- 1Faculty of Science and Engineering, National Marine Science Centre, Southern Cross University, Coffs Harbour, NSW, Australia
- 2Fisheries Research, New South Wales (NSW) Department of Primary Industries, National Marine Science Centre, Coffs Harbour, NSW, Australia
- 3The University of Western Australia (UWA) Oceans Institute & School of Biological Sciences, University of Western Australia, Perth, WA, Australia
- 4Flødevigen Research Station, Institute of Marine Research, His, Norway
- 5New South Wales Department of Primary Industries, Wollongbar Primary Industries Institute, Wollongbar, NSW, Australia
Ocean warming and short-term extreme events (e.g. marine heatwaves) are becoming more intense and frequent and have had major impacts on ecosystems. Seaweeds are foundational components of temperate reefs, providing nutrition for a diversity of species and underpinning temperate food webs. While the impacts of climate-driven environmental change on seaweed distribution, abundance and interactions are well studied, potential impacts on the provision of nutrients from seaweeds and their availability to consumers remain poorly understood. Here, we use metabolomic and lipidomic approaches to understand how the provision of nutrients from key seaweeds may be altered under climate change. We optimize a small-scale microextraction technique to test the effects of warming and marine heatwaves on the nutritional quality of two Australian habitat-forming seaweeds; Ecklonia and Sargassum. We then model changes in lipid availability in response to climate-driven ocean warming throughout Ecklonia’s eastern Australian distribution. Contrary to expectations, ocean warming and marine heatwaves had limited effects on seaweed nutritional quality, indicating resilience in the nutritional value of these species to climate-driven warming. However, nutritional quality varied significantly between species, which presents new implications for predicted species redistributions from the base of the food chain. Modelling revealed a projected net loss of 3.5% of lipids across the east coast of Australia by 2100 under RCP 8.5 in response to shifts in the redistribution of Ecklonia biomass. The climate-driven redistribution of seaweeds is likely to alter the availability of seaweed-derived nutrients to consumers, which may have broad implications for the transfer of energy within temperate marine food webs.
1 Introduction
Primary producers, such as seaweeds, are foundational components of temperate coasts providing habitat and nutrition that underpins diverse ecosystems (Christie et al., 2009; Bennett et al., 2015; Teagle et al., 2017). Seaweeds are threatened globally by ocean warming and marine heatwaves, which cause shifts in distributions and restructure temperate ecosystems (Vergés et al., 2014; Krumhansl et al., 2016; Smale, 2020; Michaud et al., 2022). Many studies suggest that continuous and discrete warming could have substantial effects on seaweed morphology (Geppi and Riera, 2022), physiology (Van Den Hoek, 1982; Wernberg et al., 2013a; Román et al., 2020), phenology (Coleman and Brawley, 2005; de Bettignies et al., 2018), abundance (Davis et al., 2021), distribution (Lima et al., 2007; Wernberg et al., 2011; Casado-Amezúa et al., 2019) and genetics (Coleman et al., 2020b; Gurgel et al., 2020). Such changes may have subsequent effects to seaweed consumers that perpetuate throughout the entire food chain (Parrish, 2009; Lozano-Montes et al., 2011; Jin et al., 2020). Despite their importance to temperate marine food webs, limited research has investigated the effects of climate change on the small bioactive compounds that contribute to seaweed nutritional quality (e.g. metabolites, but see Park et al., 2023).
Seaweeds contribute to the health of marine life through the provision of essential nutrients, such as essential fatty acids (McCauley et al., 2015; Skrzypczyk et al., 2019), antioxidants, vitamins and minerals (MacArtain et al., 2007; Wells et al., 2017). Whilst these essential nutrients form a minor component of a seaweeds composition, they have a disproportional importance in nutrition due to intrinsic bioactive properties (Lopes et al., 2020). It remains poorly understood, however, whether climate-driven environmental change will impact these nutrients in seaweeds and thus their availability to seaweed consumers. Reductions to the nutritional quality of seaweeds (e.g. lower levels of essential fatty acids) are likely to affect the trophic transfer of nutrients within marine ecosystems (Parrish, 2009; Lozano-Montes et al., 2011; Jin et al., 2020), potentially compromising reproduction in marine invertebrates and fish (Litzow et al., 2006), with potential flow on effects to higher tropic levels (Figure 1; Wanless et al., 2005). Given the implications for consumers and the subsequent trophic transfer of energy within marine food webs, understanding the impacts of climate change on the nutritional quality of seaweeds is an important research frontier. The application of molecular techniques, such as metabolomics and lipidomics, presents a new pathway to assess such impacts but have not yet been widely employed to understand the change in nutritional composition of seaweeds.
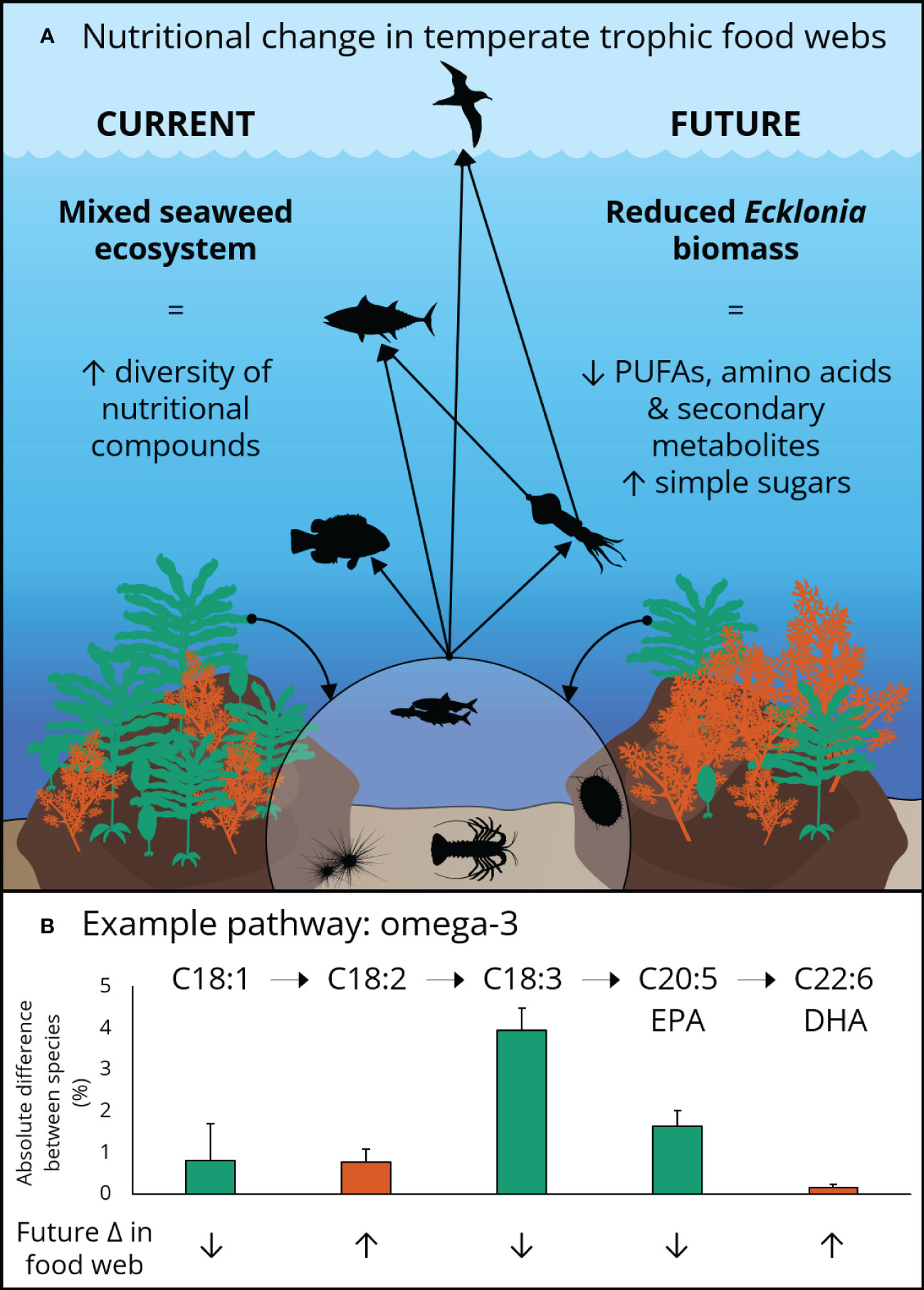
Figure 1 A representative temperate food web and biosynthetic pathway illustrating: (A) the potential trophic transfer of nutrients derived from Ecklonia (green) and Sargassum (orange) to higher trophic levels under current and future scenarios. (B) The biosynthetic pathway of omega-3 fatty acids EPA and DHA illustrating potential future changes to temperate food webs. The bars indicate the absolute difference in percent content in each fatty acid by species (shown by colour). Greater total levels of omega-3 fatty acids in Ecklonia, relative to Sargassum, indicate a likely decrease in availability at low latitude temperate marine ecosystems under future ocean conditions in east Australia.
Lipid- and metabolomics methods can be applied to quantify nutritionally valuable compounds in seaweed (e.g. omega-3 fatty acids, sugars and amino acids; Kumar et al., 2016; Britton et al., 2021; Choudhary et al., 2021). These compounds function as building blocks and end products of cellular processes in seaweeds and have beneficial properties for both seaweeds and their consumers. Differences in the composition of these compounds among species and in response to environmental change can provide insights into the health, nutritional quality and physiology of seaweeds (Britton et al., 2020; Ghaderiardakani et al., 2022; Park et al., 2023). The new application of molecular techniques to seaweeds is improving our understanding of biological responses to ocean warming and marine heatwaves, including in the concentration of key nutritional compounds. For example, Britton et al. (2020) found that exposure to marine heatwaves and future ocean conditions led to the saturation of fatty acids in the macroalga Phyllospora comosa. However, considerable scope remains to expand our understanding of biological responses to environmental change using molecular techniques. For instance, combining nutritional responses derived through molecular methods to model the condition and quality of marine species in response to climate change (Bolin et al., 2021) presents a valuable opportunity to understand likely future implications across marine food webs.
Extreme events such as marine heatwaves have had major impacts on the cooccurring temperate seaweeds, Ecklonia radiata and Sargassum linearifolium, throughout their Australian distributions (Vergés et al., 2016; Straub et al., 2019; Gurgel et al., 2020; Wernberg, 2021). Combined with Phyllospora comosa, our study species, Ecklonia radiata and S. linearifolium are the dominant habitat forming species found on subtidal rocky reefs, throughout New South Wales, Australia (Layton et al., 2020). Within the region and throughout Australia’s Great Southern Reef, these species provide essential ecosystem services (including food and habitat) supporting diverse marine communities (Coleman and Wernberg, 2017; Wernberg et al., 2019). As primary producers, these seaweeds are at the base of the food chain supporting species such as abalone, sea urchins and many species of fish, which in turn are a vital food source for larger marine species (Figure 1A; Vanderklift and Wernberg, 2010; Provost et al., 2017; Miranda et al., 2019). A better understanding of how the nutritional quality of these primary producers will be affected by climate change is vital to anticipate and manage changes throughout the temperate Australian marine environment, including the ecological and economic values it supports.
Here, we test the effects of ocean warming and MHWs on the nutritional quality of two of the most dominant seaweed species on Australian temperate reefs, E. radiata, and S. linearifolium. Specifically, we (1) tested the effects of warming and different types of MHWs on seaweed nutritional quality and the ability of these parameters to recover from impacts, (2) compared the nutritional quality of the two species’, and (3) estimated climate-driven shifts in Ecklonia lipid availability along Australia’s east coast by integrating our results with projections of the redistribution of biomass for this species under multiple climate change scenarios.
2 Materials and methods
2.1 Experimental setup and treatment conditions
Individual whole plants of Ecklonia radiata and Sargassum linearifolium (hereafter ‘Ecklonia’ and ‘Sargassum’), were collected by SCUBA in Sydney, Australia (34°03’59.0”S 151°09’44.3”E) under NSW DPI permits (P01/0059(A)-3.0 and OUT17/7525). Carefully keeping the holdfasts intact, 150 whole juvenile plants (< 30cm total length) were collected per species from a shallow subtidal rocky reef between 3 to 6m depth. Within 4h of collection, the plants were transported (by air) to the National Marine Science Centre in Coffs Harbour, Australia and placed in 1000 L mesocosms with flowing seawater, weighed down by the holdfast. Following the methods described by Straub et al. (2021) plants were acclimated for 6d before the application of experimental temperature treatments. Four ocean temperature treatments were applied over 48d. The four treatments were i) ambient (22°C), ii) warming (24°C), iii) heatwave non-variable (HWnon) – remaining at 22°C for 20d before moving to 27°C for 20d and back to 22°C for 8d, and iv) heatwave variable (HWvar) – exposed to rapid fluctuations between 22 and 24°C for 8d (temperature variability), remaining at 22°C for 6d moving to a heatwave at 27°C for 20d and back to 22°C for 8d (Figure 2). The magnitude of cumulative intensity was similar among treatments throughout the experiment (warming = 96 degree-days, HWnon = 85 degree-days, and HWvar = 97 degree-days cumulative intensity above the ambient treatment; Hobday et al., 2016).
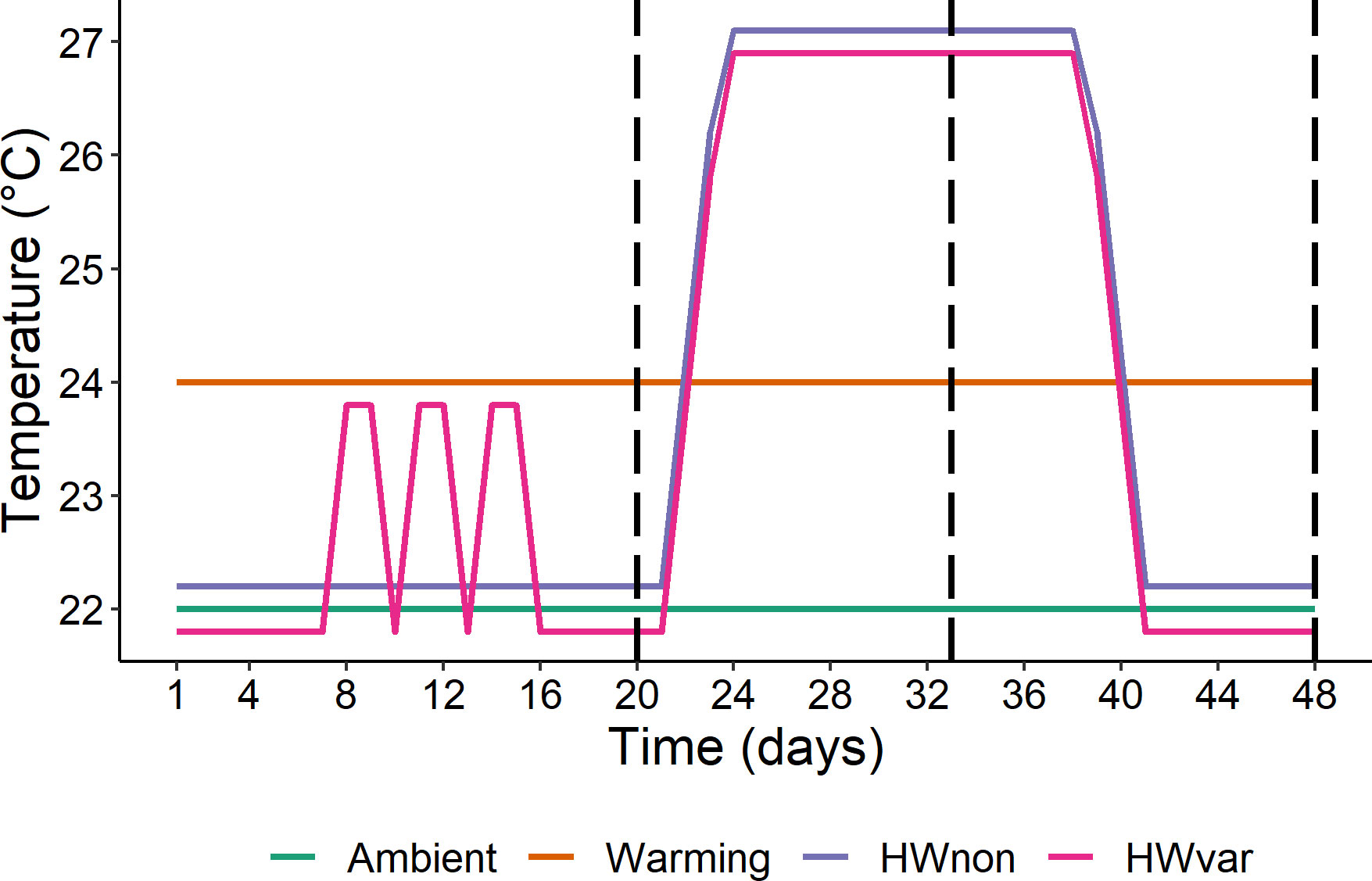
Figure 2 Experimental temperature treatment profiles: ambient (22°C), warming (24°C), HWnon (MHW from 22°C to 27°C and back to 22°C), and HWvar (initial fluctuations between 22-24°C, followed by a MHW to 27°C and back to 22°C). HWnon and HWvar treatments were offset by ± 0.2°C to avoid stacking of lines. Vertical dashed lines indicate sampling events (days 20, 33 and 48; modified from Straub et al., 2021).
Twenty aerated mesocosms (80cm diameter × 45cm high, 230L water volume) received a continuous inflow (flowrate ≈ 2L min-1) of filtered seawater (50μm) from Charlesworth Bay (30°16’3.78”S, 153° 8’25.60”E). Three header tanks with heater/chillers units (Aquahort Ltd.) controlled experimental temperatures at 22, 24, and 27°C, respectively. At the start of the experimental period, five tanks were assigned per treatment, and seven individual Ecklonia and Sargassum plants were placed within each tank (see Supplementary Material). Seaweeds acclimated at 22°C were randomly allocated to ambient, HWnon, and HWvar treatments (which start at 22°C) and seaweeds acclimated at 24°C were randomly allocated to a warming treatment tank (n = 14 plants/tank).
Tissue samples were collected on day 20 (after temperature variation, before marine heatwave), day 33 (12d into the marine heatwave), and day 48 (one-week recovery post-heatwave). At each sampling, a 9 mm hole punch was used to collect samples from the meristem of Ecklonia and tweezers were used to remove approximately 20 fronds of Sargassum. Each time, three independent plants, per species (n = 3) were sampled (see Supplementary Material). Samples were collected from three of the five independent tanks to generate experimental replicates and no plant was sampled twice, allowing the inclusion of ‘exposure time’ as an independent experimental factor. Following best practice, tissue samples were immediately snap-frozen in liquid nitrogen and frozen at – 80°C until analysis (Kumari, 2017).
2.2 Lipid and fatty acid extraction and analysis
Lipids were extracted from seaweed samples using a scaled-down approach modified from Folch et al. (1957) standard procedures, which accounted for the small sample weight obtained from the Ecklonia and Sargassum (0.07 ± 0.01 and 0.13 ± 0.07 g wet weight, respectively). Using analytical grade solvents (Sigma-Aldrich), samples were soaked in 2:1 chloroform:methanol, then polarized using 0.9% NaCl solution to obtain the lipophilic (chloroform) layer. The lipophilic layer was, transferred to a pre-weighed vial and dried using high-purity nitrogen gas to obtain the lipid extract weight. Lipid extracts were resuspended at 1mg mL-1 in dichloromethane:methanol (50/50 v/v). Total lipid concentration was expressed as a percentage of wet tissue weight for each extracted sample.
Lipid extracts were analyzed using direct infusion mass spectrometry (DIMS) using a high-resolution SCIEX 5600 Triple-TOF (Simons et al., 2012). Samples were run in both positive and negative modes with a flow rate of 200µL min-1. MS/MS reactions were obtained for peaks in the range of 500–1250 Da. Obtained spectra were analyzed using Lipidview™ 1.3 software (SCIEX) for lipid class and fatty acid composition (Ejsing et al., 2006). Thirty-three lipid classes and 43 fatty acids were detected using both positive and negative detection modes. Because the negative mode detected more lipid classes and fatty acids, the subsequent analysis used negative mode data only to avoid overrepresenting lipids detected in both modes. Lipid classes and fatty acids less than 0.01% were excluded from statistical analyses, and the remaining relative proportion of 13 lipid classes and 19 fatty acids were analyzed separately. The relative compositions (total percent) summed for saturated, monounsaturated and polyunsaturated fatty acids, the ω-3 index and ω-6/ω-3 ratio were also analyzed. The fatty acid double bond isomers cannot be identified using this method of mass spectrometry which limited our ability to distinguish between some fatty acid families (e.g. C20:3n-3/6/9 and C22:5n-3/6). Consequently, the ω-3 index was calculated as the proportion of eicosapentaenoic acid (EPA) and docosahexaenoic acid (DHA) expressed as a percent of total fatty acids. The ω-6/ω-3 PUFA ratio was calculated as arachidonic acid (ARA) divided by the sum of EPA and DHA.
2.3 Metabolomic extraction and analysis
Tissue subsamples were freeze-dried and derivatized for metabolite analysis using a modified, scaled-down approach based on methods described by Mabuchi et al. (2018; adapted from Lisec et al., 2006; Pongsuwan et al., 2007). A mixed solution of 2.5:1 methanol/ultrapure water/chloroform was used to extract hydrophilic primary metabolites in 15.73 (± 2.00) and 13.19 (± 1.30) mg of freeze-dried and macerated Ecklonia and Sargassum, respectively, with 0.2 µg mg-1 sample (dry weight) of ribitol (Sigma-Aldrich) added as an internal standard. The mixture was stirred for 5 min and centrifuged for a further 5 min (16,000xg, 4°C) and freeze-dried (~16h). Oxime formation was carried out by adding methoxyamine hydrochloride solubilized with pyridine (20mg/mL, 50µL) to the freeze-dried sample (35°C for 80 min). A trimethylsilylation reaction at 40°C for 40 min was induced using N-methyl-N-(trimethylsilyl) trifluoroacetamide (100µL).
Derivatized metabolite samples were analyzed by gas chromatography-mass spectrometry (GC-MS) using an Agilent 6890A Gas Chromatograph fitted with an Agilent 5973N Mass Selective Detector. The samples were injected with a split ratio of 50:1 using helium carrier gas at 32cm sec–1 constant flow and an inlet temperature of 280°C. Separating volatile compounds by using a Phenomenex ZB-5 column (60m x 0.25mm x 250µm) starting at 60°C with a 3 min hold, then ramped at 8°C min–1 to 330°C (44.75min) then held for 8min. The temperature of the mass spectrometer transfer line was 280°C. The mass spectra were recorded at 70 eV ionisation voltage over the mass range of 45 to 600amu. Peaks were identified by comparison of spectra with MS databases (WILEY 275 and NIST14), along with retention times and elution order. In instances where the compounds could not be positively identified, the compounds were assigned to a metabolite class when possible, based on examination of similarities in the major fragment ions corresponding to functional groups (see Supplementary Material). The relative abundance of each metabolite was quantified by TIC peak area integration from the GC and expressed as a percentage of the total in each run.
2.4 Statistical analysis
2.4.1 Nutritional quality
Linear mixed models were fitted to assess the effects of seaweed species, treatment, exposure time and their interactions on each nutritional quality response measured. Models used a gaussian distribution and included a random effect for tank ID to accommodate the nesting of tanks within treatments. Model residuals were inspected graphically to check for variance heterogeneity and non-normality. A natural log transformation of the data was required for several responses to meet the assumptions of normality and homogeneity of variance. An analysis of variance was derived from each model to test the effect of species, treatment, exposure time and their interaction. The models were then used to estimate the effects of species, treatment and time in various combinations as required. Post-hoc statistical comparisons of the estimates were made by Tukey’s test. The data analysis was conducted in the R environment (R Core Team, 2022) using the lme4 (Bates et al., 2015) and emmeans (Lenth, 2021) packages for modelling and estimation. Multivariate analysis of overall nutritional change confirmed species-specific differences in the compositional data (see Supplementary Material).
2.4.2 Climate change projections
Projections of Ecklonia lipid availability off the eastern Australian coast (28.0 – 37.5°S) were created using models of future Ecklonia biomass developed by Davis et al. (2021). Briefly, Ecklonia biomass projections were constructed using generalized additive mixed effect models (GAMMs) for the period 2090–2100 under representative concentration pathway (RCP) scenarios 2.6, 6.0 and 8.5 (IPCC, 2014). These projections incorporate ecological interaction effects with the urchin Centrostephanus rodgersii and accounted for the effects of key environmental parameters (habitat availability, light and in situ temperatures) that drive Ecklonia occurrence and cover (see more detail in Davis et al., 2021). As the total extracted lipids per gram wet weight of Ecklonia did not differ between temperature treatments (Table 1), the mean percentage of total Ecklonia lipids (1.4%) was used as a conversion factor to translate projected Ecklonia biomass values into lipid availability. The projected lipid availability maps were created in the R environment using the tidyverse suite of packages (Wickham et al., 2019). We focus our future projections of lipid availability on Ecklonia only as this is the dominant seaweed species occurring throughout the study extent and because comparable biomass projections are still underway for Sargassum.
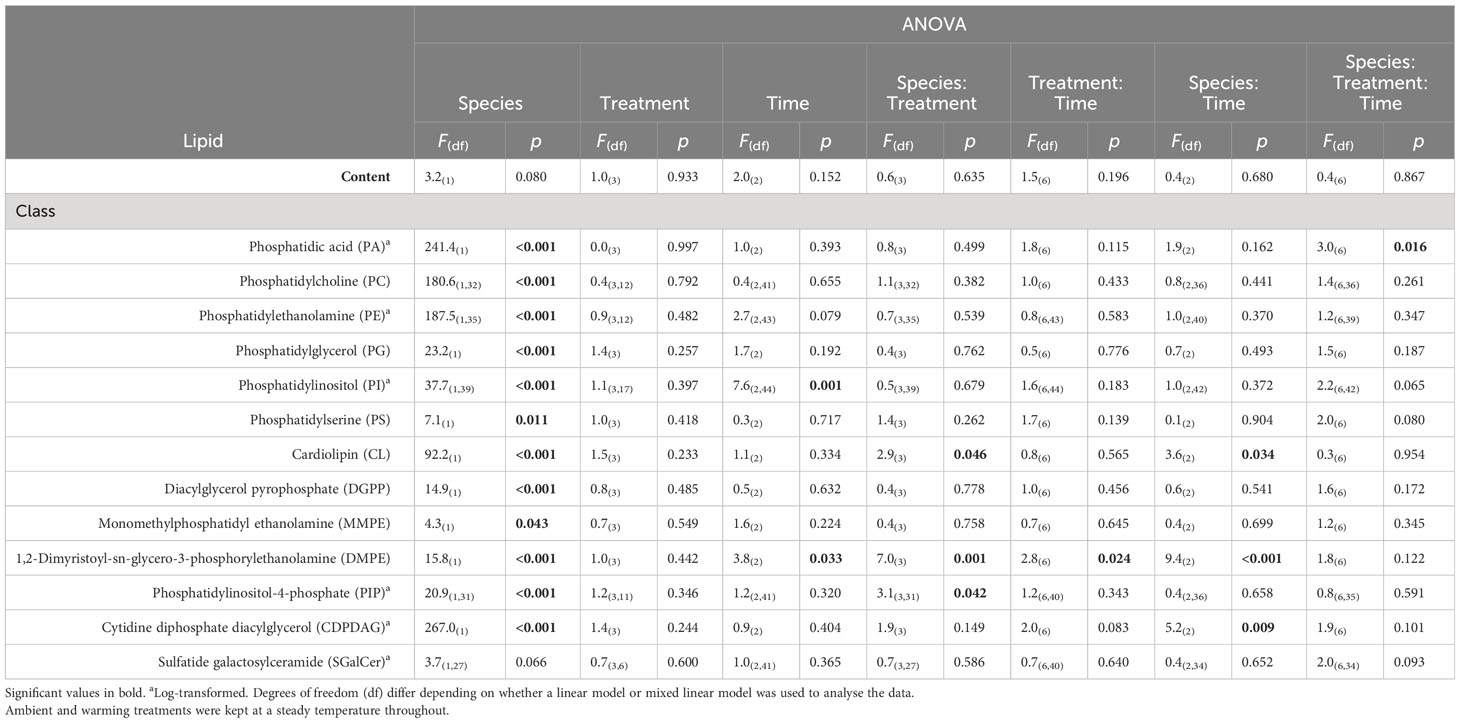
Table 1 Summary table of 3-factor ANOVA of relative percentage of lipid content (% wet weight) and lipid class composition from Ecklonia and Sargassum tissue exposed to four different temperature profiles (ambient, HWnon, HWvar, and warming), sampled before (20 days), during (33 days) and after (48 days) a simulated marine heatwave.
3 Results
3.1 Effects of ocean warming and marine heatwaves
Overall, the ocean warming and marine heatwave treatments showed minimal impacts on the nutrient content of Ecklonia and Sargassum. However, univariate analysis of the lipid classes showed that the interaction between species and treatment were statistically significant for 1,2-Dimyristoyl-sn-glycero-3-phosphorylethanolamine (DMPE), cardiolipin (CL) and phosphatidic acid (PA; Table 1). Post hoc pairwise comparisons showed that the interactive effect of species and treatment on DMPE was driven by significantly lower levels of DMPE in Sargassum from the ambient treatment relative to the HWnon treatment (p < 0.05). This difference was not observed for Ecklonia. Pair-wise tests did not detect any significant differences in CL composition among treatments for either species (p > 0.05). A significant interaction was observed for PA between species, treatment and exposure time. The post-hoc pairwise comparisons showed that after 33 days (12 days into the heatwaves) phosphatidic acid was significantly lower in Ecklonia from the HWnon temperature treatment compared to the ambient treatment (1.8%, p < 0.05); this trend was not observed for Sargassum or after a period of recovery (at 48 days). The trends in HWnon phosphatidic acid concentration throughout the experiment aligned with the HWnon maximum quantum yield of the Ecklonia meristem (as reported in Straub et al., 2021, Figure 3). The concentration of phosphatidic acid was higher under ambient conditions at this sampling time relative to both heatwave treatments.
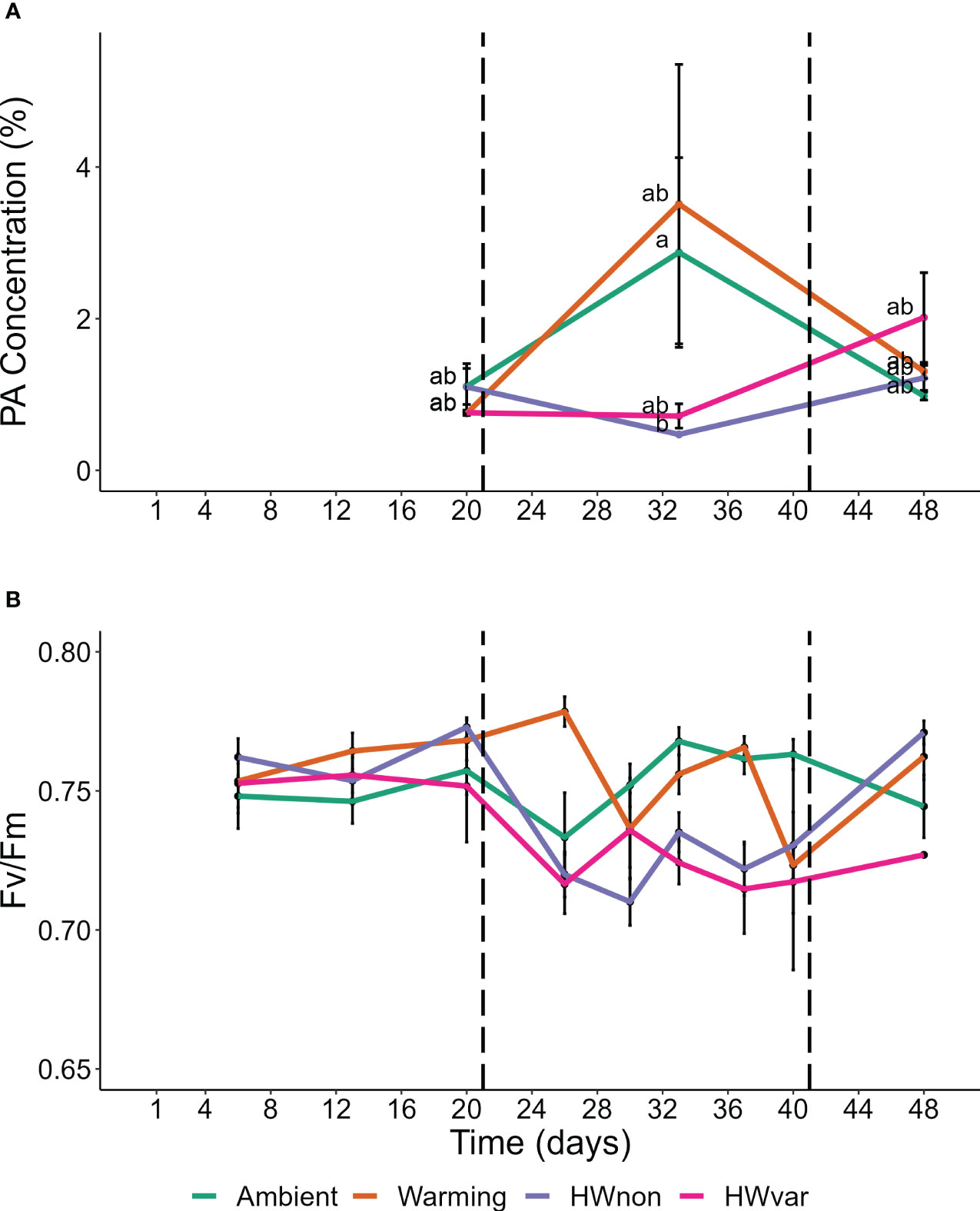
Figure 3 Ecklonia (A) phosphatidic acid concentration, and (B) maximum quantum yield [FV/FM; data derived from Straub et al. (2021)]; compared for samples exposed to the non-variable heatwave ‘HWnon’ treatment over time (means ± SE). Horizontal dotted lines mark the start and end of HWnon, letters indicate significant differences of the Tukey’s post-hoc test.
Ecklonia and Sargassum each showed minimal differences in fatty acids among treatments or among treatments over time (Table 2). However, there was a significant interaction between species and treatment for the saturated fatty acids C15:0 (Table 2). The post-hoc pairwise comparisons showed that the interaction between species and treatment for C15:0 was largely associated with a statistically significant (p < 0.05) increase under the warming treatment compared to the HWnon and HWvar treatments for Ecklonia which was not observed for Sargassum. Although statistically significant, the changes in C15:0 were less than 1% of the total fatty acid composition. A significant interaction between species, treatment and exposure time was detected for total saturated fatty acid (SFA) and the polyunsaturated fatty acids C20:3 and C20:4 (Table 2). However, these three-way interactions were not supported by the detection of any two-way interactions or main effects and no meaningful pairwise differences were detected by the post-hoc tests.
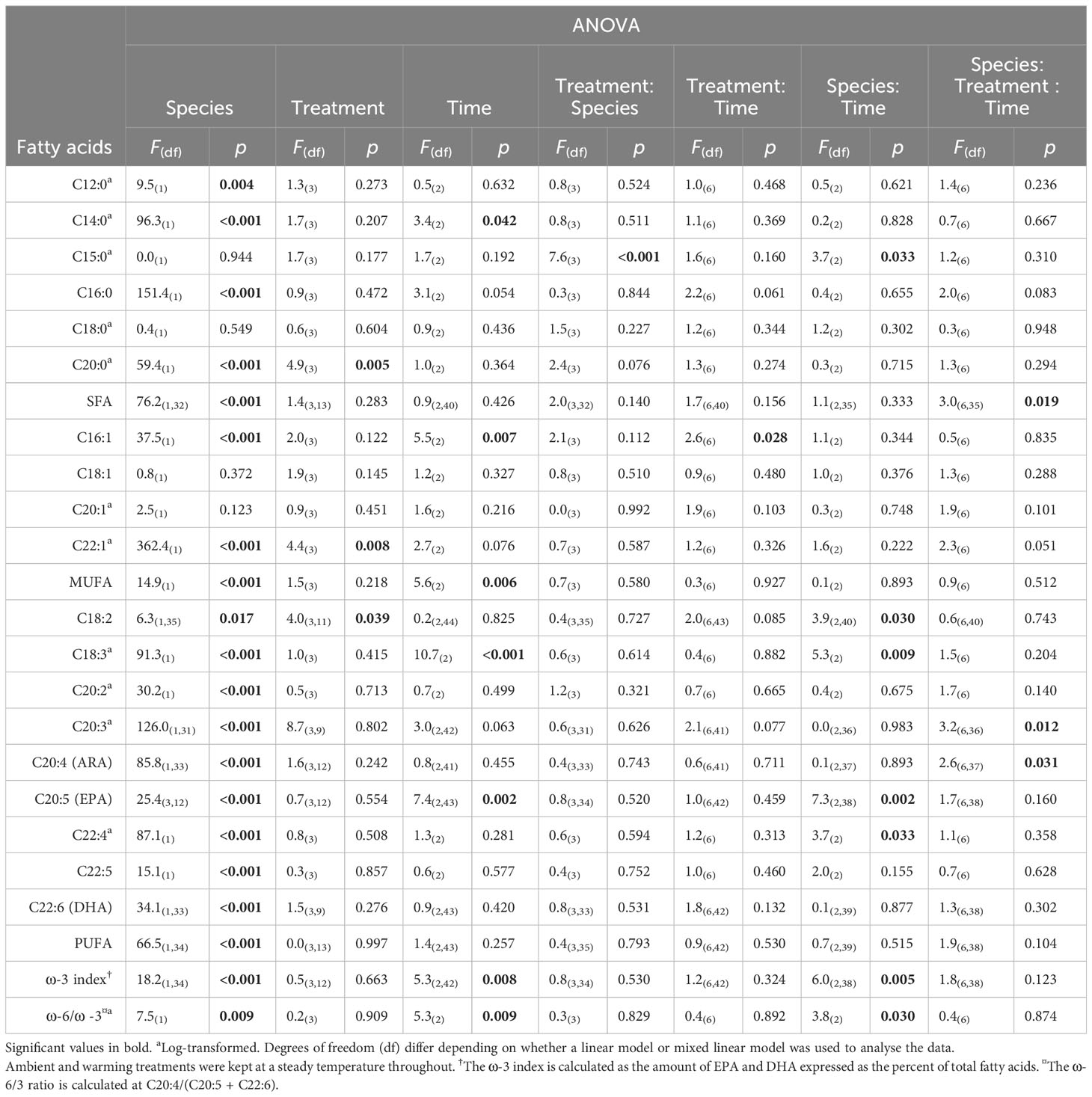
Table 2 Summary table of 3-factor ANOVA of relative percentage composition of selected fatty acids from Ecklonia and Sargassum tissue exposed to four different temperature profiles (ambient, HWnon, HWvar, and warming), sampled before (20 days), during (33 days) and after (48 days) a simulated marine heatwave.
When grouped into broad metabolite classifications, alkaloids showed a significant interaction between species and treatments. The post-hoc pairwise comparisons showed that the interaction between species and treatment was largely associated with a statistically significant (p < 0.05) decrease in alkaloids under the HWvar (p < 0.05) compared to the ambient tanks for Ecklonia which was not observed for Sargassum. No other significant interactions in broad metabolite groups, including amino acids and sugars, were found within the seaweed species in response to treatments or with treatments and exposure time (Table 3).
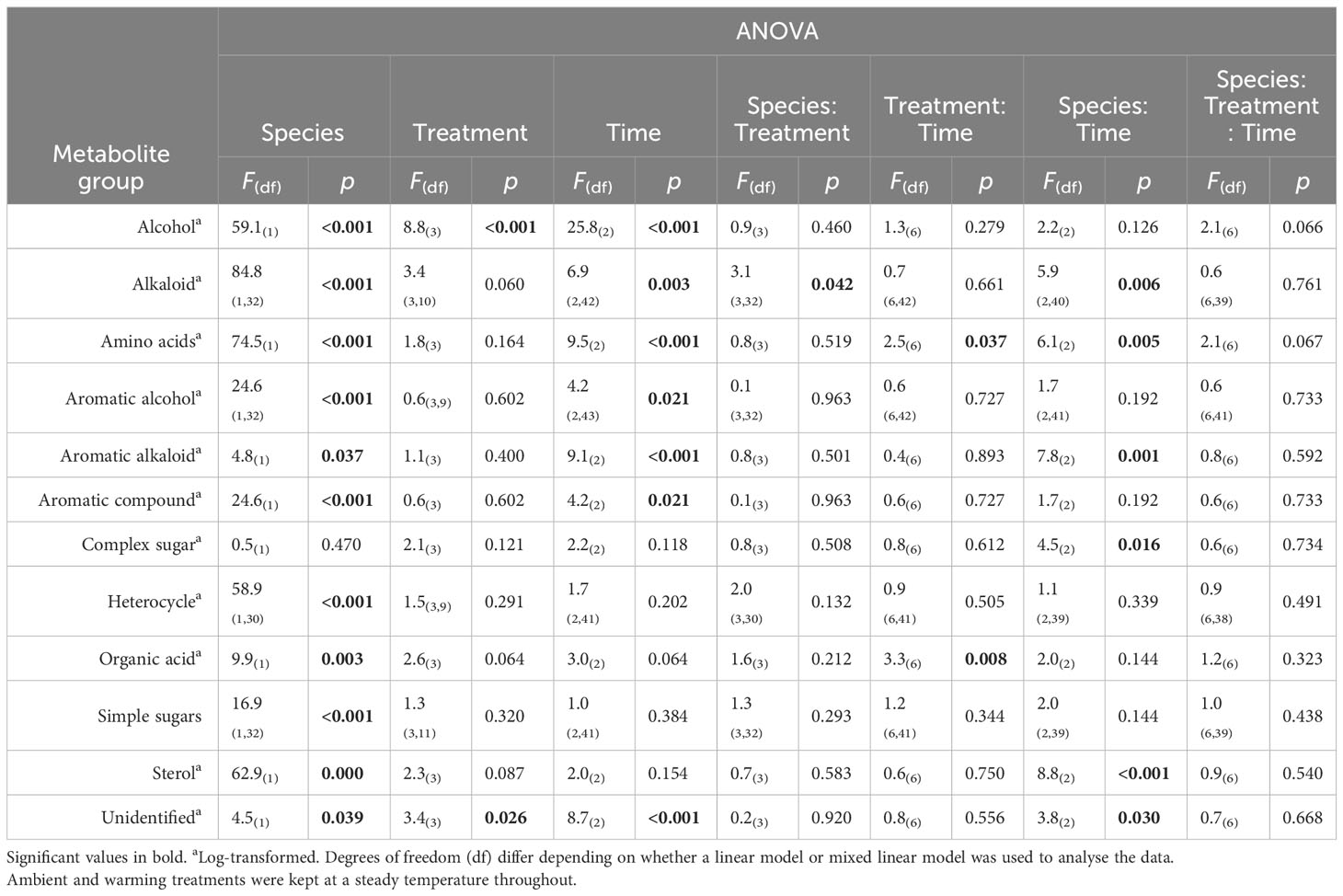
Table 3 Summary table of 3-factor ANOVA of relative percentage composition (± SE) of selected metabolites from Ecklonia and Sargassum tissue exposed to four different temperature profiles (ambient, HWnon, HWvar, and warming), sampled before (20 days), during (33 days) and after (48 days) a simulated marine heatwave.
3.2 Variation between species
The total extracted lipids of Ecklonia and Sargassum comprised on average 1.400 ± 0.113 and 1.100 ± 0.114% g-1 of the seaweeds’ total wet weight respectively, with no significant differences detected between species, experimental treatments or sampling period (Table 1). The percent composition of lipid classes showed that 92.3% (12 of 13) were significantly different between Ecklonia and Sargassum (Figure 4; Table 1). The relative concentration of phosphatidylcholine (PC), phosphatidylethanolamine (PE), phosphatidylglycerol (PG), phosphatidylinositol (PI) and monomethylphosphatidyl ethanolamine (MMPE) were significantly higher in Ecklonia compared to Sargassum (Table 1). While PA, phosphatidylserine (PS), CL, diacylglycerol pyrophosphate (DGPP), DMPE, phosphatidylinositol-4-phosphate (PIP) and cytidine diphosphate diacylglycerol (CDPDAG) were significantly higher in Sargassum in comparison to Ecklonia (Table 1). The percent of sulfatide galactosylceramide (SGalCer) was not significantly different between the two seaweed species (Table 1).
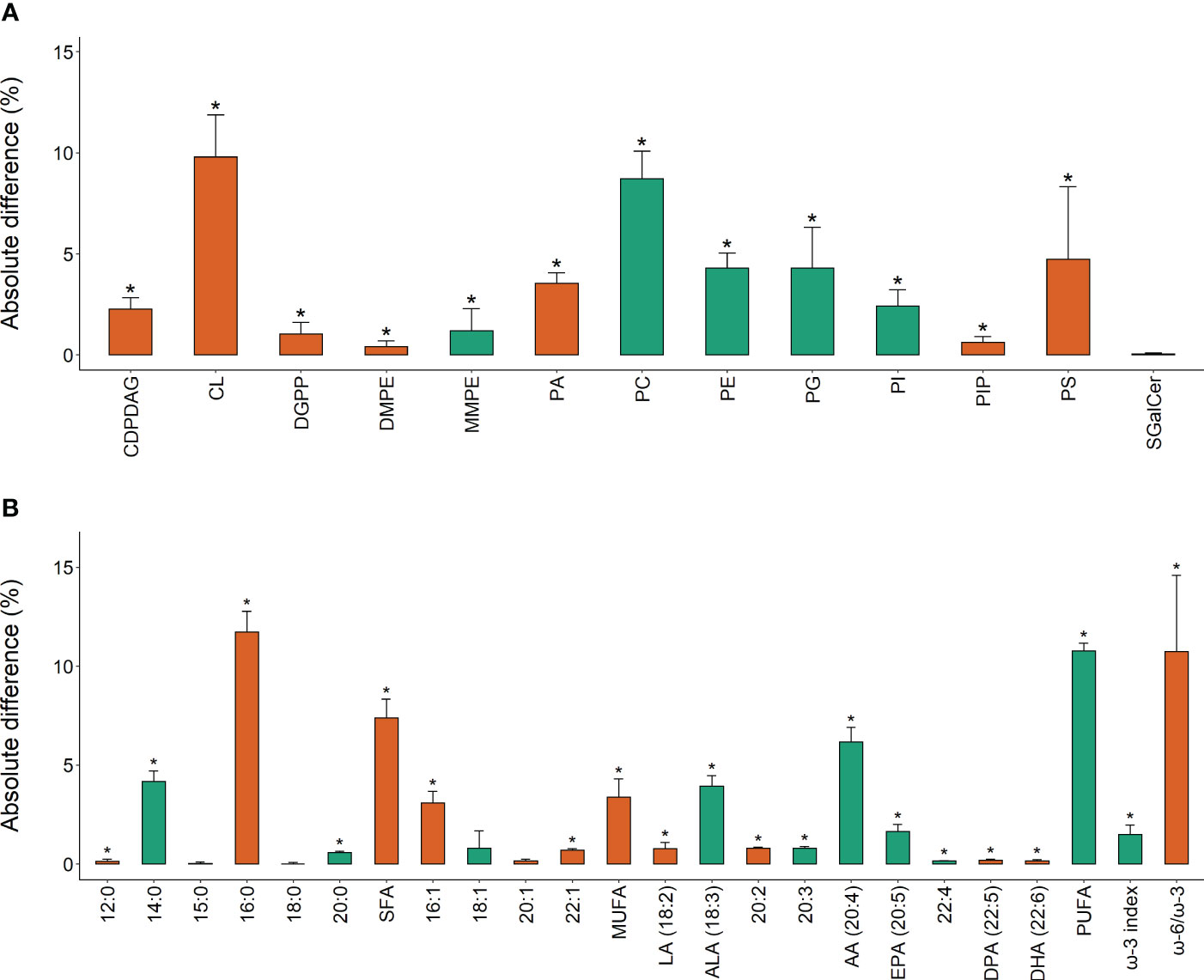
Figure 4 Absolute difference (%, +SE of the difference) in (A) lipid class; and (B) fatty acid composition between Ecklonia (green) and Sargassum (orange), for the main factor of species. The bar color indicates the species with greater percent composition of each variable. Asterisks (*) indicate a significant difference between species.
Univariate analysis of the fatty acids showed that 83% (20 of 24) were significantly different between Ecklonia and Sargassum (Table 2). Sargassum was 7.4% higher in total saturated fatty acids than Ecklonia (Figure 4; Table 2), driven by significantly higher levels of C12:0, C16:0 and C20:0 when compared to Ecklonia (Table 2). Monounsaturated fatty acids were also 3.4% higher in Sargassum compared to Ecklonia (Figure 4; Table 2), with C16:1 and C22:1 significantly higher in Sargassum than Ecklonia (Table 2). Polyunsaturated fatty acids were found to be 10.8% higher in Ecklonia when compared to Sargassum (Figure 4; Table 2). Omega-3 (C18:3, C20:5), omega-6 (C20:4, C22:4) and C20:3 fatty acids were significantly higher in Ecklonia when compared to Sargassum (Table 2). Compared to Ecklonia, Sargassum was significantly higher in C18:2 (omega-6), C20:2, C22:5 and C22:6 (omega-3; Table 2). Importantly, arachidonic acid (ARA, 20:4), a crucial long-chain polyunsaturated fatty acid in the omega-6 pathway, was 6.2% higher in Ecklonia than Sargassum (Figure 4). α–Linolenic (ALA, 18:3), important to the omega-3 pathway (Figure 1), was also 3.9% higher in Ecklonia (Figure 4). Omega-3 docosahexaenoic acid and omega-6 docosapentaenoic acid showed statistically significant changes however, with only ≤ 0.05% difference between the two species (Figure 4). Omega-3 eicosapentaenoic acid (EPA, 20:5) was, however, 1.6% higher in Ecklonia when compared to Sargassum (Figure 4). The ω-3 index was 1.5% higher in Ecklonia which shows the seaweed species has a higher omega-3 content than Sargassum (Figure 4). Sargassum had a 10.8% higher ω-6:ω-3 ratio of 16.8 compared to 6.0 in Ecklonia (Figure 4).
Metabolites were grouped into broad classes to further assess functional differences between species (see Supplementary Material). We found 91.7% (11 of 12) of metabolite classes significantly differed in content between species (see Supplementary Material). Ecklonia and Sargassum metabolites consist largely of simple sugars (93.3 and 96.5%, respectively; Figure 5A), of which Sargassum had significantly higher levels. The remaining metabolite composition of Sargassum comprised mostly of complex sugars (2.5%) and alcohols (0.5%; Figure 5B). Ecklonia metabolite composition was more diverse containing complex sugar (2.4%), and significantly higher levels of heterocycles (1.3%), aromatic alcohols (1.2%), alcohols (0.9%) and alkaloids (0.5%) than Sargassum (Figure 5B; Table 3).
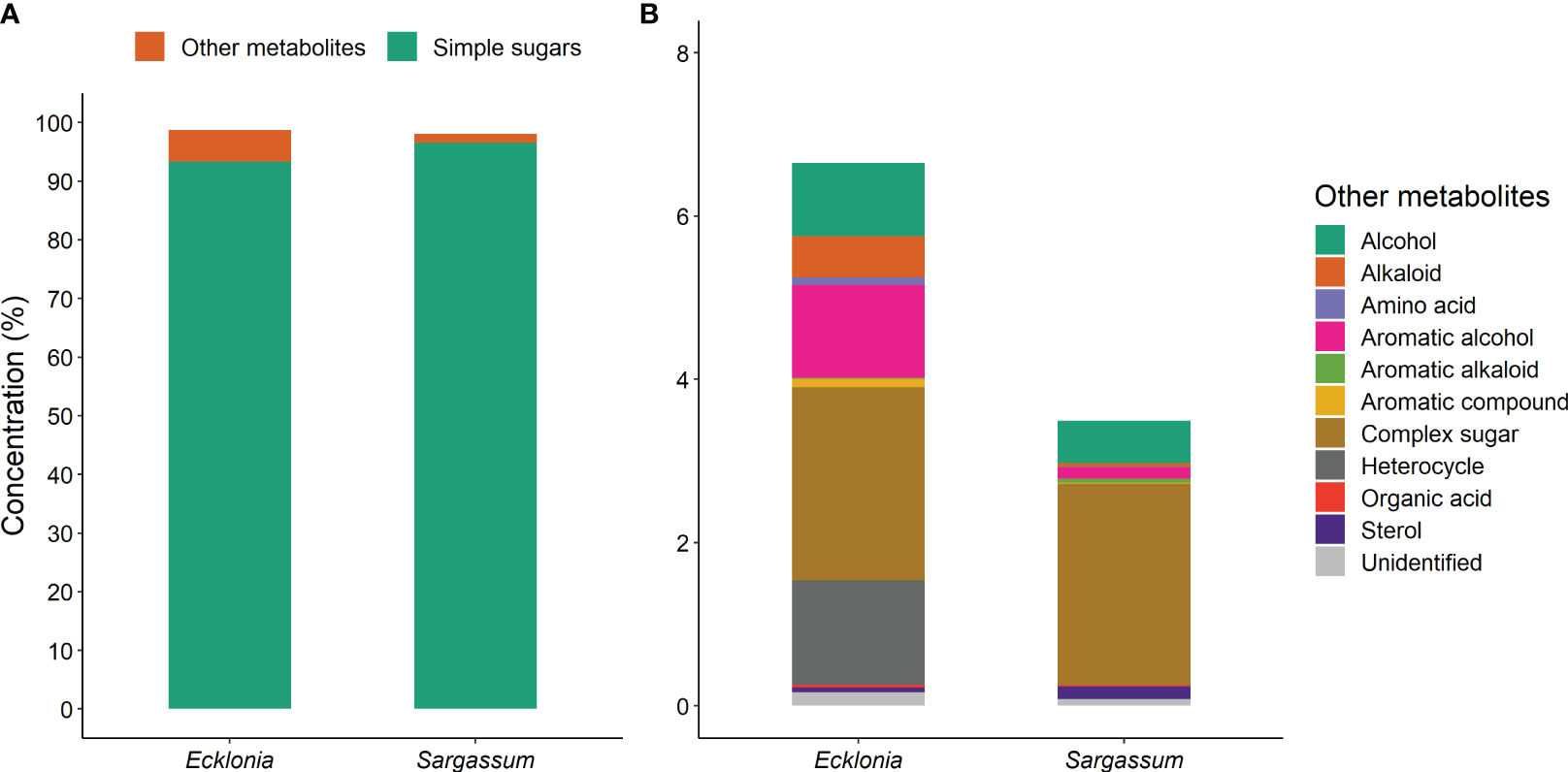
Figure 5 Comparisons of metabolite concentration between Ecklonia (n = 36) and Sargassum (n = 34) grouped based on their chemical class; (A) simple sugars (mono-, di- and tri- saccharides) compared to other metabolites, and (B) the compounds included in ‘other metabolites’, for the main factor of species. All compounds are significantly different between species except for complex sugars. The different colours within the bars represent different compounds, as detailed in the keys within each panel.
3.3 Redistribution of Ecklonia lipids under climate change
Projections of Ecklonia lipid availability off eastern Australia (28.0 – 37.5°S) revealed lipid reductions in warmer, lower latitudes where Ecklonia will range contract and increases within cooler, poleward and deeper regions of the study extent by 2100 where Ecklonia biomass will increase under all climate scenarios (Figure 6). Overall, total net lipid gains of 18.5% (4.9 g.m-2; Figure 6A) and 29.4% (7.7 g.m-2; Figure 6B) are projected under RCPs 2.6 and 6.0, respectively. In contrast, a 3.5% total net loss in Ecklonia derived lipids are projected under RCP 8.5 (-0.9 g.m-2; Figure 6C). As the majority of current Ecklonia biomass and subsequent lipid availability occurs at shallow to intermediate reef depths (i.e. <20 m), projected losses are associated with these depths and areas of the coast (primarily lower latitudes) where seabed temperatures are above ~26°C (Figure 6). Projected increases in lipid availability at high latitudes occur across the entire depth range under RCP 2.6, but are mostly restricted to deeper reefs under RCP 8.5 (>20 m; Figure 6).
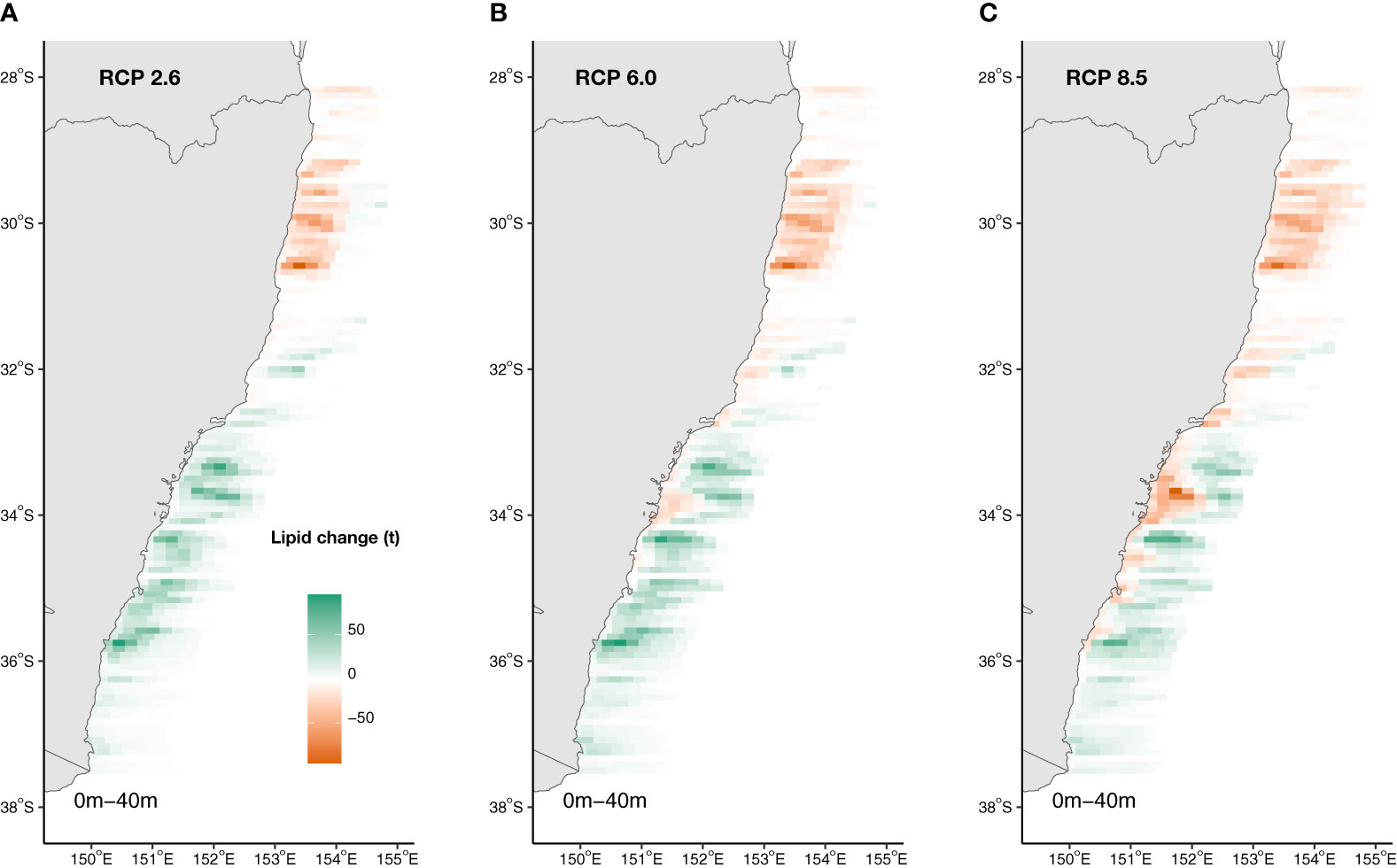
Figure 6 Projected changes to Ecklonia lipid availability (t) under climate change scenarios (A) RCP 2.6, (B) RCP 6.0 and (C) RCP 8.5 by 2100. Increases in lipid availability is represented by green and decreases by orange.
4 Discussion
Climate-driven environmental change is having profound impacts on the structure and function of temperate marine ecosystems (Wernberg et al., 2016a; Vergés et al., 2019; Holland et al., 2020). Although ocean temperature is one of the most crucial factors influencing seaweed distribution and physiological performance (Van Den Hoek, 1982; Van den Hoek et al., 1990; Krumhansl et al., 2016; Wernberg et al., 2016b; Veenhof et al., 2022), the impacts of temperature on seaweed nutrient composition and the implications for ecosystems have received limited attention (Stengel et al., 2011). In this study, we used molecular techniques to investigate the effects of warming and MHWs on the nutritional quality of two foundational Australian seaweeds, Ecklonia and Sargassum, through the optimization of a small-scale microextraction technique. We found that simulated ocean warming and MHWs had minimal impact on the nutritional properties of Ecklonia and Sargassum. However, nutritional quality differed considerably between the two species, suggesting that the climate-driven redistribution of these species could have implications for the provision of nutrients to marine food webs.
4.1 Ocean warming and marine heatwaves
Despite both ocean warming and MHWs having caused range contractions, local losses and genetic and physiological changes in Ecklonia and Sargassum in Australia (Vergés et al., 2016; Wernberg et al., 2016a; Straub et al., 2019; Coleman et al., 2020a; Gurgel et al., 2020), we observed minimal changes in the lipid and metabolite composition of these species in response to these ocean stressors. Our results were unexpected and contrary to other studies that observed changes in the fatty acid and metabolite composition of seaweeds in response to temperature changes in both field (Schmid et al., 2017; Gaubert et al., 2019) and laboratory settings (Renaud et al., 2002; Gosch et al., 2015). No biologically significant changes were detected in the fatty acids of Ecklonia or Sargassum in response to the warming and marine heatwave profiles. Increases in fatty acid saturation with increasing temperatures have been widely observed in marine algae (Renaud et al., 2002; Barbosa et al., 2020) and other marine plant species (Beca-Carretero et al., 2018). The unsaturation of fatty acids at low temperatures and saturation at high temperatures is an adaptative strategy algae use to maintain cell membrane fluidity and photosynthetic capacity with changing temperatures (Los and Murata, 2004; Schmid et al., 2021). Although this mechanism has commonly been observed in response to seasonal changes in temperature (Schmid et al., 2014; Schmid et al., 2017), this thermal adaptation may also assist seaweeds in coping with predicted ocean warming and MHWs (Britton et al., 2020). This response was not observed in our experiment for either Ecklonia or Sargassum, which could indicate that these species cannot adjust their fatty acid biosynthesis rapidly in response to unpredictable extreme temperature events. However, Britton et al. (2021) suggest that species that can also occupy intertidal or shallower areas such as Ecklonia and Sargassum may be better adapted to temperature fluctuations and potentially do not need to adjust fatty acid composition to maintain optimum membrane fluidity, or that the adjustments are small and hard to identify. Alternatively, our results could indicate a level of resilience, as the individuals sampled at each time point were survivors, which may have been better able to cope with warmer temperatures (e.g. thermally tolerant genotypes, Vranken et al., 2021; see Straub et al., 2021 for mortality throughout the experiment).
The time frame over which seaweeds might display a temperature-driven change in fatty acid composition is poorly understood. Changes in seaweed lipids in response to elevated temperature have been observed after as little as three days in the giant kelp Macrocystis pyrifera (Schmid et al., 2020), and differences in seaweed fatty acids have been observed during and after a period of recovery from MHWs (Britton et al., 2020). However, we do not yet understand whether these temperature-driven changes in physiological performance and nutritional properties are species-specific, adaptations of distinct genetic populations or correlate to natural processes (e.g. growth and reproduction). Twelve days into the simulated non-variable heatwave we observed substantially lower levels of phosphatidic acid in Ecklonia when compared to the ambient temperature treatment. However, following a period of recovery from the heatwave, this difference was no longer detected, suggesting recovery. Levels of phosphatidic acid were initially also relatively low in the warming and variable heatwave treatments but increased over time. Phosphatidic acid is an important component of seaweed chloroplast membranes (Melo et al., 2015), which are important for photosynthesis (Wang and Benning, 2012). During the marine heatwave treatments, Ecklonia photosynthetic quantum yield was significantly lower than the warming and ambient treatments but these differences were no longer observed after the recovery period (Figure 3; Straub et al., 2021). As only surviving plants were sampled at each time point, the performance improvement could be reflective of the responses of the better adapted survivors at 56 days compared to individuals sampled at 33 days. However, similar reductions in photosynthesis have been observed in other brown seaweeds (e.g. Phyllospora comosa) in response to MHWs, and following a period of recovery no lasting negative effects were detected (Britton et al., 2020). Therefore, the reduction in phosphatidic acid during the marine heatwave may be indicative of a stress response and connected to photosynthetic performance, however, further research is required to establish phosphatidic acid as a biomarker for photosynthetic stress responses in seaweeds.
Simulated ocean warming and marine heatwave treatments showed minimal effects on the metabolite content of both seaweed species. In particular, there was no evidence for impacts of temperature on complex and simple sugars which indicates there were no major effects on the potential beneficial properties (e.g. anti-inflammatory), energy content or calorific value of these species (Bayu et al., 2021). For example, fucoidan sugars like fructose have several biological activities including anti-inflammatory and anti-tumour properties which were unaffected by the temperature changes (Deniaud-Bouët et al., 2017). Amino acids function as important building blocks for protein (Maschek and Baker, 2008) and were unaffected by simulated ocean warming and marine heatwave scenarios. This suggests that temperature treatments did not trigger stress-induced protein degradation (Kumar et al., 2016), which would be expected to increase the levels of some amino acids in the tissues (Araújo et al., 2011). Additional studies are required to determine if the stability in metabolite content is a sign of resilience in the species’ or attributed to the sampling of survivors at each time point. While the application of metabolomics to marine system biology is increasing globally, the main obstacle that remains is the accurate annotation and identification of metabolites (Kumar et al., 2016; Lawson et al., 2022). A change was detected in an unidentified metabolite in response to the simulated temperatures, however, without sufficient context on the function of these compounds, the potential impacts on Ecklonia metabolism and nutritional quality could not be interpreted. For the future application of metabolomics techniques to marine seaweeds, access to advanced technology and the establishment of large mass spectral libraries centred on model organisms are needed (Kumar et al., 2016; Lawson et al., 2022).
4.2 Variation in nutritional quality between species
The nutritional composition between Ecklonia and Sargassum varied considerably. All 68 nutritional properties detected were present in both species, however, 82% differed substantially in content between species. The magnitude of difference between species was larger among lipid classes and fatty acids than metabolites. The lipid content of both Ecklonia and Sargassum was low (1.4 and 1.1%, respectively), with no difference detected between species and were similar to levels previously measured (Verma et al., 2017; Skrzypczyk et al., 2019). Ecklonia and Sargassum had lower total lipid levels than other Australian seaweed species such as Cystophora torulosa and C. polycystidea (8.4 and 5.7%, respectively), but were higher than the commercial species Sargassum fusiforme (0.7%, Skrzypczyk et al., 2019). Macroalgae are typically low in total fatty acids (in comparison to other algae e.g. microalgae) but have a high proportion of valuable polyunsaturated fatty acids (Schmid et al., 2014). This was supported by our results, as Ecklonia and Sargassum fatty acid composition was comprised of 39.3 and 28.6% polyunsaturated fatty acids, respectively. Although both species contained relatively high proportions of polyunsaturated fatty acids, higher levels were found in Ecklonia, while Sargassum contained substantially higher levels of saturated and monounsaturated fatty acids. Concentrations of polyunsaturated fatty acids tend to be higher in species from cold water and saturated fatty acids higher in species from warmer water, which could explain why temperate-tropical adapted species Sargassum displays higher levels of saturation (Choudhary et al., 2021).
The higher levels of polyunsaturated fatty acids in Ecklonia were driven by higher levels of omega-3 fatty acids (alpha-linolenic and docosapentaenoic) and arachidonic acid (an omega-6 fatty acid) when compared to Sargassum. Despite omega-3 fatty acids being primarily known for their benefits to human nutrition, these valuable fatty acids are also important to marine systems as an essential component of the cells of marine plants, animals and bacteria (Valentine and Valentine, 2004; Gladyshev et al., 2018). For example, numerous fish species have high eicosapentaenoic and docosahexaenoic acid content that is believed to support fast continuous swimming through its role in muscle cell metabolism (Gladyshev et al., 2018). Ecklonia displayed a higher omega-3 index than Sargassum and could be a better source of these fatty acids in temperate ecosystems in comparison. By contrast, Sargassum had a higher omega-6 to omega-3 ratio. Due to the opposing effects of omega-6 and omega-3 fatty acids (i.e. pro- and anti-inflammatory), a balanced consumption is recommended in human diets (Simopoulos, 2002). However, research into the importance of omega fatty acids for marine food chains is limited and the importance of this ratio for marine consumers is unclear. The species differences in nutritional composition are important in the context of nutritional seascapes because these two species are projected to change significantly and contrastingly under climate change.
Seaweed-herbivore interactions are complex (Hay and Fenical, 1988; Poore et al., 2012; Wernberg et al., 2013b). Consequently, structural shifts in seaweed availability may not equate to a suitable dietary replacement for consumers. Palatability is a key factor influencing herbivory and is influenced by macronutrient composition, for example, high protein and fat content often lead to higher palatability. Several other palatability factors including secondary metabolites, morphology and physical stress can also influence the herbivory of seaweeds (Cheng et al., 2022). Research has shown abalone species prefer a diet of brown algae over red or green algae which may be driven by high levels of polyunsaturated acids, in particular, eicosapentaenoic and arachidonic fatty acids (Nelson et al., 2002; Guest et al., 2008; Holland et al., 2021). Secondary metabolites are typically active in low concentrations and can impact the palatability for herbivores (Hay, 1996). These compounds were found in higher content in Ecklonia, including aromatic alcohols, heterocycles and alkaloids and could be used in defence against predators and pathogens (Hay, 1996; Engel et al., 2002). Some seaweeds also actively deter herbivory through chemical defences, for example, brown seaweeds contain phlorotannins which can discourage consumption by herbivores (Toth and Pavia, 2007). Simple sugars can affect the sweetness of seaweeds (Patrick et al., 2013), which may influence the consumption preferences of herbivores. These sugars were higher in Sargassum than Ecklonia, however, both species contained high quantities. These palatability factors may impact the uptake of nutrients from seaweeds despite their nutritional quality, providing a novel insight into potential nutrient transfer in marine food webs through metabolomics.
4.3 Redistribution of nutrients under climate change
Seaweeds supply coastal ecosystems with nutrients directly through macroalgal detritus (Duggins et al., 1989) and herbivory (Dunton and Schell, 1987) that are transferred to higher trophic levels (Figure 1; Vanderklift and Wernberg, 2010; Jin et al., 2020). Global changes in seaweed distribution and biomass have been driven by ocean warming (Krumhansl et al., 2016; Martínez et al., 2018), localized marine heatwave events (Wernberg et al., 2016a; Straub et al., 2019; Félix-Loaiza et al., 2022), and local anthropogenic stressors (Reed et al., 1994; Coleman et al., 2008; Strain et al., 2014), altering the nutritional seascapes of temperate environments. By 2100, the Australian distribution of Ecklonia is projected to contract by 49-71% under RCPs 2.6 and 6.0, respectively. Similarly, a 41-57% contraction in the distribution of Sargassum is projected under RCPs 2.6 and 6.0, respectively (Martínez et al., 2018). These estimates are based on correlative responses between each species and environmental variables (primarily SST) only and recent work incorporating key ecological interactions into future projections (e.g. urchin herbivory) of kelp biomass loss are more optimistic (Castro et al., 2020; Davis et al., 2021). For example, incorporating ecological interactions with urchins moderated projected net changes in Ecklonia biomass on the south-east coast of Australia to +18.5, +29.4% and -3.5% under RCPs 2.6, 6.0 and 8.5, respectively (Davis et al., 2021).
Projections of change in Ecklonia lipid availability across Australia’s east coast were made by combining our quantified lipid content with predicted alterations in distribution and biomass (Davis et al., 2021). While we found total net gains in Ecklonia lipid availability across south-east Australia under RCPs 2.6 and 6.0 and a small magnitude of total loss under RCP 8.5, the changes in lipid availability are associated with considerable spatial variation due to the shifting distribution of Ecklonia biomass off eastern Australia. For example, in northern areas of the study extent where it is projected that Ecklonia will undergo a 55-276 km range contraction (Davis et al., 2021), a complete loss of Ecklonia derived lipid availability is likely while increases are expected in more poleward regions as biomass is projected to increase due to weakening urchin herbivory. Increasing water temperatures are also projected to drive Ecklonia redistributions from shallow reefs to cooler, deeper reefs in mid-latitude areas (Davis et al., 2021). These changes may have major implications for marine consumers as the availability of nutrients shifts across not only a latitudinal but also a vertical dimension, particularly if consumers do not experience similar shifts in distribution. However, local drivers such as eutrophication may buffer the negative physiological effects of ocean warming in some areas (Fernández et al., 2020). The results also suggest that the redistribution of Ecklonia and Sargassum will considerably alter the availability of nutrients to marine ecosystems in areas where patterns of species co-occurrence are affected and there is a transition from one species to the other. For example, the predicted shift from Ecklonia to Sargassum dominated reefs at lower latitudes (Vergés et al., 2014; Vergés et al., 2019) would signify increasing saturation in available fatty acids and loss of metabolite diversity, while the loss of canopy cover, in general, would lead to an overall loss of valuable essential fatty acids for secondary consumers (Figure 1). Changes at the primary level could scale up through multiple trophic levels (e.g. primary, secondary, tertiary and finally humans) altering the trophic transfer of nutrients throughout entire marine systems (Jin et al., 2020). Combining lipidomic and metabolomic techniques with traditionally measured carbon, nitrogen and phosphate ratios will provide a greater depth to quantifying the nutritional changes in seaweeds (Peters et al., 2005). Moreover, to fully understand potential change in nutritional seascapes under climate change, we still require better understanding of food web dynamics as they relate to consumer and producer climate-redistributions and their interactions.
5 Conclusion
Lipidomic and metabolomic approaches harbor considerable value for quantifying ecosystem production and could be a powerful way to understand how nutritional seascapes may be altered under projected climate change. However, several knowledge gaps remain that must be addressed before such approaches can be widely adopted. First, future application of metabolomics techniques to marine seaweeds will rely on access to advanced technology and the establishment of large mass spectral libraries that allow the characterization of compounds and products that are currently uncharacterised (Kumar et al., 2016; Lawson et al., 2022). In addition, further research is required to establish links between seaweed compounds and biological responses (e.g. phosphatidic acid and photosynthetic stress). Nevertheless, through the optimization of a small-scale microextraction technique, we were able to demonstrate little influence of marine heatwaves and warming on the nutritional quality of two dominant habitat-forming seaweeds, but major differences between the species. These results could have major implications for climate-mediated shifts in species distributions and replacements which will fundamentally alter the nutritional seascapes of marine ecosystems under climate change.
Data availability statement
The raw data supporting the conclusions of this article will be made available by the authors, without undue reservation.
Author contributions
This project was conceptualized by TCS, MAC, TW, CC and KB. The experiment was run by MAC. Lipidomic and metabolomic analysis was undertaken by TCS and KB. Statistical analysis was carried out by TCS, SM, TD and MAC. Writing the manuscript was led by TCS with assistance from all authors. All authors contributed to the article and approved the submitted version.
Funding
This work is part of the NSW Primary Industries Climate Change Research Strategy, funded by the NSW Climate Change Fund. This work was undertaken with funding from the Australian Research Council (DP190100058 awarded to MC and TW) and the Research Training Program Scholarship awarded to TS. The SCIEX equipment used for lipid analyses was funded by the Australian Research Council Linkage Infrastructure, Equipment and Facilities grant LE170100192.
Acknowledgments
We extend our thanks to Sandra Straub, Ziggy Marzinelli, Adriana Verges, Reina Veenhof, Peter Butcherine and Endurance Ewere for their assistance and Bryan Mead and Ashley Dowell from the Southern Cross Analytical Research Facility for technical support. The vectors used in Figure 1 are courtesy of the Integration and Application Network, the University of Maryland Center for Environmental Science (ian.umces.edu/symbols/) and PhyloPic (phylopic.org/). We thank the reviewers for their invaluable feedback, which improved our manuscript.
Conflict of interest
The authors declare that the research was conducted in the absence of any commercial or financial relationships that could be construed as a potential conflict of interest.
Publisher’s note
All claims expressed in this article are solely those of the authors and do not necessarily represent those of their affiliated organizations, or those of the publisher, the editors and the reviewers. Any product that may be evaluated in this article, or claim that may be made by its manufacturer, is not guaranteed or endorsed by the publisher.
Supplementary material
The Supplementary Material for this article can be found online at: https://www.frontiersin.org/articles/10.3389/fmars.2023.1197468/full#supplementary-material
References
Araújo W. L., Tohge T., Ishizaki K., Leaver C. J., Fernie A. R. (2011). Protein degradation – an alternative respiratory substrate for stressed plants. Trends Plant Sci. 16 (9), 489–498. doi: 10.1016/j.tplants.2011.05.008
Barbosa M., Fernandes F., Pereira D. M., Azevedo I. C., Sousa-Pinto I., Andrade P. B., et al. (2020). Fatty acid patterns of the kelps Saccharina latissima, Saccorhiza polyschides and Laminaria ochroleuca: Influence of changing environmental conditions. Arabian. J. Chem. 13 (1), 45–58. doi: 10.1016/j.arabjc.2017.01.015
Bates D., Mächler M., Bolker B., Walker S. (2015). Fitting linear mixed-effects models using lme4. J. Stat. Software. 67 (1), 1–48. doi: 10.18637/jss.v067.i01
Bayu A., Warsito M. F., Putra M. Y., Karnjanakom S., Guan G. (2021). Macroalgae-derived rare sugars: Applications and catalytic synthesis. Carbon. Resour. Conversion. 4, 150–163. doi: 10.1016/j.crcon.2021.04.002
Beca-Carretero P., Guihéneuf F., Marín-Guirao L., Bernardeau-Esteller J., García-Muñoz R., Stengel D. B., et al. (2018). Effects of an experimental heat wave on fatty acid composition in two Mediterranean seagrass species. Mar. Pollut. Bull. 134, 27–37. doi: 10.1016/j.marpolbul.2017.12.057
Bennett S., Wernberg T., Connell S. D., Hobday A. J., Johnson C. R., Poloczanska E. S. (2015). The ‘Great Southern Reef’: social, ecological and economic value of Australia’s neglected kelp forests. Mar. Freshw. Res. 67 (1), 47–56. doi: 10.1071/MF15232
Bolin J. A., Schoeman D. S., Evans K. J., Cummins S. F., Scales K. L. (2021). Achieving sustainable and climate-resilient fisheries requires marine ecosystem forecasts to include fish condition. Fish. Fisheries. 1–18. doi: 10.1111/faf.12569
Britton D., Schmid M., Noisette F., Havenhand J. N., Paine E. R., McGraw C. M., et al. (2020). Adjustments in fatty acid composition is a mechanism that can explain resilience to marine heatwaves and future ocean conditions in the habitat-forming seaweed Phyllospora comosa (Labillardière) C.Agardh. Global Change Biol. 26 (6), 3512–3524. doi: 10.1111/gcb.15052
Britton D., Schmid M., Revill A. T., Virtue P., Nichols P. D., Hurd C. L., et al. (2021). Seasonal and site-specific variation in the nutritional quality of temperate seaweed assemblages: implications for grazing invertebrates and the commercial exploitation of seaweeds. J. Appl. Phycol. 33 (1), 603–616. doi: 10.1007/s10811-020-02302-1
Casado-Amezúa P., Araújo R., Bárbara I., Bermejo R., Borja Á., Díez I., et al. (2019). Distributional shifts of canopy-forming seaweeds from the Atlantic coast of Southern Europe. Biodivers. Conserv. 28 (5), 1151–1172. doi: 10.1007/s10531-019-01716-9
Castro L. C., Cetina-Heredia P., Roughan M., Dworjanyn S., Thibaut L., Chamberlain M. A., et al. (2020). Combined mechanistic modelling predicts changes in species distribution and increased co-occurrence of a tropical urchin herbivore and a habitat-forming temperate kelp. Divers. Distrib. 26 (9), 1211–1226. doi: 10.1111/ddi.13073
Cheng A., Lim W. Y., Lim P.-E., Yang Amri A., Poong S.-W., Song S.-L., et al. (2022). Marine autotroph-herbivore synergies: Unravelling the roles of macroalgae in marine ecosystem dynamics. Biology 11 (8). doi: 10.3390/biology11081209
Choudhary B., Chauhan O. P., Mishra A. (2021). Edible seaweeds: A potential novel source of bioactive metabolites and nutraceuticals with human health benefits. Front. Mar. Sci. 8. doi: 10.3389/fmars.2021.740054
Christie H., Norderhaug K. M., Fredriksen S. (2009). Macrophytes as habitat for fauna. Mar. Ecol. Prog. Ser. 396, 221–233. doi: 10.3354/meps08351
Coleman M. A., Brawley S. H. (2005). Spatial and temporal variability in dispersal and population genetic structure of a rockpool alga. Mar. Ecol. Prog. Ser. 300, 63–77. doi: 10.3354/meps300063
Coleman M. A., Kelaher B. P., Steinberg P. D., Millar A. J. K. (2008). Absence of a large brown macroalga on urbanized rocky reefs around Sydney, Australia, and evidence for historical decline. J. Phycol. 44 (4), 897–901. doi: 10.1111/j.1529-8817.2008.00541.x
Coleman M. A., Minne A. J. P., Vranken S., Wernberg T. (2020a). Genetic tropicalisation following a marine heatwave. Sci. Rep. 10 (1), 12726. doi: 10.1038/s41598-020-69665-w
Coleman M. A., Wernberg T. (2017). Forgotten underwater forests: The key role of fucoids on Australian temperate reefs. Ecol. Evol. 7 (20), 8406–8418. doi: 10.1002/ece3.3279
Coleman M. A., Wood G., Filbee-Dexter K., Minne A. J. P., Goold H. D., Vergés A., et al. (2020b). Restore or redefine: Future trajectories for restoration. Front. Mar. Sci. 7 (237). doi: 10.3389/fmars.2020.00237
Davis T. R., Champion C., Coleman M. A. (2021). Climate refugia for kelp within an ocean warming hotspot revealed by stacked species distribution modelling. Mar. Environ. Res. 166, 105267. doi: 10.1016/j.marenvres.2021.105267
de Bettignies T., Wernberg T., Gurgel C. F. D. (2018). Exploring the influence of temperature on aspects of the reproductive phenology of temperate seaweeds. Front. Mar. Sci. 5 (218). doi: 10.3389/fmars.2018.00218
Deniaud-Bouët E., Hardouin K., Potin P., Kloareg B., Hervé C. (2017). A review about brown algal cell walls and fucose-containing sulfated polysaccharides: Cell wall context, biomedical properties and key research challenges. Carbohydr. Polymers. 175, 395–408. doi: 10.1016/j.carbpol.2017.07.082
Duggins D. O., Simenstad C. A., Estes J. A. (1989). Magnification of secondary production by kelp detritus in coastal marine ecosystems. Science 245 (4914), 170–173. doi: 10.1126/science.245.4914.170
Dunton K. H., Schell D. M. (1987). Dependence of consumers on macroalgal (Laminaria solidungula) carbon in an arctic kelp community: δ13C evidence. Mar. Biol. 93 (4), 615–625. doi: 10.1007/BF00392799
Ejsing C. S., Duchoslav E., Sampaio J., Simons K., Bonner R., Thiele C., et al. (2006). Automated identification and quantification of glycerophospholipid molecular species by multiple precursor ion scanning. Analytical. Chem. 78 (17), 6202–6214. doi: 10.1021/ac060545x
Engel S., Jensen P. R., Fenical W. (2002). Chemical ecology of marine microbial defense. J. Chem. Ecol. 28 (10), 1971–1985. doi: 10.1023/A:1020793726898
Félix-Loaiza A. C., Rodríguez-Bravo L. M., Beas-Luna R., Lorda J., de la Cruz-González E., Malpica-Cruz L. (2022). Marine heatwaves facilitate invasive algae takeover as foundational kelp. Bot. Mar. 65 (5), 315–319. doi: 10.1515/bot-2022-0037
Fernández P. A., Gaitán-Espitia J. D., Leal P. P., Schmid M., Revill A. T., Hurd C. L. (2020). Nitrogen sufficiency enhances thermal tolerance in habitat-forming kelp: implications for acclimation under thermal stress. Sci. Rep. 10 (1), 3186. doi: 10.1038/s41598-020-60104-4
Folch J., Lees M., Sloane Stanley G. H. (1957). A simple method for the isolation and purification of total lipides from animal tissues. J. Biol. Chem. 226 (1), 497–509. doi: 10.1016/S0021-9258(18)64849-5
Gaubert J., Payri C. E., Vieira C., Solanki H., Thomas O. P. (2019). High metabolic variation for seaweeds in response to environmental changes: a case study of the brown algae Lobophora in coral reefs. Sci. Rep. 9 (1), 993. doi: 10.1038/s41598-018-38177-z
Geppi E. F., Riera R. (2022). Responses of intertidal seaweeds to warming: A 38- year time series shows differences of sizes. Estuarine. Coast. Shelf. Sci. 270, 107841. doi: 10.1016/j.ecss.2022.107841
Ghaderiardakani F., Langhans L., Kurbel V. B., Fenizia S., Wichard T. (2022). Metabolite profiling reveals insights into the species-dependent cold stress response of the green seaweed holobiont Ulva (Chlorophyta). Environ. Exp. Bot. 200, 104913. doi: 10.1016/j.envexpbot.2022.104913
Gladyshev M. I., Sushchik N. N., Tolomeev A. P., Dgebuadze Y. Y. (2018). Meta-analysis of factors associated with omega-3 fatty acid contents of wild fish. Rev. Fish. Biol. Fisheries. 28 (2), 277–299. doi: 10.1007/s11160-017-9511-0
Gosch B. J., Lawton R. J., Paul N. A., de Nys R., Magnusson M. (2015). Environmental effects on growth and fatty acids in three isolates of Derbesia tenuissima (Bryopsidales, Chlorophyta). Algal. Res. 9, 82–93. doi: 10.1016/j.algal.2015.02.022
Guest M. A., Nichols P. D., Frusher S. D., Hirst A. J. (2008). Evidence of abalone (Haliotis rubra) diet from combined fatty acid and stable isotope analyses. Mar. Biol. 153 (4), 579–588. doi: 10.1007/s00227-007-0831-9
Gurgel C. F. D., Camacho O., Minne A. J. P., Wernberg T., Coleman M. A. (2020). Marine heatwave drives cryptic loss of genetic diversity in underwater forests. Curr. Biol. 30 (7), 1199–1206.e1192. doi: 10.1016/j.cub.2020.01.051
Hay M. E. (1996). Marine chemical ecology: what’s known and what’s next? J. Exp. Mar. Biol. Ecol. 200 (1), 103–134. doi: 10.1016/S0022-0981(96)02659-7
Hay M. E., Fenical W. (1988). Marine plant-herbivore interactions: The ecology of chemical defense. Annu. Rev. Ecol. Syst. 19 (1), 111–145. doi: 10.1146/annurev.es.19.110188.000551
Hobday A. J., Alexander L. V., Perkins S. E., Smale D. A., Straub S. C., Oliver E. C., et al. (2016). A hierarchical approach to defining marine heatwaves. Prog. Oceanogr. 141, 227–238. doi: 10.1016/j.pocean.2015.12.014
Holland M. M., Smith J. A., Everett J. D., Vergés A., Suthers I. M. (2020). Latitudinal patterns in trophic structure of temperate reef-associated fishes and predicted consequences of climate change. Fish. Fisheries. 21 (6), 1092–1108. doi: 10.1111/faf.12488
Holland O. J., Young M. A., Sherman C. D. H., Tan M. H., Gorfine H., Matthews T., et al. (2021). Ocean warming threatens key trophic interactions supporting a commercial fishery in a climate change hotspot. Global Change Biol. 27 (24), 6498–6511. doi: 10.1111/gcb.15889
IPCC. (2014). Climate change 2014: synthesis report. Contribution of working groups I, II and III to the fifth assessment report f the Intergovernmental Panel on Climate Change (Geneva, Switzerland: IPCC).
Jin P., Hutchins D. A., Gao K. (2020). The impacts of ocean acidification on marine food quality and its potential food chain consequences. Front. Mar. Sci. 7 (780). doi: 10.3389/fmars.2020.543979
Krumhansl K. A., Okamoto D. K., Rassweiler A., Novak M., Bolton J. J., Cavanaugh K. C., et al. (2016). Global patterns of kelp forest change over the past half-century. Proc. Natl. Acad. Sci. 113 (48), 13785–13790. doi: 10.1073/pnas.1606102113
Kumar M., Kuzhiumparambil U., Pernice M., Jiang Z., Ralph P. J. (2016). Metabolomics: an emerging frontier of systems biology in marine macrophytes. Algal. Res. 16, 76–92. doi: 10.1016/j.algal.2016.02.033
Kumari P. (2017). “Seaweed lipidomics in the era of ‘omics’ biology: A contemporary perspective,” in Systems biology of Marine Ecosystems. Eds. Kumar M., Ralph P. (Cham: Springer).
Lawson C. A., Camp E., Davy S. K., Ferrier-Pagès C., Matthews J., Suggett D. J. (2022). “Informing coral reef conservation through metabolomic approaches,” in Coral reef conservation and restoration in the omics age, vol. Vol. 1 . Eds. van Oppen M. J. H., Aranda Lastra M. (Cham: Springer International Publishing) 179–202.
Layton C., Coleman M. A., Marzinelli E. M., Steinberg P. D., Swearer S. E., Vergés A., et al. (2020). Kelp forest restoration in Australia. Front. Mar. Sci. 7. doi: 10.3389/fmars.2020.00074
Lenth R. (2021) emmeans: Estimated marginal means, aka leastsquares means (Version R package 1.7.5). Available at: https://CRAN.Rproject.org/package=emmeans.
Lima F. P., Ribeiro P. A., Queiroz N., Hawkins S. J., Santos A. M. (2007). Do distributional shifts of northern and southern species of algae match the warming pattern? Global Change Biol. 13 (12), 2592–2604. doi: 10.1111/j.1365-2486.2007.01451.x
Lisec J., Schauer N., Kopka J., Willmitzer L., Fernie A. R. (2006). Gas chromatography mass spectrometry–based metabolite profiling in plants. Nat. Protoc. 1 (1), 387–396. doi: 10.1038/nprot.2006.59
Litzow M. ,. A., Bailey K. M., Prahl F. G., Heintz R. (2006). Climate regime shifts and reorganization of fish communities: the essential fatty acid limitation hypothesis. Mar. Ecol. Prog. Ser. 315, 1–11. doi: 10.3354/meps315001
Lopes D., Melo T., Rey F., Meneses J., Monteiro F. L., Helguero L. A., et al. (2020). Valuing bioactive lipids from green, red and brown macroalgae from aquaculture, to foster functionality and biotechnological applications. Molecules 25 (17). doi: 10.3390/molecules25173883
Los D. A., Murata N. (2004). Membrane fluidity and its roles in the perception of environmental signals. Biochim. Biophys. Acta (BBA). - Biomembranes. 1666 (1), 142–157. doi: 10.1016/j.bbamem.2004.08.002
Lozano-Montes H. M., Loneragan N. R., Babcock R. C., Jackson K. (2011). Using trophic flows and ecosystem structure to model the effects of fishing in the Jurien Bay Marine Park, temperate Western Australia. Mar. Freshw. Res. 62 (5), 421–431. doi: 10.1071/MF09154
Mabuchi R., Adachi M., Kikutani H., Tanimoto S. (2018). Discriminant analysis of muscle tissue type in yellowtail Seriola quinqueradiata muscle based on metabolic component profiles. Food Sci. Technol. Res. 24 (5), 883–891. doi: 10.3136/fstr.24.883
MacArtain P., Gill C. I. R., Brooks M., Campbell R., Rowland I. R. (2007). Nutritional value of edible seaweeds. Nutr. Rev. 65 (12), 535–543. doi: 10.1111/j.1753-4887.2007.tb00278.x
Martínez B., Radford B., Thomsen M. S., Connell S. D., Carreño F., Bradshaw C. J. A., et al. (2018). Distribution models predict large contractions of habitat-forming seaweeds in response to ocean warming. Divers. Distrib. 24 (10), 1350–1366. doi: 10.1111/ddi.12767
Maschek J. A., Baker B. J. (2008). “The chemistry of algal secondary metabolism,” in Algal Chemical Ecology. Ed. Amsler C. D. (Berlin, Heidelberg: Springer Berlin Heidelberg), 1–24.
McCauley J. I., Meyer B. J., Winberg P. C., Ranson M., Skropeta D. (2015). Selecting Australian marine macroalgae based on the fatty acid composition and anti-inflammatory activity. J. Appl. Phycol. 27 (5), 2111–2121. doi: 10.1007/s10811-014-0465-5
Melo T., Alves E., Azevedo V., Martins A. S., Neves B., Domingues P., et al. (2015). Lipidomics as a new approach for the bioprospecting of marine macroalgae — Unraveling the polar lipid and fatty acid composition of Chondrus crispus. Algal. Res. 8, 181–191. doi: 10.1016/j.algal.2015.02.016
Michaud K. M., Reed D. C., Miller R. J. (2022). The Blob marine heatwave transforms California kelp forest ecosystems. Commun. Biol. 5 (1), 1143. doi: 10.1038/s42003-022-04107-z
Miranda R. J., Coleman M. A., Tagliafico A., Rangel M. S., Mamo L. T., Barros F., et al. (2019). Invasion-mediated effects on marine trophic interactions in a changing climate: positive feedbacks favour kelp persistence. Proc. R. Soc. B 286 (1899), 20182866. doi: 10.1098/rspb.2018.2866
Nelson M. M., Leighton D. L., Phleger C. F., Nichols P. D. (2002). Comparison of growth and lipid composition in the green abalone, Haliotis fulgens, provided specific macroalgal diets. Comp. Biochem. Physiol. Part B: Biochem. Mol. Biol. 131 (4), 695–712. doi: 10.1016/S1096-4959(02)00042-8
Park E., Yu H., Lim J.-H., Hee Choi J., Park K.-J., Lee J. (2023). Seaweed metabolomics: A review on its nutrients, bioactive compounds and changes in climate change. Food Res. Int. 163, 112221. doi: 10.1016/j.foodres.2022.112221
Parrish C. C. (2009). “Essential fatty acids in aquatic food webs,” in Lipids in Aquatic Ecosystems. Eds. Kainz M., Brett M. T., Arts M. T. (New York, NY: Springer New York), 309–326.
Patrick J. W., Botha F. C., Birch R. G. (2013). Metabolic engineering of sugars and simple sugar derivatives in plants. Plant Biotechnol. J. 11 (2), 142–156. doi: 10.1111/pbi.12002
Peters K. J., Amsler C. D., Amsler M. O., McClintock J. B., Dunbar R. B., Baker B. J. (2005). A comparative analysis of the nutritional and elemental composition of macroalgae from the western Antarctic Peninsula. Phycologia 44 (4), 453–463. doi: 10.2216/0031-8884(2005)44[453:ACAOTN]2.0.CO;2
Pongsuwan W., Fukusaki E., Bamba T., Yonetani T., Yamahara T., Kobayashi A. (2007). Prediction of Japanese green tea ranking by gas chromatography/mass spectrometry-based hydrophilic metabolite fingerprinting. J. Agric. Food Chem. 55 (2), 231–236. doi: 10.1021/jf062330u
Poore A. G. B., Campbell A. H., Coleman R. A., Edgar G. J., Jormalainen V., Reynolds P. L., et al. (2012). Global patterns in the impact of marine herbivores on benthic primary producers. Ecol. Lett. 15 (8), 912–922. doi: 10.1111/j.1461-0248.2012.01804.x
Provost E. J., Kelaher B. P., Dworjanyn S. A., Russell B. D., Connell S. D., Ghedini G., et al. (2017). Climate-driven disparities among ecological interactions threaten kelp forest persistence. Global Change Biol. 23 (1), 353–361. doi: 10.1111/gcb.13414
R Core Team. (2022). R: A language and environment for statistical computing. (Version 2022.12.0) (Vienna, Austria: R Foundation for Statistical Computing). Available at: www.R-project.org.
Reed D. C., Lewis R. J., Anghera M. (1994). Effects of an open-coast oil-production outfall on patterns of giant kelp (Macrocystis pyrifera) recruitment. Mar. Biol. 120 (1), 25–31. doi: 10.1007/BF00381938
Renaud S. M., Thinh L.-V., Lambrinidis G., Parry D. L. (2002). Effect of temperature on growth, chemical composition and fatty acid composition of tropical Australian microalgae grown in batch cultures. Aquaculture 211 (1), 195–214. doi: 10.1016/S0044-8486(01)00875-4
Román M., Román S., Vázquez E., Troncoso J., Olabarria C. (2020). Heatwaves during low tide are critical for the physiological performance of intertidal macroalgae under global warming scenarios. Sci. Rep. 10 (1), 21408. doi: 10.1038/s41598-020-78526-5
Schmid M., Fernández P. A., Gaitán-Espitia J. D., Virtue P., Leal P. P., Revill A. T., et al. (2020). Stress due to low nitrate availability reduces the biochemical acclimation potential of the giant kelp Macrocystis pyrifera to high temperature. Algal. Res. 47, 101895. doi: 10.1016/j.algal.2020.101895
Schmid M., Guihéneuf F., Nitschke U., Stengel D. B. (2021). Acclimation potential and biochemical response of four temperate macroalgae to light and future seasonal temperature scenarios. Algal. Res. 54, 102190. doi: 10.1016/j.algal.2021.102190
Schmid M., Guihéneuf F., Stengel D. B. (2014). Fatty acid contents and profiles of 16 macroalgae collected from the Irish Coast at two seasons. J. Appl. Phycol. 26 (1), 451–463. doi: 10.1007/s10811-013-0132-2
Schmid M., Guihéneuf F., Stengel D. B. (2017). Ecological and commercial implications of temporal and spatial variability in the composition of pigments and fatty acids in five Irish macroalgae. Mar. Biol. 164 (8), 158. doi: 10.1007/s00227-017-3188-8
Simons B., Kauhanen D., Sylvänne T., Tarasov K., Duchoslav E., Ekroos K. (2012). Shotgun lipidomics by sequential precursor ion fragmentation on a hybrid quadrupole time-of-flight mass spectrometer. Metabolites 2 (1), 195–213. doi: 10.3390/metabo2010195
Simopoulos A. P. (2002). The importance of the ratio of omega-6/omega-3 essential fatty acids. Biomed. Pharmacother. 56 (8), 365–379. doi: 10.1016/S0753-3322(02)00253-6
Skrzypczyk V. M., Hermon K. M., Norambuena F., Turchini G. M., Keast R., Bellgrove A. (2019). Is Australian seaweed worth eating? Nutritional and sensorial properties of wild-harvested Australian versus commercially available seaweeds. J. Appl. Phycol. 31 (1), 709–724. doi: 10.1007/s10811-018-1530-2
Smale D. A. (2020). Impacts of ocean warming on kelp forest ecosystems. New Phytol. 225 (4), 1447–1454. doi: 10.1111/nph.16107
Stengel D. B., Connan S., Popper Z. A. (2011). Algal chemodiversity and bioactivity: Sources of natural variability and implications for commercial application. Biotechnol. Adv. 29 (5), 483–501. doi: 10.1016/j.bioteChadv.2011.05.016
Strain E. M. A., Thomson R. J., Micheli F., Mancuso F. P., Airoldi L. (2014). Identifying the interacting roles of stressors in driving the global loss of canopy-forming to mat-forming algae in marine ecosystems. Global Change Biol. 20 (11), 3300–3312. doi: 10.1111/gcb.12619
Straub S. C., Wernberg T., Marzinelli E. M., Vergés A., Kelaher B. P., Coleman M. A. (2021). Persistence of seaweed forests in the anthropocene will depend on warming and marine heatwave profiles. J. Phycol. 58. doi: 10.1111/jpy.13222
Straub S. C., Wernberg T., Thomsen M. S., Moore P. J., Burrows M. T., Harvey B. P., et al. (2019). Resistance, extinction, and everything in between – The diverse responses of seaweeds to marine heatwaves. Front. Mar. Sci. 6 (763). doi: 10.3389/fmars.2019.00763
Teagle H., Hawkins S. J., Moore P. J., Smale D. A. (2017). The role of kelp species as biogenic habitat formers in coastal marine ecosystems. J. Exp. Mar. Biol. Ecol. 492, 81–98. doi: 10.1016/j.jembe.2017.01.017
Toth G. B., Pavia H. (2007). Induced herbivore resistance in seaweeds: a meta-analysis. J. Ecol. 95 (3), 425–434. doi: 10.1111/j.1365-2745.2007.01224.x
Valentine R. C., Valentine D. L. (2004). Omega-3 fatty acids in cellular membranes: a unified concept. Prog. Lipid Res. 43 (5), 383–402. doi: 10.1016/j.plipres.2004.05.004
Van Den Hoek C. (1982). The distribution of benthic marine algae in relation to the temperature regulation of their life histories. Biol. J. Linn. Soc. 18 (2), 81–144. doi: 10.1111/j.1095-8312.1982.tb02035.x
Van den Hoek C., Breeman A. M., Stam W. T. (1990). “The geographic distribution of seaweed species in relation to temperature: Present and past,” in Expected Effects of Climatic Change on Marine Coastal Ecosystems. Eds. Beukema J. J., Wolff W. J., Brouns J. J. W. M. (Dordrecht: Springer Netherlands), 55–67.
Vanderklift M. A., Wernberg T. (2010). Stable isotopes reveal a consistent consumer–diet relationship across hundreds of kilometres. Mar. Ecol. Prog. Ser. 403, 53–61. doi: 10.3354/meps08484
Veenhof R. J., Champion C., Dworjanyn S. A., Wernberg T., Minne A. J. P., Layton C., et al. (2022). “Kelp gametophytes in changing oceans,” in Oceanography and Marine Biology: An Annual Review, Vol. 60 . Eds. Hawkins S. J., Allcock A. L., Bates A. E., Byrne M., Evans A. J., Firth L. B., Lemasson A. J., Lucas C., Marzinelli E. M., Mumby P. J., Russell B. D., Sharples J., Smith I. P., Swearer S. E., Todd P. A. (Taylor & Francis), 335–372. Available at: https://www.taylorfrancis.com/chapters/oa-edit/10.1201/9781003288602-7/kelp-gametophytes-changing-oceans-reina-veenhof-curtis-champion-symon-dworjanyn-thomas-wernberg-antoine-minne-cayne-layton-john-bolton-daniel-reed-melinda-coleman.
Vergés A., Doropoulos C., Malcolm H. A., Skye M., Garcia-Pizá M., Marzinelli E. M., et al. (2016). Long-term empirical evidence of ocean warming leading to tropicalization of fish communities, increased herbivory, and loss of kelp. Proc. Natl. Acad. Sci. 113 (48), 13791–13796. doi: 10.1073/pnas.1610725113
Vergés A., McCosker E., Mayer-Pinto M., Coleman M. A., Wernberg T., Ainsworth T., et al. (2019). Tropicalisation of temperate reefs: Implications for ecosystem functions and management actions. Funct. Ecol. 33 (6), 1000–1013. doi: 10.1111/1365-2435.13310
Vergés A., Steinberg P. D., Hay M. E., Poore A. G. B., Campbell A. H., Ballesteros E., et al. (2014). The tropicalization of temperate marine ecosystems: climate-mediated changes in herbivory and community phase shifts. Proc. R. Soc. B: Biol. Sci. 281 (1789), 20140846. doi: 10.1098/rspb.2014.0846
Verma P., Kumar M., Mishra G., Sahoo D. (2017). Multivariate analysis of fatty acid and biochemical constitutes of seaweeds to characterize their potential as bioresource for biofuel and fine chemicals. Bioresour. Technol. 226, 132–144. doi: 10.1016/j.biortech.2016.11.044
Vranken S., Wernberg T., Scheben A., Severn-Ellis A. A., Batley J., Bayer P. E., et al. (2021). Genotype–Environment mismatch of kelp forests under climate change. Mol. Ecol. 30 (15), 3730–3746. doi: 10.1111/mec.15993
Wang Z., Benning C. (2012). Chloroplast lipid synthesis and lipid trafficking through ER-plastid membrane contact sites. Biochem. Soc. Trans. 40 (2), 457–463. doi: 10.1042/BST20110752
Wanless S., Harris M. P., Redman P., Speakman J. R. (2005). Low energy values of fish as a probable cause of a major seabird breeding failure in the North Sea. Mar. Ecol. Prog. Ser. 294, 1–8. doi: 10.3354/meps294001
Wells M. L., Potin P., Craigie J. S., Raven J. A., Merchant S. S., Helliwell K. E., et al. (2017). Algae as nutritional and functional food sources: revisiting our understanding. J. Appl. Phycol. 29 (2), 949–982. doi: 10.1007/s10811-016-0974-5
Wernberg T. (2021). “Marine heatwave drives collapse of kelp forests in Western Australia,” in Ecosystem Collapse and Climate Change. Eds. Canadell J. G., Jackson R. B. (Cham: Springer International Publishing), 325–343.
Wernberg T., Bennett S., Babcock R. C., de Bettignies T., Cure K., Depczynski M., et al. (2016a). Climate-driven regime shift of a temperate marine ecosystem. Science 353 (6295), 169–172. doi: 10.1126/science.aad8745
Wernberg T., Coleman M. A., Babcock R. C., Bell S. Y., Bolton J. J., Connell S. D., et al. (2019). “Chapter 6 biology and ecology of the globally significant kelp Ecklonia radiata,” in Oceanography and Marine Biology, Vol. 57). (Taylor & Francis). Available at: https://library.oapen.org/handle/20.500.12657/24721.
Wernberg T., de Bettignies T., Joy B. A., Finnegan P. M. (2016b). Physiological responses of habitat-forming seaweeds to increasing temperatures. Limnol. Oceanogr. 61 (6), 2180–2190. doi: 10.1002/lno.10362
Wernberg T., Russell B. D., Thomsen M. S., Gurgel C.F. D., Bradshaw C. J.A., Poloczanska E. S., et al. (2011). Seaweed communities in retreat from ocean warming. Curr. Biol. 21 (21), 1828–1832. doi: 10.1016/j.cub.2011.09.028
Wernberg T., Thomsen M. S., Connell S. D., Russell B. D., Waters J. M., Zuccarello G. C., et al. (2013a). The footprint of continental-scale ocean currents on the biogeography of seaweeds. PloS One 8 (11), e80168. doi: 10.1371/journal.pone.0080168
Wernberg T., Thomsen M. S., Kotta J. (2013b). Complex plant–herbivore–predator interactions in a brackish water seaweed habitat. J. Exp. Mar. Biol. Ecol. 449, 51–56. doi: 10.1016/j.jembe.2013.08.014
Keywords: Ecklonia radiata, marine heatwaves, nutritional quality, ocean warming, Sargassum linearifolium, species distributions, fatty acids
Citation: Shalders TC, Champion C, Benkendorff K, Davis T, Wernberg T, Morris S and Coleman MA (2023) Changing nutritional seascapes of kelp forests. Front. Mar. Sci. 10:1197468. doi: 10.3389/fmars.2023.1197468
Received: 31 March 2023; Accepted: 07 November 2023;
Published: 21 November 2023.
Edited by:
George Jackson, Loma Linda University, United StatesReviewed by:
Lydia Ladah, Center for Scientific Research and Higher Education in Ensenada (CICESE), MexicoPatti Virtue, University of Tasmania, Australia
Copyright © 2023 Shalders, Champion, Benkendorff, Davis, Wernberg, Morris and Coleman. This is an open-access article distributed under the terms of the Creative Commons Attribution License (CC BY). The use, distribution or reproduction in other forums is permitted, provided the original author(s) and the copyright owner(s) are credited and that the original publication in this journal is cited, in accordance with accepted academic practice. No use, distribution or reproduction is permitted which does not comply with these terms.
*Correspondence: Tanika C. Shalders, dGFuaWthLnNoYWxkZXJzQGdtYWlsLmNvbQ==