- 1CIIMAR/CIMAR-LA, Centro Interdisciplinar de Investigação Marinha e Ambiental, Universidade do Porto, Terminal de Cruzeiros do Porto de Leixões, Matosinhos, Portugal
- 2Observatório Oceânico da Madeira, ARDITI, Madeira Tecnopolo, Funchal, Portugal
- 3ICBAS, Instituto de Ciências Biomédicas de Abel Salazar, Universidade do Porto, Rua de Jorge Viterbo Ferreira, Porto, Portugal
- 4ALGAplus, Produção e Comercialização de Algas e seus Derivados Lda., Ílhavo, Portugal
- 5SPAROS Lda., Área Empresarial de Marim, Olhão, Portugal
- 6RIASEARCH Lda., Cais da Ribeira de Pardelhas, Murtosa, Portugal
Introduction: In order to ensure lipid quality of cultured fish and an environmentally sustainable production, new alternative sources of EPA and DHA are needed to replace traditional lipid sources, such as fish oil.
Methods: Different combinations of alternative marine lipid sources rich in n-3 LC-PUFA available in the market were herein evaluated to replace traditional fish oil (sardine oil) in diets for European sea bass (Dicentrarchus labrax). A commercial-type diet (CTRL), containing 1.6% of EPA + DHA, 5% sardine oil and 8% rapeseed oil was used as a negative control. Another diet (SARDINE) formulated with 8.5% sardine oil, 4.5% rapeseed oil and 2.5% EPA + DHA was used as the positive control. Three experimental diets were formulated to completely replace sardine oil with alternative sources, targeting approximately the same EPA + DHA level as the positive control: the SALMON diet contained 9.9% salmon by-product oil mixed with 3.1% of an algal oil rich in EPA and DHA, while the ALGARAPE and the ALGASOY diets included 4.4% of the algal oil and 8.6% of either rapeseed or soybean oil, respectively. A sixth diet (ALGABLEND) was formulated to partially replace sardine oil with salmon by-product oil and rapeseed oil, balanced with 2% of algae biomass. The experimental diets were hand-fed to 118 g fish for 54 days.
Results: All diets were well-accepted by fish and no significant differences were found in feed efficiency, growth performance, somatic indexes or whole body composition among treatments. At the end of the trial, regardless the dietary EPA + DHA level, all fillets contained more than 250 mg of EPA + DHA per 100 g fresh weight, meeting EFSA recommendations for cardiovascular risk prevention for European adults (> 250 mg day -1).
Discussion: Overall, this study demonstrated that combining expensive sources of n-3 LC PUFA (Veramaris® or Algaessence Feed™ with low-priced sustainable oils (salmon by-products oil or vegetable oils) allows fortifying European sea bass flesh with EPA and DHA, without major textural changes. This approach is a successful strategy for mitigating the negative effects associated with the high inclusion of vegetable oils. However, the retention of n-3 LC-PUFA in muscle was not significantly increased, suggesting that there is a maximum dietary threshold beyond which β-oxidation might be promoted, and hence there is no advantage in increasing the dietary level of these fatty acids in European sea bass diets.
Introduction
Fish is a rich source of high-quality protein and micronutrients, such as vitamins A, B12 and D, as well as minerals like iodine and selenium. It is also one of the few sources of omega-3 long-chain polyunsaturated fatty acids (n-3 LC-PUFA) for human consumption (Xu et al., 2020). Omega-3 LC-PUFA, particularly eicosapentaenoic acid (C20:5n-3, EPA) and docosahexaenoic acid (C22:6n-3, DHA), play an important role in neural system development and help reduce the risk of chronic pathologies, such as cardiovascular diseases, diabetes and obesity in humans (Li et al., 2020). Dietary recommendations for daily intake of EPA and DHA to prevent the risk of cardiovascular accidents in European adults are between 250 and 500 mg per day, according to the European Food Safety Authority (EFSA, 2012).
Aquaculture contributes to nearly half of global fish consumption (FAO, 2022), being a major source of EPA and DHA for the burgeoning human population. However, most marine fish have limited ability to convert short-chain (C18) polyunsaturated fatty acids (PUFA) into LC-PUFA (C20-22) (Monroig et al., 2018). As a result, fish farming is dependent on the dietary supply of n-3 LC-PUFA, which is traditionally obtained from fish oil (FO) and fish meal (FM) (Tocher et al., 2019). The dependence on small pelagic stocks to support aquaculture growth compromises environmental sustainability and adds increasing pressure on fishery resources, which risk being overexploited if not well managed (Naylor et al., 2021; FAO, 2022). Therefore, finding alternative sources of EPA and DHA for aquafeeds is imperative to ensure an adequate, sustainable and secure supply of raw-materials for future formulations.
The partial or total FO replacement by vegetable oils (VO) in fish diets has been thoroughly evaluated (Turchini et al., 2010; Torrecillas et al., 2017). Many studies have shown that it is feasible to partially replace FO by VO in diets for both freshwater and marine fish species without affecting feed utilization and growth (Turchini et al., 2009; Dikel et al., 2013; Pereira et al., 2019). However, others studies have reported negative effects on fish health and fillet nutritional value due to the low levels of n-3 LC-PUFA and the imbalance of n-3 PUFA to n-6 PUFA (Glencross, 2009; Nasopoulou and Zabetakis, 2012). In European sea bass (Dicentrarchus labrax), soybean (rich in linoleic acid C18:2n-6 (LA); 57% of total fatty acids) and rapeseed oil (rich in oleic acid C18:1n-9 (OA); 54% of total fatty acids), two highly available alternative lipid sources, have been used up to a 60% FO replacement without compromising growth (Izquierdo et al., 2003), but decreasing n-3 LC-PUFA levels in the fillet of commercial-sized fish (Izquierdo et al., 2005; Mourente and Bell, 2006). Such negative effects on final product quality may limit the benefits of fish consumption. While considerable efforts have been carried out to mitigate the negative impacts of replacing FO by VO in aquafeeds (Mourente and Bell, 2006; Turchini et al., 2010), some studies have identified a range of alternative EPA and DHA sources with the potential to improve aquafeeds (Tocher, 2015; Tocher et al., 2019; Santigosa et al., 2020; Santigosa et al., 2021; Kousoulaki et al., 2022).
The pressure to use local resources and adopt a circular economy strategy can increase the supply of marine FO and strongly contribute to a reduction of its carbon footprint (Naylor et al., 2009; Klinger and Naylor, 2012; Campos et al., 2020). In fact, recycling activities such as producing FO from fish waste or by-products of the processing industry are projected to ramp up in the coming years (FAO, 2022). According to FAO (2022), the global production of Atlantic salmon (Salmo salar) in 2020 was 2.71 million tons, accounting for 32.6% of finfish in marine and coastal aquaculture. Slaughter-sized Atlantic salmon contains about 45% of its lipids in the fillet whereas the other 55% are present in the inedible portions (Ytrestøyl et al., 2011). These by-products can be used to generate added-value products such as salmon oil and could, hence, be a valuable source of n-3 LC-PUFA for aquafeeds (Ytrestøyl et al., 2011; Deepika et al., 2014).
Although the use of existing n-3 LC-PUFA sources should be considered (Tocher, 2015), it has been recently defended that recycling cannot be the only solution to ensure the supply of EPA and DHA (Tocher et al., 2019). Algal biomass and algal oils have just emerged as an alternative to FO and VO with promising results in several fish species (Miller et al., 2007; Kiron et al., 2012; Santigosa et al., 2020; Santigosa et al., 2021). Ferreira et al. (2021) have characterized the nutritional value of four commercially available micro- and macroalgae (Nannochloropsis sp., Chlorella sp., Gracilaria sp. and Ulva sp.) and a blend of the four algae species (Algaessence Feed™), reporting approximately 6% of lipids (as % dry matter, DM) in Nannochloropsis sp. and Chlorella sp., over 19 mg g-1 of EPA in Nannochloropsis sp. and approx. 5 mg g-1 of EPA in Algaessence Feed™, but none of the algae contained DHA. Other algae species (i.e. Schizochytrium and Crypthecodinium sp.) rich in DHA (30-40% of total FA) are available in the market (Adarme-Vega et al., 2012), and selected strains have been used for the production of valuable alga oils. Ferreira et al. (2022) observed an efficient accumulation of EPA+DHA in the muscle of gilthead seabream (Sparus aurata) fed a diet that included a blend of microalgae (including DHA-rich Schizochytrium sp.) to replace 33% FM. The use of a DHA and EPA rich oil obtained from Schizochytrium in FO-free diets has shown good growth and an efficient deposition of EPA and DHA in the muscle of gilthead seabream (at a 3.5% inclusion level, Santigosa et al., 2021) and rainbow trout (Oncorhynchus mykiss) (at a 5% inclusion level, Santigosa et al., 2020). Despite the encouraging results, the use of such algal oils to fully replace FO and restock the high levels of n-3 LC-PUFA of a FO-free diet is not yet economically viable (Naylor et al., 2021). Therefore, the use of multiple cost-effective lipid sources to avoid possible negative impacts of single ingredients, and maximize each ingredient’s contribution to the aquafeed nutritional balance has been envisaged (Chauton et al., 2015; Zhang et al., 2019).
In this context, this study aimed to identify the best combination of alternative lipid sources rich in LC-PUFA and currently available in the market, to replace sardine oil in diets for European sea bass, but maintaining growth performance, nutrient utilization, and flesh quality for human consumption.
Materials and methods
Ingredients and experimental diets
Commercially available salmon by-product oil (SmO; Sopropêche, France), algae oil (AO; Veramaris®, Evonik, NE, USA) and Algaessence Feed™ (AF; ALGAplus Lda., Portugal), a blend of macro and microalgae species (Gracilaria sp., Nanochloropsis sp. and Shizochitrium sp.) were selected based on their n-3 LC-PUFA richness and economic feasibility to replace sardine oil (SdO), ensuring high EPA and DHA levels. The fatty acid profile of lipid sources is presented in Table 1 and described in the next section. Based on the nutritional requirements of European sea bass (NRC, 2011), six isonitrogenous (51% DM), isolipidic (18% DM) and isoenergetic (23 kJ g−1 DM) diets were formulated with moderate levels of marine-protein ingredients (15% FM and 3.5% hydrolyzed fish protein), but differing in the oil sources (Table 2). A commercially-based diet was used as a negative control (CTRL): it provided 1.6% of EPA+DHA and included 5% SdO and 8% rapeseed oil (RO). A positive control diet (SARDINE), with 8.5% SdO and 4.5% RO, providing 2.5% of EPA+DHA was also tested. This diet implied a 6% increase in price compared to the CTRL. Three other diets were formulated to totally replace sardine oil by the selected alternative sources, targeting approximately the same EPA+DHA levels as the positive control. In the SALMON diet, 9.9% SmO and 3.1% AO were used, whereas in ALGARAPE and ALGASOY diets 4.4% AO and 8.6% of either RO or soybean oil (SyO) were included. These diets totally replaced sardine oil with a 16-17% increase in price compared to the CTRL. The last diet ALGABLEND was formulated to partially replace the SdO included in the positive control by 7.9% SdO and 4.7% RO balanced with 2% of AF biomass. This diet also targeted the same EPA+DHA level as in the positive control and explored the functionality of the algae biomass, but the drawback was a 41% price increase.
All diets were supplemented with mono-calcium phosphate, L-lysine and DL-methionine, and were extruded and produced by SPAROS Lda. (Olhão, Portugal). Proximate composition and fatty acid profile of the experimental diets are presented in Tables 2 and 3, respectively.
Lipid sources and diets fatty acid profile
The FA profile of all selected lipid sources is presented in Table 1. Major differences were found in total SFA and MUFA: while SdO, AO and AF displayed higher percentages of SFA (>28%), RO, SmO and SyO had higher levels of MUFA, which resulted from a high percentage of oleic acid (C18:1n-9) ranging from 25.4 to 60.3% in these oils. The sum of PUFA also varied between lipid sources, ranging from 27.4 to 63.8%. AO had the highest PUFA sum, followed by SyO and SdO. Within PUFA, major differences were found in the level of both LA and α-linolenic acid (C18:3n-3, ALA), which were more than 10 times higher in SmO, RO and SyO than in other lipid sources. More importantly, SdO and AO were very rich sources of EPA (>20% and >17% of total FA, respectively) which was absent in RO and SyO. The DHA level was particularly high in AO (>40% of total FA), followed by AF (>30% of total FA), and was only present at 4% of total FA in SmO, and absent in RO and SyO. These differences were reflected in the sum of EPA and DHA (highest in AO, followed by AF and SdO) and DHA/EPA ratio, which was highest in AF. The n-3/n-6 ratio of the tested ingredients was highest in AO, followed by AF and SdO (Table 1). Consequently, all the diets had very different fatty acid profiles (Table 3). The CTRL diet had the lowest percentage of SFA (18% of total FA) and PUFA levels (36% of total FA), but the highest MUFA (44% of total FA); in CTRL, 10% of total FA was EPA+DHA (corresponding to 1.6 g of EPA+DHA per 100 g DM). All diets were richer in PUFA than the CTRL diet, and as expected, their levels of EPA+DHA (15.6 - 16.7% total FA, corresponding to 2.6 - 2.8 g 100 g-1 DM) were close to the SARDINE diet (15.4% of total FA, corresponding to 2.5 g 100 g-1 DM). A 3-4% dietary inclusion of AO in SALMON, ALGARAPE and ALGASOY almost doubled the DHA concentration in these diets. This increase was reflected on DHA/EPA ratio, which was highest in ALGASOY followed by ALGARAPE and SALMON. However, n-3/n-6 ratios were still below 1 for ALGARAPE and ALGASOY, and above 1 for SARDINE, SALMON and ALGAEBLEND (Table 3).
Growth trial and fish sampling
The growth trial was conducted in Riasearch Lda. (Murtosa, Portugal), with European sea bass juveniles obtained from the commercial fish farm ALGAPlus Lda. (Ílhavo, Portugal). Fish were individually weighed and 12 homogeneous groups of 17 fish (118.6 ± 15.2 g) were established and distributed by 350 L fiberglass tanks within a saltwater recirculation system (water temperature of 20°C, salinity of 18‰, flow rate at 700 L/h (200%/h renewal) and 12 h light/12 h dark photoperiod regime). Levels of total ammonium (NH4+), nitrite (NO2-) and nitrate (NO3-), as well as pH were daily monitored to ensure levels within the recommended ranges for marine species (NH4+ < 0.05 mg L-1; NO2- < 0.5 mg L-1; NO3- < 5 mg L-1; 7.5 < pH ≤ 8). Each diet was randomly assigned to duplicate groups of fish, which were hand fed until visual apparent satiation, three times a day, seven days a week, for 54 days.
Ten fish from the initial fish stock and the 17 fish from each tank at the end of the trial were collected and killed by anaesthetic overdose (MS222, 150 mg L-1) after a 48h fasting period. All fish were individually weighed (g) and measured for growth performance evaluation. The 10 fish from the initial stock, and 12 fish from each tank at the end of trial were sampled for whole body composition analysis. Dorsal muscle samples were collected from each fish through a biopsy below the skin (~1 g per fish). For the chemical analysis of both whole body and muscle composition, two independent pools were established per tank, each containing 6 whole fish or 6 muscle samples from those 6 fish. Samples were immediately frozen in dry ice and stored at -80°C for further analysis. Five other fish per tank were collected for instrumental evaluation of skin and muscle colour, and muscle texture. The viscera and liver from these 5 fish were collected and individually weighed to determine the viscerosomatic and hepatosomatic indexes (VSI and HSI, respectively).
Proximate composition analysis
The experimental diets and whole fish were ground, homogenized and freeze-dried before being further analyzed. Proximate composition analysis followed AOAC (2006) methods: dry matter (in an oven at 105°C for 24 h); ash (incineration in a muffle furnace at 550°C for 6 h; Nabertherm L9/11/B170, Bremen, Germany); crude protein by quantification of nitrogen (N) using a Leco nitrogen analyser (Model FP-528; Leco Corporation, St. Joseph, USA) and conversion (N × 6.25) to equivalent protein; gross energy was determined in an adiabatic bomb calorimetric system (Model Werke C2000, IKA, Staufen, Germany). Total phosphorus content was determined from ash according to AOAC method 965.17 (2006) with the following adaptation: phosphates were quantified after using a 2 mM ammonium heptamolybdate reagent solution with 7 mM ascorbic acid and 0.5 M sulfuric acid at 75 °C in a water bath and later reading the absorbance at 820 nm.
Total lipids and fatty acid analysis
The experimental diets, whole fish and muscle samples were analysed for total lipids and fatty acids profile.
Total lipids of the experimental diets, whole body and dorsal muscle were extracted and quantified gravimetrically according to Folch et al. (1957) using Folch solution (dichloromethane: methanol 2:1 v/v with 0.01% BHT).
The experimental diets, whole body and muscle lipid extracts were transmethylated by acidic methylation according to Lepage and Roy (1986), with some modifications; adding 3 mL of freshly prepared 5% (v/v) acetyl chloride in anhydrous methanol and heated at 100°C for 1 h. After cooling at room temperature, 5 mL of 6% K2CO3 solution and 25 mg of BHT dissolved in 2 mL of n-hexane were added. Samples were then vortexed and centrifuged at 700 g for 5 min at room temperature, and the upper organic layer containing fatty acids methyl esters (FAME) was carefully transferred to another tube with 1 g of anhydrous sodium sulphate. This step was repeated twice, before the organic layer was filtered (125 mm Ø) into a new tube, heated at 37°C and dried under a stream of nitrogen gas. Finally, FAME was recovered in 1 mL of n-hexane and analysed in a Shimadzu Nexis GC-2030 gas chromatograph (Kyoto, Japan) equipped with a flame-ionization detector (FID) and a Shimadzu AOC-20i auto-injector. Separation was carried out on an OmegaWax 250 capillary column (30 m× 0.25 mm I.D., film thickness 0.25 µm). Operating conditions were as follows: split mode, with a split ratio of 1:50 and an injection volume of 1 µL. The injector and detector temperatures were kept at 250 and 280°C, respectively. A flow rate of 25 mL min-1 of helium as a carrier gas, 40 mL min-1 of hydrogen and 400 mL min-1 of air were provided. The column thermal gradient was as follows: initial temperature 50°C for 2 min, increased at 50°C min-1 to 174°C, hold for 14 min, then increased 2 °C min-1 to 210 °C and hold for 50 min. FAME were identified by comparison of retention times with a known standard mixture (Sigma 47,885-U Supelco 37 Component FAME Mix, USA) and quantified using the software GCsolution for GC systems (Shimadzu, Kyoto, Japan). The FAME contents in diets, whole body and muscle were expressed as % of total FAME. The amount of FA expressed in mg 100 g−1 of edible part, were calculated using the relative percentage of each peak area (% of total FA) and the lipid conversion factors calculated according to Weihrauch et al. (1977) and Garcia et al. (2019).
Colour and texture
Instrumental measurements of skin and muscle colour were performed at room temperature with a CR-400 Chroma meter (Konica Minolta Inc., Osaka, Japan) with an aperture of 8 mm, at standard illuminant D65 using the CIE 1976 (L*, lightness; a*, redness; b*, yellowness). After the calibration of the apparatus with a white plate reference standard, colour parameters were measured by applying the colorimeter onto the raw skin and flesh of the ten fish per dietary treatment (5 fish/tank). Measurements were made above the lateral line in three points of each fillet, and mean values were determined for each fish. After flashing, L*, a*, and b* reflected light values were recorded. From a* and b* values, the hue angle (H° = tan-1 b*/a*) and the Chroma (C* = (a*2 + b*2)1/2) were calculated according to Choubert et al. (1997).
Muscle texture was analysed using a TA.XT plus Texture Analyser, with a 5 kg load cell and a 2.0 mm diameter probe (Stable Micro systems Inc., Godalming, UK). Texture profile parameters [hardness (N), adhesiveness (J), springiness (-), cohesiveness (-), chewiness (J), and resilience (-)] were obtained by double penetration (probe speed of 1 mm s-1; probe penetration depth of 4 mm; wait time between penetrations of 5 s) on the thickest part of each raw fillet (Batista et al., 2020).
Calculations
Growth, feed intake and other somatic parameters were calculated as follows: Daily growth index (DGI) =100 × [(final body weight) 1/3 - (initial body weight)1/3]/days of the experiment; Final condition factor (K) = 100 × (FBW/final body length3); Voluntary feed intake (VFI) = 100 × dry feed intake/average body weight/days of experiment; Feed conversion ratio (FCR) = dry feed intake/weight gain; Protein efficiency ratio (PER) = (FBW − IBW)/total protein intake (g); HSI = 100 × (liver weight/FBW); VSI = 100 × (weight of viscera/FBW).
Fatty acids utilisation parameters were calculated as follows: FA gain = (FBW × final whole body FA content) – (IBW × initial whole body FA content/ABW/days of experiment; FA retention = 100 × (FBW × final whole body FA content − IBW × initial whole body FA content)/(dry feed intake × FA content in the diet).
Lipid quality indexes were calculated following Ulbricht and Southgate (1991): Atherogenicity index (AI) = [C12:0 + (4 × C14:0) + C16:0] / (Σ PUFAn-6 + Σ PUFAn-3 + Σ MUFA); thrombogenicity index (TI) = (C14:0 + C16:0 + C18:0) / [0.5 × Σ MUFA + 0.5 × Σ PUFAn-6 + 3× Σ PUFAn-3 + (Σ PUFAn-3 / Σ PUFAn-6)]; hypocholesterolemic/hypercholesterolemic FA ratio (h/H) = (C18:1n-9 + C18:2n-6 + C20:4n-6 + C18:3n-3 + C20:5n-3 + C22:5n-3 + C22:6n-3) / (C14:0 + C16:0); peroxidation index (PI) = 0.025 × (% Σ monoenoic FA) + 1 × (% Σ dienoic FA) + 2 × (% Σ trienoic FA) + 4 × (% Σ tetraenoic FA) + 6 × (% Σ pentaenoic FA) + 8 × (% Σ hexaenoic FA).
Statistical analysis
Statistical analyses were performed using IBM SPSS® Statistics 27.0.1 software (IBM Corporation, Chicago, USA). Data were tested for normality and homogeneity of variances using Kolmogorov-Smirnov and Levene’s tests, respectively, followed by a one-way ANOVA. If normality and/or homogeneity of variances assumptions were not met after transformation, a non-parametric test (Kruskal–Wallis H-test) was used instead. When significant differences were observed among dietary treatments, individual means were further compared by a Tukey’s multiple comparison test. In all cases, significant differences were considered for a p < 0.05.
Results
Growth performance, whole body composition and nutrient utilisation
All diets were well accepted by the European sea bass, resulting in similar voluntary feed intake (VFI) (Table 4), and no mortality occurred during the trial. After 54 days of feeding, no significant differences were observed in the fish final body weight (208 – 217g), daily growth index or condition factor. The feed conversion ratio (1.0) and protein efficiency ratio (1.9) remained similar among groups fed the different diets (Table 4). HSI and VSI were not significantly affected by dietary treatments.
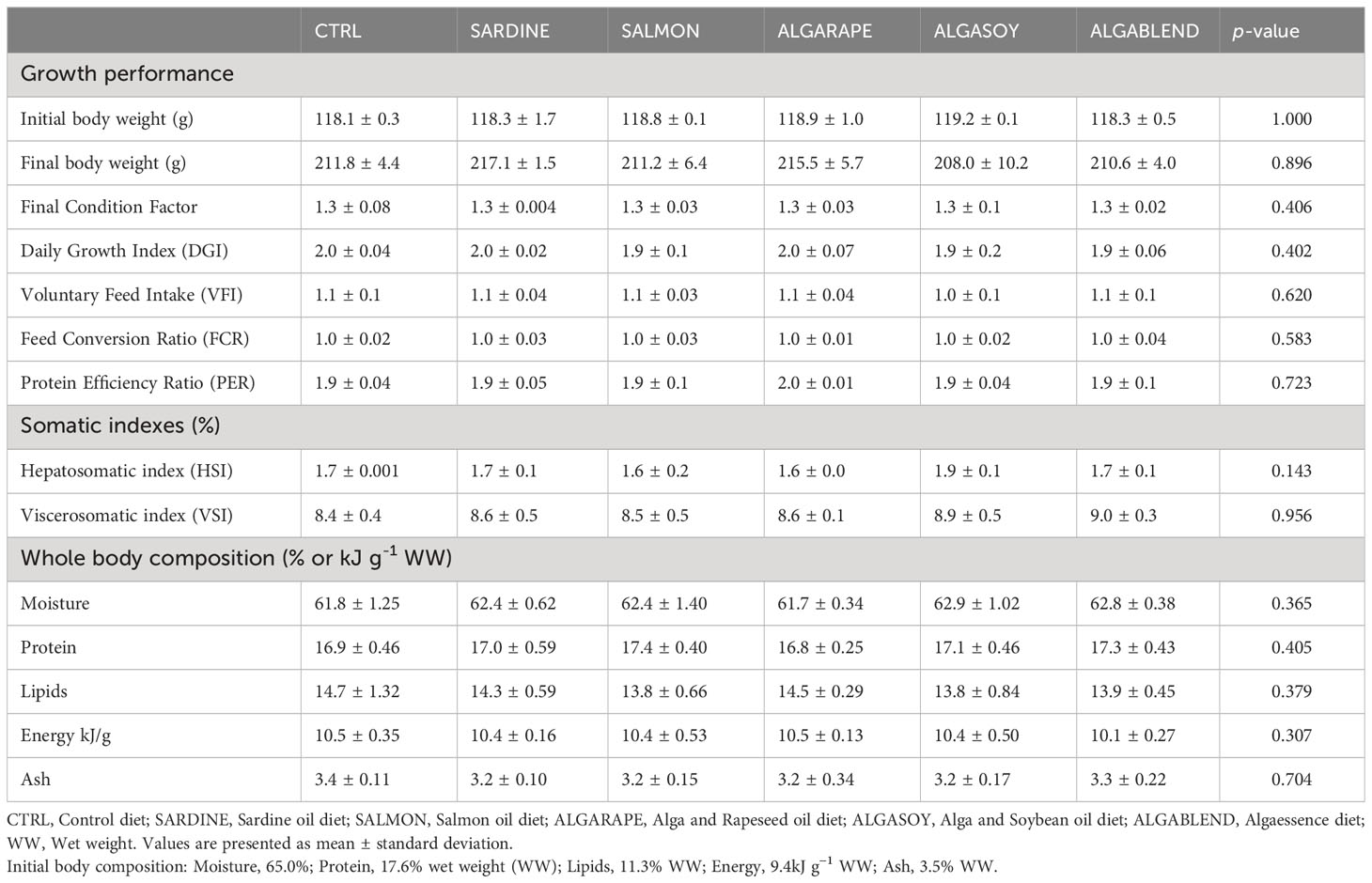
Table 4 Growth performance, somatic indexes and whole body composition of European sea bass fed experimental diets.
The changes in the dietary formulations did not significantly affect sea bass whole body final proximal composition, which remained similar among groups (Table 4). However, whole body total fatty acids profile was strongly affected by the dietary treatments, generally reflecting the dietary FA profile (Table 5; Figure 1). Whole body SFA was significantly higher in fish fed the SARDINE diet than in those fed the CTRL diet, but no other differences were found. The differences found in whole body SFA reflect the differences in palmitic acid (C16:0, PA), which was the most abundant SFA in every diet. MUFA represented the largest FAs fraction in the fish whole body, regardless of the diet (41-48%). Fish fed the CTRL diet had significantly higher MUFA levels than fish fed the diets ALGARAPE and ALGASOY (Table 5), mostly due to high percentage of OA. On the contrary, total PUFA was higher in fish fed ALGASOY or ALGARAPE than in those fed the CTRL or the SARDINE diets (Table 5). The whole body n-3/n-6 ratio was affected by the dietary treatments: the highest ratios (1.1) were observed in fish fed the SARDINE and ALGABLEND diets, and the lowest (0.7) in fish fed the ALGARAPE and ALGASOY diets. The whole-body relative content (% total FA) of either EPA or DHA were highly correlated with their respective dietary levels (R2 >0.98; Figure 1). Whole body retention of individual FA was not significantly affected by the dietary treatments (data not shown; p > 0.1), but EPA and DHA gain followed their respective dietary levels (Figure 2). EPA gain increased significantly in fish fed the SARDINE and ALGABLEND diets, whilst DHA showed the highest gain in fish fed the SALMON, ALGARAPE and ALGASOY diets (Figure 2). As a result, all dietary treatments led to a higher EPA+DHA gain when compared to the CTRL diet. The whole body EPA concentration was significantly increased in fish fed the SARDINE and ALGABLEND diets (5.7% of total FA), when compared to ALGARAPE and ALGASOY (4.1% of total FA) (Table 5). On the other hand, the whole body DHA concentration in fish fed SALMON, ALGASOY and ALGARAPE (5.9-6.1% of total FA) doubled that of fish fed the CTRL diet (3.2% of total FA). The highest DHA/EPA ratios were obtained in fish fed SALMON, ALGASOY and ALGARAPE. At the whole body level, fish fed any of the alternative diets had significantly higher EPA+DHA contents than those fed the CTRL diet (1.3 vs 1.0g 100g-1 wet weight (WW), respectively).
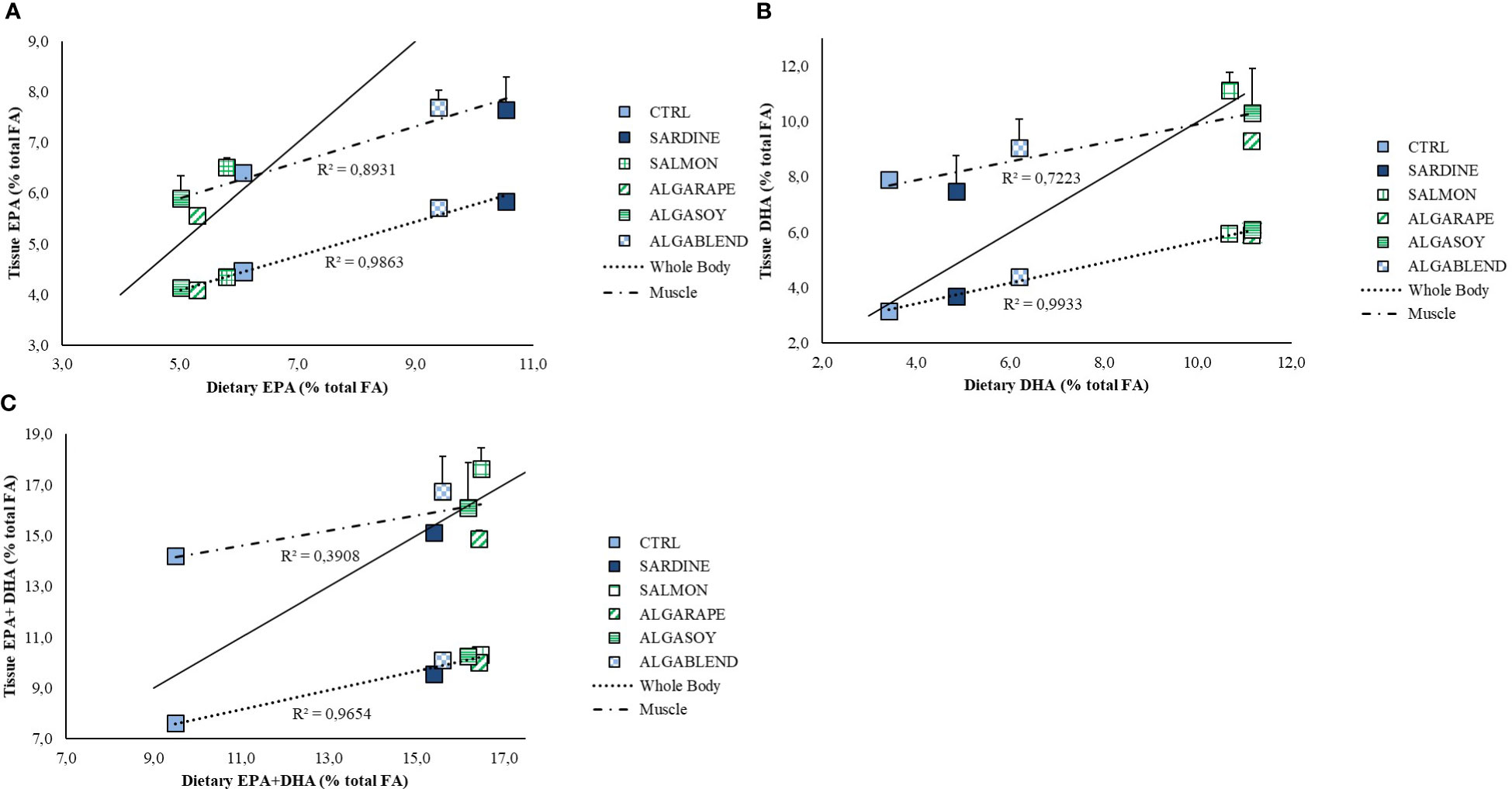
Figure 1 Relationship between dietary and whole body or muscle fatty acid content in % total fatty acids for: (A) EPA (eicosapentaenoic acid), (B) DHA (docosahexaenoic acid) and (C) EPA+DHA in European sea bass fed experimental diets for 54 days. A line of equity is represented (solid line). Data is presented as mean ± standard deviation.
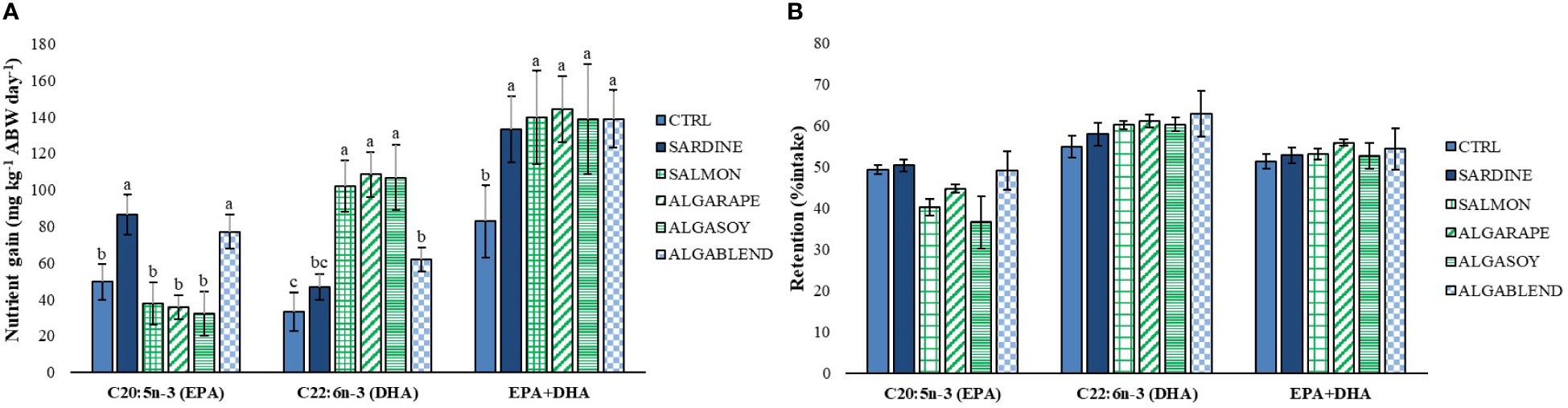
Figure 2 (A) Whole body gain and (B) retention of EPA, DHA and EPA+DHA of European seabass fed experimental diets for 54 days. CTRL, Control diet; SARDINE, Sardine oil diet; SALMON, Salmon oil diet; ALGARAPE, Alga and Rapeseed oil diet; ALGASOY, Alga and Soybean oil diet; ALGABLEND, Algaessence diet; ABW, Average body weight; Values are presented as mean ± standard deviation. Different superscript letters represent significant differences p < 0.05.
Muscle lipid content and fatty acid profile
Muscle total lipids were generally low (values ranged between 2.0 and 2.8% WW) and were not significantly affected by the dietary treatments. Muscle FA profile was strongly affected by the fat source and tended to reflect the dietary FA profile (Table 6). Muscle SFA were significantly higher in fish fed the SARDINE, ALGASOY and ALGABLEND diets, when compared to those fed the CTRL diet, mostly due to the high levels of palmitic acid. When compared to CTRL, the MUFA levels were significantly reduced in the muscle of fish fed ALGASOY, mainly due to the lower concentration of OA. The muscle PUFA was highest in fish fed ALGASOY. The high concentration of LA in the muscle of fish fed the ALGARAPE and ALGASOY resulted in the highest n-6 PUFA levels and lowest n-3/n-6 PUFA ratios (Table 6). The highest muscle EPA concentration was observed in fish fed the SARDINE and ALGABLEND diets, whilst DHA was highest in the muscle of fish fed the SALMON diet. However, the content of EPA+DHA did not vary significantly among diets. All fillets had between 303 and 369 mg EPA+DHA per 100 g wet weight. A linear correlation between the FA profile in the muscle and in the diets was established in Figure 1. A line of equity represented by a solid line corresponds to the alignment of dietary FA levels with tissue fatty acid levels. This line can suggest whether a particularly fatty acid is preferentially retained in a tissue (points above the equity line) or otherwise preferentially metabolized (points below the equity line). Both EPA and DHA tend to be preferentially retained by the muscle, but the opposite was observed in the whole body; EPA and DHA levels in the whole body are lower than those provided by the feed as evidenced by the position against the equity line (Figure 1). EPA was preferentially retained in the muscle of fish fed the ALGASOY, ALGARAPE, SALMON and CTRL diets (Figure 1A; values above the equity line), while DHA was preferentially retained in the muscle of fish fed CTRL, SARDINE, ALGABLEND and SALMON diets (Figure 1B; values above the equity line). Regarding the sum of EPA and DHA (EPA+DHA), the highest muscle retention is observed in fish fed the CTRL, the ALGABLEND, the ALGASOY and the SALMON diets (Figure 1C).
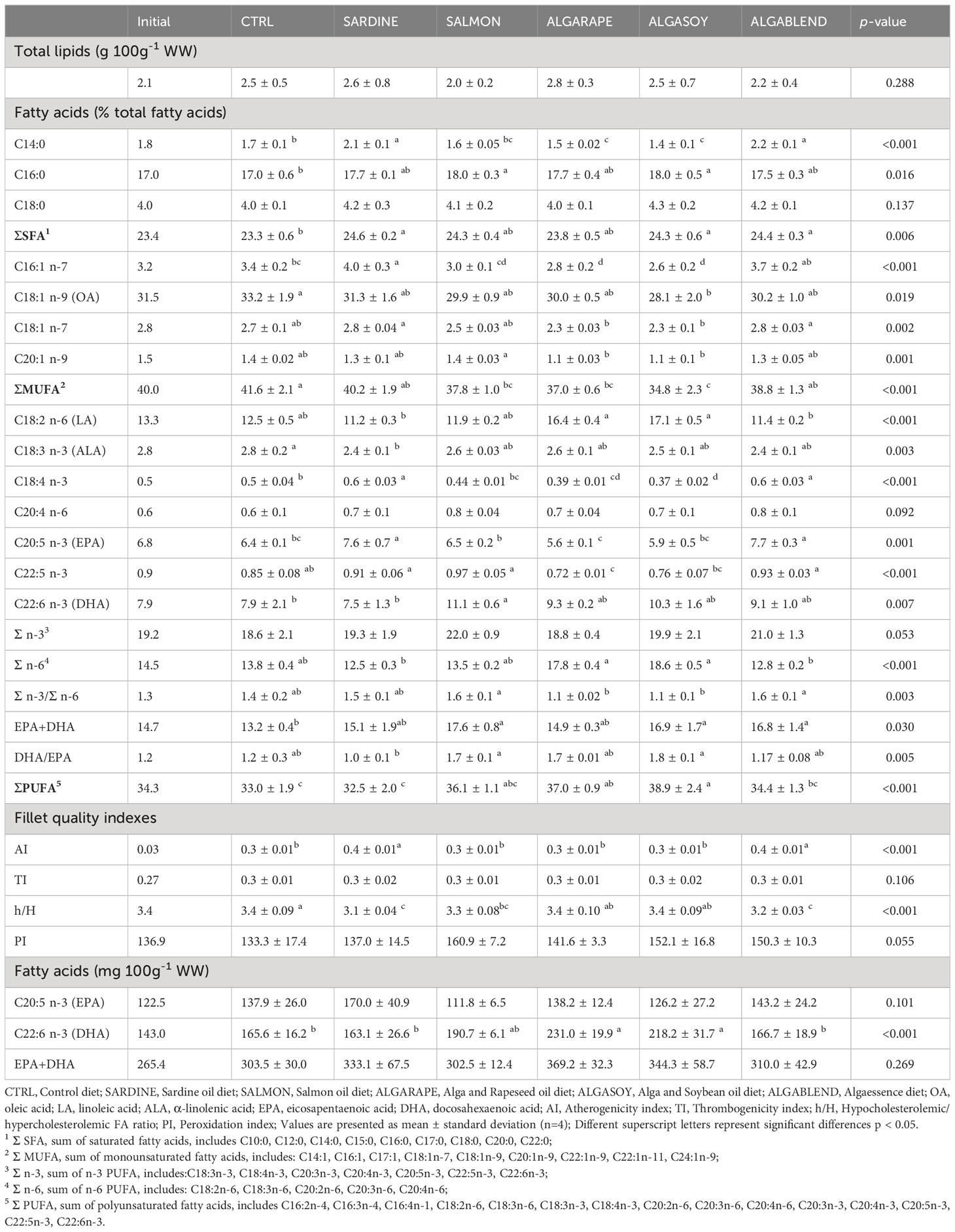
Table 6 Muscle total lipid content, quality indexes and fatty acid composition of the European sea bass fed experimental diets.
Concerning the lipid quality indexes (Table 6), the atherogenicity index (AI) was significantly higher in fillets from fish fed SARDINE and ALGABLEND when compared to the remaining diets (0.4 vs. 0.3, respectively). The hypocolesterolemic and hypercolesterolemic (h/H) FA ratio was higher in the muscle of fish fed the CTRL diet than in those fed with SARDINE, SALMON and ALGABLEND diets (3.4 vs. 3.1-3.3 respectively). No significant differences were found in thrombogenicity (TI) and peroxidation (PI) indexes between groups.
Colour and muscle texture
The skin L*, a* and the Hue (H°) angle were not affected by the dietary treatments (Table 7), but fish fed the SARDINE and ALGABLEND diets were more yellowish (higher b*) and displayed a higher colour saturation (higher C*) than those fed the CTRL diet. Muscle L* and C* were not affected by the dietary treatments, but the muscle of fish fed ALGABLEND had the highest a* and b*. The H° was highest in the muscle of fish fed the ALGARAPE diet and lowest in those fed SALMON and ALGASOY.
Concerning the fillet texture parameters, hardness, cohesiveness and chewiness were similar among groups (Table 7), but significant differences were found in adhesiveness, springiness and resilience. Fish fed the CTRL, SALMON or the ALGABLEND diets displayed a significantly higher muscle adhesiveness than fish fed the ALGARAPE diet. The highest springiness values were found in fish fed the SALMON, ALGARAPE and ALGASOY diets. Muscle of fish fed SALMON and ALGARAPE also had the highest resilience values.
Discussion
The replacement of fish oil (FO) in aquafeeds implies the identification of alternative and sustainable sources of EPA and DHA, which is nowadays one of the main challenges in aquaculture nutrition, particularly in marine fish species that have limited ability to endogenously synthesize LC-PUFA. Recent research has been focused on finding secure and cost-effective solutions to supply EPA and DHA for commercial fish diets (Tocher, 2015; Tocher et al., 2019; Santigosa et al., 2020; Santigosa et al., 2021; Kousoulaki et al., 2022). In the present study, marine n-3 LC-PUFA rich sources, such as salmon oil, algae oil and a blend of micro- and macroalgae, which are currently available in the market, proved to be viable solutions for directly replacing traditional fish oil (sardine oil - SdO) in aquafeeds. Reasonable combinations were formulated to generate diets that meet the European sea bass dietary requirements for EPA and DHA while providing consumers with differentiated fillets rich in LC-PUFA.
After a short-term growth trial of 54 days, European sea bass juveniles nearly doubled their weight, with no differences in VFI or growth performance between dietary treatments. The data showed that the performance of fish fed diets including AO in combination with either salmon-by products oil or VO was similar to fish fed the CTRL diet and within the range of values previously reported for European sea bass fed diets with FO and FM (Kaushik et al., 2004; Tibaldi et al., 2006). Moreover, neither the dietary content of EPA+DHA (1.6 in CTRL diet vs 2.5-2.8 g 100g-1 DM in the remaining experimental diets), nor the ratio n-3/n-6 (below or above 1), affected fish growth performance, which confirms that the dietary formulations met sea bass requirements for these FA. Despite the differences in the dietary lipid sources, all diets exhibited DHA/EPA ratios between 0.5-2.2, which are within the range of values (0.5-2.0) required for marine fish normal growth and development (NRC, 2011). The total absence of SdO had no significant effect on European sea bass growth, contradicting previous studies reporting growth impairment when VO was used instead of FO (Mourente et al., 2005; Yılmaz et al., 2016). This might be due to the abundance of EPA and DHA in AO that compensates for the deficiencies in VO. Microalgae oil rich in omega-3 has been successfully used without impairing growth or feed efficiency in a number of farmed species (Miller et al., 2007; Qiao et al., 2014; Betancor et al., 2016; Kousoulaki et al., 2020; Santigosa et al., 2020; Santigosa et al., 2021). Likewise, the use of a microalgae’s biomass (including Chlorella sp., Tetraselmis sp. and Schizochytrium sp.) to partially replace FM and FO in feeds for gilthead sea bream (Sparus aurata) also resulted in similar growth and feed efficiency (Ferreira et al., 2022).
Somatic indexes are good indicators of fat deposition levels, but in the present study, both HSI and VSI remained unaffected by the dietary lipid sources. This is contradictory to what has been described in several species that showed a tendency for increased HSI and VSI when dietary FO was replaced by VO (Fountoulaki et al., 2009; Bowyer et al., 2012; Willora et al., 2021). However, the present results are consistent with previous findings reported for Atlantic salmon fed diets in which FO was replaced by AO (Miller et al., 2007; Kousoulaki et al., 2022), suggesting that the incorporation of microalgae biomass is a successful strategy to mitigate the negative effects associated with high inclusion of VO.
Although proximate composition remained unaffected by the different lipid sources herein tested, the dietary FA profile clearly influenced the whole-body FA profile. Carnivorous marine species, such as European sea bass, require LC-PUFA in their diets as they have low FA bioconversion rates (Tocher, 2010). The present study shows that the fish capacity to retain n-3 LC-PUFA was not significantly affected by the dietary inclusion level of EPA and DHA. Nevertheless, the percentage of retention varied between 40-51% for EPA and 57-66% for DHA. These values are higher than those previously reported for gilthead seabream (Ferreira et al., 2022) and European seabass (Ferreira et al., 2022; Marques et al., 2022), and they are closer to those obtained for Atlantic salmon fed a FO-based diet, which retentions for EPA and DHA were 30 and 68%, respectively (Ytrestøyl et al., 2015). Moreover, European sea bass fed EPA-rich diets, containing sardine oil (SARDINE and ALGABLEND diets) had a higher EPA gain, while those fed the diets supplemented with AO, particularly rich in DHA (SALMON, ALGARAPE and ALGASOY diets), showed a higher DHA gain (Figure 2A). When compared with the CTRL, all diets promoted EPA+DHA gain, which resulted in a significant increase in fish whole body EPA+DHA content. Still, both fatty acids were metabolized at whole body level, as suggested by their correlation line below the equity line (Figure 1), whilst a selective deposition and retention of these FA was noted in muscle as previously reported in marine fish species, such as Atlantic salmon (Bell et al., 2002; Bell et al., 2003), turbot (Regost et al., 2003), Senegalese sole (Pereira et al., 2019), gilthead seabream (Fountoulaki et al., 2009) and European sea bass (Montero et al., 2005; Marques et al., 2022). In the present study, there is a trend for a higher retention of DHA, as compared to EPA (Figure 2B), which could be due to a higher beta-oxidation of EPA when compared to DHA. EPA retention also seemed to be more influenced by dietary formulation than DHA, but without significant differences among diets (Figure 2B).
From a human nutrition perspective, high n-3 LC-PUFA levels are desirable in muscle due to their health benefits, including a cardio protective effect in adults, improved immune function, cognitive function, mental and metabolic health (Simopoulos, 2000; Harris William et al., 2009). However, the higher the concentration of these FA in the diet, the more tenuous muscle retention seems to be. This was previously described by Rosenlund et al. (2016) who suggested that these FA are increasingly used for metabolism as their dietary concentrations increase. In fact, as displayed by Figure 1., EPA was more metabolized in fish fed ALGABLEND and SARDINE diets (richer in EPA), and DHA in those fed ALGASOY and ALGARAPE diets (richer in DHA), although the muscle still reflected fish dietary levels as described in previous studies (Izquierdo et al., 2005; Torrecillas et al., 2017; Santigosa et al., 2021). The balance between mechanisms underlying the selective FA deposition, namely the high specificity of fatty acyl transferases vs the relative resistance of these FA to β-oxidation likely explain the similar concentrations of EPA+DHA observed in the muscle (303-369 mg EPA+DHA per 100 g fresh weight). Dietary recommendations for EPA+DHA based on cardiovascular risk prevention for European adults are between 250 and 500 mg day-1 according to the European Food Safety Authority (EFSA, 2012). In this study, regardless the dietary EPA+DHA level, all diets were effective in providing fillets with EPA+DHA levels complying with the previously mentioned EFSA recommendations. Santigosa et al. (2020) also reported that the fillet of rainbow trout fed upon a diet with 5% AO had higher EPA+DHA content than that of trout fed a diet including 10% FO. Another study by the same author pointed out that 10% AO is enough to completely replace FO without compromising fillet quality (EPA+DHA > 250mg g-1 wet weight) (Santigosa et al., 2021), results that confirm the ability of Veramaris® oil to totally replace fish oil without compromising flesh n-3 LC-PUFA content. In the present study, when compared to the CTRL, a 10% fortification of sea bass flesh in DHA+EPA was achieved by the SARDINE diet, with a 6% price increase incurred. However, sustainable formulations with a low percentage of AO (<5%), such as ALGARAPE and ALGASOY, allowed for higher fortification (22 and 14%, respectively) at a higher cost (16-17% price increase) but remained viable, highlighting AO dietary inclusion as a feasible solution for sustainable formulations that allow for EPA and DHA fortification.
Other parameters besides total n-3 LC-PUFA have been used to evaluate the nutritional quality of fish for human consumption. The n-3/n-6 and h/H ratio, AI and TI have also been considered as useful indicators of the dietary lipid quality (Ulbricht and Southgate, 1991). In this study it is possible to observe a reduction in muscle n-3/n-6 ratio in the fish fed with either ALGARAPE or ALGASOY diets compared to those fed the remaining diets (1.1 vs. 1.4-1.6, respectively), reflecting the respective dietary composition. This was mainly due to the dietary replacement of salmon oil by the blend of RO and SyO, which have a high LA content. VO are rich in LA and contain ALA, but totally lack EPA and DHA, unbalancing its n-3/n-6 ratio towards values lower than 1. Therefore, high FO replacement levels by VO may decrease the benefits of eating fish as suggested by Simopoulos (2012), not only by decreasing EPA and DHA levels in muscle, but also because excessive intake of n-6 PUFA and consequently low n-3/n-6 ratio can have adverse health effects promoting the development/appearance of cardiovascular diseases, cancer, osteoporosis and inflammatory and autoimmune diseases (Okuyama et al., 1996; Simopoulos, 2016; Yue et al., 2021). Such FA imbalances in muscle derived from low dietary n-3/n-6 ratio might also inhibit the biosynthesis of the physiologically active n-3 LC-PUFA and hence decrease the EPA and DHA deposition levels (Power and Newsholme, 1997; Bandarra et al., 2011). Although in the present study the total replacement of sardine oil by a blend of VO and AO did not impair EPA and DHA muscle levels, these fish still had the lowest n-3/n-6 ratio. Likewise, muscle h/H ratio was affected by the experimental diets being lower in the fish fed the SARDINE, SALMON and ALGABLEND diets (h/H = 3.1 to 3.3) comparatively to the remaining. This was mainly associated with the higher content of SFA in the muscle of these fish, namely the myristic (C14:0) and palmitic acids (C16:0), resulting from a large abundance of these FA in the ingredients included in the testing diets, such as sardine oil (6.7% C14:0 and 16.7% of C16:0) and the algae’s blend (31.3% C14:0 and 15.7% of C16:0). To the best of our knowledge, there are no reference values for h/H ratio, but as suggested by Santos-Silva et al. (2002) and according to the current knowledge on the effects of specific FA on cholesterol metabolism, higher values of h/H should be considered as positive. Comparing the present results on h/H ratio with those reported in a previous study by Testi et al. (2006) in seawater-reared European sea bass selected from stocks of ready-for-sale fish (h/H = 2.1), all fish fed the experimental diets herein tested had higher h/H ratios.
Atherogenicity (AI) and thrombogenicity index (TI) are indicators of the potential impact of food on cardiovascular health. AI is defined by the ratio between the sum of the main SFA and MUFA, the first being considered pro-atherogenic (Σ SFA), and the latter anti-atherogenic (Σ PUFA n-6 + Σ PUFA n-3 + Σ MUFA), whilst TI indicates a propensity for clot formation in blood vessels and it is defined as the relationship between the pro-thrombogenetic and the anti-thrombogenetic FA (Σ SFA and Σ PUFA n-6 + Σ PUFA n-3 + Σ MUFA, respectively) (Ulbricht and Southgate, 1991). Although lower AI and TI values indicate higher nutritional quality and greater potential for reducing the risk of coronary heart disease, no recommended values for IA and IT have been established yet (Chen and Liu, 2020). Furthermore, several factors such as environmental conditions, fish weight and fish age may affect these indexes, but they seem to be predominantly related to diet, which is known to impact the FA profile (Grigorakis, 2007). The TI values herein obtained (0.2-0.3) were not affected by dietary treatments and were in line with those reported for same-sized cultured European sea bass (Alasalvar et al., 2002; Testi et al., 2006). On the other hand, the highest AI values were found in the fillets of fish fed SARDINE and ALGABLEND diets, which had sardine oil as main lipid source. Grigorakis (2007) reported even higher AI values (0.6) in market-sized European sea bass fed a FO-diet. Moreover, Álvarez et al. (2020) found that replacing FO with VO (soybean and rapeseed oils) in gilthead seabream (Sparus aurata) led to decreased AI values, which is consistent with the present data. In conclusion, replacing dietary sardine oil by alternative lipid sources can decrease AI and potentially enhance flesh fat quality. Nevertheless, it is important to keep in mind that fish still have lower AI and TI values compared to other food sources, making them an excellent choice for maintaining heart health (Olmedilla-Alonso et al., 2002).
Lipid peroxidation index (PI), represent the relationship between the FA composition of a tissue and its susceptibility to oxidation, being PUFA more sensitive to oxidation than MUFA (Hulbert et al., 2007). Despite the higher PUFA content in the muscle of fish fed the diets including AO (SALMON, ALGARAPE and ALGASOY), PI was not significantly affected. However, the flesh of fish fed the SALMON diet displayed the highest PI, which seems to indicate a higher susceptibility for FA oxidation. Further analysis throughout post-mortem could clarify the extent of the impact of dietary lipid sources on lipid oxidation.
The organoleptic quality of a final product is just as important as its nutritional value because it can influence a consumer’s purchase decision (Ramalho Ribeiro et al., 2015; Álvarez et al., 2020; Batista et al., 2020). In this study, fish fed the ALGABLEND diet showed increased colour saturation (more precisely the b*) and a more yellowish skin. Likewise, the fillet of fish fed the ALGABLEND diet had a tendency towards a more intense red (higher a* value) and yellow (higher b* value) colour, without differing significantly from the CTRL. Batista et al. (2020) also reported higher a* values in fillets of European sea bass fed with a diet containing 8% of the algae Gracilaria sp. These findings suggest that algae, being good sources of pigments, could improve the appearance of fish. Texture is also one of the most important quality attributes of fish and meat. It contributes to consumer acceptance and therefore marketability of the final product (Bland et al., 2018). Hardness is probably the most easily textural parameter perceived by consumers that generally appreciate a high muscle hardness (Cheng et al., 2014). In this study, it was found that SdO could be totally replaced by different reasonable combinations of alternative lipid sources without altering flesh hardness. This could be partly related to the absence of significant differences in muscle lipid and moisture contents, which are important chemical variables for flesh texture (Johnston et al., 2006; Xu et al., 2021). Moreover, springiness was significantly increased in diets containing AO, and resilience was lowest in fish fed with SARDINE and ALGABLEND diets (SdO inclusion > 7.9%). However, longer feeding trials and further validation by a sensorial panel are needed to fully determine the impact of alternative lipid sources on sea bass nutritional and sensory properties.
Conclusion
In this study, various marine n-3 LC-PUFA rich sources (salmon oil, algae oil and a blend of micro and macroalgae, Algaessence Feed™), currently available in the market, were demonstrated to be viable solutions for the direct replacement of a traditional fish oil (sardine oil - SdO) in diets for European sea bass, contributing to the environmental and economical sustainability of aquaculture. After a 54 days’ trial, all diets promoted fish growth equally and provided consumers differentiated fillets rich in EPA+DHA, with over 250 mg of EPA+DHA per 100 g fillet complying with EFSA recommended daily intakes to reduce the risk of cardiovascular diseases (>250 mg day-1), and without major impacts on texture. Still, the flesh of fish fed ALGARAPE and ALGASOY had the highest DHA concentrations due to the dietary inclusion of a DHA rich algae oil. SALMON and ALGABLEND fed fish displayed the highest PUFA n-3/n-6 ratios. Overall, this study showed that combining pricy sources of n-3 LC-PUFA (Veramaris® or Algaessence Feed™) with less expensive oils (salmon by-products oil or vegetable oils) was a successful strategy to fortify European sea bass flesh with EPA and DHA while mitigating the negative effects associated with high dietary inclusions of vegetable oils. However, based on FA retention, there was no apparent advantage in increasing dietary n-3 LC-PUFA in European sea bass diets, as there appears to be a threshold above which β-oxidation seems to be promoted. Future studies are required to determine the most adequate dietary levels of EPA and DHA to promote high retentions in large-sized European sea bass while ensuring desirable nutritional flesh quality and the cost-effectiveness of aquafeeds.
Data availability statement
The original contributions presented in the study are included in the article/supplementary material. Further inquiries can be directed to the corresponding author.
Ethics statement
Fish trials were conducted in compliance with the guidelines of the European Union (Directive 2010/63/EU) and approved by the Ethical Committee of Riasearch Lda., Murtosa, Portugal, overseen by the National Competence Authority. The study was conducted in accordance with the local legislation and institutional requirements.
Author contributions
AM: Methodology, validation, formal analysis, investigation, validation and writing review and editing. PC: validation and writing review. CC and FP: investigation and formal analysis. AB: investigation and writing review. MS: formal Analysis, funding acquisition and writing review. HA and JD: conceptualization and funding Acquisition. LV: conceptualization, methodology, resources, writing review & editing, supervision, project administration and funding acquisition. All authors contributed to the article and approved the submitted version.
Funding
This work is a result of the project OmegaPeixe (POCI-01-0247-FEDER-069748), supported by Operational Program for Competitiveness and Internationalization (COMPETE 2020), under the PORTUGAL 2020 Partnership Agreement, through the European Regional Development Fund (ERDF) and by FCT -Foundation for Science and Technology to CIIMAR (UIDB/04423/2020, UIDP/04423/2020). PC was supported through an ARDITI grant funded by the M1420-09-5369-0002 project.
Conflict of interest
Author HA was employed by the company Produção e Comercialização de Algas e seus Derivados Lda. and author JD was employed by the companies SPAROS Lda. and RIASEARCH Lda.
The remaining authors declare that the research was conducted in the absence of any commercial or financial relationships that could be construed as a potential conflict of interest.
The reviewer DF declared a past co-authorship with the author LV to the handling editor.
Publisher’s note
All claims expressed in this article are solely those of the authors and do not necessarily represent those of their affiliated organizations, or those of the publisher, the editors and the reviewers. Any product that may be evaluated in this article, or claim that may be made by its manufacturer, is not guaranteed or endorsed by the publisher.
References
Adarme-Vega T. C., Lim D. K. Y., Timmins M., Vernen F., Li Y., Schenk P. M. (2012). Microalgal biofactories: a promising approach towards sustainable omega-3 fatty acid production. Microbiol. Cell Factories 11, 96. doi: 10.1186/1475-2859-11-96
Alasalvar C., Taylor K. D. A., Zubcov E., Shahidi F., Alexis M. (2002). Differentiation of cultured and wild sea bass (Dicentrarchus labrax): total lipid content, fatty acid and trace mineral composition. Food Chem. 79, 145–150. doi: 10.1016/S0308-8146(02)00122-X
Álvarez A., Fontanillas R., Hernández-Contreras A., Hernández M. D. (2020). Partial replacement of fish oil with vegetal oils in commercial diets: the effect on the quality of gilthead seabream (Sparus aurata). Anim. Fedd Sci. Technol. 265, 114504. doi: 10.1016/j.anifeedsci.2020.114504
AOAC (Ed.) (2006). Official methods of analysis of AOAC International 18 ed. (Maryland, USA: AOAC International).
Bandarra N. M., Rema P., Batista I., Pousão-Ferreira P., Valente L. M. P., Batista S. M. G., et al. (2011). Effects of dietary n–3/n–6 ratio on lipid metabolism of gilthead seabream (Sparus aurata). Eur. J. Lipid Sci. Technol. 113, 1332–1341. doi: 10.1002/ejlt.201100087
Batista S., Pereira R., Oliveira B., Baião L. F., Jessen F., Tulli F., et al. (2020). Exploring the potential of seaweed Gracilaria gracilis and microalga Nannochloropsis oceanica, single or blended, as natural dietary ingredients for European seabass Dicentrarchus labrax. J. Appl. Phycol. 32, 2041–2059. doi: 10.1007/s10811-020-02118-z
Bell J. G., Henderson R. J., Tocher D. R., Mcghee F., Dick J. R., Porter A., et al. (2002). Substituting fish oil with crude palm oil in the diet of atlantic salmon (Salmo salar) affects muscle fatty acid composition and hepatic fatty acid metabolism. J. Nutr. 132, 222–230. doi: 10.1093/jn/132.2.222
Bell J. G., Tocher D. R., Henderson R. J., Dick J. R., Crampton V. O. (2003). Altered fatty acid compositions in atlantic salmon (Salmo salar) fed diets containing linseed and rapeseed oils can be partially restored by a subsequent fish oil finishing diet. J. Nutr. 133, 2793–2801. doi: 10.1093/jn/133.9.2793
Betancor M. B., Sprague M., Montero D., Usher S., Sayanova O., Campbell P. J., et al. (2016). Replacement of marine fish oil with de novo omega-3 oils from transgenic camelina sativa in feeds for Gilthead sea bream (Sparus aurata L.). Lipids 51, 1171–1191. doi: 10.1007/s11745-016-4191-4
Bland J. M., Bett-Garber K. L., Li C. H., Brashear S. S., Lea J. M., Bechtel P. J. (2018). Comparison of sensory and instrumental methods for the analysis of texture of cooked individually quick frozen and fresh-frozen catfish fillets. Food Sci. Nutr. 6, 1692–1705. doi: 10.1002/fsn3.737
Bowyer J. N., Qin J. G., Smullen R. P., Stone D. (2012). Replacement of fish oil by poultry oil and canola oil in yellowtail kingfish (Seriola lalandi) at optimal and suboptimal temperatures. Aquaculture, 356–357. doi: 10.1016/j.aquaculture.2012.05.014
Campos I., Pinheiro Valente L. M., Matos E., Marques P., Freire F. (2020). Life-cycle assessment of animal feed ingredients: Poultry fat, poultry by-product meal and hydrolyzed feather meal. J. Cleaner Prod. 252, 119845. doi: 10.1016/j.jclepro.2019.119845
Chauton M. S., Reitan K. I., Norsker N. H., Tveterås R., Kleivdal H. T. (2015). A techno-economic analysis of industrial production of marine microalgae as a source of EPA and DHA-rich raw material for aquafeed: Research challenges and possibilities. Aquaculture 436, 95–103. doi: 10.1016/j.aquaculture.2014.10.038
Chen J., Liu H. (2020). Nutritional indices for assessing fatty acids: A mini-review. Int. J. Mol. Sci. 21 (16), 5695. doi: 10.3390/IJMS21165695
Cheng J.-H., Sun D.-W., Han Z., Zeng X.-A. (2014). Texture and structure measurements and analyses for evaluation of fish and fillet freshness quality: a review. Compr. Rev. Food Sci. Food Saf. 13, 52–61. doi: 10.1111/1541-4337.12043
Choubert G., Blanc J. M., Vallée F. (1997). Colour measurement, using the CIELCH colour space, of muscle of rainbow trout, Oncorhynchus mykiss (Walbaum), fed astaxanthin: effects of family, ploidy, sex, and location of reading. Aquacult. Res. 28, 15–22. doi: 10.1046/j.1365-2109.1997.t01-1-00824.x
Deepika D., Vegneshwaran V., Julia P., Sukhinder K., Sheila T., Heather M., et al. (2014). Investigation on oil extraction methods and its Influence on omega-3 content from cultured salmon. J. Food Process. Technol. 5:1–13. doi: 10.4172/2157-7110.1000401
Dikel S., Engin K., Arslan M. (2013). Partial replacement of fish oil with vegetable oils in diets for european seabass (Dicentrarchus labrax): effects on growth performance and fatty acids profile. Turkish J. Fisheries Aquat. Sci. 13, 819–825. doi: 10.4194/1303-2712-v13_3_05
EFSA (2012). Scientific Opinion on the Tolerable Upper Intake Level of eicosapentaenoic acid (EPA), docosahexaenoic acid (DHA) and docosapentaenoic acid (DPA). EFSA J. 10:1–48. doi: 10.2903/j.efsa.2012.2815
FAO (2022). The state of worl fisheries and aquaculture 2022 - Towards Blue Transformation. Rome, FAO. doi: 10.4060/cc0461en
Ferreira M., Ribeiro P. C., Ribeiro L., Barata M., Domingues V. F., Sousa S., et al. (2022). Biofortified diets containing algae and selenised yeast: Effects on growth performance, nutrient utilization, and tissue composition of gilthead seabream (Sparus aurata). Front. Physiol. 12. doi: 10.3389/fphys.2021.812884
Ferreira M., Teixeira C., Abreu H., Silva J., Costas B., Kiron V., et al. (2021). Nutritional value, antimicrobial and antioxidant activities of micro- and macroalgae, single or blended, unravel their potential use for aquafeeds. J. Appl. Phycol. 33, 3507–3518. doi: 10.1007/s10811-021-02549-2
Folch J., Lees M., Sloane-Stanley G. H. (1957). A simple method for the isolation and purification of total lipides from animal tissues. J. Biol. Chem. 226 (1), 497–506. doi: 10.1016/S0021-9258(18)64849-5
Fountoulaki E., Vasilaki A., Hurtado R., Grigorakis K., Karacostas I., Nengas I., et al. (2009). Fish oil substitution by vegetable oils in commercial diets for gilthead sea bream (Sparus aurata L.); effects on growth performance, flesh quality and fillet fatty acid profile: Recovery of fatty acid profiles by a fish oil finishing diet under fluctuating water temperatures. Aquaculture 289, 317–326. doi: 10.1016/j.aquaculture.2009.01.023
Garcia T., Cardoso C., Afonso C., Gomes A., Mesquita C., Tanni S., et al. (2019). A study of lipid bioaccessibility in canned sardine (Sardina pilchardus) and chub mackerel (Scomber japonicus). J. Aquat. Food Prod. Technol. 28, 402–412. doi: 10.1080/10498850.2019.1594481
Glencross B. D. (2009). Exploring the nutritional demand for essential fatty acids by aquaculture species. Rev. Aquacult. 1, 71–124. doi: 10.1111/j.1753-5131.2009.01006.x
Grigorakis K. (2007). Compositional and organoleptic quality of farmed and wild gilthead sea bream (Sparus aurata) and sea bass (Dicentrarchus labrax) and factors affecting it: A review. Aquaculture 272, 55–75. doi: 10.1016/j.aquaculture.2007.04.062
Harris William S., Mozaffarian D., Rimm E., Kris-Etherton P., Rudel Lawrence L., Appel Lawrence J., et al. (2009). Omega-6 fatty acids and risk for cardiovascular disease. Circulation 119, 902–907. doi: 10.1161/CIRCULATIONAHA.108.191627
Hulbert A. J., Pamplona R., Buffenstein R., Buttemer W. A. (2007). Life and death: metabolic rate, membrane composition, and life span of animals. Physiol. Rev. 87, 1175–1213. doi: 10.1152/physrev.00047.2006
Izquierdo M. S., Montero D., Robaina L., Caballero M. J., Rosenlund G., Ginés R. (2005). Alterations in fillet fatty acid profile and flesh quality in gilthead seabream (Sparus aurata) fed vegetable oils for a long term period. Recovery of fatty acid profiles by fish oil feeding. Aquaculture 250, 431–444. doi: 10.1016/j.aquaculture.2004.12.001
Izquierdo M. S., Obach A., Arantzamendi L., Montero D., Robaina L., Rosenlund G. (2003). Dietary lipid sources for seabream and seabass: growth performance, tissue composition and flesh quality. Aquacult. Nutr. 9, 397–407. doi: 10.1046/j.1365-2095.2003.00270.x
Johnston I. A., Li X., Vieira V. L. A., Nickell D., Dingwall A., Alderson R., et al. (2006). Muscle and flesh quality traits in wild and farmed Atlantic salmon. Aquaculture 256, 323–336. doi: 10.1016/j.aquaculture.2006.02.048
Kaushik S. J., Covès D., Dutto G., Blanc D. (2004). Almost total replacement of fish meal by plant protein sources in the diet of a marine teleost, the European seabass, Dicentrarchus labrax. Aquaculture 230, 391–404. doi: 10.1016/S0044-8486(03)00422-8
Kiron V., Phromkunthong W., Huntley M., Archibald I., De Scheemaker G. (2012). Marine microalgae from biorefinery as a potential feed protein source for Atlantic salmon, common carp and whiteleg shrimp. Aquacult. Nutr. 18, 521–531. doi: 10.1111/j.1365-2095.2011.00923.x
Klinger D., Naylor R. (2012). Searching for solutions in aquaculture: charting a sustainable course. Annu. Rev. Environ. Resour. 37, 247–276. doi: 10.1146/annurev-environ-021111-161531
Kousoulaki K., Gerd Marit B., Mørkøre T., Krasnov A., Baeverfjord G., Ytrestøyl T., et al. (2020). Microalgal schizochytrium limacinum biomass improves growth and filet quality when used long-term as a replacement for fish oil, in modern salmon diets. Front. Mar. Sci. 7. doi: 10.3389/fmars.2020.00057
Kousoulaki K., Sveen L., Norén F., Espmark Å. (2022). Atlantic Salmon (Salmo salar) performance fed low trophic ingredients in a fish meal and fish oil free diet. Front. Physiol. 13. doi: 10.3389/fphys.2022.884740
Lepage G., Roy C. C. (1986). Improved recovery of fatty acid through direct transesterification without prior extraction or purification. J. Lipid Res. 25, 1391–1396. doi: 10.1016/S0022-2275(20)34457-6
Li N., Wu X., Zhuang W., Xia L., Chen Y., Wu C., et al. (2020). Fish consumption and multiple health outcomes: umbrella review. Trends Food Sci. Technol. 99, 273–283. doi: 10.1016/j.tifs.2020.02.033
Marques A., Matos E., Aires T., Melo D., Oliveira M. B. P. P., Valente L. M. P. (2022). Understanding the interaction between terrestrial animal fat sources and dietary emulsifier supplementation on muscle fatty acid profile and textural properties of European sea bass. Aquaculture 560, 738547. doi: 10.1016/j.aquaculture.2022.738547
Miller M. R., Nichols P. D., Carter C. G. (2007). Replacement of fish oil with thraustochytrid Schizochytrium sp. L oil in Atlantic salmon parr (Salmo salar L) diets. Comp. Biochem. Physiol. Part A.: Mol. Integr. Physiol. 148, 382–392. doi: 10.1016/j.cbpa.2007.05.018
Monroig O., Tocher D. R., Castro L. F. C. (2018). “Chapter 3 - polyunsaturated fatty acid biosynthesis and metabolism in fish,” in Polyunsaturated Fatty Acid Metabolism. Ed. BURDGE G. C. (AOCS Press).
Montero D., Robaina L., Caballero M. J., Ginés R., Izquierdo M. S. (2005). Growth, feed utilization and flesh quality of European sea bass (Dicentrarchus labrax) fed diets containing vegetable oils: a time-course study on the effect of a re-feeding period with a 100% fish oil diet. Aquaculture 248, 121–134. doi: 10.1016/j.aquaculture.2005.03.003
Mourente G., Bell J. G. (2006). Partial replacement of dietary fish oil with blends of vegetable oils (rapeseed, linseed and palm oils) in diets for European sea bass (Dicentrarchus labrax L.) over a long term growth study: effects on muscle and liver fatty acid composition and effectiveness of a fish oil finishing diet. Comp. Biochem. Physiol. Part B.: Biochem. Mol. Biol. 145, 389–399. doi: 10.1016/j.cbpb.2006.08.012
Mourente G., Dick J. R., Bell J. G., Tocher D. R. (2005). Effect of partial substitution of dietary fish oil by vegetable oils on desaturation and β-oxidation of [1-14C]18:3n–3 (LNA) and [1-14C]20:5n–3 (EPA) in hepatocytes and enterocytes of European sea bass (Dicentrarchus labrax L.). Aquaculture 248, 173–186. doi: 10.1016/j.aquaculture.2005.04.023
Nasopoulou C., Zabetakis I. (2012). Benefits of fish oil replacement by plant originated oils in compounded fish feeds. A review. LWT 47, 217–224. doi: 10.1016/j.lwt.2012.01.018
Naylor R. L., Hardy R. W., Bureau D. P., Chiu A., Elliott M., Farrell A. P., et al. (2009). Feeding aquaculture in an era of finite resources. Proc. Natl. Acad. Sci. 106, 15103. doi: 10.1073/pnas.0905235106
Naylor R. L., Kishore A., Sumaila U. R., Issifu I., Hunter B. P., Belton B., et al. (2021). Blue food demand across geographic and temporal scales. Nat. Commun. 12, 5413. doi: 10.1038/s41467-021-25516-4
NRC (2011). Nutrient requirements of fish and shrimp. 1st ed (Washington, DC, USA: The National Academy).
Okuyama H., Kobayashi T., Watanabe S. (1996). Dietary fatty acids - The n-6n-3 balance and chronic elderly diseases. Excess linoleic acid and relative n-3 deficiency syndrome seen in Japan. Prog. Lipid Res. 35, 409–457. doi: 10.1016/S0163-7827(96)00012-4
Olmedilla-Alonso B., Granado-Lorencio F., Herrero-Barbudo C., Blanco-Navarro I. (2002). Comparison of the effects of egg yolk, fish oil and palm oil on serum lipids and lipoproteins in normolipidemic subjects. Eur. J. Clin. Nutr. 56, 352–357. doi: 10.1038/sj.ejcn.1601311
Pereira R., Basto A., Conde-Sieira M., Linares F., Rodríguez Villanueva J. L., Sieira G. P., et al. (2019). Growth performance and nutrient utilisation of Senegalese sole fed vegetable oils in plant protein-rich diets from juvenile to market size. Aquaculture 511, 734229. doi: 10.1016/j.aquaculture.2019.734229
Power G. W., Newsholme E. A. (1997). Dietary fatty acids influence the activity and metabolic control of mitochondrial carnitine palmitoyltransferase I in rat heart and skeletal muscle. J. Nutr. 127, 2142–2150. doi: 10.1093/jn/127.11.2142
Qiao H., Wang H., Song Z., Ma J., Li B., Liu X., et al. (2014). Effects of dietary fish oil replacement by microalgae raw materials on growth performance, body composition and fatty acid profile of juvenile olive flounder, Paralichthys olivaceus. Aquacult. Nutr. 20, 646–653. doi: 10.1111/anu.12127
Ramalho Ribeiro A., Gonçalves A., Colen R., Nunes M. L., Dinis M. T., Dias J. (2015). Dietary macroalgae is a natural and effective tool to fortify gilthead seabream fillets with iodine: effects on growth, sensory quality and nutritional value. Aquaculture 437, 51–59. doi: 10.1016/j.aquaculture.2014.11.028
Regost C., Arzel J., Robin J., Rosenlund G., Kaushik S. J. (2003). Total replacement of fish oil by soybean or linseed oil with a return to fish oil in turbot (Psetta maxima). Growth performance, flesh fatty acid profile, and lipid metabolism. Aquaculture 217, 465–482. doi: 10.1016/S0044-8486(02)00259-4
Rosenlund G., Torstensen B. E., Stubhaug I., Usman N., Sissener N. H. (2016). Atlantic salmon require long-chain n-3 fatty acids for optimal growth throughout the seawater period. J. Nutr. Sci. 5, 19. doi: 10.1017/jns.2016.10
Santigosa E., Brambilla F., Milanese L. (2021). Microalgae oil as an effective alternative source of EPA and DHA for gilthead seabream (Sparus aurata) aquaculture. Animals 11:1–17. doi: 10.3390/ani11040971
Santigosa E., Constant D., Prudence D., Wahli T., Verlhac-Trichet V. (2020). A novel marine algal oil containing both EPA and DHA is an effective source of omega-3 fatty acids for rainbow trout (Oncorhynchus mykiss). J. World Aquacult. Soc. 51, 649–665. doi: 10.1111/jwas.12699
Santos-Silva J., Bessa R. J. B., Santos-Silva F. (2002). Effect of genotype, feeding system and slaughter weight on the quality of light lambs: II. Fatty acid composition of meat. Livestock Prod. Sci. 77, 187–194. doi: 10.1016/S0301-6226(02)00059-3
Simopoulos A. P. (2000). Human requirement for n-3 polyunsaturated fatty acids. Poultry Sci. 79, 961–970. doi: 10.1093/ps/79.7.961
Simopoulos (2012). Health effects of polyunsaturated fatty acids in seafoods [edition unavailable] (Elsevier Science). Available at: https://www.perlego.com/book/1857929/health-effects-of-polyunsaturated-fatty-acids-in-seafoods-pdf (Accessed: 15 October 2022).
Simopoulos A. P. (2016). Evolutionary aspects of the dietary omega–6:omega–3 fatty acid ratio: medical implications. In: A. Alvergne, C. Jenkinson, C. Faurie (eds), Evolutionary thinking in medicine. Advances in the evolutionary analysis of human behaviour. (Cham: Springer). doi: 10.1007/978-3-319-29716-3_9
Testi S., Bonaldo A., Gatta P. P., Badiani A. (2006). Nutritional traits of dorsal and ventral fillets from three farmed fish species. Food Chem. 98, 104–111. doi: 10.1016/j.foodchem.2005.05.053
Tibaldi E., Hakim Y., Uni Z., Tulli F., De Francesco M., Luzzana U., et al. (2006). Effects of the partial substitution of dietary fish meal by differently processed soybean meals on growth performance, nutrient digestibility and activity of intestinal brush border enzymes in the European sea bass (Dicentrarchus labrax). Aquaculture 261, 182–193. doi: 10.1016/j.aquaculture.2006.06.026
Tocher D. R. (2010). Fatty acid requirements in ontogeny of marine and freshwater fish. Aquacult. Res. 41, 717–732. doi: 10.1111/j.1365-2109.2008.02150.x
Tocher D. R. (2015). Omega-3 long-chain polyunsaturated fatty acids and aquaculture in perspective. Aquaculture 449, 94–107. doi: 10.1016/j.aquaculture.2015.01.010
Tocher D. R., Betancor M. B., Sprague M., Olsen R. E., Napier J. A. (2019). Omega-3 long-chain polyunsaturated fatty acids, EPA and DHA: bridging the gap between supply and demand. Nutrients 11:1–20. doi: 10.3390/nu11010089
Torrecillas S., Robaina L., Caballero M. J., Montero D., Calandra G., Mompel D., et al. (2017). Combined replacement of fishmeal and fish oil in European sea bass (Dicentrarchus labrax): production performance, tissue composition and liver morphology. Aquaculture 474, 101–112. doi: 10.1016/j.aquaculture.2017.03.031
Turchini G. M., Ng W.-K., Tocher D. R. (2010). Fish oil replacement and alternative lipid sources in aquaculture feeds (Boca Raton, FL 33487-2742: CRC Press).
Turchini G. M., Torstensen B. E., Ng W.-K. (2009). Fish oil replacement in finfish nutrition. Rev. Aquacult. 1, 10–57. doi: 10.1111/j.1753-5131.2008.01001.x
Ulbricht T. L. V., Southgate D. (1991). Coronary heart disease: seven dietary factors. Lancet 338, 985–992. doi: 10.1016/0140-6736(91)91846-M
Weihrauch J. L., Posati L. P., Anderson B. A., Exler J. (1977). Lipid conversion factors for calculating fatty acid contents of foods. J. Am. Oil Chem. Soc. 54, 36–40. doi: 10.1007/BF02671370
Willora F. P., Grønevik B., Liu C., Palihawadana A., Sørensen M., Hagen Ø. (2021). Total replacement of marine oil by rapeseed oil in plant protein rich diets of juvenile lumpfish (Cyclopterus lumpus): Effects on growth performance, chemical and fatty acid composition. Aquacult. Rep. 19, 100560. doi: 10.1016/j.aqrep.2020.100560
Xu H., Bi Q., Liao Z., Sun B., Jia L., Wei Y., et al. (2021). Long-term alternate feeding between fish oil- and terrestrially sourced oil-based diets mitigated the adverse effects of terrestrially sourced oils on turbot fillet quality. Aquaculture 531, 735974. doi: 10.1016/j.aquaculture.2020.735974
Xu H., Turchini G. M., Francis D. S., Liang M., Mock T. S., Rombenso A., et al. (2020). Are fish what they eat? A fatty acid’s perspective. Prog. Lipid Res. 80, 101064. doi: 10.1016/j.plipres.2020.101064
Yılmaz H. A., Corraze G., Panserat S., Eroldoğan O. T. (2016). Effects of alternate feeding with different lipid sources on fatty acid composition and bioconversion in European sea bass (Dicentrarchus labrax). Aquaculture 464, 28–36. doi: 10.1016/j.aquaculture.2016.06.013
Ytrestøyl T., Aas T. S., Åsgård T. (2015). Utilisation of feed resources in production of Atlantic salmon (Salmo salar) in Norway. Aquaculture 448, 365–374. doi: 10.1016/j.aquaculture.2015.06.023
Ytrestøyl T., Aas T. S., Berge G. M., Hatlen B., Sørensen M., Ruyter B., et al. (2011). Resource utilisation and eco-efficiency of norwegian salmon farming in 2010. Nofima Rapportserie, 11250/283692.
Yue H., Liu W., Zhang W., Jia M., Huang F., Du F., et al. (2021). Dietary low ratio of n-6/n-3 polyunsaturated fatty acids improve type 2 diabetes mellitus via activating brown adipose tissue in male mice. J. Food Sci. 86, 1058–1065. doi: 10.1111/1750-3841.15645
Keywords: algal oil, EPA and DHA, fish flesh quality, fish oil replacement, omega-3 fatty acids, sustainable aquaculture
Citation: Marques A, Canada P, Costa C, Basto A, Piloto F, Salgado MA, Abreu H, Dias J and Valente LMP (2023) Replacement of fish oil by alternative n-3 LC-PUFA rich lipid sources in diets for European sea bass (Dicentrarchus labrax). Front. Mar. Sci. 10:1189319. doi: 10.3389/fmars.2023.1189319
Received: 18 March 2023; Accepted: 03 November 2023;
Published: 05 December 2023.
Edited by:
Imtiaz Ahmed, University of Kashmir, IndiaReviewed by:
Giulia Secci, University of Florence, ItalyDébora Machado Fracalossi, Federal University of Santa Catarina, Brazil
Copyright © 2023 Marques, Canada, Costa, Basto, Piloto, Salgado, Abreu, Dias and Valente. This is an open-access article distributed under the terms of the Creative Commons Attribution License (CC BY). The use, distribution or reproduction in other forums is permitted, provided the original author(s) and the copyright owner(s) are credited and that the original publication in this journal is cited, in accordance with accepted academic practice. No use, distribution or reproduction is permitted which does not comply with these terms.
*Correspondence: Luisa M. P. Valente, bHZhbGVudGVAaWNiYXMudXAucHQ=