- 1Institute of Aquaculture, Nha Trang University, Nha Trang, Vietnam
- 2Department of Biological Sciences (BIO), University of Bergen, Bergen, Norway
- 3Institute of Marine Research, Norwegian Institute of Nutrition and Seafood Research, Bergen, Norway
- 4SPAROS (Portugal), Olhão, Portugal
- 5Instituto de Ciencias Marinas de Andalucía (ICMAN-CSIC), Puerto Real, Spain
- 6Centre of Marine Sciences of Algarve (CCMAR), University of Algarve, Faro, Portugal
This study aimed to determine the impact of elevated temperature combined with different levels of dietary methionine concentrations on feed intake (FI) and brain expression of selected neuropeptides and one receptor involved in appetite control in juvenile cobia (approximately 3.7 g body weight). The genes studies were neuropeptide y, npy; agouti-related protein, agrp; cocaine- and amphetamine-regulated transcript, cart; cholecystokinin, cck and melanocortin 4 receptor; mc4r. The cobia were reared at typical sea water temperature in Vietnam (30 °C) and elevated temperature (34°C; selected as one of the predicted scenarios of climate change). The fish were fed diets with different levels of methionine: deficient (M9; 9.1 g/kg), sufficient (M12; 12.8 g/kg) and surplus (M16, 16.8 g/kg) for 6 weeks (triplicate tanks per treatment). Both dietary methionine concentration and temperature affected FI in cobia. Dietary methionine deficiency (i.e., M9) and elevated temperature reduced FI in cobia. Temperature significantly influenced the mRNA expression of agrp, cart, cck and mc4r. Expression of the orexigenic neuropeptide npy was consistently higher before the meal than after the meal for all diets and at both temperatures. At 30°C, prefeeding levels of npy correlated with both increased methionine levels and FI. The interaction between dietary methionine and temperature on the levels of brain npy expression was significant (P<0.05). There was higher brain expression of agrp, cart and cck in cobia at 34°C than in fish at 30°C, which was correlated with a lower FI. In conclusion, both feeding, temperature and/or dietary methionine levels affected the brain expression of npy and agrp, cart, cck and mc4r. This suggests that these neuropeptides as well as the mc4r receptor are actively involved in adjusting feed intake to compensate for changing energetic demands, as well as metabolic adjustments due to the variable availability of methionine at elevated temperature.
1 Introduction
Due to the ectothermic nature of fish, the environmental temperature is a key and main extrinsic factor that directly and significantly affects the rates of all physiological processes and metabolic pathways. Temperature strongly influences voluntary feed intake, feed utilization, and growth performance [see review by (Volkoff and Rønnestad, 2020)]. Based on the sixth assessment report (AR6) of climate change by IPCC (2021), under the worst scenario (SCP5-8.5), the highest increased level of net global temperature will be approximately 4°C by 2100. Such elevated temperatures will negatively affect most fish species (Glencross and Bermudes, 2011; Glencross and Bermudes, 2012; Sandersfeld et al., 2015; Pham et al., 2021), including cobia. Cobia (Rachycentron canadum) is a candidate species in aquaculture due to its high quality flesh and remarkable rapid growth (Chou et al., 2001). However, elevated temperatures reduce growth performance in the species (Sun and Chen, 2014; Nguyen et al., 2019), potentially representing a severe challenge to the large-scale commercial aquaculture of cobia worldwide and in Vietnam. A previous study in this species revealed that elevated temperature affects digestion with changes in the daily pattern of gastrointestinal pH, enzymatic proteolytic digestive activity and resulting increased feed transit time (Yúfera et al., 2019). However, it is still unknown to what extent cobia can adapt to high temperatures and whether dietary modifications may alleviate some of the potentially affected physiological systems controlling appetite and feed intake.
Methionine is an indispensable amino acid (IAA) in mammals and in fish. Besides being a precursor to protein synthesis, this IAA is also involved in several metabolic pathways, including fatty acid oxidation, phospholipid status, creatine synthesis, bile acid production and polyamine availability (Bender, 2012; Andersen et al., 2016; Espe et al., 2016). Supplementation of methionine in the diets influences the epigenetic modification of DNA methylation and gene expression and thus affects the growth and health of animals [reviewed by (Zhang, 2018)]. Methionine is a limiting AA in most plant ingredients, the dominant protein-rich source in aquafeeds, and is increasingly used in carnivorous species (Conceição et al., 2012). In cobia, the requirement of methionine is established at 11.9 g kg-1 dry diet (26.4 g kg-1 dietary protein) in the presence of 6.7 g kg-1 cysteine (Zhou et al., 2006). Supplementation of crystalline DL-methionine in plant protein-based diets results in better growth and feed conversion ratio in cobia (Nguyen et al., 2019) as a consequence of the synchronization of the absorption rate between crystalline DL-methionine and protein-bound methionine (He et al., 2021).
Feed intake in fish, as in other vertebrates, is controlled by neuropeptides released in neural circuits in the brain that also receive inputs from afferent nerves, hormones and metabolic factors released in peripheral tissues (Rønnestad et al., 2016; Volkoff, 2016b; Soengas et al., 2018; Soengas, 2021). Among the appetite-controlling neuropeptides, neuropeptide Y (Npy) and Agouti-related peptide (Agrp) , are considered the most potent orexigenic (feeding stimulatory) factors (Nguyen et al., 2013; Rønnestad et al., 2016; Volkoff, 2016b; Soengas, 2021; Tolås et al., 2021). Intracerebroventricular injections of Npy in goldfish Carassius auratus, trout Oncorhynchus mykiss and channel catfish Ictalurus punctatus resulted in a dose-dependent increase in voluntary feed intake (López-Patiño et al., 1999; de Pedro et al., 2000; Narnaware et al., 2000; Silverstein and Plisetskaya, 2000; Aldegunde and Mancebo, 2006; Matsuda et al., 2012). A lower feed intake is correlated with reduced mRNA expression of agrp (Delgado et al., 2017; Soengas, 2021), whereas fasting leads to increased hypothalamic expression levels of agrp in goldfish (Cerdá-Reverter and Peter, 2003), zebrafish Danio rerio (Song et al., 2003) and Yafish Schizothorax prenanti (Wei et al., 2013), supporting the orexigenic role of Agrp in these species. In cobia, the presence and role of this neuropeptide in the brain is still not determined.
In contrast, cocaine- and amphetamine-regulated transcript, CART (Butler et al., 2017) and cholecystokinin (CCK) are believed to play anorexigenic (feeding inhibitory) roles in feeding control pathways in terrestrial animals and fish (Schwartz et al., 2000; Morton et al., 2014; Rønnestad et al., 2016; Volkoff et al., 2017). Increased expression levels of cart after feeding, suggesting a satiety-inducing effect, have been reported in several fish species, such as medaka Oryzias latipes (Murashita and Kurokawa, 2011), blind cavefish Astyanax mexicanus (Volkoff, 2016a), pacu Piaractus mesopotamicus (Volkoff et al., 2017) and Atlantic salmon Salmo salar (Murashita et al., 2009; Burt et al., 2013; Kousoulaki et al., 2013). CCK produced and secreted in the intestine has a key role in digestion in the vertebrates studied and is also believed to provide a key signal to the brain inducing satiety that terminates a meal. CCK is also produced in the brain, where a reduced feed intake is correlated with increased expression levels (summarized by (Volkoff, 2016b). While CCK previously have been described in cobia (Nguyen et al., 2013), no information is available on the structure and role of CART in cobia.
The melanocortin 4 receptor (MC4R) was characterized and reported to be involved in feeding behavior and the regulation of metabolism, homeostasis and growth in vertebrates, including fish (Volkoff, 2016b; Delgado et al., 2017; Rønnestad et al., 2017; Kalananthan et al., 2020a; Kalananthan et al., 2020b). This receptor is part of the melanocortin system (Anderson et al., 2016). In rainbow trout, Oncorhynchus mykiss (Schjolden et al., 2009), and goldfish (Cerdá-Reverter and Peter, 2003; Schjolden et al., 2009), there was a reduced feed intake after treatment with an Mcr agonist, while Mcr antagonist injection led to an increased feed intake (Cerdá-Reverter and Peter, 2003), supporting the anorexigenic effects of Mc4r in the studied species. However, no information is available on the structure and the role of Mcr4 in cobia.
Previous studies in mammalian species have shown that AAs participate in controlling feed intake via nutrient signaling pathways. This includes the olfactory and gustatory systems that affect feeding behavior and modulate the motivation to search and ingest food. Additionally, sensors in enteroendocrine cells releasing hormones such as CCK and postabsorptive signals such as nutrient sensing mechanisms in the liver, adipose tissue and brain are sensitive to AA levels (reviewed by (Fromentin et al., 2012). Recent research on how specific nutrients affect appetite and nutrient sensing pathways in fish has revealed more details on some of the involved mechanisms. For instance, intracerebroventricular (IC) injection of valine in rainbow trout resulted in increased feed intake, while IC injections of leucine led to reduced levels of npy and agrp (Conde-Sieira and Soengas, 2017; Delgado et al., 2017; Comesaña et al., 2018). Wang et al. (2021) reported that a dietary deficiency of methionine in largemouth bass, Micropterus salmoides, affected AA concentrations in plasma and activated nutrient signaling pathways at both cellular and systemic levels to regulate metabolism and control growth. In cobia, studies (Chi et al., 2020; He et al., 2021) have shown the involvement of methionine in peripheral signaling pathways that affect growth (Chi et al., 2020; He et al., 2021). However, the link between methionine and appetite control was not explored in any of these studies.
The present study aimed to target the role of the selected neuropeptides npy, agrp, cart, cck and receptor mc4r in appetite regulation pathway; and to explore to what extent the selected neuropeptides are related to the modulation of feed intake caused by dietary methionine concentrations (9.1, 12.8 and 16.8 g kg-1, representing deficient, sufficient, and surplus levels of methionine, respectively) and temperatures (elevated 34°C vs. control 30°C). This study also aimed to determine potential interactions, if any between dietary methionine level and temperature on feed intake and brain expression of npy, agrp, cart, cck and mc4r in cobia.
2 Materials and methods
2.1 Experimental diet
Three experimental diets were prepared as described by (Nguyen et al., 2019), all isoenergetic (approximately 20.0 MJ/kg) with approximately 46,5% protein and 10,3% lipid and nutritionally balanced for all indispensable AAs except for methionine. Briefly, crystalline DL-methionine was added at different levels to make final diets that were deficient in methionine M9 (9.1 g kg-1), sufficient in methionine M12 (12.8 g kg-1) and surplus in methionine M16 (16.8 g kg-1) for cobia juveniles, according to (Zhou et al., 2006; NRC, 2011; Nguyen et al., 2019). The dietary AA composition is presented in Table 1S (Supplemental data). All diets were produced at SPAROS Lda. (Olhão, Portugal).
2.2 Fish and experimental design
Cobia juveniles purchased from a local hatchery in Nha Trang, Vietnam, were stocked into two fiberglass tanks (5 m3; 600 individuals per tank), assigned to elevated and control temperature fish groups, and acclimated for 1 week. During this time, fish were fed to satiation twice daily (at 8:00 and 16:00) with pelleted feed (INVE, Ltd. Ho Chi Minh City, Vietnam), and all uneaten feed was collected (Figure 1S). During the experiment, seawater temperature in the hatchery (indoor condition) varied between 27.0°C (nighttime) and 30.5°C (daytime). At the start of the trial, water temperature in the elevated temperature fish group tanks was gradually increased, with a rate of 1°C per day, until it reached 34°C by using thermal controllers (Chuan Kang Ltd., Taiwan). Thereafter the temperature for both fish groups was continuously maintained at 34°C and 30°C for remainder of the trial. Other water parameters were controlled and remained at 29±3.1 ppt salinity, pH of 7.8–8.3, 4.6±0.5 mg L-1 oxygen and < 0.03 mg L-1 total ammonia.
After this initial acclimation period, 180 fish from each temperature group (30 and 34°C) with uniform size (3.7±0.1 and 3.8±0.1 g body weight, respectively) were collected and distributed randomly into the experimental tanks at a density of 20 fish per tank. Eighteen experimental tanks (80×50×60 cm, 200 L) were connected to two recirculation subunits (nine tanks per unit with the same temperature) with continuous aeration. Output water from the rearing tanks of each unit was collected via perpendicular pipes (Ø 27 mm) in the middle of each tank and gathered in a reservoir tank (1.0 x 2.0 x 2.0 m3) before it was pumped into a two-chamber organic filtered fiberglass tank (1.0 x 1.5 x 2.0 m3) (for details see Nguyen et al., 2014). There seawater was passed through a sediment filter, sterilized and finally temperature adjusted before joining the recirculation system subunits. Thermal controllers (Chuan Kang Ltd., Taiwan) maintained the temperature at 30±0.1°C and 34±0.1°C in the two subunits. Other water parameters were maintained as in the acclimation period.
The trial started when fish were introduced to the experimental diet (feeding protocol as in the acclimation period) and lasted six weeks. At each temperature, groups of three tanks (triplicates) were randomly assigned to one of three diets: M9, M12 or M16 (as described in 2.1). Feed intake and growth performance were measured and calculated as described by Nguyen et al. (2019).
2.3 Sampling and sample preparation
Juvenile cobia were weighed individually and measured for length at the beginning and end of the experiment. The fish were anesthetized by MS-222 (Merck, Darmstadt, Germany; 0.4 mg L-1) prior to any handling. To target the role of the selected neuropeptide and receptor genes npy, agrp, cart, cck and mc4r in the appetite controlling pathways and determine the impact of dietary methionine and elevated temperature on expressions, cobia brain samples were collected just before feeding (prefeeding) and after feeding a meal (postfeeding). The prefeeding and postfeeding cobia from the same tank were sampled on different days to prevent the potential impact of handling stress on the meal effects on the expression of appetite genes. Two individuals from each tank were collected at the relevant sampling time.
The prefeeding unfed cobia were collected prior to the morning meal, from 08:00-09:00, while the postfeeding fed fish were collected 30 minutes after the meal was terminated. The fish were sampled by dip net and immediately anesthetized (MS-222; 0.4 mg L-1), and then the whole brains were dissected and transferred into 4 mL cryo-vials prefilled with RNAlater (Thermo Fisher Scientific, MA, USA) on ice. The samples were kept overnight at 4°C in a refrigerator and then stored at −80 ± 3°C until they were transported (on dry ice) to the University of Bergen, Norway, where molecular cloning and quantitative PCR (Q-PCR) were performed.
2.4 Molecular cloning and Q-PCR
2.4.1 RNA isolation
Both npy and cck have previously been cloned in cobia (Nguyen et al., 2013), while the gene transcript sequences for agrp, cart and mc4r for this species are described in this study. We used the whole brain for cloning and establishment of Q-PCR assays for these genes. The tissues were homogenized using FastPrep FP120 (Savant Instruments, Holbrook, NY, USA), and the procedures followed the manufacturer’s protocol. Total RNA was isolated using TRI Reagent (Sigma-Aldrich, MO, USA), and the RNA purity and concentration were checked in a NanoDrop ND-1000 (Thermo Fisher Scientific, MO, USA). The RNA integrity number (RIN) was assessed using the Agilent RNA 6000 Nano Assay protocol (Agilent Technologies).
2.4.2 cDNA synthesis
For each sample, 2 µg and 4 µg of total RNA templates were used to synthesize the first-strand cDNA for cloning and for qPCR, respectively, following the SuperScriptTM III Reverse Transcriptase protocol (Invitrogen, CA, USA) with oligo (dT)20 primers (Sigma-Aldrich, MO, USA). For each run, negative controls (no reverse transcriptase enzyme and no template controls) pooled RNA from all running samples were included.
2.4.3 Molecular cloning
Primers for the cloning of agrp, cart and mc4r were made based on sequence homology searches to similar species. The primers used are shown in Table 1. Nested PCR for cloning agrp was performed using the following thermal program: 98°C for 30 sec; 35 cycles at 98°C for 10 sec, 65°C for 30 sec, 72°C for 60 sec; and 72°C for 2 min using 50 ng of template. To clone agrp, Q5® High-Fidelity DNA Polymerase Enzyme (New England Biolabs, Ipswich, MA, USA) with enhancer was used. To clone mc4r and cart OneTaq® DNA Polymerase enzyme (New England Biolabs, Ipswich, MA, USA) with GC buffer were used.
Nested PCR for cloning of mc4r was performed according to the following thermal program: 94°C for 2 min; 35 cycles at 94°C for 30 sec, 51°C for 60 sec, and 68°C for 30 sec; and 68°C for 5 min using 50 ng of template. Nested PCR for cloning of cart was performed using the thermal program 94°C for 2 min; 45 cycles at 94°C for 30 sec, 50°C for 60 sec, 68°C for 60 sec; followed by 68°C for 5 min using 50 ng of template. TOPO cloning (Invitrogen, Carlsbad, CA, USA) was performed according to the manufacturer’s protocol, and sequencing was performed using the University of Bergen sequencing service. Q-PCR products were sequenced to verify the amplicon.
2.4.4 Quantitative PCR
Relative expression of gene transcripts was determined by Q-PCR using 1X iTaqTM SYBR® Green supermix (Bio-Rad), with 25 ng cDNA template (1:40) in 25 μl reactions following the manufacturer’s protocol using 40 nM specific forward and reverse primers (Table 1). qPCRs were set up in multiple plates using the sample-maximization strategy (Bustin et al., 2010), with triplicates for each sample. Setups included no template and no reverse transcriptase control and between-plate positive controls. The thermal profile was 95°C for 3 min, followed by 40 cycles of 95°C for 30 sec, 58°C for 30 min, and 72°C for 30 sec, with a final denaturation step of 10 sec at 95°C. Among three housekeeping genes, beta-actin (β-actin; (Mohamed et al., 2007), elongation Factor 1alpha (ef1a; Gene Bank1, 2) and ubiquitin protein ligase E3A [ube3a; (Betancur et al., 2013)], tested with cobia brain samples, ef1a was the most stable and thus was used as the reference gene for normalizing gene expression. Gene expression efficiencies for ef1a, npy, agrp, cart, mc4r and cck were calculated as 92.0, 98.1, 101.4, 97.4, 96.7 and 98.7%, respectively, using a dilution series for each assay. Mean normalized expressions (MNE) were calculated in the Microsoft Excel-based Application coded in Visual Basic for Applications, Q-Gene software package (Muller et al., 2002).
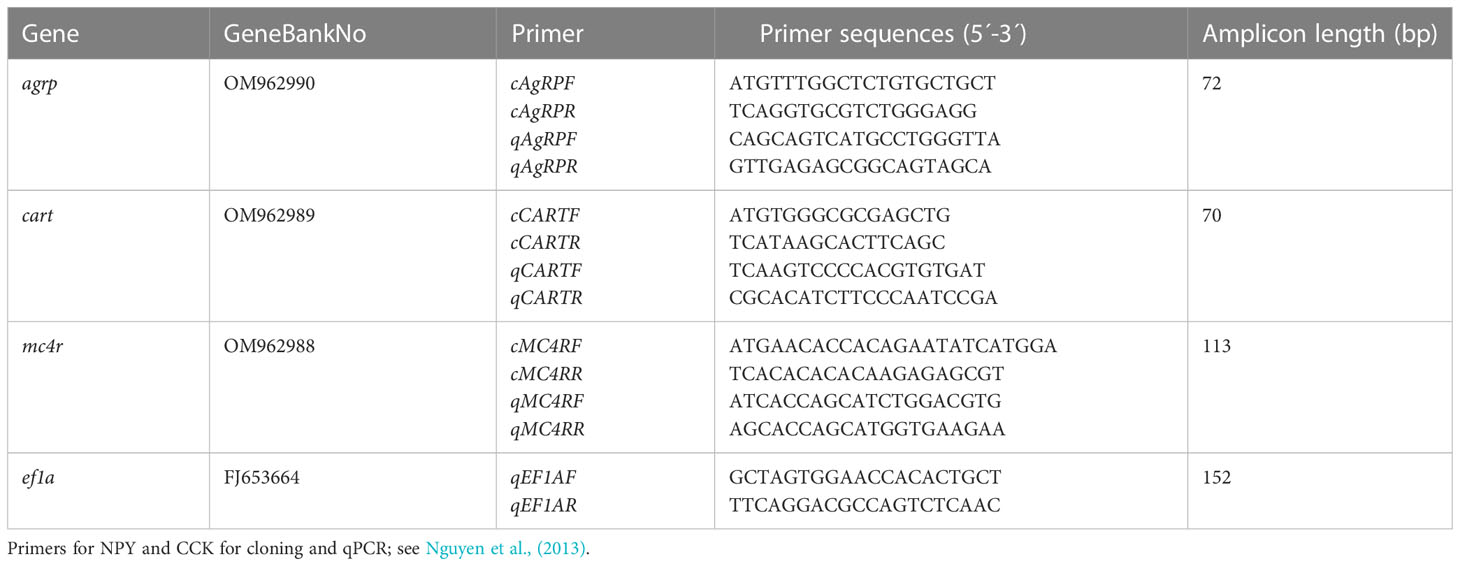
Table 1 Primer sequences for cloning and Q-PCR. Primers for cloning indicated with c, primers for Q-PCR denoted with q. Amplicon length (bp) indicated for q-pcr assays.
2.5 Statistical analyses
Statistical analysis was performed using SPSS for Windows (IBM® SPSS® Statistics version 25). Linear mixed models (in which Tanks is a random factor) were applied to test any differences and/or the interaction between feeding status (prefeeding vs. postfeeding), dietary methionine concentrations and temperature (as fixed factors) on FI, body growth and mRNA expression levels. Pairwise comparisons using Tukey's post hoc test were then applied to evaluate the differences between treatment means. Prior to performing these analyses, all data were checked for normal distribution (Kolmogorov-Smirnov test), homogeneity in variance (Levene’s test) and heteroscedasticity (F test). Data on the mean normalized expression (MNE) of npy were found to be inhomogeneous in variance and heteroscedasticity, and a natural logarithm was applied for MNE (x1000) before applying the tests. Correlation of MNE among genes was performed by applying the Pearson test. Graphs were generated using the ggplot2 package version 3.3.5 (Wickham, 2016) in R 3.1.3 and Rstudio 1.1.463.
2.6 Multiple alignments, structural analysis, and phylogeny
Predicted protein sequences for cobia Agrp, Cart and Mc4r were aligned using Genious software. Signal peptide cleavage analysis for Agrp was performed using SignalP Ver. 5.03, 4 program. Putative signal peptides were predicted using PrediSi5, 6, and proteolytic cleavage sites were predicted using Neuropred7, 8. Transmembrane regions, including extracellular and intracellular loops, as well as identifying cysteines involved in disulfide bonds, were retrieved by blasting cobia Mc4r in UniProt9, 10 and performing transmembrane topology prediction using the deep TMHMM11 sequence predictor transmembrane domains.
All peptide sequences were aligned using MUSCLE with the default parameters (UPGMA clustering method, Gap opening penalty −2.90, Gap extension 0.0) from MEGAX (Hall, 2013). Phylogenetic analysis was performed using the predicted mature peptide sequences. Based on best-fit substitution model analysis in MEGAX, a phylogenetic tree was constructed using maximum likelihood (Huggett et al., 2005) with a Jones-Taylor-Thornton (JTT) model (Jones et al., 1992; Kumar et al., 2018) with a fixed gamma distribution (+G) parameter with five rate categories and 500 bootstrap replicates. The trees were rooted to the human, Homo sapiens, sequences. Cobia Cart were aligned to all known paralogs and rooted to the human, elephant shark, Callorhinchus milii, coelacanth, Latimeria chalumnae, and Cart sequences before being subjected to phylogenetic analysis using only Cart2 transcript variants. In the initial analysis, we included fish species as old teleost Mexican tetra, Astyanax mexicanus, Atlantic herring, Clupea harengus and salmonids, rainbow trout and Atlantic salmon (that went through a 4 round of whole genome duplication; 4R WGD), fish species before the whole teleost specific genome duplication event (Ts WGD) (spotted gar, Lepisosteus oculatus, and elephant shark), and species that diverged before 4R WGD (northern pike, Esox lucius) and those that went to the very recent 4R WGD (goldfish) and zebrafish which did not go to 4R WGD (Ravi and Venkatesh, 2018).
3 Results
3.1 Feed intake and growth
The results on feed intake and growth in cobia were reported and discussed by (Nguyen et al., 2019) and are only summarized here (Figure 1). Generally, cobia were very active during feeding in all groups, and initiated feeding as soon as the first pellet was dropped into the tank. The fish were apparently satiated and typically stopped ingesting pellets after 5-7 min of feeding. The levels of feed intake at M12 and M16, calculated over the six-week duration of the trial, did not differ, while growth was better at M12. Both Groups M12 and M16 had a higher feed intake and better growth compared to the M9 fish. Higher feed intake at 30°C resulted in higher growth in this treatment compared to the fish reared at 34°C. While interaction effects between dietary levels of methionine and temperature were present in growth, these two factors acted independently on feed intake.
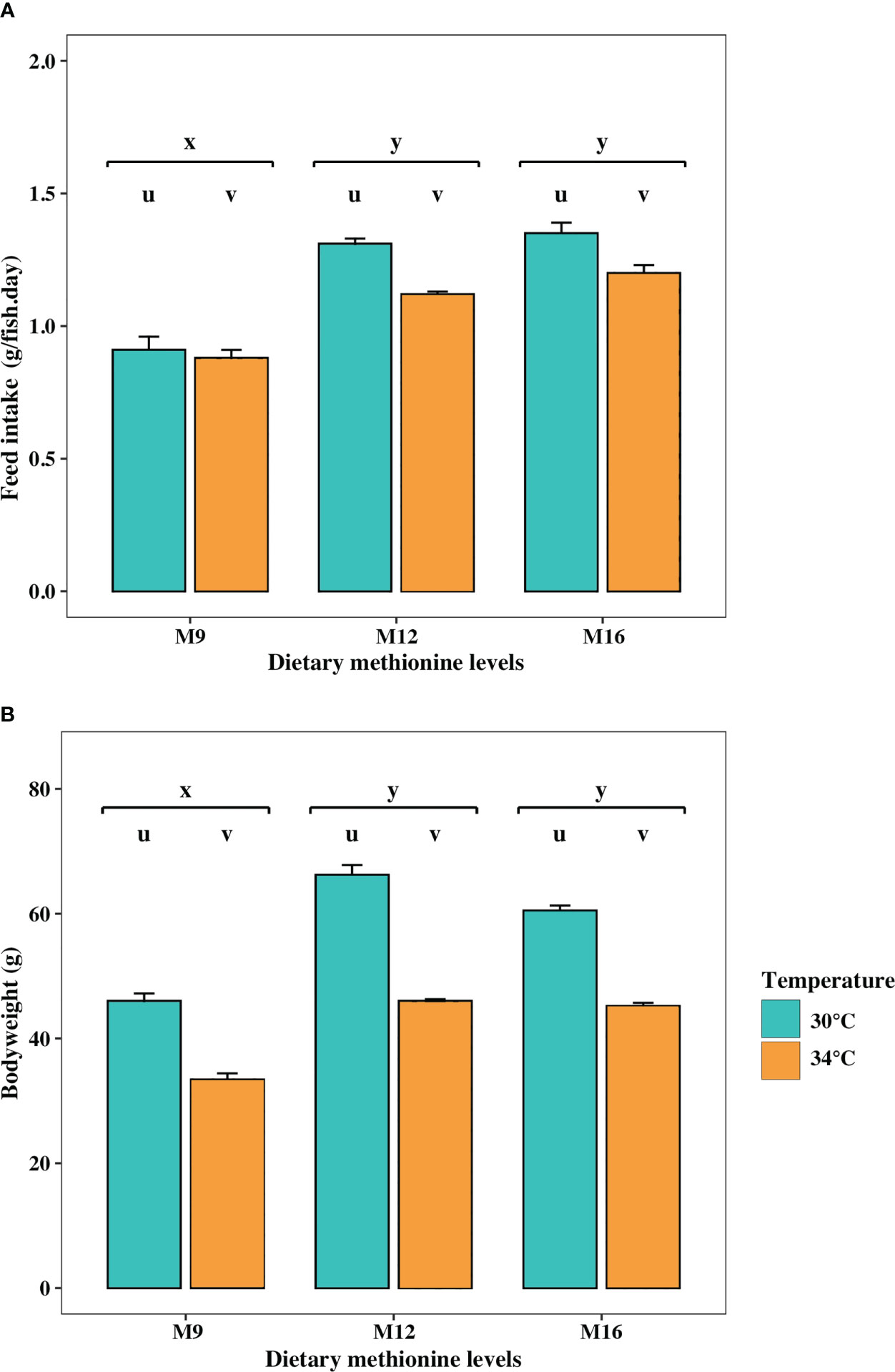
Figure 1 (A) Feed intake (FI) and (B) final body weight in cobia fed different methionine diets [9.1 gkg-1 (M9, deficient), 12.8 gkg-1 (M12, sufficient) and 16.8 gkg-1 (M16, surplus)] and at two temperatures (30°C and 34°C). Bars present mean with standard errors, using tanks as the statistical unit (N=3). Different letters on top of boxes indicate significant difference (p<0.05) between temperatures (u, v) and among methionine levels (x, y).
3.2 Molecular cloning and phylogeny
The cobia npy and cck nucleotide sequences were reported in our previous study [GenBank accession no, KC284716 and KC284715 (Nguyen et al., 2013)]. For the cobia agrp, cart and mc4r, we obtained the ORF sequences from the PCR: Agrp (GenBank accession no OM962990), Cart (GenBank accession no OM962989) and Mc4r (GenBank accession no OM962988).
The cobia Agrp (OM962990) is 426 bp in length and encodes sequences of 141 AAs (Figure 2S). The molecular characterization of cobia Agrp is shown in Figure 2S (alignment of AA sequences) and Figure 2 (phylogenetic analysis of mature peptide sequence). The signal peptide cleavage site is between pos. 20 and 21: SSS-LV (P:0.2927) (Figure 2S). The cobia Agrp sequence clusters well within the teleost Agrp1 clade and differs significantly from Agrp2 identified in teleosts. The cobia Agrp showed 50.7% amino acid identity to human AGRP (Figure 2).
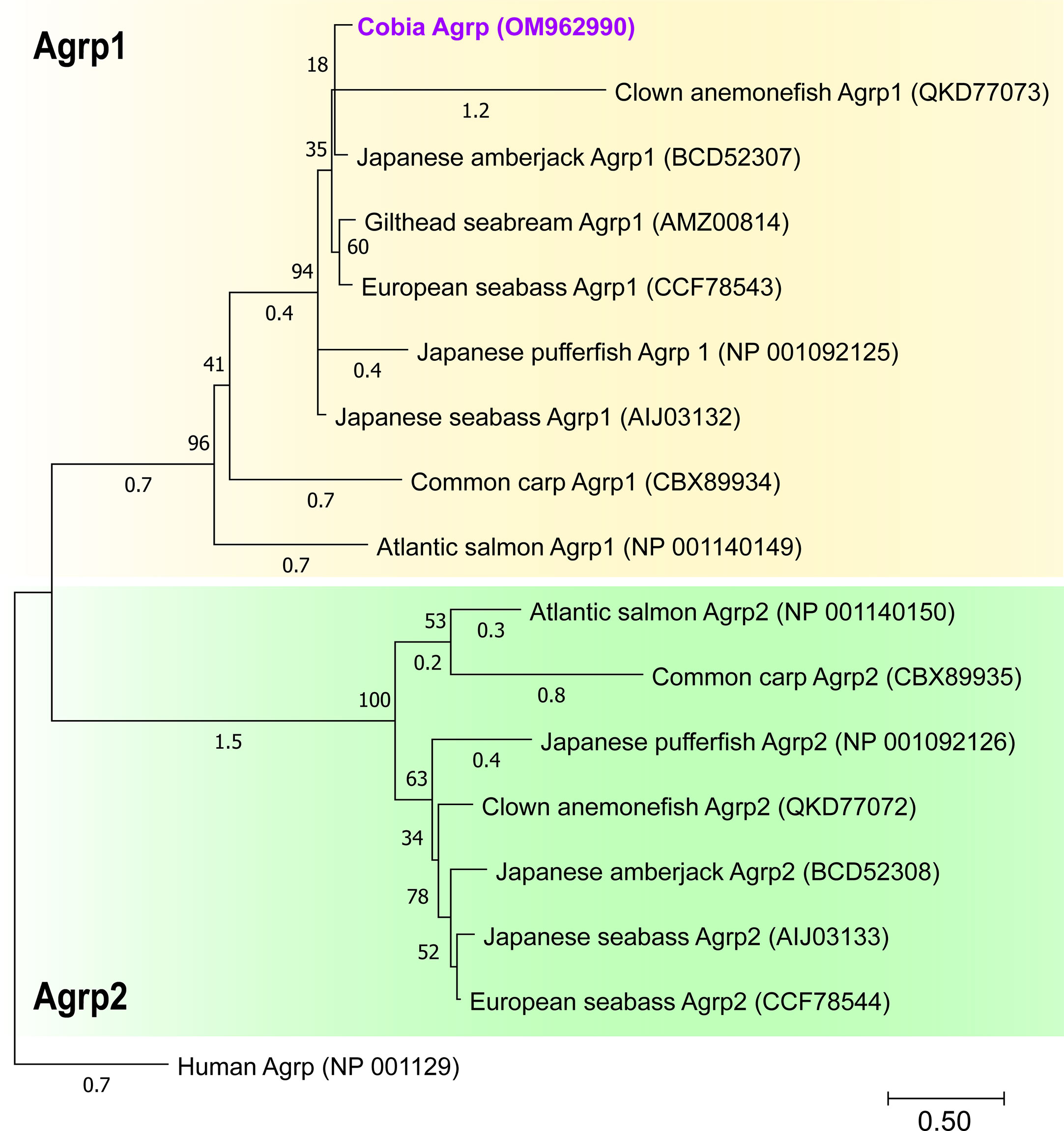
Figure 2 Phylogenetic analysis of the predicted cobia Agrp mature peptide sequence. Alignments were made using the submitted GenBank protein sequences in MEGA-X software. All peptide sequences were aligned using MUSCLE with the default parameters. The phylogenetic tree was constructed using Maximum Likelihood (ML) with a Jones-Taylor-Thornton (JTT) model with fixed Gamma distribution (+G) parameter with five rate categories and 500 bootstrap replicates. The tree was rooted to the human Agrp sequence. GenBank Accession Nos are as follows: cobia (Agrp, OM962990), Clown anemonefish (Agrp1, QKD77073), Japanese amberjack (Agrp1, BCD52307), Gilthead seabream (Agrp1, AMZ00814) European seabass (Agrp1, CCF78543), Japanese pufferfish (Agrp1, NP_001092125), Japanese seabass (Agrp1, AIJ03132), common carp (Agrp1, CBX89934), Atlantic salmon (Agrp1, NP_001140149), Atlantic salmon (Agrp2, NP_001140150), common carp (Agrp2, CBX89935), Japanese pufferfish (Agrp2, NP_001092126), Japanese seabass (Agrp2, AIJ03133), clown anemonefish (Agrp2, QKD77072), Japanese amberjack (Agrp2, BCD52308) and European seabass (Agrp2, CCF78544) and outgroup Human (Agrp, NP_001129).
The cobia Cart paralog identified in this study (OM962989) is 312 bp in length and encodes a sequence of 103 AAs (Figure 3S). The molecular characterization based on AA alignment of cobia Cart clusters within the Cart2a clade (Figure 3). Cobia Cart2a contains an 18-amino acid residue putative signal peptide12. The deduced cobia Cart2a protein has six cysteine residues for a putative disulfide bond that is conserved in Cart proteins, and the processing signals (KR and KK) are conserved in cobia Cart (Figure 3S). Cobia Cart2a structural analysis indicated a cleavage site between positions 23 and 24 of TEA-ER13 (results not shown). Cobia Cart2a showed 65.3% amino acid identity to human CARTs (Figure 3).
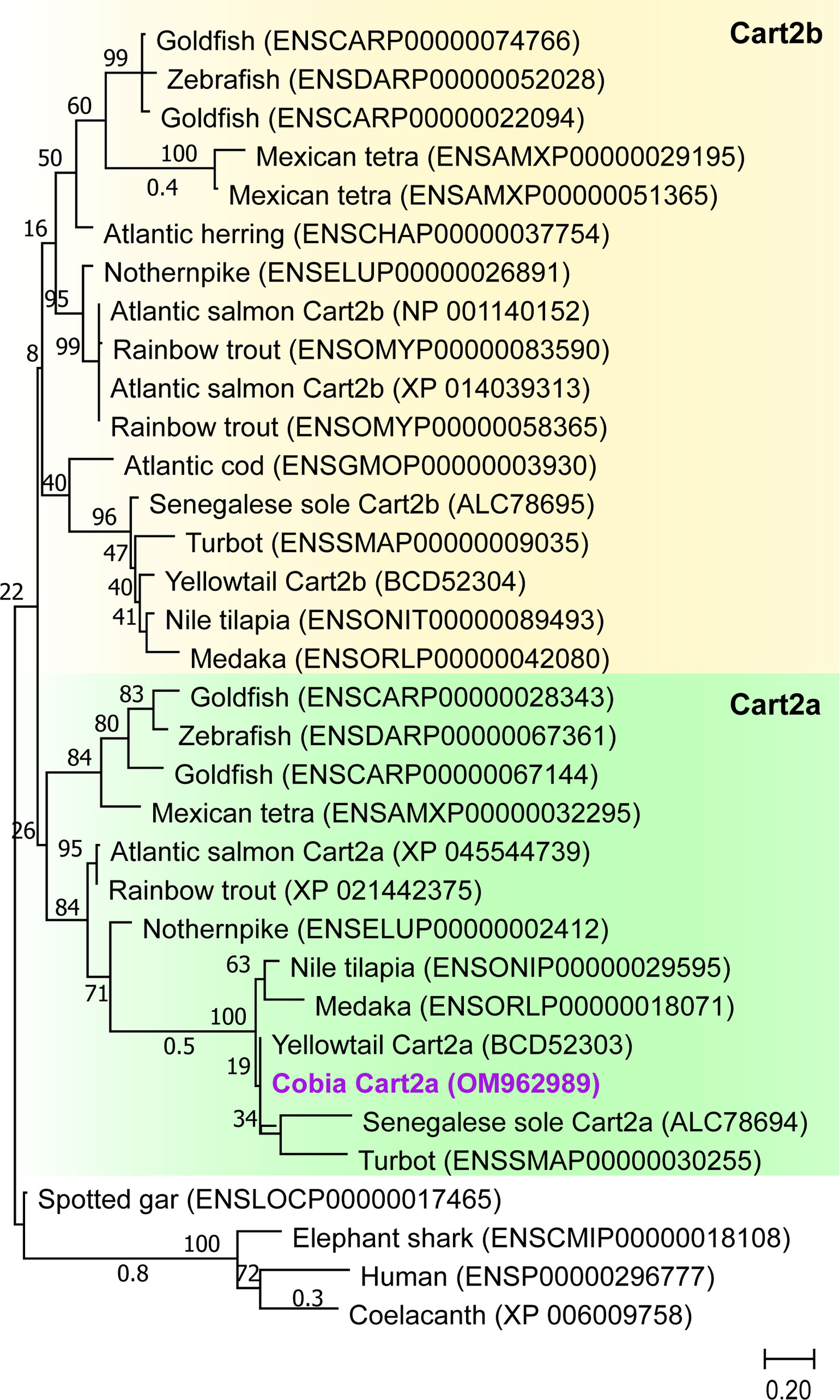
Figure 3 Phylogenetic analysis of the predicted mature peptide sequence of cobia Cart. Alignments were made using the submitted GenBank peptide sequences in MEGA-X software. All peptide sequences were aligned using MUSCLE with the default parameters. The phylogenetic tree was constructed using Maximum Likelihood (ML) with a Jones-Taylor-Thornton (JTT) model with fixed Gamma distribution (+G) parameter with five rate categories and 500 bootstrap replicates. The tree was rooted to the human, elephant shark and coelacanth Cart sequences. GenBank Accession Nos and ENSEMBL are as follows: Cobia (Cart2a, OM962989), Atlantic salmon (Cart2a, XP_045544739) Atlantic salmon (Cart2b, NP_001140152 and XP_014039313), Yellowtail (Cart2a, BCD52303), Yellowtail (Cart2b, BCD52304), human (Cart, ENSP00000296777), Coelacanth_(Cart, XP_006009758), Spotted gar_(Cart, ENSLOCP00000017465), Goldfish (Cart2, ENSCARP00000074766, ENSCARP00000067144, ENSCARP00000028343 and ENSCARP00000022094), zebrafish (Cart2, ENSDARP00000052028 and ENSDARP00000067361), Nothernpike (Cart2, ENSELUP00000002412 and ENSELUP00000026891), Rainbow_trout_(Cart2, ENSOMYP00000058365, ENSOMYP00000083590 and XP_021442375), Atlantic_herring (Cart, _ENSCHAP00000037754), Atlantic_cod (Cart, _ENSGMOP00000003930), Nile_tilapia_(Cart2, ENSONIT00000089493 and ENSONIP00000029595) and Senegalese_sole_(Cart2a, ALC78694), Senegalese_sole_(Cart2b, ALC78695), Turbot (Cart2, ENSSMAP00000030255 and ENSSMAP00000009035), Medaka (Cart2, ENSORLP00000042080 and ENSORLP00000018071), Mexican tetra (Cart2, ENSAMXP00000029195, ENSAMXP00000051365 and ENSAMXP00000032295) and Elephant_shark (Cart, ENSCMIP00000018108).
The cobia Mc4r (OM962988) is 975 bp in length and encodes sequences of 324 AAs (Figure 4S). The molecular characterization of cobia Mc4r is provided in Figure 4S (AA alignment) and Figure 4 as a phylogenetic analysis. By blasting the cobia Mc4r sequence in UniProt and the deep TMHMM sequence predictor14, the transmembrane domains and extracellular and intracellular loops for cobia Mc4r were identified. The cysteines involved in the disulfide bonds in human MC4R are conserved in cobia Mc4r (results not shown). The cobia Mc4r sequence clusters well within the teleost Mc4r clade (Figure 4). The cobia Mc4r showed 68.1% amino acid identity to human Mc4R (Figures 4, 4S).
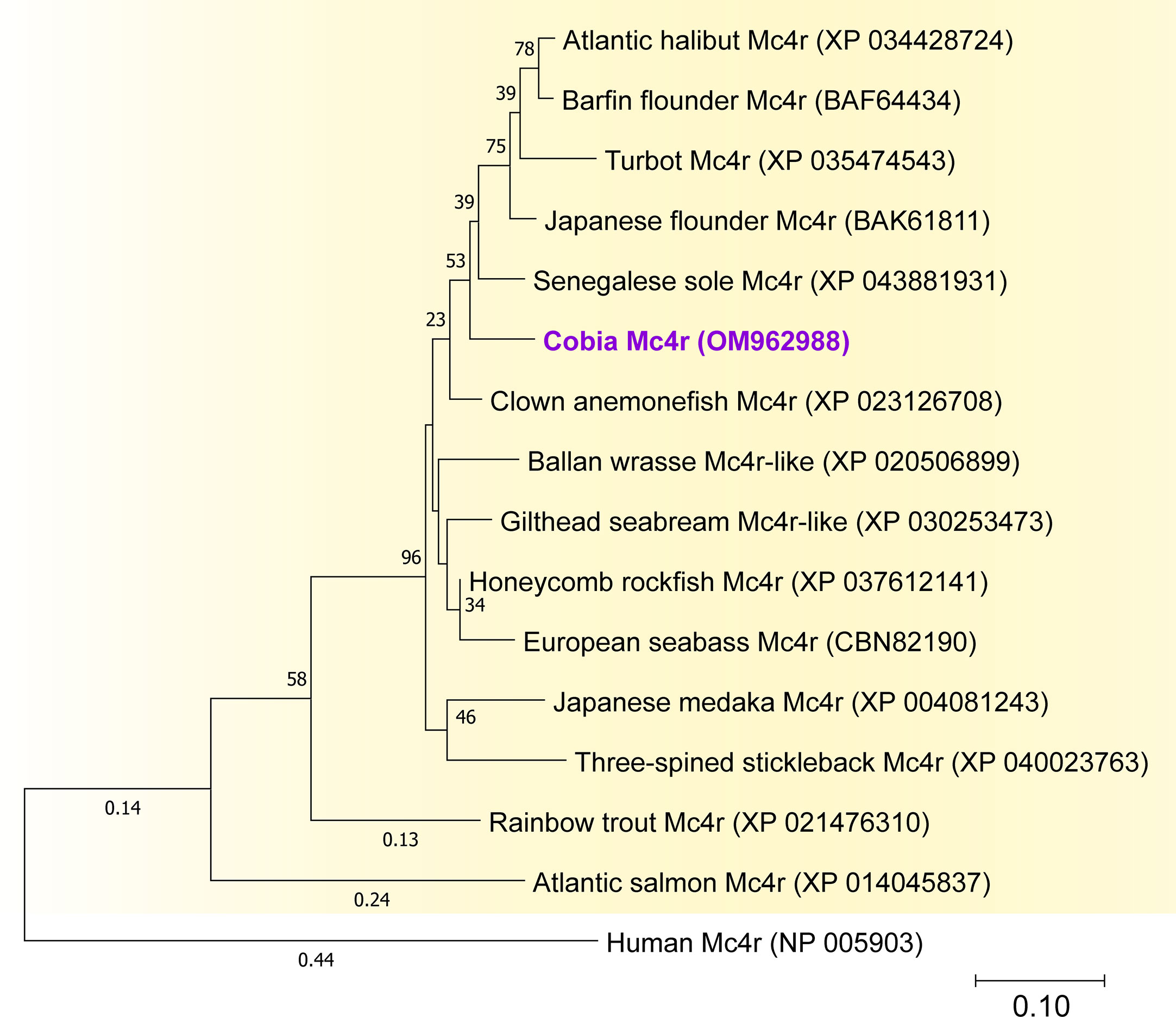
Figure 4 Phylogenetic analysis of the mature peptide sequence of cobia Mc4r. Alignments were made using the submitted GenBank amino acid sequence in MEGA-X software. All peptide sequences were aligned using MUSCLE with the default parameters. The phylogenetic three was constructed using Maximum Likelihood (ML) with a Jones-Taylor-Thornton (JTT) model with fixed Gamma distribution (+G) parameter with five rate categories and 500 bootstrap replicates. The tree was rooted to the human Mc4r sequence. GenBank Accession Nos are as follows: Japanese medaka (Mc4r, XP_004081243), Cobia (Mc4r, OM962988), Three-spined stickleback (Mc4r, XP_040023763), Senegalese sole (Mc4r, XP_043881931), Japanese flounder (Mc4r, BAK61811), Gilthead seabream (Mc4r-like, XP_030253473), Atlantic halibut (Mc4r, XP_034428724), Clown anemonefish (Mc4r, XP_023126708), Honeycomb rockfish (Mc4r, XP_037612141), Barfin flounder (Mc4r, BAF64434), Ballan wrasse (Mc4r-like, XP_020506899), Turbot (Mc4r, XP_035474543), European seabass (Mc4r, CBN82190), Rainbow trout (Mc4r, XP_021476310), Atlantic salmon (Mc4r, XP_014045837) and outgroup Human (Mc4r, NP_005903).
3.3 Expression of brain neuropeptides
3.3.1 Quality control of results in Q-PCR analysis
The mean RIN value of the extracted RNA brain samples was 8.8 (SD=0.6). All samples had RIN values above eight and could therefore be used for downstream applications (Fleige and Pfaffl, 2006). To evaluate the potential for a minor degradation of RNA in the brain samples during sampling or storage, regression analysis was performed, indicating a weak correlation between RIN values and Cq values for efa1, npy, agrp, cart and cck (results not shown). This indicates that minor degradation did not affect the gene expression results.
3.3.2 npy expression- effects of temperature, methionine levels and a meal
Temperature did not significantly affect the expression of npy (P>0.005). However, dietary methionine levels affected the cobia’s brain expression of npy. Cobia fed the M16 diet showed a higher level of npy than those fish fed the M9 diet; while cobia fed the M12 diet showed comparable brain expression of npy to the fish fed the other diets. In addition, levels of brain npy in the cobia analyzed around the first meal in the morning were higher before (pre) feeding than after (post-) feeding in all experimental treatments (P = 0.001, Figure 5A).
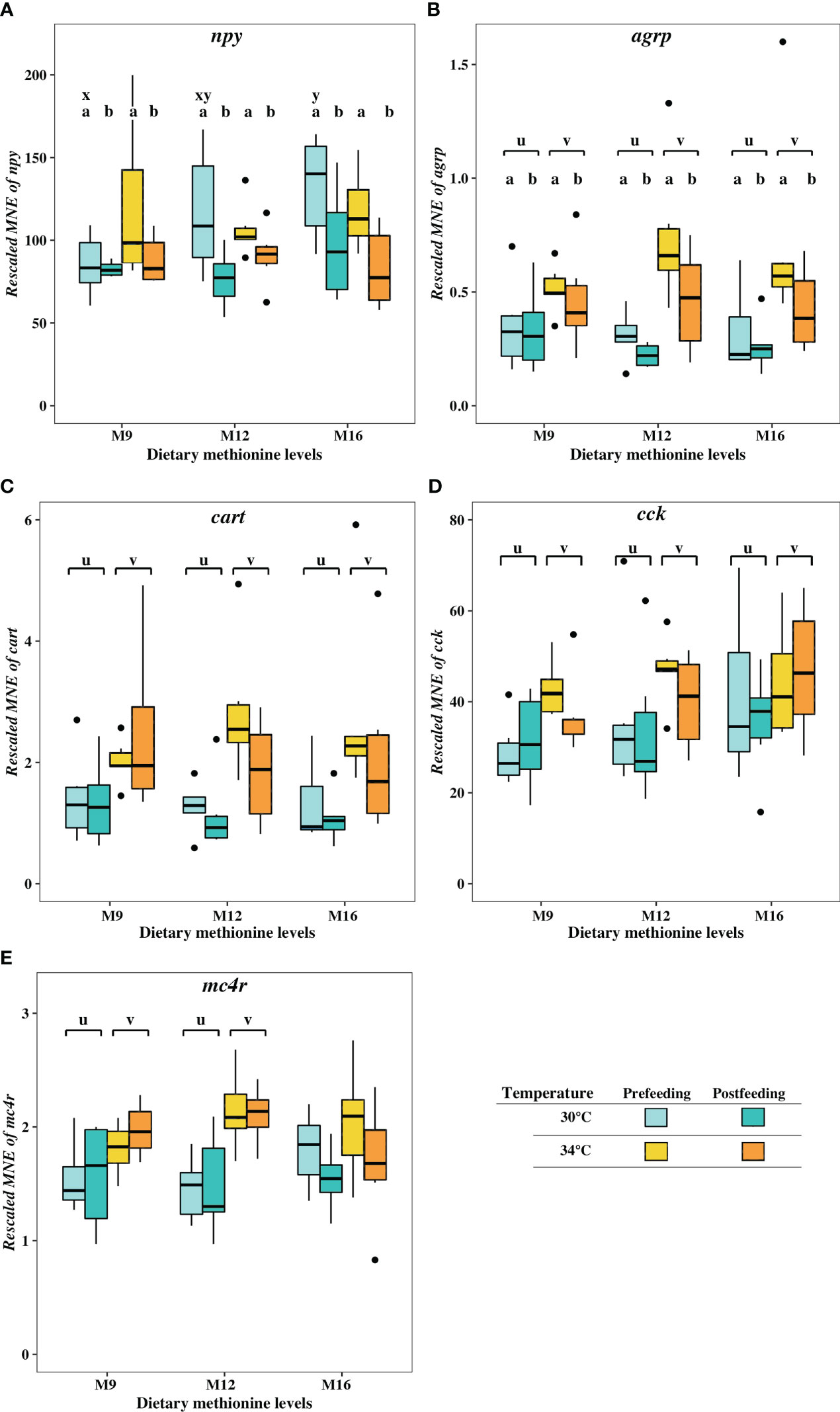
Figure 5 Relative mRNA expression of neuropeptides (A) npy, (B) agrp, (C) cart, (D) cck and (E) the melanocortin receptor mc4r in the brain of cobia fed three dietary methionine levels (9.1 gkg-1 (M9), 12.8 gkg-1 (M12) and 16.8 gkg-1 (M16)) (x-axis) at two temperatures (30°C and 34°C and; before (Pre-feeding) and after (Post-feeding) a meal. Values shown are for triplicate tanks with six fish per group (n = 3). Y-axis shows rescaled mean normalized expression (MNEx103). Data is shown as boxplots with median (black line within the box), 25th and 75th percentiles (boundaries closest and farthest to zero) and 10th and 90th percentiles (whiskers above and below the box). Points outside the 10th and 90th percentiles are outliers. Different letters on top of boxes indicate the significant difference (p<0.05) between temperatures (u, v), two feeding states (a, b) and among methionine levels (x, y).
There was an interaction between dietary methionine and temperature on npy expression of cobia’s brain (P=0.014) that led to higher level of npy in cobia fed M16 diet than those fish fed the M9 diet at control temperature (30°C), but not at elevated temperature (34°C).
3.3.3 agrp expression- effects of temperature, methionine levels and a meal
Expression of agrp in the brain of cobia was affected by both temperature (P < 0.001) and intake of a meal (pre vs. post feeding of the first meal in the morning) (P = 0.009), but not methionine levels (Figure 5B). agrp mRNA levels were higher in the fish reared at elevated temperature (34°C) than the ones reared at control temperature (30°C). Also, the prefeeding cobia showed higher agrp than the postfeeding fish. There were no significant combined effects among feeding, dietary methionine, and rearing temperature on the expression levels of this gene.
3.3.4 cart, cck and mc4r expression- effects of temperature, methionine levels and a meal
Temperature affected expression of these neuropeptides and the receptor. The elevated temperature group had higher expression levels of cart (P< 0.001), cck (P=0.002) and mc4r (P< 0.0001) in the cobia brain (Figures 5C–E). There were no significant differences in brain expressions of cart, cck and mc4r amongst the cobia fed different dietary methionine levels, nor between the prefeeding and postfeeding fish (P>0.05).
3.3.5 Correlation analysis of gene expression
The correlation analysis of gene expression (Figure 5S) showed values ranging from -0.02 (cart and npy) to 0.83 (agrp and cart).
In summary, dietary methionine affected the brain expression levels of npy, while elevated temperature caused significantly upregulated expression of agrp, cart, cck and mc4r. Expression levels of both npy and agrp were higher in cobia before the meal (prefeeding) compared to after the meal had been terminated (postfeeding). There was a combined effect of dietary methionine and rearing temperature on the expression levels of npy.
4 Discussion
4.1 Effects of dietary methionine levels and temperatures on feed intake and growth performance
In the present study, cobia fed the M9 diet had the lowest feed intake, indicating that a dietary deficiency of methionine reduced appetite in the fish (Figure 1A). As expected, the fish that was fed diet deficient in methionine (M9 diet) also grew less (Figure 1B) and had a poorer FCR, compared to those fish fed either the M12 or the M16 diets. The dietary deficiency of one indispensable AA will lead to an increased oxidation of other indispensable and dispensable AAs present in the diets, thereby reducing growth in the cobia [see a more detailed discussion in (Nguyen et al., 2019)]. These results are in line with previous studies on cobia related to methionine availability, growth and feed utilization (Zhou et al., 2006; Van Nguyen et al., 2014; Wang et al., 2016; Chi et al., 2020), as well as in other fish species (Walton et al., 1982; Murthy and Varghese, 1998; Elmada et al., 2016; Gao et al., 2019; Wang et al., 2021). The finding that dietary deficiency of methionine is also involved in the loss of appetite and reduction of FI, resulting in reduced growth and feed efficiency in cobia, has also been observed in European sea bass (Thebault et al., 1985), grouper Epinephelus coioides (Luo et al., 2005), turbot Scophthalmus maximus (Peres and Oliva-Teles, 2005), gilthead sea bream Sparus aurata (Sánchez-Lozano et al., 2011), large yellow croaker Pseudosciaena crocea (Mai et al., 2006; Li et al., 2021) and golden pompano, Trachinotus ovatus (Niu et al., 2013). Although, feed intake in cobia fed M12 and M16 diets did not differ, growth was better in the fish fed the M12 diet (Nguyen et al., 2019).
The elevated temperature had a negative effect on fish performance. Both feed intake and growth were lower in cobia juveniles reared at 34°C than at 30°C (Figure 1). With increasing temperature, the rates for all metabolic and physiological processes in an ectothermic fish will increase up to a certain level (Hevrøy et al., 2011; Volkoff and Rønnestad, 2020). Performance is maximal in an optimal temperature range but will then start to decline and proceed toward zero at the upper critical temperature (Volkoff and Rønnestad, 2020). The data in this study indicate that 34°C is beyond the optimum temperature for cobia but still below the critical thermal maximum (Sun and Chen, 2014). Sun and Chen (2014) reported that cobia shows the maximal growth rate at 31°C, which shows that this species can effectively ingest and allocate sufficient energy to growth at this temperature.
The growth result from the current study suggests that there were higher costs for routine metabolism, and combined with a lower feed intake, a smaller fraction of the energy budget could be allocated to growth in cobia kept at 34°C compared to the fish at 30°C. This result compares well with that reported for other fish species exposed to temperatures above the optimum range (Glencross and Bermudes, 2011; Glencross and Bermudes, 2012; Sandersfeld et al., 2015; Pham et al., 2022). Although fish will reduce their feed intake when the temperature increases over their optimal range, there is little information on the underlying signaling pathways and the involvement of central appetite-controlling neuropeptides.
4.2 npy and agrp expression- effects of temperature, methionine levels and a meal
Feeding resulted in a decline in the expression levels of both brain npy and agrp in the cobia after the meal at both temperatures and for all three diets (Figures 5A, B). This supports the notion that Npy and Agrp peptides are involved in the control of appetite and feeding and that both act as orexigenic factors in fish (Volkoff, 2016b; Delgado et al., 2017; Rønnestad et al., 2017), including cobia (Nguyen et al., 2013). The described time course fits an orexigenic peptide that stimulates feeding behavior and intake of feed with a higher activity before than after the meal, when the fish is satiated. The present study thus supports other notions that orexigenic activity is also reflected in the mRNA expression of specific peptides.
Previous studies have suggested that Npy is the most potent orexigenic player involved in central control of appetite and feed intake of fish. Several studies have reported that the expression levels of npy decline after a meal; Atlantic cod, Gadus morhua (Kehoe and Volkoff, 2007), zebrafish (Tian et al., 2015), and grass carp, Ctenopharyngodon idella (Zhou et al., 2013). In goldfish, brain npy mRNA levels increase before and are reduced after a meal, again supporting this notion (Narnaware et al., 2000). Feed deprivation and fasting also result in elevated levels of npy. This suggests, as expected, that hunger signals increase in fasted fish, including coho salmon (Oncorhynchus kisutch), chinook salmon (Oncorhynchus tshawytscha), goldfish, and winter skate (Leucoraja ocellata) (Silverstein et al., 1998; Narnaware and Peter, 2001; MacDonald and Volkoff, 2009). Following the same argument, refeeding goldfish resulted in a reduction in npy to normal levels, which also supports an orexigenic role of Npy (Narnaware and Peter, 2001).
An orexigenic effect of Npy is also reflected in the fact that cobia fed the M9 diet had a lower npy expression level and a lower feed intake, and therefore also a lower growth rate compared to the fish fed either the M12 or M16 diets. Thus, the imbalanced dietary AA profile did not induce a compensatory orexigenic drive resulting in an increased feed intake. In contrast, our data show high expression levels of npy before the onset of feeding in the M12 and M16 groups (Figure 5A). It should be noted that the prefeeding expression levels were analyzed 30 min before the meal was administered. The underlying mechanisms for having an orexigenic drive or a sense of hunger before the meal are partly expected to be due to intrinsic signals from reduced filling of the gastrointestinal tract and directly or indirectly from the nutrient and energy status of the body. However, given that the fish were fed according to a fixed feeding schedule, this could also involve a conditioned anticipatory response, with the fish expecting a meal at this time. Such conditional anticipation, assessed as altered behavior when food is offered on a periodic basis, has been reported for several species, including Atlantic salmon (Fernö et al., 1995; Folkedal et al., 2012).
The mechanism for why insufficient dietary methionine led to a lower orexigenic action compared to sufficient and surplus methionine may involve a range of nutrient sensing pathways; either methionine acts as an attractant and increasing palatability in cobia or other intrinsic signaling pathways, possibly directly in the brain or in the liver acting via secondary endocrines, which both may induce an orexigenic action in npy expression. The underlying mechanism of this action in the brain may involve methionine sensing pathways that affect mTOR (target of rapamycin), ERK [extracellular signal-regulated kinase, and mitochondrial metabolism of AAs, which results in the activation of ROS (reactive oxygen species) and elevated levels of ATP/ADP from the mitochondria of the cell, as proposed for branched-chain AA by (Conde-Sieira and Soengas, 2017)]. In mammals, it has been shown that hepatic FGF21 is the key mediator of the physiological and behavioral effects related to metabolism and energy balance caused by methionine-restricted diets (Forney et al., 2018).
Interestingly, Forney et al. (2017) showed that restriction of dietary methionine induced hyperphagia in mice. They proposed that a methionine-sensing system is linked to a compensatory hyperphagic mechanism, but only when there is sufficient methionine in the diet to prevent weight loss through a compensatory increase in feed intake, thus pointing to a concentration-dependent and essential AA-specific effect of the AA composition of the diet. Further studies are required to clarify to what extent similar links between the sensing of specific AAs and appetite control are found in cobia as well as in other ectotherms.
With regard to temperature effects, it is also interesting to note that the npy behavior related to the intake of a meal differed between the two temperatures. The correlation between npy expression and the methionine level was not observed at the elevated temperature. This could be due to a possible stress response caused by temperatures beyond the optimum. A link between NPY and stress has been reported for mammals (Reichmann and Holzer, 2016), but whether there is a relationship between Npy and stress in cobia remains to be explored. On the other hand, our data suggest that the appetite signaling pathways via Npy action in cobia are impacted mainly by the presence of food (prefeeding vs. postfeeding) and food quality (methionine deficiency) rather than environmental temperature.
Agrp has also been reported to be involved in the regulation of energy homeostasis and stimulation of food intake in teleosts (Sohn, 2015; Takeuchi, 2016). Fasting leads to increased expression levels of agrp in the hypothalamus of goldfish (Cerdá-Reverter and Peter, 2003), zebrafish (Song et al., 2003), and Ya fish Schizothorax prenanti (Wei et al., 2013), indicating an orexigenic role of this neuropeptide in appetite control in fish (Volkoff, 2016a). To date, we have only identified one paralog of agrp in the cobia brain (Figures 2, 2S), compared to two reported paralogs of agrp (agrp 1 & 2) that play different roles in controlling appetite in mammals and some fish species (e.g., clownfish (Amphiprion ocellaris), (Pham et al., 2021), Atlantic salmon (Murashita et al., 2009; Murashita and Kurokawa, 2011; Kalananthan et al., 2020b; Lai et al., 2021) and zebrafish (Shainer et al., 2017)). Our analysis shows that the presently reported agrp in cobia aligns well with other teleost agrp1 paralogs (Figure 2). Additionally, our results on cobia agrp are in line with those reports for agrp in Arctic charr, Salvelinus alpinus (Striberny et al., 2015), and for agrp1 in transgenic coho salmon, (Kim et al., 2015) where agrp levels decline after feeding. act as an orexigenic neuropeptide. In contrast, the other agrp paralog, agrp2, showed an increased expression level after feeding in clownfish (Pham et al., 2021). In Atlantic salmon, the two agrp paralogs have different distributions in the brain and respond differently to feeding status. (Kalananthan et al., 2020b) showed that agrp1 had the highest expression in the hypothalamus and was affected by feeding status (fasting), while agrp2 had higher expression levels in other brain regions (i.e., in the saccus vasculosus) and did not respond to fasting. The reported agrp2 is also known as asip2 and may therefore serve different roles than appetite control, i.e., in zebrafish, it has been described to provide negative regulation of cortisol secretion and pigmentation15.
In the current study, dietary methionine did not affect the brain expression of agrp in cobia, indicating that this neuropeptide did not respond to any nutrient-sensing mechanisms for methionine. In addition, elevated temperature resulted in higher expression levels of agrp, even though the FI in this treatment was lower than that in the control. These results may indicate that agrp responds to signals related to long-term energy status and demands rather than appetite in response to elevated temperature.
The upregulation of agrp at the mRNA level in cobia at prefeeding and at elevated temperature suggests that this neuropeptide not only acts as an orexigenic factor but is also involved in the regulation of energy homeostasis in fish, as reported in mammals (see (Sohn, 2015) for a review). To cope with elevated temperature (an unfavorable thermal condition), a higher abundance of agrp could be expected in cobia for the fish to control its body homeostasis as an adaptive strategy. Thus, upregulation of the agrp gene may partly contribute to maintaining appetite to compensate for the higher metabolic cost due to elevated temperature. However, for cobia kept at 34°C, any potential compensatory mechanisms to increase FI to keep up with the metabolic demands were inhibited by anorexigenic mechanisms, resulting in a lower FI (Figure 1). In zebrafish, acute stress induced a rapid increase in total body cortisol and increased central expression levels of npy and agrp as a coordinated response to counteract the inhibitory effects of stress on feed intake (Cortes et al., 2018). In the present study, fish were acclimated to elevated temperatures for a long time, and any stress-related response to the increased temperature was not acute but long-term. In addition, we only observed increased expression levels of agrp and not npy. It is thus possible that in cobia kept at elevated and unfavorable temperatures, two orexigenic neuropeptides respond differently based on their individual involvement in signaling pathways that include both stress and energy homeostasis. More studies are encouraged to explore and elucidate such mechanisms.
In short, the results from the present study indicate that npy and agrp act as orexigenic neuropeptides in pathways of appetite and feeding control in cobia, but with different roles and final effects on the feed intake and impacts of elevated temperature. Dietary methionine significantly affected the expression levels of npy, while elevated temperature led to increased agrp expression in cobia.
4.3 cart and cck expression- effects of temperature, methionine levels and a meal
In this study, we were able to identify and characterize a single Cart transcript gene in the cobia transcriptome, while there are multiple paralogs identified in some teleosts, such as ten in Atlantic salmon (Kalananthan et al., 2021) seven in Senegal sole, Solea senegalensis (Bonacic et al., 2015), and six in medaka (Murashita and Kurokawa, 2011). The cobia cart aligns with the cart2a gene family reported for other teleost species (Figure 3) and responds to feeding status. The transcriptome of cobia larvae and pooled tissue has recently been described (Ebeneezar et al., 2023), but as of now, no characterization of brain cobia is available. Thus, a more detailed exploration of the cobia genome and brain regional analysis expression profiles is required to conclude if cobia has other cart paralogs involved in appetite control. It is known that the early evolution of vertebrates was accompanied by two rounds of WGD and that thereafter WGD is only known in ray-finned fish and amphibian lineages. Similar to salmonids, suckers have also experienced recent tetraploidization events (Ravi and Venkatesh, 2018).
Both cart and cck mRNA levels were higher at higher temperatures. Since both are expected to give anorexigenic effects, this may be the direct link to lower appetite and feed intake at 34°C in cobia. The higher temperature might have caused a stress effect that stimulated increased activity of these neuropeptides. Although not directly comparable, a study of the effects of short-term stress on appetite-related genes in zebrafish indicated that while the expression of orexigenic genes was not affected by handling, the anorexigenic genes analyzed, including cart, showed increased expression after stress, thereby suggesting a direct involvement of Cart in the anorexigenic effect of stress (Cortes et al., 2018). Our data support this notion in cobia.
However, the brain expression of cart and cck did not change during a meal, nor were there any differences among the three dietary methionine concentrations (Figures 5C, D ). Thus, there was no clear direct role of Cart and Cck levels in appetite control in the brain related to meal or methionine level control of cobia. CART and CCK have been reported to act as satiety factors that suppress feed intake and terminate ingestion in many vertebrates, including fish (Crawley and Corwin, 1994; Himick and Peter, 1994; Peyon et al., 1999; Gélineau and Boujard, 2001; Volkoff et al., 2003; Matsuda et al., 2012; Morton et al., 2014; Conde-Sieira and Soengas, 2017). For instance, intracerebroventricular (ICV) administration of Cart resulted in reduced feed intake in several species of fish, such as goldfish (Volkoff and Peter, 2000) and channel catfish (Kobayashi et al., 2008). In addition, feed deprivation led to decreased expression levels of cart in Atlantic cod (Kehoe and Volkoff, 2007), goldfish (Volkoff and Peter, 2001), and Atlantic salmon (Murashita et al., 2009), while refeeding resulted in increased cart abundance in channel catfish (Peterson et al., 2012), goldfish (Volkoff and Peter, 2001), and dourado, Salminus brasiliensis (Volkoff et al., 2017). Insulin treatment increased cart in rainbow trout (Libran-Perez et al., 2015) and catfish (Subhedar et al., 2011). Both central and peripheral injections of Cck reduced feed intake in rainbow trout (Gélineau and Boujard, 2001; Jönsson et al., 2006), coho salmon (White et al., 2016), goldfish (Himick and Peter, 1994; Volkoff et al., 2003; Kang et al., 2010), catfish (Silverstein and Plisetskaya, 2000), sea bass (Rubio et al., 2008), and winter flounder, Pseudopleuronectes americanus (MacDonald and Volkoff, 2009). Higher cck mRNA levels after ingesting feed have been reported in goldfish brain (Reimers et al.), yellowtail, Seriola lalandi pyloric cecum (Reimers et al.) and Atlantic salmon brain (Peyon et al., 1999; Murashita et al., 2007; Valen et al., 2011). Meanwhile, the administration of CCK antagonists resulted in an increase in feed intake in rainbow trout (Gélineau and Boujard, 2001).
Whereas the highest expression levels of cck in the goldfish brain were observed in the hypothalamus (Peyon et al., 1999), this information is not available for juvenile cobia. Therefore, regional differences in changes in cobia brain cck levels were not clarified. CCK is found not only in the brain but also in the digestive tract, where it serves as a key regulator of the digestive process, regulates gallbladder release, stimulates the release of pancreatic enzymes, enhances motility, and regulates stomach evacuation (Jensen et al., 2001; Murashita et al., 2006). CCK acts locally in the gut and via enteric and vagal signaling, where it acts on appetite and behavioral control of feed intake. Ingestion of nutrients induces the release of CCK, which relays first to the hindbrain and ultimately to the hypothalamus and brain reward regions (Shechter and Schwartz, 2018). Expression levels of both cck-l and cck-n from the gastrointestinal tract increased within 1.5 hours after a meal (Rønnestad et al., 2010). Thus, to obtain the complete involvement of CCK in the gut-brain axis during a meal, both organs must be assessed in parallel.
Any change in cck expression after a meal may take some time, and some of the dynamic changes in cck expression could have been missed in our experiment due to the sampling strategy. In goldfish, the expression levels of cck in the brain increased 2 h postprandial (Peyon et al., 1999). In Atlantic salmon, there were different patterns of changes in the expression of different isoforms of brain cck after feeding; levels of cck-l were elevated at 12 h postprandial, while there were no changes in the expression of cck-n (Valen et al., 2011).
The gene for pro-opiomelanocortin (POMC) is a precursor for well-known neuropeptide appetite suppressors in the hypothalamus that is also coexpressed with CART in mammals (Schwartz et al., 2000; Soengas et al., 2018). However, pomc was not successfully cloned in cobia, and primers for qPCR were not specific for the detection of levels in pomc cobia brain samples. Thus, we do not currently have a good understanding of the integration of these two important neuropeptides related to metabolic and endocrine signals involved in the control of feed intake.
However, higher levels of cart and cck in cobia at 34°C, concomitant with lower feed intake compared to those fish at 30°C (Figure 1A), indicate that these two neuropeptides may act as anorexigenic factors that reduce feed intake in cobia. Prolonged exposure to unfavorable elevated temperatures likely led to the induction of anorectic signals, resulting in reduced appetite and thus feed intake and thus having negative effects on growth performance in cobia. The apparent paradoxical reduction in feed intake at elevated temperatures is possibly linked to a decrease in aerobic scope that results from higher temperatures (Nilsson et al., 2009). Based on data on clownfish, (Pham et al., 2022) proposed that a reduced meal size limits the standard dynamic action (SDA; increased oxygen consumption after a meal) beyond the aerobic scope to prevent impacts that reduce performance.
4.4 mc4r expression- effects of temperature, methionine levels and a meal
The integration between nutrient sensing and neuroendocrine signaling in regulating appetite is very complex and not yet fully understood in fish (Soengas, 2021). MC4R is known to be involved in the regulation of feed intake and homeostasis/energy expenditure and growth in vertebrates, including fish (Cerdá-Reverter and Peter, 2003; Tao, 2010; Rønnestad et al., 2017). MC4R serves as an integrating receptor in second-order neurons, where its activity is inhibited when stimulated by orexigenic neuropeptides and its activity is increased by competitive anorexigenic neuropeptides (Reimers et al., 1993; Cone, 2006). In cobia, we found only one form of Mc4r that is clustered together within the teleost clade, suggesting an important role in integrating neuronal activity on appetite control and feed intake in cobia. In many teleost species, Mc4r is characterized by more than one paralog (e.g., four Mc4r in Atlantic salmon (Kalananthan et al., 2020a), three in goldfish (Reimers et al.) and one in common carp (Reimers et al.)). To what extent these paralogs play different roles remains to be explored.
In the present trial, mc4r expression was higher at the higher temperature, possibly suggesting a link with the general lower feed intake in cobia at 34°C (Figure 5E). In rats, administration of an MC4R antagonist (SHU9119) increased feeding and induced obesity, suggesting the anorexigenic effects of MC4R on feed intake and homeostasis of the animals (Hagan et al., 1999; Hwa et al., 2001). Additionally, long-term ICV infusions with MC4R antagonists such as HS014 and HS024 in rats result in increased food intake and body weight (Kask et al., 1998; Skuladottir et al., 1999). Mc4r has been characterized in several fish species (Rønnestad et al., 2017; Liu et al., 2019; Kalananthan et al., 2020a), even though the impact of expression levels and the role of this receptor in appetite regulation is still unclear (Kobayashi et al., 2008; Sanchez et al., 2009; Pham et al., 2021). For instance, studies in rainbow trout (Schjolden et al., 2009) and goldfish (Cerdá-Reverter and Peter, 2003; Schjolden et al., 2009) showed reduced feed intake after treatment with an Mcr agonist, and Mcr antagonist injection led to increased feed intake in these species (Cerdá-Reverter and Peter, 2003), supporting the anorexigenic effects of Mc4r. On the other hand, progressive fasting did not affect the expression of mc4r in the hypothalamus of barfin flounder Verasper moseri (Kobayashi et al., 2008) and European sea bass (Sanchez et al., 2009). In the current study, there were no significant differences between prefeeding and postfeeding cobia among dietary methionine and temperature. It is possible that mc4r expression levels vary in different brain regions, with high expression in the preoptic and arcuate nuclei of the brain (Tao, 2010; Liu et al., 2019; Wang et al., 2020). In addition, the role of Mc4rs varies from tissue to tissue. MC4R expressed in the paraventricular nucleus of the hypothalamus is correlated with anorexigenic effects, i.e., inhibition of feed intake, while MC4R expressed by sympathetic neurons within the intermediolateral column of the spinal cord is believed to increase energy expenditure (Sohn, 2015). However, higher levels of mc4r at 34°C correlated with lower FI and poorer growth in cobia, suggesting that Mc4r is involved in the anorexigenic stimulation that suppresses feed intake in this species.
Although we cannot demonstrate the link between dietary methionine levels and mc4r expression, the response of mc4r expression at high temperature differed in fish fed different levels of methionine. In addition, in humans, the protein profile in restricted diets influenced appetite and craving differently in individuals with different gene variants of MC4R (Huang et al., 2017). In this regard, more attention is needed to clarify the role of dietary methionine levels on appetite in fish, including the impact of Mc4r.
To date, some reports in mammals have shown that the limitation of essential AAs alters the expression level of anorexigenic neuropeptides in the hypothalamus [reviewed by (Forney et al., 2018)]. However, our results show that in cobia, the main response was observed in orexigenic npy. The signaling pathways in which restricted dietary methionine affects appetite in mammals seem to involve peripheral organs (e.g., hepatic FGF21) (Forney et al., 2018; Chi et al., 2020), but the pathways in the hypothalamus are still unclear.
5 Conclusion
Elevated temperature (34°C) and dietary methionine deficiency both reduced feed intake in cobia, resulting in retarded growth. Feeding cobia a dietary methionine concentration above the requirement did not promote growth performance, neither in the control nor at the higher temperature. Dietary methionine affected the expression levels of npy. Meanwhile, elevated temperature significantly affected and upregulated the expression of agrp, cart, cck and mc4r. There was a combined effect of dietary methionine and rearing temperature on cobia’s brain expression levels of npy. In addition, expression levels of both npy and agrp were higher before compared to after the meal. At 30°C, prefeeding levels of npy were correlated with both increased methionine levels and feed intake. Taken together, these results support an orexigenic role of Npy and Agrp, as well as the anorexigenic role, and possibly stress-related effects caused by temperature, of Cart and Cck in the signaling pathway of appetite and feeding behavior in cobia. In summary, both feeding, temperature and/or dietary methionine levels affected the brain expression of npy, agrp, cart, cck suggesting that these neuropeptides and the receptor mc4r, are actively involved in adjusting feed intake to compensate for changing energetic demands as well as metabolic adjustments due to variable availability of an essential AA at elevated temperature.
Data availability statement
The gene datasets presented in this study can be found in online repositories. The names of the repository/repositories and accession number(s) can be found below: GenBank, OM962988-OM962990. The remainder of the data supporting the conclusions of this article will be made available by the authors upon request, without undue reservation.
Ethics statement
The animal study was reviewed and approved by Center for Experiments and Practices at Nha Trang University, Nha Trang, Vietnam, chaired by Dr. Nguyen Van Hoa.
Author contributions
MN, LP, A-EJ, ME, LC, MY, SE, ML, and IR conceived and designed the study. MN and ML conducted the experiment. MN, LP, A-EJ, ME, LC, MY, SE, ML, and IR contributed to the sampling. MN and A-EJ performed the primer design, preparatory lab work and qPCR. A-EJ performed the phylogenetic analysis. MN and LP performed the statistical analysis. LP and MN drafted the manuscript. All authors contributed to the article and approved the submitted version.
Funding
The study received funding from the European Union’s H2020 programme (Marie Skłodowska-Curie grant No 691150; WISEFEED). Additional funding was received from the Norwegian Agency for Development Cooperation NORHED, No. QZA-0485 SRV-13/0010, and MCIU-AEI + ERDF Spain project THERMODIGEST RTI2018-096134-B-I00. SE acknowledges an investigator grant IF/00482/2014/CP1217/CT0005 and national funds through projects UIDB/04326/2020, UIDP/04326/2020 and LA/P/0101/2020 from FCT. IR also acknowledges funds from the Research Council of Norway (grant No. 311627).
Conflict of interest
Author LC was employed by SPAROS Lda.
The remaining authors declare that the research was conducted in the absence of any commercial or financial relationships that could be construed as a potential conflict of interest.
Publisher’s note
All claims expressed in this article are solely those of the authors and do not necessarily represent those of their affiliated organizations, or those of the publisher, the editors and the reviewers. Any product that may be evaluated in this article, or claim that may be made by its manufacturer, is not guaranteed or endorsed by the publisher.
Supplementary material
The Supplementary Material for this article can be found online at: https://www.frontiersin.org/articles/10.3389/fmars.2023.1183967/full#supplementary-material
Footnotes
- ^ https://www.ncbi.nlm.nih.gov/nuccore/257062718
- ^ https://services.healthtech.dtu.dk/service.php?SignalP-5.0
- ^ https://services.healthtech.dtu.dk/service.php?SignalP-5.0
- ^ http://stagbeetle.animal.uiuc.edu/cgi-bin/neuropred.py
- ^ http://www.predisi.de/home.html
- ^ https://dtu.biolib.com/DeepTMHMM/
- ^ http://stagbeetle.animal.uiuc.edu/cgi-bin/neuropred.py
- ^ http://stagbeetle.animal.uiuc.edu/cgi-bin/neuropred.py
- ^ https://www.uniprot.org/blast/
- ^ https://zfin.org/ZDB-GENE-150212-1#summary
- ^ https://dtu.biolib.com/DeepTMHMM/
- ^ http://www.predisi.de/index.html
- ^ http://stagbeetle.animal.uiuc.edu/cgi-bin/neuropred.py
- ^ https://dtu.biolib.com/DeepTMHMM/
- ^ https://zfin.org/ZDB-GENE-150212-1#summary
References
Aldegunde M., Mancebo M. (2006). Effects of neuropeptide y on food intake and brain biogenic amines in the rainbow trout (Oncorhynchus mykiss). Peptides 27 (4), 719–727. doi: 10.1016/j.peptides.2005.09.014
Andersen S. M., Waagbø R., Espe M. (2016). Functional amino acids in fish health and welfare. Front. Biosci. 8, 143–169. doi: 10.2741/e757
Anderson E. J., Cakir I., Carrington S. J., Cone R. D., Ghamari-Langroudi M., Gillyard T., et al. (2016). 60 YEARS OF POMC: regulation of feeding and energy homeostasis by alpha-MSH. J. Mol. Endocrinol. 56 (4), T157–T174. doi: 10.1530/JME-16-0014
Bender D. A. (2012). “Amino acids synthesized from aspartate: lysine, methionine (and cysteine), threonine and isoleucine,” in Amino acid metabolism. Ed. Bender D. A. (UK: John Wiley & Sons, Ltd.), 225–277.
Betancur R. R., Li C., Munroe T. A., Ballesteros J. A., Ortí G. (2013). Addressing gene tree discordance and non-stationarity to resolve a multi-locus phylogeny of the flatfishes (Teleostei: pleuronectiformes). Syst. Biol. 62 (5), 763–785. doi: 10.1093/sysbio/syt039
Bonacic K., Martínez A., Martín-Robles Á.J., Muñoz-Cueto J. A., Morais S. (2015). Characterization of seven cocaine- and amphetamine-regulated transcripts (CARTs) differentially expressed in the brain and peripheral tissues of solea senegalensis (Kaup). Gen. Comp. Endocrinol. 224, 260–272. doi: 10.1016/j.ygcen.2015.08.017
Burt K., Hamoutene D., Perez-Casanova J., Kurt Gamperl A., Volkoff H. (2013). The effect of intermittent hypoxia on growth, appetite and some aspects of the immune response of Atlantic salmon (Salmo salar). Aquacul. Res. 45 (1), 124–137. doi: 10.1111/j.1365-2109.2012.03211.x
Bustin S. A., Beaulieu J.-F., Huggett J., Jaggi R., Kibenge F. S. B., Olsvik P. A., et al. (2010). MIQE précis: practical implementation of minimum standard guidelines for fluorescence-based quantitative real-time PCR experiments. BMC Mol. Biol. 11 (1), 74. doi: 10.1186/1471-2199-11-74
Butler A. A., Girardet C., Mavrikaki M., Trevaskis J. L., Macarthur H., Marks D. L., et al. (2017). A life without hunger: the ups (and downs) to modulating melanocortin-3 receptor signaling. Front. Neurosci. 11. doi: 10.3389/fnins.2017.00128
Cerdá-Reverter J. M., Peter R. E. (2003). Endogenous melanocortin antagonist in fish: structure, brain mapping, and regulation by fasting of the goldfish agouti-related protein gene. Endocrinology 144 (10), 4552–4561. doi: 10.1210/en.2003-0453
Chi S., He Y., Zhu Y., Tan B., Dong X., Yang Q., et al. (2020). Dietary methionine affects growth and the expression of key genes involved in hepatic lipogenesis and glucose metabolism in cobia (Rachycentron canadum). Aquacul. Nutr. 26 (1), 123–133. doi: 10.1111/anu.13006
Chou R.-L., Su M.-S., Chen H.-Y. (2001). Optimal dietary protein and lipid levels for juvenile cobia (Rachycentron canadum). Aquaculture 193 (1), 81–89. doi: 10.1016/S0044-8486(00)00480-4
Comesaña S., Velasco C., Ceinos R. M., López-Patiño M. A., Míguez J. M., Morais S., et al. (2018). Evidence for the presence in rainbow trout brain of amino acid-sensing systems involved in the control of food intake. Am. J. Physiol. Regul. Integr. Comp. Physiol. 314 (2):R201–R215. doi: 10.1152/ajpregu.00283.2017
Conceição L. E. C., Aragão C., Dias J., Costas B., Terova G., Martins C., et al. (2012). Dietary nitrogen and fish welfare. Fish Physiol. Biochem. 38 (1), 119–141. doi: 10.1007/s10695-011-9592-y
Conde-Sieira M., Soengas J. L. (2017). Nutrient sensing systems in fish: impact on food intake regulation and energy homeostasis. Front. Neurosci. 10:1–21 doi: 10.3389/fnins.2016.00603
Cone R. D. (2006). Studies on the physiological functions of the melanocortin system. Endocrine Rev. 27 (7), 736–749. doi: 10.1210/er.2006-0034
Cortes R., Teles M., Oliveira M., Fierro-Castro C., Tort L., Cerda-Reverter J. M. (2018). Effects of acute handling stress on short-term central expression of orexigenic/anorexigenic genes in zebrafish. Fish Physiol. Biochem. 44 (1), 257–272. doi: 10.1007/s10695-017-0431-7
Crawley J. N., Corwin R. L. (1994). Biological actions of cholecystokinin. Peptides 15 (4), 731–755. doi: 10.1016/0196-9781(94)90104-X
Delgado M. J., Cerdá-Reverter J. M., Soengas J. L. (2017). Hypothalamic integration of metabolic, endocrine, and circadian signals in fish: involvement in the control of food intake. Front. Neurosci. 11. doi: 10.3389/fnins.2017.00354
de Pedro N., López-Patiño M. A., Guijarro A. I., Pinillos M. L., Delgado M. J., Alonso-Bedate M. (2000). NPY receptors and opioidergic system are involved in NPY-induced feeding in goldfish. Peptides 21 (10), 1495–1502. doi: 10.1016/s0196-9781(00)00303-x
Ebeneezar S., Krupesha Sharma S. R., Vijayagopal P., Sebastian W., Sajina K. A., Tamilmani G., et al. (2023). Full-length transcriptome from different life stages of cobia (Rachycentron canadum, rachycentridae). Sci. Data 10 (1), 97. doi: 10.1038/s41597-022-01907-0
Elmada C. Z., Huang W., Jin M., Liang X., Mai K., Zhou Q. (2016). The effect of dietary methionine on growth, antioxidant capacity, innate immune response and disease resistance of juvenile yellow catfish (Pelteobagrus fulvidraco). Aquacul. Nutr. 22 (6), 1163–1173. doi: 10.1111/anu.12363
Espe M., Veiseth-Kent E., Zerrahn J.-E., Rønnestad I., Aksnes A. (2016). Juvenile Atlantic salmon decrease white trunk muscle IGF-1 expression and reduce muscle and plasma free sulphur amino acids when methionine availability is low while liver sulphur metabolites mostly is unaffected by treatment. Aquacul. Nutr. 22 (4), 801–812. doi: 10.1111/anu.12294
Fernö A., Huse I., Juell J.-E., Bjordal Å. (1995). Vertical distribution of Atlantic salmon (Salmo solar l.) in net pens: trade-off between surface light avoidance and food attraction. Aquaculture 132 (3), 285–296. doi: 10.1016/0044-8486(94)00384-Z
Fleige S., Pfaffl M. W. (2006). RNA Integrity and the effect on the real-time qRT-PCR performance. Mol. Aspects Med. 27 (2-3), 126–139. doi: 10.1016/j.mam.2005.12.003
Folkedal O., Stien L. H., Torgersen T., Oppedal F., Olsen R. E., Fosseidengen J. E., et al. (2012). Food anticipatory behaviour as an indicator of stress response and recovery in Atlantic salmon post-smolt after exposure to acute temperature fluctuation. Physiol. Behav. 105 (2), 350–356. doi: 10.1016/j.physbeh.2011.08.008
Forney L. A., Stone K. P., Wanders D., Gettys T. W. (2018). Sensing and signaling mechanisms linking dietary methionine restriction to the behavioral and physiological components of the response. Front. Neuroendocrinol 51, 36–45. doi: 10.1016/j.yfrne.2017.12.002
Forney L. A., Wanders D., Stone K. P., Pierse A., Gettys T. W. (2017). Concentration-dependent linkage of dietary methionine restriction to the components of its metabolic phenotype. Obes. (Silver Spring) 25 (4), 730–738. doi: 10.1002/oby.21806
Fromentin G., Darcel N., Chaumontet C., Marsset-Baglieri A., Nadkarni N., Tomé D. (2012). “Peripheral and central mechanisms involved in the control of food intake by dietary amino acids and proteins,” in Nutrition research reviews. (Cambridge University Press) 25(1):29–39
Gao Z., Wang X., Tan C., Zhou H., Mai K., He G. (2019). Effect of dietary methionine levels on growth performance, amino acid metabolism and intestinal homeostasis in turbot (Scophthalmus maximus l.). Aquaculture 498, 335–342. doi: 10.1016/j.aquaculture.2018.08.053
Gélineau A., Boujard T. (2001). Oral administration of cholecystokinin receptor antagonists increase feed intake in rainbow trout. J. Fish Biol. 58 (3), 716–724. doi: 10.1111/j.1095-8649.2001.tb00524.x
Glencross B. D., Bermudes M. (2011). The effect of high water temperatures on the allometric scaling effects of energy and protein starvation losses in juvenile barramundi, Lates calcarifer. Comp. Biochem. Physiol. Part A: Mol. Integr. Physiol. 159 (2), 167–174. doi: 10.1016/j.cbpa.2011.02.013
Glencross B. D., Bermudes M. (2012). Adapting bioenergetic factorial modelling to understand the implications of heat stress on barramundi (Lates calcarifer) growth, feed utilisation and optimal protein and energy requirements – potential strategies for dealing with climate change? Aquacul. Nutr. 18 (4), 411–422. doi: 10.1111/j.1365-2095.2011.00913.x
Hagan M. M., Rushing P. A., Schwartz M. W., Yagaloff K. A., Burn P., Woods S. C., et al. (1999). Role of the CNS melanocortin system in the response to overfeeding. J. Neurosci. 19 (6), 2362–2367. doi: 10.1523/jneurosci.19-06-02362.1999
Hall B. G. (2013). Building phylogenetic trees from molecular data with MEGA. Mol. Biol. Evol. 30 (5), 1229–1235. doi: 10.1093/molbev/mst012
He Y., Chi S., Tan B., Dong X., Yang Q., Liu H., et al. (2021). Effects of three methionine sources in diets on temporal B0AT1 and ASCT2 mRNA expression in different intestinal segments of cobia, Rachycentron canadum. J. World Aquacul. Soc. 52 (1), 171–183. doi: 10.1111/jwas.12743
Hevrøy E. M., Azpeleta C., Shimizu M., Lanzén A., Kaiya H., Espe M., et al. (2011). Effects of short-term starvation on ghrelin, GH-IGF system, and IGF-binding proteins in Atlantic salmon. Fish Physiol. Biochem. 37 (1), 217–232. doi: 10.1007/s10695-010-9434-3
Himick B. A., Peter R. E. (1994). CCK/gastrin-like immunoreactivity in brain and gut, and CCK suppression of feeding in goldfish. Am. J. Physiol. 267 (3 Pt 2), R841–R851. doi: 10.1152/ajpregu.1994.267.3.R841
Huang T., Zheng Y., Hruby A., Williamson D. A., Bray G. A., Shen Y., et al. (2017). Dietary protein modifies the effect of the MC4R genotype on 2-year changes in appetite and food craving: the POUNDS lost trial. J. Nutr. 147 (3), 439–444. doi: 10.3945/jn.116.242958
Huggett J., Dheda K., Bustin S., Zumla A. (2005). Real-time RT-PCR normalisation; strategies and considerations. Genes Immun. 6 (4), 279–284. doi: 10.1038/sj.gene.6364190
Hwa J. J., Ghibaudi L., Gao J., Parker E. M. (2001). Central melanocortin system modulates energy intake and expenditure of obese and lean zucker rats. Am. J. Physiol. Regul. Integr. Comp. Physiol. 281 (2), R444–R451. doi: 10.1152/ajpregu.2001.281.2.R444
IPCC (2021). Climate change 2021: the physical science basis. contribution of working group I to the sixth assessment report of the intergovernmental panel on climate change. (Switzerland: Alisa Singer)
Jensen H., Rourke I. J., Møller M., Jønson L., Johnsen A. H. (2001). Identification and distribution of CCK-related peptides and mRNAs in the rainbow trout, Oncorhynchus mykiss. Biochim. Biophys. Acta (BBA) - Gene Structure Expression 1517 (2), 190–201. doi: 10.1016/S0167-4781(00)00263-3
Jones D. T., Taylor W. R., Thornton J. M. (1992). The rapid generation of mutation data matrices from protein sequences. Bioinformatics 8 (3), 275–282. doi: 10.1093/bioinformatics/8.3.275
Jönsson E., Forsman A., Einarsdottir I. E., Egnér B., Ruohonen K., Björnsson B. T. (2006). Circulating levels of cholecystokinin and gastrin-releasing peptide in rainbow trout fed different diets. Gen. Comp. Endocrinol. 148 (2), 187–194. doi: 10.1016/j.ygcen.2006.02.016
Kalananthan T., Gomes A. S., Lai F., Tolås I., Jordal A.-E. O., Norland S., et al. (2021). Brain distribution of 10 cart transcripts and their response to 4 days of fasting in Atlantic salmon (Salmo salar l.). Front. Mar. Sci. 8. doi: 10.3389/fmars.2021.763766
Kalananthan T., Lai F., Gomes A. S., Murashita K., Handeland S., Rønnestad I. (2020a). The melanocortin system in Atlantic salmon (Salmo salar l.) and its role in appetite control. Front. Neuroanat. 14. doi: 10.3389/fnana.2020.00048
Kalananthan T., Murashita K., Rønnestad I., Ishigaki M., Takahashi K., Silva M. S., et al. (2020b). Hypothalamic agrp and pomc mRNA responses to gastrointestinal fullness and fasting in Atlantic salmon (Salmo salar, l.). Front. Physiol. 11. doi: 10.3389/fphys.2020.00061
Kang K. S., Yahashi S., Azuma M., Matsuda K. (2010). The anorexigenic effect of cholecystokinin octapeptide in a goldfish model is mediated by the vagal afferent and subsequently through the melanocortin- and corticotropin-releasing hormone-signaling pathways. Peptides 31 (11), 2130–2134. doi: 10.1016/j.peptides.2010.07.019
Kask A., Mutulis F., Muceniece R., Paühkla R., Mutule I., Wikberg J. E. S., et al. (1998). Discovery of a novel superpotent and selective melanocortin-4 receptor antagonist (HS024): evaluation in vitro and in vivo. Endocrinology 139 (12), 5006–5014. doi: 10.1210/endo.139.12.6352
Kehoe A. S., Volkoff H. (2007). Cloning and characterization of neuropeptide y (NPY) and cocaine and amphetamine regulated transcript (CART) in Atlantic cod (Gadus morhua). Comp. Biochem. Physiol. Part A: Mol. Integr. Physiol. 146 (3), 451–461. doi: 10.1016/j.cbpa.2006.12.026
Kim J.-H., Leggatt R. A., Chan M., Volkoff H., Devlin R. H. (2015). Effects of chronic growth hormone overexpression on appetite-regulating brain gene expression in coho salmon. Mol. Cell. Endocrinol. 413, 178–188. doi: 10.1016/j.mce.2015.06.024
Kobayashi Y., Peterson B. C., Waldbieser G. C. (2008). Association of cocaine- and amphetamine-regulated transcript (CART) messenger RNA level, food intake, and growth in channel catfish. Comp. Biochem. Physiol. Part A: Mol. Integr. Physiol. 151 (2), 219–225. doi: 10.1016/j.cbpa.2008.06.029
Kousoulaki K., Rønnestad I., Olsen H. J., Rathore R., Campbell P., Nordrum S., et al. (2013). Krill hydrolysate free amino acids responsible for feed intake stimulation in Atlantic salmon (Salmo salar). Aquacul. Nutr. 19 (s1), 47–61. doi: 10.1111/anu.12094
Kumar S., Stecher G., Li M., Knyaz C., Tamura K. (2018). MEGA X: molecular evolutionary genetics analysis across computing platforms. Mol. Biol. Evol. 35 (6), 1547–1549. doi: 10.1093/molbev/msy096
Lai F., Royan M. R., Gomes A. S., Espe M., Aksnes A., Norberg B., et al. (2021). The stress response in Atlantic salmon (Salmo salar l.): identification and functional characterization of the corticotropin-releasing factor (crf) paralogs. Gen. Comp. Endocrinol. 313, 113894. doi: 10.1016/j.ygcen.2021.113894
Li J., Xu W., Lai W., Kong A., Zhang Z., Pang Y., et al. (2021). Effect of dietary methionine on growth performance, lipid metabolism and antioxidant capacity of large yellow croaker (Larimichthys crocea) fed with high lipid diets. Aquaculture 536, 736388. doi: 10.1016/j.aquaculture.2021.736388
Libran-Perez M., Velasco C., Otero-Rodino C., Lopez-Patino M. A., Miguez J. M., Soengas J. L. (2015). Effects of insulin treatment on the response to oleate and octanoate of food intake and fatty acid-sensing systems in rainbow trout. Domest Anim. Endocrinol. 53, 124–135. doi: 10.1016/j.domaniend.2015.06.004
Liu R., Kinoshita M., Adolfi M. C., Schartl M. (2019). Analysis of the role of the MC4R system in development, growth, and puberty of medaka. Front. Endocrinol. 10. doi: 10.3389/fendo.2019.00213
López-Patiño M. A., Guijarro A. I., Isorna E., Delgado M. J., Alonso-Bedate M., de Pedro N. (1999). Neuropeptide y has a stimulatory action on feeding behavior in goldfish (Carassius auratus). Eur. J. Pharmacol. 377 (2), 147–153. doi: 10.1016/S0014-2999(99)00408-2
Luo Z., Liu Y.-j., Mai K.-s., Tian L.-x., Yang H.-j., Tan X.-y., et al. (2005). Dietary l-methionine requirement of juvenile grouper Epinephelus coioides at a constant dietary cystine level. Aquaculture 249 (1), 409–418. doi: 10.1016/j.aquaculture.2005.04.030
MacDonald E., Volkoff H. (2009). Neuropeptide y (NPY), cocaine- and amphetamine-regulated transcript (CART) and cholecystokinin (CCK) in winter skate (Raja ocellata): cDNA cloning, tissue distribution and mRNA expression responses to fasting. Gen. Comp. Endocrinol. 161 (2), 252–261. doi: 10.1016/j.ygcen.2009.01.021
Mai K., Wan J., Ai Q., Xu W., Liufu Z., Zhang L., et al. (2006). Dietary methionine requirement of large yellow croaker, Pseudosciaena crocea r. Aquaculture 253 (1), 564–572. doi: 10.1016/j.aquaculture.2005.08.010
Matsuda K., Sakashita A., Yokobori E., Azuma M. (2012). Neuroendocrine control of feeding behavior and psychomotor activity by neuropeptideY in fish. Neuropeptides 46 (6), 275–283. doi: 10.1016/j.npep.2012.09.006
Mohamed J. S., Benninghoff A. D., Holt G. J., Khan I. A. (2007). Developmental expression of the G protein-coupled receptor 54 and three GnRH mRNAs in the teleost fish cobia. J. Mol. Endocrinol. 38 (1-2), 235–244. doi: 10.1677/jme.1.02182
Morton G. J., Meek T. H., Schwartz M. W. (2014). Neurobiology of food intake in health and disease. Nat. Rev. Neurosci. 15 (6), 367–378. doi: 10.1038/nrn3745
Muller P. Y., Janovjak H., Miserez A. R., Dobbie Z. (2002). Processing of gene expression data generated by quantitative real-time RT-PCR. Biotechniques 32 (6), 1378–1379.
Murashita K., Fukada H., Hosokawa H., Masumoto T. (2006). Cholecystokinin and peptide y in yellowtail (Seriola quinqueradiata): molecular cloning, real-time quantitative RT-PCR, and response to feeding and fasting. Gen. Comp. Endocrinol. 145 (3), 287–297. doi: 10.1016/j.ygcen.2005.09.008
Murashita K., Fukada H., Hosokawa H., Masumoto T. (2007). Changes in cholecystokinin and peptide y gene expression with feeding in yellowtail (Seriola quinqueradiata): relation to pancreatic exocrine regulation. Comp. Biochem. Physiol. Part B: Biochem. Mol. Biol. 146 (3), 318–325. doi: 10.1016/j.cbpb.2006.11.009
Murashita K., Kurokawa T. (2011). Multiple cocaine- and amphetamine-regulated transcript (CART) genes in medaka, Oryzias latipes: cloning, tissue distribution and effect of starvation. Gen. Comp. Endocrinol. 170 (3), 494–500. doi: 10.1016/j.ygcen.2010.11.005
Murashita K., Kurokawa T., Ebbesson L. O. E., Stefansson S. O., Rønnestad I. (2009). Characterization, tissue distribution, and regulation of agouti-related protein (AgRP), cocaine- and amphetamine-regulated transcript (CART) and neuropeptide y (NPY) in Atlantic salmon (Salmo salar). Gen. Comp. Endocrinol. 162 (2), 160–171. doi: 10.1016/j.ygcen.2009.03.015
Murthy, Varghese (1998). Total sulphur amino acid requirement of the Indian major carp, Labeo rohita (Hamilton). Aquacul. Nutr. 4 (1), 61–65. doi: 10.1046/j.1365-2095.1998.00045.x
Narnaware Y. K., Peter R. E. (2001). Effects of food deprivation and refeeding on neuropeptide y (NPY) mRNA levels in goldfish. Comp. Biochem. Physiol. B Biochem. Mol. Biol. 129 (2-3), 633–637. doi: 10.1016/S1096-4959(01)00359-1
Narnaware Y. K., Peyon P. P., Lin X., Peter R. E. (2000). Regulation of food intake by neuropeptide y in goldfish. Am. J. Physiol. Regul. Integr. Comp. Physiol. 279 (3), R1025–R1034. doi: 10.1152/ajpregu.2000.279.3.R1025
Nguyen M. V., Espe M., Conceição L. E. C., Le H. M., Yúfera M., Engrola S. A. D., et al. (2019). The role of dietary methionine concentrations on growth, metabolism and n-retention in cobia (Rachycentron canadum) at elevated water temperatures. Aquacul. Nutr. 25 (2), 495–507. doi: 10.1111/anu.12875
Nguyen M. V., Jordal A. E. O., Espe M., Buttle L., Lai H. V., Rønnestad I. (2013). Feed intake and brain neuropeptide y (NPY) and cholecystokinin (CCK) gene expression in juvenile cobia fed plant-based protein diets with different lysine to arginine ratios. Comp. Biochem. Physiol. - A Mol. Integr. Physiol. 165 (3), 328–337. doi: 10.1016/j.cbpa.2013.04.004
Nilsson G. E., Crawley N., Lunde I. G., Munday P. L. (2009). Elevated temperature reduces the respiratory scope of coral reef fishes. Global Change Biol. 15 (6), 1405–1412. doi: 10.1111/j.1365-2486.2008.01767.x
Niu J., Du Q., Lin H.-Z., Cheng Y.-Q., Huang Z., Wang Y., et al. (2013). Quantitative dietary methionine requirement of juvenile golden pompano trachinotus ovatus at a constant dietary cystine level. Aquacul. Nutr. 19 (5), 677–686. doi: 10.1111/anu.12015
NRC (2011). Nutrient requirements of fish and shrimp (Washington, DC: The National Academies Press).
Peres H., Oliva-Teles A. (2005). The effect of dietary protein replacement by crystalline amino acid on growth and nitrogen utilization of turbot Scophthalmus maximus juveniles. Aquaculture 250 (3), 755–764. doi: 10.1016/j.aquaculture.2005.04.046
Peterson B. C., Waldbieser G. C., Riley L. G., Upton K. R., Kobayashi Y., Small B. C. (2012). Pre- and postprandial changes in orexigenic and anorexigenic factors in channel catfish (Ictalurus punctatus). Gen. Comp. Endocrinol. 176 (2), 231–239. doi: 10.1016/j.ygcen.2012.01.022
Peyon P., Saied H., Lin X., Peter R. E. (1999). Postprandial, seasonal and sexual variations in cholecystokinin gene expression in goldfish brain. Mol. Brain Res. 74 (1), 190–196. doi: 10.1016/S0169-328X(99)00282-X
Pham L. P., Nguyen M. V., Jordal A.-E. O., Rønnestad I. (2022). Metabolic rates, feed intake, appetite control, and gut transit of clownfish Amphiprion ocellaris exposed to increased temperature and limited feed availability. Comp. Biochem. Physiol. Part A: Mol. Integr. Physiol. 111318:1–8. doi: 10.1016/j.cbpa.2022.111318
Pham L. P., Olderbakk Jordal A.-E., Nguyen M. V., Rønnestad I. (2021). Food intake, growth, and expression of neuropeptides regulating appetite in clown anemonefish (Amphiprion ocellaris) exposed to predicted climate changes. Gen. Comp. Endocrinol. 304, 113719–113719. doi: 10.1016/j.ygcen.2021.113719
Ravi V., Venkatesh B. (2018). The divergent genomes of teleosts. Annu. Rev. Anim. Biosci. 6, 47–68. doi: 10.1146/annurev-animal-030117-014821
Reichmann F., Holzer P. (2016). Neuropeptide y: a stressful review. Neuropeptides 55 (1), 99–109. doi: 10.1016/j.npep.2015.09.008
Reimers E., Kjørrefjord A. G., Stavøstrand S. M. (1993). Compensatory growth and reduced maturation in second sea winter farmed Atlantic salmon following starvation in February and march. J. Fish Biol. 43 (5), 805–810. doi: 10.1111/j.1095-8649.1993.tb01156.x
Rønnestad I., Gomes A. S., Murashita K., Angotzi R., Jönsson E., Volkoff H. (2017). Appetite-controlling endocrine systems in teleosts. Front. Endocrinol. 8. doi: 10.3389/fendo.2017.00073
Rønnestad I., Jordal A.-E. O., Gomes A. S. (2016). Nutrient sensing in Atlantic salmon - exploring the sensory systems for amino acids in the gastrointestinal tract. FASEB J. 30 (S1), 1020.1022–1020.1022. doi: 10.1096/fasebj.30.1_supplement.1020.2
Rønnestad I., Valen R., Murashita K., Jordal A.-E. O. (2010). Postprandial changes in GI-tract peptide hormones in the teleost Atlantic salmon. FASEB J. 24 (S1), lb620–lb620. doi: 10.1096/fasebj.24.1_supplement.lb620
Rubio V. C., Sánchez-Vázquez F. J., Zamora S., Madrid J. A. (2008). Endogenous modification of macronutrient selection pattern in sea bass (Dicentrarchus labrax, l.). Physiol. Behav. 95 (1), 32–35. doi: 10.1016/j.physbeh.2008.03.006
Sanchez E., Rubio V. C., Thompson D., Metz J., Flik G., Millhauser G. L., et al. (2009). Phosphodiesterase inhibitor-dependent inverse agonism of agouti-related protein on melanocortin 4 receptor in sea bass (Dicentrarchus labrax). Am. J. Physiol. Regul. Integr. Comp. Physiol. 296 (5), R1293–R1306. doi: 10.1152/ajpregu.90948.2008
Sánchez-Lozano N. B., Martínez-Llorens S., Tomás-Vidal A., Jover Cerdá M. (2011). Amino acid retention of gilthead sea bream (Sparus aurata, l.) fed with pea protein concentrate. Aquacul. Nutr. 17 (2), e604–e614. doi: 10.1111/j.1365-2095.2010.00803.x
Sandersfeld T., Davison W., Lamare M. D., Knust R., Richter C. (2015). Elevated temperature causes metabolic trade-offs at the wholeorganism level in the Antarctic fish Trematomus bernacchii. J. Exp. Biol. 218 (15), 2373–2381. doi: 10.1242/jeb.122804
Schjolden J., Schiöth H. B., Larhammar D., Winberg S., Larson E. T. (2009). Melanocortin peptides affect the motivation to feed in rainbow trout (Oncorhynchus mykiss). Gen. Comp. Endocrinol. 160 (2), 134–138. doi: 10.1016/j.ygcen.2008.11.003
Schwartz M. W., Woods S. C., Porte D., Seeley R. J., Baskin D. G. (2000). Central nervous system control of food intake. Nature 404 (6778), 661–671. doi: 10.1038/35007534
Shainer I., Buchshtab A., Hawkins T. A., Wilson S. W., Cone R. D., Gothilf Y. (2017). Novel hypophysiotropic AgRP2 neurons and pineal cells revealed by BAC transgenesis in zebrafish. Sci. Rep. 7(44777):1–10. doi: 10.1038/srep44777
Shechter A., Schwartz G. J. (2018). Gut-brain nutrient sensing in food reward. Appetite 122, 32–35. doi: 10.1016/j.appet.2016.12.009
Silverstein J. T., Breininger J., Baskin D. G., Plisetskaya E. M. (1998). Neuropeptide y-like gene expression in the salmon brain increases with fasting. Gen. Comp. Endocrinol. 110 (2), 157–165. doi: 10.1006/gcen.1998.7058
Silverstein J. T., Plisetskaya E. M. (2000). The effects of NPY and insulin on food intake regulation in fish. Am. Zoologist 40296–308, 213 (2). doi: 10.1093/icb/40.2.296
Skuladottir G. V., Jonsson L., Skarphedinsson J. O., Mutulis F., Muceniece R., Raine A., et al. (1999). Long term orexigenic effect of a novel melanocortin 4 receptor selective antagonist. Br. J. Pharmacol. 126 (1), 27–34. doi: 10.1038/sj.bjp.0702264
Soengas J. L. (2021). Integration of nutrient sensing in fish hypothalamus. Front. Neurosci. 15:1–8 doi: 10.3389/fnins.2021.653928
Soengas J. L., Cerdá-Reverter J. M., Delgado M. J. (2018). Central regulation of food intake in fish: an evolutionary perspective. J. Mol. Endocrinol. 60 (4), R171–R199. doi: 10.1530/JME-17-0320
Sohn J.-W. (2015). Network of hypothalamic neurons that control appetite. BMB Rep. 48 (4), 229–233. doi: 10.5483/bmbrep.2015.48.4.272
Song Y., Golling G., Thacker T. L., Cone R. D. (2003). Agouti-related protein (AGRP) is conserved and regulated by metabolic state in the zebrafish, Danio rerio. Endocrine 22 (3), 257–265. doi: 10.1385/ENDO:22:3:257
Striberny A., Ravuri C. S., Jobling M., Jørgensen E. H., Fuentes J. (2015). Seasonal differences in relative gene expression of putative central appetite regulators in arctic charr (Salvelinus alpinus) do not reflect its annual feeding cycle. PloS One 10 (9), e0138857–e0138857. doi: 10.1371/journal.pone.0138857
Subhedar N., Barsagade V. G., Singru P. S., Thim L., Clausen J. T. (2011). Cocaine- and amphetamine-regulated transcript peptide (CART) in the telencephalon of the catfish, clarias gariepinus: distribution and response to fasting, 2-deoxy-D-glucose, glucose, insulin, and leptin treatments. J. Comp. Neurol. 519 (7), 1281–1300. doi: 10.1002/cne.22569
Sun L., Chen H. (2014). Effects of water temperature and fish size on growth and bioenergetics of cobia (Rachycentron canadum). Aquaculture 426-427, 172–180. doi: 10.1016/j.aquaculture.2014.02.001
Takeuchi S. (2016). Subchapter 8B - Agouti-Related Protein In: Y. Takei, H. Ando & K. Tsutsui, eds. Handbook of Hormones, (San Diego: Academic Press), 70-71.
Tao Y. X. (2010). The melanocortin-4 receptor: physiology, pharmacology, and pathophysiology. Endocrine Rev. 31 (4), 506–543. doi: 10.1210/er.2009-0037
Thebault H., Alliot E., Pastoureaud A. (1985). Quantitative methionine requirement of juvenile sea-bass (Dicentrarchus labrax). Aquaculture 50 (1), 75–87. doi: 10.1016/0044-8486(85)90154-1
Tian J., He G., Mai K., Liu C. (2015). Effects of postprandial starvation on mRNA expression of endocrine-, amino acid and peptide transporter-, and metabolic enzyme-related genes in zebrafish (Danio rerio). Fish Physiol. Biochem. 41 (3), 773–787. doi: 10.1007/s10695-015-0045-x
Tolås I., Kalananthan T., Gomes A. S., Lai F., Norland S., Murashita K., et al. (2021). Regional expression of npy mRNA paralogs in the brain of Atlantic salmon (Salmo salar, l.) and response to fasting. Front. Physiol. 12. doi: 10.3389/fphys.2021.720639
Valen R., Jordal A. E. O., Murashita K., Rønnestad I. (2011). Postprandial effects on appetite-related neuropeptide expression in the brain of Atlantic salmon, Salmo salar. Gen. Comp. Endocrinol. 171 (3), 359–366. doi: 10.1016/j.ygcen.2011.02.027
Van Nguyen M., Rønnestad I., Buttle L., Van Lai H., Espe M. (2014). Imbalanced lysine to arginine ratios reduced performance in juvenile cobia (Rachycentron canadum) fed high plant protein diets. Aquacul. Nutr. 20 (1), 25–35. doi: 10.1111/anu.12043
Volkoff H. (2016a). “Chapter 14 - feeding behavior, starvation response, and endocrine regulation of feeding in Mexican blind cavefish (Astyanax fasciatus mexicanus),” in Biology and evolution of the Mexican cavefish. Eds. Keene A. C., McGaugh M.Y. &S. E. (Amsterdam: Academic Press), 269–290.
Volkoff H. (2016b). The neuroendocrine regulation of food intake in fish: a review of current knowledge. Front. Neurosci 10:1–31. doi: 10.3389/fnins.2016.00540
Volkoff H., Esatevan Sabioni R., Coutinho L. L., Cyrino J. E. P. (2017). Appetite regulating factors in pacu (Piaractus mesopotamicus): tissue distribution and effects of food quantity and quality on gene expression. Comp. Biochem. Physiol. -Part A Mol. Integr. Physiol. 203, 241–254. doi: 10.1016/j.cbpa.2016.09.022
Volkoff H., Eykelbosh A. J., Peter R. E. (2003). Role of leptin in the control of feeding of goldfish carassius auratus: interactions with cholecystokinin, neuropeptide y and orexin a, and modulation by fasting. Brain Res. 972 (1-2), 90–109. doi: 10.1016/s0006-8993(03)02507-1
Volkoff H., Peter R. E. (2000). Effects of CART peptides on food consumption, feeding and associated behaviors in the goldfish, Carassius auratus: actions on neuropeptide y- and orexin a-induced feeding. Brain Res. 887 (1), 125–133. doi: 10.1016/S0006-8993(00)03001-8
Volkoff H., Peter R. E. (2001). Characterization of two forms of cocaine- and amphetamine-regulated transcript (CART) peptide precursors in goldfish: molecular cloning and distribution, modulation of expression by nutritional status, and interactions with leptin. Endocrinology 142 (12), 5076–5088. doi: 10.1210/endo.142.12.8519
Volkoff H., Rønnestad I. (2020). Effects of temperature on feeding and digestive processes in fish. Temperature 7 (4), 307–320. doi: 10.1080/23328940.2020.1765950
Walton M. J., Cowey C. B., Adron J. W. (1982). Methionine metabolism in rainbow trout fed diets of differing methionine and cystine content. J. Nutr. 112 (8), 1525–1535. doi: 10.1093/jn/112.8.1525
Wang Z., Mai K., Xu W., Zhang Y., Liu Y., Ai Q. (2016). Dietary methionine level influences growth and lipid metabolism via GCN2 pathway in cobia (Rachycentron canadum). Aquaculture 454, 148–156. doi: 10.1016/j.aquaculture.2015.12.019
Wang K., Mao W., Zhang X., Zhao Y., Fan K., Pan D., et al. (2020). Neuroanatomy of melanocortin-4 receptor pathway in the mouse brain. Open Life Sci. 15 (1), 580–587. doi: 10.1515/biol-2020-0063
Wang W., Yang P., He C., Chi S., Li S., Mai K., et al. (2021). Effects of dietary methionine on growth performance and metabolism through modulating nutrient-related pathways in largemouth bass (Micropterus salmoides). Aquacul. Rep. 20, 100642–100642. doi: 10.1016/j.aqrep.2021.100642
Wei R. B., Yuan D. Y., Wang T., Zhou C. W., Lin F. J., Chen H., et al. (2013). Characterization, tissue distribution and regulation of agouti-related protein (AgRP) in a cyprinid fish (Schizothorax prenanti). Gene 527 (1), 193–200. doi: 10.1016/j.gene.2013.06.003
White S. L., Volkoff H., Devlin R. H. (2016). Regulation of feeding behavior and food intake by appetite-regulating peptides in wild-type and growth hormone-transgenic coho salmon. Horm. Behav. 84, 18–28. doi: 10.1016/j.yhbeh.2016.04.005
Yúfera M., Nguyen M. V., Navarro-Guillén C., Moyano F. J., Jordal A. E. O., Espe M., et al. (2019). Effect of increased rearing temperature on digestive function in cobia early juvenile. Comp. Biochem. Physiol. -Part A Mol. Integr. Physiol. 230 (September 2018), 71–80. doi: 10.1016/j.cbpa.2019.01.007
Zhang N. (2018). Role of methionine on epigenetic modification of DNA methylation and gene expression in animals. Anim. Nutr. 4 (1), 11–16. doi: 10.1016/j.aninu.2017.08.009
Zhou Y., Liang X.-F., Yuan X., Li J., He Y., Fang L., et al. (2013). Neuropeptide y stimulates food intake and regulates metabolism in grass carp, Ctenopharyngodon idellus. Aquaculture 380-383, 52–61. doi: 10.1016/j.aquaculture.2012.11.033
Keywords: cobia, appetite, feed intake, neuropeptide, gene expression, elevated temperature, dietary methionine
Citation: Nguyen MV, Pham LP, Jordal A-EO, Espe M, Conceição LEC, Yúfera M, Engrola S, Le MH and Rønnestad I (2023) Elevated sea temperature combined with dietary methionine levels affect feed intake and appetite-related neuropeptide expression in the brains of juvenile cobia (Rachycentron canadum). Front. Mar. Sci. 10:1183967. doi: 10.3389/fmars.2023.1183967
Received: 10 March 2023; Accepted: 16 June 2023;
Published: 04 July 2023.
Edited by:
Marta Conde-Sieira, University of Vigo, SpainReviewed by:
Tianming Wang, Zhejiang Ocean University, ChinaXiaofang Liang, Chinese Academy of Agricultural Sciences, China
Copyright © 2023 Nguyen, Pham, Jordal, Espe, Conceição, Yúfera, Engrola, Le and Rønnestad. This is an open-access article distributed under the terms of the Creative Commons Attribution License (CC BY). The use, distribution or reproduction in other forums is permitted, provided the original author(s) and the copyright owner(s) are credited and that the original publication in this journal is cited, in accordance with accepted academic practice. No use, distribution or reproduction is permitted which does not comply with these terms.
*Correspondence: Minh V. Nguyen, bWluaG5ndXllbkBudHUuZWR1LnZu; I. Rønnestad, aXZhci5yb25uZXN0YWRAdWliLm5v
†These authors have contributed equally to this work and share first authorship