 16, and 18) were then sedimented in an Utermöhl chamber for 24 h and analyzed under an inverted microscope (Olympus IX70). Organisms were counted and identified at the family level (Kraberg et al., 2010) and organized into different groups: naked ciliates, tintinnids, heterotrophic dinoflagellates and mixotrophic dinoflagellates.
Metazooplankton samples were collected on days 1 and 17 using 5 L Niskin bottles that were deployed four times in each mesocosm to collect 20 L, which were screened through a 20 µm mesh size net (Hydrobios). Samples were then fixed with formaldehyde (4% final dilution) until analysis. Mesozooplankton were taxonomically counted and identified to the genus level using a Macroscope (Leica Z16AP0) (Trégouboff and Rose 1957a; Trégouboff and Rose 1957b) and were grouped into broader ecological groups, such as: copepods, copepods nauplii, mollusks, rotifers, worms, and polychaetes larvae, echinodermata, appendicularians, and eggs.
2.3 Statistical analysis
All data analyses were performed using R software (version 3.6). All physical, chemical, and biological time series data were analyzed using repeated measure analysis of variance (RM-ANOVA) to assess significant differences between the runoff treatment and the control. For each variable, treatment was set as a fixed factor and time was set as a random factor. Prior to the RM-ANOVA, the normality of the residuals and random factors was checked. If these assumptions could not be met, the Kruskal-Wallis test was performed. We considered a p value of 0.05 as the level of significance for every test performed. All the RM-ANOVA results are presented in Tables 1, 2.
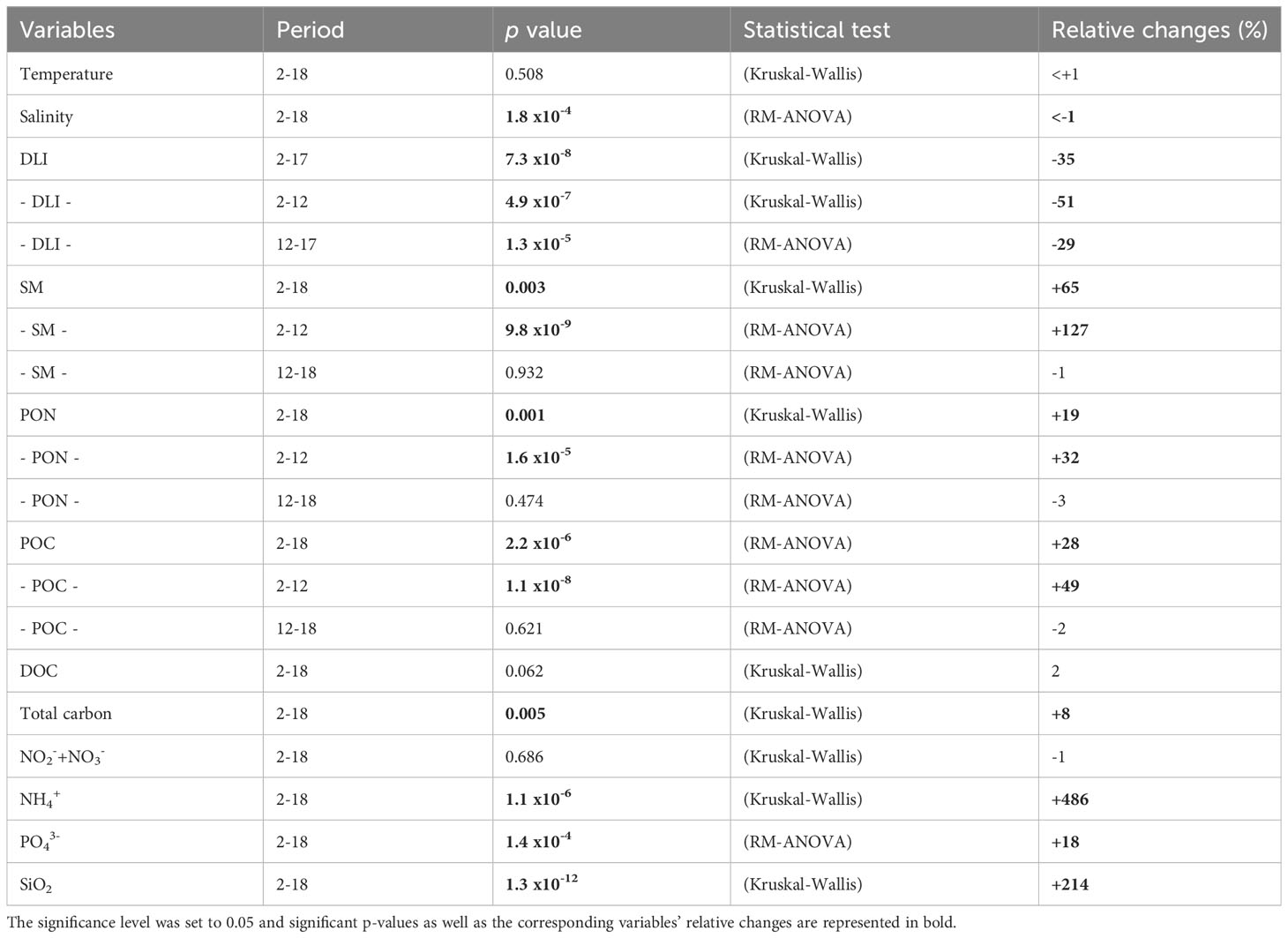
Table 1 Summary table of statistical comparison and relative changes between the control and runoff treatments (from days 2 to 18) for physical and chemical variables.
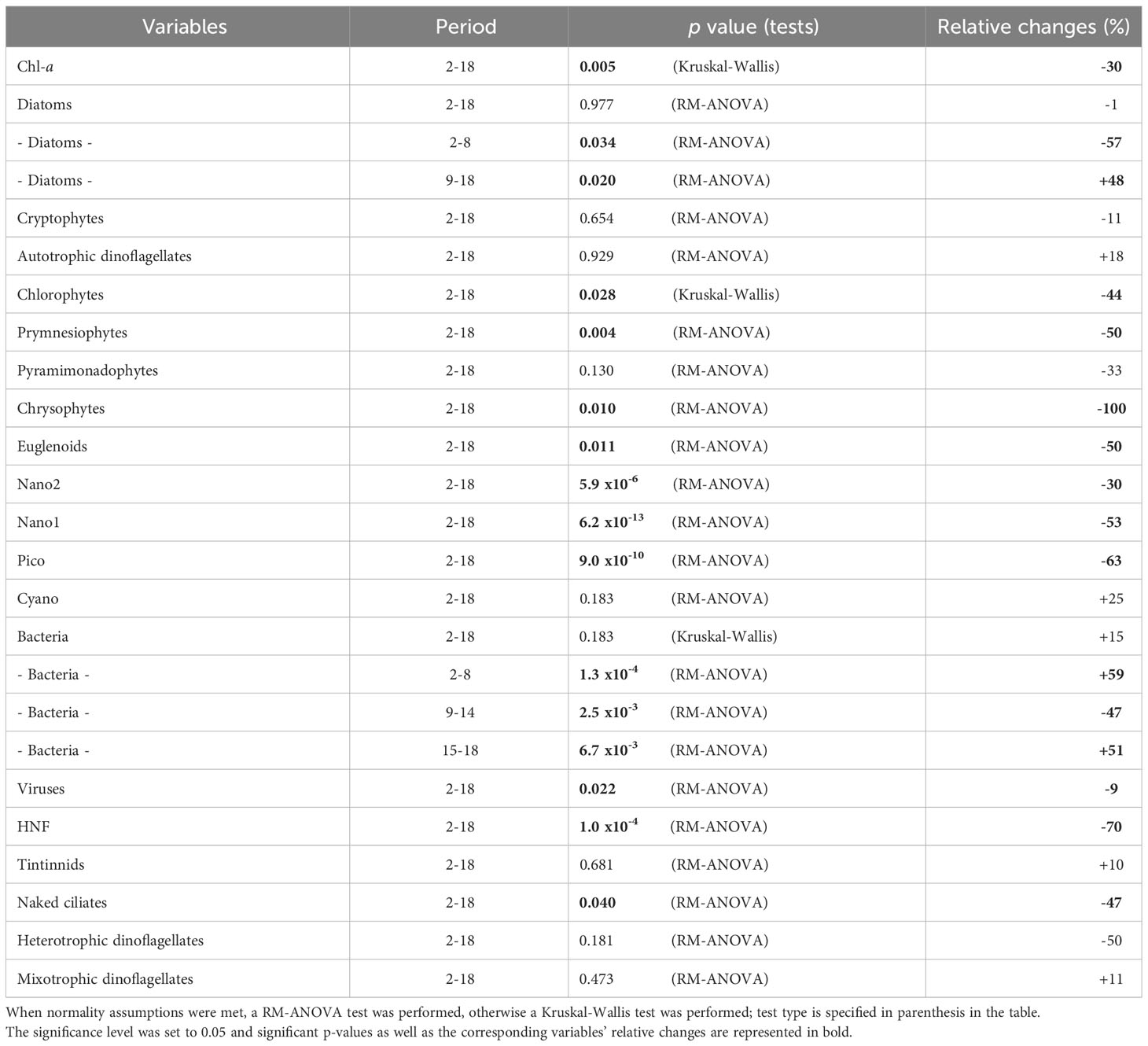
Table 2 Summary table of statistical comparison and relative changes between the control and runoff treatment, for biological variables.
To better analyse the effects of the runoff treatment on the covariance of the physical, chemical and biological variables, Principal Component Analysis (PCA) was performed on the log response ratio of the different variables investigated in the present study from days 3 to 18. More specifically, the DLI, salinity, inorganic nutrients, particulate and dissolved organic matter, suspended matter, Chl-a concentrations, as well as the abundance of viruses, HNF, heterotrophic and mixotrophic dinoflagellates, tintinnids, and naked ciliates were integrated into the analysis. To account for the difference in data frequency, the NAs were imputed by the mean of the corresponding variable. Metazooplankton data were not included in the PCA analysis because of the low availability of data (two data points), and phytoplankton abundances from flow cytometry and microscopy analyses were not integrated into the analysis as they were already represented through Chl-a concentrations.
3 Results
3.1 Physical and chemical variables
Water temperature varied from 16.48 ± 0.01 to 18.01 ± 0.01°C, and was not affected by the terrestrial runoff treatment (Figure 2A, Table 1). Salinity in the control was 38.42 ± 0.07 on average, whereas in the terrestrial runoff treatment the salinity was significantly lower, 38.17 ± 0.09 on average, due to the addition of the soil maturated in river water (Figure 2B). The DLI varied from 5.47 ± 0.21 to24.26 ± 1.98 mol m−2 d−1 in the control treatment. The DLI was significantly reduced in the terrestrial runoff treatment by 43% overall, although this reduction attenuated over time (Figure 2C, Table 1).
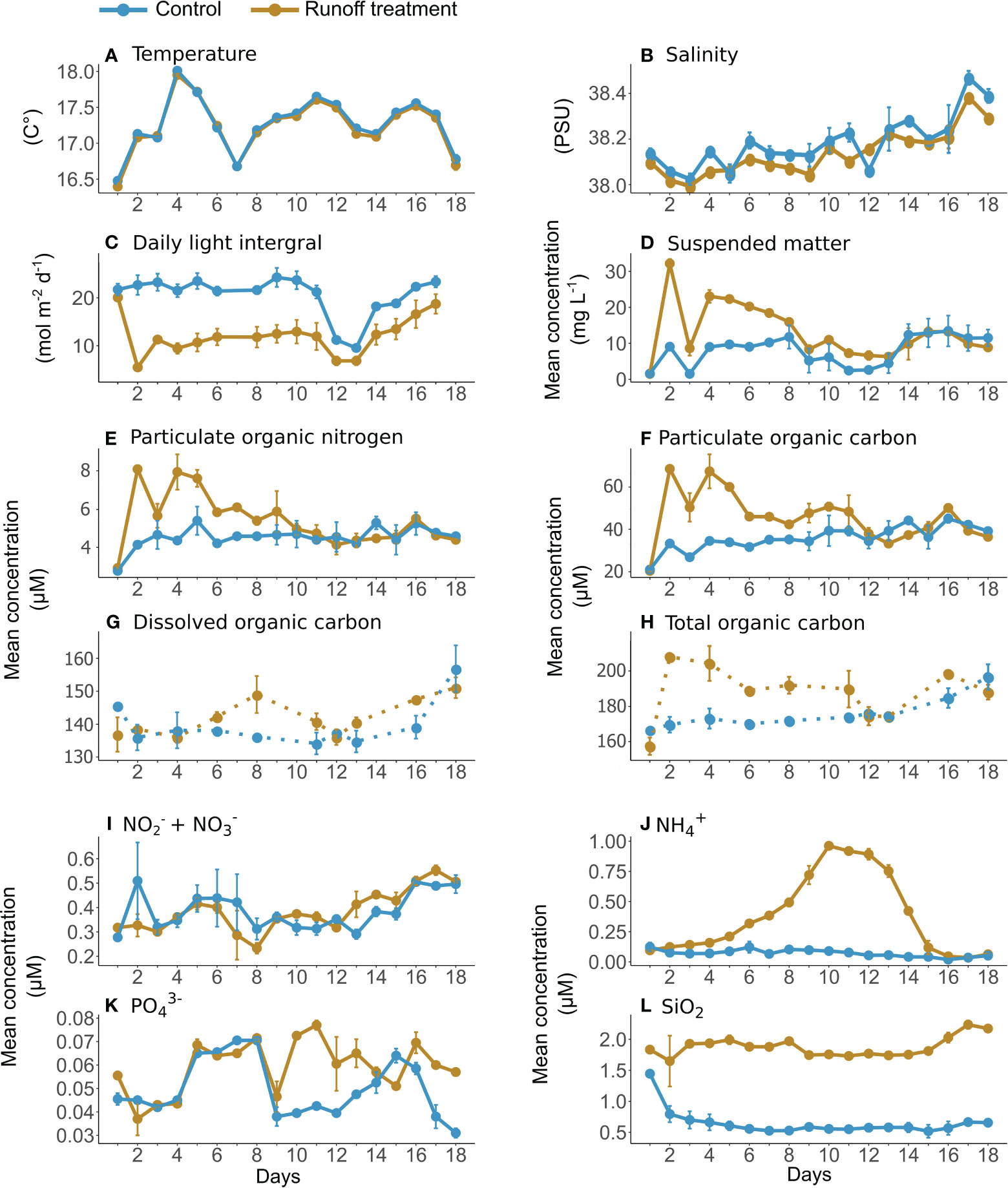
Figure 2 Daily mean water temperature (A), salinity (B), daily light integral (C), suspended matter (D), particulate organic nitrogen (E), particulate organic carbon (F), dissolved organic carbon (G), total organic carbon (H), nitrate + nitrite (I), ammonium (J), orthophosphate (K) and silicate (L) in the control (blue) and terrestrial runoff treatment (gold). The error bars represent the range of the observations. Daily measurements are represented in solid lines whereas data with a lower sampling frequency are represented in dotted lines.
Suspended matter, POC and PON concentrations in the control were on average 8.02 ± 4.12 mg L−1, 0.44 ± 0.07 mg L−1 and 0.06 ± 0.01 mg L−1, respectively. On day 2, 24 h after the addition of the maturated soil, the suspended matter, POC, and PON concentrations in the runoff treatment were higher than in the control, by 255, 104, and 94%, respectively, being significantly higher from days 2 to 12 (Figures 2D–F, Table 1). DOC and total organic carbon concentrations were on average 1.72 ± 0.14 mg L−1 and 2.15 ± 0.13 mg L−1, respectively in the control. However, only total organic carbon concentrations were significantly affected by the runoff treatment (Figures 2G, H, Table 1), whereas DOC concentrations did not differ between treatments.
Inorganic nutrient concentration in the control were on average 0.39 ± 0.08 µM of NO2−+NO3−, 0.07 ± 0.03 µM of NH4+, 0.05 ± 0.01 µM of PO43−, and 0.65 ± 0.21 µM of SiO2. Silicate (SiO2) concentrations increased immediately after the maturated soil addition and were 107% higher in the runoff treatment than in the control on day 2 and were overall 214% significantly higher during the entire experiment (Figure 2L, Table 1). In contrast, nitrate (NO2−+NO3−), ammonium (NH4+), and phosphate (PO43−) concentrations were not directly affected by maturated soil addition, as their concentrations on day 2 were not significantly different between the two treatments. Nonetheless, ammonium increased during the experiment in the runoff treatment, attaining a maximum concentration of 0.96 ± 0.03 µM on day 10, before reducing until the end of the experiment (Figure 2J). Overall, ammonium concentrations were 486% significantly higher in the runoff treatment compared to the control (Table 1). Phosphate concentrations were significantly higher in the runoff treatment than in the control, especially from days 9 to 18 by an average of 37% (Figure 2K, Table 1). Nitrate concentrations were not significantly affected by the runoff treatment over the course of the experiment (Figure 2I, Table 1).
3.2 Phytoplankton community
In the control treatment, Chl-a concentrations varied from 0.57 ± 0.00 to 1.46 ± 0.13 µg L−1 (Figure 3A). In the runoff treatment, Chl-a concentration was significantly lower than in the control by 30% over the course of the experiment, and more specifically from day 2 to 12 by 70% (Figure 3A, Table 2). In the second half of the experiment (from days 12 to 18), Chl-a concentrations quickly increased in the terrestrial runoff treatment forming a bloom-like dynamic, characterized by a fast accumulation of the phytoplankton biomass during consecutive days and followed by a depression (Trombetta et al., 2019), that surpassed the concentrations in the control treatment and reached a maximum value of 1.87 ± 0.36 µg L−1 on day 15 (Figure 3A).
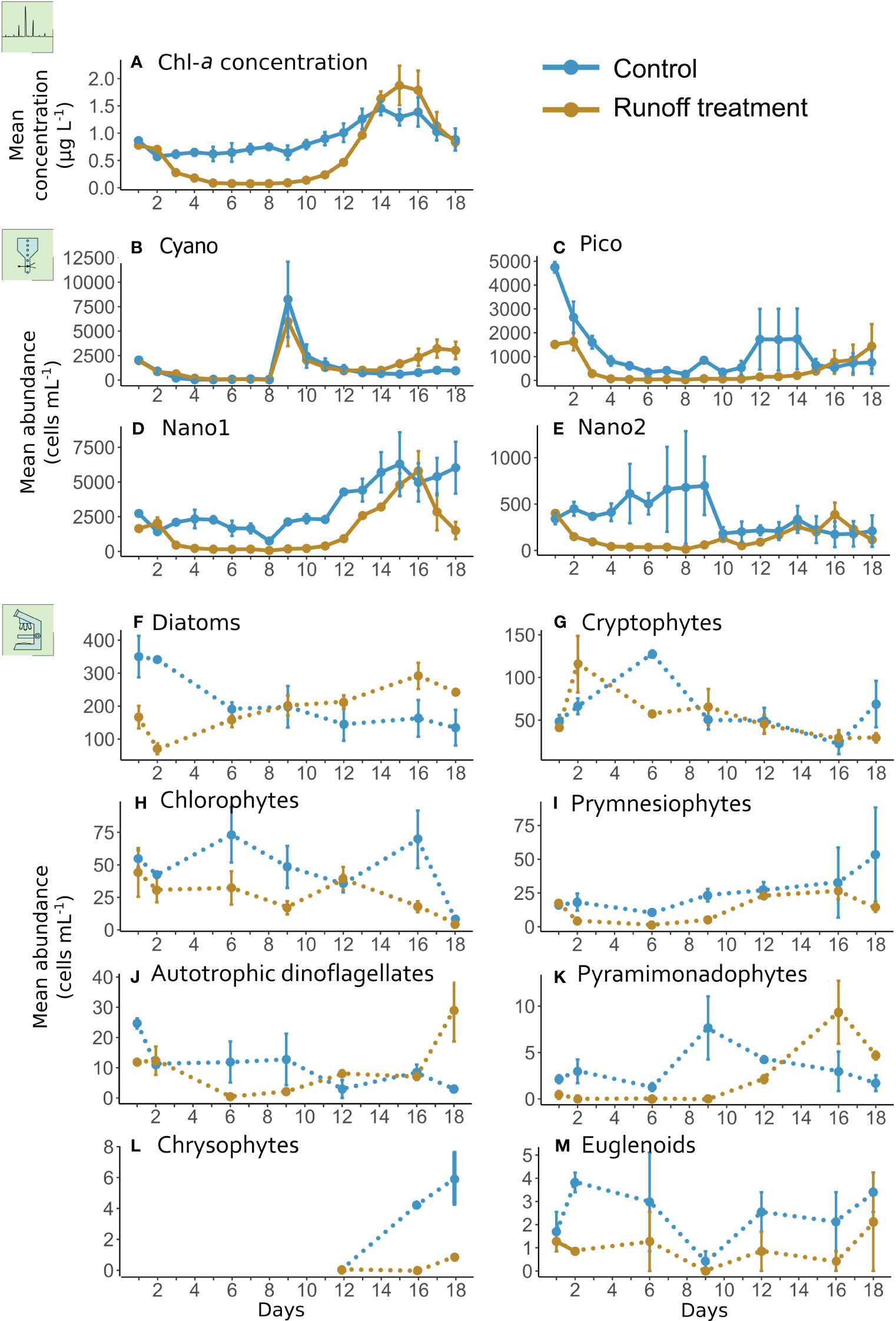
Figure 3 Daily mean Chl-a concentrations (A) and different phytoplankton groups abundance analyzed by flow cytometry (B–E) or using an inverted microscope (F–M), in the control (blue) and terrestrial runoff treatment (gold). The error bars represent the range of the observations. Daily measurements are represented in solid lines whereas data with a lower sampling frequency are represented in dotted lines.
Based on microscopic analysis, the eukaryotic phytoplankton community in the control treatment was dominated by diatoms (135 ± 54 to 350 ± 63 cells mL−1) and cryptophytes (23 ± 12 to 127 ± 4 cells mL−1) (Figures 3B–D). Other phytoplankton groups were present in lower abundances, such as chlorophytes, prymnesiophytes, and autotrophs dinoflagellates (Figures 3E, F). Pyramimonadophytes, chrysophytes, and euglenoids also composed the phytoplankton community but in much lower abundances, with less than 10 cells mL-1 in the control (Figures 3G–I). Flow cytometry analysis revealed that small phytoplankton were generally dominant during the experiment as reflected by the high abundance of Nano1, Pico and Nano2, with abundances ranging from 174 ± 137 to 6297 ± 2281 cells mL−1, respectively, in the control treatment (Figures 3J–L). Cyanobacterial abundance peaked on day 9 in the control, reaching a maximum abundance of 8240 ± 3849 cells mL−1 (Figure 3M).
Almost all phytoplankton abundances were significantly lower in the terrestrial runoff treatment than in the control (Table 2). However, diatom, pyramimonadophyte, and Nano2 abundances increased in the terrestrial runoff treatment, reflecting a bloom-like dynamic, in the second half of the experiment as previously described regarding the Chl-a concentrations. Toward the end of the experiment, autotrophic dinoflagellate, Pico, and Cyano abundances were higher in the terrestrial runoff treatment than in the control (Figure 3).
3.3 Bacterial and virus communities
Bacterial abundance ranged from 0.8×106 ± 0.3×106 to 1.9×106 ± 0.8×106 cells mL−1 over the course of the experiment in the control (Figure 4A). In both the control and runoff treatments, bacterial abundance fluctuated a lot; however, this trend was reversed between the two treatments. From days 2 to 8 and 15 to 18, bacterial abundances were 59 and 51% significantly higher in the runoff treatment compared to the control, respectively. In comparison from days 9 to 14, bacterial abundance was 47% significantly lower in the runoff treatment relative to the control (Figure 4A, Table 2). Overall, over the 18 days of experiment bacterial abundance was 15% higher in the runoff treatment than in the control.
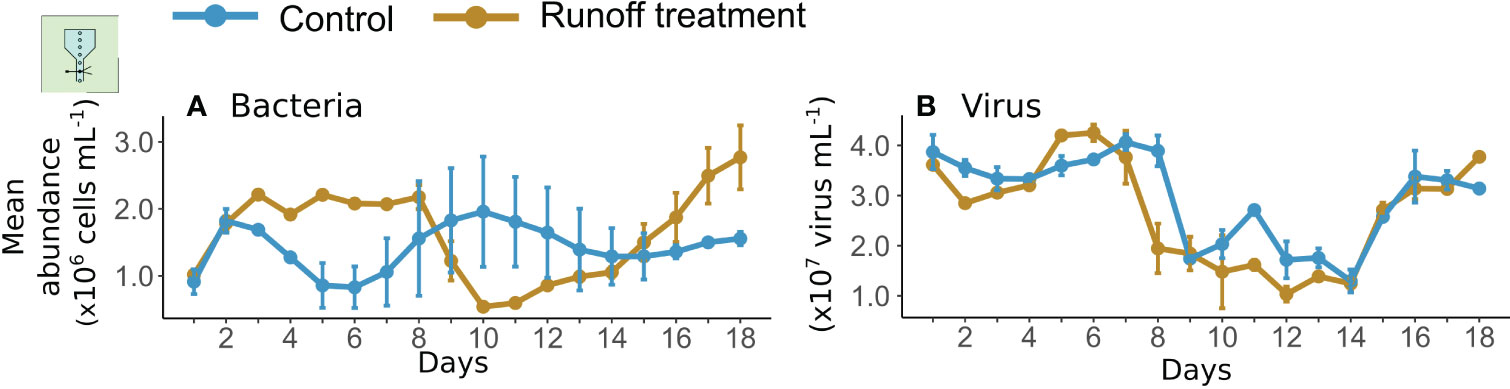
Figure 4 Daily mean abundances of bacteria (A) and viruses (B) in the control (blue) and terrestrial runoff treatment (gold). The error bars represent the range of the observations.
Viruses abundances ranged from 1.3×107 ± 2.3×106 to 4.1×107 ± 1.6×106 viruses mL−1 in the control. Dynamics of the virus abundance were similar in both treatments, although virus abundance was significantly lower in the terrestrial runoff treatment than in the control by 9% (Figure 4B, Table 2).
3.4 Protozooplankton community
Microscopic identification revealed that the dinoflagellate community was mainly composed of small naked dinoflagellates, Oblea sp. and Gyrodinium sp. genus characterized as heterotrophs, as well as Prorocentrum micans and Dinophysis sp., characterized as mixotrophs. The naked ciliate community was mainly composed of Strombidium sp., Cyrtostrombidium sp., Strobilidium sp., and Tiarina sp. whereas tintinnids were represented solely by the genus Tintinnopsis sp.
Heterotrophic nanoflagellates and dinoflagellates were the most abundant protozooplankton groups with abundances ranging from 995 ± 26 to 4959 ± 1622 cells mL−1 and from 4.13 ± 0.63 to 32.91 ± 6.76 cells mL−1 respectively in the control treatment (Figures 5A, D). Mixotrophic dinoflagellates and naked ciliates had lower abundances (<10 cells mL−1) (Figures 5C, E), but tintinnids were the less abundant protozooplankton group in the control treatment, with abundances lower than 0.3 cells mL−1.
The terrestrial runoff treatment had a strong significant negative effect on heterotrophic nanoflagellate and naked ciliate abundances, which were depressed by 70% and 47%, respectively compared to the control (Figure 5B, Table 2). Heterotrophic dinoflagellate abundances in the runoff treatment were 50% less than in control but overall, the difference was not statistically significant. Tintinnids and mixotrophic dinoflagellates did not show any statistically significant response to the treatment.
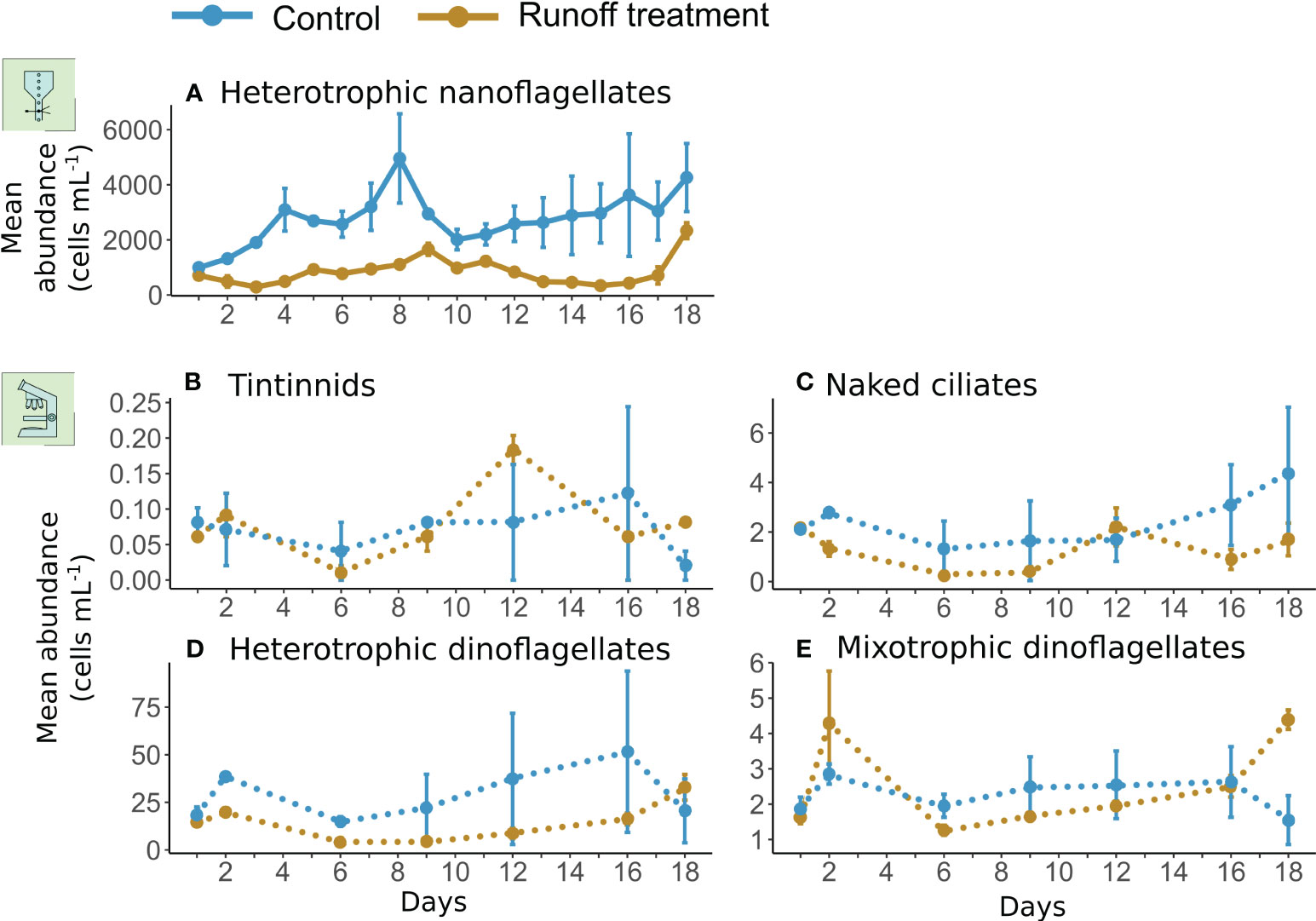
Figure 5 Mean abundances of protozooplankton groups: heterotrophic nanoflagellates (A), tintinnids (B), naked ciliates (C), heterotrophic dinoflagellates (D), mixotrophic dinoflagellates (E), in the control (blue) and runoff treatment (gold). Heterotrophic nanoflagellates were monitored daily by flow cytometry whereas tintinnids, naked ciliates and heterotrophic and mixotrophic dinoflagellates were identified and counted through microscopic analysis on days 1, 2, 6, 9, 12, 16 and 18. The error bars represent the range of the observations. Daily abundances are represented in solid lines whereas data with a lower sampling frequency are represented in dotted lines.
3.5 Metazooplankton community
The initial community of metazooplankton was almost identical in the control and runoff treatments. It was dominated by mollusks, which mostly consisted of D-stage Bivalvia larvae (106.2 ± 13.8 individuals L−1) and nauplii of copepods (25.2 ± 5.4 individuals L−1). Appendicularia (19.3 ± 4.1 individuals L−1), rotifers (5.4 ± 1.6 individuals L−1) and larvae (mainly constituted of early-stage polychaete larvae 10.4 ± 2.0 individuals L−1) were also relatively abundant groups (Figure 6). Other metazooplankton groups such as Gastropoda, sea urchin, starfish, abalone, and adults and juvenile copepods of multiple genera, Acartia sp., Oncea sp., Clytemnestra sp., Microsetella sp., Euterpina sp., and Calanoida sp. were in much lower abundances. Since some organisms can actively avoid the Niskin sampling bottle, results on the abundance of metazooplankton should be taken cautiously, however the comparison of the metazooplankton abundances between treatments was possible as the sampling was identical in both treatments. By the end of the experiment, all metazooplankton groups’ abundances decreased by 59% and 36% in the control and runoff treatments compared to the start of the experiment, and there were no more appendicularians compared to the beginning of the experiment. However, in the terrestrial runoff treatment, the metazooplankton were 70% more abundant than in the control at the end of the experiment. More specifically, there were clearly more mollusks larvae, rotifers, and copepod nauplii, which were 98, 49, and 33% more abundant than in the control respectively.
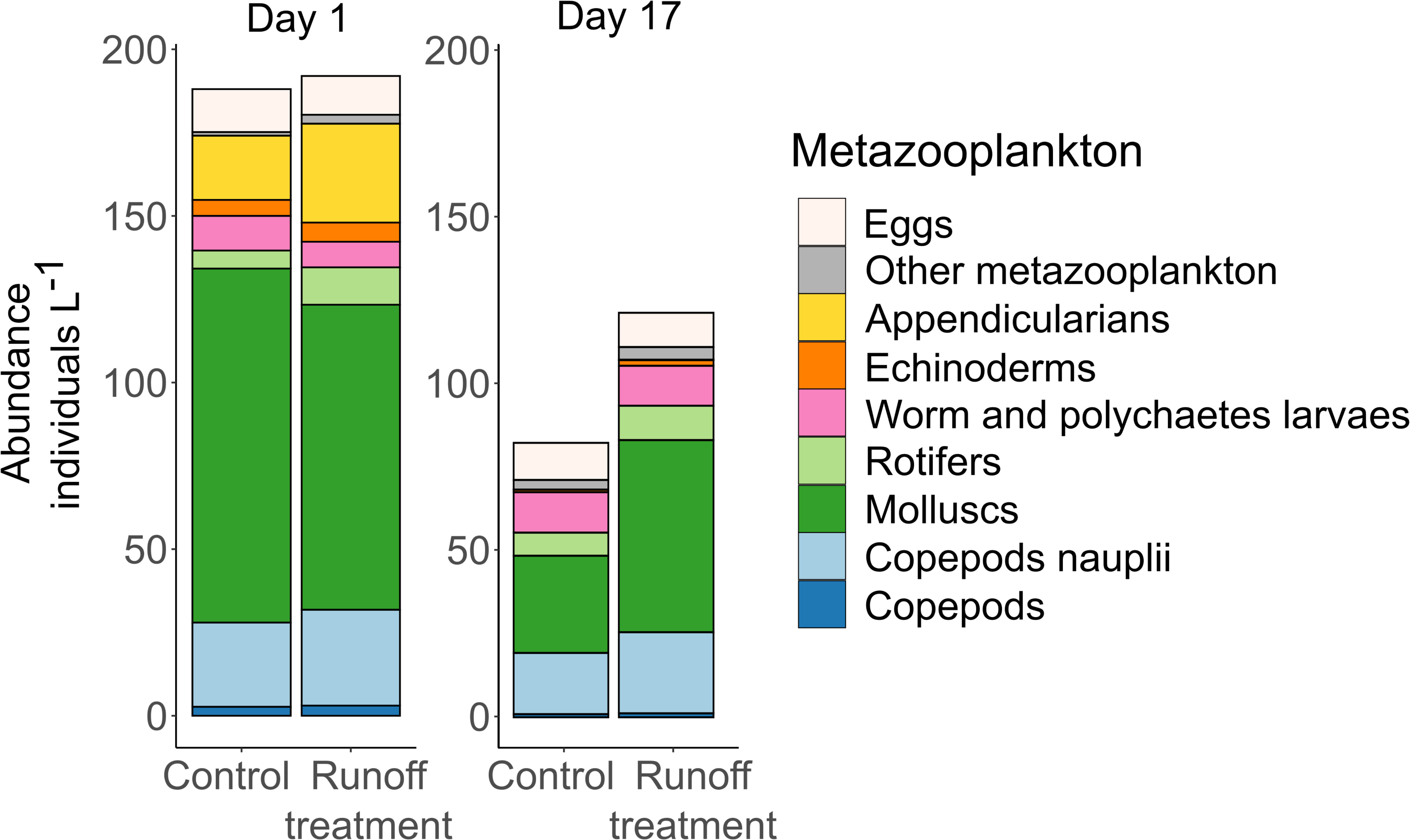
Figure 6 Histograms of the cumulative average metazooplankton groups abundance in the control and runoff treatment on days 1 and 17. Metazooplankton groups with very low abundances (<2 individuals L-1) were gathered into the “Other metazooplankton” category.
3.6 Effects of the runoff treatment on the microbial food web
The PCA analysis was conducted for the dataset from days 3 to 18. The first two dimensions of the PCA performed on the log response ratio represented respectively 35.7 and 24.7% of the variance of the dataset (Figure 7). The first dimension was positively represented by the DLI and Chl-a concentrations, which were broadly opposed to POC, PON, suspended matter, and DOC concentrations. The second dimension was well represented by bacterial and virus abundances on the positive scale, which were inversely related to the NH4+ concentrations.
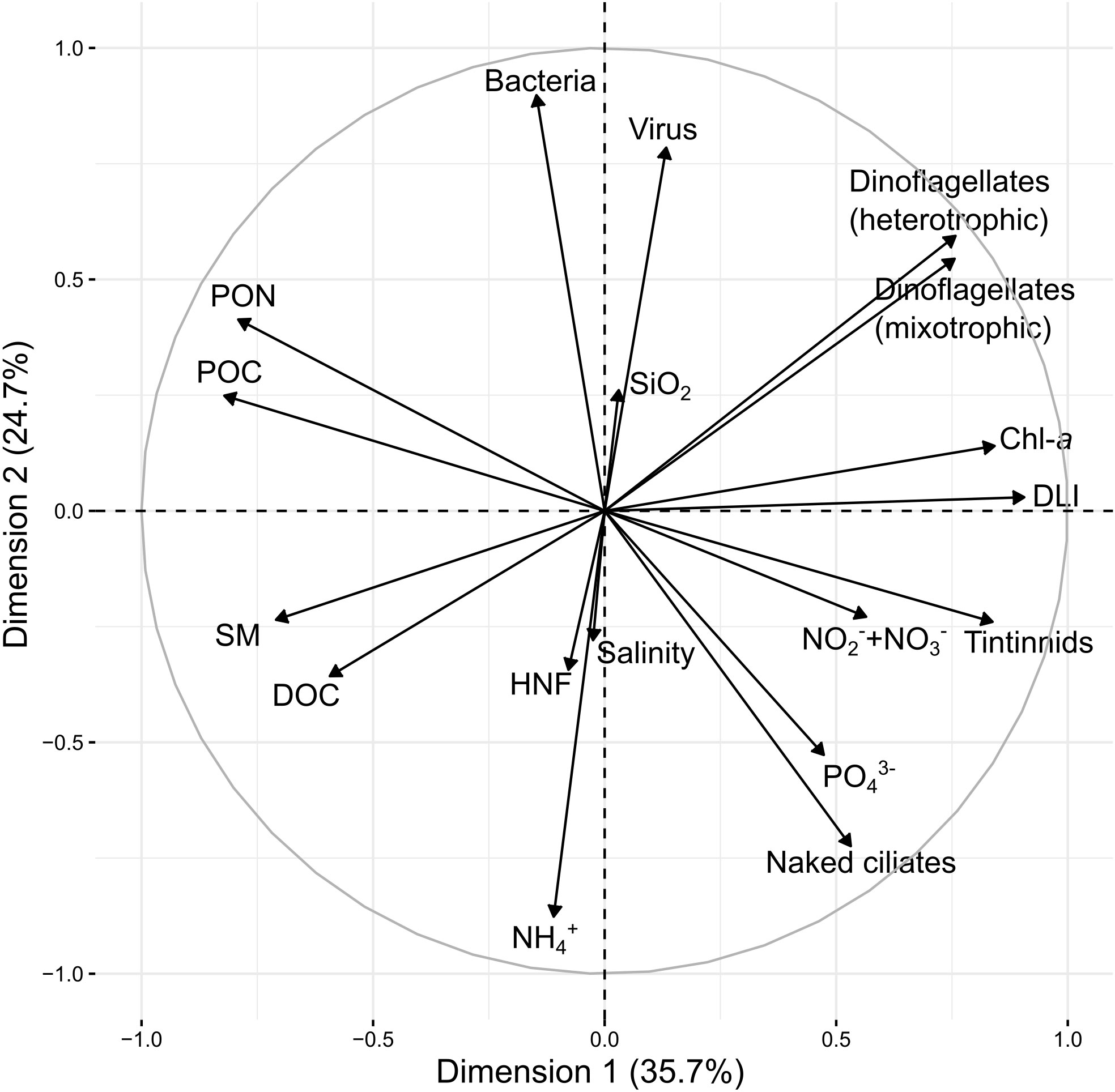
Figure 7 Principal component analyses (PCA) of the log response ratio of the physical, chemical, and biological data from the control and runoff treatment from days 3 to 18.
4 Discussion
4.1 Overall effects of the runoff treatment
In the present experiment, the addition of the forest soil maturated in the river water induced a short-term negative effect on phytoplankton followed by a mid-term positive one. The responses among the different phytoplankton groups were similar, whereas the responses of heterotrophic organisms varied quite differently from one group to another. Bacteria and small metazooplankton overall benefited from the runoff treatment, while heterotrophic protists, such as HNF and ciliates, were negatively affected. The forest soil added to the mesocosms to simulate terrestrial runoff was a clay-based forest soil, which is typical of the Mediterranean region’s oak forests (Allard et al., 2008). Compared to what has been measured in the Thau Lagoon after a natural terrestrial runoff, the concentrations of SM, PON, and DOC measured in the terrestrial runoff treatment after the addition of maturated soil were much lower (10, 1.6, and 2 times lower, respectively), whereas the POC concentration was 1.4 times higher (Fouilland et al., 2012).
4.2 The terrestrial runoff induced a rapid decline in phytoplankton community followed by a diatom bloom
As mentioned previously, the runoff treatment induced two distinct effects on the phytoplankton community over the course of the experiment. At first, phytoplankton was negatively affected by the runoff treatment. Both microscopy and flow cytometry analyses reflected the same trend for phytoplankton groups of different size classes and taxa, indicating that this negative effect was widespread among phytoplankton communities. As shown by the PCA analysis, high concentrations of POC, PON, and SM in the terrestrial runoff caused a decrease in light availability (by 51% on average in the first 12 days), which was the main driver correlated with the decrease in phytoplankton abundance and biomass during the experiment. These results were in agreement with previous studies that also reported a negative effect on the phytoplankton community related to a decrease in light availability following the addition of humic substances to the water or because of higher turbidity (Guadayol et al., 2009; Soulié et al., 2022). In addition to this decrease in light availability, the high quantity of particulate matter in the water column of the mesocosms could also have facilitated the flocculation and sedimentation of phytoplankton (Kiorboe et al., 1990) which could accentuate the negative effect of terrestrial runoff treatment on phytoplankton.
From days 12 to 17 of the experiment, phytoplankton, and especially diatoms, seemed to have acclimated to the terrestrial runoff conditions and beneficiated from it, as they formed a bloom. Diatoms certainly benefited from the increase in NH4+ and PO43− concentrations that occurred in the terrestrial runoff treatment in the middle period of the experiment, potentially because of bacterial remineralization of the added organic matter (Nixon, 1981) rather than from a direct enrichment. Diatoms could also have benefitted from the higher silicate concentrations in the runoff treatment, although no silicate drawdown was observed, even after day 12, when diatoms started to grow. This could indicate that silicate was taken up and released to the environment on a faster timescale than what the daily sampling could identify. Simultaneously, during this period (days 12–17), POC, PON, and SM concentrations were similar in both treatments, indicating that matter had been sedimented or solubilized (Goldthwait et al., 2005). The difference in DLI between the two treatments remained significant from days 12 to 18 (29% of decrease), but was less important than during the first 12 days of the experiment (51%), indicating that even though light availability increased over time in the terrestrial runoff treatment, diatoms were able to bloom under low light conditions. Previous studies similarly reported almost constant diatom blooms following terrestrial runoffs in Mediterranean waters due to higher nutrient concentrations (Spatharis et al., 2008; Pecqueur et al., 2011; Liess et al., 2016). Diatoms are fast-growing phytoplankton that respond rapidly to nutrient inputs from the land (Deininger et al., 2016), and can do so even under low light conditions regarding the present results, suggesting that diatoms are well adapted to a post-terrestrial runoff aquatic environment. Following the diatom bloom, the abundance of small phytoplankton such as picophytoplankton, cyanobacteria, and larger phytoplankton such as dinoflagellates started to increase in the present experiment, in accordance with in situ observations during natural terrestrial runoff in the Thau Lagoon (Pecqueur et al., 2011).
The increase in phytoplankton that occurred in the present study was lower and occurred later than that in the experiment of Deininger et al. (2016), which was performed at the same location. However, the protocol used by Deininger et al. (2016) to simulate terrestrial runoff treatment was quite different from ours and included the use of a resin that allows a better extraction of organic matter and nutrients (Lefébure et al., 2013). The processed soil they added to their mesocosms was therefore richer in DOC (3.6 mg C L–1 in their soil treatment) and induced a stronger positive effect on phytoplankton than that observed in the present study. In this regard, comparing studies that investigate the effects of terrestrial runoff, brownification, or discharges of terrestrial matter into an aquatic environment should be done cautiously, as the protocols to simulate terrestrial runoff can be quite different and therefore yield different and even contrasted results (Scharnweber et al., 2021). In the present study, we added to the marine water a mixture of soil and river water that was maturated naturally for two weeks to better mimic natural dragging of the soil along the river watershed (Müller et al., 2018). Therefore, the quantity of particulate and dissolved matter that was naturally extracted and added to the mesocosms might be different from chemically extracted or artificial humic substances (Scharnweber et al., 2021) but it is also the closest to a natural phenomenon.
4.3 Positive responses of bacteria to terrestrial runoff at the beginning and end of the experiment
The bacterial abundance responded to the runoff conditions in opposite ways as the experiment went by, as bacteria were first favoured (days 2-8), then depressed (days 9-14), before being finally favoured again by the end of the experiment (days 15-18). Simultaneously with the strong decrease in phytoplankton biomass at the beginning of the experiment, bacterial abundance was significantly higher in the terrestrial runoff treatment during the first eight days of the experiment. This result is in agreement with previous studies that reported a similar increase in bacterial abundance after terrestrial matter addition in mesocosms (Guadayol et al., 2009; Rasconi et al., 2015; Liess et al., 2016), and after a natural flood event (Pecqueur et al., 2011). In these later studies, the increase in bacteria was linked to the input of DOC-rich soil, but in the present experiment, there was no increase in the DOC concentrations in the runoff treatment 24 h after the addition of maturated soil. Similarly, in their study Liess et al. (2016) reported that after one day, all the DOC added through soil addition in their mesocosms was immediately consumed by bacteria, explaining why they did not measure any increase in DOC concentrations in their experiment. In the present study, DOC measurements were performed before the addition of maturated soil in the mesocosms (day 1) and 24 h after (day 2); therefore, it cannot be excluded that bacteria could have rapidly assimilated the DOC contained in the maturated soil. However, the gradual increase in bacterial abundance in the terrestrial runoff treatment until day 8, coupled with the continuous decrease in POC and PON concentrations during this period, suggests a solubilization of POC into DOC, which could then have been rapidly taken up by bacteria (Zweifel et al., 1993). This increase in bacterial abundance that benefited from the input of terrestrial organic matter, coinciding with the decrease in phytoplankton caused by the lower light availability linked to the same matter input, typically highlights how terrestrial runoff can immediately decouple the two communities (Berglund et al., 2007; Wikner and Andersson, 2012).
Subsequently, bacterial abundance decreased from days 9 to 14 in the runoff treatment. At the same time, the particulate matter reached concentrations similar to those in the control during this period, having been solubilized and/or sedimented. Therefore, it is possible that there was not enough bioavailable organic matter to support the increase in bacterial abundance during this period. As phytoplankton were less abundant in the runoff treatment, bacteria would not have benefited from phytoplankton DOC release, indicating that the decoupling between phytoplankton and bacteria (Berglund et al., 2007) was prolonged during this period. However, the increase in PO43– concentrations in the runoff treatment concomitantly with the decrease in bacterial abundance suggested a potential intracellular nutrient release from bacterial grazing or cell lysis (Pomeroy, 1974; Bratbak et al., 1994). This nutrient release concurrent with the decrease in bacterial abundance during this period could be due to grazing mortality from Bivalvia larvae (Arapov et al., 2010; Marshall et al., 2010) and copepod nauplii (Roff et al., 1995; Turner, 2004) as they were positively affected by the terrestrial runoff treatment, whereas protozooplankton and viruses, other potential factors of mortality for bacteria (Azam et al., 1983; Sherr & Sherr, 1988; Bratbak et al., 1994; Baudoux et al., 2008), were clearly negatively affected by the terrestrial runoff treatment during this period. Indeed, while virus abundances followed a similar trend in both treatments, they were significantly lower in the runoff treatment compared to the control.
By the end of the experiment, bacterial abundance increased again in the runoff treatment, concomitantly with the increase in phytoplankton abundance and biomass, which could indicate a recoupling between these two communities, with phytoplankton exudating DOC that bacteria could use and bacteria providing remineralized inorganic nutrients to phytoplankton (Kirchman, 1994).
4.4 Terrestrial runoff strengthened the role of metazooplankton at the expense of mixotrophic and heterotrophic protozooplankton as a link to higher trophic levels
Numerous studies reported that mixotrophs generally benefit from humic-matter-rich water (Bergström et al., 2003; Calderini et al., 2022) or sediment addition (Wilken et al., 2018). In the present study, the genus or species of dinoflagellates that are described as mixotrophic (Kraberg et al., 2010) did not significantly benefit from the addition of the maturated soil, although they showed higher abundances on days 2 and 18 in the runoff treatment compared to the control (Figure 5E). Similarly, in a study by Liess et al. (2016), the authors reported that during their mesocosm experiment, the addition of soil benefited more diatoms than mixotrophs in the Thau Lagoon. These results suggest that in the Thau Lagoon, mixotrophs are not likely to be more competitive than the strictly autotrophic plankton community (notably diatoms), which displays a rather fast adaptation to the changes in light and nutrients after a terrestrial runoff. The protozooplankton abundances of heterotrophic nanoflagellates, dinoflagellates, naked ciliates, and tintinnids were negatively affected by the terrestrial runoff treatment. These negative effects can be explained by both bottom-up and top-down controls. On one hand, the decrease in phytoplankton abundance could have negatively affected protozooplankton through a limitation in their prey abundance. Additionally, non-living particles contained in the maturated soil could have affected protozooplankton predation (Sommaruga and Kandolf, 2015). A similar effect was observed during an experiment in an alpine lake, where mineral particles induced a negative effect on HNF predation on bacteria (Sommaruga and Kandolf, 2015). On the other hand, as metazooplankton abundance was also higher in the terrestrial runoff treatment than in the control by the end of the experiment, it is possible that protozooplankton were under higher grazing pressure from some groups of metazooplankton, such as filter feeding mollusc larvae or copepod nauplii (Turner, 2004; Arapov et al., 2010; Marshall et al., 2010). It must be noted that metazooplankton abundance was lower at the end of the experiment compared to the beginning in both treatments, possibly suffering from the confinement in the mesocosm bags. The negative effect of terrestrial runoff treatment on protozooplankton was simultaneous with the positive effect on metazooplankton, indicating that the structure of the plankton food web might have been greatly affected by this treatment. Because both protozooplankton and mixotrophic organisms were negatively affected by the runoff treatment, metazooplankton may have replaced their role for carbon biomass transfer within the plankton food web instead. The metazooplankton composition was dominated by molluscs larvae, copepod nauplii, and rotifers, which are able to feed on a wide range of prey sizes (Rougier et al., 2000; Turner, 2004; Arapov et al., 2010; Marshall et al., 2010) and could therefore participate to the transfer of biomass at different trophic levels. Since some zooplankton can also directly consume terrigenous organic matter (Carpenter et al., 2005; Cole et al., 2006; Cole et al., 2011), the higher metazooplankton abundances observed by the end of the experiment in the terrestrial runoff treatment also suggest that metazooplankton could directly transfer terrestrial carbon biomass to higher trophic levels in the plankton food web during a terrestrial runoff in Thau lagoon. This also implies that the transfer of carbon biomass would be more efficient after a terrestrial runoff, with less trophic levels than in a classical plankton food web (Sommer et al., 2002; Lefébure et al., 2013) and with a main transfer pathway from bacteria and/or phytoplankton directly to metazooplankton without passing through the intermediate level of protozooplankton.
4.5 Conclusion
The main result observed in the present study was that the effects of the simulated terrestrial runoff spread along the plankton food web, significantly affecting all trophic levels of the natural plankton community studied. This occurred in a relatively short time considering that the experiment lasted less than three weeks. The lower light availability in the terrestrial runoff treatment compared to the control resulted in a net decrease of approximately one-third of Chl-a concentration and phytoplankton abundance over the entire experiment. Even though higher remineralized nutrient concentrations in the runoff treatment induced a bloom of diatoms afterward, this was not enough to counterbalance the depression of phytoplankton during the first half of the experiment. Simultaneously, bacteria generally benefited from the addition of maturated soil and were, overall, 15% more abundant in the runoff treatment. Despite these changes in the food source at the base of the food web, represented by the phytoplankton/bacteria ratio, it appears that the terrestrial runoff treatment favoured metazooplankton rather than protozooplankton, resulting hypothetically in a more efficient channelling of the biomass through the food web via small metazooplankton, while subsiding intermediate trophic levels such as protozooplankton.
Data availability statement
The raw data supporting the conclusions of this article will be made available by the authors, without undue reservation.
Author contributions
FV and BM designed experiments. All co-authors participated in the experiment and produced the datasets that were integrated into the study. JC wrote the original draft with the input of all co-authors. All authors contributed to the article and approved the submitted version.
Funding
This study was part of the RESTORE project funded by the French National Research Agency (contract no. ANR-19-CE32-0013). It was also opened to transnational access through the AQUACOSM-plus European project (grant agreement: 871081).
Acknowledgments
We would like to thank the MEDIMEER crew for their crucial implications in the experimental set up, sampling, and analysis, and the students who participated in the sampling and analysis during the experiment. We also thank Florian Voron for the nutrient analysis and Barbara Marie for the DOC analysis. Lastly, we would like to thank David Pecqueur and Christophe Salmeron from the SU/CNRS BioPIC Imaging and Cytometry platform of Banyuls/M Oceanologic Observatory and the technical support of EMBRC-France, whose French state funds are managed by the ANR within the Investments of the Future program under reference ANR-10-INBS-02. Microscopy analysis were done with the support of LabEx CeMEB funded by the ANR-10-LABX-04-01. Lastly, we would like to thank the reviewers for their meaningful comments, that helped improve the quality of the manuscript.
Conflict of interest
The authors declare that the research was conducted in the absence of any commercial or financial relationships that could be construed as a potential conflict of interest.
Publisher’s note
All claims expressed in this article are solely those of the authors and do not necessarily represent those of their affiliated organizations, or those of the publisher, the editors and the reviewers. Any product that may be evaluated in this article, or claim that may be made by its manufacturer, is not guaranteed or endorsed by the publisher.
References
Allard V., Ourcival J. M., Rambal S., Joffre R., Rocheteau A. (2008). Seasonal and annual variation of carbon exchange in an evergreen Mediterranean forest in southern France. Glob Chang Biol. 14, 714–725. doi: 10.1111/j.1365-2486.2008.01539.x
Alpert P., Ben-Gai T., Baharad A., Benjamini Y., Yekutieli D., Colacina M., et al. (2002). The paradoxical increase of Mediterranean extreme daily rainfall in spite of decrease in total values. Geophys Res. Lett. 29, 1536. doi: 10.1029/2001GL013554
Arapov J., Ezgeta-Balic D., Peharda M., Gladan Z. N. (2010). Bivalve feeding — How and what they eat? Ribarstvo 68, 105–116.
Ask J., Karlsson J., Persson L., Ask P., Byström P., Jansson M. (2009). Terrestrial organic matter and light penetration: Effects on bacterial and primary production in lakes. Limnol Oceanogr 54, 2034–2040. doi: 10.4319/lo.2009.54.6.2034
Azam F., Fenchel T., Field J. G., Gray J. S., Meyer-Reil L. A., Thingstad T. (1983). The ecological role of water-column microbes in the sea. Mar. Ecol. Prog. Ser. 10, 257–263. doi: 10.3354/meps010257
Baudoux A. C., Veldhuis M. J. W., Noordeloos A. A. M., Van Noort G., Brussaard C. P. D. (2008). Estimates of virus- vs. grazing induced mortality of picophytoplankton in the North Sea during summer. Aquat. Microbial Ecol. 52 (1), 69–82. doi: 10.3354/ame01207
Benner R., Strom M. (1993). A critical evaluation of the analytical blank associated with DOC measurements by high-temperature catalytic oxidation. Mar. Chem. 41, 153–160. doi: 10.1016/0304-4203(93)90113-3
Berglund J., Müren U., Båmstedt U., Andersson A. (2007). Efficiency of a phytoplankton-based and a bacterial-based food web in a pelagic marine system. Limnol Oceanogr 52, 121–131. doi: 10.4319/lo.2007.52.1.0121
Bergström A. K., Jansson M., Drakare S., Blomqvist P. (2003). Occurrence of mixotrophic flagellates in relation to bacterioplankton production, light regime and availability of inorganic nutrients in unproductive lakes with differing humic contents. Freshw. Biol. 48, 868–877. doi: 10.1046/j.1365-2427.2003.01061.x
Bratbak G., Thingstad F., Heldal M. (1994). Viruses and the microbial loop. Microb. Ecol. 28, 209–221. doi: 10.1007/BF00166811
Brett M. T., Bunn S. E., Chandra S., Galloway A. W.E., Guo F., Kainz M. J., et al. (2017). How important are terrestrial organic carbon inputs for secondary production in freshwater ecosystems? Freshw. Biol. 62, 833–853. doi: 10.1111/fwb.12909
Brussaard C. P. D. (2004). Optimization of procedures for counting viruses by flow cytometry. Appl. Environ. Microbiol. 70, 1506–1513. doi: 10.1128/AEM.70.3.1506-1513.2004
Calderini M. L., Salmi P., Rigaud C., Peltomaa E., Taipale S. J. (2022). Metabolic plasticity of mixotrophic algae is key for their persistence in browning environments. Mol. Ecol. 31, 4726–4738. doi: 10.1111/mec.16619
Carpenter S. R., Cole J. J., Pace M. L., Van de Bogert M., Bade D. L., Bastviken D., et al. (2005). Ecosystem subsidies: Terrestrial support of aquatic food webs from 13 C addition to contrasting lakes. Ecology 86, 2737–2750. doi: 10.1890/04-1282
Christaki U., Courties C., Massana R., Catala P., Lebaron P., Gasol J. M., et al. (2011). Optimized routine flow cytometric enumeration of heterotrophic flagellates using SYBR Green I. Limnol Oceanogr Methods 9, 329–339. doi: 10.4319/lom.2011.9.329
Cole J. J., Carpenter S. R., Kitchell J., Pace M. L., Solomon C. T., Weidel B. (2011). Strong evidence for terrestrial support of zooplankton in small lakes based on stable isotopes of carbon, nitrogen, and hydrogen. Proc. Natl. Acad. Sci. 108, 1975–1980. doi: 10.1073/pnas.1012807108
Cole J. J., Carpenter S. R., Pace M. L., Van de Bogert M. C., Kitchell J. L., Hodgson J. R. (2006). Differential support of lake food webs by three types of terrestrial organic carbon. Ecol. Lett. 9, 558–568. doi: 10.1111/j.1461-0248.2006.00898.x
Deininger A., Faithfull C. L., Lange K., Bayer T., Vidussi F., Liess A. (2016). Simulated terrestrial runoff triggered a phytoplankton succession and changed seston stoichiometry in coastal lagoon mesocosms. Mar. Environ. Res. 119, 40–50. doi: 10.1016/j.marenvres.2016.05.001
Fouilland E., Trottet A., Bancon-Montigny C., Bouvy M., Le Floc’h E., Gonzalez J. L., et al. (2012). Impact of a river flash flood on microbial carbon and nitrogen production in a Mediterranean Lagoon (Thau Lagoon, France). Estuar. Coast. Shelf Sci. 113, 192–204. doi: 10.1016/j.ecss.2012.08.004
Goldthwait S., Carlson C., Henderson G., Alldredge A. (2005). “Effects of physical fragmentation on remineralization of marine snow,” in Marine ecology progress series, vol. 305. , 59–65. doi: 10.3354/meps305059
Guadayol Ò., Peters F., Marrasé C., Gasol J., Roldán C., Berdalet E., et al. (2009). Episodic meteorological and nutrient-load events as drivers of coastal planktonic ecosystem dynamics: a time-series analysis. Mar. Ecol. Prog. Ser. 381, 139–155. doi: 10.3354/meps07939
Holmes R. M., Aminot A., Kérouel R., Hooker B. A., Peterson B. J. (1999). A simple and precise method for measuring ammonium in marine and freshwater ecosystems. Can. J. Fish. Aq. Sci. 56 (10), 1801–1808. doi: 10.1139/f99-128
Kiorboe T., Andersen K. P., Dam H. G. (1990). Coagulation efficiency and aggregate formation in marine phytoplankton. Mar. Biol. 107, 235–245. doi: 10.1007/BF01319822
Kirchman D. L. (1994). The uptake of inorganic nutrients by heterotrophic bacteria. Microbial Ecol. 28 (2), 255–271. doi: 10.1007/BF00166816
Kraberg A., Baumann M., Dürselen C. (2010). Coastal phytoplank- ton: Photo guide for Northern Europena Seas (München: Pfeil Verlag), 204.
Lefébure R., Degerman R., Andersson A., Larsson S., Eriksson L. O., Båmstedt U., et al. (2013). Impacts of elevated terrestrial nutrient loads and temperature on pelagic food-web efficiency and fish production. Glob Chang Biol. 19, 1358–1372. doi: 10.1111/gcb.12134
Liess A., Faithfull C., Reichstein B., Rowe O., Guo J., Pete R., et al. (2015). Terrestrial runoff may reduce microbenthic net community productivity by increasing turbidity: a Mediterranean coastal lagoon mesocosm experiment. Hydrobiologia 753, 205–218. doi: 10.1007/s10750-015-2207-3
Liess A., Rowe O., Francoeur S. N., Guo J., Lange K., Schröder A., et al. (2016). Terrestrial runoff boosts phytoplankton in a Mediterranean coastal lagoon, but these effects do not propagate to higher trophic levels. Hydrobiologia 766, 275–291. doi: 10.1007/s10750-015-2461-4
Marie D., Partensky F., Jacquet S., Vaulot D. (1997). Enumeration and cell cycle analysis of natural populations of marine picoplankton by flow cytometry using the nucleic acid stain SYBR green I. Appl. Environ. Microbiol. 63, 186–193. doi: 10.1128/AEM.63.1.186-193.1997
Marshall R., McKinley S., Pearce C. M. (2010). Effects of nutrition on larval growth and survival in bivalves. Rev. Aquac 2, 33–55. doi: 10.1111/j.1753-5131.2010.01022.x
Meunier C. L., Liess A., Andersson A., Brugel S., Paczkowska J., Rahman H., et al. (2017). Allochthonous carbon is a major driver of the microbial food web – A mesocosm study simulating elevated terrestrial matter runoff. Mar. Environ. Res. 129, 236–244. doi: 10.1016/j.marenvres.2017.06.008
Mostajir B., Roques C., Bouvier C., Bouvier T., Fouilland É., Got P., et al (2015). Microbial food web structural and functional responses to oyster and fish as top predators. Mar. Ecol. Prog. Ser. 515, 11–27. doi: 10.3354/meps11429
Müller O., Seuthe L., Bratbak G., Paulsen M. L. (2018). Bacterial response to permafrost derived organic matter input in an Arctic Fjord. Front. Mar. Sci. 5. doi: 10.3389/fmars.2018.00263
Nixon S. W. (1981). “Remineralization and nutrient cycling in coastal marine ecosystems,” in Estuaries and nutrients (Totowa, NJ: Humana Press), 111–138.
Nunes J. P., Seixas J., Keizer J. J., Ferreira A. J. D. (2009). Sensitivity of runoff and soil erosion to climate change in two Mediterranean watersheds. Part II: assessing impacts from changes in storm rainfall, soil moisture and vegetation cover. Hydrol Process 23, 1212–1220. doi: 10.1002/hyp.7250
Pecqueur D., Vidussi F., Fouilland E., Le Floc’h E., Mas S., Roques C., et al. (2011). Dynamics of microbial planktonic food web components during a river flash flood in a Mediterranean coastal lagoon. Hydrobiologia 673, 13–27. doi: 10.1007/s10750-011-0745-x
Pomeroy L. R. (1974). The ocean’s food web, A changing paradigm. Bioscience 24, 499–504. doi: 10.2307/1296885
Rambal S., Joffre R., Ourcival J. M., Cavender-Bares J., Rocheteau A. (2004). The growth respiration component in eddy CO2 flux from a Quercus ilex mediterranean forest. Glob Chang Biol. 10, 1460–1469. doi: 10.1111/j.1365-2486.2004.00819.x
Rasconi S., Gall A., Winter K., Kainz M. J. (2015). Increasing water temperature triggers dominance of small freshwater plankton. PloS One 10, e0140449. doi: 10.1371/journal.pone.0140449
Robinson C. (2008). Heterotrophic bacterial respiration. In: microbial ecology of the oceans (Hoboken, NJ, USA: John Wiley & Sons, Inc.), 299–334.
Roff J., Turner J., Webber M., Hopcroft R. (1995). Bacterivory by tropical copepod nauplii: extent and possible significance. Aquat Microb. Ecol. 9, 165–175. doi: 10.3354/ame009165
Rougier C., Pourriot R., Lam-Hoai T. (2000). The genus Synchaeta ( rotifers ) in a north-western Mediterranean coastal lagoon ( Etang de Thau , France ): taxonomical and ecological remarks. Hydrobiologia 436 (1-3), 105–117. doi: 10.1023/A:1026579212772
Sánchez E., Gallardo C., Gaertner M. A., Arribas A., Castro M. (2004). Future climate extreme events in the Mediterranean simulated by a regional climate model: a first approach. Glob Planet Change 44, 163–180. doi: 10.1016/j.gloplacha.2004.06.010
Scharnweber K., Peura S., Attermeyer K., Bertilsson S., Bolender L., Buck M., et al. (2021). Comprehensive analysis of chemical and biological problems associated with browning agents used in aquatic studies. Limnol Oceanogr Methods 19, 818–835. doi: 10.1002/lom3.10463
Sherr E., Sherr B. (1988). Role of microbes in pelagic food webs: A revised concept. Limnology Oceanography 33 (5), 1225–1227. doi: 10.4319/lo.1988.33.5.1225
Souchu P., Bec B., Smith V. H., Laugier T., Fiandrino A., Benau L., et al. (2010). Patterns in nutrient limitation and chlorophyll a along an anthropogenic eutrophication gradient in French Mediterranean coastal lagoons. Canadian Journal of Fisheries and Aquatic Sciences 67 (4), 743–753. doi: 10.1139/F10-018
Sommaruga R., Kandolf G. (2015). Negative consequences of glacial turbidity for the survival of freshwater planktonic heterotrophic flagellates. Sci. Rep. 4, 4113. doi: 10.1038/srep04113
Sommer U., Stibor H., Katechakis A., Sommer F., Hansen T. (2002). Pelagic food web configurations at different levels of nutrient richness and their implications for the ratio fish production:primary production. Hydrobiologia 484, 11–20. doi: 10.1023/A:1021340601986
Soulié T., Stibor H., Mas S., Braun B., Knechtel J., Nejstgaard J. C., et al. (2022). Brownification reduces oxygen gross primary production and community respiration and changes the phytoplankton community composition: An in situ mesocosm experiment with high-frequency sensor measurements in a North Atlantic bay. Limnol Oceanogr 67, 874–887. doi: 10.1002/lno.12041
Spatharis S., Mouillot D., Danielidis D. B., Karydis M., Chi T. D., Tsirtsis G., et al. (2008). Influence of terrestrial runoff on phytoplankton species richness-biomass relationships: A double stress hypothesis. J. Exp. Mar. Bio Ecol. 362, 55–62. doi: 10.1016/j.jembe.2008.06.003
Trégouboff G., Rose M. (1957a). Manuel de planctonologie méditerranéenne Vol. I (Paris: Centre National de la Recherche Scientifique).
Trégouboff G., Rose M. (1957b). Manuel de planctonologie méditerranéenne Vol. II (Paris: Centre National de la Recherche Scientifique).
Trombetta T., Vidussi F., Mas S., Parin D., Simier M., Mostajir B. (2019). Water temperature drives phytoplankton blooms in coastal waters. PloS One 14 (4), e0214933. doi: 10.1371/journal.pone.0214933
Turner J. T. (2004). The importance of small planktonic copepods and their roles in pelagic marine food webs. Zool Stud. 43, 255–266.
Vidussi F., Mostajir B., Fouilland E., Le Floc'H E., Nouguier J., Roques C. (2011). Effects of experimental warming and increased ultraviolet B radiation on the Mediterranean plankton food web. Limnol. Oceanogr. 56, 216–218. doi: 10.4319/lo.2011.56.1.0206
Wikner J., Andersson A. (2012). Increased freshwater discharge shifts the trophic balance in the coastal zone of the northern Baltic Sea. Glob Chang Biol. 18, 2509–2519. doi: 10.1111/j.1365-2486.2012.02718.x
Wilken S., Soares M., Urrutia-Cordero P., Ratcovich J., Ekvall M. K., Van Donk E., et al. (2018). Primary producers or consumers? Increasing phytoplankton bacterivory along a gradient of lake warming and browning. Limnol Oceanogr 63, S142–S155. doi: 10.1002/lno.10728
Zapata M., Rodríguez F., Garrido J. (2000). Separation of chlorophylls and carotenoids from marine phytoplankton:a new HPLC method using a reversed phase C8 column and pyridine-containing mobile phases. Mar. Ecol. Prog. Ser. 195, 29–45. doi: 10.3354/meps195029
Keywords: terrestrial runoff, phytoplankton, bacteria, virus, zooplankton, in situ mesocosm, Thau Lagoon, food web
Citation: Courboulès J, Vidussi F, Soulié T, Nikiforakis E, Heydon M, Mas S, Joux F and Mostajir B (2023) Effects of an experimental terrestrial runoff on the components of the plankton food web in a Mediterranean coastal lagoon. Front. Mar. Sci. 10:1200757. doi: 10.3389/fmars.2023.1200757
Received: 05 April 2023; Accepted: 18 September 2023;
Published: 02 October 2023.
Edited by:
Michelle Jillian Devlin, Fisheries and Aquaculture Science (CEFAS), United KingdomReviewed by:
Gayantonia Franzè, Norwegian Institute of Marine Research (IMR), NorwayJuris Aigars, Latvian Institute of Aquatic Ecology, Latvia
Copyright © 2023 Courboulès, Vidussi, Soulié, Nikiforakis, Heydon, Mas, Joux and Mostajir. This is an open-access article distributed under the terms of the Creative Commons Attribution License (CC BY). The use, distribution or reproduction in other forums is permitted, provided the original author(s) and the copyright owner(s) are credited and that the original publication in this journal is cited, in accordance with accepted academic practice. No use, distribution or reproduction is permitted which does not comply with these terms.
*Correspondence: Justine Courboulès, anVzdGluZS5jb3VyYm91bGVzQGhvdG1haWwuZnI=; Behzad Mostajir, YmVoemFkLm1vc3RhamlyQHVtb250cGVsbGllci5mcg==