- 1Department of Life Sciences, University of Trieste Oceanography, Trieste, Italy
- 2Oceanography, National Institute of Oceanography and Applied Geophysics - OGS, Trieste, Italy
- 3Department of Environmental Sciences, Informatics and Statics, Ca’Foscari, University of Venice, Venice, Italy
In the Mediterranean, Cystoseira sensu lato (s.l.) (Phaeophyceae) forests have sharply declined and restoration measures are needed to compensate for the loss. Assisted regeneration through the outplanting of seedlings grown ex-situ has proven to be a sustainable option. Optimizing mesocosm culture can maximize survival of the most critical embryonic stages and reduce long-term maintenance costs. Host-microbiome interactions could also play a crucial role in seedling development and welfare. In this context, we aimed to advance a cultivation protocol that stimulates the growth and fitness of Ericaria amentacea (Phaeophyceae) seedlings and identify the associated microbial biofilm communities. Seedlings were cultured in 6 treatments [i.e., filtered seawater (SW, C, Control), von Stoch-enriched SW (VS), VS + algal extract (VSA), algal extract-enriched SW: A1< A2< A3]. After the field, A2 seedlings had the highest cover (1372 ± 53.66 mm2), which was 1.8 and 1.9 times greater than in VS and VSA, respectively. The addition of the algal extract and nutrients significantly affected the structure and composition of the microbial community that shifted over time in each culture medium. We identified a treatment-specific microbial fingerprint. After the mesocosm phase, A2 was characterized by 4 unique taxa: Postechiella, Winogradskyella, Roseovarius and Arenibacter (Bacteria). Given the success of A2 seedlings, we propose the probiotic consortia candidates characterized by the unique treatment-taxa in conjunction with the shared taxon Psychroserpens (Bacteria, present in A1, A2, VSA, VS) and the reminder community. Within the holobiont concept, the effect of algal extract or nutrients on the algae and/or biofilm could have important consequences for tuning the overall interaction networks. Our study has shown that macroalgal restoration could benefit from both the use of commercial algal extract and tailored nutrient enrichment in ex-situ cultures and the identification of probiotic consortia candidates that promote seedling growth.
1 Introduction
In the Mediterranean Sea, Cystoseira sensu lato (s.l.) (Phaeophyceae) form extensive forests, which are among the most productive habitats in the coastal zone (Fabbrizzi et al., 2020). In recent decades, Cystoseira s.l. canopies have declined significantly in many coastal regions and are now critically threatened (e.g. Mangialajo et al., 2008; Falace et al., 2010; Vergés et al., 2014; Thibaut et al., 2015; Blanfuné et al., 2016; Valdazo et al., 2017), due to multiple stressors such as overgrazing, eutrophication, habitat fragmentation and climate change (e.g Thibaut et al., 2005; Mangialajo et al., 2008; Thibaut et al., 2015; Blanfuné et al., 2016; Mancuso et al., 2018; Bevilacqua et al., 2019; Falace et al., 2021). There is little evidence of natural recovery of damaged populations, hampered by low zygote and embryo dispersal (Clayton, 1990; Buonomo et al., 2017), so proactive actions are needed to support the regeneration of such populations. Restoration is increasingly recognized as useful tool to trigger the recovery of degraded coastal habitats (Abelson et al., 2020), as stated by the UN Decade of Ecosystem Restoration (2021-2030; https://www.decadeonrestoration.org/). Several techniques have been advanced for the restoration of Cystoseira s.l.: transplantation of adult thalli (Falace et al., 2006; Susini et al., 2007), in situ deployment of receptacles (Verdura et al., 2018; Medrano et al., 2020) and outplantation of seedlings grown ex situ (Falace et al., 2006; Sales et al., 2011; Falace et al., 2018a; Verdura et al., 2018; De La Fuente et al., 2019; Orlando-Bonaca et al., 2021; Savonitto et al., 2021; Lardi et al., 2022; Orlando-Bonaca et al., 2022). The latter two techniques are preferable to avoid further depletion of donor populations (De La Fuente et al., 2019).
Optimizing growth conditions can maximize zygote settlement and survival of the most critical embryonic stages and reduce the cost of long-term maintenance (Savonitto et al., 2021; Clausing et al., 2022; Orlando-Bonaca et al., 2022). Nevertheless, overgrowth of bacteria, epiphytic diatoms and filamentous algae could strongly limit growth and development of zygotes (Orlando-Bonaca et al., 2021; Clausing et al., 2022; Lardi et al., 2022). To overcome these issues, commercially manufactured extracts of a variety of seaweeds have recently been used to reduce epiphyte attachment (Jiksing et al., 2022) and as biostimulants to increase survival, growth and stress tolerance of macroalgae, including brown algae (Hurtado and Critchley, 2018; Umanzor et al., 2019; Hurtado and Critchley, 2020; Umanzor et al., 2020a; Umanzor et al., 2020b; Ali et al., 2021; Han et al., 2022; Jiksing et al., 2022; Umanzor et al., 2022). Macroalgae-derived biostimulants are also used as a sustainable option to improve agronomic production, plant growth and health (Crouch and Van Staden, 1993; Battacharyya et al., 2015; Hurtado and Critchley, 2020; Samuels et al., 2022), thanks to their broad spectrum of constituents (i.e., macro- and microelements, amino acids, hormones, phenolic compounds and saccharides) (Khan et al., 2009; Ali et al., 2021; Sujeeth et al., 2022). Biostimulants are defined as substances and materials, excluding pesticides and nutrients, that can modify the physiological processes of plants improving their growth, development and/or responses to stress (du Jardin, 2012; Rouphael and Colla, 2020). In particular, they promote natural processes for efficient nutrient uptake and utilization, chlorophyll content and photosynthesis, stress resistance, root development and also trigger early flowering and seed germination (Blunden and Wildgoose, 1977; Crouch and Van Staden, 1993; Van Oosten et al., 2017; Ali et al., 2021; Shukla et al., 2021; Sujeeth et al., 2022).
The interactions between host and microbiome can also play a crucial role in development and welfare in the early life stage, as has been shown for corals and sponges (Taylor et al., 2007; Cleary et al., 2022; Peixoto et al., 2022; Thatcher et al., 2022). In the marine field, microbe-assisted crop improvement is still in its infancy compared to agriculture, where plants benefit from mixtures of diverse microbes to protect them from pests or pathogens, improve nutrient supply and directly promote growth through microbial antagonistic interactions (Berg et al., 2021). A growing body of literature (reviewed in Florez et al., 2017 and Duarte et al., 2018) on microbe-algae highlights the paramount importance of the tiny unseen majority in morphological development (Matsuo et al., 2003; Grueneberg et al., 2016), antimicrobial activity and control of microbial colonization via quorum sensing (Manefield et al., 1999; Holmström et al., 2002; Rao et al., 2005; Wang et al., 2009) and algal status under healthy and stressful conditions across the lifespan (Ivanova et al., 2002; Mancuso et al., 2016; Minich et al., 2018; Juhmani et al., 2020). The microbial biofilm forms an outer layer over the host epidermis that plays a functional role between the host and the environment, and is almost considered a new and functionally different “tissue” (Steinberg et al., 2011). The microbes living on the algal surface have an abundance of 106 to 109 bacteria per cm2 and a high phylogenetic diversity (Martin et al., 2014), and differ from the surrounding seawater community (Bengtsson et al., 2012; Michelou et al., 2013; Florez et al., 2017). The algae provide a rich substrate for the microorganisms to thrive, and antagonistic relationships can strongly shape the community alongside algal physiology. As the macroalgal surface is highly dynamic, the functioning of the microbial interface intimately depends on the complex relationships between the host and all-associated organisms (Trevathan-Tackett et al., 2019; Dittami et al., 2021). Hence it is useful to view it as a network of interactions forming a functional synergistic unit called a holobiont (Zilber-Rosenberg and Rosenberg, 2008). The associated microbes play a crucial role in the biology, ecology, and well-being of their hosts, and therefore altering these associations can lead to a pathological state within the holobiont (Egan and Gardiner, 2016; Pitlik and Koren, 2017; Sullivan et al., 2018; Longford et al., 2019; van der Loos et al., 2019).
In the context of ex-situ restoration, it may therefore be relevant to investigate the microbial biofilm associated with the algae in mesocosm cultures to identify possibly probiotic communities with beneficial effects on the algal fitness once they are outplanted in the field. As the application of algal-derived biostimulants in ecological restoration also needs to be explored (Hurtado and Critchley, 2020; Jiksing et al., 2022), in the present study, we used a commercial extract derived from Macrocystis pyrifera (Linnaeus) C. Agardh (Laminariales, Ochrophyta) as an additive to culture media for the cultivation of Ericaria amentacea (C.Agardh) Molinari & Guiry (Fucales, Ochrophyta) seedlings.
Within this framework, the aims of this study were: (i) to investigate the effects of different culture media on the survival, growth and photosynthetic efficiency of E. amentacea seedlings both in culture and in the field, (ii) to identify the microbial biofilm associated with E. amentacea seedlings during an ex-situ experiment and (iii) to create conditions to promote potentially beneficial microbes by using a seaweed extract.
2 Materials and methods
2.1 Fieldwork
Fertile apices of E. amentacea were collected in July 2021 at Bogliasco in the Ligurian Sea (NW Italy, 44°22’31.4” N, 9°04’35.2” E) and transported to the facilities of the University of Trieste in the dark and at 4°C.
2.2 Effects of culture media on seedling survival and growth
2.2.1 Culture in mesocosms
Apices were rinsed with filtered seawater, carefully cleared of epiphytes and stored in the dark at 4°C for 24 hours to promote gamete release.
Six culture media were tested: i) autoclaved filtered seawater (SW) (0.22 µm Durapore membrane philtre, Merck millipore Ltd) as control (C); ii) SW enriched with Von Stosch’s solution (VS) (Von Stosch, 1963; Guiry and Cunningham, 1984; Falace et al., 2018a); iii) SW enriched with the commercial macroalgal extract AlgatronCifo® [Cifo S.p.A., San Giorgio di Piano, Bologna, Italy] (here after Algatron) at three concentrations (i.e., A1 = 2 mL L-1; A2 = 4.5 mL L-1; A3 = 9 mL L-1). The concentrations of the algal extract for solutions A2 and A3 correspond to the manufacturer’s recommendations for foliar and soil application, respectively, while concentration A1 was chosen to test whether E. amentacea could benefit from a lower concentration; iv) VS solution containing 4.5 mL L-1 Algatron as in A2 (VSA). Algatron contains N, P, alginic acid, mannitol, other carbohydrates, polyphenols and essential amino acids (Prisa, 2021). Information on the culture media and N:P ratio are reported in Supplementary Table 1.
To test the effects of the different culture media on the microbial biofilm communities associated with the seedlings and the overall performance of the culture, we set up tanks containing 2 L of culture medium (n= 3 tanks per treatment), and 11 clay tiles (4.5 cm diameter with a central hole of 0.8 cm diameter) (Figure 1), each containing four randomly assigned fertile apices. The tiles were sterilized by autoclaving and the tanks were cleaned with 1% HCl. The apices were removed after 24 hours and the tiles left undisturbed for another 24 hours to ensure attachment of the zygotes.
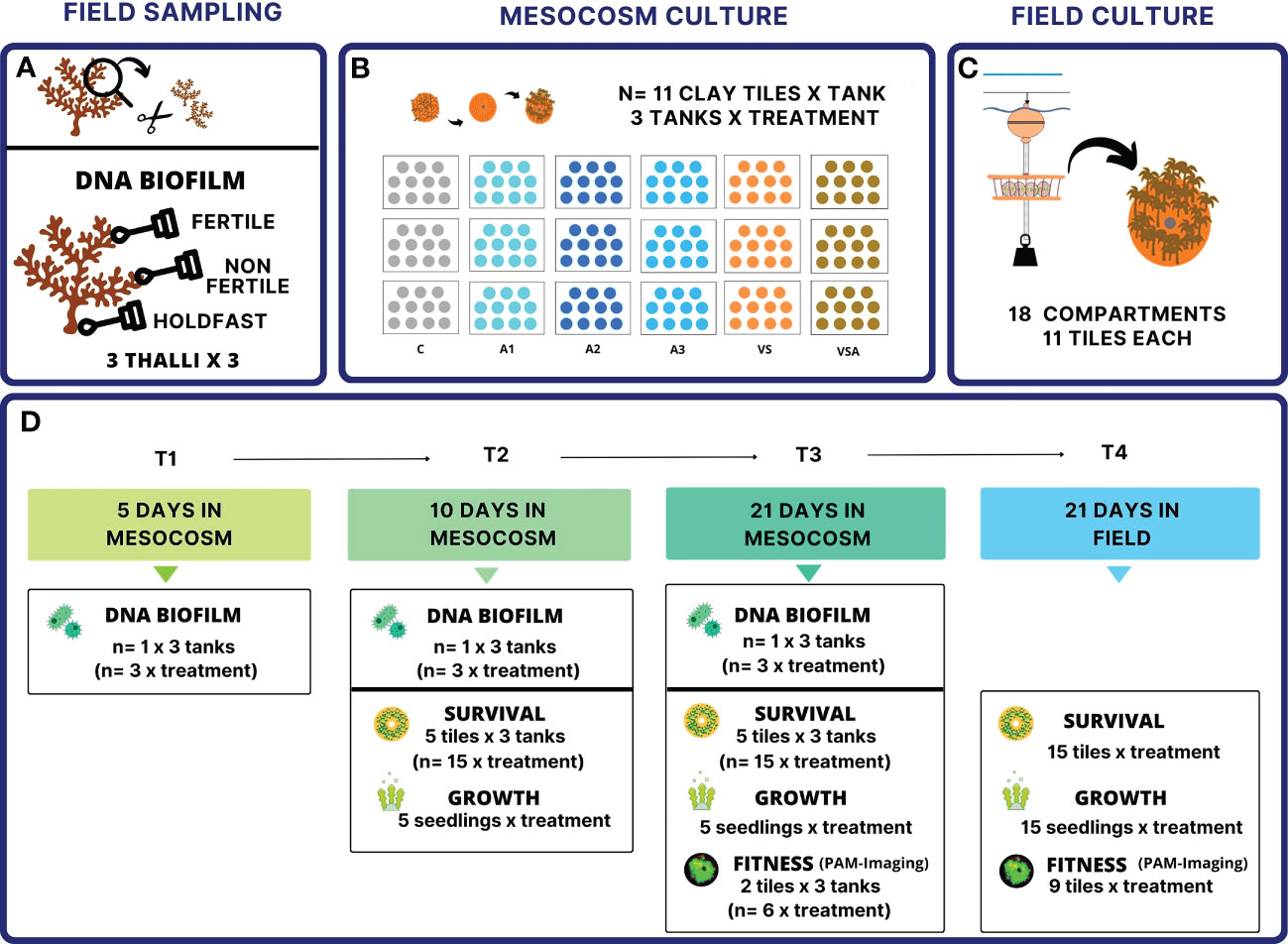
Figure 1 Experimental design. (A) field sampling, (B) mesocosm culture, (C) field culture. Culture media: A1 = 2 mL L-1 Algatron; A2 = 4.5 mL L-1 Algatron; A3 = 9 mL L-1 Algatron; C= filtered seawater; VS, SW enriched with Von Stosch’s solution; VSA, VS solution with 4.5 mL L-1 Algatron. (D). Summary of collected data. The days (T) are counted from the release of the zygotes (Supplementary Table 1). the tiles from the same tank are considered pseudo-replicates.
Seedlings were grown for three weeks in environmentally controlled rooms under optimal conditions as previously determined by Falace et al. (2018a). The temperature was 20°C, the light intensity 125 μmol photons m-2 s-1 (LED lamps AM366 Sicce USA Inc., Knoxville, USA) with 15:9 h light:dark photoperiod. Air temperature was automatically set by the room controller and water temperature was measured daily with a thermometer in each aquarium to ensure it was constant. The culture medium was changed every four days to avoid nutrient depletion and the tanks were cleaned accordingly with 1% HCl. The tanks were oxygenated using air pumps and bubblers. Treatment aquaria as well as tiles within the aquaria were randomly repositioned each time the medium was changed to control for positional variations in light and temperature intensity.
The following data were collected (Figure 1):
- Seedling survival (i.e., tile area covered by seedlings, mm2) was measured on day 10 (T2) and 21 (T3) post fertilization (PF). The area was estimated from images (Pentax k5 MarkII, Pentax, Tokyo, Japan). At each time point, n= 5 tiles per tank (n= 15 per treatment) were randomly selected for measurements. Images were processed using ImageJ software (Schneider et al., 2012).
- Seedling growth (i.e., length, mm) was measured at T2 and T3 with an inverted microscope (Leica, DM IL LED) and photographed with a Canon Powershot G9. At each time point, n= 5 seedlings per treatment were randomly selected from the tiles in the tanks. The limited replication for this variable was necessary because the measure implies destructive sampling.
- Seedling fitness (i.e., Fv/Fm); in vivo chlorophyll-a fluorescence (ChlaF) of the photosystem II (PSII) was measured at T3 on 2 tiles per tank (n= 6 per treatment) using the PAM -Imaging Fluorometer Open FluorCam (Photon Systems Instruments, Brno, Czech Republic). Shutter time and sensitivity of the charge-coupled device (CCD) camera were set to 1 and 10, respectively. Each tile was placed at a constant distance of 17.5 cm below the camera objective, and the lamps were placed at an angle of 45° to the center of the measurement area. Prior to measurements, the tiles with seedlings were dark adapted for 20 min to allow complete oxidation of the PSII reaction centers prior to measurements, then the basal fluorescence (F0) was measured (Krause and Weis, 1984). Then a saturating pulse of actinic light (4040 µmol m-2 s-1, 0.8 s) was administered to induce maximum fluorescence (Fm). The maximum quantum yield of PSII was calculated as follows: Fv/Fm= [Fm - F0]/Fm (Maxwell and Johnson, 2000).
2.2.2 Cultivation in the field
After cultivation in the mesocosms, all the tiles were transferred outdoors for the next phase of cultivation in the sea. The tiles were attached to a hanging structure in a way that they could surface regularly, as the species naturally does in the intertidal. The hanging structure consisted of 18 contiguous compartments; each set of 11 tiles (i.e., one set for each tank of each treatment) was labeled and randomly assigned to one of the 18 compartments.
After 21 days (T4), the tiles were returned to the laboratory and measured as in the first phase (Figure 1):
- Seedling survival was estimated from images for which 5 tiles per compartment (n= 15 per treatment) had been randomly selected.
- Seedling growth was measured from 5 randomly selected seedlings per compartment (n= 15 per treatment).
- Seedling fitness was measured on 3 randomly selected tiles per compartment (n= 9 per treatment).
2.2.3 Statistical analyses
Analysis of Variance (ANOVA, R Core Team, 2022) was performed to test the effects of culture medium and exposure time on seedling survival and growth (i.e., cover, length) and fitness (i.e., Fv/Fm). For cover, the design for the analysis consisted of the two factors Time (Ti, fixed, 2 levels), and Medium (Me, fixed, 6 levels, crossed), with n=3. For Fv/Fm, the design included only factor Me (as this variable was measured at the end of the lab experiment, at T3), with n=3. For these two variables, the average values measured in the three tanks were used as independent replicates for each treatment and each time (Millar and Anderson, 2004). For seedling length, measurements were made on a total of n=5 seedlings across tanks to avoid excessive tile manipulation (see above). In this case, the design for ANOVA included the factors Ti and Me, with n=5 seedlings randomly selected across all tanks for each treatment (i.e., medium culture) and sampling time.
For the field experiment, ANOVA was used to test for differences among treatments on seedling survival, growth and welfare. In this case, the design for the analysis included only factor Me with n=3 replicates per each level of the factors. Analogously to the analysis of laboratory data, the average values measured in the three sections corresponding to the original laboratory tanks were used as independent replicates for each level of factor Me.
The assumption of normality of response variables was tested using the Shapiro-Wilk test, while Cochran’s C-test (Underwood, 1997) was used to test the assumption of homogeneity of variances prior to analysis. For all response variables, since non-normality and/or variance heterogeneity remained after data transformation, untransformed data were analyzed with a permutational multivariate analysis of variance (PERMANOVA, Anderson, 2001) based on Euclidean distance with 5,000 permutations. Significant interaction terms involving the factor Me or its main effect were examined by post hoc pairwise t-tests. PERMANOVA makes no assumptions about the data distribution and is robust to variance heterogeneity in experiments with balanced designs (Anderson, 2014), as in our case.
2.3 Microbiome: DNA extraction, PCR conditions and sequencing
On the day of sampling, microbial biofilms associated with three whole thalli were collected in triplicate by rubbing three areas with sterile cotton swabs: basal holdfast, non-fertile and fertile apices. Thalli that were overgrown with epiphytic algae or animals were avoided. The swabs were immediately placed in sterile 1.5 mL Eppendorf tubes and stored in DNA-RNA shield solution (Zymo Research). Samples were transported at -20°C in the dark and stored at -80°C until DNA extraction.
During the mesocosm experiment, microbial biofilm was sampled in triplicate per treatment with sterile cotton swabs from independent tiles of Ericaria amentacea seedlings at T1 (i.e., 5 days post fertilization, PF), T2 (i.e., 10 days PF) and T3 (i.e., 21 days PF, Figure 1). We followed the microbial abundance in the water compartment over the experiment. Samples were analyzed at the BD FacsCanto cytometer after SYBRGreenTM I staining according to Gasol and Del Giorgio (2000) (Supplementary Data Sheet). It was beyond the scope of this work to investigate the free-living microbial community in the bulk-water during the mesocosm phase. We used the E.Z.N.A Soil DNA Kit (Omega Bio-Tek) to extract DNA from the swabs. Extracted DNA was quantified with a NanoDrop 2000 spectrophotometer (Thermo Fisher Scientific). We targeted the V4 hypervariable region of the 16S rRNA gene with 515F primer (Caporaso et al., 2011), and a mix of 802R (Claesson et al., 2009) and 806R (Caporaso et al., 2011) primers. Primers were tailed with two different GC rich sequences enabling barcoding with a second amplification step. We run three technical replicates for each sample in 20 μL of volume reaction containing 10 μL AccuStartII PCR ToughMix 2X (Quanta Bio), 1 μL EvaGreen™ 20X (Biotium), 0.8 μL 515 F (10 μM— 5’ modified with unitail 1-CAGGACCAGGGTACGGTG-), 0.4 μL 802 R (10 μM— 5’ modified with unitail 2-CGCAGAGAGGCTCCGTG-), 0.4 μL 806 R (10 μM— 5’ modified with initial 2-CGCAGAGAGGCTCCGTG-), and 50 ng of DNA template. We used a CFX 96™ PCR System (Bio-Rad) with a real-time limited number of cycles (94°C for 20 s, 55°C for 20 s, 72°C for 60 s). The second PCR amplification (outer PCR) was required to uniquely label each sample. We used a forward primer composed of the ‘A’ adaptor, a sample-specific 10 bp barcode and the tail 1 of the primary PCR primers, and a reverse primer composed of the P1 adaptor sequence and the tail 2. We followed the protocol of Giglio et al. (2021) for creating the library and setting the sequence reaction with Ion 316 chip (Life Technologies) in the Ion Torrent PGM System.
2.3.1 Microbiome bioinformatics pipeline and analyses
The CLC Microbial Genomics Module as part of CLC Genomics Workbench 20.0 (QIAGEN Digital Insights, Aarhus, Denmark) was used to analyze alpha and beta diversity, and bacterial community composition. Raw sequencing reads were imported into the CLC environment, and we performed quality control, primers and adapter sequences removal and minimum size cut-off of 150 bp, chloroplast, mitochondrial, unassigned and Eukarya sequences were removed from the database. Operational Taxonomic Units, OTUs, were picked by mapping sequences against the SILVA 16S v138 97% database (Quast et al., 2013) at the same identity percentage to observe OTU at the species level. We focused on the most abundant top twenty OTUs. We computed the alpha diversity and then performed the Kruskal-Wallis H test to assess statistical significance Results with q-value < 0.05 were considered significant. Alpha diversity, the diversity within the sample (Whittaker, 1972) based on OTUs, was computed among the three adult thalli and seedlings using the Chao 1 index. A principal coordinate analysis (PCoA) of the beta diversity distance matrices was performed. We used the Bray–Curtis distance where we considered the abundance of each OTU balanced with the cladistic information (weighted UniFrac index). We generated a heat-map by clustering the z-score normalized relative abundance OTU data using the Euclidean distance and the average linkage to identify the shared taxa present in adult thalli and in the seedlings. We ran indicator species analysis using the R package indicspecies (De Cáceres and Legendre, 2009) to identify associations among OTUs and treatments with statistical significance. 148 OTUs at the genus level with a relative abundance >1% were selected and uniquely associated with one or more treatment combinations.
3 Results
3.1 Effects of culture media on seedling survival and growth
Seedling survival was significantly affected by the interaction of treatment and time during the mesocosm culture (p-value=0.0036) and by treatment after the field period (p-value=0.0002) (Supplementary Table 2).
At T2, significant differences were observed between the culture media in terms of survival (i.e., tile coverage) (Figure 2A). A2 seedlings had the highest survival (85.31 ± 6.43 mm2), while C and A3 had the lowest survival (28.99 ± 4.40 and 22.43 ± 1.77 mm2, respectively). The differences between treatments increased with time. At T3, the seedlings cultured in seawater (C) and A3 had the lowest survival (5.09 ± 0.90 mm2 and 8.23 ± 1.49 mm2 respectively) (Figure 2A). Seedling survival in A1, A2, VS and VSA was comparable at T3 (Figure 2A), with the highest values for VSA (201.00 ± 30.26 mm2) and A2 (187.00 ± 21.29 mm2).
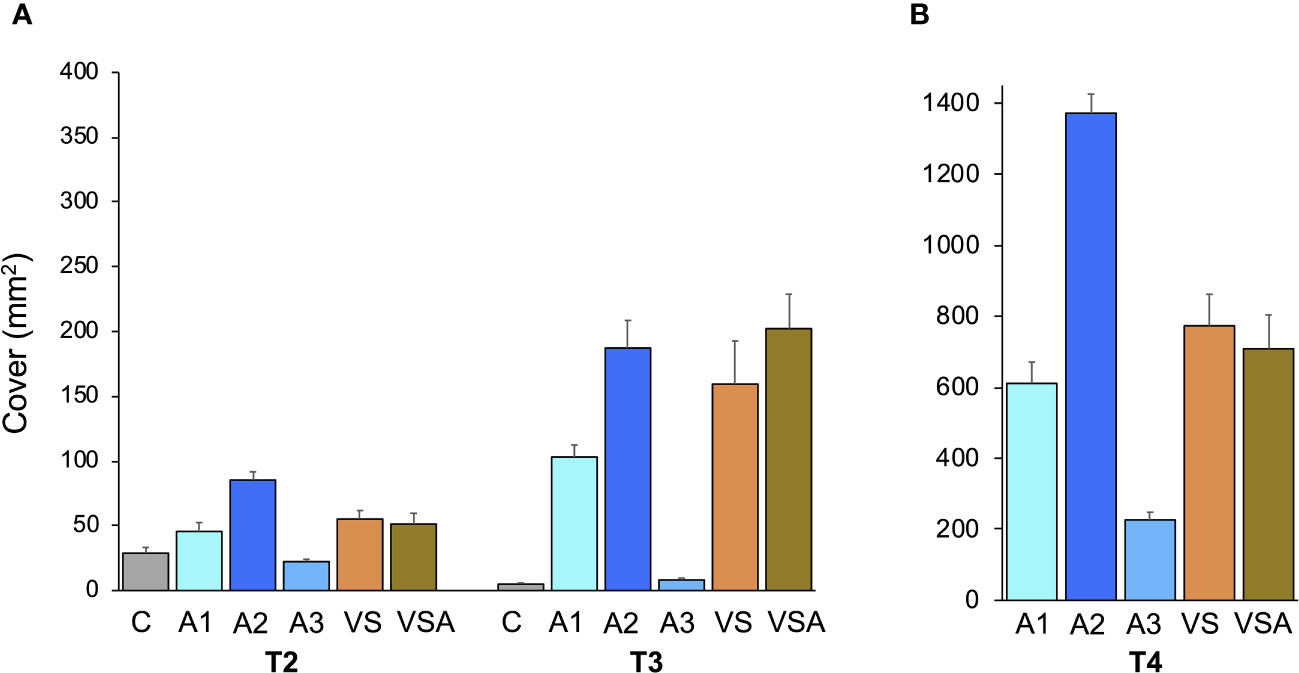
Figure 2 Mean values (± SE) of tile area covered by Ericaria amentacea seedlings: (A) in mesocosm at T2 (10 days post fertilization) and T3 (21 days post fertilization); (B) after 3 weeks in field culture (T4). C (seawater, SW); A1 (SW with Algatron 2 mL L-1); A2 (SW with Algatron 4.5 mL L-1); A3 (SW with Algatron 9 mL L-1); VS (SW with addition of von Stoch); VSA (SW with addition of von Stoch and Algatron 4.5 mL L-1). At T4, no seedlings could be measured for C, as the tiles were free of seedlings.
The lowest growth was observed at T3 for the C condition (length= 0.45 ± 0.03 mm), while it was highest for VSA (length= 2.30 ± 0.21 mm) and VS (length= 2.24 ± 0.15 mm). However, seedlings growing in VSA and VS had the lowest photosynthetic efficiency (i.e., Fv/Fm 0.51 ± 0.02 and 0.46 ± 0.02, respectively) (Figure 3A). In contrast, seedlings cultivated in Algatron (i.e., A1, A2, A3) and in C showed optimal photophysiological fitness (Fv/Fm > 0.69) (Figure 3A). At T3, tiles and seedlings cultivated in VS and VSA were covered by epiphytes, while they were absent in the Algatron and seawater treatments (Figure 4).
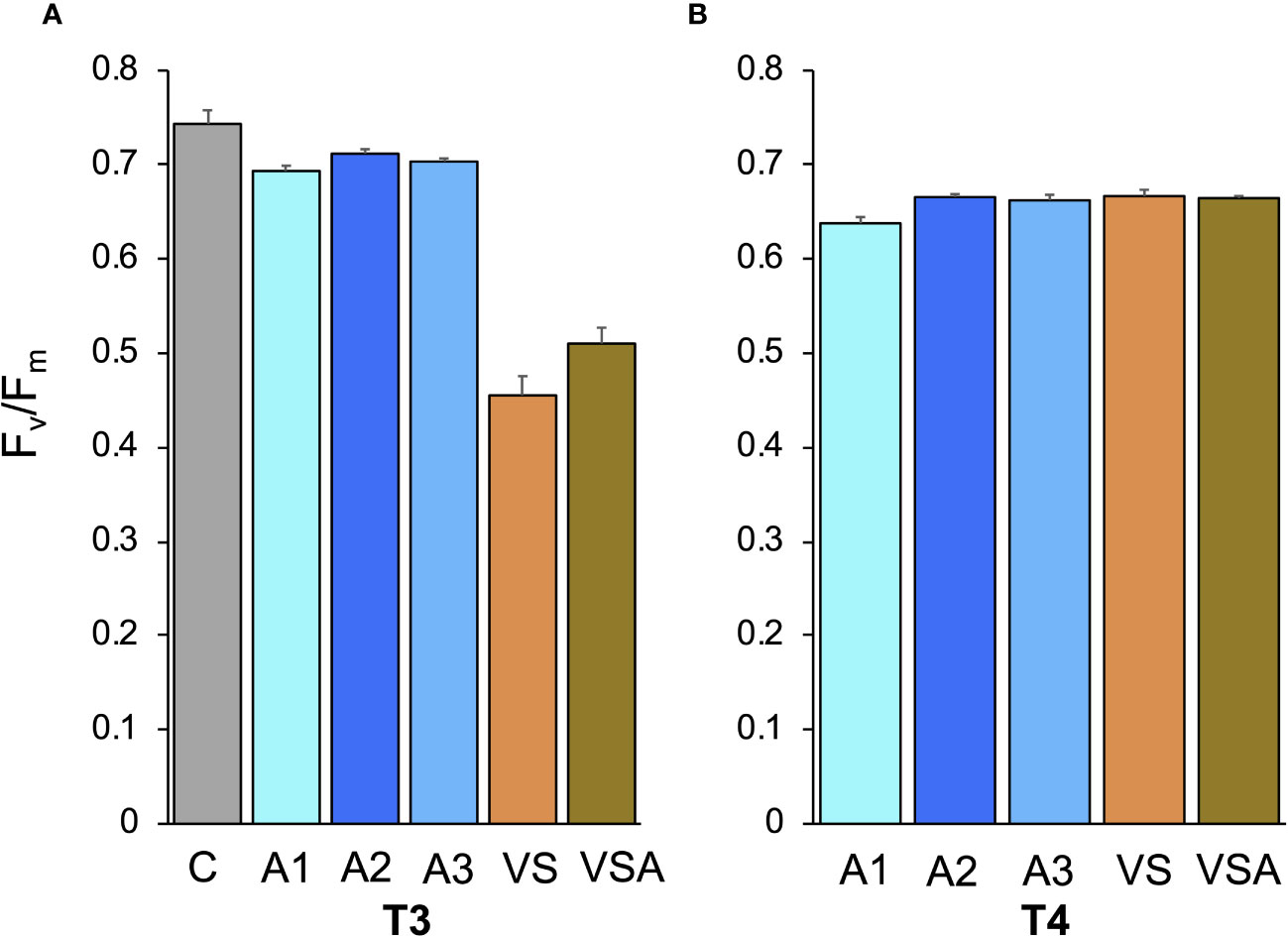
Figure 3 Mean values (± SE) of the maximum quantum yield of PSII (Fv/Fm) of Ericaria amentacea seedlings; (A) at the end of laboratory culture (T3); (B) after 3 weeks of culture in the field (T4). C (seawater, SW); A1 (SW with Algatron 2 mL L-1); A2 (SW with Algatron 4.5 mL L-1); A3 (SW with Algatron 9 mL L-1); VS (SW with addition of von Stoch); VSA (SW with addition of von Stoch and Algatron 4.5 mL L-1). At T4 C-treatment tiles were free of seedlings.
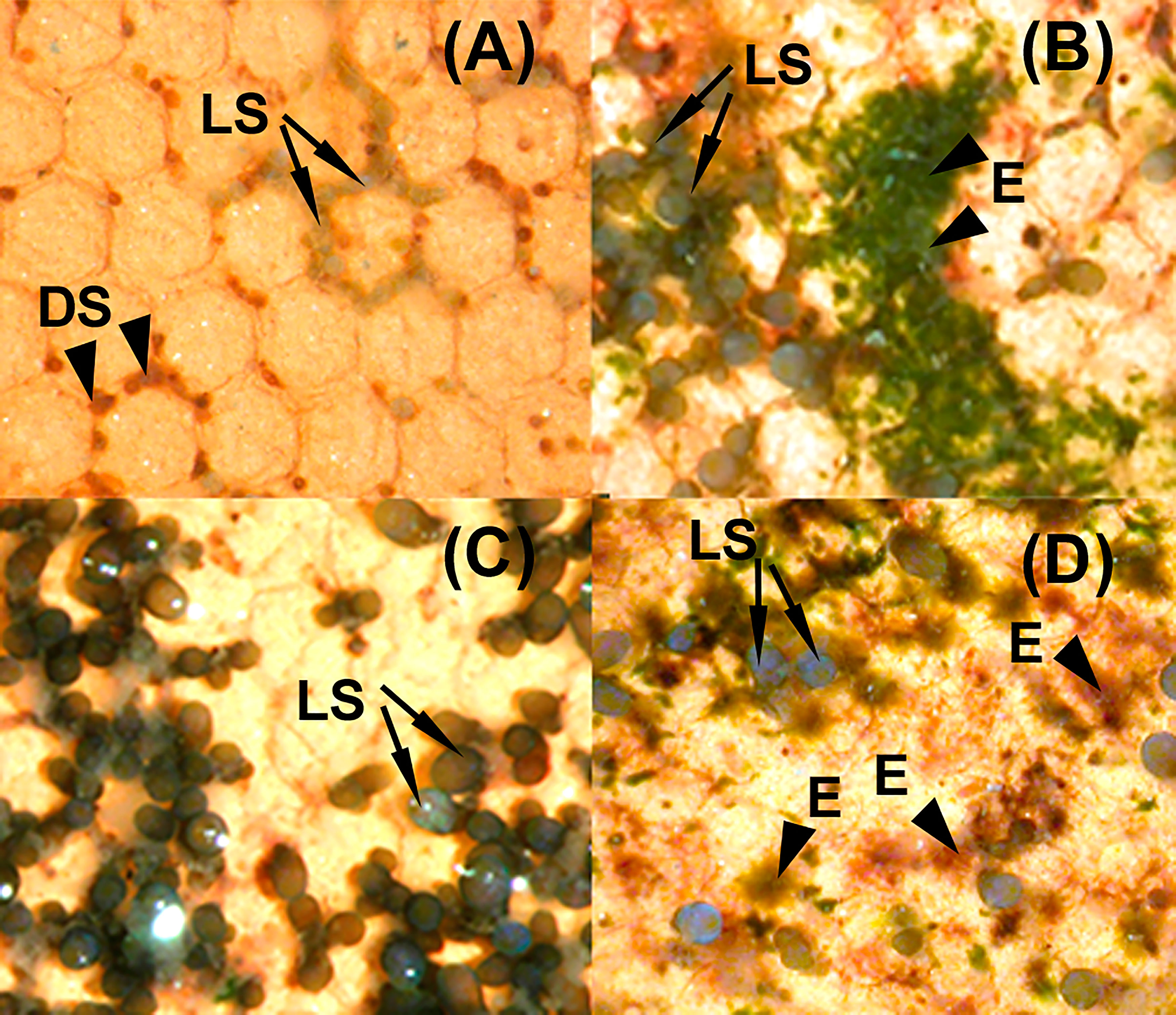
Figure 4 Clay tiles with Ericaria amentacea seedlings at the end of the laboratory culture (T3) under different culture media: (A) C (seawater, SW) with living seedlings (LS, arrows) and dead seedlings (DS, arrowheads). (B) VS (SW with addition of von Stoch) with living seedlings (LS, arrows) and epiphytes (E, arrowheads). (C) A2 (SW with Algatron 4.5 mL L-1) with living seedlings (LS, arrows). (D) VSA (SW with addition of von Stoch and Algatron 4.5 mL L-1) with living seedlings (LS, arrows) and epiphytes (E, arrowheads).
After the seedlings were exposed to the field (T4), there were still significant differences in survival between treatments (Supplementary Table 2). Seedlings cultured in seawater (C) did not survive, while seedlings in A2 had the highest coverage (1372.00 ± 53.66 mm2), which was 1.8 and 1.9 times higher than seedlings from VS and VSA, respectively (Figure 2B).
At T4, seedling length was comparable in all treatments (Supplementary Table 2). Seedlings from A3 showed the lowest growth (length= 3.53 ± 0.17 mm), while the highest growth was recorded in seedlings from VSA (4.69 ± 0.20 mm) and A2 (4.45 ± 0.20 mm).
The Fv/Fm values of all treatments were within the range of values indicating healthy PSII (Figure 3B), although a significant difference was observed between A1 and the other treatments (Supplementary Table 2).
3.2 Microbiome: structure and dynamics
The analysis of the 16S rRNA genes allowed the identification of the most abundant microbial taxa associated with the seedlings and adult thalli of E. amentacea. A grand total of 2373362 sequences were generated and 97.5% were assigned to OTUs. The rarefaction curves showed that the sequencing effort sufficed sample biodiversity (Supplementary Data Sheet). Seedling microbial biofilm replicates were significantly correlated within treatment (p < 0.05). Alpha diversity analysis (Chao 1 index, Figure 5) showed that the whole thalli were more diverse than the seedlings. The alpha diversity index of the microbial biofilm among the different treatments for the total number of families (Figure 6) showed that A2 and A3 were not significantly different, while A2 was different from the other treatments (see p-values in Figure 6), and had the less diverse biofilm communities. Over time, diversity increased in all other treatments.
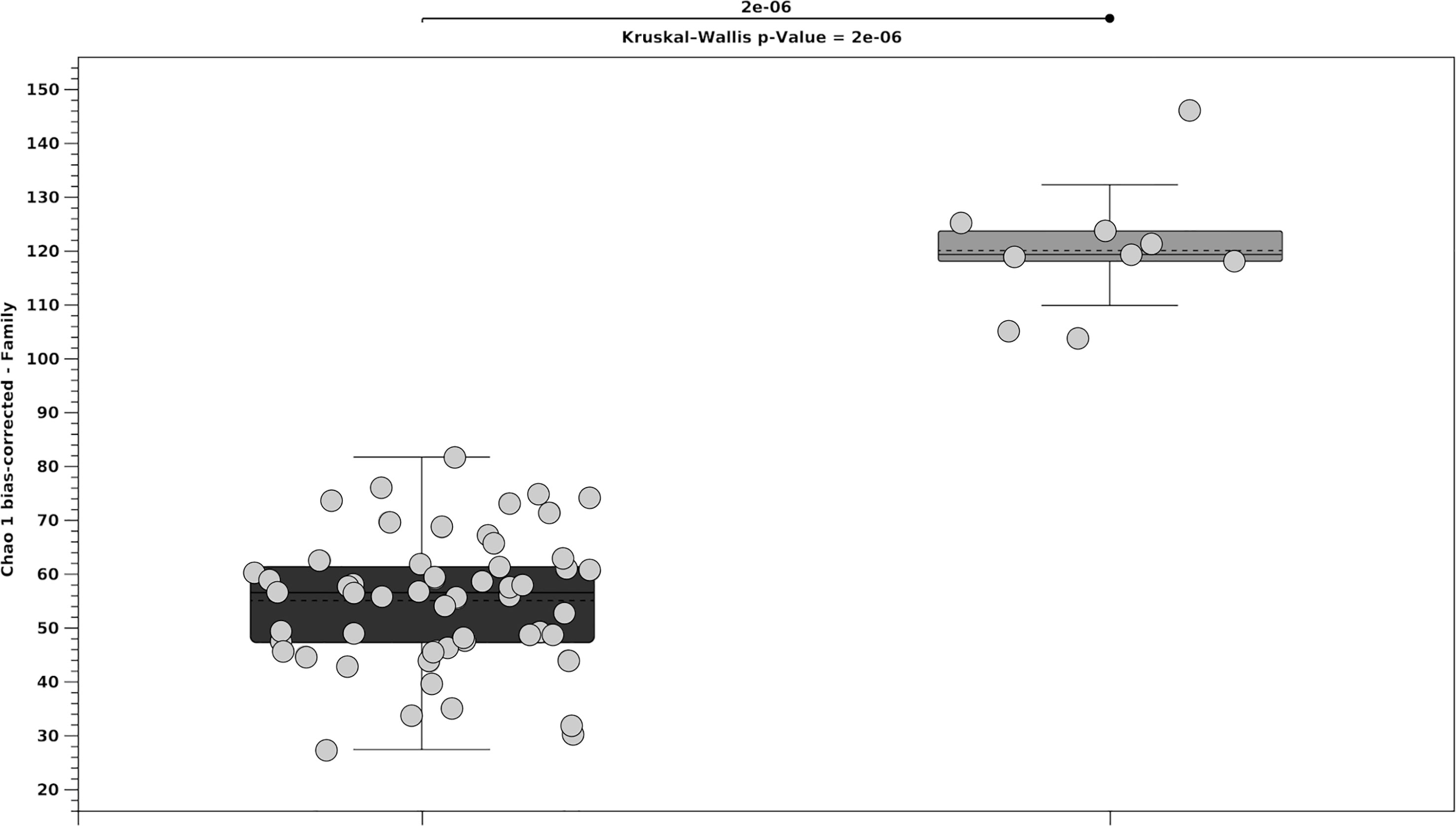
Figure 5 Median value 25th and 75th percentiles of alpha diversity for the microbial biofilms (total number of families) for the total seedlings in black and the three adult whole thalli in gray. Circles indicate treatments and time in seedlings whereas positions (i.e., basal holdfast, fertile and not fertile apices) in adult thalli.
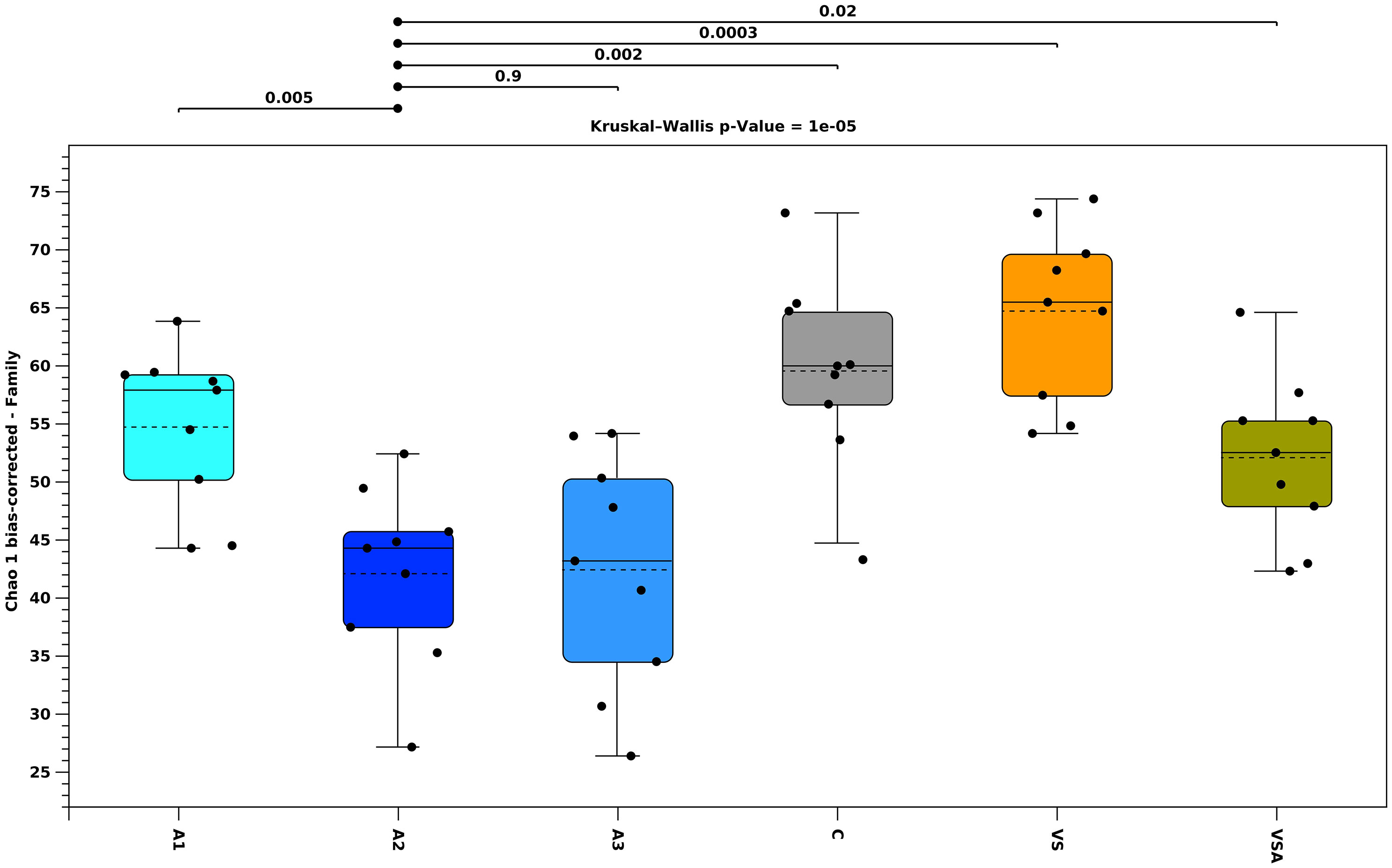
Figure 6 Median value 25th and 75th percentiles of alpha diversity for the microbial biofilm (total number of families), measured in 6 different treatments: Algatron (A1), Algatron (A2), Algatron and (A3), Control (C), von Stosch (VS), von Stosch with Algatron (VSA) for the entire dataset. C (Control, gray), VSA (brown), VS (orange), A1 (baby-blue), A2 (blue), A3 (navy-blue). Circles indicate replicates over time.
Beta diversity showed the differences among microbial communities when comparing adult thalli vs seedlings (Figure 7) and treatment vs treatment (Figure 8). PCoA showed that the adult thalli were separated from the seedlings (Figure 7). PCoA showed that the treatments had their own trajectories over time (Figure 8). At T3, C (i.e., the seawater control) was separate from the others, supporting the hypothesis that the treatments affected beta-diversity. We also noted a gradient, along the PCo2 axis according to Algatron concentration.
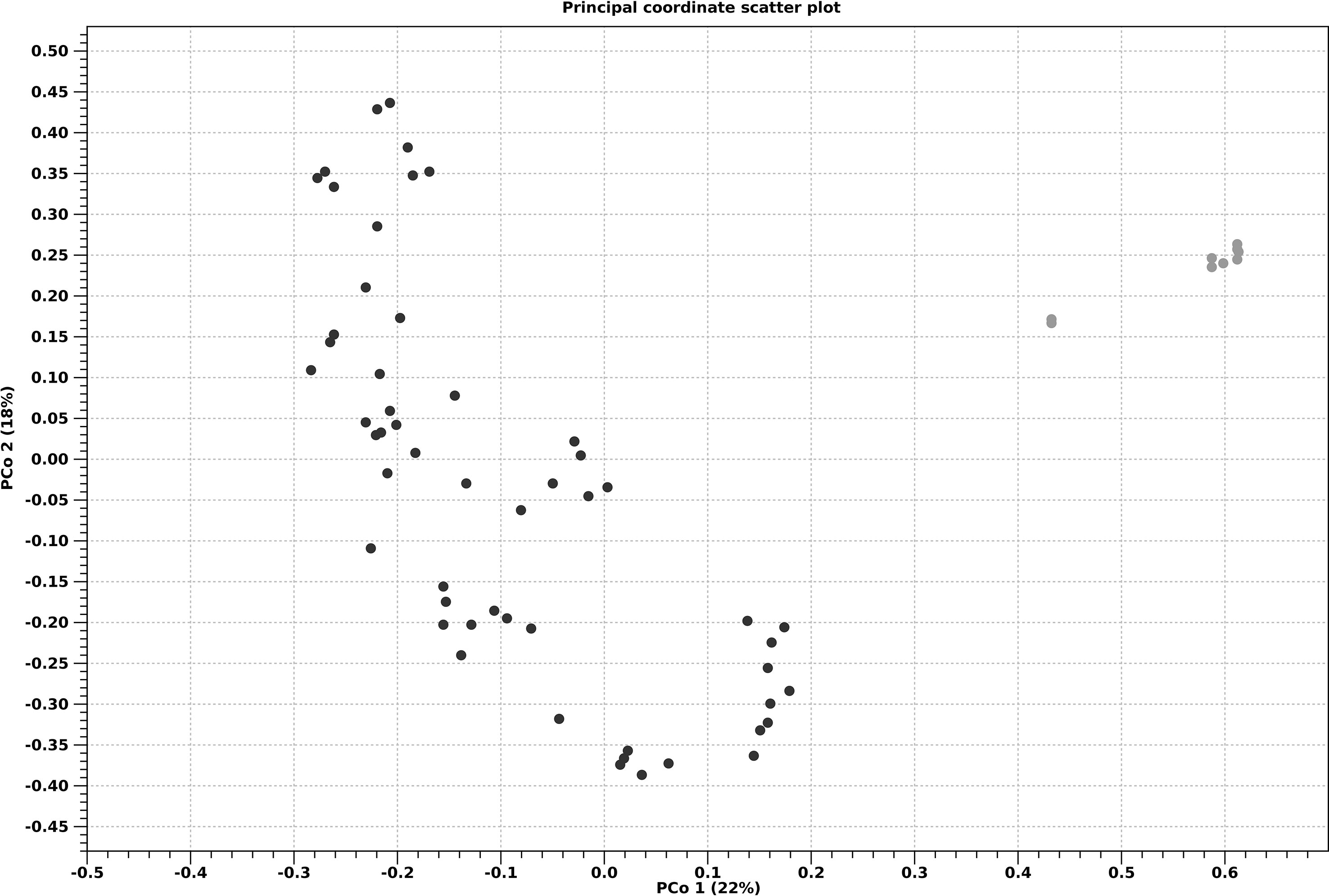
Figure 7 Principal coordinate analysis ordination (PCoA) of a Bray-Curtis dissimilarity matrix for adult thalli (gray) and seedlings (black).
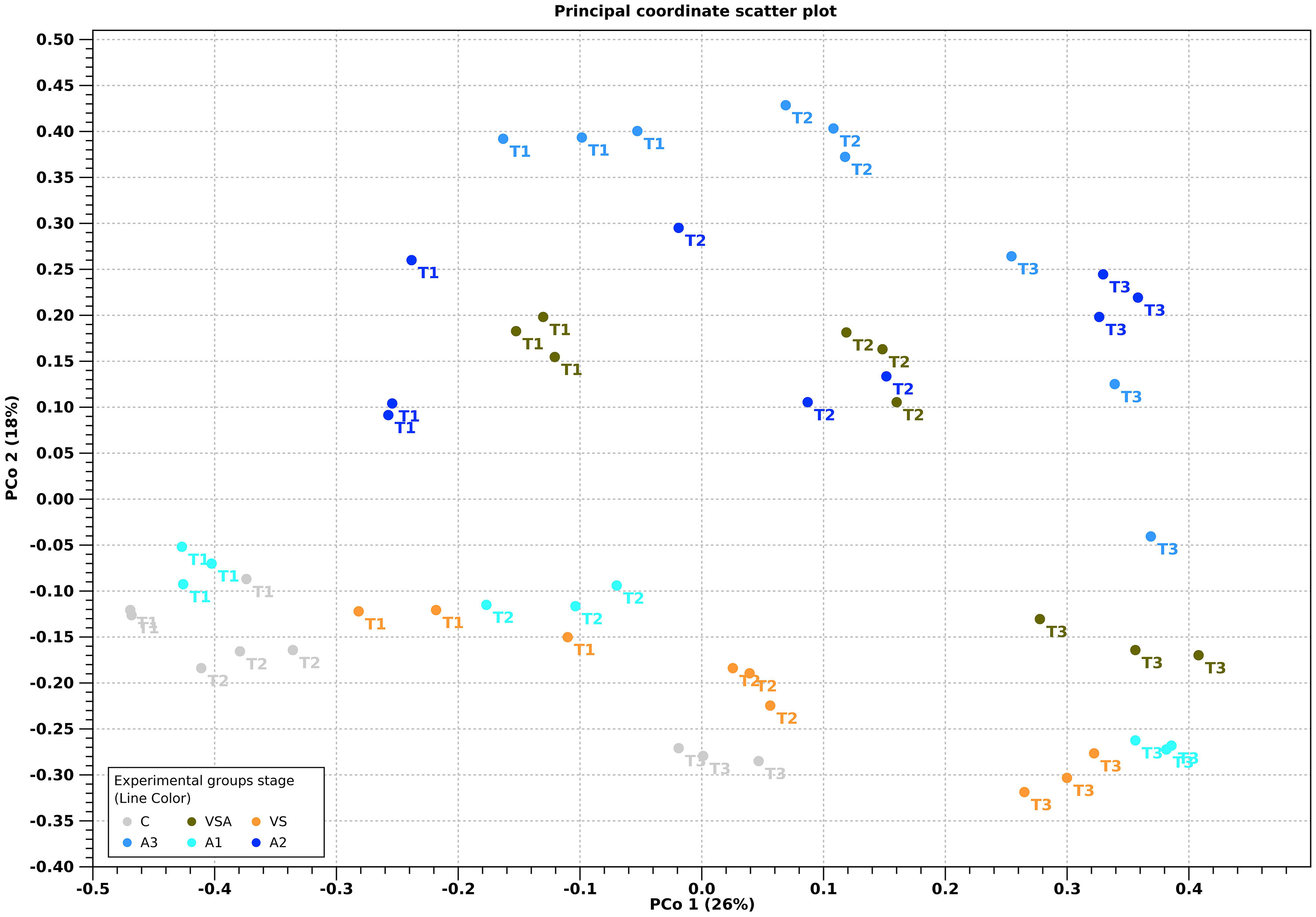
Figure 8 Principal coordinate analysis ordination (PCoA) of a Bray-Curtis dissimilarity matrix for the treatment over time. Treatments are color coded: C (Control, gray), VSA (brown), VS (orange), A1 (baby-blue), A2 (blue), A3 (navy-blue).
Phylogenetic characterization identified 303 families (Bacteria) in the seedling microbial biofilm communities (Figure 9) and only 32 families had at least one OTU scoring >1% of the relative abundance in a treatment. In general, T1 was characterized by the dominance of four families: Rhodobacteraceae, Flavobacteriaceae, Alteromonadaceae and Vibrionaceae. This fingerprint changed at T3, where only the Rhodobacteraceae and Flavobacteriaceae emerged as major groups. The twenty most abundant families account for more than 95% of OTU abundance. The biofilm communities of adult thalli are discussed in SI.
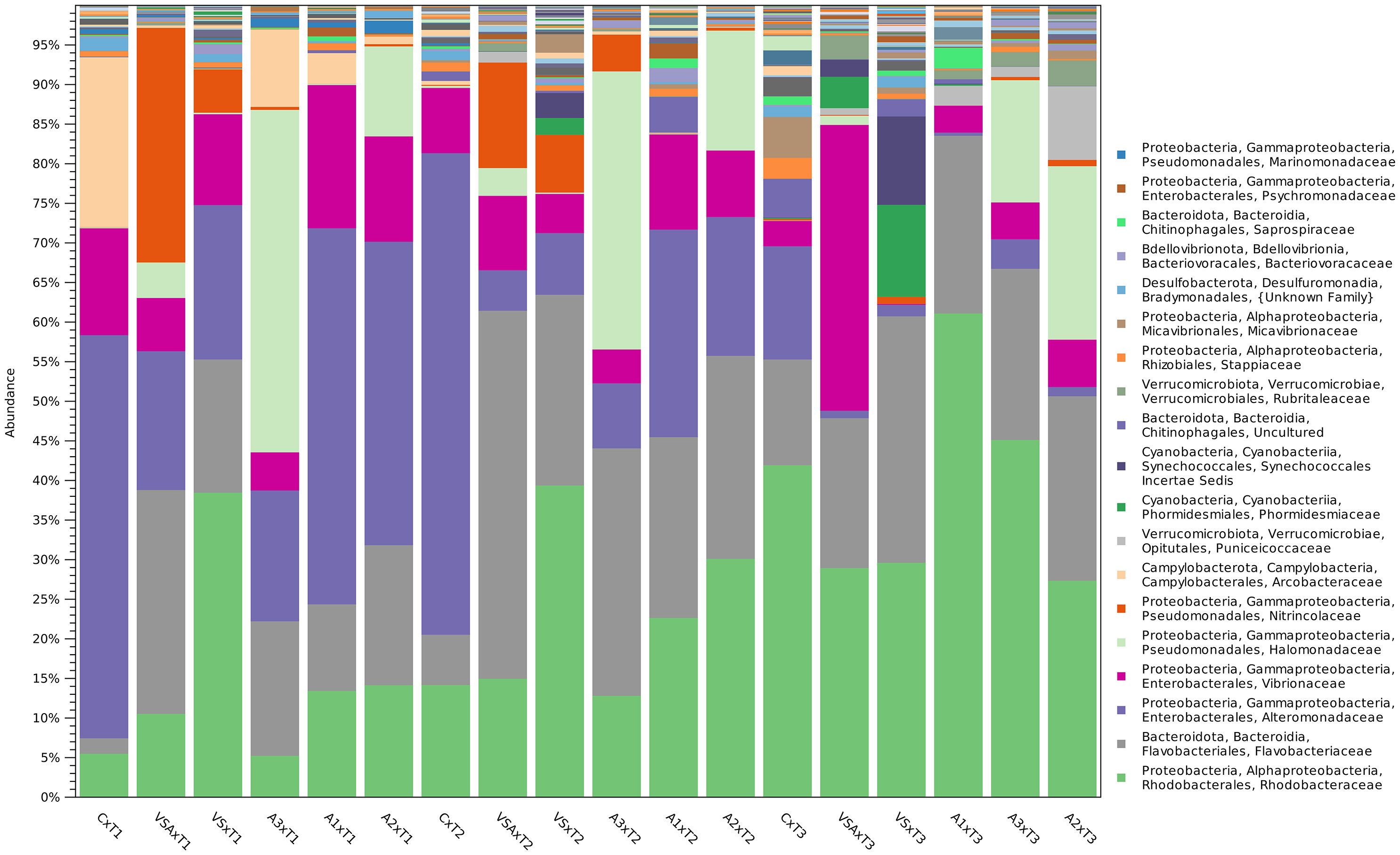
Figure 9 OTU relative abundance (20 most abundant families) aggregated per treatment over time of seedlings microbial biofilm. Bar height is proportional to taxa abundance.
On E. amentacea seedlings, the 20 most dominant families were: Rhodobacteraceae, Flavobacteriaceae, Alteromonadacea, Vibrionaceae, Halomonadaceae, Nitricolaceae, Acrobacteriaceae, Puniceicoccaceae, Phormidesmiaceae (Cyanobacteria), a family belonging to Synechoccales (Cyanobacteria), a family belonging to the Chitinophagales, Rubriraleaceae, Stappiaceae, Micavibrionaceae, a family belonging to the Bradymonadales, Bacteriovoraceae, Saprospiraceae, Psychromonadaceae and Marinomonadaceae (Figure 10).
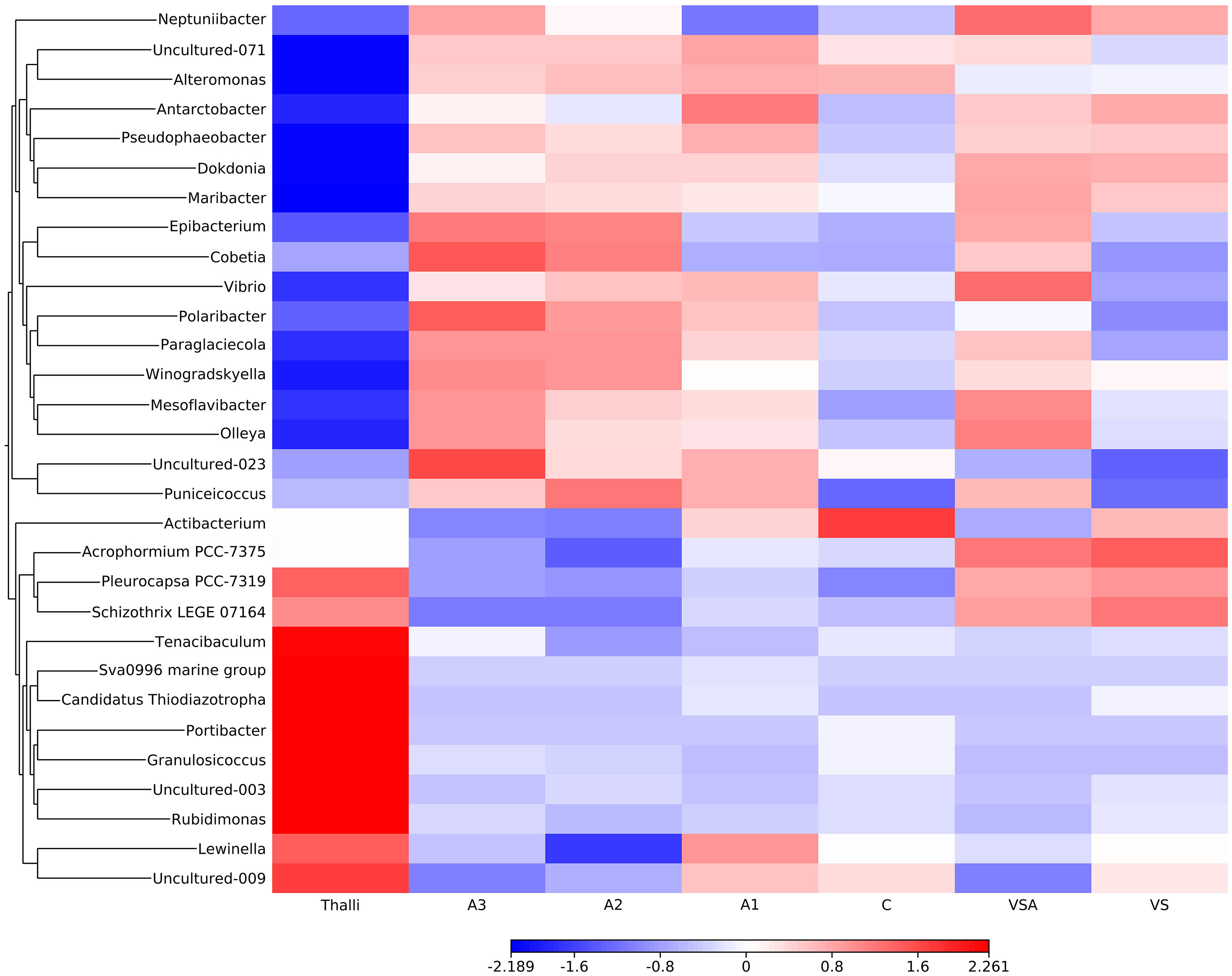
Figure 10 Heat-map of OTU relative abundance in the adult thalli and the seedlings community. Z score bar indicates enrichment (red) or depletion (blue) factor in comparison to the mean OTU value.
In the seawater treatment (C), the microbial biofilm structure was characterized over time by an increase in Rhodobacteraceae (T1-T3: from 5.8% to 39.9%), Flavobacteriaceae (T1-T3: from 1.8% to 12.6%), an uncultured family of Chitinofagales (T1-T3: <1% to 4.7%) and Stappiaceae (T1-T3: from <1% to 2.5%), Micavibrionaceae (T1-T3: from <1% to 5.0%), Saprospirareae (T1-T3: from <1% to >1%), Phycisphaeraceae (T1-T3: from <1% to 2.2%), Nisaeceacea, Rhizobiaceae and Talassobaculaceae (T1-T3: from <1% to >1%). Over time, the microbial biofilm structure in C was characterized by the decrease of Alteromonadaceae (T1-T3: from 47.0% to 13.0%), Vibrionaceae (T1-T3: from 12.6% to 3%), Acrobacteraceae (T1-T3: from 19.8% to < 1%).
In VS, the microbial biofilm structure was characterized over time by the increase in Flavobacteriaceae (T1-T3: from 16.0% to 30.4%), Phormidesmiaceae (Cyanobacteria, T1-T3: from <1% to 11.4%), an uncultured family of Synechococcales (Cyanobacteria, T1-T3: from <1% to 11.0%), an uncultured family of Chitinofagales (T1-T3: from <1% to 2.1%), Phycisphaeraceae (T1-T3: from <1% to >1%). Furthermore, the microbial biofilm structure in VS was characterized over time by the decrease in Rhodobacteraceae (T1-T3: from 36.6% to 29.1%), Alteromonadaceae (T1-T3: from 18.6% to 1.4%), Vibrionaceae (T1-T3: from 11.0% to < 1%), Nitricolaceae (T1-T3: from 5.2% to < 1%).
In VSA, the microbial biofilm structure was characterized over time by an increase in Rhodobacteraceae (T1-T3: from 10.5% to 28.9%), Vibrionaceae (T1-T3: from 6.7% to 36.1%), an uncultured family of Synechococcales (Cyanobacteria, T1-T3: from <1% to 2.1%), Rubritaleaceae (T1-T3: from <1% to 3.0%). Furthermore, the VSA microbial biofilm structure was characterized by a decrease of Flavobacteriaceae (T1-T3: from 27.9% to 18.8%), Alteronoadeaecae (T1-T3: from 17.3% to <1%), Halomonadaceae (T1-T3: from 4.4% to 1.2%), Nitrincolaceae (T1-T3: from 29.2% to <1%).
In A1, over time, the microbial biofilm structure was characterized by an increase of Rhodobacteraceae (T1-T3: from 13.0% to 60.9%), Flavobacteriaceae (T1-T3: from 10.5% to 22.3%), Puniceicoccaceae (T1-T3: from <1% to 2.4%), Rubritaleaceae (T1-T3: from <1% to 1.2%), Saprospiraceae (T1-T3: from <1% to 2.6%) and NS9 marine group of the Flavobacteriales (T1-T3: from <1% to 1.6%). Furthermore over time, the A1 microbial biofilm structure was characterized by a decrease of Alteromonadaceae (T1-T3: from 47.7% to < 1%), Vibrionaceae (T1-T3: from 17.5% to 3.4%), Arcobacteraceae (T1-T3: from 3.7% to < 1%), Psychoromonadaceae (T1-T3: from 1.1% to < 1%).
In A2, over time, the microbial biofilm structure was characterized by an increase of Rhodobacteraceae (T1-T3: from 14.1% to 27.2%), Flavobacteriaceae (T1-T3: from 17.5% to 23.0%), Halomonadaceae (T1-T3: from 11.2% to 21.6%), Puniceicoccaceae (T1-T3: from <1% to 9.2%), Rubritaleaceae (T1-T3: from <1% to 3.2%) and Micavibrionaceae (T1-T3: from <1% to 1.1%). Furthermore over time, the A2 microbial biofilm structure was characterized by a decrease of Alteromonadaceae (T1-T3: from 37.9% to 1.2%), Vibrionaceae (T1-T3: from 13.3% to 6.0%), Marinomonadaceae (T1-T3: from 1.6% to < 1%), Pseudoalteromonadaceae (T1-T3: from 1.2% to < 1%).
In A3, over time, the microbial biofilm structure was characterized by an increase of Rhodobacteraceae (T1-T3: from 5.3% to 45.5%), Flavobacteriaceae (T1-T3: from 16.9% to 21.5%), Puniceicoccaceae (T1-T3: from <1% to 1.3%) and Rubritaleaceae (T1-T3: from <1% to 1.8.%). Vibrionaceae stayed stable from T1 to T3, accounting for 4%. Furthermore, over time, the A3 microbial biofilm structure was characterized by a decrease of Alteromonadaceae (T1-T3: from 16.7% to 3.7%), Halomonadaceae (T1-T3: from 43.0% to 15.3%), Acrobacteriaceae (T1-T3: from 9.8% to < 1%) and Marinomonadaceae (T1-T3: from 1.1% to < 1%).
The most abundant taxa of adult thalli decreased during the cultivation phase in the seedling (Figure 10). The adult thalli were characterized by Pleurocapsa (Cyanobacteria), Schizotrhrix (Cyanobacteria), and Bacteria: Tenacibaculum, uncultured OTU from SVa0996 marine group, Candidatus Thiodiaxotropha, Portibacter, Ganulosicoccus, Rudimonas and Lewinella. On the other hand, the most abundant taxa in the seedlings were less abundant on the adult thalli (Figure 11).
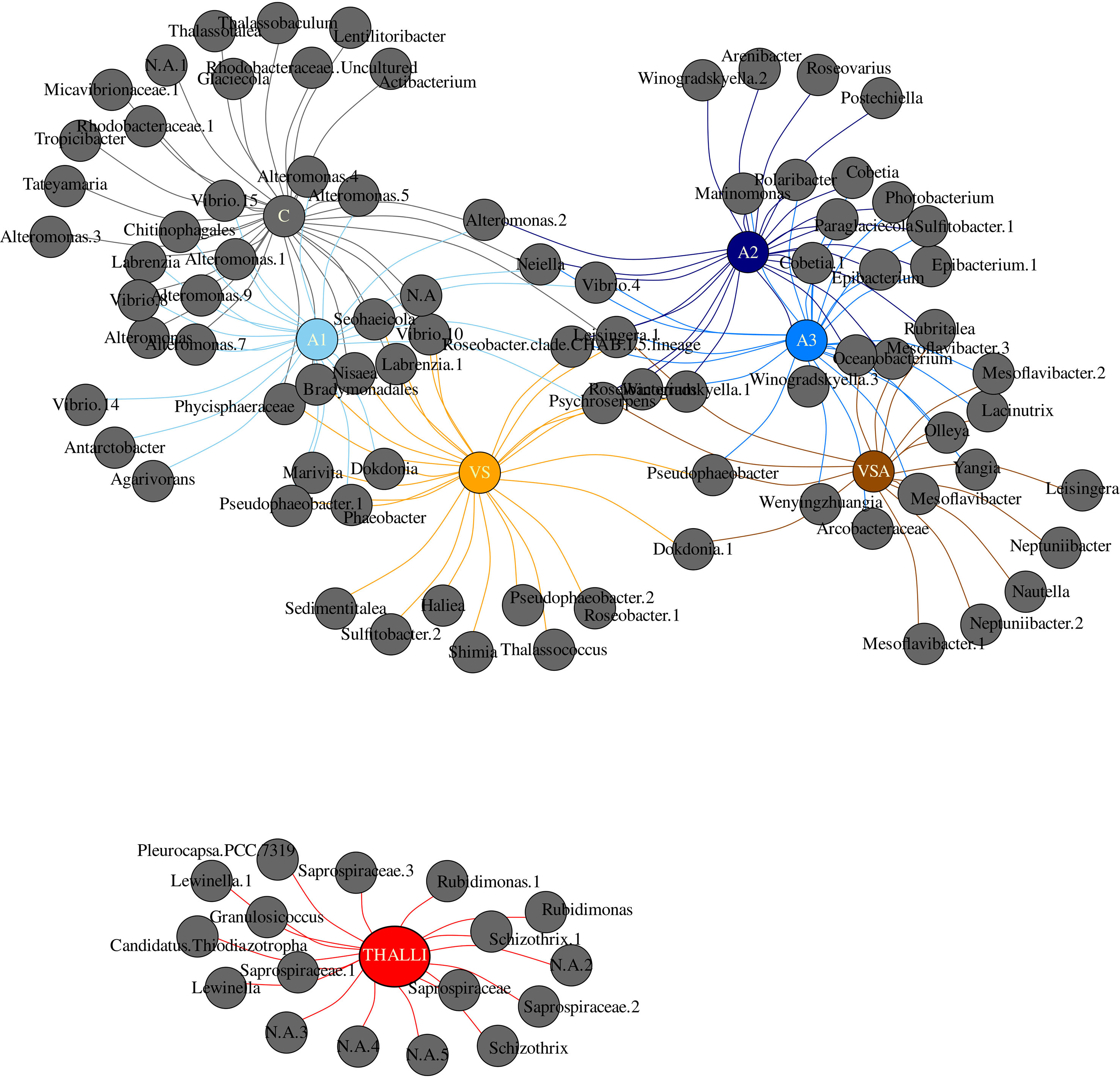
Figure 11 Indicator species analysis based on OTU > 1% abundance at the genus level of the adult thalli (red) and seedlings in each treatment. Treatments are color coded: C-gray, A1-baby blue, A2-blue, A3-navy blue, VS-orange, VSA-brown.
The A series were characterized by Neptuniibacter, Alteromonas, Antarctobacter, Pseudophaeobacter, Dokdonia, Maribacter, Epibacterium, Cobetia, Vibrio, Polaribacter, Paraglaciecola, Winogradskyella, Mesoflavibacter, Olleya and Puniceicoccus.
The indicator species analysis depicted no statistically significant shared OTUs in both the adult thalli and seedling microbial biofilm communities (Figure 11; Supplementary Data Sheet). Each treatment was characterized by specific OTUs at the genus level, respectively: C by 12, VS by 7, VSA by 5, A1 by 3, A2 by 4, A3 by 3, and adult thalli by 17. Adult thalli were not sharing unique taxa within the seedling communities. C shared OTUs with A1(10) and VS (6) uniquely. A2 was uniquely characterized by Postechiella, Roseovarius, Winogradskyella.2 and Arenibacter. A1, A2, VS, VSA shared Psychroserpens.
Whereas, A2 shared only with VS Roseibacterium; with VSA Rubritalea and with A3 10 OTUs (Cobetia, Epibacterium, Cobetia.1, Epibacterium.1, Sulfitobacter.1, Photobacterium, Winogradskyella.3, Paraglaciecola, Polaribacter and Marinomonas). A1, A2 and A3 shared Vibrio 4 and Amylibacter. A1, A2, A3 and VS shared Roseobacter.clade.CHAB.I.5.lineage. A2, A3, VSA shared Oceanobacterium and Mesoflavibacter.3. Finally, VS and VSA shared Dokdonia 1. A1 was uniquely characterized by Agarivorans, Antarctobacter and Vibrio 14. VS was uniquely characterized by Sulfitobacter.2, Sedimentitalea, Roseobacter.1, Thalassococcus, Pseudophaeobacter.2, Haliea and Shimia. VSA was uniquely characterized by Nautella, Neptuniibacter.2 and 1, Leisingera, Neptuniibacter and Mesoflavibacter.1.
4 Discussion
Our results show that the commercial seaweed extract AlgatronCifo® and von Stoch medium (VS) influenced seedling survival and growth as well as microbial biofilm communities. Overall, the A2 culture survived best in the field. VS, VSA and A1 were the second- best treatments, A3 performed poorly, while C seedlings did not survive in the field.
Brown algae extract with biostimulant properties have recently been used to improve harvest in aquaculture (Hurtado and Critchley, 2018; Umanzor et al., 2019; Hurtado and Critchley, 2020; Umanzor et al., 2020a; Umanzor et al., 2020b; Han et al., 2022; Jiksing et al., 2022; Umanzor et al., 2022). The priming effect is likely due to their complex composition and the presence of compounds with biostimulatory activities like polysaccharides (Shukla et al., 2021) and secondary metabolites. Phenolic compounds (Stirk et al., 2020; Sujeeth et al., 2022) play a crucial role in cell wall formation and early development of brown algae (Schoenwaelder, 2002), help adapt to environmental stressors and defend against biological pressures such as grazers, pathogens and epiphytes (Stiger-Pouvreau et al., 2014; Generalić Mekinić et al., 2019). In plants, algal-derived extracts have been shown to induce changes in the microbial community and inhibit plant pathogens (see references in Ali et al., 2021; Shukla et al., 2021).
In our study, the culture media (i.e., VS) and the addition of Algatron had a significant effect on the structure and composition of the biofilm communities associated with the seedlings (Figure 11). The biofilm communities shifted in distinct ways over time, with an overall decrease in the diversity of OTUs compared to adult thalli. This could be due to the more controlled and less variable culture environment compared to the intertidal zone where E. amentacea thrives, where desiccation, wave dynamics, temperature stress and ever-changing seawater conditions (i.e., nutrients, salinity, pH, pollution, etc.) strongly influence the algae and their biofilm.
In our experimental setting, we cannot unambiguously distinguish between the effect of the additive on algal physiology, which in turn modified the microbes (indirect effect of biostimulant and nutrients, via algal metabolism, Ren et al., 2022) and the direct effect of biostimulant/nutrients on microbial growth and community structure.
At the end of the mesocosm culture (T3), the treatments with VS (i.e., VS, VSA) and A1 and A2 showed comparable seedling coverage. However, A2 and A1 also showed optimal photophysiological fitness (Fv/Fm > 0.69). The VS and VSA treatments had the lowest Fv/Fm values (0.51 ± 0.02 and 0.46 ± 0.02, respectively), indicating stress to the photosynthetic apparatus (Celis-Plá et al., 2014; Smolina et al., 2016; Falace et al., 2018b; Savva et al., 2018; Verdura et al., 2021). After the field period, all treatments showed good photosynthetic efficiency, with A2 having the highest coverage, being 1.8, 1.9, 2.2 and 6.0 times higher than VS, VSA, A1 and A3, respectively.
We hypothesize that the higher survival is due to the interplay of nutrients, presence/absence of epiphytes and their antagonistic or beneficial relationships with the E. amentacea seedlings and finally priming by the algal extract.
While E. amentacea is mainly restricted to oligotrophic waters and is sensitive to eutrophication (Pinedo et al., 2007; Mangialajo et al., 2008; Thibaut et al., 2014), seedlings may have a higher nutrient uptake capacity than adult thalli, as observed in other Fucales (Thomas et al., 1985; Sánchez de Pedro et al., 2022). Although culture media were frequently renewed, C-culture seedlings likely suffered from nutrient limitation (Supplementary Table 1), which may have affected many processes like photosynthetic capacity, embryonic development and growth (Duarte, 1992; Roleda and Hurd, 2019). Lower growth of E. amentacea seedlings in seawater compared to VS was also observed by Susini (2006). The lowest performance in terms of survival and growth of C seedlings was followed by total failure in the field. We found that the C biofilm differed the most from the other treatments (in the PCoA plot) despite the high alpha diversity. This treatment shared fewer OTUs with the adult thalli (13/64 scoring > 1% relative abundance) than the other treatments with nutrient addition, indicating the importance of microbial interactions and algal growth in co-shaping the microbial biofilm, as reported in Sargassum (Hervé et al., 2021) and Macrocystis (Michelou et al., 2013).
The VS and VSA treatments had high nutrient concentrations, with the highest nitrate () and phosphate () levels of all treatments (Supplementary Table 1), which were 5.25 and 5.32 fold and 26.5 and 32.3 fold higher than the C-treatment, respectively. is one of the two main sources of nitrogen (N), which is essential for macroalgal growth as it is a major component of the photosynthetic apparatus, amino acids and cellular enzymes, and thus can be a critical limiting factor in the marine environment (Hurd et al., 2014). As well, phosphorus (P) is a vital nutrient for macroalgae, involved in algal photosynthesis and respiration, particularly energy transfer through the synthesis of adenosine triphosphate (ATP) and other energy-rich compounds (Lobban and Harrison, 1994). The nutrient-rich conditions, characterized by a lower N:P ratio in VS and VSA (10.29 and 65.26 respectively, Supplementary Table 1) which fall within the optimal range for seaweeds growth (i.e., from 10:1 to 80:1; Suthar et al., 2019), simultaneously promoted the highest growth of seedlings, but also, as expected, of epiphytes. Indeed, fast-growing opportunistic epiphytes (i.e., filamentous algae, diatoms, cyanobacteria) have a competitive advantage at high nutrient concentrations by maximizing rapid nutrient uptake and photosynthetic efficiency (Fujita, 1985; Carpenter, 1990; Duarte, 1995). Furthermore, when growing on the outer surface of seedlings, they compete for light (Pang et al., 2011) and act as a barrier to carbon uptake (Sand-Jensen, 1977), resulting in lower photosynthetic efficiency of their hosts. In algal cultures, epiphyte infestation has been shown to impair algal productivity (Lüning and Pang, 2003; Ward et al., 2020) and is one of the main barriers to ex situ culture of Cystoseira s.l. (e.g. Orlando-Bonaca et al., 2021; Clausing et al., 2022; Lardi et al., 2022). In addition, epiphytes can in some cases damage host tissues (Hayashi et al., 2010) and facilitate colonization by opportunistic bacteria (Vairappan et al., 2008; Jiksing et al., 2022) that cause disease.
Contrary to the treatments with VS (i.e., VS, VSA), in the Algatron treatment, the main source of N was ammonium () (Supplementary Table 1), which may be a more efficient form of N fertilizer for brown algal growth (Dā Costa Braga and Yoneshigue-Valentin, 1996; Smart et al., 2022). Among these treatments, A3 showed the lowest performance in terms of growth and survival. Seedlings cultivated in A3 treatment were exposed to the highest ammonium concentration () (Supplementary Table 1), which might have been the cause of the low cover despite the good Fv/Fm. In an indoor culture of Sargassum spp. seedlings, was shown to effectively promote their growth (Han et al., 2018) and increase photosynthesis (Hong et al., 2021), but a too high ammonium content (i.e., 900 μmol·L-1) can negatively affect growth (Hong et al., 2021). It has also been noted that algal extracts can have both positive and negative effects depending on the concentration (Kapoore et al., 2021). A1 and A2 seedlings had on average the same Fv/Fm, with A2 seedlings showing higher growth and survival, indicating a more suitable concentration than A1. In treatments A1 and A2, was supplied at concentrations 4.5 and 2 times lower, respectively, than in A3 (Supplementary Table 1), which promoted the growth of E. amentacea without favoring the development of epiphytes. Apart from high availability, other constituents of the algal extract Algatron may have induced changes in seedlings metabolism and signaling pathways specifically related to nutrient uptake and/or nutrient translocation improving nutrient use efficiency, as observed in plants (Jannin et al., 2013; Saa et al., 2015; Sujeeth et al., 2022). Nevertheless, in our experimental settings we cannot unambiguously assess the effect of the biostimulant versus the ammonium () per se. The toxicity of A3 medium to the seedlings could be due to high ammonium concentration. On the other hand, the lower cover (%) of seedlings under A1 than in A2 media showed indeed an effect of biostimulant because nutrients (i.e., nitrate and ammonium) were not limiting in the A1 medium. Further studies are required to disentangle a possible biostimulant action from the effect on the seedling growth. Moreover, complementary studies using -omics techniques (i.e., metabolomics and/or transcriptomics) could identify the modes of actions and the metabolic pathways by which the complex extract might improve seedlings performance.
The lower occurrence of opportunistic epiphytes can also be attributed to the lower concentrations compared to VS and VSA, since fast-growing species have much higher P-demands than slower growing algae (Pedersen et al., 2010). According to the N:P ratio at which nutrients were supplied in Algatron treatments (A1 = 278.5; A2 = 324.58; A3 = 350.93, respectively), there would be an external P-limitation also for seedlings development, nevertheless the culture media were suitable for seedling growth, especially in A2, where the was 5.85 times higher than in the C treatment despite a relatively more balanced N:P ratio in the latest (i.e., 60.1) (Supplementary Table 1). Indeed, to avoid misuse, the N:P ratio should be interpreted along with the absolute amounts of each nutrient (Dodds, 2003).
The good performance in terms of growth, survival and photophysiological fitness of the seedlings in A2 treatment might have played an important role in the success of this treatment in the field. In previous ex-situ outplantings, high detachment of E. amentacea seedlings was documented after transfer to the field (De La Fuente et al., 2019; Clausing et al., 2022). The high survival of A2 seedlings in the field might be related to the ability of the seedlings to anchor to the tiles due to the alginate-enriched algal extract, which can act as a metabolic stimulant and trigger favorable physiological responses (Briceño-Domínguez et al., 2014; Shukla et al., 2021). Although the synthetic pathways of alginates are not fully understood, it is thought that alginates are first synthesized as a polymer of mannuronic acid (a component of alginic acid) by alginate synthase and that some of the mannuronic acid is converted to guluronic acid (Michel et al., 2010). Alginates can influence the mechanical properties of cell walls in the rhizoids of developing zygotes of Fucales (Linardic, 2018; Yonamine et al., 2021) and provide for a stronger rhizoid system. We can assume that the A2 seedlings had stronger rhizoids that allowed them to adhere more firmly to the tiles. Considering that E. amentacea thrives in wave-exposed sites (Agardh, 1842; Boudouresque, 1971; Thibaut et al., 2014), it follows that well-developed rhizoids could promote better seedling survival.
The 20 most dominant families we found on the seedlings and adult thalli of E. amentacea were also identified as the most abundant on other brown algae (Florez et al., 2017 and reference), as Fucales and Laminariales. This suggests a great microbial plasticity that allows them to thrive with ecologically diverse algae. Within these families, there are many multitudes of microbial adaptive strategies for growth, nutrient uptake, and antagonistic interactions. From an algal perspective (Florez et al., 2017), studies have shown that Rhodobacteraceae, Flavobacteriaceae, Alteromonadaceae, Vibrionaceae, and Halomonadaceae can induce morphogenesis and degrade algal compounds. Rhodobacteraceae, Flavobacteriaceae, Alteromonadaceae, Vibrionaceae, Halomonadaceae Bacteriovoraceae, Saprospiraceae may be related to pathogenesis and antibacterial activity. Furthermore, microbially induced diseases have been identified in Laminaria, such as hole-rotten disease, red spot disease, spot-wounded fronds and swollen gametophytes and filamentous fading caused by Pseudomonas, Vibrio, Halomonas and Alteromonas strains (Egan et al., 2014). It follows that biofilm-associated microbes can become opportunistic pathogens when the host is stressed by temperature, UV and nutrient stress, eutrophication or other anthropogenic induced challenges (Egan et al., 2014; Duarte et al., 2018; van der Loos et al., 2019).
Overall, the adult thalli have structured seedlings biofilm community composition. Taxa that were dominant in the adult thalli decreased in the seedlings and vice versa. Taxa that were dominant in the seedlings were copiotrophs, particle- or biofilm-associated (Lauro et al., 2009; Heins and Harder, 2023) and high-efficient organic matter degraders (Cottrell and Kirchman, 2000). Given these features, we can surmise that these microbes were closely associated with algal growth. The seedling biofilm communities were characterized by metabolisms predicted by the FAPROTAX annotation, which primarily used chemical energy released by breaking chemical bonds to degrade organic carbon from the primary production of algae and alginates contained in the algal extract Algatron.
Index species analysis based on pre-infield biofilm communities revealed that the best performing treatment, A2, was uniquely characterized by 5 Gram-negative aerobic genera Postechiella, Roseovarius, Winogradskyella and Arenibacter. Many strains from these genera have been isolated from diverse marine systems, indicating the huge metabolic flexibility (Lee et al., 2012; Luo and Moran, 2014; Zhuang and Luo, 2020). Furthermore, these unique taxa have been reported as easy to cultivate and purify, in relation to the fact that not more than 1% of the microbes in every environment can be cultivated. This is an extremely important and desirable feature for planning future probiotic-focused bacterial strain cultivation efforts. Postechiella, Winogradskyella and Arenibacter (Flavobacteriaceae) have been shown to be able to degrade agar, DNA, starch and many diverse sugars beside proteins and lipids (Lee et al., 2012; Gutierrez et al., 2014; Kurilenko et al., 2019). The microbial degradative activities could be important for supplying the algae with nutrients from the carbohydrate, protein and lipid pools of the algal extract, which can then be used for primary production. Another possible function is the control of biofilm structure and dynamics by DNase and sugar and protein hydrolysis activities (Whitchurch et al., 2002; Wang et al., 2004). Arenibacter is also able to degrade polycyclic aromatic hydrocarbon compounds. This is interesting given that E. amentacea, like the other brown algae, produces polyphenols (Generalić Mekinić et al., 2019) and Algatron contains these compounds. Polyphenols are important for chemical defense against herbivory (i.e., non palatability) and microbes, and for protection against oxidative stress by absorbing harmful UV and excessive irradiation. Thus, for microbes being able to degrade a toxic substance as phenols could therefore be beneficial for more efficient microbial competition for space and nutrients. The Roseovarius (family Rhodobacteraceae) genome presents pathways for the production of thiamine and cobalamin (Luo and Moran, 2014). Thiamine and cobalamin are important micronutrients for algae (Croft et al., 2006), playing key roles in their central metabolism. Many genera belonging to the Rhodobacteraceae produce these vitamins and share them with primary producers within the virtuous cycle of organic matter production and remineralization (Bertrand and Allen, 2012; Luo and Moran, 2014).
A2, A1, VSA and VS shared the taxon Psychroserpens (Flavobacteriaceae, Ping et al., 2022), which is able to degrade alginate and casein, suggesting a role in degrading sugar-rich and phospho-protein rich compounds within the biofilm. Interestingly, some strains require vitamins for growth thus being a competitor for these micronutrients in the algal biofilm.
A few studies have found that secondary metabolites from macroalgal extracts have strong effects on microbial surface colonization, altering the bacterial biofilm formation and community composition under laboratory and field conditions (Sneed and Pohnert, 2011; Egan et al., 2013; Lachnit et al., 2013).
Further experiments should elucidate the direct or indirect effect of nutrients and biostimulants in promoting a probiotic microbial community by investigating the gene expression of algae and microbes along the diverse treatments and their degree of interdependence. It would be important to track O2 evolution using optode technique in the key treatments to guide microbial sampling when changes in algal performance occur. Such an approach will allow coupling algal performance with microbial community response and fill the gap in the functional role of microbes in macroalgal holobiont health (Duarte et al., 2018).
Given the seedling success of A2, we propose treatment tailored probiotic consortia candidates characterized by the unique treatment-taxa (A2: Postechiella, Roseovarius, Winogradskyella and Arenibacter) and the reminder community. At this stage, we cannot disentangle the net contribution of the unique vs. shared vs. reminder community in relation to the overall seedling fitness. Within the holobiont concept, we can hypothesize that the nature and intensity of interactions might be important in defining probiotic consortia. Furthermore, the effect of algal extract or nutrients on the algae and/or biofilm could have important consequences for tuning the overall interaction network. Our study has shown that ex-situ restoration of macroalgae could benefit from both the use of commercial algal extracts in ex-situ cultures and the identification of probiotic consortia candidates that promote seedling growth and optimal protection against biotic and abiotic stressors.
Data availability statement
The datasets presented in this study can be found in online repositories. The names of the repository/repositories and accession number(s) can be found below: BioProject, PRJNA922753.
Author contributions
AF, SK, FM designed the study. LA, FM, SK, AS, CB, FG, AF performed the laboratory measurements. AP, FM, SB, AS, SN performed the formal data analysis. FM, SK, AF wrote the original draft of the manuscript. All authors contributed to the article and approved the submitted version.
Funding
This work was supported by grants from the LIFE financial instrument of the European Community, project REEForest-LIFE (101074309 LIFE21-NAT-IT-REEForest) and from the Davines support to the ‘Tuteliamo il Mare’ campaign.
Acknowledgments
The authors would like to thank Prof. Monia Renzi for the Algatron nutrient analysis and the Agenzia Regionale per la Protezione dell’Ambiente del Friuli Venezia Giulia (ARPA-FVG) for providing the seawater nutrient data. We would also like to thank Saul Ciriaco for field work and Dr Marina Srijemsi for help with laboratory culture.
Conflict of interest
The authors declare that the research was conducted in the absence of any commercial or financial relationships that could be construed as a potential conflict of interest.
Publisher’s note
All claims expressed in this article are solely those of the authors and do not necessarily represent those of their affiliated organizations, or those of the publisher, the editors and the reviewers. Any product that may be evaluated in this article, or claim that may be made by its manufacturer, is not guaranteed or endorsed by the publisher.
Supplementary material
The Supplementary Material for this article can be found online at: https://www.frontiersin.org/articles/10.3389/fmars.2023.1181685/full#supplementary-material
References
Abelson A., Reed D. C., Edgar G. J., Smith C. S., Kendrick G. A., Orth R. J., et al. (2020). Challenges for restoration of coastal marine ecosystems in the anthropocene. Front. Mar. Sci. 7. doi: 10.3389/fmars.2020.544105
Agardh J. G. (1842). Algae maris mediterranei et adriatici, observationes in diagnosin specierum et dispositionem generum (Paris: Parisiis: Apud Fortin, Masson et Cie.), 164.
Ali O., Ramsubhag A., Jayaraman J. (2021). Biostimulant properties of seaweed extracts in plants: implications towards sustainable crop production. Plants 10, 531. doi: 10.3390/plants10030531
Anderson M. J. (2001). Permutation tests for univariate or multivariate analysis of variance and regression. Can. J. Fish. Aquat. Sci. 58, 626–639. doi: 10.1139/f01-004
Anderson M. J. (2014). Permutational multivariate analysis of variance (PERMANOVA) (Wiley statsref: statistics reference online), 1–15. doi: 10.1002/9781118445112.stat07841
Battacharyya D., Babgohari M. Z., Rathor P., Prithiviraj B. (2015). Seaweed extracts as biostimulants in horticulture. Sci. Hortic. (Amsterdam) 196, 39–48. doi: 10.1016/j.scienta.2015.09.012
Bengtsson M. M., Sjøtun K., Lanzén A., Øvreås L. (2012). Bacterial diversity in relation to secondary production and succession on surfaces of the kelp Laminaria hyperborea. ISME J. 6, 2188–2198. doi: 10.1038/ismej.2012.67
Berg G., Kusstatscher P., Abdelfattah A., Cernava T., Smalla K. (2021). Microbiome modulation-toward a better understanding of plant microbiome response to microbial inoculants. Front. Microbiol. 12, 650610. doi: 10.3389/fmicb.2021.650610
Bertrand E. M., Allen A. E. (2012). Influence of vitamin b auxotrophy on nitrogen metabolism in eukaryotic phytoplankton. Front. Microbiol. 3, 375. doi: 10.3389/fmicb.2012.00375
Bevilacqua S., Savonitto G., Lipizer M., Mancuso P., Ciriaco S., Srijemsi M., et al. (2019). Climatic anomalies may create a long-lasting ecological phase shift by altering the reproduction of a foundation species. Ecology 100, 1–4. doi: 10.1002/ecy.2838
Blanfuné A., Boudouresque C. F., Verlaque M., Beqiraj S., Kashta L., Nasto I., et al. (2016). Response of rocky shore communities to anthropogenic pressures in Albania (Mediterranean sea): ecological status assessment through the CARLIT method. Mar. pollut. Bull. 109, 409–418. doi: 10.1016/j.marpolbul.2016.05.041
Blunden G., Wildgoose P. B. (1977). The effects of aqueous seaweed extract and kinetin on potato yields. J. Sci. Food. Agric. 28, 121–125. doi: 10.1002/jsfa.2740280203
Boudouresque C. F. (1971). Contribution à l’étude phytosociologique des peuplements algaux des côtes varoises. Vegetatio 22 (1-3), 83–184. doi: 10.1007/BF01955721
Briceño-Domínguez D., Hernández-Carmona G., Moyo M., Stirk W., van Staden J. (2014). Plant growth promoting activity of seaweed liquid extracts produced from Macrocystis pyrifera under different pH and temperature conditions. J. Appl. Phycol. 26, 2203–2210. doi: 10.1007/s10811-014-0237-2
Buonomo R., Assis J., Fernandes F., Engelen A. H., Airoldi L., Serrão E. A. (2017). Habitat continuity and stepping-stone oceanographic distances explain population genetic connectivity of the brown alga Cystoseira amentacea. Mol. Ecol. 26, 766–780. doi: 10.1111/mec.13960
Caporaso J. G., Lauber C. L., Walters W. A., Berg-Lyons D., Lozupone C. A., Turnbaugh P. J., et al. (2011). Global patterns of 16S rRNA diversity at a depth of millions of sequences per sample. Proc. Natl. Acad. Sci. U S A. 108 Suppl 1 (Suppl 1), 4516–4522. doi: 10.1073/pnas.1000080107
Carpenter R. C. (1990). Competition among marine macroalgae: a physiological perspective. J. Phycol. 26, 6–12. doi: 10.1111/j.0022-3646.1990.00006.x
Celis-Plá P. S. M., Korbee N., Gómez-Garreta A., Figueroa F. L. (2014). Seasonal photoacclimation patterns in the intertidal macroalga Cystoseira tamariscifolia (Ochrophyta). Sci. Mar. 78, 377–388. doi: 10.3989/scimar.04053.05A
Claesson M. J., O’Sullivan O., Wang Q., Nikkilä J., Marchesi J. R., Smidt H., et al. (2009). Comparative analysis of pyrosequencing and a phylogenetic microarray for exploring microbial community structures in the human distal intestine. PloS One 4 (8), e6669. doi: 10.1371/journal.pone.0006669
Clausing R. J., de la Fuente G., Falace A., Chiantore M. (2022). Accounting for environmental stress in restoration of intertidal foundation species. J. Appl. Ecol. 1365-2664, 14334. doi: 10.1111/1365-2664.14334
Clayton M. N. (1990). The adaptive significance of life history characters in selected orders of marine brown macroalgae. Aust. J. Ecol. 15, 439–452. doi: 10.1111/j.1442-9993.1990.tb01469.x
Cleary D. F. R., Polónia A. R. M., Swierts T., Coelho F. J. R. C., de Voogd N. J., Gomes N. C. M. (2022). Spatial and environmental variables structure sponge symbiont communities. Mol. Ecol. 31 (19), 4932–4948. doi: 10.1111/mec.16631
Cottrell M. T., Kirchman D. L. (2000). Natural assemblages of marine proteobacteria and members of the cytophaga-flavobacter cluster consuming low- and high-molecular-weight dissolved organic matter. Appl. Environ. Microbiol. 66 (4), 1692–1697. doi: 10.1128/AEM.66.4.1692-1697.2000
Croft M. T., Warren M. J., Smith A. G. (2006). Algae need their vitamins. Eukaryot Cell. 5 (8), 1175 83. doi: 10.1128/EC.00097-06
Crouch I. J., Van Staden J. (1993). Commercial seaweed products as biostimulants in horticulture. J. Home. Consum. Hortic. 1, 19–76. doi: 10.1300/J280v01n01_03
Dā Costa Braga A., Yoneshigue-Valentin Y. (1996). “Nitrogen and phosphorus uptake by the Brazilian kelp laminaria abyssalis (Phaeophyta) in culture,” in Fifteenth international seaweed symposium. Eds. Lindstrom S. C., Chapman D. J. (Dordrecht: Springer), 445–450.
De Cáceres M., Legendre P. (2009). Associations between species and groups of sites: indices and statistical inference. Ecology 90, 3566–3574. doi: 10.1890/08-1823.1
De La Fuente G., Chiantore M., Asnaghi V., Kaleb S., Falace A. (2019). First ex situ outplanting of the habitat-forming seaweed Cystoseira amentacea var. stricta from a restoration perspective. PeerJ 7, 1–16. doi: 10.7717/peerj.7290
Dittami S. M., Arboleda E., Auguet J.-C., Bigalke A., Briand E., Cárdenas P., et al. (2021). A community perspective on the concept of marine holobionts: current status, challenges, and future directions. PeerJ 9, e10911. doi: 10.7717/peerj.10911
Dodds W. K. (2003). Misuse of inorganic n and soluble reactive p concentrations to indicate nutrient status of surface waters. J. North Am. Benthol Soc. 22, 171–181. doi: 10.2307/1467990
Duarte C. M. (1992). Nutrient concentration of aquatic plants: patterns across species. Limnol. Oceanogr. 37, 882–889. doi: 10.4319/lo.1992.37.4.0882
Duarte C. M. (1995). Submerged aquatic vegetation in relation to different nutrient regimes. Ophelia 41, 87–112. doi: 10.1080/00785236.1995.10422039
Duarte B., Martins I., Rosa R., Matos A. R., Roleda M. Y., Reusch T. B. H., et al. (2018). Climate change impacts on seagrass meadows and macroalgal forests: an integrative perspective on acclimation and adaptation potential. Front. Mar. Sci. 5. doi: 10.3389/fmars.2018.00190
du Jardin P. (2012) The science of plant biostimulants-a bibliographic analysis. Available at: http://ec.europa.eu/enterprise/sectors/chemicals/files/fertilizers/final_report_bio_2012_en.pdf.
Egan S., Fernandes N. D., Kumar V., Gardiner M., Thomas T. (2014). Bacterial pathogens, virulence mechanism and host defense in marine macroalgae. Environ. Microbiol. 16 (4), 925–938. doi: 10.1111/1462-2920.12288
Egan S., Gardiner M. (2016). Microbial dysbiosis: rethinking disease in marine ecosystems. Front. Microbiol. 7. doi: 10.3389/fmicb.2016.00991
Egan S., Harder T., Burke C., Steinberg P., Kjelleberg S. (2013). The seaweed holobiont: understanding seaweed-bacteria interactions. FEMS Microbiol. Rev. 37, 462–476. doi: 10.1111/1574-6976.12011
Fabbrizzi E., Scardi M., Ballesteros E., Benedetti-Cecchi L., Cebrian E., Ceccherelli G., et al. (2020). Modeling macroalgal forest distribution at Mediterranean scale: present status, drivers of changes and insights for conservation and management. Front. Mar. Sci. 7, 20. doi: 10.3389/fmars.2020.00020
Falace A., Alongi G., Cormaci M., Furnari G., Curiel D., Cecere E., et al. (2010). Changes in the benthic algae along the Adriatic Sea in the last three decades. Chem. Ecol. 26, 77–90. doi: 10.1080/02757541003689837
Falace A., Kaleb S., de la Fuente G., Asnaghi V., Chiantore M. (2018a). Ex situ cultivation protocol for Cystoseira amentacea var. stricta (Fucales, phaeophyceae) from a restoration perspective. PloS One 13, 1–16. doi: 10.1371/journal.pone.0193011
Falace A., Marletta G., Savonitto G., Candotto Carniel F., Srijemsi M., Bevilacqua S., et al. (2021). Is the south-Mediterranean canopy-forming Ericaria giacconei (= Cystoseira hyblaea) a loser from ocean warming? Front. Mar. Sci. 8. doi: 10.3389/fmars.2021.760637
Falace A., Tamburello L., Guarnieri G., Kaleb S., Papa L., Fraschetti S. (2018b). Effects of a glyphosate-based herbicide on fucus virsoides (Fucales, ochrophyta) photosynthetic efficiency. Environ. pollut. 243, 912–918. doi: 10.1016/j.envpol.2018.08.053
Falace A., Zanelli E., Bressan G. (2006). Algal transplantation as a potential tool for artificial reef management and environmental mitigation. Bull. Mar. Sci. 78, 161–166.
Florez J. Z., Camus C., Hengst M. B., Buschmann A. H. (2017). A functional perspective analysis of macroalgae and epiphytic bacterial community interaction. Front. Microbiol. 8. doi: 10.3389/fmicb.2017.02561
Fujita R. M. (1985). The role of nitrogen status in regulating transient ammonium uptake and nitrogen storage by macroalgae. J. Exp. Mar. Biol. Ecol. 92, 283–301. doi: 10.1016/0022-0981(85)90100-5
Gasol J. M., Del Giorgio P. A. (2000). Using flow cytometry for counting natural planktonic bacteria and understanding the structure of planktonic bacterial communities. Scientia Marina 64 (2), 197–224. doi: 10.3989/scimar.2000.64n2197
Generalić Mekinić I., Skroza D., Šimat V., Hamed I., Čagalj M., Popović Perković Z. (2019). Phenolic content of brown algae (Pheophyceae) species: extraction, identification, and quantification. Biomolecules 9 (6), 244. doi: 10.3390/biom9060244
Giglio A., Vommaro M. L., Gionechetti F., Pallavicini A. (2021). A. gut microbial community response to herbicide exposure in a ground beetle. J. Appl. Entomology 145, 986–1000. doi: 10.1111/jen.12919
Grueneberg J., Engelen A. H., Costa R., Wichard T. (2016). Macroalgal morphogenesis induced by waterborne compounds and bacteria in coastal seawater. PloS One 11, e0146307. doi: 10.1371/journal.pone.0146307
Guiry M. D., Cunningham E. M. (1984). Photoperiodic and temperature responses in the reproduction of northeastern Atlantic Gigartina acicularis (Rhodophyta: gigartinales). Phycologia 23 (3), 357–367. doi: 10.2216/i0031-8884-23-3-357.1
Gutierrez T., Rhodes G., Mishamandani S., Berry D., Whitman W. B., Nichols P. D., et al. (2014). Polycyclic aromatic hydrocarbon degradation of phytoplankton-associated arenibacter spp. and description of arenibacter algicola sp. nov., an aromatic hydrocarbon-degrading bacterium. Appl. Environ. Microbiol. 80 (2), 618–628. doi: 10.1128/AEM.03104-13
Han T., Qi Z., Huang H., Liao X., Zhang W. (2018). Nitrogen uptake and growth responses of seedlings of the brown seaweed Sargassum hemiphyllum under controlled culture conditions. J. Appl. Phycol 30, 507–515. doi: 10.1007/s10811-017-1216-1
Han S., Song H. I., Park J. S., Kim Y. J., Umanzor S., Yarish C., et al. (2022). Sargassum horneri And Ascophyllum nodosum extracts enhance thermal tolerance and antioxidant activity of Neopyropia yezoensis. J. Appl. Phycol. 35 (1), 201–207. doi: 10.1007/s10811-022-02870-4
Hayashi L., Hurtado A. Q., Msuya F. E., Bleicher-Lhonneur G., Critchley A. T. (2010). “A review of kappaphycus farming: prospects and constraints,” in Seaweeds and their role in globally changing environments. Eds. Seckbach J., Einav R., Israel A. (Dordrecht: Springer Netherlands), 251–283.
Heins A., Harder J. (2023). Particle-associated bacteria in seawater dominate the colony-forming microbiome on ZoBell marine agar. FEMS Microbiol. Ecol. 99, 1–11. doi: 10.1093/femsec/fiac151
Hervé V., Lambourdière J., René-Trouillefou M., Devault D. A., Lopez P. J. (2021). Sargassum Differentially shapes the microbiota composition and diversity at coastal tide sites and inland storage sites on Caribbean islands. Front. Microbiol. 12. doi: 10.3389/fmicb.2021.701155
Holmström C., Egan S., Franks A., McCloy S., Kjelleberg S. (2002). Antifouling activities expressed by marine surface associated pseudoalteromonas species. FEMS Microbiol. Ecol. 41, 47–58. doi: 10.1016/S0168-6496(02)00239-8
Hong M., Ma Z., Wang X., Shen Y., Mo Z., Wu M., et al. (2021). Effects of light intensity and ammonium stress on photosynthesis in Sargassum fusiforme seedlings. Chemosphere. 273. doi: 10.1016/j.chemosphere.2020.128605
Hurd C. L., Harrison P. J., Bischof K., Lobban C. S. (2014). Seaweed ecology and physiology. ed. 2 (Cambridge, UK: Cambridge University Press), 551.
Hurtado A. Q., Critchley A. T. (2018). A review of multiple biostimulant and bioeffector benefits of AMPEP, an extract of the brown alga Ascophyllum nodosum, as applied to the enhanced cultivation and micropropagation of the commercially important red algal carrageenophyte Kappaphycus alvarezii and its selected cultivars. J. Appl. Phycol. 30, 2859–2873. doi: 10.1007/s10811-018-1407-4
Hurtado A. Q., Critchley A. T. (2020). “Time for applications of biostimulants in phyconomy: seaweed extracts for enhanced cultivation of seaweeds (SEECS),” in: Torres M. D., Kraan S., Dominguez H.. (Eds). Sustainable seaweed technologies (Elsevier), 103–127. doi: 10.1016/B978-0-12-817943-7.00024-X
Ivanova E. P., Bakunina I. Y., Sawabe T., Hayashi K., Alexeeva Y. V., Zhukova N. V., et al. (2002). Two species of culturable bacteria associated with degradation of brown algae Fucus evanescens. Microb. Ecol. 43, 242–249. doi: 10.1007/s00248-001-1011-y
Jannin L., Arkoun M., Etienne P., Laîné P., Goux D., Garnica M., et al. (2013). Brassica napus Growth is promoted by Ascophyllum nodosum (L.) le jol. seaweed extract: microarray analysis and physiological characterization of n, c, and s metabolisms. J. Plant Growth. Regul. 32, 31–52. doi: 10.1007/s00344-012-9273-9
Jiksing C., Ongkudon M. M., Thien V. Y., Rodrigues K. F., Lym Yong W. T. (2022). Recent advances in seaweed seedling production: a review of eucheumatoids and other valuable seaweeds. Algae 37, 105–121. doi: 10.4490/algae.2022.37.5.11
Juhmani A. S., Vezzi A., Wahsha M., Buosi A., Pascale F. D., Schiavon R., et al. (2020). Diversity and dynamics of seaweed associated microbial communities inhabiting the lagoon of venice. Microorganisms 8 (11), 1657. doi: 10.3390/microorganisms8111657
Kapoore R. V., Wood E. E., Llewellyn C. A. (2021). Algae biostimulants: a critical look at microalgal biostimulants for sustainable agricultural practices. Biotechnol. Adv. 49, 107754. doi: 10.1016/j.biotechadv.2021.107754
Khan W., Rayirath U. P., Subramanian S., Jithesh M. N., Rayorath P., D. Hodges M., et al. (2009). Seaweed extracts as biostimulants of plant growth and development. J. Plant Growth. Regul. 28, 386–399. doi: 10.1007/s00344-009-9103-x
Krause G. H., Weis E. (1984). Chlorophyll fluorescence as a tool in plant physiology. Photosynthesis Res. 5 (2), 139–157. doi: 10.1007/BF00028527
Kurilenko V. V., Romanenko L. A., Isaeva M. P., Isaeva M. P., Svetashev V. I., Mikhailov V. V. (2019). Winogradskyella algae Sp. nov., a marine bacterium isolated from the brown alga. Antonie van Leeuwenhoek 112, 731–739. doi: 10.1007/s10482-018-1207-5
Lachnit T., Fischer M., Künzel S., Baines J. F., Harder T. (2013). Compounds associated with algal surfaces mediate epiphytic colonization of the marine macroalga Fucus vesiculosus. FEMS Microbiol. Ecol. 84 (2), 411–420. doi: 10.1111/1574-6941.12071
Lardi P. I., Varkitzi I., Tsiamis K., Orfanidis S., Koutsoubas D., Falace A., et al. (2022). Early development of Gongolaria montagnei (Fucales, phaeophyta) germlings under laboratory conditions, with a view to enhancing restoration potential in the Eastern Mediterranean. Bot. Mar. 65 (4), 279–287. doi: 10.1515/bot-2021-0105
Lauro F. M., McDougald D., Thomas T., Williams T. J., Egan S., Rice S., et al. (2009). The genomic basis of trophic strategy in marine bacteria. PNAS 106 (37), 5527–15533. doi: 10.1073/pnas.0903507106
Lee D. H., Cho S. J., Kim S. M., Lee S. B. (2012). Postechiella marina Gen. nov., sp. nov., isolated from seawater. Int. J. Syst. Evol. Microbiol. 62 (Pt 7), 1528–1535. doi: 10.1099/ijs.0.031302-0
Linardic M. (2018). The role of brown algal cell walls in morphogenesis and development. doi: 10.17863/CAM.22084
Lobban C. S., Harrison P. H. (1994). Seaweed ecology and physiology (New York: Cambridge University Press). doi: 10.1017/CBO9780511626210
Longford S. R., Campbell A. H., Nielsen S., Case R. J., Kjelleberg S., Steinberg P. D. (2019). Interactions within the microbiome alter microbial interactions with host chemical defences and affect disease in a marine holobiont. Sci. Rep. 9 (1), 1363. doi: 10.1038/s41598-018-37062-z
Lüning K., Pang S. (2003). Mass cultivation of seaweeds: current aspects and approaches. J. Appl. Phycol. 15, 115–119. doi: 10.1023/A:1023807503255
Luo H., Moran M. A. (2014). Evolutionary ecology of the marine Roseobacter clade. Microbiol. Mol. Biol. Rev. 78 (4), 573–587. doi: 10.1128/MMBR.00020-14
Mancuso F. P., D’Hondt S., Willems A., Airoldi L., De Clerck O. (2016). Diversity and temporal dynamics of the epiphytic bacterial communities associated with the canopy-forming seaweed Cystoseira compressa (Esper) gerloff and nizamuddin. Front. Microbiol. 7. doi: 10.3389/fmicb.2016.00476
Mancuso F. P., Strain E. M. A., Piccioni E., De Clerck O., Sarà G., Airoldi L. (2018). Status of vulnerable Cystoseira populations along the Italian infralittoral fringe, and relationships with environmental and anthropogenic variables. Mar. pollut. Bull. 129, 762–771. doi: 10.1016/j.marpolbul.2017.10.068
Manefield M., de Nys R., Naresh K., Roger R., Givskov M., Steinberg P., et al. (1999). Evidence that halogenated furanones from Delisea pulchra inhibit acylated homoserine lactone (AHL)-mediated gene expression by displacing the AHL signal from its receptor protein. Microbiology 145, 283–291. doi: 10.1099/13500872-145-2-283
Mangialajo L., Chiantore M., Cattaneo-Vietti R. (2008). Loss of fucoid algae along a gradient of urbanization, and structure of benthic assemblages. Mar. Ecol. Prog. Ser. 358, 63–74. doi: 10.3354/meps07400
Martin M., Portetelle D., Michel G., Vandenbol M. (2014). Microorganisms living on macroalgae: diversity, interactions, and biotechnological applications. Appl. Microbiol. Biotechnol. 98 (7), 2917–2935. doi: 10.1007/s00253-014-5557-2
Matsuo Y., Suzuki M., Kasai H., Shizuri Y., Harayama S. (2003). Isolation and phylogenetic characterization of bacteria capable of inducing differentiation in the green alga Monostroma oxyspermum. Environ. Microbiol. 5, 2535. doi: 10.1046/j.1462-2920.2003.00382.x
Maxwell K., Johnson G. N. (2000). Chlorophyll fluorescence-a practical guide. J. Exp. Bot. 51, 659–668. doi: 10.1093/jexbot/51.345.659
Medrano A., Hereu B., Cleminson M., Pagès-Escolà M., Rovira G., Solà J., et al. (2020). From marine deserts to algal beds: Treptacantha elegans revegetation to reverse stable degraded ecosystems inside and outside a no-take marine reserve. Restor. Ecol. 28, 632–644. doi: 10.1111/rec.13123
Michel G., Tonon T., Scornet D., Cock J. M., Kloareg B. (2010). The cell wall polysaccharide metabolism of the brown alga ectocarpus siliculosus.Insights into the evolution of extracellular matrix polysaccharides in eukaryotes. New Phytol. 188, 82–97. doi: 10.1111/j.1469-8137.2010.03374.x
Michelou V. K., Caporaso J. G., Knight R., Palumbi S. R. (2013). The ecology of microbial communities associated with Macrocystis pyrifera. PloS One 8, e67480. doi: 10.1371/journal.pone.0067480
Millar R. B., Anderson M. J. (2004). Remedies for pseudoreplication. Fisheries Res. 70, 397–407. doi: 10.1016/j.fishres.2004.08.016
Minich J., Morris M., Brown M., Doane M., Edwards M., Michael T., et al. (2018). Elevated temperature drives kelp microbiome dysbiosis, while elevated carbon dioxide induces water microbiome disruption. PloS One 13, 192772. doi: 10.1371/journal.pone.0192772
Orlando-Bonaca M., Pitacco V., Slavinec P., Šiško M., Makovec T., Falace A. (2021). First restoration experiment for Gongolaria barbata in Slovenian coastal waters. what can go wrong? Plants 10, 1–17. doi: 10.3390/plants10020239
Orlando-Bonaca M., Savonitto G., Asnaghi V., Trkov D., Pitacco V., Šiško M., et al. (2022). Where and how - new insight for brown algal forest restoration in the Adriatic. Front. Mar. Sci. 9. doi: 10.3389/fmars.2022.988584
Pang T., Liu J., Liu Q., Lin W. (2011). Changes of photosynthetic behaviours in Kappaphycus alvarezii infected by epiphytes. Evidence-Based Complementary Altern. Med. 2011, 658906. doi: 10.1155/2011/658906
Pedersen M. F., Borum J., Fotel F. (2010). Phosphorus dynamics and limitation of fast- and slow-growing temperate seaweeds in oslofjord, Norway. Mar. Ecol. Prog. Ser. 399, 103–115. doi: 10.3354/meps08350
Peixoto R. S., Voolstra C. R., Sweet M., Duarte C. M., Carvalho S., Villela H., et al. (2022). Harnessing the microbiome to prevent global biodiversity loss. Nat. Microbiol. 7, 1726–1735. doi: 10.1038/s41564-022-01173-1
Pinedo S., García M., Satta M. P., de Torres M., Ballesteros E. (2007). Rocky-shore communities as indicators of water quality: a case study in the northwestern Mediterranean. Mar. pollut. Bull. 55 (1–6), 125–135. doi: 10.1016/j.marpolbul.2006.08.044
Ping X. Y., Wang K., Zhang J. Y., Wang S. X., Du Z. J., Mu D. S. (2022). Psychroserpens luteolus Sp. nov., isolated from Gelidium, reclassification of Ichthyenterobacterium magnum as Psychroserpens magnus comb. nov., Flavihalobacter algicola as Psychroserpens algicola comb. nov., Arcticiflavibacter luteus as Psychroserpens luteus comb. nov. Arch. Microbiol. 204, 279. doi: 10.1007/s00203-022-02895-w
Pitlik S. D., Koren O. (2017). How holobionts get sick–toward a unifying scheme of disease. Microbiome 5, 1–4. doi: 10.1186/s40168-017-0281-7
Prisa D. (2021). Biological mixture of brown algae extracts influences the microbial community of Lobivia arachnacantha, Lobivia aurea, Lobivia jojoiana and Lobivia grandiflora in pot cultivation. GSC Advanced Res. Rev. 08 (03), 043–053. doi: 10.30574/gscarr.2021.8.3.0190
Quast C., Pruesse E., Yilmaz P., Gerken J., Schweer T., Yarza P., et al. (2013). The SILVA ribosomal RNA gene database project: improved data processing and web-based tools. Nucl. Acids Res. 41 (D1), D590–D596. doi: 10.1093/nar/gks1219
Rao D., Webb J. S., Kjelleberg S. (2005). Competitive interactions in mixed-species biofilms containing the marine bacterium Pseudoalteromonas tunicata. appl. Environ. Microbiol. 71, 1729–1736. doi: 10.1128/AEM.71.4.1729-1736.2005
R Core Team (2022). R: a language and environment for statistical computing (Vienna, Austria: R Foundation for Statistical Computing). Available at: https://www.R-project.org/.
Ren C. G., Liu Z. Y., Wang X. L., Qin S. (2022). The seaweed holobiont: from microecology to biotechnological applications. Microb. Biotechnol. 15 (3), 738–754. doi: 10.1111/1751-7915.14014
Roleda M. Y., Hurd C. L. (2019). Seaweed nutrient physiology: application of concepts to aquaculture and bioremediation. Phycologia 58, 552–562. doi: 10.1080/00318884.2019.1622920
Rouphael Y., Colla G. (2020). Editorial: biostimulants in agriculture. Front. Plant Sci. 11. doi: 10.3389/fpls.2020.00040
Saa S., Olivos-Del Rio A., Castro S., Brown P. H. (2015). Foliar application of microbial and plant based biostimulants increases growth and potassium uptake in almond (Prunus dulcis [Mill.] D.A. Webb). Front. Plant Sci. 6. doi: 10.3389/fpls.2015.00087
Sales M., Cebrian E., Tomas F., Ballesteros E. (2011). Pollution impacts and recovery potential in three species of the genus Cystoseira (Fucales, heterokontophyta). Estuar. Coast. Shelf. Sci. 92 (3), 347–357. doi: 10.1016/j.ecss.2011.01.008
Samuels L. J., Setati M. E., Blancquaert E. H. (2022). Towards a better understanding of the potential benefits of seaweed based biostimulants in Vitis vinifera l. Cultivars. Plants 11, 348. doi: 10.3390/plants11030348
Sánchez de Pedro R., Fernández A. N., García-Sánchez M. J., Flores-Moya A., Bañares-España E. (2022). Parental environment modulates offspring thermal tolerance in a foundational intertidal seaweed. Eur. J. Phycol. 1–24. doi: 10.1080/09670262.2022.2081731
Sand-Jensen K. (1977). Effect of epiphytes on eelgrass photosynthesis. Aquat. Bot. 3 (Suppl. C), 55–63. doi: 10.1016/0304-3770(77)90004-3
Savonitto G., de la Fuente G., Tordoni E., Ciriaco S., Srijemsi M., Bacaro G., et al. (2021). Addressing reproductive stochasticity and grazing impacts in the restoration of a canopy forming brown alga by implementing mitigation solutions. Aquat. Conserv. Mar. Freshw. Ecosyst. 31, 1611–1623. doi: 10.1002/aqc.3555
Savva I., Bennett S., Roca G., Jordà G., Marbà N. (2018). Thermal tolerance of Mediterranean marine macrophytes: vulnerability to global warming. Ecol. Evol. 8, 12032–12043. doi: 10.1002/ece3.4663
Schneider C. A., Rasband W. S., Eliceiri K. W. (2012). NIH Image to ImageJ: 25 years of image analysis. Nat. Methods 9 (7), 671–675. doi: 10.1038/nmeth.2089
Schoenwaelder M. E. A. (2002). The occurrence and cellular significance of physodes in brown algae. Phycologia 41 (2), 125–139. doi: 10.2216/i0031-8884-41-2-125.1
Shukla P. S., Borza T., Critchley A. T., Prithiviraj B. (2021). Seaweed-based compounds and products for sustainable protection against plant pathogens. Mar. Drugs 19, 59. doi: 10.3390/md19020059
Smart J. N., Schmid M., Paine E. R., Britton D., Revill A., Hurd C. L. (2022). Seasonal ammonium uptake kinetics of four brown macroalgae: implications for use in integrated multi-trophic aquaculture. J. Appl. Phycol. 34, 1693–1708. doi: 10.1007/s10811-022-02743-w
Smolina I., Kollias S., Jueterbock A., Coyer J. A., Hoarau G. (2016). Variation in thermal stress response in two populations of the brown seaweed, Fucus distichus, from the Arctic and subarctic intertidal. R. Soc Open Sci. 3 (1), 150429. doi: 10.1098/rsos.150429
Sneed J. M., Pohnert G. (2011). The green alga Dictyosphaeria ocellata and its organic extracts alter natural bacterial biofilm communities. Biofouling 27, 347–356. doi: 10.1080/08927014.2011.576317
Steinberg P. D., Rice S. A., Campbell A. H., McDougald D., Harder T. (2011). Interfaces between bacterial and eukaryotic “neuroecology”. Integr. Comp. Biol. 51 (5), 794–806. doi: 10.1093/icb/icr115
Stiger-Pouvreau V., Jégou C., Cérantola S., Guérard F., Le Lann K. (2014). “Phlorotannins in sargassaceae species from Brittany (France): interesting molecules for ecophysiological and valorisation purposes,” in: Bourgougnon N. (Eds). Advances in botanical research, Academic Press, 379–411. doi: 10.1016/B978-0-12-408062-1.00013-5
Stirk W. A., Rengasamy K. R. R., Kulkarni M. G., van Staden J. (2020). “Plant biostimulants from seaweed: an overview,” in Geelan D., Xu L. (Eds). The chemical biology of plant biostimulants. Wiley, Hoboken, USA (Hoboken, NJ, USA, John Wiley & Sons, Ltd), 31–55.
Sujeeth N., Petrov V., Guinan K. J., Rasul F., O’Sullivan J. T., Gechev T. S. (2022). Current insights into the molecular mode of action of seaweed-based biostimulants and the sustainability of seaweeds as raw material resources. Int. J. Mol. Sci. 23 (14), 7654. doi: 10.3390/ijms23147654
Sullivan B. K., Trevathan-Tackett S. M., Neuhauser S., Govers L. L. (2018). Host-pathogen dynamics of seagrass diseases under future global change. Mar. pollut. Bull. 134, 75–88. doi: 10.1016/j.marpolbul.2017.09.030
Susini M. L. (2006). Statut et biologie de cystoseira amentacea var. stricta (France: Université Nice-Sophia Antipolis). Available at: ftp://nephi.unice.fr/users/ecomers/2006/2006%20Susini%20Th%C3%A8se.pdf.
Susini M. L., Mangialajo L., Thibaut T., Meinesz A. (2007). Development of a transplantation technique of Cystoseira amentacea var. stricta and Cystoseira compressa. Hydrobiologia 580, 241–244. doi: 10.1007/s10750-006-0449-9
Suthar P., Gajaria T. K., Reddy C. R. K. (2019). Production of quality seaweed biomass through nutrient optimization for the sustainable land-based cultivation. Algal Res. 42, 101583. doi: 10.1016/j.algal.2019.101583
Taylor M. W., Radax R., Steger D., Wagner M. (2007). Sponge-associated microorganisms: evolution, ecology, and biotechnological potential review. Microbiol. Mol. Biol. Rev. 71 (2), 295–347. doi: 10.1128/MMBR.00040-06
Thatcher C., Høj L., Bourne D. G. (2022). Probiotics for coral aquaculture: challenges and considerations. Curr. Opin. Biotechnol. 73, 380–386. doi: 10.1016/j.copbio.2021.09.009
Thibaut T., Blanfune A., Boudouresque C. F., Verlaque M. (2015). Decline and local extinction of fucales in French Riviera: the harbinger of future extinctions? Mediterr. Mar. Sci. 16 (1), 206–224. doi: 10.12681/mms.1032
Thibaut T., Blanfuné A., Markovic L., Verlaque M., Boudouresque C. F., Perret-Boudouresque M., et al. (2014). Unexpected abundance and long-term relative stability of the brown alga Cystoseira amentacea, hitherto regarded as a threatened species, in the north-western Mediterranean Sea. Mar. pollut. Bull. 89, 305–323. doi: 10.1016/j.marpolbul.2014.09.043
Thibaut T., Pinedo S., Torras X., Ballesteros E. (2005). Long-term decline of the populations of fucales (Cystoseira spp. and sargassum spp.) in the albères coast (France, north-western Mediterranean). Mar. pollut. Bull. 50, 1472–1489. doi: 10.1016/j.marpolbul.2005.06.014
Thomas T. E., Harrison P. J., Taylor E. B. (1985). Nitrogen uptake and growth of the germlings and mature thalli of Fucus distichus. Mar. Biol. 84, 267–274. doi: 10.1007/BF00392496
Trevathan-Tackett S. M., Sherman C. D., Huggett M. J., Campbell A. H., Laverock B., Hurtado-McCormick V., et al. (2019). A horizon scan of priorities for coastal marine microbiome research. Nat. Ecol. Evol. 3, 1509–1520. doi: 10.1038/s41559-019-0999-7
Umanzor S., Han S., Song H. I., Park J. S., Critchley A. T., Yarish C., et al. (2022). Ascertaining the interactions of brown seaweed-derived biostimulants and seawater temperature on spore release, germination, conchocelis, and newly formed blades of the commercially important red alga Neopyropia yezoensis. Algal. Res. 64, 102692. doi: 10.1016/j.algal.2022.102692
Umanzor S., Jang S., Antosca R., Critchley A. T., Yarish C., Kim J. K. (2020a). Optimizing the application of selected biostimulants to enhance the growth of Eucheumatopsis isiformis, a carrageenophyte with commercial value, as grown in land-based nursery systems. J. Appl. Phycol. 32, 1917–1922. doi: 10.1007/s10811-020-02091-7
Umanzor S., Shin S., Marty-Rivera M., Augyte S., Yarish C., Kim J. K. (2019). Preliminary assessment on the effects of the commercial seaweed extract, AMPEP, on growth and thermal tolerance of the kelp saccharina spp. from the Northwest Atlantic. J. Appl. Phycol. 31, 3823–3829. doi: 10.1007/s10811-019-01852-3
Umanzor S., Shin S., Yarish C., Augyte S., Kim J. K. (2020b). Exploratory evaluation of the effects of kelpak® seaweed extract on cultivated kelp saccharina spp. exposed to sublethal and lethal temperatures. J. World. Aquac. Soc 51, 960–969. doi: 10.1111/jwas.12687
Underwood A. J. (1997). Experiments in ecology: their logical design and interpretation using analysis of variances (Cambridge: Cambridge University Press). doi: 10.1017/CBO9780511806407
Vairappan C. S., Chung C. S., Hurtad A. Q., Soya F. E., Lhonneur G. B., Critchley A. (2008). Distribution and symptoms of epiphyte infection in major carrageenophyte-producing farms. J. Appl. Phycol. 20, 477–483. doi: 10.1007/s10811-007-9299-8
Valdazo J., Viera-Rodríguez M. A., Espino F., Haroun R., Tuya F. (2017). Massive decline of Cystoseira abies-marina forests in gran canaria island (Canary islands, eastern Atlantic). Sci. Mar. 81, 499–507. doi: 10.3989/scimar.04655.23A
van der Loos L. M., Eriksson B. K., Salles J. F. (2019). The macroalgal holobiont in a changing Sea. Trends Microbiol. 27 (7), 635–650. doi: 10.1016/j.tim.2019.03.002
Van Oosten M. J., Pepe O., De Pascale S., Silletti S., Maggio A. (2017). The role of biostimulants and bioeffectors as alleviators of abiotic stress in crop plants. Chem. Biol. Technol. Agric. 4, 5. doi: 10.1186/s40538-017-0089-5
Verdura J., Sales M., Ballesteros E., Cefalì M. E., Cebrian E. (2018). Restoration of a canopy-forming alga based on recruitment enhancement: methods and long-term success assessment. Front. Plant Sci. 9. doi: 10.3389/fpls.2018.01832
Verdura J., Santamaría J., Ballesteros E., Smale D. A., Cefalì M. E., Golo R., et al. (2021). Local-scale climatic refugia offer sanctuary for a habitat-forming species during a marine heatwave. J. Ecol. 109, 1758–1773. doi: 10.1111/1365-2745.13599
Vergés A., Steinberg P. D., Hay M. E., Poore A. G. B., Campbell A. H., Ballesteros E., et al. (2014). The tropicalization of temperate marine ecosystems: climate-mediated changes in herbivory and community phase shifts. Proc. R. Soc B. Biol. Sci. 281, 20140846. doi: 10.1098/rspb.2014.0846
Von Stosch H. A. (1963). Wirkungen von jod und arsenit auf meeresalgen in kultur. Proc. Int. Seaweed Symp. 4, 142–150.
Wang X., Preston J. F. 3rd, Romeo T. (2004). The pgaABCD locus of escherichia coli promotes the synthesis of a polysaccharide adhesin required for biofilm formation. J. Bacteriol. 186 (9), 2724–2734. doi: 10.1128/JB.186.9.2724-2734.2004
Wang Z., Xiao T., Pang S., Liu M., Yue H. (2009). Isolation and identification of bacteria associated with the surfaces of several algal species. Chin. J. Oceanol. Limn. 27, 487–492. doi: 10.1007/s00343-009-9165-4
Ward G. M., Faisan J. P., Cottier-Cook E. J., Gachon C., Hurtado A. Q., Lim P. E., et al. (2020). A review of reported seaweed diseases and pests in aquaculture in Asia. J. World. Aquacult. Soc 51 (4), 815–828. doi: 10.1111/jwas.12649
Whitchurch C. B., Tolker-Nielsen T., Ragas P. C., Mattick J. S. (2002). Extracellular DNA required for bacterial biofilm formation. Science 295 (5559), 1487. doi: 10.1126/science.295.5559.1487
Whittaker R. H. (1972). Evolution and measurement of species diversity. Taxon 21 (2/3), 213–251. doi: 10.2307/1218190
Yonamine R., Ichihara K., Tsuyuzaki S., Hervé C., Motomura T., Nagasato C. (2021). Changes in cell wall structure during rhizoid formation of Silvetia babingtonii (Fucales, phaeophyceae) zygotes. J. Phycol. 57, 1356–1367. doi: 10.1111/jpy.13178
Zhuang L., Luo L. (2020). Roseovarius spongiae Sp. nov., a bacterium isolated from marine sponge. Int. J. Syst. Evol. Microbiol. 70 (1), 274–281. doi: 10.1099/ijsem.0.003750
Keywords: Fucales, microbe, biofilm, ecological restoration, ex-situ culture, algal extract
Citation: Malfatti F, Kaleb S, Saidi A, Pallavicini A, Agostini L, Gionechetti F, Natale S, Balestra C, Bevilacqua S and Falace A (2023) Microbe-assisted seedling crop improvement by a seaweed extract to address fucalean forest restoration. Front. Mar. Sci. 10:1181685. doi: 10.3389/fmars.2023.1181685
Received: 07 March 2023; Accepted: 17 April 2023;
Published: 02 May 2023.
Edited by:
Roberto Danovaro, Marche Polytechnic University, ItalyReviewed by:
Sotiris Orfanidis, Institute of Fisheries Research, GreeceLeonel Pereira, University of Coimbra, Portugal
Copyright © 2023 Malfatti, Kaleb, Saidi, Pallavicini, Agostini, Gionechetti, Natale, Balestra, Bevilacqua and Falace. This is an open-access article distributed under the terms of the Creative Commons Attribution License (CC BY). The use, distribution or reproduction in other forums is permitted, provided the original author(s) and the copyright owner(s) are credited and that the original publication in this journal is cited, in accordance with accepted academic practice. No use, distribution or reproduction is permitted which does not comply with these terms.
*Correspondence: Francesca Malfatti, fmalfatti@units.it