- 1Integrative Science Center of Germplasm Creation in Western China (CHONGQING) Science City & Southwest University, Chongqing, China
- 2Key Laboratory of Freshwater Fish Reproduction and Development (Ministry of Education), Key Laboratory of Aquatic Science of Chongqing, Southwest University, Chongqing, China
- 3School of Life Sciences, Southwest University, Chongqing, China
The distribution pattern of species is determined by the environment and their adaptability to the environment. Qinghai-Tibet Plateau has become a natural laboratory for studying adaptive evolution due to its extreme environmental characteristics such as low temperature, low oxygen, high salinity and high ultraviolet radiation (UVR). Fish are sensitive to the environmental stress, so they are ideal materials for studying high-altitude adaptation of animals. Previous studies have mainly focused on the adaptability of plateau species, but the reasons why plain species cannot spread to the plateau have been ignored. In this study, stress experiments and histological experiments were used to compare the tolerance of six Barbini fishes (family: Cyprinidae) distributed at different altitudes and regions to low temperature, low oxygen, salinity and UVR. Results showed that the tolerance of fishes to high-altitude environmental stress factors was closely related to the environmental stress of their main habitats. The high-altitude fish Gymnocypris eckloni had strong tolerance to all stress factors, while the other five fishes from middle and low altitudes could not adapt to single or multiple stress factors, with significant interspecific differences. Among these factors, middle- and low-altitude fishes showed common low tolerance to UVR, suggesting that high UVR, the factor lacking at low altitude areas, plays an important role. Moreover, during the uplift of the Qinghai-Tibet Plateau, Schizothorax fish disappeared from the middle of the plateau. We speculate that this was caused by its intolerance to the increasingly extreme plateau environment, especially salinity.
1 Introduction
Adaptation refers to the process of survival and reproduction in which the morphological structure and physiological function of organisms are suitable for certain environmental conditions (Brandon, 1978). In nature, the environment is constantly changing, and organisms respond to various environmental pressures (Bijlsma and Loeschcke, 2012). For individuals, they can regulate their phenotypes through phenotypic plasticity, thereby increasing their tolerance to environmental stress (West-Eberhard, 2003; Pigliucci, 2005; Ghalambor et al., 2015); for populations, specific environmental pressures will accelerate the corresponding genetic variation (Rosenberg, 2001; Galhardo et al., 2007; Martincorena et al., 2012), and will also play a selective role in the genetic variation of populations (Haldane, 1932; Charlesworth et al., 2017). These processes together constitute the adaptive evolution of organisms (Bijlsma and Loeschcke, 2012), and the distribution pattern of species is gradually formed in these processes (Zhao Z. et al., 2022).
Qinghai-Tibet Plateau, with an average altitude of more than 4500 meters, is known as the “roof of the world” and the “third pole” (Anand et al., 1986; Qiu, 2008). It is the highest plateau in the world and a hot spot for studying adaptive evolution (Qu et al., 2013; Mao et al., 2021; Li et al., 2022). Under the collision and extrusion of Indian Ocean plate and Eurasian plate, the plateau gradually uplifted during the Neogene (23 ~ 2.6 Ma) (Tapponnier et al., 2001; Su et al., 2019; Spicer et al., 2021), and the rising altitude brought severe cold, low oxygen, strong UVR and drought (Machta and Hughes, 1970; Li and Fang, 1999; Zhou and Zhang, 2005; Liu W. et al., 2009; Liu Y. et al., 2009). Plateau animals formed their own unique adaptation mechanisms to resist the extreme environment, and the distinct species distribution pattern of the Qinghai-Tibet Plateau and its surroundings was also formed (Wang X. et al., 2015).
As poikilothermal animals living in water, fish are very sensitive to water temperature, dissolved oxygen and salinity (Scholander et al., 1953; Lindsey, 1966; Kültz, 2015). Moreover, the lack of hair on the surface of fish causes direct effect of light on the skin, making fish more vulnerable to UVR (Hunter et al., 1979; Hunter et al., 1981). Therefore, fish is ideal material for studying high-altitude adaptation of animals. There are unique fish groups distributed on the Qinghai-Tibet Plateau, among which the Schizothoracinae fishes (family: Cyprinidae) are the most representative. For example, Gymnocypris eckloni lives in the waters with an altitude of more than 3000 m. These fish experience cold and hypoxic water, meanwhile, high-altitude clear rivers and lakes have low UVR attenuation (Bullock, 1988; Liu et al., 2021), and fish need to face more intense UVR. In addition, drought leads to a large amount of salt deposition in the water bodies, which makes the water on the Qinghai-Tibet Plateau more salty (Liu W. et al., 2009). It can be seen that the stress faced by plateau fish is multifaceted, and single or multiple plateau stress factors may cause plain fish to fail to adapt to the plateau environment.
Previous studies have mainly focused on the high-altitude adaptability of plateau species, but little is known about the reasons why plain species cannot adapt to the plateau, because it is not clear which plateau stress factors play a major role (Cao et al., 2008; Yang et al., 2015; Xu et al., 2016; Chen et al., 2020). Under such unclear conditions, it may cause some studies to blindly select plain control species. For example, Cyprinus carpio with strong adaptability has strong tolerance to low temperature and low oxygen, for some plateau stress factors also exist in the plain areas. If it is selected as a control to study the adaptability of plateau fish to low temperature or low oxygen, it may cause the failure of experimental design. In addition, previous studies on the tolerance of plateau fish often focused on a single stress factor, and mostly used genetic analysis methods (Xu et al., 2016; Yang et al., 2020; Jin et al., 2022), lacking of stress tolerance experiments to verify. Therefore, the comparison of multi-group and multi-factor stress experiments can help us better understand the adaptation process of plateau fish and the causes of distribution pattern, so as to answer “why middle- and low-altitude fish cannot reach the plateau”.
In our study, we compared the tolerance of six Barbini fishes (family: Cyprinidae) distributed at different altitudes and regions (Figure 1) to low temperature, low oxygen, salinity and UVR. Through experiments, we want to answer two scientific questions: (1) What are the differences in the tolerance of fish at different altitudes to different high-altitude environmental stress factors? (2) What factors limit the spread of middle- and low-altitude fish to the plateau? Single factor or multiple factors?
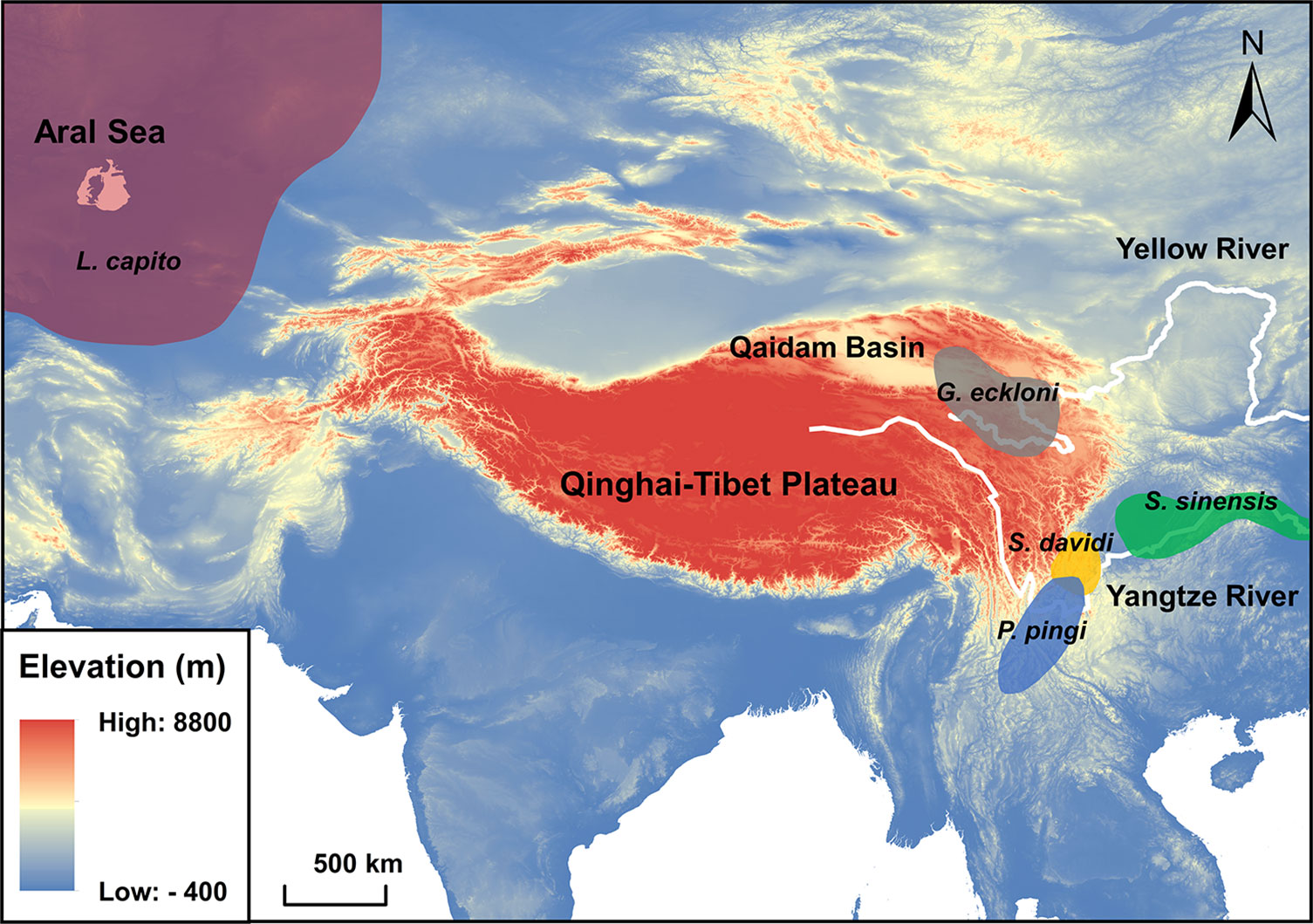
Figure 1 Main distribution of the wild populations of experimental fishes. Grey area: Gymnocypris eckloni. Orange area: Schizothorax davidi. Blue area: Percocypris pingi. Green area: Spinibarbus sinensis. Red area: Luciobarbus capito. Cyprinus carpio is widely distributed in the wild, and its distribution is not shown in the figure.
2 Materials and Methods
2.1 Ethics statement
All the animal procedures were conducted with the approval of the Committee of Laboratory Animal Experimentation at Southwest University, Chongqing, China and were in full compliance with the Committee’s guidelines [protocol number (2014)25].
2.2 Experimental animals
Gymnocypris eckloni (subfamily: Schizothoracinae) is mainly distributed in the upper reaches of the Yellow River and Qaidam Basin (average altitude > 3000 m) on the Qinghai-Tibet Plateau. Schizothorax davidi (subfamily: Schizothoracinae) and Percocypris pingi (subfamily: Barbinae) live in the middle altitude (average altitude: 1000 ~ 2000 m) of the upper reaches of the Yangtze River. Spinibarbus sinensis (subfamily: Barbinae), Luciobarbus capito (subfamily: Barbinae) and Cyprinus carpio (subfamily: Cyprininae) come from low altitude plains (average altitude < 1000 m) (Ding, 1994). These six tetraploid fishes with close phylogenetic relationships experience different types and intensities of environmental stress in their respective habitats, which is an ideal model to explore the relationship between fish habitat characteristics and their stress tolerance (Wang et al., 2016; Yang et al., 2022). The experimental fishes with body length of 10 ± 2 cm are artificially bred offspring of the wild population and are in the juvenile stage to exclude the effects of gender (Júnior et al., 2012). They were domesticated in the laboratory circulating aquariums (60×32×28 cm) holding 50 L of water each at 14 ± 1°C on a 12 L: 12 D photoperiod where they were fed twice a day. Before experiments, the experimental fishes were fasted for 24 h (Figueroa et al., 2000).
2.3 Stress experiments
The response of fish to chronic stress and acute stress is different (Barton and Iwama, 1991; Wendelaar Bonga, 1997; Barcellos et al., 2006), so for the factors of low temperature, low oxygen and salinity, chronic stress experiments and acute stress experiments were set up to more accurately reflect the tolerance of fishes to high-altitude environmental stress. In these experiments, the loss of balance of fish was used as a sign of reaching the tolerance limit (Belanger et al., 1986), the unbalanced fish were kept in normal water and no longer participated in subsequent experiments. For the UVR exposure experiment, we set the UVR intensity according to the actual UVR intensity on the Qinghai-Tibet Plateau. All experiments were carried out in Chongqing, China (250 m above sea level), the low temperature, low oxygen and salinity experiments were carried out from November to December 2020, and the UVR experiments were carried out from March to April 2021.
2.3.1 Low temperature
2.3.1.1 Chronic low temperature experiment
Two 65 L white breeding buckets (with a bottom diameter of 34 cm) were filled with about 50 L dechlorinated tap water and put into the refrigerator (Haier BC/BD-519HCM), and the water temperature was reduced to 12°C as the initial temperature. The oxygenating heads were added to provide sufficient oxygen. Then, we put 10 fish of each specie into 2 buckets (5 fish per bucket) and closed the door of the refrigerator so that the water temperature gradually decreased at a certain rate (decreased by about 2°C per minute in the first 5 minutes, about 1°C in 5-6 minutes, and finally stabilized at 1 ± 0.1°C), and the change of water temperature was monitored with a thermometer (Figure 2A). During the experiment, we opened the refrigerator door every 10 min to observe, recorded water temperature and fished out the unbalanced fish, then quickly closed the refrigerator door.
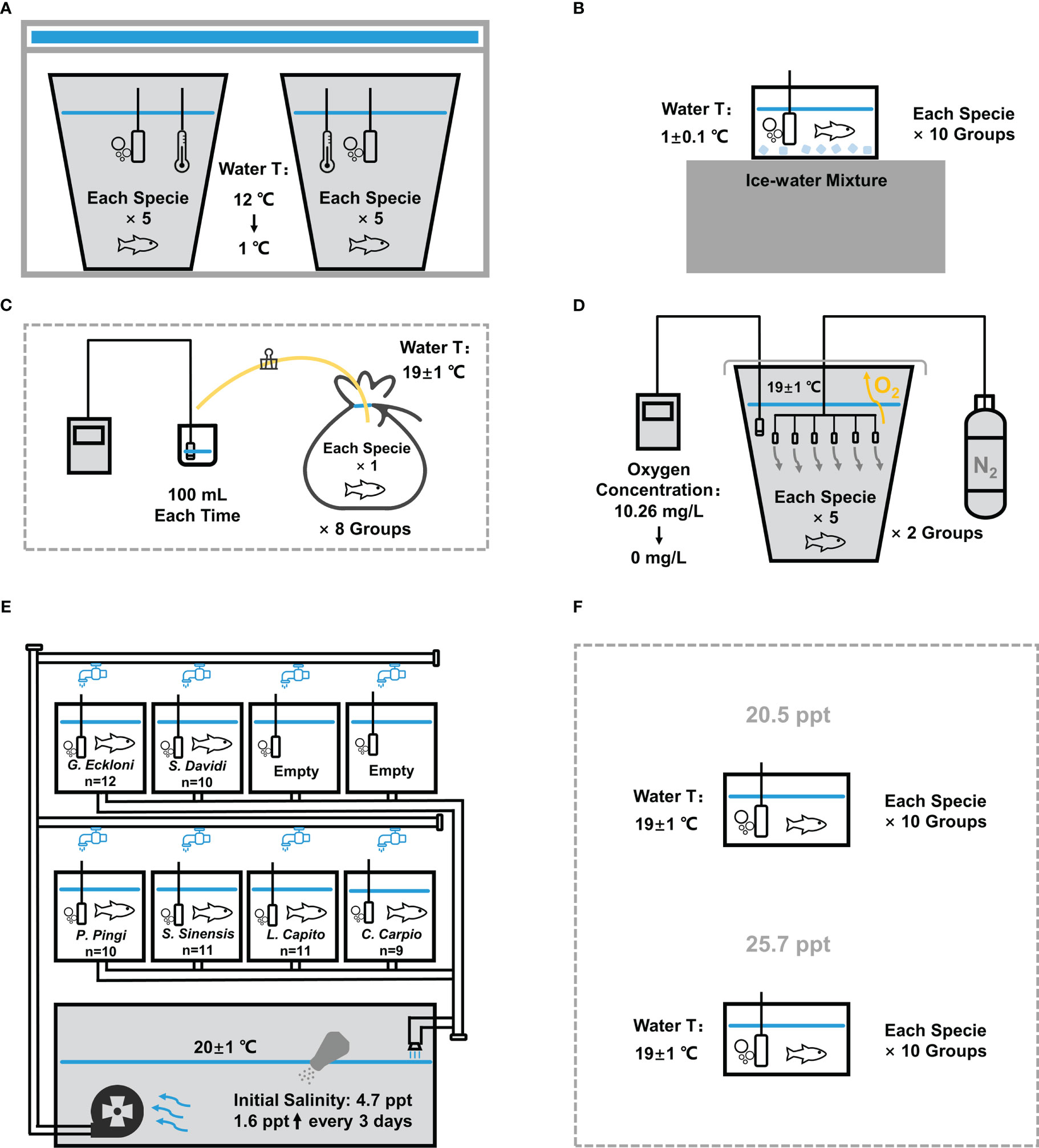
Figure 2 Experimental device diagram of stress experiments. (A) Chronic low temperature experiment. (B) Acute low temperature experiment. (C) Chronic hypoxia experiment. (D) Acute hypoxia experiment. (E) Chronic salinity experiment. (F) Acute salinity experiment. The experimental device of the UVR exposure experiment is shown in our previous study.
2.3.1.2 Acute low temperature experiment
The fish tank (25×15×20 cm) was filled with 5 L of dechlorinated ice-water mixture maintained at 1 ± 0.1°C, and an oxygenating head was added to provide sufficient oxygen (Figure 2B). We put one fish at a time, recorded the time when the fish lost balance, and fished it out. The water was replaced and the water temperature was adjusted each time.
2.3.2 Low oxygen
2.3.2.1 Chronic hypoxia experiment
Eight transparent PE plastic bags were packed into 5 L dechlorinated tap water at 19 ± 1°C (between the water temperature of warm water fish and cold water fish). We put one fish of each specie into each plastic bag, inserted a rubber tube clamped with a clip. Then, we discharged the air in the plastic bags to keep the same volume of each bag, sealed the bags, and observed the activity of fish. When the fish was out of balance, we opened the clamp on the rubber tube, and extruded 100 mL water from the plastic bag for measurement. The dissolved oxygen concentration was measured with a dissolved oxygen meter (HACH HQd, HACH, America) (Figure 2C), and the fish number and oxygen concentration were recorded.
2.3.2.2 Acute hypoxia experiment
A 65 L white breeding bucket was filled with about 50 L dechlorinated tap water at 19 ± 1°C. The experiment was carried out in two times, with 5 fish of each specie each time. After 1 h adaptation, the fish were treated with low oxygen by nitrogen replacement method (Zhang et al., 2014): six aeration heads connected to the nitrogen tank were evenly put into the bucket to introduce nitrogen, the dissolved oxygen meter probe was put into the water, and the bucket was covered with plastic film to reduce the contact between water and air (Figure 2D). In the first hour, the oxygen concentration was reduced from 10.26 mg/L to 2.5 mg/L; after half an hour, it gradually decreased to 1.5 mg/L; subsequently, it decreased by 0.1 mg/L every 10 min; after reaching 0.5 mg/L, it decreased by 0.1 mg/L every 20 min until it decreased to 0. During the process, the corresponding oxygen concentration of each fish was recorded when it lost balance, and the unbalanced fish was fished out.
2.3.3 Salinity
2.3.3.1 Chronic salinity experiment
The circulating water system (with 8 aquariums (60×32×28 cm) holding 50 L of water each and a circulating flume with 230 L capacity) was filled with about 630 L dechlorinated tap water at 20 ± 1°C, and each aquarium was equipped with an oxygenating head. Six species were placed in six aquariums of the system to adapt for 2 days. After that, we added 3 kg NaCl to the system with a salinometer (HACH HQd, HACH, America) measured a salinity of 4.7 ppt, and 1 kg NaCl was added every 3 days to increase the salinity of water in the system by about 1.6 ppt each time (Figure 2E). During the experiment, we checked the fish every day, fished out the unbalanced fish and recorded the salinity at that time.
2.3.3.2 Acute salinity experiment
According to the result of chronic experiment, the acute experiment was divided into two higher salinity levels of 20.5 ppt and 25.7 ppt, which also met the salinity range set in previous studies. (Sun et al., 1995; Karsiotis et al., 2012; Ghosh et al., 2019). Two fish tanks (25×15×20 cm) equipped with oxygenating heads were filled with 5 L of dechlorinated tap water at 19 ± 1°C, and salt was added to make the salinity in the two tanks reach 20.5 ppt and 25.7 ppt (Figure 2F). We put one fish into each of the two fish tanks at a time, recorded the tolerance time, and then fished it out. Before each species replacement, we replaced the water, and re-adjusted the salinity and temperature.
2.3.4 UVR
In our previous studies, we have compared the UVR tolerance of Gymnocypris eckloni, Schizothorax davidi, Spinibarbus sinensis, Luciobarbus capito and Cyprinus carpio (Gu et al., 2022a). In this study, we supplemented the experiment of Percocypris pingi with the same experimental method, and the specific method can refer to our previous research. The brief method is as follows:
2.3.4.1 UVR exposure
Twelve Percocypris pingi with body length of 10 ± 2 cm were selected and divided into experimental group (n=6) and control group (n=6), which were placed in two 65 L white breeding buckets respectively. The water kept flowing and updated, and the water temperature was controlled at 16 ± 1°C. The bucket of the experimental group was placed under a 275 W full-spectrum solar lamp (Sparkzoo, China), and the UVR intensity at the center of the water surface was about 0.108 MJ/m2/h (UVB/UVA≈0.09). The experimental group was exposed to the solar lamp for 12 hours every day for 7 days from 7:00 to 19:00, and the UVA and UVB daily dose was approximately 1.30×103 kJ/m2/d. In contrast, the bucket of the control group was covered with a transparent acrylic sheet, which resulted in nearly undetectable UVR.
2.3.4.2 Histology
The experimental fishes were euthanized with an overdose of MS-222 (tricaine methane sulfonate, 500 mg/L) on the 7th night. The lateral skin tissue was collected, fixed in 4% paraformaldehyde for approximately 12 hours at 4 °C and then transferred to a 70% alcohol solution for preservation. Traditional paraffin sections (6 μm) were obtained using a microtome (Leica 2245, Leica, Germany), and were stained with an AB-PAS staining kit (DG1014-6×100 mL, Dingguo). All sections were observed using a microscope (Nikon SMZ25, Japan), a complete and representative section was selected for each sample, and three random images of this section were photographed (100×) for measurement.
2.3.4.3 Damage levels
The damage caused by UVR exposure was classified into 0-5 levels (Bullock, 1988; Gu et al., 2022a).
2.4 Phylogenetic analysis
The complete mitochondrial genome sequences of six experimental fishes was downloaded from GenBank (https://www.ncbi.nlm.nih.gov/nuccore/), and the DNA sequences were then aligned using MAFFT Online Service (Katoh et al., 2019). The maximum likelihood phylogenetic tree was constructed using the default setting of IQ-TREE with default models (Trifinopoulos et al., 2016).
2.5 Statistical analyses
Data was analyzed by One-Way ANOVA and expressed as the mean ± standard deviation (SD) with GraphPad prism 9.0 software (GraphPad, USA). The Duncan ‘s range test was used to analyze the differences in tolerance among species with IBM SPSS Statistics (version 27.0, Armonk, New York, United States), and significant differences (P < 0.05) were expressed by different lowercase letters. The correlation between fish habitat stress levels and their stress tolerance was analyzed using Spearman rank correlation with IBM SPSS Statistics. All graphs were generated with GraphPad prism 9.0 software. The images of sections were measured with Photoshop 2020 (Adobe, San Jose, CA, USA).
3 Results
3.1 Low temperature
3.1.1 Chronic low temperature experiment
Spinibarbus sinensis was the first to lose balance when the water temperature was reduced to 3.3°C at 4.33 h. At 4.75 h (2.1°C), all individuals reached the tolerance limit, and the average tolerance time was 4.54 ( ± 0.16) h. The tolerance of Percocypris pingi is similar to that of Spinibarbus sinensis, they successively lost their balance during the 4.5 h (2.7°C) to 5.17 h (1.9°C) of the experiment, and the average tolerance time was 4.72 ( ± 0.25) h. After 5.5 h of the experiment, the water temperature remained stable at 1 ± 0.1°C. At 6 h, intolerance began to appear in Schizothorax davidi and Luciobarbus capito, individuals of the two fishes gradually lost their balance in the following 6 h, with an average tolerance time of 6.27 ( ± 0.24) h and 7.01 ( ± 1.91) h, respectively. When the experiment continued to 13 h, all individuals of Gymnocypris eckloni and Cyprinus carpio could still swim normally and did not reach the tolerance limit (Figure 3A). Compared with the first four species, they showed stronger tolerance to chronic low temperature.
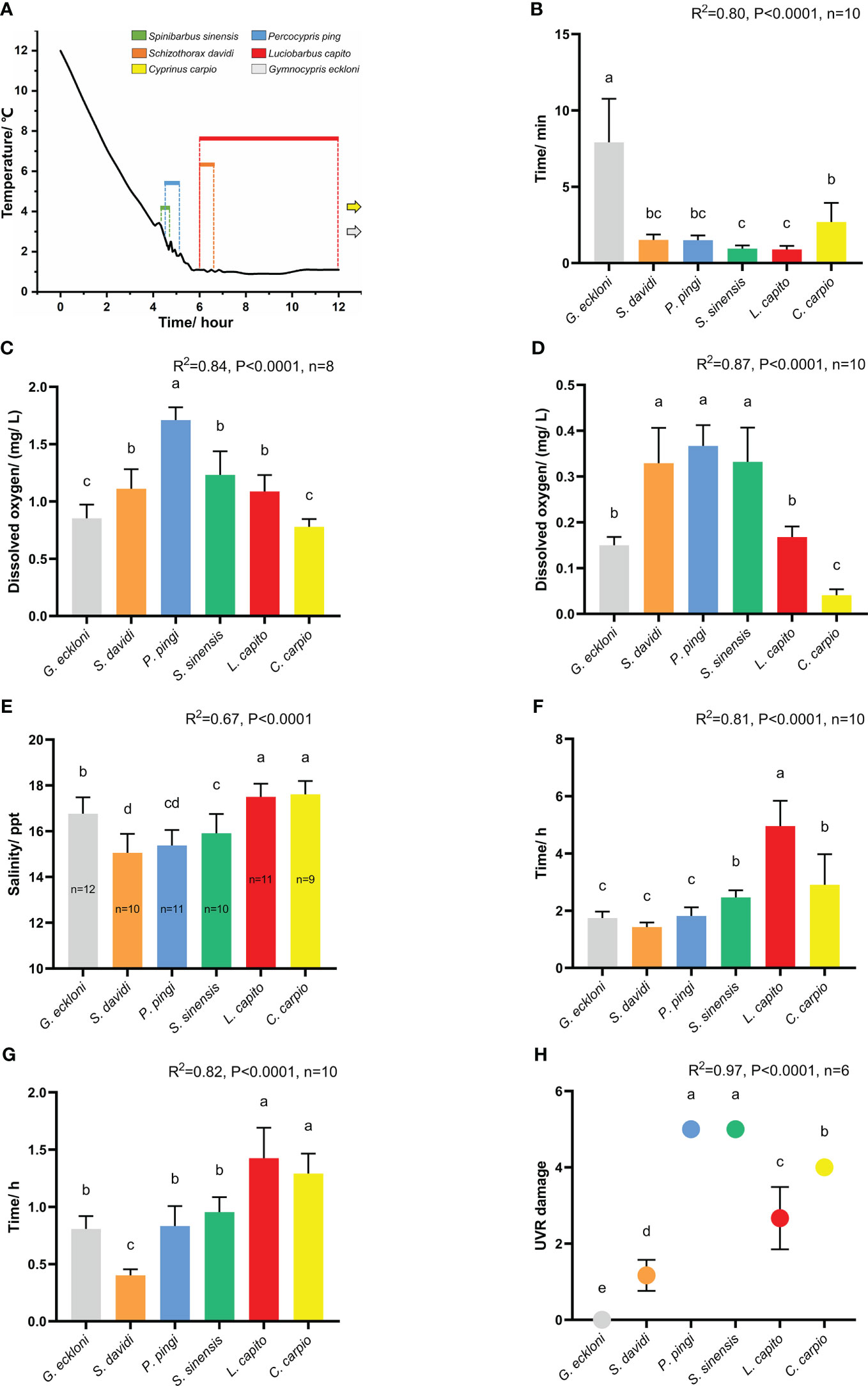
Figure 3 The tolerance of experimental fishes to stress factors in stress experiments. (A) Chronic low temperature experiment (n=10). The black curve indicates temperature change; the colored lines indicate that the experimental fishes lost their balance at the corresponding time; the grey and yellow arrows indicate that Gymnocypris eckloni and Cyprinus carpio are not out of balance in the experiment. (B) Acute low temperature experiment. (C) Chronic hypoxia experiment. (D) Acute hypoxia experiment. (E) Chronic salinity experiment. (F) Acute salinity experiment (20.5 ppt). (G) Acute salinity experiment (25.7 ppt). (H) UVR exposure experiment. The height and whiskers indicate the mean ± SD. Different lowercase letters (a–d) indicate significant differences in Duncan ‘s range test (α=0.05).
3.1.2 Acute low temperature experiment
Compared with the chronic experiment, the tolerance time of all fishes was significantly shortened (P < 0.01). The acute low temperature tolerance of Gymnocypris eckloni (7.91 ± 2.85 min) was significantly stronger than that of Cyprinus carpio (2.69 ± 1.25 min), Schizothorax davidi (1.52 ± 0.36 min), Percocypris pingi (1.50 ± 0.31 min), Spinibarbus sinensis (0.96 ± 0.21 min) and Luciobarbus capito (0.89 ± 0.24 min) (Figure 3B, P < 0.05), and the tolerance time of some Gymnocypris eckloni individuals even exceeded 10 min.
3.2 Low oxygen
3.2.1 Chronic hypoxia experiment
Percocypris pingi first began to lose balance when the dissolved oxygen in the water was reduced to 1.86 mg/L, and the average dissolved oxygen concentration they tolerated was 1.71 ( ± 0.11) mg/L. The chronic hypoxia tolerance of Spinibarbus sinensis (1.23 ± 0.21 mg/L), Schizothorax davidi (1.11 ± 0.17 mg/L) and Luciobarbus capito (1.09 ± 0.14 mg/L) was similar, and slightly stronger than that of Percocypris pingi. The tolerance of Gymnocypris eckloni (0.85 ± 0.12 mg/L) and Cyprinus carpio (0.78 ± 0.07 mg/L) was similar, which was significantly stronger than that of the other four species (Figure 3C, P < 0.05).
3.2.2 Acute hypoxia experiment
Percocypris pingi (0.37 ± 0.05 mg/L), Schizothorax davidi (0.33 ± 0.08 mg/L) and Spinibarbus sinensis (0.33 ± 0.07 mg/L) had weak acute hypoxia tolerance. The adaptability of Gymnocypris eckloni (0.15 ± 0.02 mg/L) to acute hypoxia was similar to that of Luciobarbus capito (0.17 ± 0.02 mg/L), while the tolerance of Cyprinus carpio (0.04 ± 0.01 mg/L) to acute hypoxia stress was significantly stronger than that of the two (Figure 3D, P < 0.05).
3.3 Salinity
3.3.1 Chronic salinity experiment
Salinity has acute toxicological effects on freshwater fish, and there are great differences in salinity tolerance among different species (Kefford et al., 2004; Varsamos et al., 2005; Bachman and Rand, 2008). Under the chronic salinity stress, Schizothorax davidi (15.06 ± 0.83 mg/L), Percocypris pingi (15.38 ± 0.67 mg/L) and Spinibarbus sinensis (15.91 ± 0.85 mg/L) quickly reached the tolerance limit, and the tolerance of the three was slightly different. The chronic salinity tolerance of Gymnocypris eckloni (16.77 ± 0.72 mg/L) was significantly stronger than that of the first three fishes, but weaker than that of Luciobarbus capito (17.50 ± 0.57 mg/L) and Cyprinus carpio (17.61 ± 0.58 mg/L) (Figure 3E, P < 0.05).
3.3.2 Acute salinity experiment
At the salinity level of 20.5 ppt, Schizothorax davidi (1.43 ± 0.16 h), Gymnocypris eckloni (1.75 ± 0.22 h), Percocypris pingi (1.82 ± 0.30 h), Spinibarbus sinensis (2.46 ± 0.25 h) and Cyprinus carpio (2.91 ± 1.07 h) were out of balance at about 2 h. The tolerance time of Luciobarbus capito (4.96 ± 0.88 h) to acute salinity stress at 20.5 ppt level was significantly longer than that of the other five species (Figure 3F, P < 0.05). At the salinity level of 25.7 ppt, all the six species reached the tolerance limit in a very short time, especially Schizothorax davidi (0.40 ± 0.05 h) showed intolerance at 0.33 h of the experiment (Figure 3G). The acute experiment showed different results compared with the chronic experiment, it was speculated that 20.5 ppt exceeded the short-term salt tolerance limit of five species except Luciobarbus capito, while 25.7 ppt exceeded the limit of all species in the experiment. Therefore, fishes with strong tolerance in chronic salinity experiment did not perform better in acute experiment.
3.4 UVR
UVR can cause a series of acute damage to fish, especially the direct damage to skin (Bullock and Coutts, 1985). High-intensity UVR can lead to severe degeneration of fish epidermis, loss of scales, increase of epidermis thickness, rupture of club cells, and reduction, necrosis or shedding of mucous cells (Osman and Harabawy, 2010). In previous experiments, Spinibarbus sinensis (5), Cyprinus carpio (4) and Luciobarbus capito (2.67 ± 0.82) had weak UVR tolerance, Schizothorax davidi (1.17 ± 0.41) was slightly stronger, and Gymnocypris eckloni (0) had the strongest UVR tolerance. In this experiment, all the Percocypris pingi individuals in the experimental group died in the last three days of exposure, and the damage level was 5 (Figures 3H, 4A–L). Only the skin of control group was taken and made into paraffin sections.
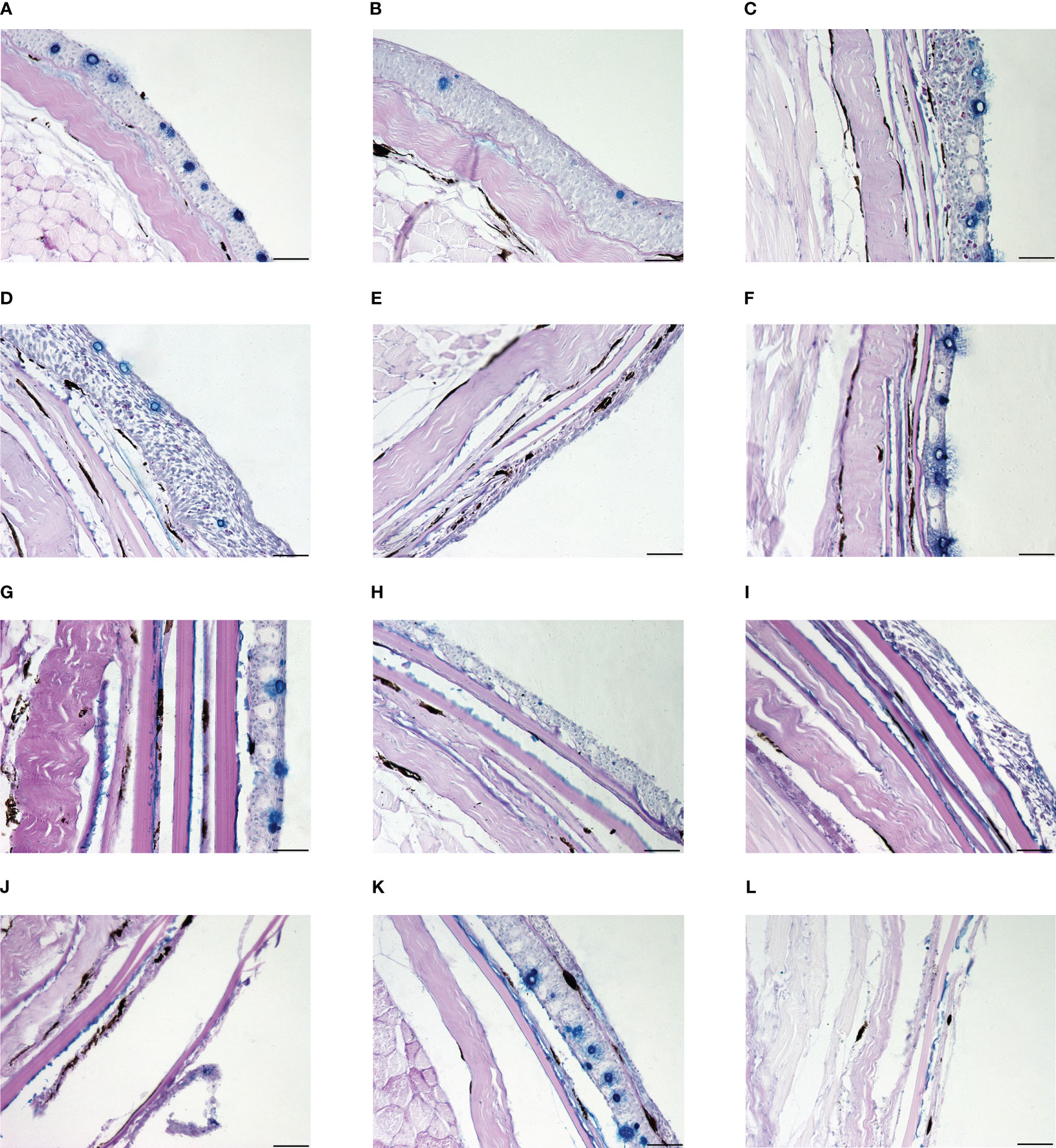
Figure 4 The skin histological structure of experimental fishes in UVR exposure experiment. The scale is 50 μm. (A) Control group of Gymnocypris eckloni. (B) Experimental group of Gymnocypris eckloni (0 level damage). (C) Control group of Schizothorax davidi. (D) Experimental group of Schizothorax davidi (1 level damage). (E) Experimental group of Schizothorax davidi (2 level damage). (F) Control group of Percocypris pingi. (G) Control group of Spinibarbus sinensis. (H) Control group of Luciobarbus capito. (I) Experimental group of Luciobarbus capito (2 level damage). (J) Experimental group of Luciobarbus capito (3 level damage). (K) Control group of Cyprinus carpio. (L) Experimental group of Cyprinus carpio (4 level damage).
4 Discussion
4.1 Factors limiting the spread of low-altitude fishes to the plateau
In the long evolutionary process, the tolerance of animals to environmental stress is compatible with the environment in which they live (Wu et al., 2014; Witt and Huerta-Sánchez, 2019). Qinghai-Tibet Plateau has extreme environmental conditions that are not available in low-altitude plain areas, plateau fish have acquired the ability to resist high-altitude environmental stress during long-term adaptive evolution (Zhuang et al., 2012; Little and Seebacher, 2013; Guan et al., 2014; Kültz, 2015; Mustafa et al., 2015; Sushchik et al., 2018; Nobrega et al., 2019; Evans and Kültz, 2020; Yuan et al., 2020). In this study, the stress experiments of low temperature, low oxygen, salinity and UVR were carried out. As a high-altitude fish, Gymnocypris eckloni showed high tolerance in all stress experiments, including salinity experiments. During the “Gonghe Movement” (0.15 Ma), Qinghai Lake was separated from Yellow River, and Gymnocypris przewalskii (living in the saltwater lake Qinghai Lake) and Gymnocypris eckloni were two groups that differentiated after this event (Zhao et al., 2005; Zhao et al., 2007; Qi et al., 2013). Due to the short differentiation time, most of the genetic similarities between the two are still retained, and some other Gymnocypris fishes are distributed in saltwater lakes of the central Qinghai-Tibet Plateau (e.g., Gymnocypris namensis) (Yuan et al., 2002), which explains the reason why Gymnocypris eckloni living in fresh water has strong salinity tolerance. The experiment results of it also proved that this experimental method can effectively reflect the actual adaptability of fish to plateau stress factors. Interestingly, fishes at middle and low altitudes were not intolerant to all high-altitude environmental stress factors, but were related to their own habitat environment (Supplementary Table, P < 0.05), with large interspecific differences. Luciobarbus capito is an euryhaline fish native to salt lakes in Central Asia, the salinity of the environment in which its wild population lives is the highest among the six Barbini species. Long-term adaptation resulted in its strong resistance to salinity, and it can rapidly regulate its osmotic pressure balance by Na +/K + -ATPase under salinity stress (Geng et al., 2016). However, its tolerance to low temperature, low oxygen and UVR was weak, which limited its diffusion to the plateau. The tolerance of Schizothorax davidi to low temperature and UVR was slightly stronger than that of Spinibarbus sinensis, but its salinity tolerance was the weakest among the six species. As a carnivorous fish, Percocypris pingi has a higher oxygen consumption rate under emergency conditions (Bau and Parent, 2001), and histological sections showed that the density of mucous cells and club cells in its epidermis was higher, while the epithelial cells that play a major role in resisting UVR were thinner (Gu et al., 2022a; Gu et al., 2022b). Therefore, its tolerance to low oxygen and UVR was slightly lower than that of Schizothorax davidi with similar altitude distribution. Cyprinus carpio is a well-known freshwater fish with strong adaptability, it is widely distributed and there are a variety of environmental stress factors in its habitat. They sometimes feed on the larvae of Diptera insects, which are usually produced in anoxic, high-salt polluted water. Furthermore, Cyprinus carpio are also found in the cold waters of northern Eurasia, and they can maintain membrane fluidity by increasing the synthesis of long-chain unsaturated fatty acids (Tiku et al., 1996; Zhao R. et al., 2022). It has acquired the ability to resist low temperature, low oxygen and salinity stress during long-term adaptation, but UVR is also a limiting condition for its diffusion to high altitude (Figure 5).
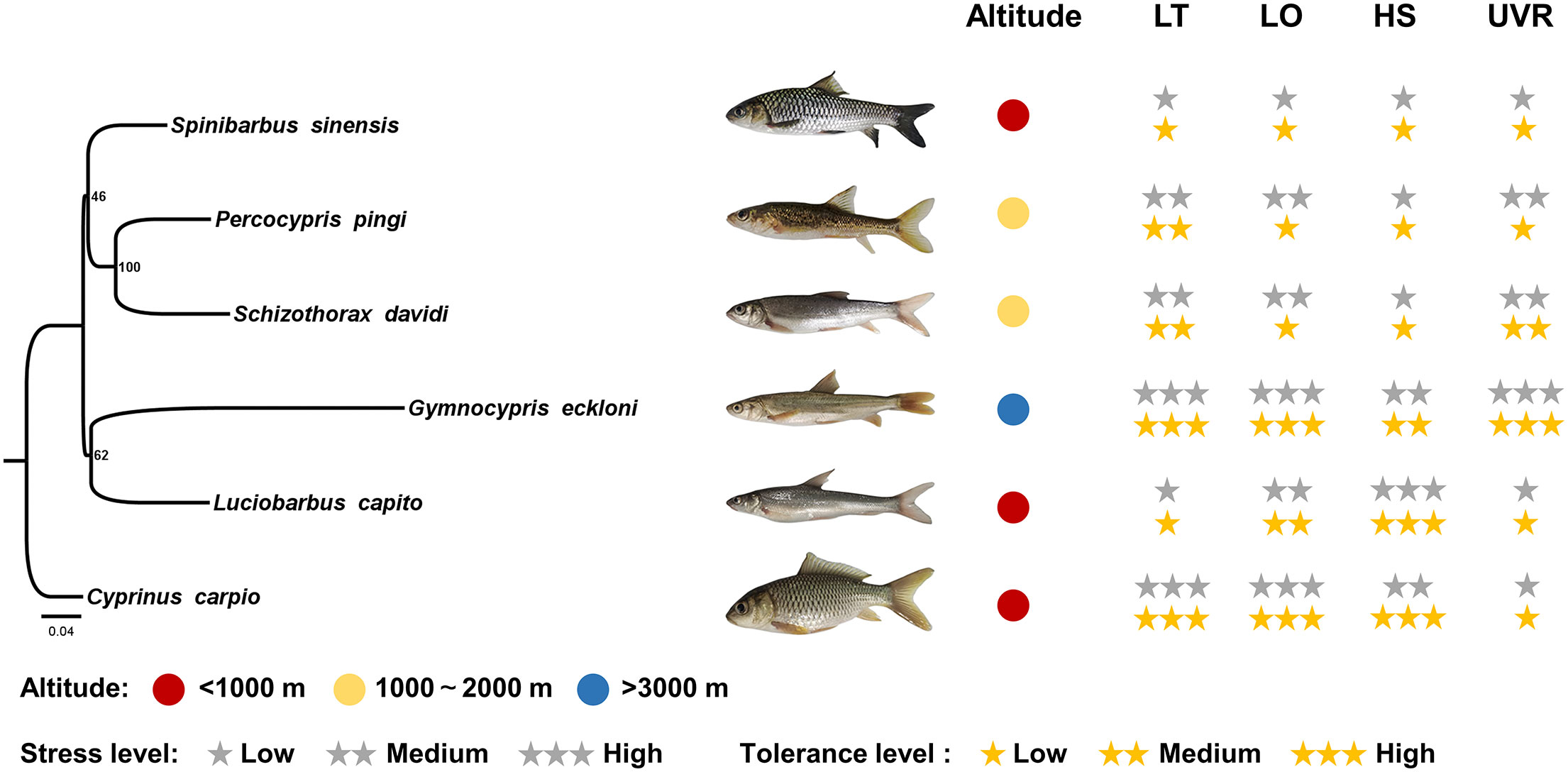
Figure 5 Environmental stress intensity of the habitats and tolerance levels of experimental fishes. LT, low temperature; LO, low oxygen; HS, high salinity. The gray stars indicate the stress intensity of fish habitats, and the yellow stars indicate the tolerance levels of fishes.
For low-altitude fishes, any stress conditions can be fatal, and there are always one or several stress factors that limit their access to the plateau. It is worth noting that in this study, fishes at middle and low altitudes showed common low tolerance to UVR, while Gymnocypris eckloni showed strong UVR tolerance. This suggests that among these high-altitude environmental stress factors, UVR, which is generally lacking in low altitude areas, may play a decisive role.
Low temperature, low oxygen and high salinity stress also exist in plain areas, and low-altitude fishes will also acquire adaptive evolutionary characteristics in the process of resisting to environmental stresses. Therefore, when selecting the plain control species for the studies of fish high-altitude adaptability, in addition to paying attention to its altitude distribution, the adaptability of the control species itself should also be considered.
4.2 Uplift of Qinghai-Tibet Plateau and distribution change of Schizothorax fish
Qinghai-Tibet Plateau has experienced an uplift process of millions of years (Li and Fang, 1999; Tapponnier et al., 2001). In the Paleogene (66 ~ 23 Ma), the central part of the plateau was a deep valley with an average altitude of less than 2300 m, surrounded by a mountain system with an altitude of more than 4000 m (Su et al., 2019). Fossil evidence indicates that during that period, the organisms adapted to the tropical climate lived in the Lunpola Basin in the central Tibetan Plateau (Cyr et al., 2005; Deng et al., 2012; Sun et al., 2014; Su et al., 2019). In the Neogene (23 ~ 2.6 Ma), the central part of the Tibetan Plateau gradually uplifted to about 4600 m above sea level, forming the present Tibetan Plateau (Spicer et al., 2021) (Figure 6).
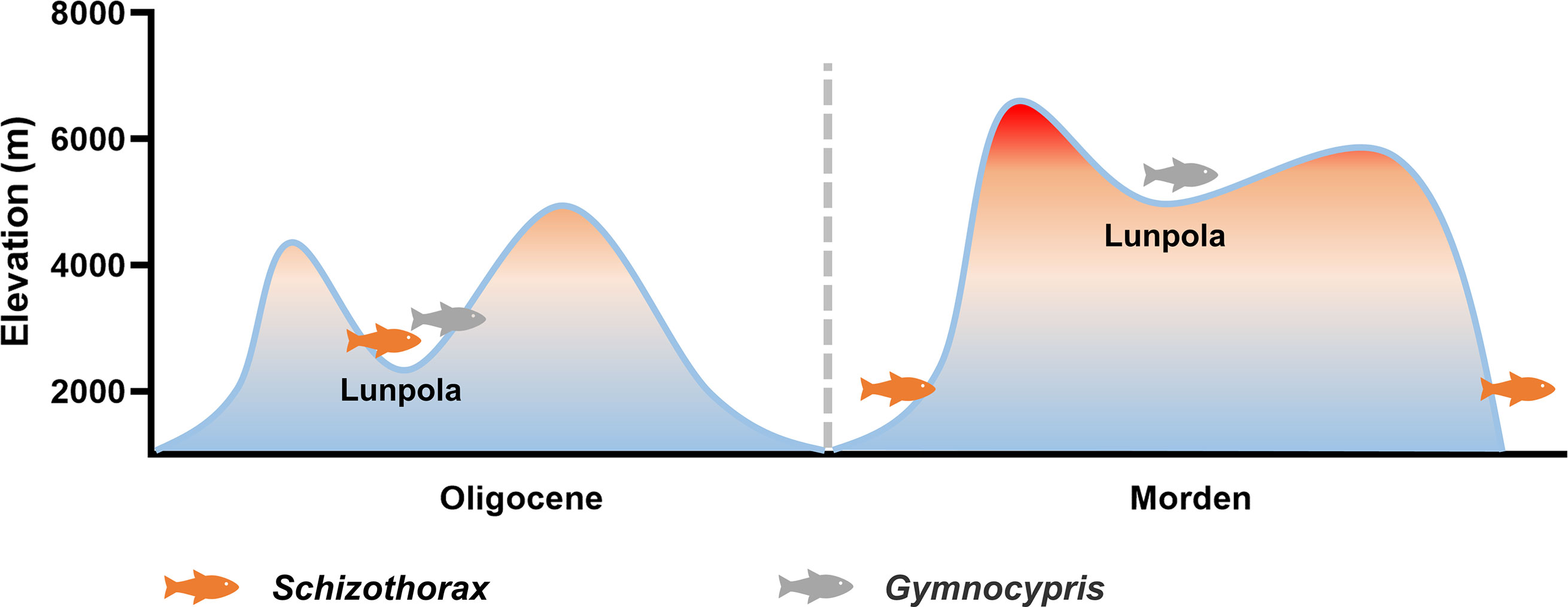
Figure 6 The uplift of Qinghai-Tibet Plateau and the distribution changes of Schizothorax and Gymnocypris fishes since Oligocene.
Schizothoracinae fish is a group mainly distributed in the Qinghai-Tibet Plateau and its surrounding areas. Studies have shown that Schizothoracinae fish evolved from Barbinae (family: Cyprinidae) fish in Tertiary (66 ~ 2.6 Ma). According to the modifications of scales, pharyngeal teeth and barbels, the subfamily Schizothoracinae was divided into three grades: primitive [e.g., Schizothorax davidi (genus: Schizothorax)], specialized, and highly specialized [e.g., Gymnocypris eckloni (genus: Gymnocypris)] schizothoracine fishes (Cao et al., 1981). The groups with higher specialization grade are more adaptable to the extreme environment of the plateau. Fossil evidence indicates that the extant Schizothorax fish originated from the Qaidam Basin (3500 m above sea level) before Oligocene (34 ~ 23 Ma) (Yang et al., 2021). Before the Eocene, Qaidam Basin was an inland sea (Wu et al., 2013; Wang Y. et al., 2015). Since the Eocene, it has rapidly dried up, forming some scattered salt lakes, and the water salinity has gradually increased (Gao et al., 2019; Ye et al., 2020; Tang et al., 2021). Schizothorax fish should theoretically have strong salinity tolerance. But strangely, in our experiments, the salt tolerance of Schizothorax davidi was the weakest among six species, which seemed to be inconsistent with the environmental characteristics of its origin. We propose two possible scenarios: (1) the salinity of lakes in the ancient Qaidam Basin is low; (2) the primitive Schizothorax fish lived in the freshwater system outside the basin. This may provide new insights into the reconstruction of the paleoenvironment of ancient Qaidam and the origin of Schizothorax fish.
In the 1970s, Miocene (23 ~ 5.3 Ma) Schizothorax fish fossils were unearthed from the Lunpola Basin (4500 m above sea level) (Wu and Chen, 1980). This indicates that during the Oligocene-Miocene period, the primitive Schizothorax fish was distributed in the central part of the Qinghai-Tibet Plateau, while the distribution of existing Schizothorax fish was limited to the eastern, southern and western marginal areas of the Qinghai-Tibet Plateau (Cao et al., 1981), suggesting that its distribution has changed since the Neogene (Figure 6). During the uplift of the central Tibetan Plateau in the Neogene, the climate here gradually became extreme. Meanwhile, geological changes of the plateau have also changed the distribution of water system, rivers were blocked and lakes were divided, the original fishes here were extinct or migrated (Wu and Tan, 1991). We speculate that the intolerance of Schizothorax fish to low temperature, low oxygen, and especially salinity led to its extinction in the central Tibetan Plateau. This also suggests that the adaptation to salinity is also an important feature of fish high-altitude adaptation.
Data availability statement
The original contributions presented in the study are included in the article/Supplementary Material. Further inquiries can be directed to the corresponding author.
Ethics statement
All the animal procedures were conducted with the approval of the Committee of Laboratory Animal Experimentation at Southwest University, Chongqing, China and were in full compliance with the Committee’s guidelines [protocol number (2014)25].
Author contributions
SL, HG and ZW conceived the ideas and designed this study. SL wrote the original manuscript and completed data analysis. HG and YW completed stress experiments. HG and SL completed histology experiments. SL, HG and ZW participated in the discussion of the experimental results and the improvement of the manuscript. All authors contributed to the article and approved the submitted version.
Funding
This research was supported by Regional Science and Technology Collaborative Innovation Project (F2023268).
Acknowledgments
Thanks to all the authors for contributing to this article. Thanks are due to Deyong Pu and Yingqiang Guan for assistance with the experiments and to Yuchao Song for valuable discussion.
Conflict of interest
The authors declare that the research was conducted in the absence of any commercial or financial relationships that could be construed as a potential conflict of interest.
Publisher’s note
All claims expressed in this article are solely those of the authors and do not necessarily represent those of their affiliated organizations, or those of the publisher, the editors and the reviewers. Any product that may be evaluated in this article, or claim that may be made by its manufacturer, is not guaranteed or endorsed by the publisher.
Supplementary material
The Supplementary Material for this article can be found online at: https://www.frontiersin.org/articles/10.3389/fmars.2023.1193699/full#supplementary-material
References
Anand I., Harris E., Ferrari R., Pearce P., Harris P. (1986). Pulmonary haemodynamics of the yak, cattle, and cross breeds at high altitude. Thorax 41 (9), 696–700. doi: 10.1136/thx.41.9.696
Bachman P. M., Rand G. M. (2008). Effects of salinity on native estuarine fish species in south Florida. Ecotoxicology 17, 591–597. doi: 10.1007/s10646-008-0244-7
Barcellos L. J. G., Kreutz L. C., Quevedo R. M. (2006). Previous chronic stress does not alter the cortisol response to an additional acute stressor in jundiá (Rhamdia quelen, quoy and gaimard) fingerlings. Aquaculture 253 (1-4), 317–321. doi: 10.1016/j.aquaculture.2005.05.035
Barton B. A., Iwama G. K. (1991). Physiological changes in fish from stress in aquaculture with emphasis on the response and effects of corticosteroids. Annu. Rev. Fish. Dis. 1, 3–26. doi: 10.1016/0959-8030(91)90019-G
Bau F., Parent J. (2001). Physiological responses of seven lacustrine fish species to capture stress (gill net and landing net). Bull. Francais. la. Peche. la. Piscicult. 357-360, 157–168. doi: 10.1051/kmae/2001042
Belanger S. E., Schurr K., Allen D. J., Gohara A. (1986). Effects of chrysotile asbestos on coho salmon and green sunfish: evidence of behavioral and pathological stress. Environ. Res. 39 (1), 74–85. doi: 10.1016/S0013-9351(86)80009-3
Bijlsma R., Loeschcke V. (2012). Genetic erosion impedes adaptive responses to stressful environments. Evol. Appl. 5 (2), 117–129. doi: 10.1111/j.1752-4571.2011.00214.x
Brandon R. N. (1978). Adaptation and evolutionary theory. Stud. History Philosophy. Sci. 9 (3), 181–206. doi: 10.1016/0039-3681(78)90005-5
Bullock A. M. (1988). Solar ultraviolet radiation: a potential environmental hazard in the cultivation of farmed finfish. Recent Adv. Aquacult. 3, 139–224. doi: 10.1007/978-94-011-9743-4_3
Bullock A., Coutts R. (1985). The impact of solar ultraviolet radiation upon the skin of rainbow trout, salmo gairdneri Richardson, farmed at high altitude in Bolivia. J. Fish. Dis. 8 (3), 263–272. doi: 10.1111/j.1365-2761.1985.tb00942.x
Cao Y. B., Chen X. Q., Wang S., Wang Y. X., Du J. Z. (2008). Evolution and regulation of the downstream gene of hypoxia-inducible factor-1α in naked carp (Gymnocypris przewalskii) from lake qinghai, China. J. Mol. Evol. 67 (5), 570–580. doi: 10.1007/s00239-008-9175-4
Cao W., Chen Y., Wu Y., Zhu S. (1981). Origin and evolution of schizothoracine fishes in relation to the upheaval of the xizang plateau. Stud. Period. Amplitude. Type. Uplift. Qinghai-Xizang. Plateau. Sci. Press. Beijing, 118–130.
Charlesworth D., Barton N. H., Charlesworth B. (2017). The sources of adaptive variation. Proc. R. Soc. B.: Biol. Sci. 284 (1855), 20162864. doi: 10.1098/rspb.2016.2864
Chen X., Wang J., Yue W., Lei S., Dobjay S., Li Z, et al. (2020). Integrated transcriptome provides resources and insights into the adaptive evolution of colonized brown trout (Salmo trutta fario) in the Tibetan plateau. J. World Aquacult. Soc. 51 (3), 763–774. doi: 10.1111/jwas.12621
Cyr A. J., Currie B. S., Rowley D. B. (2005). Geochemical evaluation of fenghuoshan group lacustrine carbonates, north-central Tibet: implications for the paleoaltimetry of the Eocene Tibetan plateau. J. Geol. 113 (5), 517–533. doi: 10.1086/431907
Deng T., Wang S., Xie G., Li Q., Hou S., Sun B. (2012). A mammalian fossil from the dingqing formation in the lunpola basin, northern Tibet, and its relevance to age and paleo-altimetry. Chin. Sci. Bull. 57, 261–269. doi: 10.1007/s11434-011-4773-8
Evans T. G., Kültz D. (2020). The cellular stress response in fish exposed to salinity fluctuations. J. Exp. Zool. Part A.: Ecol. Integr. Physiol. 333 (6), 421–435. doi: 10.1002/jez.2350
Figueroa R., Rodríguez-Sabarís R., Aldegunde M., Soengas J. (2000). Effects of food deprivation on 24 h-changes in brain and liver carbohydrate and ketone body metabolism of rainbow trout. J. Fish. Biol. 57 (3), 631–646. doi: 10.1111/j.1095-8649.2000.tb00265.x
Galhardo R. S., Hastings P. J., Rosenberg S. M. (2007). Mutation as a stress response and the regulation of evolvability. Crit. Rev. Biochem. Mol. Biol. 42 (5), 399–435. doi: 10.1080/10409230701648502
Gao C., Yu J., Min X., Cheng A., Hong R., Zhang L., et al. (2019). The sedimentary evolution of da qaidam salt lake in qaidam basin, northern Tibetan plateau: implications for hydro-climate change and the formation of pinnoite deposit. Environ. Earth Sci. 78, 1–15. doi: 10.1007/s12665-019-8480-0
Geng L., Tong G., Jiang H., Xu W. (2016). Effect of salinity and alkalinity on luciobarbus capito gill Na+/K+ -ATPase enzyme activity, plasma ion concentration, and osmotic pressure. BioMed. Res. Int. 2016, 4605839 doi: 10.1155/2016/4605839
Ghalambor C. K., Hoke K. L., Ruell E. W., Fischer E. K., Reznick D. N., Hughes K. A. (2015). Non-adaptive plasticity potentiates rapid adaptive evolution of gene expression in nature. Nature 525 (7569), 372–375. doi: 10.1038/nature15256
Ghosh T. K., Chauhan Y. H., Mandal R. N. (2019). Growth performance of labeo bata (Hamilton 1822) in freshwater and its acclimatization in brackish water with betaine as feed additive. Aquaculture 501, 128–134. doi: 10.1016/j.aquaculture.2018.11.020
Gu H., Li S., Wang H., Zhu S., Yuan D., Wang Z. (2022a). Interspecific differences and ecological correlations of ultraviolet radiation tolerance in low-and high-altitude fishes. Front. Mar. Sci. 9, 10351402290. doi: 10.3389/fmars.2022.1035140
Gu H., Wang H., Zhu S., Yuan D., Dai X., Wang Z. (2022b). Interspecific differences and ecological correlations between scale number and skin structure in freshwater fishes. Curr. Zool. zoac059. doi: 10.1093/cz/zoac059
Guan L., Chi W., Xiao W., Chen L., He S. (2014). Analysis of hypoxia-inducible factor alpha polyploidization reveals adaptation to Tibetan plateau in the evolution of schizothoracine fish. BMC Evol. Biol. 14 (1), 1–13. doi: 10.1186/s12862-014-0192-1
Hunter J. R., Kaupp S. E., Taylor J. H. (1981). Effects of solar and artificial ultraviolet-b radiation on larval northern anchovy, engraulis mordax. Photochem. Photobiol. 34 (4), 477–486. doi: 10.1111/j.1751-1097.1981.tb09028.x
Hunter J. R., Taylor J. H., Moser H. G. (1979). Effect of ultraviolet irradiation on eggs and larvae of the northern anchovy, engraulis mordax, and the pacific mackerel, scomber japonlcus, during the embryonic stage. Photochem. Photobiol. 29 (2), 325–338. doi: 10.1111/j.1751-1097.1979.tb07055.x
Jin L., Li Z., Wang C., Wang Y., Li X., Yang J., et al. (2022). Contrasting population differentiation in two sympatric triplophysa loaches on the qinghai–Tibet plateau. Front. Genet. 13. doi: 10.3389/fgene.2022.958076
Júnior A. B., Alves F. L., Pereira A., Ide L. M., Hoffmann A. (2012). Behavioral characterization of the alarm reaction and anxiolytic-like effect of acute treatment with fluoxetine in piauçu fish. Physiol. Behav. 105 (3), 784–790. doi: 10.1016/j.physbeh.2011.10.007
Karsiotis S. I., Pierce L. R., Brown J. E., Stepien C. A. (2012). Salinity tolerance of the invasive round goby: experimental implications for seawater ballast exchange and spread to north American estuaries. J. Great. Lakes. Res. 38 (1), 121–128. doi: 10.1016/j.jglr.2011.12.010
Katoh K., Rozewicki J., Yamada K. D. (2019). MAFFT online service: multiple sequence alignment, interactive sequence choice and visualization. Briefings Bioinf. 20 (4), 1160–1166. doi: 10.1093/bib/bbx108
Kefford B. J., Papas P. J., Metzeling L., Nugegoda D. (2004). Do laboratory salinity tolerances of freshwater animals correspond with their field salinity? Environ. pollut. 129 (3), 355–362. doi: 10.1016/j.envpol.2003.12.005
Kültz D. (2015). Physiological mechanisms used by fish to cope with salinity stress. J. Exp. Biol. 218 (12), 1907–1914. doi: 10.1242/jeb.118695
Li J., Fang X. (1999). Uplift of the Tibetan plateau and environmental changes. Chin. Sci. Bull. 44 (23), 2117–2124. doi: 10.1007/BF03182692
Li B., Jia G., Wen D., Zhao X., Zhang J., Xu Q., et al. (2022). Rumen microbiota of indigenous and introduced ruminants and their adaptation to the qinghai–Tibetan plateau. Front. Microbiol. 13. doi: 10.3389/fmicb.2022.1027138
Lindsey C. (1966). Body sizes of poikilotherm vertebrates at different latitudes. Evolution 20 (4), 456–465. doi: 10.2307/2406584
Little A. G., Seebacher F. (2013). Thyroid hormone regulates muscle function during cold acclimation in zebrafish (Danio rerio). J. Exp. Biol. 216 (18), 3514–3521. doi: 10.1242/jeb.089136
Liu Y., An Z., Linderholm H. W., Chen D., Song H., Cai Q., et al. (2009). Annual temperatures during the last 2485 years in the mid-eastern Tibetan plateau inferred from tree rings. Sci. China Ser. D.: Earth Sci. 52 (3), 348–359. doi: 10.1007/s11430-009-0025-z
Liu W., Li X., Zhang L., An Z., Xu L. (2009). Evaluation of oxygen isotopes in carbonate as an indicator of lake evolution in arid areas: the modern qinghai lake, qinghai–Tibet plateau. Chem. Geol. 268 (1-2), 126–136. doi: 10.1016/j.chemgeo.2009.08.004
Liu C., Zhu L., Li J., Wang J., Ju J., Qiao B., et al. (2021). The increasing water clarity of Tibetan lakes over last 20 years according to MODIS data. Remote Sens. Environ. 253, 112199. doi: 10.1016/j.rse.2020.112199
Machta L., Hughes E. (1970). Atmospheric oxygen in 1967 to 1970. Science 168 (3939), 1582–1584. doi: 10.1126/science.168.3939.158
Mao K. S., Wang Y., Liu J. Q. (2021). Evolutionary origin of species diversity on the qinghai–Tibet plateau. J. Syst. Evol. 59 (6), 1142–1158. doi: 10.1111/jse.12809
Martincorena I., Seshasayee A. S., Luscombe N. M. (2012). Evidence of non-random mutation rates suggests an evolutionary risk management strategy. Nature 485 (7396), 95–98. doi: 10.1038/nature10995
Mustafa S. A., Karieb S. S., Davies S. J., Jha A. N. (2015). Assessment of oxidative damage to DNA, transcriptional expression of key genes, lipid peroxidation and histopathological changes in carp cyprinus carpio l. following exposure to chronic hypoxic and subsequent recovery in normoxic conditions. Mutagenesis 30 (1), 107–116. doi: 10.1093/mutage/geu048
Nobrega R. O., Batista R. O., Corrêa C. F., Mattioni B., Filer K., Pettigrew J. E., et al. (2019). Dietary supplementation of aurantiochytrium sp. meal, a docosahexaenoic-acid source, promotes growth of Nile tilapia at a suboptimal low temperature. Aquaculture 507, 500–509. doi: 10.1016/j.aquaculture.2019.04.030
Osman, Harabawy A. S. A. (2010). Hematotoxic and genotoxic potential of ultraviolet-a radiation on the African catfish clarias gariepinus (Burchell 1822). J. Fisheries. Int. 5 (3), 44–53. doi: 10.3923/jfish.2010.44.53
Pigliucci M. (2005). Evolution of phenotypic plasticity: where are we going now? Trends Ecol. Evol. 20 (9), 481–486. doi: 10.1016/j.tree.2005.06.001
Qi D., Chao Y., Zhao L., Shen Z., Wang G. (2013). Complete mitochondrial genomes of two relatively closed species from gymnocypris (Cypriniformes: cyprinidae): genome characterization and phylogenetic considerations. Mitochondrial. DNA 24 (3), 260–262. doi: 10.3109/19401736.2012.744975
Qu Y., Zhao H., Han N., Zhou G., Song G., Gao B., et al. (2013). Ground tit genome reveals avian adaptation to living at high altitudes in the Tibetan plateau. Nat. Commun. 4 (1), 1–9. doi: 10.1038/ncomms3071
Rosenberg S. M. (2001). Evolving responsively: adaptive mutation. Nat. Rev. Genet. 2 (7), 504–515. doi: 10.1038/35080556
Scholander P. F., Flagg W., Walters V., Irving L. (1953). Climatic adaptation in arctic and tropical poikilotherms. Physiol. Zool. 26 (1), 67–92. doi: 10.1086/physzool.26.1.30152151
Spicer R. A., Su T., Valdes P. J., Farnsworth A., Wu F.-X., Shi G., et al. (2021). Why ‘the uplift of the Tibetan plateau’is a myth. Natl. Sci. Rev. 8 (1), nwaa091. doi: 10.1093/nsr/nwaa091
Su T., Farnsworth A., Spicer R. A., Huang J., Wu F.-X., Liu J., et al. (2019). No high Tibetan plateau until the neogene. Sci. Adv. 5 (3), eaav2189. doi: 10.1126/sciadv.aav2189
Sun L., Chen G., Chang C. (1995). Acute responses of blood parameters and comatose effects in salt-acclimated tilapia exposed to low temperatures. J. Thermal. Biol. 20 (3), 299–306. doi: 10.1016/0306-4565(94)00066-R
Sun J., Xu Q., Liu W., Zhang Z., Xue L., Zhao P. (2014). Palynological evidence for the latest oligocene– early Miocene paleoelevation estimate in the lunpola basin, central Tibet. Palaeogeogr. Palaeoclimatol. Palaeoecol. 399, 21–30. doi: 10.1016/j.palaeo.2014.02.004
Sushchik N., Zuev I., Kalachova G., Ageev A., Gladyshev M. (2018). Content of highly unsaturated fatty acids in fish from rivers of contrasting temperature. River. Res. Appl. 34 (6), 565–574. doi: 10.1002/rra.3286
Tang W., Yi F., Chen X., Tang H., Li F., Xia G., et al. (2021). Abrupt aridification in the upper eocene of the western qaidam basin, northeastern Tibetan plateau. Palaeogeogr. Palaeoclimatol. Palaeoecol. 577, 110515. doi: 10.1016/j.palaeo.2021.110515
Tapponnier P., Zhiqin X., Roger F., Meyer B., Arnaud N., Wittlinger G., et al. (2001). Oblique stepwise rise and growth of the Tibet plateau. science 294 (5547), 1671–1677. doi: 10.1126/science.105978
Tiku P. E., Gracey A. Y., Macartney A. I., Beynon R. J., Cossins A. R. (1996). Cold-induced expression of Δ9-desaturase in carp by transcriptional and posttranslational mechanisms. Science 271 (5250), 815–818. doi: 10.1126/science.271.5250.815
Trifinopoulos J., Nguyen L.-T., von Haeseler A., Minh B. Q. (2016). W-IQ-TREE: a fast online phylogenetic tool for maximum likelihood analysis. Nucleic Acids Res. 44 (W1), W232–W235. doi: 10.1093/nar/gkw256
Varsamos S., Nebel C., Charmantier G. (2005). Ontogeny of osmoregulation in postembryonic fish: a review. Comp. Biochem. Physiol. Part A.: Mol. Integr. Physiol. 141 (4), 401–429. doi: 10.1016/j.cbpb.2005.01.013
Wang X., Gan X., Li J., Chen Y., He S. (2016). Cyprininae phylogeny revealed independent origins of the Tibetan plateau endemic polyploid cyprinids and their diversifications related to the neogene uplift of the plateau. Sci. China Life Sci. 59 (11), 1149–1165. doi: 10.1007/s11427-016-0007-7
Wang X., Wang Y., Li Q., Tseng Z. J., Takeuchi G. T., Deng T., et al. (2015). Cenozoic Vertebrate evolution and paleoenvironment in Tibetan plateau: progress and prospects. Gondwana. Res. 27 (4), 1335–1354. doi: 10.1016/j.gr.2014.10.014
Wang Y., Wang X., Wang Y. (2015). Cambrian Ichnofossils from the zhoujieshan formation (Quanji group) overlying tillites in the northern margin of the qaidam basin, NW China. J. Earth Sci. 26, 203–210. doi: 10.1007/s12583-015-0532-0
Wendelaar Bonga S. E. (1997). The stress response in fish. Physiol. Rev. 77 (3), 591–625. doi: 10.1152/physrev.1997.77.3.591
Witt K. E., Huerta-Sánchez E. (2019). Convergent evolution in human and domesticate adaptation to high-altitude environments. Philos. Trans. R. Soc. B. 374 (1777), 20180235. doi: 10.1098/rstb.2018.0235
Wu Y., Chen Y. (1980). Fossil cyprinid fishes from the late tertiary of north xizang, China. Vert. PalAsiat. 18, 15–20. doi: 10.19615/j.cnki.1000-3118.1980.01.003
Wu H., Guang X., Al-Fageeh M. B., Cao J., Pan S., Zhou H., et al. (2014). Camelid genomes reveal evolution and adaptation to desert environments. Nat. Commun. 5 (1), 5188. doi: 10.1038/ncomms6188
Wu Z., Hu D., Ye P., Wu Z. (2013). Early Cenozoic tectonics of the Tibetan plateau. Acta Geol. Sinica-English. Edition. 87 (2), 289–303. doi: 10.1111/1755-6724.12051
Wu Y., Tan Q. (1991). Characteristics of the fish-fauna of the characteristics of qinghai-xizang plateau and its geological distribution and formation. Acta Zool. Sin. 37 (2), 135–152.
Xu Q., Zhang C., Zhang D., Jiang H., Peng S., Liu Y., et al. (2016). Analysis of the erythropoietin of a Tibetan plateau schizothoracine fish (Gymnocypris dobula) reveals enhanced cytoprotection function in hypoxic environments. BMC Evol. Biol. 16 (1), 1–17. doi: 10.1186/s12862-015-0581-0
Yang T., Liang W., Cai J., Gu H., Han L., Chen H., et al. (2021). A new cyprinid from the oligocene of qaidam basin, north-eastern Tibetan plateau, and its implications. J. Syst. Palaeontol. 19 (17), 1161–1182. doi: 10.1080/14772019.2021.2015470
Yang T., Meng W., Guo B. (2020). Population genomic analysis of two endemic schizothoracins reveals their genetic differences and underlying selection associated with altitude and temperature. Animals 10 (3), 447. doi: 10.3390/ani10030447
Yang L., Naylor G. J., Mayden R. L. (2022). Deciphering reticulate evolution of the largest group of polyploid vertebrates, the subfamily cyprininae (Teleostei: cypriniformes). Mol. Phylogenet. Evol. 166, 107323. doi: 10.1016/j.ympev.2021.107323
Yang L., Wang Y., Zhang Z., He S. (2015). Comprehensive transcriptome analysis reveals accelerated genic evolution in a Tibet fish, gymnodiptychus pachycheilus. Genome Biol. Evol. 7 (1), 251–261. doi: 10.1093/gbe/evu279
Ye C., Yang Y., Fang X., Zhang W., Song C., Yang R. (2020). Paleolake salinity evolution in the qaidam basin (NE Tibetan plateau) between~ 42 and 29 ma: links to global cooling and paratethys sea incursions. Sedimentary. Geol. 409, 105778. doi: 10.1016/j.sedgeo.2020.105778
Yuan D., Chen X., Gu H., Zou M., Zou Y., Fang J., et al. (2020). Chromosomal genome of triplophysa bleekeri provides insights into its evolution and environmental adaptation. GigaScience 9 (11), giaa132. doi: 10.1093/gigascience/giaa132
Yuan J., Gao J., Lu X., Chen K. (2002). Assessment on wetland resources in namucuo and countermeasures for conservation and rational use. Resour. Sci. 24 (4), 29–34. doi: 10.3321/j.issn:1007-7588.2002.04.006
Zhang A., Cao Z., Fu S. (2014). Comparison of hypoxia tolerance and locomotor performance in two cyprinids with incompletely overlapped habitat. Acta Ecol. Sin. 34 (20), 8. doi: 10.5846/stxb201301230130
Zhao K., Duan Z., Yang G., Peng Z., He S., Chen Y. (2007). Origin of gymnocypris przewalskii and phylogenetic history of gymnocypris eckloni (Teleostei : cyprinidae). Prog. Natural Sci. 17 (5), 520–528. doi: 10.1080/10020070708541031
Zhao Z., Hou Z.-E., Li S.-Q. (2022). Cenozoic Tethyan changes dominated Eurasian animal evolution and diversity patterns. Zool. Res. 43 (1), 3. doi: 10.24272/j.issn.2095-8137.2021.322
Zhao K., Li J., Yang G., Duan Z., He S., Chen Y. (2005). Molecular phylogenetics of gymnocypris (Teleostei : cyprinidae) in lake qinghai and adjacent drainages. Chin. Sci. Bull. 50 (13), 1325–1333. doi: 10.1360/982005-223
Zhao R., Wang Y., Yang C., Li S., Li J., Sun X., et al. (2022). Dominant elongase activity of Elovl5a but higher expression of Elovl5b in common carp (Cyprinus carpio). Int. J. Mol. Sci. 23 (23), 14666. doi: 10.3390/ijms232314666
Zhou S., Zhang R. (2005). Decadal variations of temperature and geopotential height over the Tibetan plateau and their relations with Tibet ozone depletion. Geophys. Res. Lett. 32 (18), L18705 doi: 10.1029/2005GL023496
Keywords: plateau fish, Schizothoracinae, environmental stress, high-altitude adaptation, fishes distribution
Citation: Li S, Gu H, Wang Y and Wang Z (2023) Factors limiting the spread of middle- and low-altitude fishes to the Qinghai-Tibet plateau. Front. Mar. Sci. 10:1193699. doi: 10.3389/fmars.2023.1193699
Received: 25 March 2023; Accepted: 15 May 2023;
Published: 26 May 2023.
Edited by:
Susana Puntarulo, University of Buenos Aires, ArgentinaReviewed by:
Xidong Mu, Chinese Academy of Fishery Sciences, ChinaCunfang Zhang, Qinghai University, China
Copyright © 2023 Li, Gu, Wang and Wang. This is an open-access article distributed under the terms of the Creative Commons Attribution License (CC BY). The use, distribution or reproduction in other forums is permitted, provided the original author(s) and the copyright owner(s) are credited and that the original publication in this journal is cited, in accordance with accepted academic practice. No use, distribution or reproduction is permitted which does not comply with these terms.
*Correspondence: Zhijian Wang, d2FuZ3pqMTk2OUAxMjYuY29t
†These authors have contributed equally to this work